- 1Department of Pharmacology, School of Pharmacy, Southwest Medical University, Luzhou, Sichuan, China
- 2School of Basic Medical Sciences, Southwest Medical University, Luzhou, Sichuan, China
- 3Clinical Trial Center, The Affiliated Traditional Chinese Medicine Hospital of Southwest Medical University, Luzhou, Sichuan, China
- 4Drug Discovery Research Center, Southwest Medical University, Luzhou, Sichuan, China
- 5Laboratory for Cardiovascular Pharmacology of Department of Pharmacology, The School of Pharmacy, Southwest Medical University, Luzhou, Sichuan, China
Introduction: Radiation-induced thrombocytopenia (RIT) is a serious complication of cancer radiotherapy, for which therapeutic options are limited. This study investigates wedelolactone (WED), a metabolite of a botanical drug, as a potential treatment for RIT.
Methods: In vitro experiments were conducted using Meg-01 and K562 cell lines to evaluate the effects of WED on megakaryocyte differentiation and maturation. Flow cytometry and phalloidin staining were employed to assess the expression of megakaryocyte-specific markers CD41 and CD61, as well as nuclear polyploidization. A mouse model of RIT was established to assess the efficacy of WED in restoring platelet counts and regulating hematopoiesis. RNA sequencing and western blot analyses were performed to explore the underlying molecular mechanisms.
Results: In vitro experiments revealed that WED enhanced megakaryocyte differentiation in a dose-dependent manner, increasing the expression of lineage-specific markers CD41 and CD61, and promoting polyploidization and cytoskeletal reorganization. In vivo, WED significantly restored platelet counts in the mouse model of RIT and promoted the production of hematopoietic stem cells (HSCs), megakaryocytes, and reticulated platelets. RNA sequencing and western blot revealed that WED-induced megakaryocyte differentiation involves the regulation of mitochondrial oxidative phosphorylation mediated by the AMPK signaling pathway and activation of the MAPK signaling pathway. Inhibition of mitochondrial oxidative phosphorylation or MAPK signaling suppressed WED-induced megakaryocyte differentiation, highlighting the central role of these pathways.
Discussion: These findings indicate that WED could be a promising therapeutic candidate for RIT, acting through the modulation of oxidative phosphorylation and MAPK signaling pathways to enhance thrombopoiesis.
1 Introduction
Thrombocytopenia is a prevalent hematological disorder defined by a peripheral blood platelet count below the normal threshold of 150 × 109/L (Raghunathan et al., 2022). It often results in bleeding tendencies, coagulation abnormalities, and, in severe cases, life-threatening conditions (Greenberg, 2017). The etiology of thrombocytopenia is multifactorial, frequently linked to either impaired platelet production or accelerated platelet clearance (Chen et al., 2022). This condition can arise from diverse causes, including genetic predispositions, acquired disorders, microbial infections, or as a consequence of radiotherapy and chemotherapy. Thrombocytopenia significantly worsens patient prognosis and restricts the efficacy of treatments, particularly in those undergoing radiotherapy and chemotherapy (Du et al., 2023). Additionally, it can precipitate serious complications, such as arterial and venous thrombosis, ischemic stroke, and myocardial infarction, thereby contributing substantially to global morbidity and mortality (Xu et al., 2016). Consequently, the rapid and effective elevation of platelet counts is a critical therapeutic objective in the management of thrombocytopenia.
Platelets are small cytoplasmic fragments shed from megakaryocytes in the bone marrow (BM), playing critical roles in several physiological processes, including hemostasis, thrombosis, and immunomodulation. With an average lifespan of 7–10 days, continuous platelet production is essential to maintain normal platelet counts (Liu et al., 2014). In healthy individuals, platelets are produced from hematopoietic stem cells (HSCs) through a complex series of differentiation and maturation processes known as thrombopoiesis. HSCs differentiate into megakaryocytes, which undergo differentiation and polyploidization to form platelet precursors that release platelets into the vascular lumen (Ghalloussi et al., 2019). This process is regulated by several key signaling pathways, such as JAK/STAT, PI3K/AKT, and MAPK. These activated pathways regulate the expression of critical transcription factors, including GATA1, RUNX1, NF-E2, FOS, and TAL1, which are pivotal in megakaryocyte differentiation, maturation, and thrombopoiesis (Yang et al., 2022; Lai et al., 2023). Furthermore, recent studies have suggested that mitochondrial metabolism may represent a novel regulatory mechanism in megakaryocyte differentiation. For instance, atmospheric particulate matter has been reported to induce thrombopoiesis by modulating mitochondrial oxidative phosphorylation (Jin et al., 2021). Similarly, virodhamine has been shown to promote megakaryocyte differentiation by regulating mitochondrial function (Sharma et al., 2021), while Justicia adhatoda L. [Acanthaceae] induces differentiation via mitochondrial reactive oxygen species (ROS) production (Gutti et al., 2018). These findings underscore the emerging significance of mitochondrial pathways in regulating megakaryocyte maturation and thrombopoiesis.
The primary treatments for thrombocytopenia currently include pharmacological therapy, platelet transfusion, and dietary modification. Pharmacological interventions typically involve glucocorticoids, immunoglobulins, and thrombopoietin receptor agonists to enhance platelet production. However, these approaches often come with significant drawbacks, including high relapse rates, limited efficacy, and the development of drug tolerance (Lasne et al., 2020). Platelet transfusion, while capable of rapidly increasing platelet levels, is not a sustainable long-term solution due to limited donor resources and the risk of transfusion-related reactions (Katsube et al., 2019). Consequently, significant challenges persist in the management of thrombocytopenia. There is an essential demand for the development of innovative and effective interventions that can safely and rapidly elevate platelet counts and improve patient outcomes.
The metabolites of botanical drugs are widely recognized as valuable precursors for drug development due to their unique chemical structures and diverse biological activities (Sun et al., 2020). The metabolites derived from plants, microorganisms, and marine organisms offer significant chemical diversity, providing novel insights for drug discovery. Wedelolactone (WED), a polyphenol sharing a coumarin skeleton with a benzofuran moiety at C-3 and C-4, belongs to a class of secondary metabolites renowned for diverse pharmacological effects. Isolated from traditional medicinal plants such as Eclipta prostrata (L.) L. [Asteraceae], Sphagneticola calendulacea (L.) Pruski [Asteraceae], WED has been extensively studied for its potent bioactivities, including antifibrotic, anti-inflammatory, anticancer, and antidiabetic properties (Tu et al., 2021; Swami et al., 2023; Zhang J. et al., 2023). These effects are primarily attributed to its molecular structure, which facilitates interactions with various cellular targets, influencing key signaling pathways (Tu et al., 2021; Zhang J. et al., 2023). The intrinsic molecular properties of WED, such as its stability and reactivity, drive ongoing research in the biomedical and pharmaceutical fields, particularly for the development of novel therapeutic interventions (Swami et al., 2023). In addition, the use of botanical drug metabolites like WED is increasingly recognized as a promising alternative to synthetic drugs, offering a more sustainable and often less toxic approach to treatment. The potential of WED as an effective therapeutic agent underscores the importance of exploring botanical drug metabolites in modern medicine (Swami et al., 2023). WED was previously identified as a potential therapy for thrombocytopenia through virtual screening, demonstrating its ability to restore platelet counts in radiation-induced thrombocytopenia (RIT) mice models without systemic toxicity (Mo et al., 2023). However, the precise mechanism underlying WED’s efficacy in treating thrombocytopenia remains unclear. The molecular mechanism underlying WED-induced megakaryocyte differentiation was investigated using RNA sequencing. Experimental validation further confirmed that WED promotes megakaryocyte differentiation and maturation by regulating mitochondrial metabolism and activating the MAPK pathway. These findings emphasize the potential impact of mitochondrial metabolism in hematopoiesis and indicate that WED could be a prospective candidate for treating RIT clinically.
2 Materials and methods
2.1 Chemicals
WED, with a purity exceeding 99.66% was ascertained through High Performance Liquid Chromatography, was procured by Chengdu Pusi Biotechnology Co., Ltd. and dissolved as per the instructions.
2.2 Cell culture
The human chronic myeloid leukemia cell line (K562) and human megakaryocytic leukemia cell line (Meg-01) were obtained from the American Type Culture Collection (Bethesda, MD, United States). These cells were cultured in RPMI-1640 medium, with 10% fetal bovine serum (FBS) and 1% penicillin/streptomycin.
2.3 Quantification of cells surface markers
Following the 5-day treatment involving WED (2.5, 5, and 10 μM) along with Phorbol 12-myristate 13-acetate (PMA) (Mo et al., 2023), the cells were collected and were subsequently incubated with FITC-CD41 and PE-CD61(4A Biotech, Beijing, China) antibodies for a duration of 30 min, shielded from light. The quantification of CD41 and CD61 expression levels were quantified using flow cytometer (BD Biosciences, San Jose, CA, USA).
2.4 DNA ploidy analysis
Cells were harvested and washed three times with phosphate-buffered saline (PBS), as previously described. They were then fixed overnight at 4°C, followed by two additional washes with PBS. Next, the cells were incubated with propidium iodide/RNase staining buffer for 15 min at room temperature. Finally, the stained cells were analyzed using flow cytometry.
2.5 Phalloidin staining
Cells were collected as previously outlined and fixed with 4% paraformaldehyde for 10 min, followed by permeabilization with 0.5% Triton X-100. They were then shielded from light and stained with tetramethylrhodamine-labeled ghost pen cyclic peptide (Solarbio, Beijing, China) for 1 h. Afterward, the nuclei were counterstained with 100 nM 4′,6-diamidino-2-phenylindole (Solarbio, Beijing, China) for 30 s. Fluorescence images were captured using a fluorescence microscope (Leica, Wetzlar, Germany).
2.6 Animals
Kunming (KM) mice, aged 8–10 weeks, were acquired from Liaoning Changsheng Biological Co. Ltd. They were provided a standard diet and kept in an environment with a 12-hour light/dark cycle.
