- Department of Pharmacology, School of Medicine, University of California, Davis, Davis, CA, United States
The transmembrane protein Synapse Differentiation Induced Gene 4 (SynDIG4) functions as an auxiliary factor of AMPA receptors (AMPARs) and plays a critical role in excitatory synaptic plasticity as well as hippocampal-dependent learning and memory. Mice lacking SynDIG4 have reduced surface expression of GluA1 and GluA2 and are impaired in single tetanus-induced long-term potentiation and NMDA receptor (NMDAR)-dependent long-term depression. These findings suggest that SynDIG4 may play an important role in regulating AMPAR distribution through intracellular trafficking mechanisms; however, the precise roles by which SynDIG4 governs AMPAR distribution remain unclear. Here, we characterized the endocytosis and recycling of GluA1-containing AMPARs under basal conditions. We did not observe any change in baseline endocytosis; however, we did observe a significant decrease in recycling of GluA1-containing AMPARs in cultured hippocampal neurons from mice lacking SynDIG4. This resulted in a significant increase in the levels of internal GluA1 and GluA2, along with greater colocalization of these subunits with Rab4-positive recycling endosomes. Notably, the overlap between Rab4-positive and Rab11-positive vesicles was elevated in hippocampal neurons lacking SynDIG4, suggesting an impairment in the trafficking between these compartments. Furthermore, our findings revealed a reduction in surface GluA1 within synaptic regions of hippocampal neurons lacking SynDIG4. Collectively, these results indicate that SynDIG4 regulates the distribution of GluA1-containing AMPARs via the Rab4-dependent endosomal recycling pathway, thereby maintaining AMPAR levels at synaptic regions under baseline conditions. This regulatory function of SynDIG4 may contribute to the deficits in GluA1-dependent synaptic plasticity and impairment of hippocampal-dependent behaviors observed in SynDIG4 deficient mice.
Introduction
The spatial and temporal organization of neurotransmitter receptors at the surface of postsynaptic sites is fundamental to efficient synaptic function, which governs how the brain processes and responds to information (Lüscher and Malenka, 2012; Kennedy, 2013; Choquet, 2018). At excitatory synapses, α-amino-3-hydroxy-5-methyl-4-isoxazolepropionic acid (AMPA) receptors (AMPARs) are critical for rapid synaptic transmission. Changes in the number of synaptic AMPARs reflect changes in synaptic strength under both basal and activity-dependent conditions, making them a key molecular mechanism underlying learning and memory (Caya-Bissonnette and Béïque, 2024; Derkach et al., 2007; Huganir and Nicoll, 2013). Thus, the regulatory mechanisms governing AMPAR trafficking within synapses is an area of intense and on-going investigation.
Many studies highlight the importance of dynamic AMPAR trafficking to and from synapses in regulating synaptic expression and modulating synaptic strength. For example, AMPARs can undergo differential sorting in endosomal compartments, rapid recycling to the plasma membrane, and lateral diffusion between synaptic and extrasynaptic sites (Huganir and Nicoll, 2013; Man et al., 2000; Groc et al., 2004; Zheng et al., 2015; Hastings and Man, 2018). The endocytic machinery localized at the postsynaptic region is essential for maintaining basal synaptic transmission and plasticity to ensure the availability of a cycling pool of AMPARs near the postsynaptic density (Ehlers, 2000; Chowdhury et al., 2006; Granger et al., 2013). For instance, the physical coupling of dynamin-3 to Homer anchors the endocytic zone, sustaining mobile surface AMPARs that are essential for regulating synaptic strength (Lu et al., 2007; Petrini et al., 2009).
Small GTPases of the Rab family have emerged as regulators of AMPAR vesicular trafficking (Zerial and McBride, 2001). For instance, Rab4 and Rab11 mediate the recycling of endocytosed AMPARs from sorting and recycling endosomes, respectively, during basal conditions (Gu et al., 2016; Hausser and Schlett, 2019). Impaired fusion between Rab4- and Rab11-positive endosomal compartments has been shown to reduce the surface expression of GluA1-and GluA2-containing AMPARs (Hoogenraad et al., 2010; Chiu et al., 2017). These studies demonstrate that dynamic trafficking of AMPARs is essential for maintaining synaptic function and plasticity, relying on coordinated processes of endocytosis and exocytosis, via diverse endosomal sorting mechanisms to regulate their synaptic expression.
AMPAR auxiliary proteins play critical roles in regulating AMPAR distribution and gating properties (Jackson and Nicoll, 2011; Díaz, 2010; Greger et al., 2017; Jacobi and von Engelhardt, 2017; Qneibi et al., 2024). One such AMPAR auxiliary factor is Synapse Differentiation-Induced Gene 4 (SynDIG4), also known as Proline-Rich Transmembrane Protein 1 (PRRT1), was identified in multiple proteomics studies (Shanks et al., 2012; Schwenk et al., 2012; Chen et al., 2014) and shown to be present in native AMPAR complexes through cryo-electron microscopy (EM) structural analysis (Yu et al., 2021). Notably, the transmembrane domain of SynDIG4 was observed to align approximately parallel to the transmembrane helices of the AMPAR, with the putative interaction site being the M4 helix of GluA1 (Yu et al., 2021). Consistent with these findings, the membrane-associated region of SynDIG4 has been shown to be crucial for the clustering of GluA1 and GluA2 in heterologous cells (Plambeck et al., 2022). Moreover, using heterologous expression in Xenopus oocytes, SynDIG4 was shown to modulate AMPAR gating properties in a subunit-dependent manner (Matt et al., 2018), consistent with a direct interaction. In SynDIG4 knockout (KO) mice, while total levels of AMPARs are unchanged, surface expression of GluA1 and GluA2 was significantly decreased (Troyano-Rodriguez et al., 2019). SynDIG4 overlaps primarily with extrasynaptic AMPARs (Kirk et al., 2016) and localizes to early and recycling endosomes (Martin et al., 2021). Indeed, there is a significant decrease in extrasynaptic GluA1 and GluA2 in SynDIG4 KO neurons (Matt et al., 2018). Furthermore, NMDA receptor (NMDAR)-dependent long-term depression (LTD) (Troyano-Rodriguez et al., 2019) and single tetanus-induced long-term potentiation (LTP) (Matt et al., 2018) are impaired in SynDIG4 KO neurons while baseline synaptic transmission is intact. As these synaptic plasticity mechanisms require calcium-permeable AMPARs (Sanderson et al., 2016), which are mostly GluA1 homomers, together these results suggest that SynDIG4 is required for establishing pools of extrasynaptic AMPARs (both GluA1/GluA2 heteromers and GluA1 homomers) at baseline.
These results motivated us to investigate the mechanisms by which SynDIG4 regulates the trafficking of AMAPRs under basal conditions. We analyzed endocytosis and recycling of GluA1-containing AMPARs in wild type (WT) and SynDIG4 KO hippocampal neurons and brain tissue. While basal endocytosis was unchanged, AMPAR recycling was significantly reduced in SynDIG4 KO neurons, leading to increased intracellular accumulation of GluA1 and GluA2 in Rab4-positive recycling endosomes. Furthermore, there was an increase in the overlap between Rab4-positive and Rab11-positive compartments in SynDIG4 KO neurons, suggesting impaired trafficking between these two compartments. Based on these results, we propose a model in which SynDIG4 plays a critical role in the recycling process of GluA1-containing AMPARs, particularly at the interface between Rab4- and Rab11-mediated pathways, thereby influencing their surface expression and ultimately their synaptic distribution.
Materials and methods
Animals
All animal experiments were conducted in protocols approved by the Institutional Animal Care and Use Committee (IACUC) at the University of California, Davis, following the guidelines of the US Public Health Service and NIH. SynDIG4 KO mice were generated and maintained on a C57BL/6J background as described (Matt et al., 2018). Littermates of both sexes from heterozygous breeding pairs were used for experiments.
Primary hippocampal neuron culture
Hippocampal neurons were isolated from P0∼P2 mice of both sexes and plated onto poly-L-lysine-coated glass coverslips in 6-well plates at a density of 100,000 cells per well. The neurons were cultured in astrocyte-conditioned neuron maintenance medium (NMM), consisting of Neurobasal medium (Gibco, Cat# 21103049) supplemented with GlutaMAX (Gibco, Cat# 35050079) and B27 (Gibco, Cat# A3582801). Half of the NMM volume was changed every 5 days, and neurons were fixed for experiments between days in vitro (DIV) 18 and 22.
Bis-sulfosuccinimidyl suberate (BS3) surface-protein cross-linking assay
Acute coronal brain slices (400 μm thick) were obtained from P14 mice, targeting the hippocampal region, in ice-cold dissection buffer containing (in mM): 127 NaCl, 1.9 KCl, 1.2 KH2PO4, 26 NaHCO3, 10 D-glucose, 23 MgSO4, and 1.1 CaCl2, saturated with 95% O2 and 5% CO2. P14 was selected because SynDIG4 has peak expression at a similar developmental stage in rats (Kirk et al., 2016), making it a suitable time point for our analysis. Following dissection, the slices were maintained in artificial cerebrospinal fluid (ACSF) composed of (in mM): 127 NaCl, 26 NaHCO3, 1.2 KH2PO4, 1.9 KCl, 2.2 CaCl2, 1 MgSO4, and 10 D-glucose, oxygenated with 95% O2 and 5% CO2 for 2 h at 30°C to allow recovery. After recovery, the slices were transferred to 1 mL of ice-cold ACSF and treated with 40 μL of 52 mM BS3 at 4°C for 30 min with gentle mixing. The cross-linking reaction was terminated by adding 100 μL of 1 M glycine and incubating for 10 min. The samples were then centrifuged at 20,000 × g for 2 min at 4°C, after which the supernatant was removed, and the pellet was lysed for protein extraction.
Immunoblotting
Protein samples were heated at 70°C for 10 min and separated by gel electrophoresis using the Bio-Rad Mini-Protean system with 4%–20% gradient gels (Bio-Rad, Cat# 456-1094). After electrophoresis, proteins were transferred to nitrocellulose membranes, which were blocked in 5% milk prepared in Tris-buffered saline (TBS) with 0.1% Tween-20 (TBST) for 60 min at room temperature (RT). Membranes were incubated overnight at 4°C with the following primary antibodies diluted in 5% TBST: GluA1-C (Millipore, Cat# AB1504, 1:1000), GluA2-C (NeuroMab, Cat# SKU: 75-002, 1:1000), GluN1 (BD Bioscience, Cat# 556308, 1:1000) and β-tubulin (Millipore, Cat# 05-661, 1:5000). The next day, membranes were washed three times with TBST and incubated for 2 h at RT with secondary antibodies: Goat anti-Mouse IgG1 488 (Jackson ImmunoResearch Labs, Cat# 112-545-205, 1:500), Goat anti-Mouse IgG IR700 (AzureSpectra, Cat# AC2129, 1:1000), and Goat anti-Rabbit IgG IR800 (AzureSpectra, Cat# AC2134, 1:1000). After incubation, membranes were washed three times with TBST and once with TBS at RT before imaging. Fluorescent immunoblots were imaged using the Sapphire Bioimager (Azure Biosystems, Model# Sapphire RGBNIR) and quantified with Azure Spot software (version 2.0). For analysis, the intensity of GluA1, GluA2, and GluN1 bands at their predicted molecular weight region was normalized to β-tubulin. The internal-to-total ratios of GluA1, GluA2, and GluN1 were compared between WT and SynDIG4 KO samples.
Immunofluorescence
Hippocampal neurons at DIV18∼22 were fixed with 4% paraformaldehyde and 4% sucrose in phosphate buffered saline (PBS) for 7 min (surface labeling) or 10 min (standard labeling) at RT. After fixation, coverslips were washed once with ice-cold PBS and blocked with 5% fetal bovine serum (FBS) in PBS at RT for 2 h. For surface labeling, neurons were incubated overnight at 4°C with primary antibodies before permeabilization. The antibodies used were GluA1-N (NeuroMab, SKU: 75-327, 1:100) and GluA2-N (Millipore, Cat# MAB397, 1:100). For standard labeling, neurons were permeabilized with 0.25% Triton X-100 in PBS for 8 min after fixation. After permeabilization, neurons were incubated at RT for 2 h with primary antibodies. The primary antibodies included MAP2 (Millipore, Cat# AB5622-1, 1:300), PSD95 (Synaptic Systems, Cat# 124014, 1:300), GluA1-C (Millipore, Cat# AB1504, 1:200), GluA2-C (NeuroMab, SKU: 75-002, 1:200), EEA1 (Cell Signaling, Cat# 2411, 1:100), Rab4 (Cell Signaling, Cat# 2167, 1:50), Rab4 (Santa Cruz, Cat# sc-517263, 1:50), and Rab11 (Cell Signaling, Cat# 5589, 1:50), and Rab11 (ThermoFisher, Cat# MA5-49197, 1:50).
After the primary antibody incubation, coverslips were washed with 0.01% Triton X-100 in PBS and incubated at RT for 2 h with secondary antibodies. The secondary antibodies included Goat anti-Rabbit 405 (Jackson ImmunoResearch, Cat# 111-475-003, 1:100), Donkey anti-Rabbit 488 (Jackson ImmunoResearch, Cat# 711-545-152, 1:200), Goat anti-Mouse IgG2a 488 (Jackson ImmunoResearch, Cat# 115-546-206, 1:300), Goat anti-Mouse IgG2b 488 (Jackson ImmunoResearch, Cat# 115-545-207, 1:300), Goat anti-Mouse IgG2a 647 (Jackson ImmunoResearch, Cat# 115-605-206, 1:300), Goat anti-Guinea Pig 647 (Invitrogen, Cat# A-21450, 1:200), and Goat anti-Mouse IgG1 555 (Invitrogen, Cat# A-21127, 1:300). As a cell tracer, neurons were incubated with DiA (4-(4-Dihexadecylaminostyryl)-N-methylpyridinium iodide), a lipophilic dye that inserts into the plasma membranes, overnight at 4°C, where indicated.
Antibody-feeding assay
The antibody-feeding assay was based on published protocols (Zheng et al., 2015; Chiu et al., 2017; Ghane et al., 2019). Hippocampal neurons at DIV18∼22 were incubated with primary antibodies (GluA1-N, 1:50; GluA2-N, 1:50) diluted in conditioned media for 15 min at RT. Following three washes with conditioned media, the neurons were returned to a 37°C incubator for 30 min. For AMPAR endocytosis experiments, neurons were fixed and incubated with fragment antigen-binding (Fab) antibodies (80 μg/mL, Jackson ImmunoResearch Labs, Cat# 715-007-003) for 20 min at RT, followed by permeabilization and subsequent staining using the standard labeling protocol described previously. For AMPAR recycling experiments, after a 30 min internalization step, neurons were treated with Fab antibodies (80 μg/mL) at RT for 20 min and washed three times with conditioned media. The neurons were then treated with dynasore (80 μM; Millipore, SML0340), a dynamin-dependent endocytosis inhibitor (Macia et al., 2006), and incubated at 37°C for 45 min to allow recycling of internalized, live-labeled AMPARs while inhibiting the endocytosis of the remaining surface AMPAR pool. After 45 min, neurons were fixed with 4% paraformaldehyde and 4% sucrose in PBS for 7 min, permeabilized, and subjected to the standard labeling protocol.
Imaging and analysis
Images were acquired using a Leica SP8 instrument in either confocal or Stimulated Emission Depletion (STED) mode, employing a ×63 or ×100 objective, respectively. Z-stack images were captured throughout the entire cell volume. Prior to analysis, images were thresholded, with thresholds for each experiment determined by averaging the thresholds of at least 25% of the images within the dataset. Following maximum projection of the z-stacks, image analysis (integrated density and puncta size) was conducted using FIJI/ImageJ (ver. 2.14.0/1.54f). For STED images, deconvolution was performed using Huygens Software (Scientific Volume Imaging) prior to thresholding. Overlap between signals was calculated using Manders Correlation Coefficient analysis via the JaCoP plugin. For quantification of the amount of surface GluA1 (sGluA1) overlapped with PSD95 puncta, colocalization of sGluA1 and PSD95 was identified by using a PSD95 mask overlaid on sGluA1 signals. The level of sGluA1 at PSD95 was quantified as the ratio of the number of sGluA1 PSD95 colocalized puncta to the total number of sGluA1 puncta. To calculate the association between sGluA1 and PSD95, cross-correlation confidence analysis in Fuji/ImageJ was performed. All the sGluA1 signals excluding the signals overlapped with MAP2 were subjected to calculation. For figure presentation, representative images were adjusted using identical linear level adjustments across all panels within a figure using Adobe Photoshop (Adobe Systems), to ensure consistent signal representation visible to the viewer.
Statistics
Statistical analyses were conducted using GraphPad Prism (version 10.3.1).
Results
Intracellular pools of GluA1 and GluA2 are increased in the absence of SynDIG4
Previous studies demonstrated that surface GluA1 (sGluA1) and surface GluA2 (sGluA2) are decreased in brain lysates from SynDIG4 KO mice through surface-biotinylation assays (Troyano-Rodriguez et al., 2019). To investigate whether intracellular GluA1 (inGluA1) and intracellular GluA2 (inGluA2) are subsequently affected in SynDIG4 KO mice, we applied bis-sulfosuccinimidyl suberate (BS3), a membrane-impermeable reagent that covalently cross-links proteins at the plasma membrane (Boudreau et al., 2012), to acute brain slices obtained from WT and SynDIG4 KO mice. This method allowed the separation of surface and intracellular protein pools, which were subsequently visualized via immunoblotting. After BS3 treatment on one hemisphere of the brain slice, no aggregation of β-tubulin was observed, confirming that BS3 selectively cross-links surface proteins (Figure 1A). Concurrently, sGluA1 and sGluA2 formed aggregates observable in higher molecular weight regions, while inGluA1 and inGluA2 appeared at their predicted apparent molecular weight by using an antibody against an intracellular epitope (Figure 1A). It is worth noting that the signals at higher molecular weight regions may not be quantifiable, as antibody access to the epitope can be hindered following protein cross-linking and aggregation. By comparing the ratio of the intracellular signal from the treated hemisphere to total signal intensity from the untreated hemisphere of the same slice, we found that inGluA1 and inGluA2 levels were both increased in SynDIG4 KO brain slices (Figure 1B).