2.7 Establishment and treatment of RIT mice model
The mice were divided randomly into four groups: control group, RIT model group, recombinant human thrombopoietin (TPO) positive group (3000 U/kg) (Lin et al., 2021), and WED (2.5, 5, and 10 mg/kg) group (Mo et al., 2023). All groups, except the control group, received total body irradiation (TBI) with 4 Gy X-rays at a dose rate of 4 Gy/min to establish thrombocytopenic model mice. Briefly, KM mice were irradiated in well-ventilated acrylic plastic boxes at room temperature. The boxes were positioned at the center of the irradiation field within the 95% isodose region of the irradiator to ensure uniform dose delivery across multiple samples (Du et al., 2023; Wang et al., 2023). Following irradiation, both the control and model groups received daily intraperitoneal injections of normal saline. The TPO and WED groups received intraperitoneal injections of TPO or WED daily for 12 consecutive days, respectively. Blood samples of 40 µL were collected from the ocular venous plexus (days 0, 3, 7, 10, 12) and analysed using a haematology analyser (Sysmex XT-2000iV, Kobe, Japan).
2.8 Flow cytometry analysis of BM
Femur cells of mice were harvested (Liu et al., 2024). Antibody labeling followed standard protocols. For haematopoietic stem progenitor analysis, cells were labelled with FITC-conjugated anti-CD34 and PE-conjugated anti-CD117. For megakaryocyte-erythroid progenitor cell analysis, cells were labelled with FITC-conjugated anti-CD41 and PE-conjugated anti-CD117 For megakaryocyte analysis, cells were labelled with FITC-conjugated anti-CD41 and PE-conjugated anti-CD42d. All antibodies were incubated for 20 min and tested by flow cytometry. All antibodies are from Beijing Sizhenbai Biotechnology Co.
2.9 Reticulocyte platelet assay
Whole blood from mice orbits was collected and diluted in Tyrode’s solution. Platelets were labeled with anti-CD61-APC antibody and thiazole orange, then incubated for 20 min in the dark before analysis by flow cytometry.
2.10 Immunohistochemical analysis
Mice were euthanized, and the femur and spleen were isolated and fixed in 10% paraformaldehyde. The femur was decalcified in a decalcification solution for over a month. Organs were subsequently embedded in paraffin, sectioned, and stained with antibodies against CD41 (Abmart, Shanghai, China).
2.11 Mitochondrial assay
Investigated mitochondrial metabolic function using established methods (Chen et al., 2023). Mitochondrial mass was assessed by staining with Mito-Tracker Green (MTG), while Mitochondrial Membrane Potential (MMP) was evaluated using the Mitochondrial Membrane Potential Assay Kit JC-1. Levels of mitochondrial ROS were measured with the ROS Assay Kit. After staining, cells were analyzed by flow cytometry. Additionally, adenosine triphosphate (ATP) levels were quantified following the manufacturer’s instructions using the ATP Assay Kit. All assay reagents were obtained from Beyotime Biotechnology Technologies Ltd.
2.12 RNA sequencing
Briefly, total RNA was extracted using TRIzol (Invitrogen, Carlsbad, CA) following the treatment of cells with WED (10 μM) for 3 days. Libraries were sequenced on the Illumina HiSeq xten/NovaSeq 6,000 platform by Shanghai Metso BioMedical Biotechnology Co. Differentially expressed genes (DEGs) were identified using a fold change (FC) of ≥ 1.5 and a p-value of < 0.05. DEGs were then subjected to enrichment analyses. The RNA sequencing data were deposited to Sequence Read Archive of National Center for Biotechnology Information, and the accession number was PRJNA1162465.
2.13 Western blot
Briefly, samples were loaded onto 10% sodium dodecyl sulfate polyacrylamide gel electrophoresis followed by polyvinylidene difluoride membrane (BioRad, Hercules, CA). Transfer of membrane was performed. Add primary antibody and incubate overnight, then wash three times with PBST (PBS with 2% Tween-20), the secondary antibody was added and incubated at 37°C for 1 h. Antibodies against RAS (Abmart, T56672S), p-MEK1/2 (CST, 9154S), MEK1/2 (CST, 8727 S), p-ERK1/2 (Abmart, TA1015S), ERK1/2 (Abmart, TA0155), p-AMPK (Abmart, T55608F), AMPK (Abmart, T55326F), NF-E2 (Proteintech, United States, 11089-1-AP), GATA1 (Proteintech, United States, 10917-2-AP), FOS (Proteintech, United States, 66590-1-Ig), GAPDH (Proteintech, United States, 10494-1-AP).
2.14 Statistical analysis
The mean ± standard deviation (SD) of at least three independent repeated trials were used to illustrate all calculated data. Statistical analysis was performed using either Student’s t-test or two-way ANOVA to compare the different groups in this study, with a significance level set at p < 0.05.
3 Results
3.1 WED promotes megakaryocyte differentiation and maturation in vitro
Megakaryocyte development involves a process of “excessive hypertrophy”, marked by substantial cell volume expansion, polyploidy formation, and elevated expression of megakaryocyte-specific markers (Tang et al., 2024). To assess whether WED directly influences megakaryocyte differentiation and maturation, we treated Meg-01 and K562 cells with varying doses of WED (2.5, 5, and 10 μM). PMA served as a positive control for in vitro megakaryocyte differentiation (Ogura et al., 1988). Flow cytometry analysis, conducted after 5 days of treatment, revealed a dose-dependent increase in the expression of megakaryocyte lineage-specific markers CD41 and CD61 in both WED-treated and PMA-treated groups (Figure 1A).
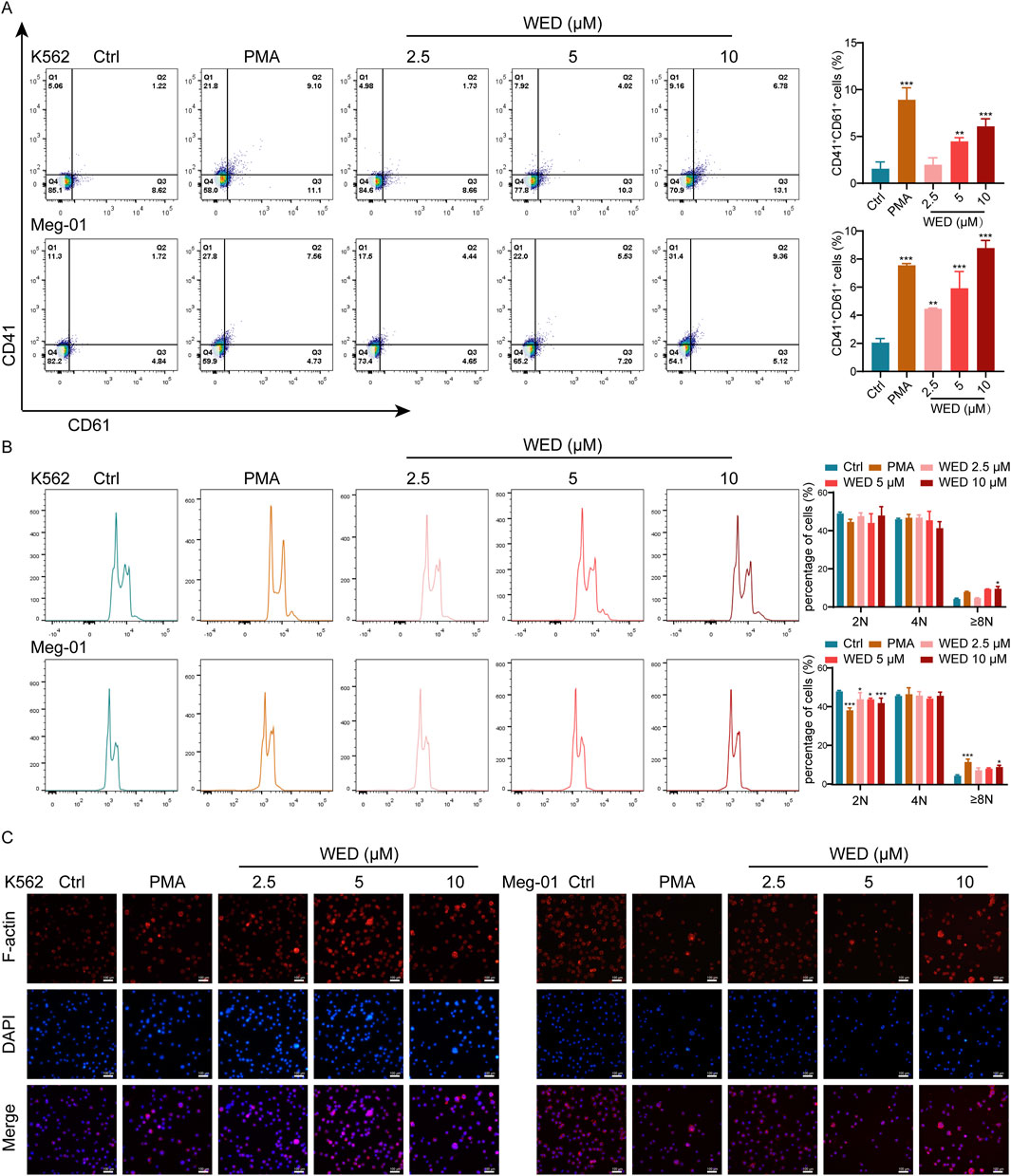
Figure 1. WED induces megakaryocyte differentiation and maturation. (A) CD41 and CD61 expression in the cells after 5 days of PMA and WED treatment. The histograms illustrate the proportion of CD41+ CD61+ cells in each group. (B) DNA ploidy analysis of cells using flow cytometry after 5 days of PMA and WED intervention. The histograms display the percentage of 2N, 4N, and ≥ 8N cells. (C) Phalloidin staining was conducted on two cell types treated with WED and PMA for 5 days. Scale bar = 100 μm. Data are displayed as mean ± SD (n = 3). *p < 0.05, **p < 0.01, ***p < 0.001 vs. the control group. Ctrl: Control.
Polyploidy, a hallmark of mature megakaryocytes (Chase et al., 2010), was also evaluated. Flow cytometry indicated that WED significantly enhanced DNA ploidy (Figure 1B). Given that maturation of megakaryocytes and the formation of proplatelets necessitate the reorganization of the actin cytoskeleton (Machlus et al., 2016), we conducted phalloidin staining. The results indicated that cells in the WED-treated and PMA-treated groups displayed a notable enlargement of cell size and exhibited multilobed, multinucleated nuclei, in contrast to the control group, which showed minimal nuclear maturation (Figure 1C). Together, these findings suggest that WED effectively promotes megakaryocyte differentiation and maturation.
3.2 WED promotes platelet recovery in RIT models
To evaluate the in vivo efficacy of WED in treating thrombocytopenia, a RIT mouse model was established using whole-body irradiation with 4 Gy X-rays. Following irradiation, treatment included varying doses of WED (2.5, 5, and 10 mg/kg), with TPO (3000 U/kg) serving as a positive control (Figure 2A). Throughout the experimental period, the health status of the mice was monitored daily. On the seventh day post-irradiation, the mice exhibited lethargy and reduced physical activity. However, by day twelve, no significant differences were observed in body weight, mental state, locomotor activity, or food and water intake among any of the treatment groups. These findings suggest that the mice tolerated WED well at the tested dosage. In irradiated mice, platelet counts declined to their lowest point on day 7, while counts in the control group remained stable. However, from day 7 to day 12, both WED and TPO-treated mice exhibited significant platelet recovery compared to the model group, indicating that WED effectively promotes platelet production in the RIT model (Figure 2B). Platelet distribution width (PDW), mean platelet volume (MPV), and platelet-large cell ratio (P-LCR) were significantly elevated in irradiated mice compared to normal controls (Figures 2C–E). This observation aligns with previous studies showing that surviving megakaryocytes produce larger, more reactive platelets following irradiation, which may serve as an emergency response to compensate for the reduced hemostatic function caused by cytotoxic damage (Du et al., 2023).
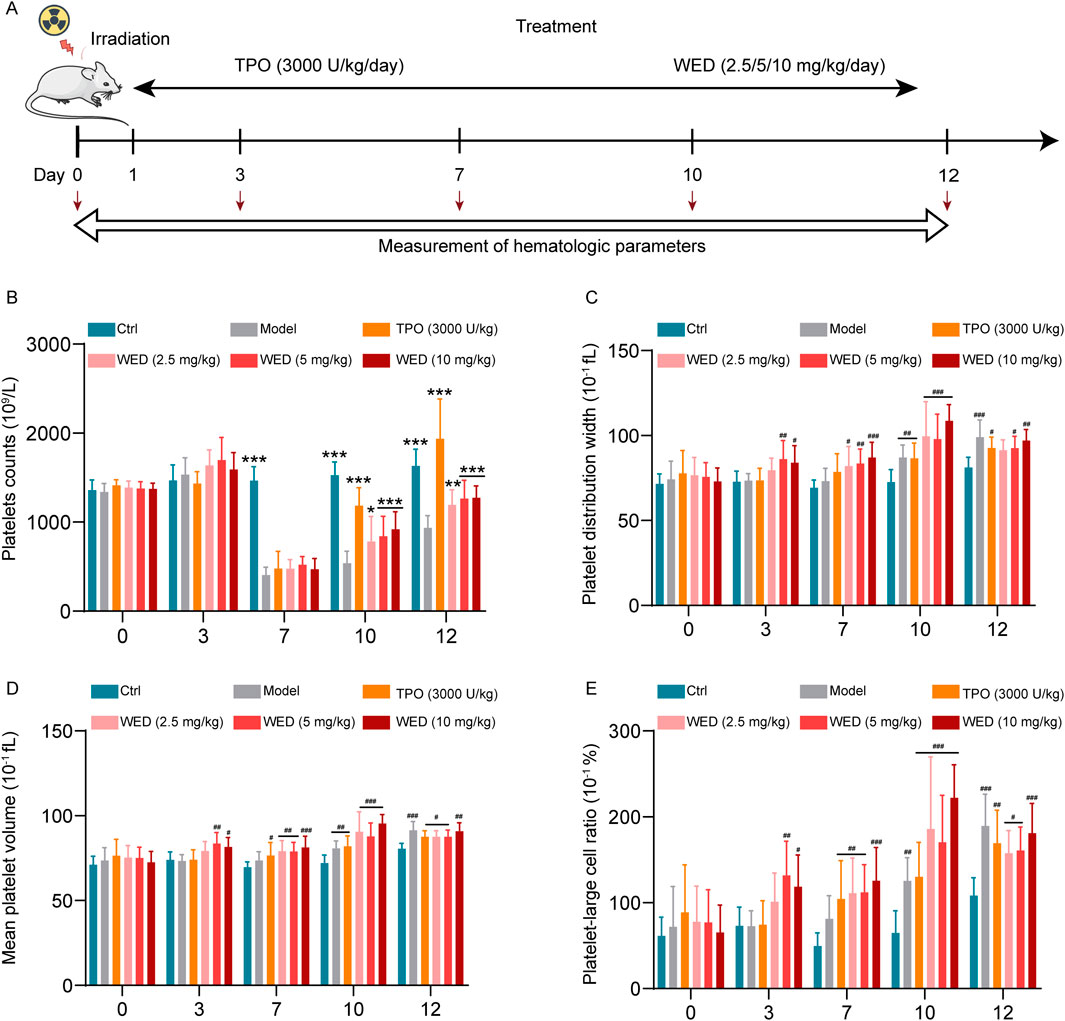
Figure 2. WED promotes platelet recovery in RIT mice. (A) Schematic diagram illustrating the study design for the treatment of RIT model mice with WED. (B–E) Changes in platelet parameters, including (B) platelet counts, (C) PDW, (D) MPV, (E) P-LCR, were measured in peripheral blood on days 0, 3, 7, 10, and 12 after RIT mice were treated with WED. Data are displayed as mean ± SD (n = 10). *p < 0.05, **p < 0.01, ***p < 0.001 vs. the model. #p < 0.05, ##p < 0.01, ###p < 0.001 vs. control group. Ctrl: Control.
3.3 WED promotes the production of HSCs, megakaryocytes, and reticulated platelets in RIT mice
It is well established that hematopoietic cells are highly sensitive to radiation and undergo apoptosis immediately following exposure (Li et al., 2016). As the BM is the primary hematopoietic organ (Guo et al., 2021), we examined the percentages of hematopoietic cells in the BM of each group of mice. Flow cytometry analysis revealed significant increases in the percentages of c-Kit+CD34+ (hematopoietic stem progenitor) (Figure 3A), c-Kit+CD41+ (megakaryocytic progenitor) (Figure 3B), and CD41+CD42d+ (megakaryocyte) (Figure 3C) cells in the TPO- and WED-treated groups compared to the model group. These results suggest that WED can promote various stages of megakaryopoiesis.
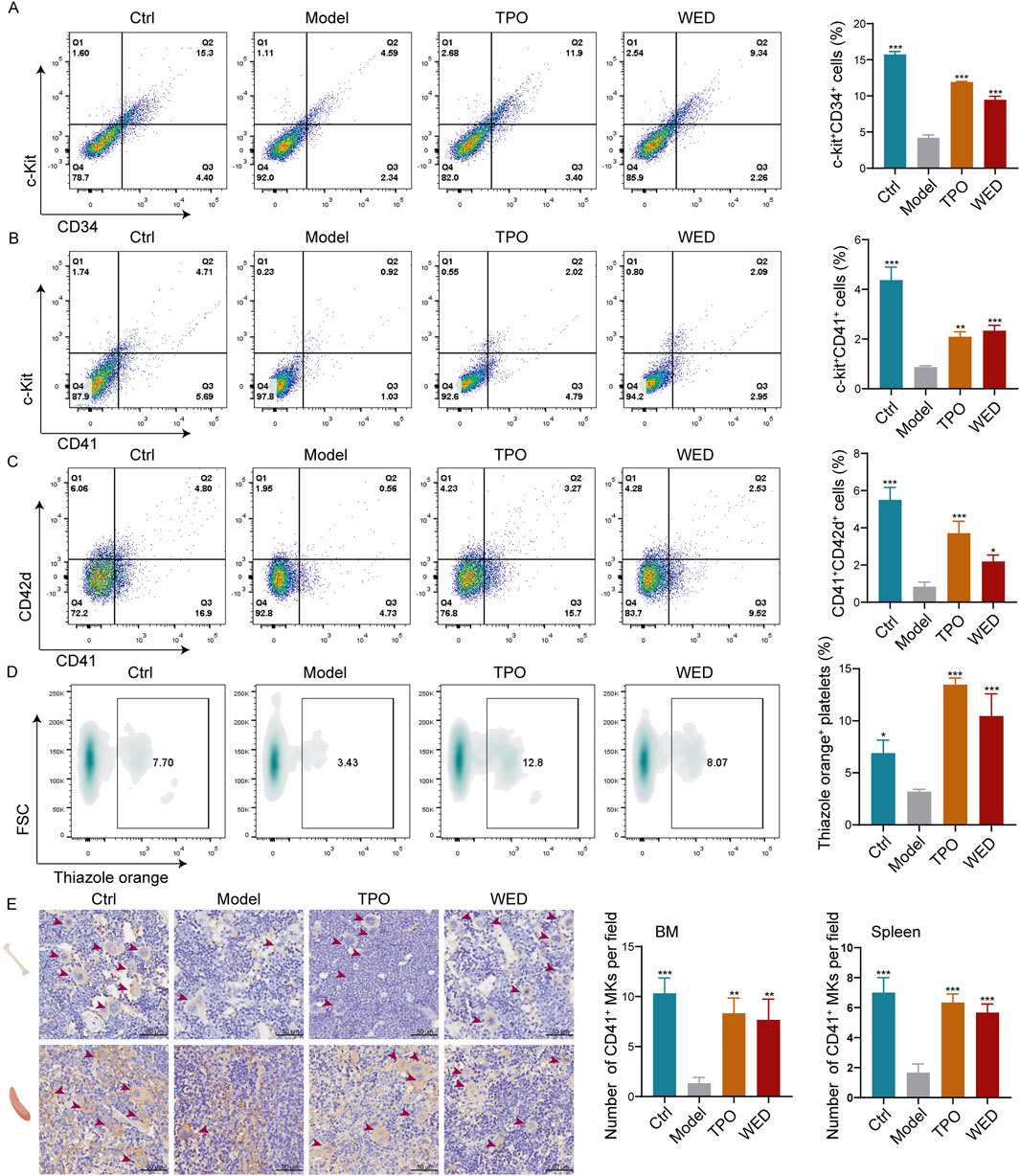
Figure 3. WED stimulates the production of both megakaryocytes and platelets in vivo. (A–C) Flow cytometry analyses of c-Kit+CD34+ (A), c-Kit+CD41+ (B), and CD41+CD42d+ (C) cell percentages in the BM after 10 days of treatment. Histograms represent the proportion of positive cells in each group. (D) Thiazole orange-positive platelets in peripheral blood. Histograms represent the proportion of positive platelets in each group. (E) Immunohistochemical staining of CD41 in the BM and spleen tissue. The CD41+ MKs are indicated by arrows. Scale bar = 50 μm. Histograms represent the proportion of CD41+ cells in BM and spleen across groups. Data are displayed as mean ± SD (n = 3). *p < 0.05, **p < 0.01, ***p < 0.001 vs. model group.