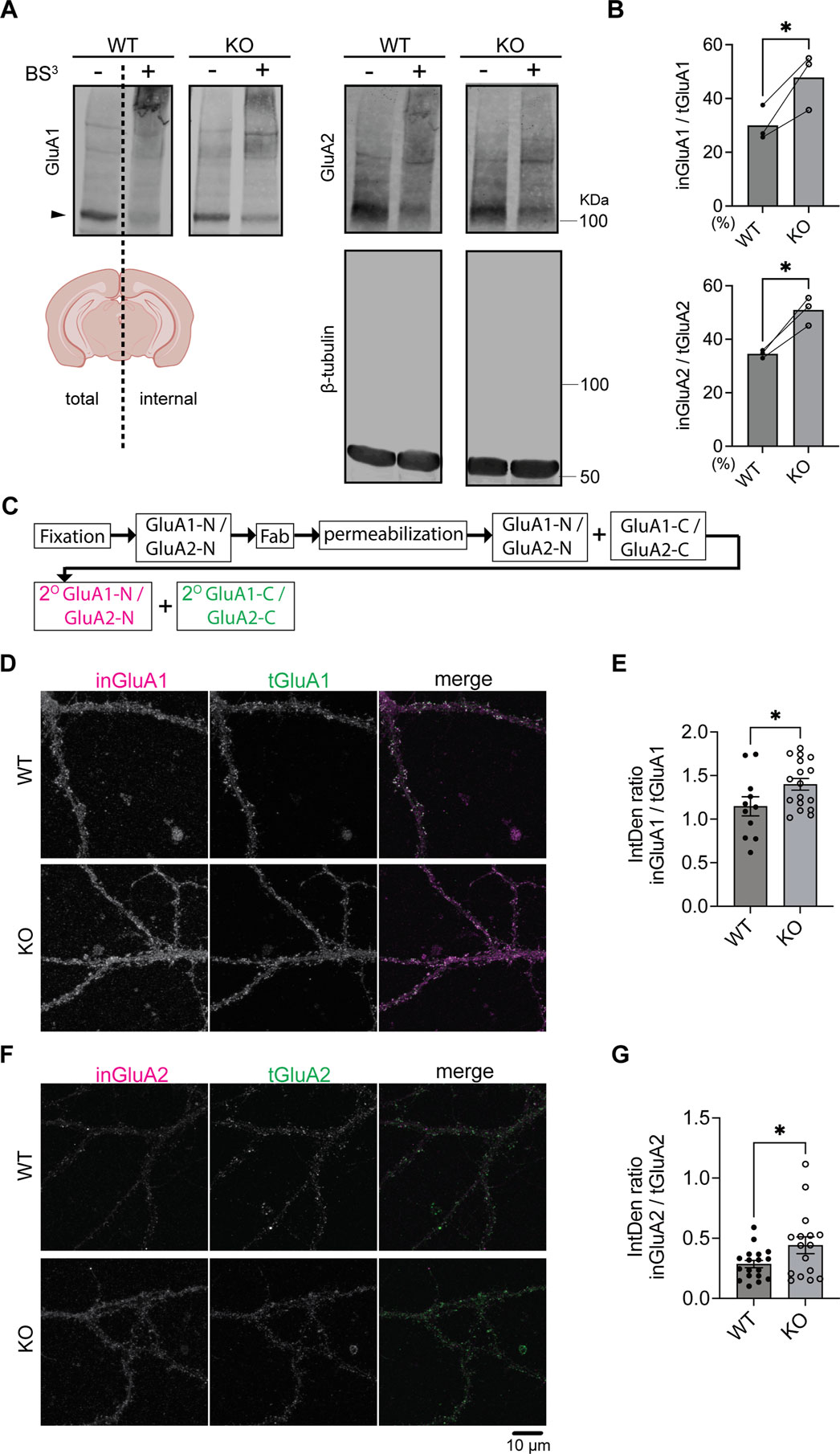
Figure 1. Increased intracellular GluA1 and GluA2 levels in SynDIG4 KO neurons (A) Representative immunoblots showing the separation of surface and intracellular GluA1 and GluA2 in brain slices from P14 WT and SynDIG4 KO mice after BS3 treatment. Arrowheads indicate the protein bands subjected to quantification. (B) Quantification of the ratio of intracellular to total GluA1 and GluA2 signals in WT and SynDIG4 KO brain slices. Data are presented as mean with statistical significance determined using ratio paired t-test; n = 3 biological replicates (each from a different litter with paired WT and KO littermates); *p < 0.05. (C) Schematic diagram illustrating the experimental procedure for intracellular labeling of AMPARs. (D–G) Representative confocal images of WT and SynDIG4 KO neurons showing internal and total GluA1 (D) and GluA2 (F) signals. Graphs depict the integrated density (IntDen) ratio of internal to total GluA1 (E) and internal to total GluA2 (G) signals in WT and SynDIG4 KO neurons. Data are presented as mean ± SEM, with statistical significance determined using unpaired t-test; n = 15-20 images, each image from a different neuron; *p < 0.05. Three biological replicates (independent cultures, each from a different litter with paired WT and KO littermates) were performed for each group, and representative results shown are from one of these replicates. Scale bar, 10 µm.
To determine if the effect is specific for AMPAR subunits, we re-probed the blots for the GluN1 subunit of the NMDA receptor (NMDAR) and did not observe any difference between WT and SynDIG4 KO slices (Supplementary Figures S1A, B). Although the intracellular levels of GluN1 remained comparable between WT and SynDIG4 KO slices, no detectable aggregation of surface GluN1 was observed using an antibody against an extracellular region between the third and fourth membrane domains, likely due to the epitope being destroyed by the cross-linking procedure. The total signal intensities of GluA1, GluA2, and GluN1 were comparable between WT and SynDIG4 KO in brain slices (Supplementary Figure S1C), indicating that there is no change in the overall level of the subunits consistent with previous results from whole brain (Matt et al., 2018) and hippocampal lysates (Troyano-Rodriguez et al., 2019).
To determine whether levels of inGluA1 and inGluA2 between acute brain slices and cultured hippocampal neurons from SynDIG4 KO and WT mice are comparable, fragment antigen-binding (Fab) antibodies were applied to fixed neurons to block surface signals by preventing secondary antibodies from accessing primary antibodies bound to the extracellular N-terminal (NT) regions of GluA1 (GluA1-N) and GluA2 (GluA2-N) prior to permeabilization (Supplementary Figure S1D). As a control, sGluA1 and sGluA2 signals cannot be detected after adding Fab (Supplementary Figure S1E). After permeabilization, the same primary antibodies against GluA1-N and GluA2-N followed by fluorophore-conjugated secondary antibodies were added to detect inGluA1 and inGluA2 (Figure 1C). By comparing the ratio of intracellular signals detected by the antibody against the NT to total signals detected by the antibody against the C-terminal (CT) regions of GluA1 (GluA1-C) and GluA2 (GluA2-C), we found that inGluA1 and inGluA2 levels were increased in cultured hippocampal neurons (Figures 1D–G), consistent with BS3 cross-linking results from brain slices (Figures 1A–C) and surface biotinylation results from brain lysates (Troyano-Rodriguez et al., 2019).
SynDIG4 regulates the recycling of GluA1-containing AMPARs
Previous findings showed no significant changes in total AMPAR levels in SynDIG4 KO mice (Matt et al., 2018; Troyano-Rodriguez et al., 2019). Combined with the current results, these findings indicate that SynDIG4 likely regulates the trafficking and distribution of AMPARs rather than their expression. To pinpoint the trafficking mechanism impaired in SynDIG4 KO neurons, we employed an antibody-feeding approach to examine the endocytosis and recycling of GluA1-containing AMPARs (Zheng et al., 2015; Chiu et al., 2017; Ghane et al., 2019). To label endocytosed GluA1 (enGluA1), live hippocampal neurons were incubated with an antibody targeting the extracellular NT region of GluA1 (GluA1-N), followed by incubation at 37°C to allow internalization of labeled receptors. Fab antibodies were subsequently applied to block surface GluA1-N epitopes, preventing further labeling. After permeabilization, antibodies against the CT region of GluA1 (GluA1-C) were applied, followed by secondary antibody incubation (Figure 2A). During the GluA1-N antibody-feeding process, some receptors were internalized (Supplementary Figure S2A). After 30 min of internalization, the levels of enGluA1 were comparable between WT and SynDIG4 KO neurons (Figures 2B,C).
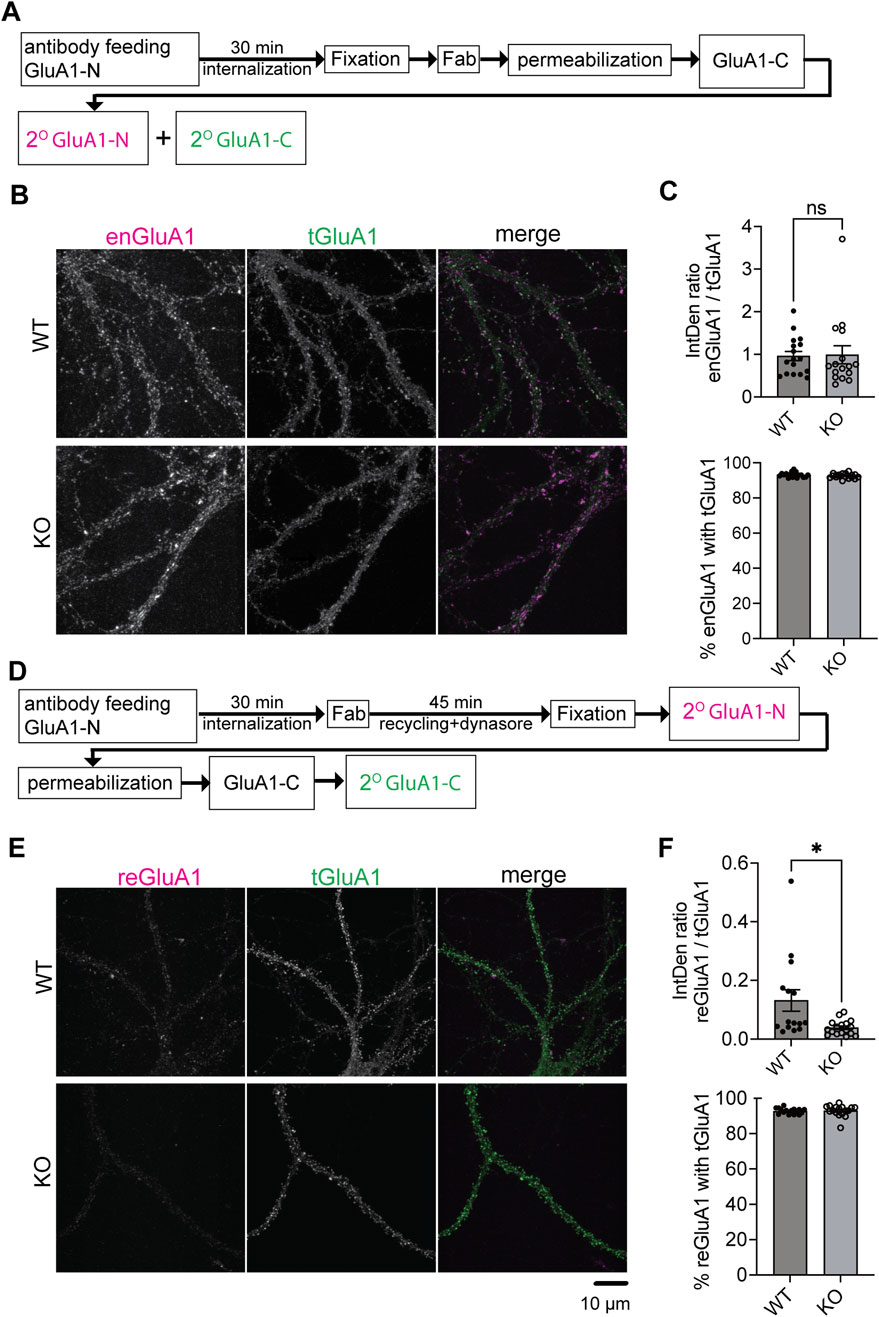
Figure 2. Impaired recycling of GluA1-containing AMPARs in SynDIG4 KO neurons (A) Schematic diagram illustrating the experimental procedure for AMPAR endocytosis. After antibody feeding, neurons were incubated for 30 min, fixed, treated with Fab, permeabilized, and subjected to standard labeling. (B) Representative confocal images of WT and SynDIG4 KO neurons showing endocytosed and total GluA1 signals. (C) The ratio of the integrated density of endocytosed GluA1 (enGluA1) to total GluA1 (tGluA1) signals and the percentage of enGluA1 colocalized with tGluA1 were analyzed in WT and SynDIG4 KO neurons. (D) Schematic diagram illustrating the experimental procedure for AMPAR recycling. (E) Representative confocal images of WT and SynDIG4 KO neurons showing recycled and total GluA1 signals. (F) Graphs depict the ratio of integrated density (IntDen) for recycled GluA1 (reGluA1) to tGluA1 signals and the percentage of reGluA1 colocalized with tGluA1 in WT and SynDIG4 KO neurons. Data are presented as mean ± SEM, with statistical significance determined using unpaired t-test; n = 15-20 images, each image from a different neuron; *p < 0.05, ns, not significant. Three biological replicates (independent cultures, each from a different litter with paired WT and KO littermates) were performed for each group, and representative results shown are from one of these replicates. Scale bar, 10 µm.