Previous studies demonstrated a gradual increase in peripheral platelet counts in RIT mice following WED treatment. To investigate the origin of this increase, we used thiazole orange staining to assess the production of new platelets (Kostyak et al., 2014). Flow cytometry analysis showed a significant increase in thiazole orange-positive platelets in both the TPO and WED-treated groups (Figure 3D), indicating enhanced de novo platelet production following WED administration. Additionally, as megakaryocytes, predominantly located in the BM and partially in the spleen, are responsible for platelet production (Ma et al., 2019), we conducted immunohistochemical staining for CD41 in the BM and spleen. The data demonstrated a notable increase in megakaryocyte numbers in the BM of TPO- and WED-treated mice compared with model group, with analogous findings in the spleen (Figure 3E). These findings suggest that WED promotes the generation of both megakaryocytes and platelets in RIT mice.
3.4 Gene expression profile associated with WED-induced megakaryocyte differentiation
The molecular mechanisms underlying WED-induced megakaryocyte differentiation were investigated through RNA sequencing. A heat map highlighted the changes in overall gene expression between control and WED-treated groups (Figure 4A). Volcano plot revealed 1869 DEGs, with 732 upregulated and 1,137 downregulated in the WED-treated group compared to control (Figure 4B). To further understand the functional roles of the WED-regulated DEGs, GO and KEGG pathway enrichment analyses were conducted. GO analysis revealed that the DEGs were predominantly associated with mitochondrial metabolism-related processes, such as ATP metabolic process, positive regulation of mitochondrial translation, positive regulation of megakaryocyte differentiation, and ATPase activity (Figure 4C). KEGG analysis revealed that these DEGs were significantly enriched in metabolic pathways, oxidative phosphorylation, and related processes (Figure 4D). These findings suggest that mitochondrial metabolism is crucial for WED-induced megakaryocyte differentiation.
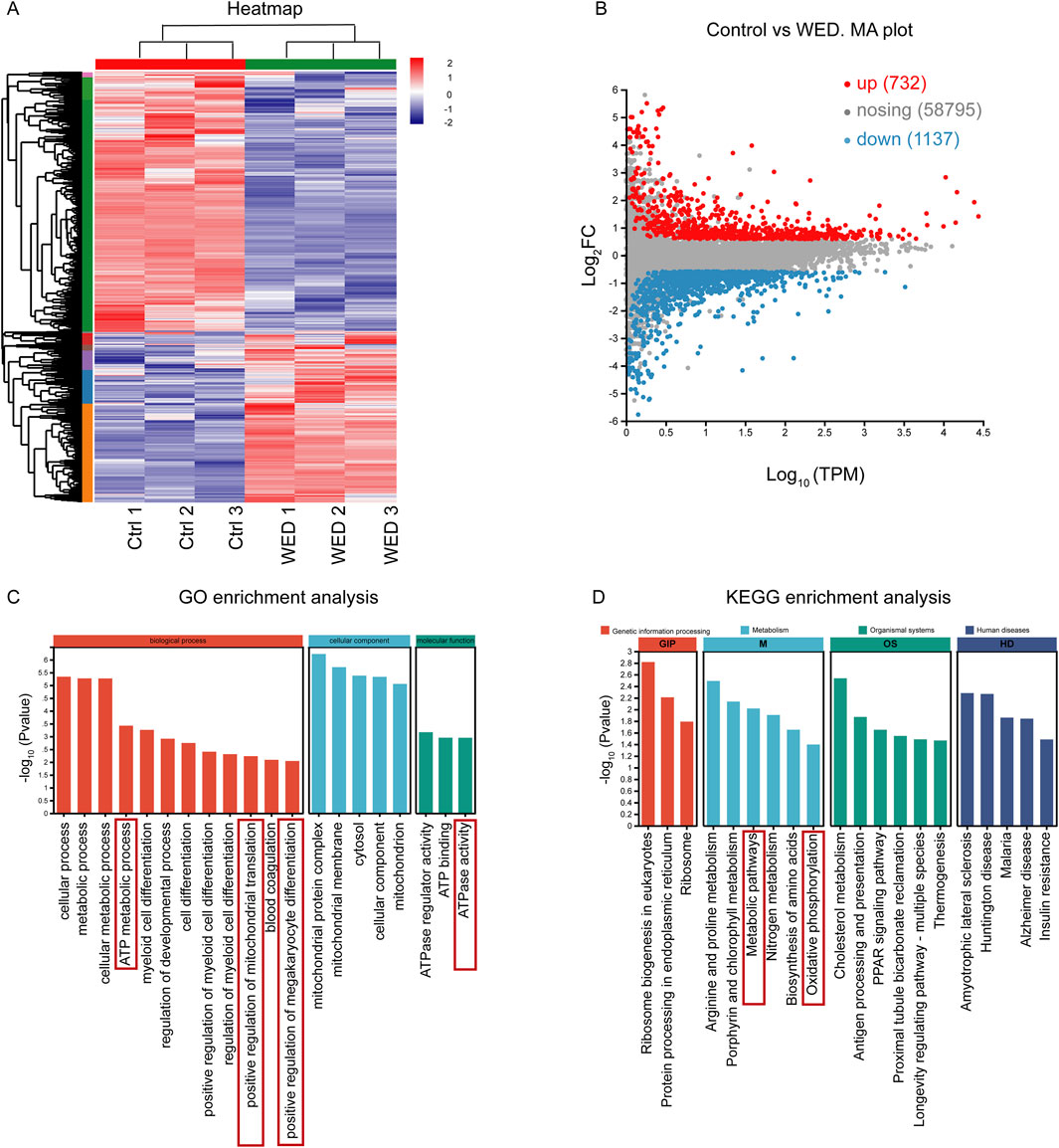
Figure 4. RNA sequencing analysis. (A) Heatmap displaying gene expression changes. (B) MA plot showing DEGs with upregulated (red) or downregulated (blue). (C) GO enrichment analysis. (D) KEGG enrichment analysis.
3.5 WED induces megakaryocyte differentiation by regulating oxidative phosphorylation
To elucidate the involvement of mitochondrial metabolism and oxidative phosphorylation in WED-induced megakaryocyte differentiation, several mitochondrial metabolism-related indicators were examined, including MMP, cellular ATP levels, ROS content, and mitochondrial mass. The data revealed that WED treatment resulted in a decrease in MMP (Figures 5A,C), accompanied by increased ATP levels (Figure 5B), elevated ROS production (Figure 5D), and enhanced mitochondrial mass (Figure 5E). Analogous results were detected in BM cells of RIT mice (Supplementary Figure S1B,C). The MMP of BM cells increased in both the TPO and WED-treated groups compared to the model group, suggesting that WED may regulate MMP (Supplementary Figure S1A). To further validate the involvement of mitochondrial oxidative phosphorylation in megakaryocyte differentiation, Carbonyl Cyanide m-Chlorophenylhydrazone (CCCP), an inhibitor of mitochondrial oxidative phosphorylation, was added, and flow cytometry analysis showed that CCCP significantly inhibited WED-induced expression of CD41 and CD61 (Figure 5F). Indicating that mitochondrial oxidative phosphorylation is integral to WED-induced megakaryocyte differentiation.