To investigate recycling of GluA1-containing AMPARs, hippocampal neurons were treated with dynasore, a dynamin-dependent endocytosis inhibitor (Macia et al., 2006), following GluA1-N antibody feeding and internalization (Figure 2D). This treatment prevented further endocytosis, allowing recycled GluA1 (reGluA1) to reinsert into the plasma membrane. Dynasore treatment decreased enGluA1 levels in WT neurons as expected (Supplementary Figures S2C, D). Interestingly, reGluA1 levels were reduced in SynDIG4 KO neurons (Figures 2E,F), indicating that SynDIG4 is important for the recycling but not for the endocytosis process of GluA1-containing AMPARs at the plasma membrane.
As an internal control to validate the staining specificity, we examined the colocalization of both enGluA1 and reGluA1 with total GluA1 (tGluA1). The percentages of enGluA1 and reGluA1 colocalizing with tGluA1 were greater than 90% in both WT and SynDIG4 KO neurons (Figures 2C,F). These findings confirm that, despite the presence of some background noise, the staining procedure exhibits high specificity and adequate efficiency.
Retention of AMPARs in Rab4-positive endosomes in SynDIG4 deficient neurons
The above results suggest that AMPAR endosomal recycling is impaired in SynDIG4 KO neurons, though the specific intracellular compartments that SynDIG4 is involved in remains unclear. To identify the impaired compartments of AMPAR trafficking in SynDIG4 KO neurons, GluA1 and GluA2 subcellular distribution was investigated with different endosomal markers (Grant and Donaldson, 2009) in WT and SynDIG4 KO neurons. The results showed that the distribution of GluA1 and GluA2 in EEA1-positive endosomes were similar between WT and SynDIG4 KO neurons (Figures 3A, D; Supplementary Figures S3A, D), suggesting that trafficking to EEA1-positive early endosomes is unaffected, consistent with the results from the antibody-feeding assay (Figures 2B,C).
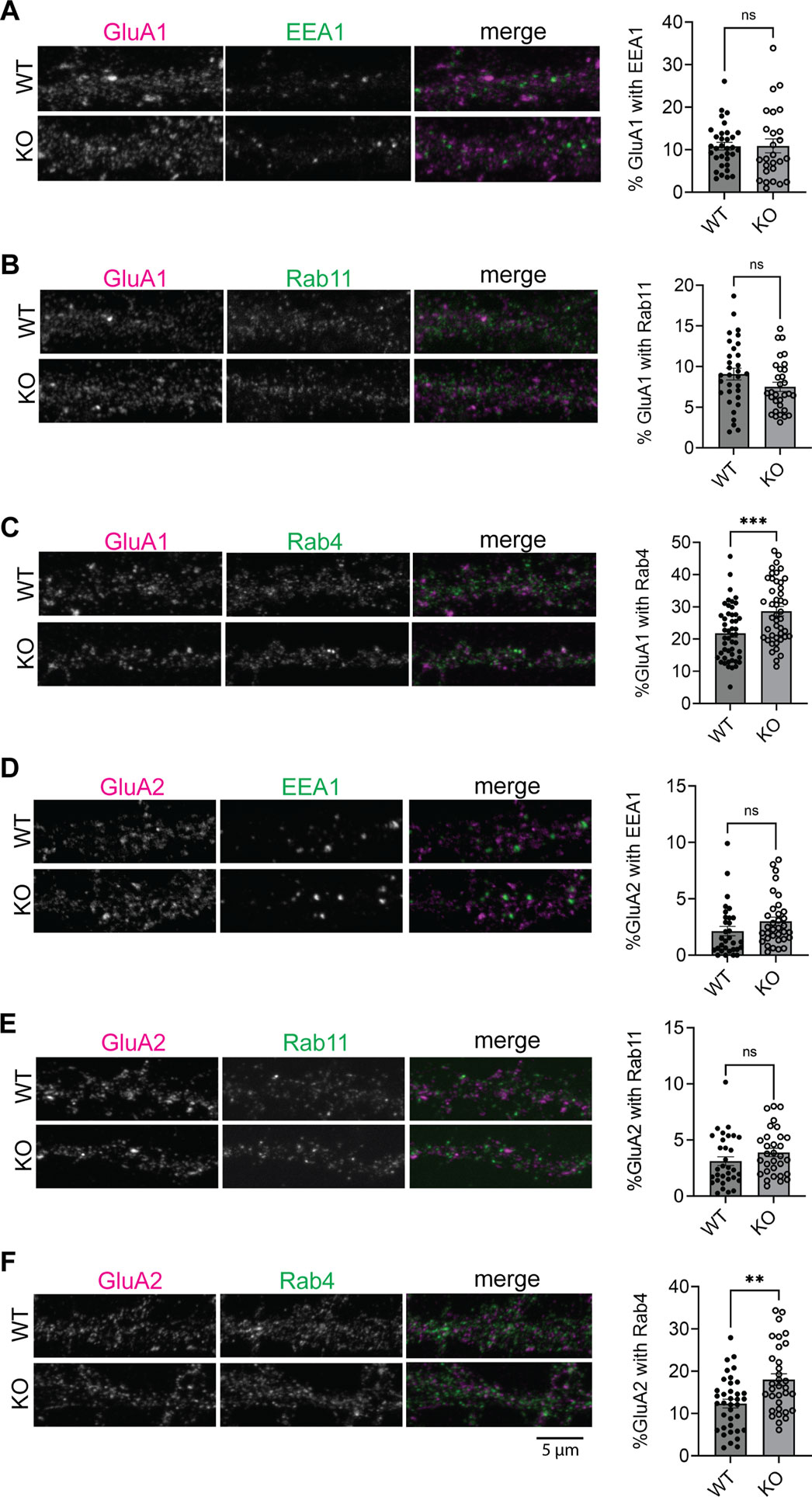
Figure 3. Accumulation of AMPARs in Rab4-positive endosomes in SynDIG4 KO neurons (A) Representative images showing the colocalization of EEA1 and GluA1 and quantification of Manders’ colocalization coefficient for EEA1 and GluA1 in WT and SynDIG4 KO neurons. (B) Representative images showing the colocalization of Rab11 and GluA1 and quantification of Manders’ colocalization coefficient for Rab11 and GluA1 in WT and SynDIG4 KO neurons. (C) Representative images showing the colocalization of Rab4 and GluA1 and quantification of Manders’ colocalization coefficient for Rab4 and GluA1 in WT and SynDIG4 KO neurons. (D) Representative images showing the colocalization of EEA1 and GluA2 and quantification of Manders’ colocalization coefficient for EEA1 and GluA2 in WT and SynDIG4 KO neurons. (E) Representative images showing the colocalization of Rab11 and GluA2 and quantification of Manders’ colocalization coefficient for Rab11 and GluA2 in WT and SynDIG4 KO neurons. (F) Representative images showing the colocalization of Rab4 and GluA2 and quantification of Manders’ colocalization coefficient for Rab4 and GluA2 in WT and SynDIG4 KO neurons. Data are presented as mean ± SEM, with statistical significance determined using unpaired t-test; n = ∼40 dendrite stretches, 2–3 dendritic stretches were cropped from individual neuron images; **p < 0.01, ***p < 0.001, ns, not significant. Three biological replicates (independent cultures, each from a different litter with paired WT and KO littermates) were performed for each group, and representative results shown are from one of these replicates. Scale bar, 5 µm.
AMPARs can be recycled to the plasma membrane through different endosomal trafficking pathways (Grant and Donaldson, 2009). To investigate which specific recycling process that SynDIG4 is involved in, we stained Rab4-positive and Rab11-positive compartments, markers for fast and slow recycling endosomes, respectively (Grant and Donaldson, 2009), along with GluA1 or GluA2 in WT and SynDIG4 KO neurons. The results revealed that the levels of GluA1 and GluA2 in Rab11-positive endosomes were comparable between WT and SynDIG4 KO neurons (Figures 3B, E; Supplementary Figures S3B, E). In contrast, both GluA1 and GluA2 were significantly elevated in Rab4-positive endosomes in SynDIG4 KO neurons (Figures 3C, F; Supplementary Figures S3C, F).
To further evaluate the subcellular localization of AMPARs, Van Steensel’s analysis was performed to assess the Pearson correlation between GluA1 or GluA2 and endosomal markers. The peak Pearson correlation occurred at a 0-pixel shift of the original images, and the correlation values progressively decreased with increasing pixel shifts, indicating spatial colocalization (Supplementary Figures S3A–F). In contrast, the Pearson correlation between GluA1 or GluA2 and randomized endosome marker images remained close to zero, suggesting that the observed correlations are not due to random overlap. Notably, the correlation coefficients for GluA1/Rab4 and GluA2/Rab4 were significantly higher in SynDIG4 KO neurons compared to WT neurons (Supplementary Figures S3C, F). These findings suggest that GluA1 and GluA2 are retained in Rab4-positive endosomes in the absence of SynDIG4, thereby impairing their recycling to the plasma membrane.
Loss of SynDIG4 disrupts endosomal trafficking between Rab4-positive and Rab11-positive endosomes
Previous work proposed a model that impaired fusion between Rab4-positive and Rab11-positive endosomes leads to increased overlap between Rab4 and Rab11 compartments, thereby disrupting AMPAR trafficking (Hoogenraad et al., 2010). This disruption leads to a decrease in the surface expression of AMPARs and an increased colocalization of AMPARs with syntaxin13, a recycling endosome marker (Chiu et al., 2017). To determine where SynDIG4 functions in this process, we co-stained for Rab4 and Rab11 with SynDIG4 in WT neurons. We found that the levels of SynDIG4 are comparable between Rab4-positive and Rab11-positive endosomes (Figures 4A, D; Supplementary Figure S4A), indicating that some SynDIG4 (∼25%) localizes to both compartments. Further analysis showed that 59.74% of Rab4-Rab11 colocalization sites contained SynDIG4; however, this represented only 12.63% of all SynDIG4 signals that overlapped with Rab4-Rab11 colocalization sites (data not shown). These findings suggest that SynDIG4 is not specifically enriched in Rab4-positive or Rab11-positive endosomes; rather it is equally distributed across the two compartments and likely plays functional roles at or between Rab4-positive and Rab11-positive endosomes.
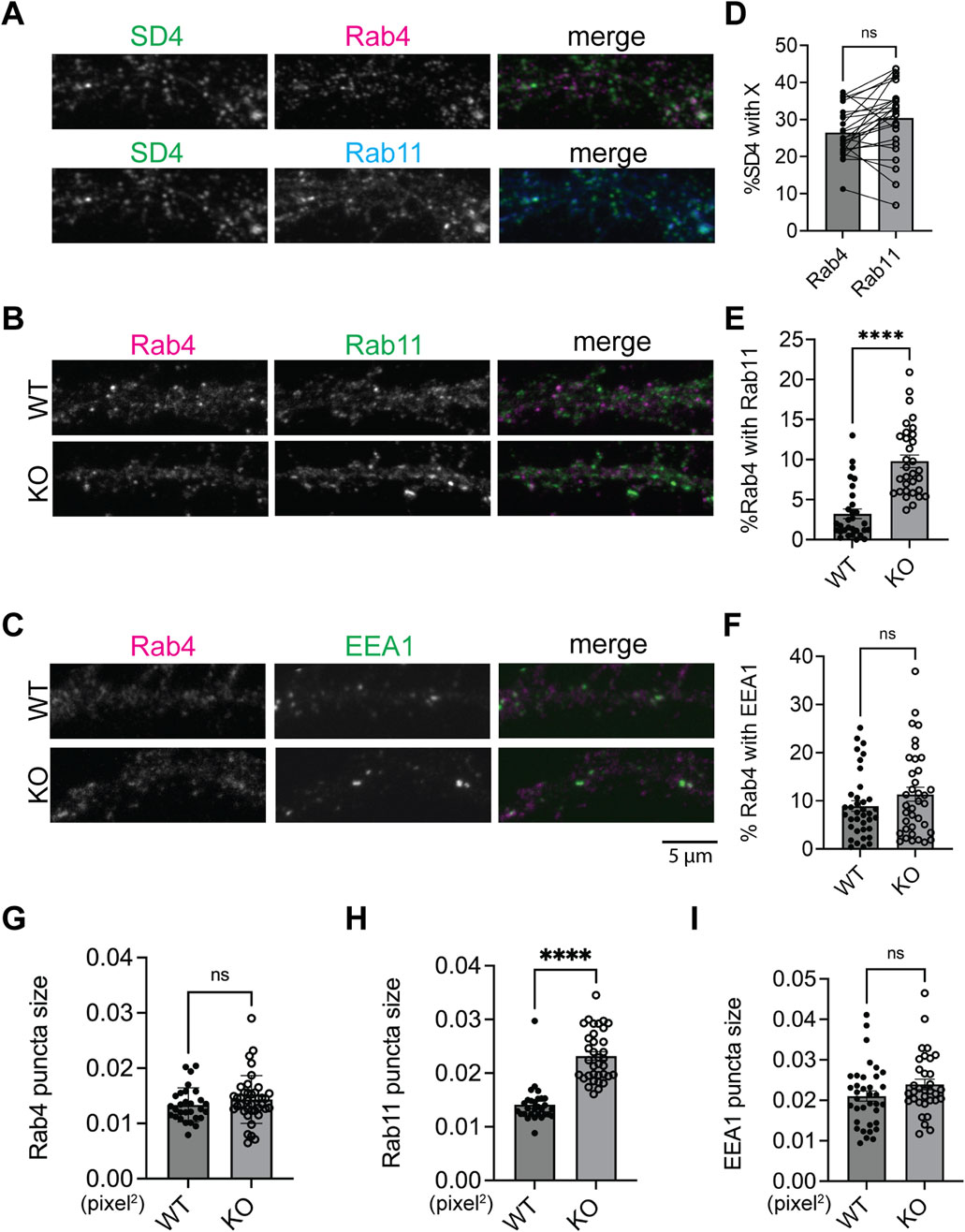
Figure 4. Increased colocalization of Rab4-positive and Rab11-positive compartments in SynDIG4 KO neurons (A) Representative images showing the colocalization of SynDIG4 and Rab4 or Rab11. (B) Representative images showing the colocalization of Rab4 and Rab11. (C) Representative images showing the colocalization of Rab4 and EEA1. (D) Quantification of Manders’ colocalization coefficient for SynDIG4 and Rab4 or Rab11 in WT neurons. (E) Quantification of Manders’ colocalization coefficient for Rab4 and Rab11 in WT and SynDIG4 KO neurons. (F) Quantification of Manders’ colocalization coefficient for Rab4 and EEA1 in WT and SynDIG4 KO neurons. (G–I) Quantification of puncta size of Rab4, Rab11 and EEA1 in WT and SynDIG4 KO neurons, respectively. Data are presented as mean ± SEM, with statistical significance determined using unpaired t-test; n = ∼40 dendrite stretches, 2–3 dendritic stretches were cropped from individual neuron images; ****p < 0.0001, ns, not significant. Three biological replicates (independent cultures, each from a different litter with paired WT and KO littermates) were performed for each group, and representative results shown are from one of these replicates. Scale bar, 5 µm.
To test whether the trafficking between Rab4-positive and Rab11-positive endosomes is affected in SynDIG4 KO neurons, Rab4 and Rab11 were co-stained in WT and SynDIG4 KO neurons. Interestingly, the overlap between Rab4 signals and Rab11 signals was increased in SynDIG4 KO neurons, suggesting that endosomal trafficking between Rab4-positive and Rab11-positive endosomes is disrupted in SynDIG4 KO neurons (Figures 4B, E; Supplementary Figure S4B). At the same time, the overlap between EEA1 and Rab4 remained unchanged between WT and SynDIG4 KO neurons (Figures 4C, F; Supplementary Figure S4C). Additionally, we observed that Rab11 puncta size was significantly increased in SynDIG4 KO neurons, whereas the sizes of Rab4 and EEA1 puncta remained unchanged (Figures 4G–I). Meanwhile, the total expression levels of Rab4, Rab11 and EEA1 were comparable between WT and SynDIG4 KO neurons (Supplementary Figures S4D–I). These results suggest that the increased overlap between Rab4 and Rab11 signals in SynDIG4 KO neurons could be due to the enlargement of the Rab11 compartment due to impaired trafficking between Rab4-positive and Rab11-positive endosomes and strengthen the idea that SynDIG4 functions in endosome recycling between Rab4-positive and Rab11-positive compartments to regulate the distribution of AMPARs.
Decreased surface expression of GluA1-containing AMPARs at synaptic regions in SynDIG4 deficient neurons
We have demonstrated impaired AMPAR recycling in SynDIG4 KO neurons. Previous work showed decreased surface expression of GluA1 and GluA2 (Troyano-Rodriguez et al., 2019) and reduced density of extrasynaptic GluA1 and GluA2 puncta in SynDIG4 KO neurons (Matt et al., 2018). These findings suggest that SynDIG4 plays a critical role in both the surface expression and synaptic localization of AMPARs. To investigate SynDIG4 effects on the synaptic localization of surface AMPARs at the same time, sGluA1 was visualized using Stimulated Emission Depletion (STED) super-resolution microscopy to enhance resolution of molecular distributions within synapses by applying antibodies against GluA1-N to neurons prior to permeabilization (Figure 5A). As a control, antibodies against MAP2 added before permeabilization were undetectable, confirming that this surface-labeling protocol exclusively detects extracellular epitopes located on the plasma membrane (Supplementary Figure S5). The percentage of sGluA1 puncta overlapped with PSD95 puncta was decreased in SynDIG4 KO neurons (Figure 5B). To further investigate whether the association of sGluA1 with PSD95 was affected in SynDIG4 KO neurons, cross-correlation confidence analysis was performed. These results showed that the overlap of sGluA1 with PSD95 was reduced in SynDIG4 KO neurons (Figure 5C). Together, these results suggest that SynDIG4 contributes to the synaptic localization of surface GluA1-containing AMPARs.