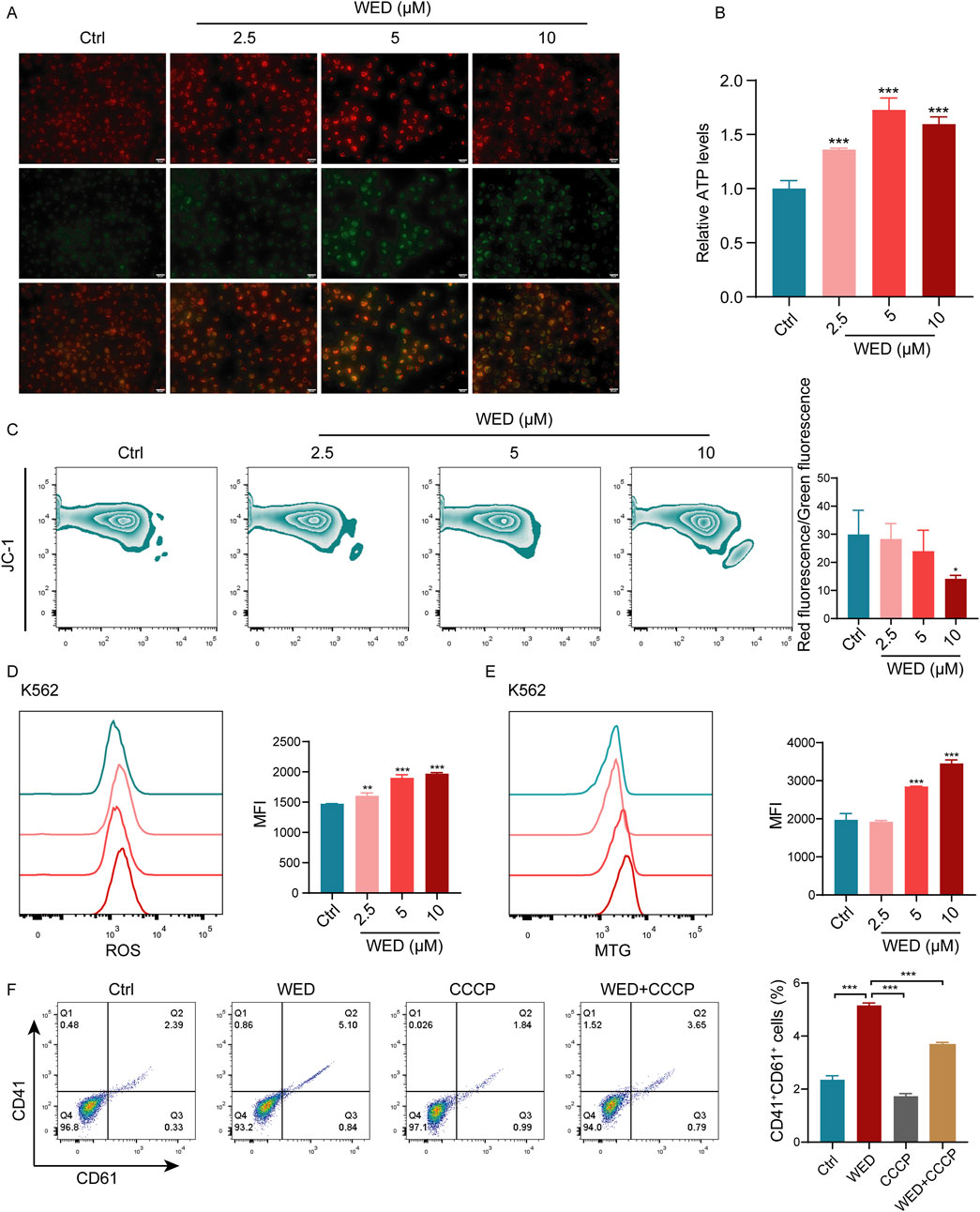
Figure 5. Mitochondrial oxidative phosphorylation contributes to WED-induced megakaryocyte differentiation. (A) Fluorescence images of JC-1-stained K562 cells showing changes in MMP. (B) Intracellular ATP levels in K562 cells after 5 days of WED treatment. (C–E) Flow cytometry evaluation of MMP (C), ROS levels (D), and mitochondrial mass (E) in K562 cells after 5 days of WED intervention. *p < 0.05, **p < 0.01, ***p < 0.001. vs the control group. (F) Expression of CD41 and CD61. Histograms display the percentage of CD41+CD61+ cells. Data are displayed as mean ± SD (n = 3). ***p < 0.001 vs. the WED group.
Moreover, RNA sequencing indicated that WED-induced differential genes were significantly enriched in ATP metabolic processes, ATPase regulator activity, ATP binding, ATPase activity, and other pathways related to energy metabolism. Given that AMPK serves as a key regulator of energy metabolism (Herzig and Shaw, 2018), we further investigated the effect of WED on the AMPK signaling pathway. Western blot analysis confirmed that WED significantly promoted AMPK phosphorylation (Supplementary Figure S1D), while the addition of the AMPK inhibitor compound C inhibited WED-induced ATP production (Supplementary Figure S1E). These findings imply that AMPK phosphorylation is essential for WED-regulated mitochondrial oxidative phosphorylation, which may subsequently induce the differentiation of megakaryocytes.
3.6 The MAPK signaling pathway is activated during WED-promoted megakaryocyte differentiation
GO analysis revealed that DEGs were enriched in the positive regulation of megakaryocyte differentiation. The MAPK signaling pathway is a crucial intracellular network that governs cellular proliferation and differentiation (Lieu et al., 2017), with numerous studies reporting its role in regulating megakaryocyte differentiation (Manne et al., 2022; Zhou et al., 2024). Additionally, previous research has shown that WED attenuates pulmonary fibrosis in part by activating AMPK and modulating the MAPK signaling pathway (Yang et al., 2019). Therefore, we investigated the role of the MAPK signaling pathway in WED-induced megakaryocyte differentiation. The results show that WED significantly induced the expression of RAS protein and promoted the phosphorylation of MEK and ERK (Figures 6A–C). Additionally, WED enhanced the expression of transcription factors FOS, NF-E2, and GATA1 (Figures 6D–F), which are essential for megakaryocyte differentiation. Flow cytometry analysis further demonstrated that both the AMPK inhibitor compound C and the MAPK inhibitor SCH772984 suppressed WED-induced CD41 and CD61 expressions (Figure 6G). These findings suggest that AMPK-mediated oxidative phosphorylation and the MAPK signaling pathway are essential for WED-induced megakaryocyte differentiation.
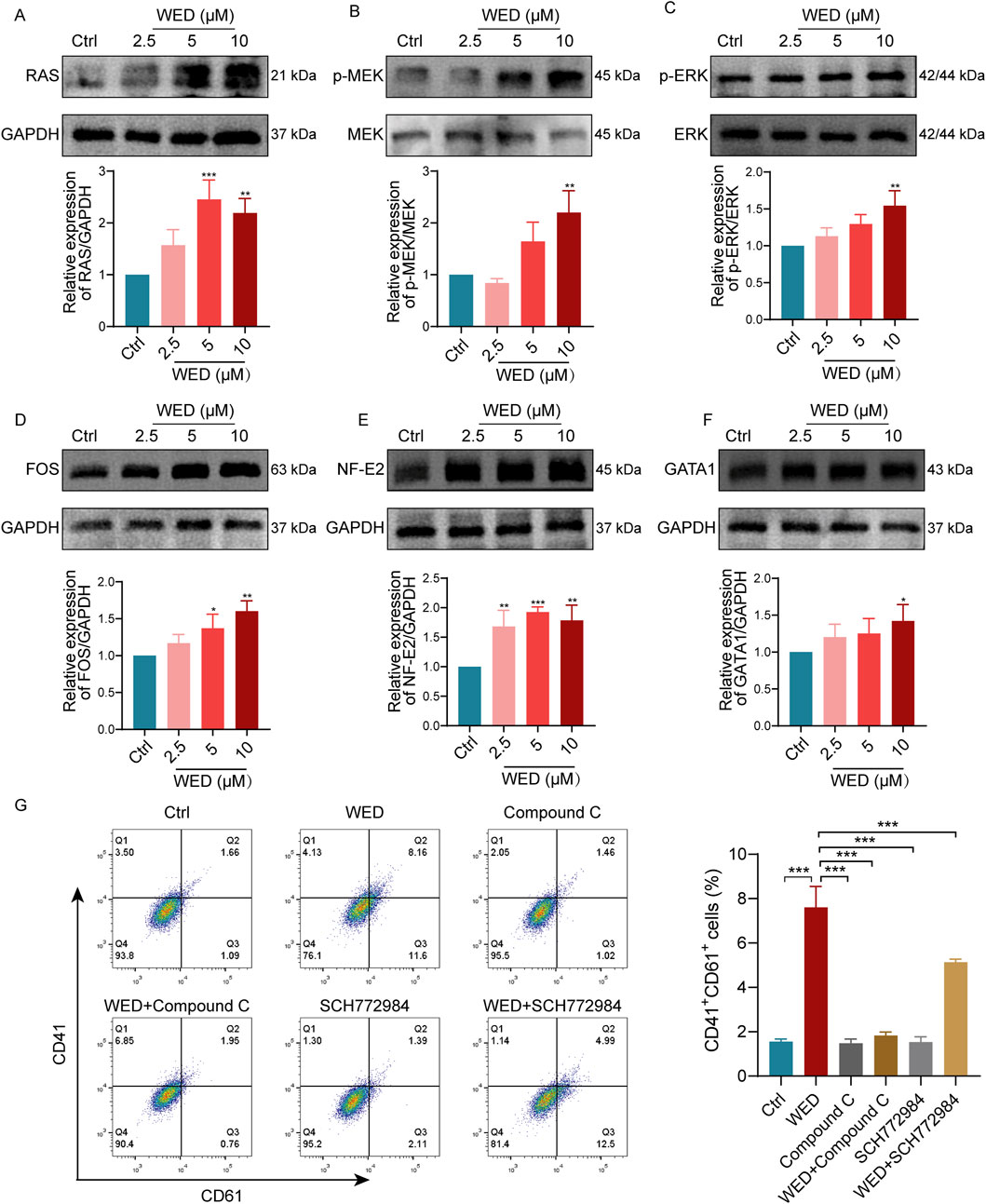
Figure 6. MAPK signaling pathway is necessary for WED-induced megakaryocyte maturation. (A-F) Western blot analysis showing the expression levels of RAS, p-MEK, p-ERK, FOS, NF-E2, and GATA1 in cells exposed to WED (2.5, 5, and 10 μM). *p < 0.05, **p < 0.01, ***p < 0.001 vs. the control group. (G) Flow cytometry analysis, with histograms displaying the proportion of CD41+CD61+ cells. Data are displayed as mean ± SD (n = 3). ***p < 0.001 vs. the WED group.
4 Discussion
Thrombocytopenia is a prevalent and acute complication of chemotherapy and radiotherapy, leading to symptoms such as severe bleeding and increased infection risk, which can interrupt treatment and negatively impact patient outcomes (Zhou et al., 2024). Although current therapeutic strategies like platelet transfusions, thrombopoietin receptor agonists, and glucocorticoids are used to manage thrombocytopenia, their limitations, including side effects and restricted applicability, have driven the search for alternative treatments (Zhang T. et al., 2023). The metabolites of botanical drugs offer a promising avenue for drug development due to their diverse biological activities and low toxicity (Kim et al., 2015). Extensive biomedical research on WED has demonstrated its diverse pharmacological properties, including anticancer, anti-inflammatory, antidiabetic, anti-obesity, antioxidant, antiviral, and anti-aging activities. Additionally, WED exhibits cardiovascular benefits and serine protease inhibition, with notable protective effects on vital organs such as the liver, lungs, bones, and teeth (Cheng et al., 2019; Das et al., 2019; Sarwar et al., 2021; Ha et al., 2023). In this research, we explored the therapeutic potential of WED, a natural bioactive metabolite, in promoting megakaryocyte differentiation and platelet recovery in the context of radiation-induced thrombocytopenia.