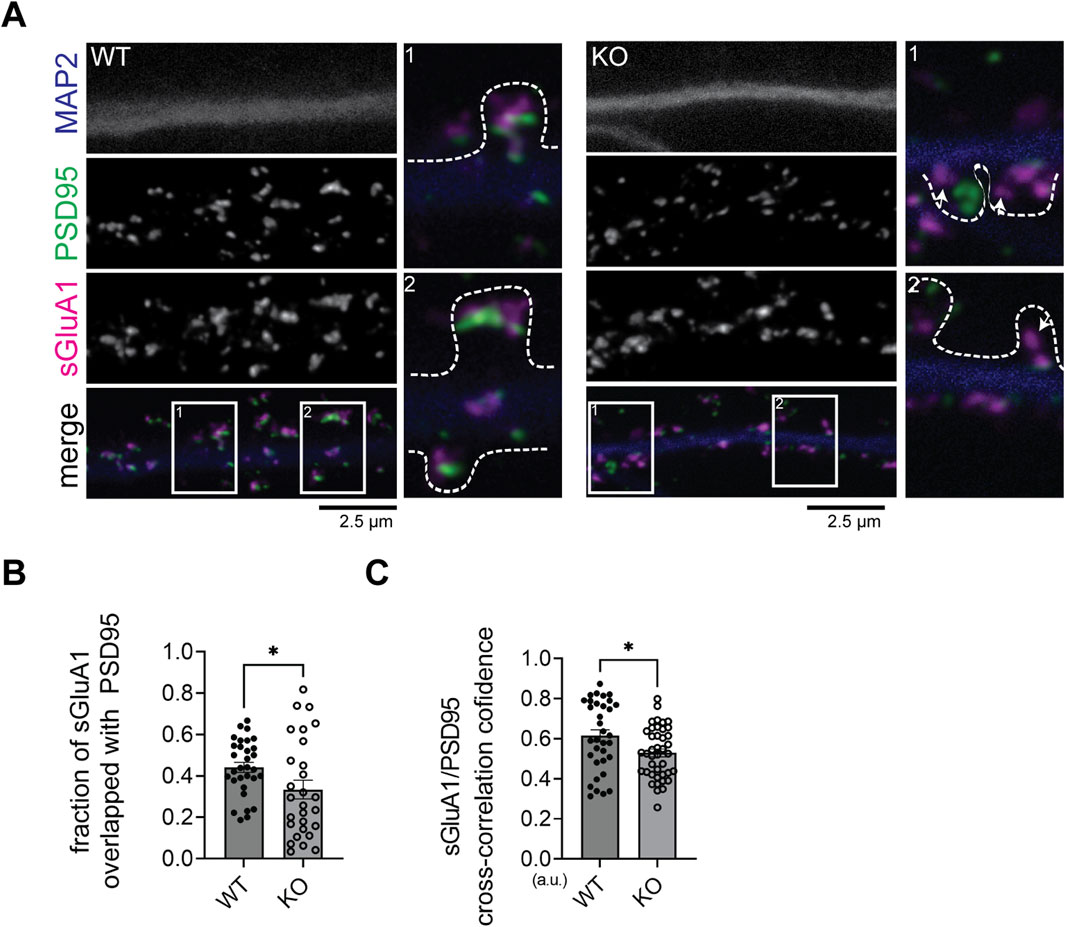
Figure 5. Association between sGluA1 and PSD95 is decreased in SynDIG4 KO neurons (A) Representative STED images showing that sGluA1 is less associated or overlapped with PSD95 in SynDIG4 KO neurons (indicated by arrowhead). The outline of the spine is identified by the signals from the cell tracer DiA. (B, C) Quantitative analysis of sGluA1 and PSD95 colocalization using puncta overlap (B) and cross-correlation coefficient methods (C). Data are presented as mean ± SEM, with statistical significance determined using unpaired t-test; n = ∼40 dendrite stretches, 2–3 dendritic stretches were cropped from individual neuron images; *p < 0.05. Three biological replicates (independent cultures, each from a different litter with paired WT and KO littermates) were performed for each group, and representative results shown are from one of these replicates. Scale bar, 2.5 µm.
Discussion
Overall, our findings suggest that SynDIG4 promotes the surface expression of GluA1-containing AMPARs via the Rab4-dependent recycling pathway and regulates the synaptic localization of sGluA1. We found that the levels of endocytosed GluA1-containing AMPARs and their localization in early endosomes were comparable between WT and SynDIG4 KO neurons, indicating that SynDIG4 does not play a role in the endocytosis of GluA1-containing AMPARs under basal conditions. In contrast, recycling of GluA1-containing AMPARs was impaired in SynDIG4 KO neurons likely due to trafficking defects between Rab4-positive and Rab11-positive recycling endosomes, thereby reducing the levels of sGluA1 at synaptic sites (Figure 6).
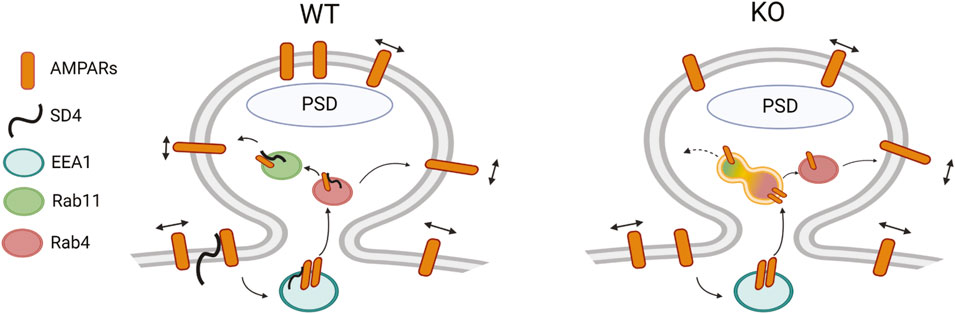
Figure 6. Model for the role of SynDIG4 in AMPAR distribution via endosomal recycling mechanism. In WT neurons, SynDIG4 (SD4) facilitates the trafficking of AMPARs between Rab4-positive and Rab11-positive endosomes under baseline conditions. In SynDIG4 KO neurons, the recycling of GluA1-containing AMPARs is reduced, and Rab11-positive endosomes fail to undergo fission from Rab4-positive endosomes. Consequently, the levels of intracellular AMPARs are increased, impairing the Rab4-Rab11-dependent recycling process. This SD4-mediated mechanism is crucial for the synaptic distribution of surface AMPARs. Created in BioRender. He, C. (2025) https://BioRender.com/k52o982.
SynDIG4 promotes endosomal trafficking of AMPARs
Previous studies have shown that some SynDIG4 colocalizes with the early endosomal marker EEA1 and with the transferrin receptor which is recycled between endosomes and the plasma membrane (Martin et al., 2021), suggesting that SynDIG4 is involved in recycling of AMPARs via the endosomal pathway. The endosomal pathway represents a collage of distinct yet overlapping compartments that are regulated by Rab proteins. Here we discovered that some SynDIG4 also overlaps with both the rapid and slow recycling endosomal markers Rab4 and Rab11, respectively (Zerial and McBride, 2001). Rab11 is involved in the continuous recycling of endocytosed GluA1-containing AMPARs to the postsynaptic membrane via an EEA1-Rab4-Rab11 endosomal pathway (Esteves da Silva et al., 2015; Lisé et al., 2006; Wang et al., 2008; Park et al., 2004); however, AMPARs can also return to the surface directly from Rab4-positive compartments as was shown for GluA2 (Gu et al., 2016). Previous work proposed a model that impaired fusion between Rab4-positive and Rab11-positive endosomes leads to increased overlap between Rab4 and Rab11 compartments, thereby disrupting AMPAR trafficking (Hoogenraad et al., 2010). We demonstrate that upon loss of SynDIG4, the overlap between Rab4 and Rab11 increases significantly, which leads to a significantly increased accumulation of GluA1 and GluA2 in Rab4-positive recycling endosomes but not in Rab11-positive recycling endosomes, suggesting that SynDIG4 is involved specifically in the continuous and rapid recycling mediated by Rab4-dependent transport.
An interesting possibility is that SynDIG4 may be involved in mediating trafficking between Rab4-positive and Rab11-positive endosomes and point to a potential role for SynDIG4 in promoting the fission of Rab11-positive endosomes from Rab4-positive compartments. In this study, the enlargement of Rab11-positive endosomes was observed in SynDIG4 KO neurons, supporting the idea that SynDIG4 is critical for this fission process, with the enlargement likely resulting from a failure in the separation between Rab4-positive and Rab11-positive endosomes. In addition, SynDIG4 belongs to a larger superfamily named “Dispanins” based on the prediction of two hydrophobic helical segments across the plasma membrane; however, later studies identified them as single pass type II transmembrane proteins with intracellular NT and extracellular CT, including SynDIG4 (Kirk et al., 2016). Members of the Dispanin superfamily are thought to function as “fusogens”, facilitating or inhibiting membrane fusion (Coomer et al., 2021). For example, interferon-induced transmembrane protein 3 (IFITM3), another member of the Dispanin superfamily, restricts viral entry in endosomes, by inhibiting fusion pore formation (Klein et al., 2023). Similarly, PRRT2 (Proline-Rich Transmembrane protein 2), to which SynDIG4 is related, has been shown to regulate the fusion of synaptic vesicles with the presynaptic plasma membrane, thereby controlling neurotransmitter release (Coleman et al., 2018). Together, these findings strengthen the hypothesis that SynDIG4 contributes to trafficking between Rab4-positive and Rab11-positive endosomes by regulating the vesicle fusion and/or fission processes. Furthermore, considering that other membrane receptors are also recycled to the plasma membrane via the Rab4–Rab11 pathway, the possibility that SynDIG4 plays a broader role in the trafficking of additional receptor types cannot be excluded.
The retention of GluA1 and GluA2 in Rab4-positive endosomes, combined with possible defects in Rab4-Rab11 fission observed in SynDIG4 KO neurons, suggests that impaired endosomal trafficking between Rab4 and Rab11 disrupts the recycling of AMPARs. Interestingly, recycling of AMPARs through Rab4-Rab11-associated recycling endosomes and directly from Rab4-positive endosomes are both important for the supply for synaptic AMPARs (Gu et al., 2016; Hausser and Schlett, 2019). Our results support a model that SynDIG4 plays a pivotal role in AMPAR trafficking within the Rab4-Rab11 recycling pathway (Figure 6). However, we cannot exclude the possibility that SynDIG4 also contributes to AMPAR recycling via direct transport from Rab4-positive endosomes or through other potential mechanisms. For example, the increased GluA1 in Rab4-positive endosomes in SynDIG4 KO neurons could be due to a shift in trafficking pathways. That is, in response to the block in Rab4-Rab11-plasma membrane trafficking pathway, AMPAR transport moves to utilize the direct Rab4-plasma membrane pathway.
It is also possible that SynDIG4 regulates the trafficking of GluA1-containing AMPARs differently than GluA2-containing AMPARs, which may complicate interpretations of our results. Although SynDIG4 associates with both GluA1-and GluA2-containing AMPARs (Shanks et al., 2012; Schwenk et al., 2012; Chen et al., 2014) and loss of SynDIG4 leads to decreased surface expression of GluA1 and GluA2 in brain lysates (Troyano-Rodriguez et al., 2019) and reduced density of extrasynaptic GluA1 and GluA2 in cultured neurons (Matt et al., 2018), there are some aspects of SynDIG4 function that are selective for GluA1. In Xenopus oocytes, SynDIG4 was shown to modulate AMPAR gating properties in a subunit-dependent manner (Matt et al., 2018). Similar to other AMPAR auxiliary factors (Jackson and Nicoll, 2011), SynDIG4 slows deactivation kinetics of both GluA1 homomers and GluA1/GluA2 heteromers. In contrast, SynDIG4 reduces desensitization only of GluA1 homomers and has no significant effect on desensitization of heteromeric GluA1/GluA2 (Matt et al., 2018). In our recently published study, we demonstrated that SynDIG4 mutants lacking an endocytic signal co-localize with GluA1 but less so with GluA2 on the surface of heterologous cells (Speca et al., 2025). In native cryo-EM structures, SynDIG4 association with AMPARs appears to be through the M4 helix of GluA1 (Yu et al., 2021). Intriguingly, both NMDAR-dependent LTD (Troyano-Rodriguez et al., 2019) and single tetanus-induced LTP (Matt et al., 2018), which require calcium-permeable AMPARs (Sanderson et al., 2016), which are mostly GluA1 homomers, are impaired in SynDIG4 deficient neurons while baseline synaptic transmission is intact. Thus, it is possible that SynDIG4 is required for trafficking of both GluA1/GluA2 heteromers as well as GluA1 homomers at baseline through independent endosomal pathways. Additional experiments beyond the scope of this study are necessary to investigate this interesting possibility.
SynDIG4 regulates the distribution of surface AMPARs at synapses
Using STED microscopy, we found that the overlap between sGluA1 and PSD95 were decreased in SynDIG4 KO neurons. These findings suggest a reduction in sGluA1-containing AMPARs at or near PSD95, consistent with the observed decrease in miniature excitatory postsynaptic current (mEPSC) amplitude in SynDIG4 KO mice (Matt et al., 2018). Interestingly, under confocal microscopy, the density of GluA1 puncta was increased in synaptic regions identified by overlap with the presynaptic marker vGLUT1 (Matt et al., 2018). These findings indicate that while the total GluA1 level at synapses is increased, its surface expression was reduced, implying that intracellular GluA1 may be retained within the synaptic region. However, this interpretation should be considered with caution, as different imaging methods (STED vs. confocal) and distinct synaptic markers (PSD95 vs. vGLUT1) were used, which could lead to potential misinterpretations.
Role of SynDIG4 in endocytosis of AMPARs
Interestingly, our prior study investigating the distribution of SynDIG4 and AMPARs in heterologous cells proposed that endocytosis is likely required for the clustering of live-labeled GluA1 and GluA2, but not GluK2, with SynDIG4 (Plambeck et al., 2022). This raises the possibility that SynDIG4 may be involved in the endocytosis-dependent clustering of GluA1/GluA2 on the plasma membrane, despite not affecting the overall amount of AMPARs being internalized. However, even though the total amount of endocytosed GluA1 was comparable between WT and SynDIG4 KO neurons, SynDIG4 KO neurons had less surface GluA1 available initially. This suggests that, relative to the available surface pool, GluA1 endocytosis may occur more frequently in SynDIG4 KO neurons. Alternatively, SynDIG4 and AMPARs may undergo endocytosis independently and associate within endosomes to promote bi-directional clustering. Importantly, the clustering experiments were conducted at 37°C, a temperature that facilitates both endocytosis and exocytosis, leaving open the possibility that exocytosis also contributes to GluA1/GluA2 clustering in heterologous cells. Our findings here demonstrate that SynDIG4 is dispensable for the internalization of GluA1-containing AMPARs at baseline. However, they do not exclude the possibility that SynDIG4 influences AMPAR trafficking through mechanisms involving endocytosis such as during synaptic plasticity.
In summary, our findings demonstrate that SynDIG4 plays a critical role in the endosomal trafficking of GluA1-containing AMPARs via Rab4-positive recycling pathways and is essential for the proper distribution of surface AMPARs at synapses. SynDIG4 KO neurons display deficits in LTD (Troyano-Rodriguez et al., 2019) and single-tetanus LTP (Matt et al., 2018), both of which require GluA1. SynDIG4 KO mice also display deficits in hippocampal-dependent learning and memory (Matt et al., 2018), and downregulation of SynDIG4 has been found in patients with Alzheimer’s disease (Li et al., 2021). These findings highlight the importance of investigating SynDIG4 function in synaptic plasticity, its contributions to learning and memory, and its potential role in the pathology of Alzheimer’s disease. These areas are the focus of our current research.
Data availability statement
The raw data supporting the conclusions of this article will be made available by the authors, without undue reservation.
Ethics statement
The animal study was approved by the Institutional Animal Care and Use Committee of the University of California, Davis. The study was conducted in accordance with the local legislation and institutional requirements.
Author contributions
C-WH: Formal Analysis, Investigation, Writing – original draft, Writing – review and editing. ED: Formal Analysis, Funding acquisition, Methodology, Supervision, Writing – original draft, Writing – review and editing.
Funding
The author(s) declare that financial support was received for the research and/or publication of this article. This work was supported by NIH grant #R01MH119347 (ED).
Acknowledgments
We extend our sincere gratitude to Drs. Johannes Hell, John Gray, and Madeline Nieves-Cintrón (University of California, Davis) for their invaluable insights and feedback throughout the research process. We thank members of the Díaz Lab, particularly Dr. David Speca, for their constructive suggestions on the research and comments on the manuscript. We are especially grateful to Dr. Ingrid Brust-Mascher (Advanced Imaging Facility, University of California, Davis School of Veterinary Medicine) for her expert assistance with fluorescent microscopy. Additionally, we thank Dr. James Trimmer and members of Dr Hell’s lab, with special recognition to Zoila Estrada-Tobar, (University of California, Davis), for generously sharing antibodies and reagents used in this research.
Conflict of interest
The authors declare that the research was conducted in the absence of any commercial or financial relationships that could be construed as a potential conflict of interest.
The author(s) declared that they were an editorial board member of Frontiers, at the time of submission. This had no impact on the peer review process and the final decision.
Generative AI statement
The author(s) declare that no Generative AI was used in the creation of this manuscript.
Publisher’s note
All claims expressed in this article are solely those of the authors and do not necessarily represent those of their affiliated organizations, or those of the publisher, the editors and the reviewers. Any product that may be evaluated in this article, or claim that may be made by its manufacturer, is not guaranteed or endorsed by the publisher.
Supplementary material
The Supplementary Material for this article can be found online at: https://www.frontiersin.org/articles/10.3389/fphar.2025.1568908/full#supplementary-material
References
Boudreau, A. C., Milovanovic, M., Conrad, K. L., Nelson, C., Ferrario, C. R., and Wolf, M. E. (2012). A protein cross-linking assay for measuring cell surface expression of glutamate receptor subunits in the rodent brain after in vivo treatments. Curr. Protoc. Neurosci. Chapter 5, 1–19. doi:10.1002/0471142301.ns0530s59
Caya-Bissonnette, L., and Béïque, J. C. (2024). Half a century legacy of long-term potentiation. Curr. Biol. CB 34 (13), R640–R662. doi:10.1016/j.cub.2024.05.008
Chen, N., Pandya, N. J., Koopmans, F., Castelo-Székelv, V., van der Schors, R. C., Smit, A. B., et al. (2014). Interaction proteomics reveals brain region-specific AMPA receptor complexes. J. Proteome Res. 13 (12), 5695–5706. doi:10.1021/pr500697b
Chiu, S. L., Diering, G. H., Ye, B., Takamiya, K., Chen, C. M., Jiang, Y., et al. (2017). GRASP1 regulates synaptic plasticity and learning through endosomal recycling of AMPA receptors. Neuron 93 (6), 1405–1419. doi:10.1016/j.neuron.2017.02.031
Choquet, D. (2018). Linking nanoscale dynamics of AMPA receptor organization to plasticity of excitatory synapses and learning. J. Neurosci. Off. J. Soc. Neurosci. 38 (44), 9318–9329. doi:10.1523/JNEUROSCI.2119-18.2018
Chowdhury, S., Shepherd, J. D., Okuno, H., Lyford, G., Petralia, R. S., Plath, N., et al. (2006). Arc/Arg3.1 interacts with the endocytic machinery to regulate AMPA receptor trafficking. Neuron 52 (3), 445–459. doi:10.1016/j.neuron.2006.08.033
Coleman, J., Jouannot, O., Ramakrishnan, S. K., Zanetti, M. N., Wang, J., Salpietro, V., et al. (2018). PRRT2 regulates synaptic fusion by directly modulating SNARE complex assembly. Cell Rep. 22 (3), 820–831. doi:10.1016/j.celrep.2017.12.056