The findings of this research indicate that WED significantly promotes the differentiation of megakaryocytes and enhances platelet recovery in the RIT mice model. In vitro, WED markedly increased the expression of megakaryocyte lineage markers CD41 and CD61 and promoted megakaryocyte polyploidization, a key step in platelet biogenesis. These findings were further confirmed in vivo, where WED-treated mice exhibited significant recovery of platelet counts following irradiation. Flow cytometry analysis revealed that WED enhanced the production of HSCs, megakaryocyte progenitors, mature megakaryocytes, and new platelets in the BM. These results were corroborated by immunohistochemical staining, which showed an elevation in megakaryocyte numbers in both the BM and spleen. Transcriptomic analysis provided insights into the molecular basis of WED’s action, with GO analysis revealed that the DEGs were predominantly associated with mitochondrial metabolism-related processes, such as ATP metabolic process, positive regulation of mitochondrial translation and ATPase activity. Notably, KEGG pathway analyses identified enrichment of oxidative phosphorylation and metabolic processes. To validate the results from RNA sequencing and enhance the reliability of our findings, the molecular mechanism of WED-induced megakaryocyte differentiation was further systematically confirmed using Western blot and flow cytometry.
Mitochondria are essential for cellular energy production through oxidative phosphorylation, which generates ATP, a critical energy source for cell differentiation and proliferation (Valentin-Vega et al., 2012). In this study, WED was found to enhance mitochondrial activity by increasing ATP production and mitochondrial mass while regulating MMP. These changes indicate that WED modulates mitochondrial function to support the energy demands of megakaryocyte differentiation. Furthermore, the addition of CCCP, a mitochondrial oxidative phosphorylation inhibitor, significantly suppressed WED-induced expression of CD41 and CD61, confirming the involvement of mitochondrial oxidative phosphorylation in this process. The process of oxidative phosphorylation leads to the production of ROS (Lu et al., 2022), and increased ROS has been reported to promote megakaryocyte maturation and platelet production (Beaulieu et al., 2011). Additionally, WED activates the AMPK signaling pathway, a key regulator of energy metabolism (Lian et al., 2019). AMPK activation is known to enhance mitochondrial biogenesis and promote oxidative phosphorylation (Weimer et al., 2014; Herzig and Shaw, 2018). Western blot analysis revealed that WED significantly increased the phosphorylation of AMPK, while inhibition of AMPK with compound C attenuated WED-induced ATP production and megakaryocyte differentiation. These results suggest that AMPK-mediated regulation of mitochondrial metabolism is critical for the pro-differentiation effects of WED. The MAPK pathway, particularly the MEK/ERK cascade, is identified as modulating cell proliferation, differentiation, and survival (Wang et al., 2022). Western blot analysis confirmed that WED significantly stimulated the expression of RAS and the phosphorylation of MEK and ERK. Furthermore, WED enhanced the expression of transcription factors FOS, NF-E2, and GATA1, which are vital for the concluding phases of megakaryocyte development and proplatelet synthesis (Kamal et al., 2018; Zhou et al., 2024). Inhibition of the MAPK pathway with SCH772984 suppressed WED-induced CD41/CD61 expression, demonstrating the importance of MAPK signaling in WED-mediated megakaryocyte differentiation.
However, like many polyphenols, WED has been identified as a potential pan-assay interference compound. These compounds are known for their ability to interact with multiple molecular targets and exhibit so-called “promiscuous” binding, which can result in non-specific or artifactual outcomes in various bioassays (Heinrich et al., 2020). To address this limitation, future studies will incorporate additional technical approaches—such as drug target identification techniques, gene knockdown strategies, and multi-omics analyses—to rigorously elucidate the molecular basis of WED’s pro-hematopoietic effects.
In summary, this study demonstrates that mitochondrial oxidative phosphorylation and MAPK signaling pathways play a key role in WED-mediated promotion of megakaryocyte differentiation and maturation. Moreover, WED was found to enhance hematopoiesis and restore platelet counts in mice following irradiation-induced injury. Based on both our in vitro and in vivo findings, WED may represent a promising therapeutic candidate for the treatment of thrombocytopenia. These results provide a theoretical foundation and offer new insights for the development of novel thrombocytopenia therapies (Figure 7).
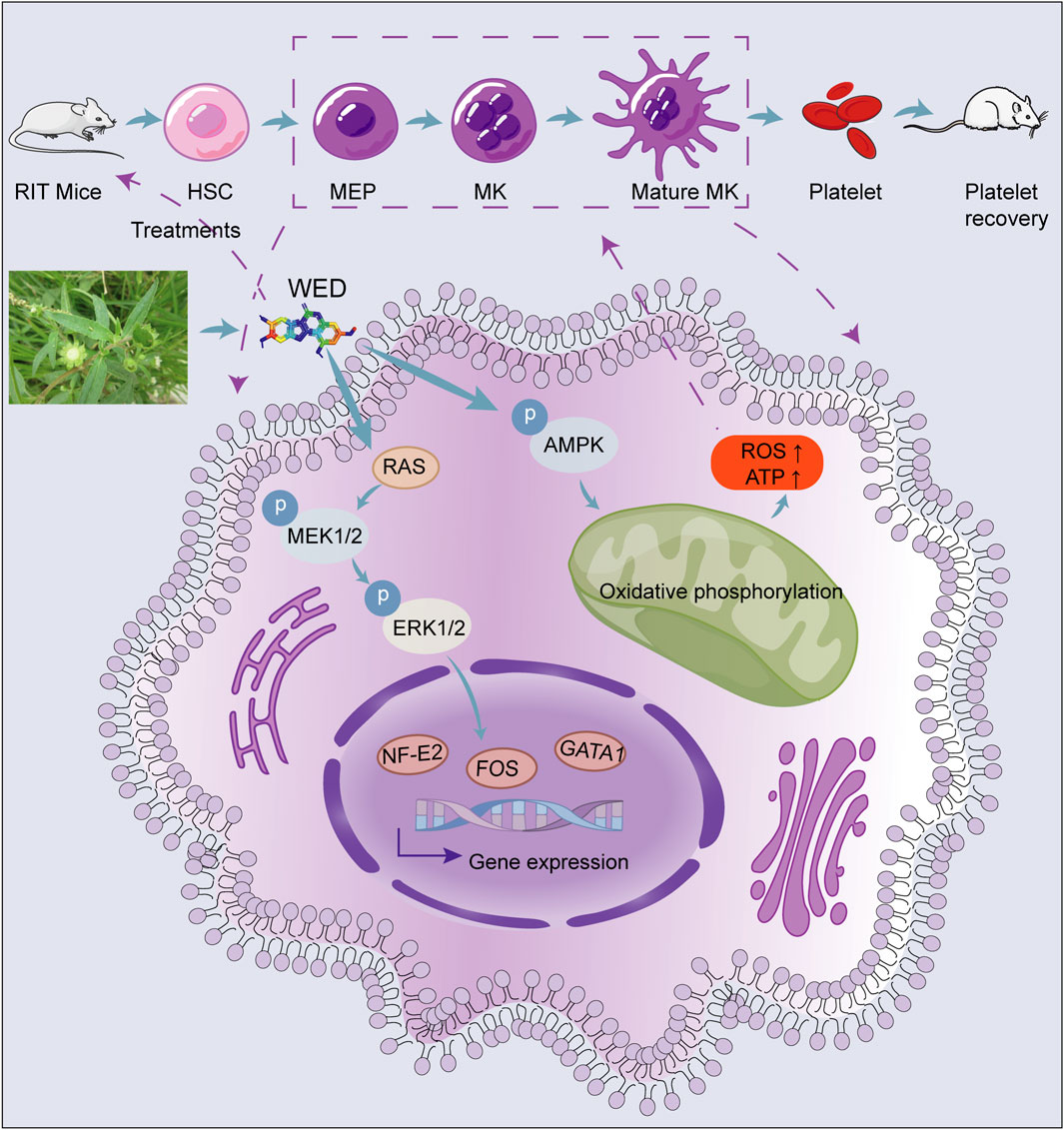
Figure 7. Schematic model showing the role of WED in regulating megakaryocyte differentiation and platelet generation. WED promotes megakaryocyte differentiation and platelet generation by activating the MAPK signaling pathway (RAS/MEK/ERK1/2) and modulating oxidative phosphorylation via the AMPK signaling pathway. Activation of MAPK signaling results in the upregulation of key hematopoietic transcription factors, including FOS, NF-E2, and GATA1, which are essential for megakaryocyte differentiation and maturation. This process ultimately leads to the restoration of platelet counts, as demonstrated in the RIT mice model. MEP: Megakaryocyte-erythroid progenitor.
5 Conclusion
These results offer fresh perspectives on the mechanisms by which WED regulates megakaryocyte differentiation and highlight its potential as a therapeutic agent for thrombocytopenia. Future research should explore the clinical applicability of WED and investigate the broader implications of mitochondrial regulation in haematopoietic diseases.
Data availability statement
The datasets presented in this study can be found in online repositories. The names of the repository/repositories and accession number(s) can be found below: https://www.ncbi.nlm.nih.gov/, PRJNA1162465.
Ethics statement
Ethical approval was not required for the studies on humans in accordance with the local legislation and institutional requirements because only commercially available established cell lines were used. The animal study was approved by this study was conducted in accordance with ARRIVE guidelines and approved by the laboratory animal ethics committee of the Southwest Medical University (Luzhou, China, License No. 20220228-024). The study was conducted in accordance with the local legislation and institutional requirements.