Coomer, C. A., Rahman, K., and Compton, A. A. (2021). CD225 proteins: a family portrait of fusion regulators. Trends Genet. TIG 37 (5), 406–410. doi:10.1016/j.tig.2021.01.004
Derkach, V. A., Oh, M. C., Guire, E. S., and Soderling, T. R. (2007). Regulatory mechanisms of AMPA receptors in synaptic plasticity. Nat. Rev. Neurosci. 8 (2), 101–113. doi:10.1038/nrn2055
Díaz, E. (2010). Regulation of AMPA receptors by transmembrane accessory proteins. Eur. J. Neurosci. 32 (2), 261–268. doi:10.1111/j.1460-9568.2010.07357.x
Ehlers, M. D. (2000). Reinsertion or degradation of AMPA receptors determined by activity-dependent endocytic sorting. Neuron 28 (2), 511–525. doi:10.1016/s0896-6273(00)00129-x
Esteves da Silva, M., Adrian, M., Schätzle, P., Lipka, J., Watanabe, T., Cho, S., et al. (2015). Positioning of AMPA receptor-containing endosomes regulates synapse architecture. Cell Rep. 13 (5), 933–943. doi:10.1016/j.celrep.2015.09.062
Ghane, M. A., Yakout, D. W., and Mabb, A. M. (2019). A high-content assay for monitoring AMPA receptor trafficking. J. Vis. Exp. JoVE 28, 143. doi:10.3791/59048
Granger, A. J., Shi, Y., Lu, W., Cerpas, M., and Nicoll, R. A. (2013). LTP requires a reserve pool of glutamate receptors independent of subunit type. Nature 493 (7433), 495–500. doi:10.1038/nature11775
Grant, B. D., and Donaldson, J. G. (2009). Pathways and mechanisms of endocytic recycling. Nat. Rev. Mol. Cell Biol. 10 (9), 597–608. doi:10.1038/nrm2755
Greger, I. H., Watson, J. F., and Cull-Candy, S. G. (2017). Structural and functional architecture of AMPA-type glutamate receptors and their auxiliary proteins. Neuron 94 (4), 713–730. doi:10.1016/j.neuron.2017.04.009
Groc, L., Heine, M., Cognet, L., Brickley, K., Stephenson, F. A., Lounis, B., et al. (2004). Differential activity-dependent regulation of the lateral mobilities of AMPA and NMDA receptors. Nat. Neurosci. 7 (7), 695–696. doi:10.1038/nn1270
Gu, Y., Chiu, S. L., Liu, B., Wu, P. H., Delannoy, M., Lin, D. T., et al. (2016). Differential vesicular sorting of AMPA and GABAA receptors. Proc. Natl. Acad. Sci. U. S. A. 113 (7), E922–E931. doi:10.1073/pnas.1525726113
Hastings, M. H., and Man, H. Y. (2018). Synaptic capture of laterally diffusing AMPA receptors - an idea that stuck. Trends Neurosci. 41 (6), 330–332. doi:10.1016/j.tins.2018.03.016
Hausser, A., and Schlett, K. (2019). Coordination of AMPA receptor trafficking by Rab GTPases. Small GTPases 10 (6), 419–432. doi:10.1080/21541248.2017.1337546
Hoogenraad, C. C., Popa, I., Futai, K., Martinez-Sanchez, E., Wulf, P. S., van Vlijmen, T., et al. (2010). Neuron specific Rab4 effector GRASP-1 coordinates membrane specialization and maturation of recycling endosomes. PLoS Biol. 8 (1), e1000283. doi:10.1371/journal.pbio.1000283
Huganir, R. L., and Nicoll, R. A. (2013). AMPARs and synaptic plasticity: the last 25 years. Neuron 80 (3), 704–717. doi:10.1016/j.neuron.2013.10.025
Jackson, A. C., and Nicoll, R. A. (2011). The expanding social network of ionotropic glutamate receptors: TARPs and other transmembrane auxiliary subunits. Neuron 70 (2), 178–199. doi:10.1016/j.neuron.2011.04.007
Jacobi, E., and von Engelhardt, J. (2017). Diversity in AMPA receptor complexes in the brain. Curr. Opin. Neurobiol. 45, 32–38. doi:10.1016/j.conb.2017.03.001
Kennedy, M. B. (2013). Synaptic signaling in learning and memory. Cold Spring Harb. Perspect. Biol. 8 (2), a016824. doi:10.1101/cshperspect.a016824
Kirk, L. M., Ti, S. W., Bishop, H. I., Orozco-Llamas, M., Pham, M., Trimmer, J. S., et al. (2016). Distribution of the SynDIG4/proline-rich transmembrane protein 1 in rat brain. J. Comp. Neurol. 524 (11), 2266–2280. doi:10.1002/cne.23945
Klein, S., Golani, G., Lolicato, F., Lahr, C., Beyer, D., Herrmann, A., et al. (2023). IFITM3 blocks influenza virus entry by sorting lipids and stabilizing hemifusion. Cell Host Microbe 31 (4), 616–633.e20. doi:10.1016/j.chom.2023.03.005
Li, M., Geng, R., Li, C., Meng, F., Zhao, H., Liu, J., et al. (2021). Dysregulated gene-associated biomarkers for Alzheimer’s disease and aging. Transl. Neurosci. 12 (1), 83–95. doi:10.1515/tnsci-2021-0009
Lisé, M. F., Wong, T. P., Trinh, A., Hines, R. M., Liu, L., Kang, R., et al. (2006). Involvement of myosin Vb in glutamate receptor trafficking. J. Biol. Chem. 281 (6), 3669–3678. doi:10.1074/jbc.M511725200
Lu, J., Helton, T. D., Blanpied, T. A., Rácz, B., Newpher, T. M., Weinberg, R. J., et al. (2007). Postsynaptic positioning of endocytic zones and AMPA receptor cycling by physical coupling of dynamin-3 to Homer. Neuron 55 (6), 874–889. doi:10.1016/j.neuron.2007.06.041
Lüscher, C., and Malenka, R. C. (2012). NMDA receptor-dependent long-term potentiation and long-term depression (LTP/LTD). Cold Spring Harb. Perspect. Biol. 4 (6), a005710. doi:10.1101/cshperspect.a005710
Macia, E., Ehrlich, M., Massol, R., Boucrot, E., Brunner, C., and Kirchhausen, T. (2006). Dynasore, a cell-permeable inhibitor of dynamin. Dev. Cell 10 (6), 839–850. doi:10.1016/j.devcel.2006.04.002
Man, H. Y., Ju, W., Ahmadian, G., and Wang, Y. T. (2000). Intracellular trafficking of AMPA receptors in synaptic plasticity. Cell Mol. Life Sci. CMLS 57 (11), 1526–1534. doi:10.1007/pl00000637
Martin, E. E., Wleklinski, E., Hoang, H. T. M., and Ahmad, M. (2021). Interaction and subcellular association of PRRT1/SynDIG4 with AMPA receptors. Front. Synaptic Neurosci. 13, 705664. doi:10.3389/fnsyn.2021.705664
Matt, L., Kirk, L. M., Chenaux, G., Speca, D. J., Puhger, K. R., Pride, M. C., et al. (2018). SynDIG4/Prrt1 is required for excitatory synapse development and plasticity underlying cognitive function. Cell Rep. 22 (9), 2246–2253. doi:10.1016/j.celrep.2018.02.026
Park, M., Penick, E. C., Edwards, J. G., Kauer, J. A., and Ehlers, M. D. (2004). Recycling endosomes supply AMPA receptors for LTP. Science. 305 (5692), 1972–1975. doi:10.1126/science.1102026
Petrini, E. M., Lu, J., Cognet, L., Lounis, B., Ehlers, M. D., and Choquet, D. (2009). Endocytic trafficking and recycling maintain a pool of mobile surface AMPA receptors required for synaptic potentiation. Neuron 63 (1), 92–105. doi:10.1016/j.neuron.2009.05.025
Plambeck, K. E., He, C. W., Navarro, H. H., and Díaz, E. (2022). Mutually dependent clustering of SynDIG4/PRRT1 and AMPA receptor subunits GluA1 and GluA2 in heterologous cells and primary neurons. Front. Mol. Neurosci. 15, 788620. doi:10.3389/fnmol.2022.788620
Qneibi, M., Bdir, S., Bdair, M., Aldwaik, S. A., Heeh, M., Sandouka, D., et al. (2024). Exploring the role of AMPA receptor auxiliary proteins in synaptic functions and diseases. FEBS J. doi:10.1111/febs.17287
Sanderson, J. L., Gorski, J. A., and Dell’Acqua, M. L. (2016). NMDA receptor-dependent LTD requires transient synaptic incorporation of Ca2+-permeable AMPARs mediated by akap150-anchored PKA and calcineurin. Neuron. 89 (5), 1000–1015. doi:10.1016/j.neuron.2016.01.043
Schwenk, J., Harmel, N., Brechet, A., Zolles, G., Berkefeld, H., Müller, C. S., et al. (2012). High-resolution proteomics unravel architecture and molecular diversity of native AMPA receptor complexes. Neuron 74 (4), 621–633. doi:10.1016/j.neuron.2012.03.034
Shanks, N. F., Savas, J. N., Maruo, T., Cais, O., Hirao, A., Oe, S., et al. (2012). Differences in AMPA and kainate receptor interactomes facilitate identification of AMPA receptor auxiliary subunit GSG1L. Cell Rep. 1 (6), 590–598. doi:10.1016/j.celrep.2012.05.004
Speca, D. J., He, C. W., Meyer, C. M., Scott, E. C., and Díaz, E. (2025). Functional characterization of endocytic signals in the SynDIG/PRRT family members SynDIG1 and SynDIG4 in heterologous cells and neurons. Front. Cell Neurosci. 18, 1526034. doi:10.3389/fncel.2024.1526034
Troyano-Rodriguez, E., Mann, S., Ullah, R., and Ahmad, M. (2019). PRRT1 regulates basal and plasticity-induced AMPA receptor trafficking. Mol. Cell Neurosci. 98, 155–163. doi:10.1016/j.mcn.2019.06.008
Wang, Z., Edwards, J. G., Riley, N., Provance, D. W., Karcher, R., Li, X. D., et al. (2008). Myosin Vb mobilizes recycling endosomes and AMPA receptors for postsynaptic plasticity. Cell 135 (3), 535–548. doi:10.1016/j.cell.2008.09.057
Yu, J., Rao, P., Clark, S., Mitra, J., Ha, T., and Gouaux, E. (2021). Hippocampal AMPA receptor assemblies and mechanism of allosteric inhibition. Nature 594 (7863), 448–453. doi:10.1038/s41586-021-03540-0
Zerial, M., and McBride, H. (2001). Rab proteins as membrane organizers. Nat. Rev. Mol. Cell Biol. 2 (2), 107–117. doi:10.1038/35052055
Keywords: SynDIG4, PRRT1, AMPA receptor, endosomes, recycling, Rab4, Rab11
Citation: He C-W and Díaz E (2025) Loss of SynDIG4/PRRT1 alters distribution of AMPA receptors in Rab4- and Rab11-positive endosomes and impairs basal AMPA receptor recycling. Front. Pharmacol. 16:1568908. doi: 10.3389/fphar.2025.1568908
Received: 30 January 2025; Accepted: 29 April 2025;
Published: 21 May 2025.
Edited by:
Linda Marie Nowak, Cornell University, United StatesReviewed by:
Shiyi Wang, Duke University, United StatesJochen Schwenk, University of Freiburg, Germany
Copyright © 2025 He and Díaz. This is an open-access article distributed under the terms of the Creative Commons Attribution License (CC BY). The use, distribution or reproduction in other forums is permitted, provided the original author(s) and the copyright owner(s) are credited and that the original publication in this journal is cited, in accordance with accepted academic practice. No use, distribution or reproduction is permitted which does not comply with these terms.
*Correspondence: Elva Díaz, ZWRpYXpAdWNkYXZpcy5lZHU=