Author contributions
ZL: Data curation, Formal Analysis, Investigation, Methodology, Validation, Visualization, Writing - original draft. QL: Investigation, Methodology, Validation, Writing - original draft. SW: Investigation, Validation, Writing - original draft. XM: Methodology, Validation, Writing - original draft. XQ: Methodology, Validation, Writing - original draft. SL: Software, Validation, Writing - original draft. GQ: Investigation, Methodology, Writing - original draft. HS: Conceptualization, Resources, Writing - original draft. JL: Software, Visualization, Writing - original draft. JZ: Conceptualization, Formal Analysis, Writing - original draft. FH: Methodology, Writing - original draft. RL: Conceptualization, Data curation, Resources, Supervision, Writing - review and editing. LW: Conceptualization, Data curation, Formal Analysis, Funding acquisition, Project administration, Resources, Supervision, Writing - original draft, Writing - review and editing.
Funding
The author(s) declare that financial support was received for the research and/or publication of this article. This work was supported by the National Science Foundation of China (82204666, 82074129, 81774013 and 82273889), the Sichuan Provincial Natural Science Foundation General Project (2024NSFSC0711, 2023NSFSC0657), the Joint Project of Luzhou Municipal People’s Government and Southwest Medical University (2024LZXNYDJ030), the Science and Technology Program Joint Innovation Project of Sichuan Province (2022YFS0635 and 2022YFS0635-B1), the Sichuan Outstanding Youth Fund Project (2022JDJQ0061), and the National Innovation and Entrepreneurship Training Program for College Students of China (202410632006, 202210632031, 202310632102 and S202310632303).
Conflict of interest
The authors declare that the research was conducted in the absence of any commercial or financial relationships that could be construed as a potential conflict of interest.
Generative AI statement
The authors declare that no Generative AI was used in the creation of this manuscript.
Publisher’s note
All claims expressed in this article are solely those of the authors and do not necessarily represent those of their affiliated organizations, or those of the publisher, the editors and the reviewers. Any product that may be evaluated in this article, or claim that may be made by its manufacturer, is not guaranteed or endorsed by the publisher.
Supplementary material
The Supplementary Material for this article can be found online at: https://www.frontiersin.org/articles/10.3389/fphar.2025.1508215/full#supplementary-material
Abbreviations
RIT, Radiation-induced thrombocytopenia; WED, Wedelolactone; HSCs, Hematopoietic stem cells; MK, Megakaryocyte; BM, Bone marrow; K562, Human chronic myeloid leukemia cell line; Meg-01, Human megakaryocytic leukemia cell line; PMA, Phorbol 12-myristate 13-acetate; PBS, Phosphate Buffered Saline; KM, Kunming; TPO, Thrombopoietin; MTG, Mito-Tracker green; ROS, Reactive oxygen species; MMP, Mitochondrial membrane potential; ATP, Adenosine triphosphate; DEGs, Differentially expressed genes; PBST, PBS with 2% Tween-20; PDW, Platelet distribution width; MPV, Mean platelet volume; P-LCR, platelet-large cell ratio; KEGG, Kyoto encyclopedia of genes and genomes; GO, Gene ontology; CCCP, Carbonyl cyanide m-chlorophenylhydrazone.
References
Beaulieu, L. M., Lin, E., Morin, K. M., Tanriverdi, K., and Freedman, J. E. (2011). Regulatory effects of TLR2 on megakaryocytic cell function. Blood 117 (22), 5963–5974. doi:10.1182/blood-2010-09-304949
Chase, T. H., Lyons, B. L., Bronson, R. T., Foreman, O., Donahue, L. R., Burzenski, L. M., et al. (2010). The mouse mutation “thrombocytopenia and cardiomyopathy” (trac) disrupts Abcg5: a spontaneous single gene model for human hereditary phytosterolemia/sitosterolemia. Blood 115 (6), 1267–1276. doi:10.1182/blood-2009-05-219808
Chen, S., Sun, K., Xu, B., Han, S., Wang, S., Xu, Y., et al. (2023). Akt-mediated mitochondrial metabolism regulates proplatelet formation and platelet shedding post vasopressin exposure. J. Thromb. Haemost. 21 (2), 344–358. doi:10.1016/j.jtha.2022.11.018
Chen, Y., Luo, L., Zheng, Y., Zheng, Q., Zhang, N., Gan, D., et al. (2022). Association of platelet desialylation and circulating follicular helper T cells in patients with thrombocytopenia. Front. Immunol. 13, 810620. doi:10.3389/fimmu.2022.810620
Cheng, M., Lin, J., Li, C., Zhao, W., Yang, H., Lv, L., et al. (2019). Wedelolactone suppresses IL-1β maturation and neutrophil infiltration in Aspergillus fumigatus keratitis. Int. Immunopharmacol. 73, 17–22. doi:10.1016/j.intimp.2019.04.050
Das, S., Mukherjee, P., Chatterjee, R., Jamal, Z., and Chatterji, U. (2019). Enhancing chemosensitivity of breast cancer stem cells by downregulating SOX2 and ABCG2 using wedelolactone-encapsulated nanoparticles. Mol. Cancer Ther. 18 (3), 680–692. doi:10.1158/1535-7163.Mct-18-0409
Du, C. H., Wu, Y. D., Yang, K., Liao, W. N., Ran, L., Liu, C. N., et al. (2023). Apoptosis-resistant megakaryocytes produce large and hyperreactive platelets in response to radiation injury. Mil. Med. Res. 10 (1), 66. doi:10.1186/s40779-023-00499-z
Ghalloussi, D., Dhenge, A., and Bergmeier, W. (2019). New insights into cytoskeletal remodeling during platelet production. J. Thromb. Haemost. 17 (9), 1430–1439. doi:10.1111/jth.14544
Greenberg, E. M. (2017). Thrombocytopenia: a destruction of platelets. J. Infus. Nurs. 40 (1), 41–50. doi:10.1097/nan.0000000000000204
Guo, H., Chang, Y. J., Hong, Y., Xu, L. P., Wang, Y., Zhang, X. H., et al. (2021). Dynamic immune profiling identifies the stronger graft-versus-leukemia (GVL) effects with haploidentical allografts compared to HLA-matched stem cell transplantation. Cell Mol. Immunol. 18 (5), 1172–1185. doi:10.1038/s41423-020-00597-1
Gutti, U., Komati, J. K., Kotipalli, A., Saladi, R. G. V., and Gutti, R. K. (2018). Justicia adhatoda induces megakaryocyte differentiation through mitochondrial ROS generation. Phytomedicine 43, 135–139. doi:10.1016/j.phymed.2018.04.038
Ha, N. M., Hop, N. Q., and Son, N. T. (2023). Wedelolactone: a molecule of interests. Fitoterapia 164, 105355. doi:10.1016/j.fitote.2022.105355
Heinrich, M., Appendino, G., Efferth, T., Fürst, R., Izzo, A. A., Kayser, O., et al. (2020). Best practice in research - overcoming common challenges in phytopharmacological research. J. Ethnopharmacol. 246, 112230. doi:10.1016/j.jep.2019.112230
Herzig, S., and Shaw, R. J. (2018). AMPK: guardian of metabolism and mitochondrial homeostasis. Nat. Rev. Mol. Cell Biol. 19 (2), 121–135. doi:10.1038/nrm.2017.95
Jin, X., Yu, H., Wang, B., Sun, Z., Zhang, Z., Liu, Q. S., et al. (2021). Airborne particulate matters induce thrombopoiesis from megakaryocytes through regulating mitochondrial oxidative phosphorylation. Part Fibre Toxicol. 18 (1), 19. doi:10.1186/s12989-021-00411-4
Kamal, T., Green, T. N., Hearn, J. I., Josefsson, E. C., Morel-Kopp, M. C., Ward, C. M., et al. (2018). N-methyl-d-aspartate receptor mediated calcium influx supports in vitro differentiation of normal mouse megakaryocytes but proliferation of leukemic cell lines. Res. Pract. Thromb. Haemost. 2 (1), 125–138. doi:10.1002/rth2.12068
Katsube, T., Shimizu, R., Fukuhara, T., Kano, T., and Wajima, T. (2019). Pharmacokinetic/pharmacodynamic modelling and simulation of lusutrombopag, a novel thrombopoietin receptor agonist, for the treatment of thrombocytopenia in patients with chronic liver disease undergoing invasive procedures. Clin. Pharmacokinet. 58 (11), 1469–1482. doi:10.1007/s40262-019-00770-4
Kim, N. H., Pham, N. B., Quinn, R. J., Shim, J. S., Cho, H., Cho, S. M., et al. (2015). The small molecule R-(-)-β-O-methylsynephrine binds to nucleoporin 153 kDa and inhibits angiogenesis. Int. J. Biol. Sci. 11 (9), 1088–1099. doi:10.7150/ijbs.10603
Kostyak, J. C., Bhavanasi, D., Liverani, E., McKenzie, S. E., and Kunapuli, S. P. (2014). Protein kinase C δ deficiency enhances megakaryopoiesis and recovery from thrombocytopenia. Arterioscler. Thromb. Vasc. Biol. 34 (12), 2579–2585. doi:10.1161/atvbaha.114.304492
Lai, J., Li, Y., Ran, M., Huang, Q., Huang, F., Zhu, L., et al. (2023). Xanthotoxin, a novel inducer of platelet formation, promotes thrombocytopoiesis via IL-1R1 and MEK/ERK signaling. Biomed. Pharmacother. 163, 114811. doi:10.1016/j.biopha.2023.114811
Lasne, D., Pascreau, T., Darame, S., Bourrienne, M. C., Tournoux, P., Philippe, A., et al. (2020). Measuring beta-galactose exposure on platelets: standardization and healthy reference values. Res. Pract. Thromb. Haemost. 4 (5), 813–822. doi:10.1002/rth2.12369
Li, X. H., Ha, C. T., and Xiao, M. (2016). MicroRNA-30 inhibits antiapoptotic factor Mcl-1 in mouse and human hematopoietic cells after radiation exposure. Apoptosis 21 (6), 708–720. doi:10.1007/s10495-016-1238-1
Lian, X., Wu, X., Li, Z., Zhang, Y., Song, K., Cai, G., et al. (2019). The combination of metformin and 2-deoxyglucose significantly inhibits cyst formation in miniature pigs with polycystic kidney disease. Br. J. Pharmacol. 176 (5), 711–724. doi:10.1111/bph.14558
Lieu, C. H., Hidalgo, M., Berlin, J. D., Ko, A. H., Cervantes, A., LoRusso, P., et al. (2017). A phase ib dose-escalation study of the safety, tolerability, and pharmacokinetics of cobimetinib and duligotuzumab in patients with previously treated locally advanced or metastatic cancers with mutant KRAS. Oncologist 22 (9), 1024–1e89. doi:10.1634/theoncologist.2017-0175
Lin, J., Zeng, J., Liu, S., Shen, X., Jiang, N., Wu, Y. S., et al. (2021). DMAG, a novel countermeasure for the treatment of thrombocytopenia. Mol. Med. 27 (1), 149. doi:10.1186/s10020-021-00404-1
Liu, X., Lai, J., Zhang, X., Wu, A., Zhou, L., Li, Y., et al. (2024). Harmine promotes megakaryocyte differentiation and thrombopoiesis by activating the Rac1/Cdc42/JNK pathway through a potential target of 5-HTR2A. Phytother. Res. 38, 5134–5149. doi:10.1002/ptr.8317
Liu, Z. J., Hoffmeister, K. M., Hu, Z., Mager, D. E., Ait-Oudhia, S., Debrincat, M. A., et al. (2014). Expansion of the neonatal platelet mass is achieved via an extension of platelet lifespan. Blood 123 (22), 3381–3389. doi:10.1182/blood-2013-06-508200
Lu, C., Yang, D., Klement, J. D., Colson, Y. L., Oberlies, N. H., Pearce, C. J., et al. (2022). H3K9me3 represses G6PD expression to suppress the pentose phosphate pathway and ROS production to promote human mesothelioma growth. Oncogene 41 (18), 2651–2662. doi:10.1038/s41388-022-02283-0
Ma, Q., Zhu, C., Zhang, W., Ta, N., Zhang, R., Liu, L., et al. (2019). Mitochondrial PIP3-binding protein FUNDC2 supports platelet survival via AKT signaling pathway. Cell Death Differ. 26 (2), 321–331. doi:10.1038/s41418-018-0121-8
Machlus, K. R., Wu, S. K., Stumpo, D. J., Soussou, T. S., Paul, D. S., Campbell, R. A., et al. (2016). Synthesis and dephosphorylation of MARCKS in the late stages of megakaryocyte maturation drive proplatelet formation. Blood 127 (11), 1468–1480. doi:10.1182/blood-2015-08-663146
Manne, B. K., Campbell, R. A., Bhatlekar, S., Ajanel, A., Denorme, F., Portier, I., et al. (2022). MAPK-interacting kinase 1 regulates platelet production, activation, and thrombosis. Blood 140 (23), 2477–2489. doi:10.1182/blood.2022015568
Mo, Q., Zhang, T., Wu, J., Wang, L., and Luo, J. (2023). Identification of thrombopoiesis inducer based on a hybrid deep neural network model. Thromb. Res. 226, 36–50. doi:10.1016/j.thromres.2023.04.011
Ogura, M., Morishima, Y., Okumura, M., Hotta, T., Takamoto, S., Ohno, R., et al. (1988). Functional and morphological differentiation induction of a human megakaryoblastic leukemia cell line (MEG-01s) by phorbol diesters. Blood 72 (1), 49–60. doi:10.1182/blood.v72.1.49.bloodjournal72149
Raghunathan, S., Rayes, J., and Sen Gupta, A. (2022). Platelet-inspired nanomedicine in hemostasis thrombosis and thromboinflammation. J. Thromb. Haemost. 20 (7), 1535–1549. doi:10.1111/jth.15734
Sarwar, S., Alamro, A. A., Alghamdi, A. A., Naeem, K., Ullah, S., Arif, M., et al. (2021). Enhanced accumulation of cisplatin in ovarian cancer cells from combination with wedelolactone and resulting inhibition of multiple epigenetic drivers. Drug Des. Devel Ther. 15, 2211–2227. doi:10.2147/dddt.S288707
Sharma, D. S., Raghuwanshi, S., Kovuru, N., Dahariya, S., Gautam, D. K., Paddibhatla, I., et al. (2021). Virodhamine, an endocannabinoid, induces megakaryocyte differentiation by regulating MAPK activity and function of mitochondria. J. Cell Physiol. 236 (2), 1445–1453. doi:10.1002/jcp.29949
Sun, G., Hu, C., Mei, Q., Luo, M., Chen, X., Li, Z., et al. (2020). Uncovering the cytochrome P450-catalyzed methylenedioxy bridge formation in streptovaricins biosynthesis. Nat. Commun. 11 (1), 4501. doi:10.1038/s41467-020-18336-5
Swami, R. K., Nimker, S., Narula, A., and Farooqi, H. (2023). Enhanced wedelolactone content in in vitro-raised genetically uniform Wedelia chinensis under the influence of CuSO(4). Front. Plant Sci. 14, 1281445. doi:10.3389/fpls.2023.1281445
Tang, X., Liao, R., Zhou, L., Yi, T., Ran, M., Luo, J., et al. (2024). Genistin: a novel estrogen analogue targeting ERβ to alleviate thrombocytopenia. Int. J. Biol. Sci. 20 (6), 2236–2260. doi:10.7150/ijbs.90483
Tu, Y., Yang, Y., Li, Y., and He, C. (2021). Naturally occurring coumestans from plants, their biological activities and therapeutic effects on human diseases. Pharmacol. Res. 169, 105615. doi:10.1016/j.phrs.2021.105615
Valentin-Vega, Y. A., Maclean, K. H., Tait-Mulder, J., Milasta, S., Steeves, M., Dorsey, F. C., et al. (2012). Mitochondrial dysfunction in ataxia-telangiectasia. Blood 119 (6), 1490–1500. doi:10.1182/blood-2011-08-373639
Wang, L., Liu, S., Luo, J., Mo, Q., Ran, M., Zhang, T., et al. (2023). Targeting a thrombopoietin-independent strategy in the discovery of a novel inducer of megakaryocytopoiesis, DMAG, for the treatment of thrombocytopenia. Haematologica 108 (5), 1394–1411. doi:10.3324/haematol.2022.282209
Wang, Y., Zhang, Y., Mi, J., Jiang, C., Wang, Q., Li, X., et al. (2022). ANKFN1 plays both protumorigenic and metastatic roles in hepatocellular carcinoma. Oncogene 41 (29), 3680–3693. doi:10.1038/s41388-022-02380-0
Weimer, S., Priebs, J., Kuhlow, D., Groth, M., Priebe, S., Mansfeld, J., et al. (2014). D-Glucosamine supplementation extends life span of nematodes and of ageing mice. Nat. Commun. 5, 3563. doi:10.1038/ncomms4563
Xu, X. R., Zhang, D., Oswald, B. E., Carrim, N., Wang, X., Hou, Y., et al. (2016). Platelets are versatile cells: new discoveries in hemostasis, thrombosis, immune responses, tumor metastasis and beyond. Crit. Rev. Clin. Lab. Sci. 53 (6), 409–430. doi:10.1080/10408363.2016.1200008
Yang, J. Y., Tao, L. J., Liu, B., You, X. Y., Zhang, C. F., Xie, H. F., et al. (2019). Wedelolactone attenuates pulmonary fibrosis partly through activating AMPK and regulating raf-MAPKs signaling pathway. Front. Pharmacol. 10, 151. doi:10.3389/fphar.2019.00151
Yang, S., Tang, X., Wang, L., Ni, C., Wu, Y., Zhou, L., et al. (2022). Targeting TLR2/rac1/cdc42/JNK pathway to reveal that ruxolitinib promotes thrombocytopoiesis. Int. J. Mol. Sci. 23 (24), 16137. doi:10.3390/ijms232416137
Zhang, J., Zhang, M., Huo, X. K., Ning, J., Yu, Z. L., Morisseau, C., et al. (2023a). Macrophage inactivation by small molecule wedelolactone via targeting sEH for the treatment of LPS-induced acute lung injury. ACS Cent. Sci. 9 (3), 440–456. doi:10.1021/acscentsci.2c01424
Zhang, T., Mo, Q., Jiang, N., Wu, Y., Yang, X., Chen, W., et al. (2023b). The combination of machine learning and transcriptomics reveals a novel megakaryopoiesis inducer, MO-A, that promotes thrombopoiesis by activating FGF1/FGFR1/PI3K/Akt/NF-κB signaling. Eur. J. Pharmacol. 944, 175604. doi:10.1016/j.ejphar.2023.175604
Keywords: wedelolactone, thrombocytopenia, oxidative phosphorylation, megakaryocyte differentiation, thrombopoiesis
Citation: Li Z, Li Q, Wu S, Mei X, Qi X, Liu S, Qiao G, Shen H, Luo J, Zeng J, Huang F, Li R and Wang L (2025) Regulating mitochondrial oxidative phosphorylation and MAPK signaling: wedelolactone as a novel therapeutic for radiation-induced thrombocytopenia. Front. Pharmacol. 16:1508215. doi: 10.3389/fphar.2025.1508215
Received: 09 October 2024; Accepted: 11 April 2025;
Published: 30 April 2025.
Edited by:
Michael Heinrich, University College London, United KingdomReviewed by:
Ahmed Esmat Abdel Moneim, Helwan University, EgyptRavi Kant Swami, Jamia Hamdard University, India
Meng Wang, Tianjin University of Traditional Chinese Medicine, China
Copyright © 2025 Li, Li, Wu, Mei, Qi, Liu, Qiao, Shen, Luo, Zeng, Huang, Li and Wang. This is an open-access article distributed under the terms of the Creative Commons Attribution License (CC BY). The use, distribution or reproduction in other forums is permitted, provided the original author(s) and the copyright owner(s) are credited and that the original publication in this journal is cited, in accordance with accepted academic practice. No use, distribution or reproduction is permitted which does not comply with these terms.
*Correspondence: Long Wang, d2FuZ2xvbmcxMjI2QHN3bXUuZWR1LmNu; Rong Li, bHIyMDA4QHN3bXUuZWR1LmNu
†These authors have contributed equally to this work and share first authorship