- 1Cancer Center, The First Hospital of Jilin University, Changchun, China
- 2Cancer Research Institute of Jilin University, The First Hospital of Jilin University, Changchun, China
- 3International Center of Future Science, Jilin University, Changchun, China
- 4Department of Pharmacy, China-Japan Union Hospital of Jilin University, Changchun, China
- 5Department of Clinical Pharmacy, The First Hospital of Jilin University, Changchun, China
Chimeric antigen receptor (CAR) T-cell therapy has emerged as a groundbreaking immunotherapeutic approach, particularly for oncohematological patients who are refractory to conventional treatments. As clinical trials expand the applications of CAR T-cell therapy beyond hematologic malignancies, a critical understanding of its associated toxicities, particularly cardiovascular complications, becomes imperative. This review synthesizes current literature on the interplay between cytokine release syndrome (CRS) and cardiotoxicity related to CAR T-cell therapy, emphasizing the potential severity of these adverse events. While significant progress has been made in managing CRS, the cardiac manifestations—ranging from mild events to life-threatening complications—remain underreported in pivotal studies. We explore the incidence and nature of cardiotoxicity in real-world and clinical trial settings, identify risk factors contributing to cardiovascular events, and propose guidelines for pre-therapy evaluations, post-infusion monitoring, and management strategies. By highlighting the urgent need for heightened awareness and proactive care, this review aims to enhance patient safety and optimize outcomes in the evolving landscape of CAR T-cell therapy.
Introduction
Cancer has become the leading cause of death globally, which has a profound impact on the health of the population, the national economy, and the progress of society around the world (Hafeez et al., 2020; Huang et al., 2024). Over the past three decades, remarkable progress has been achieved in cancer treatment, evolving from surgery, radiotherapy, and chemotherapy to targeted therapy, and more recently, immunotherapy (Baskar et al., 2012). Immunotherapy encompasses a range of strategies, such as immune checkpoint inhibitors (ICI), Chimeric antigen receptor (CAR) T-cell therapy, bispecific T cell engaging antibodies, cytokine-based treatments (e.g., high-dose interleukin-2 [IL-2] and interferon-α[IFN-α]), and monoclonal antibody therapies (June and Sadelain, 2018). Among these immunotherapies, CAR T-cell therapy represents an innovative immunotherapy approach that has achieved remarkable results in the treatment of hematological malignancies (Huang et al., 2024). Up to now, the CAR T cells approved by the United States Food and Drug Administration (FDA) are designed to target the CD19 antigen, which is highly expressed in B-cell malignancies (Crees and Ghobadi, 2021). However, data from clinical trials and observational studies indicate that this therapy may be associated with a range of toxicities, especially cardiac toxicities (Brudno and Kochenderfer, 2019). In this review, we summarized the adverse cardiac events observed in clinical trials of CAR T-cell therapy. We found that the underlying mechanisms for their occurrence seem to be related to the abnormal inflammatory activation observed in cytokine release syndrome (CRS) (Camilli et al., 2023a). Based on the key pathways involved in the currently understood mechanisms of CRS, we provide monitoring and management strategies. These strategies aim to enhance the safety of CAR T-cell therapy and expand its scope of application (Brudno and Kochenderfer, 2024).
Chimeric antigen receptor (CAR) T-cell therapy
T lymphocytes, also known as T cells, are a subtype of lymphocytes that play a vital role in the adaptive immune system by defending against disease-causing pathogens as well as protecting the body from abnormal cells (such as tumor cells), which is called immune surveillance (Schmid et al., 2006). Normal T cells trigger an immune response when they recognize antigens presented to them by antigen-presenting cells (APCs) that are coupled to major histocompatibility complex (MHC) molecules. Cancer cells can also express MHC molecules on their surface membranes (Darragh and Karam, 2022). In addition, conventional T cell therapy requires co-stimulation of other immune cells to trigger cytokine release, cytotoxic activity, and proliferation to be sufficient to induce an immune response (Gray et al., 2006).
CAR T-cell therapy, an innovative adoptive cell therapy that represents a new paradigm for cancer management, gained attention in 2012 after it was used to treat a pediatric patient with relapsed acute lymphoblastic leukemia (ALL) (Gill, 2023). It stimulates a direct anti-tumor response through the cell therapy mechanism of in vitro transgenic T cells, and the preparation process takes approximately 2–4 weeks (Nunes et al., 2024). CAR T cells are not existing products, but autologous T cells are isolated from peripheral blood of patients and collected by leukocyte apheresis (Padmanabhan, 2018). Lentivirus or γ retrovirus vectors are used for gene modification, allowing the required engineered CAR sequence to be inserted and integrated into the genome of autologous T cells, thereby leading to the expression of CAR on the surface of T cells, for example, CD19 (Freyer and Porter, 2020; Perry and Rayat, 2021). The transfected cells are then amplified in the laboratory using various methods that rapidly proliferate in vitro to produce therapeutic amounts, such as artificial APCs expressing CD80 and stimulating cytokines such as IL-15 (Yoshikai and Nishimura, 2000; Herrero-Beaumont et al., 2012). After expanding these cells in vitro, they are reintroduced to the patient, who receives lymphodepleting conditioning regime (LDC) with cyclophosphamide or fludarabine 3–5 days prior to administration, which inhibits the patient’s regulatory immune cell population to promote the activation and proliferation of the transfused CAR T cells in vivo (Shank et al., 2017). Thus, the therapeutic effect of CAR T cells was enhanced. Preoperative administration of acetaminophen and diphenhydramine should be given 30–60 min before CAR T cell infusion (Geiger and Howard, 2007). Shortly after infusion, the CAR T cells bind to the target antigen and are activated, causing them to rapidly multiply in the body and release inflammatory cytokines, which in turn recruit other immune cells to the tumor site, such as macrophages. These CAR T cells also exert cytotoxic effects by releasing cytotoxic particles containing granzyme and perforin, and directly stimulate tumor cell apoptosis by activating Fas/Fas-L and TNF-R pathways that lead to tumor cell destruction (Figure 1) (Hay and Slansky, 2022). Most patients receive CAR T cell transfusions and are monitored in the hospital for several days to weeks (Bachier et al., 2019).
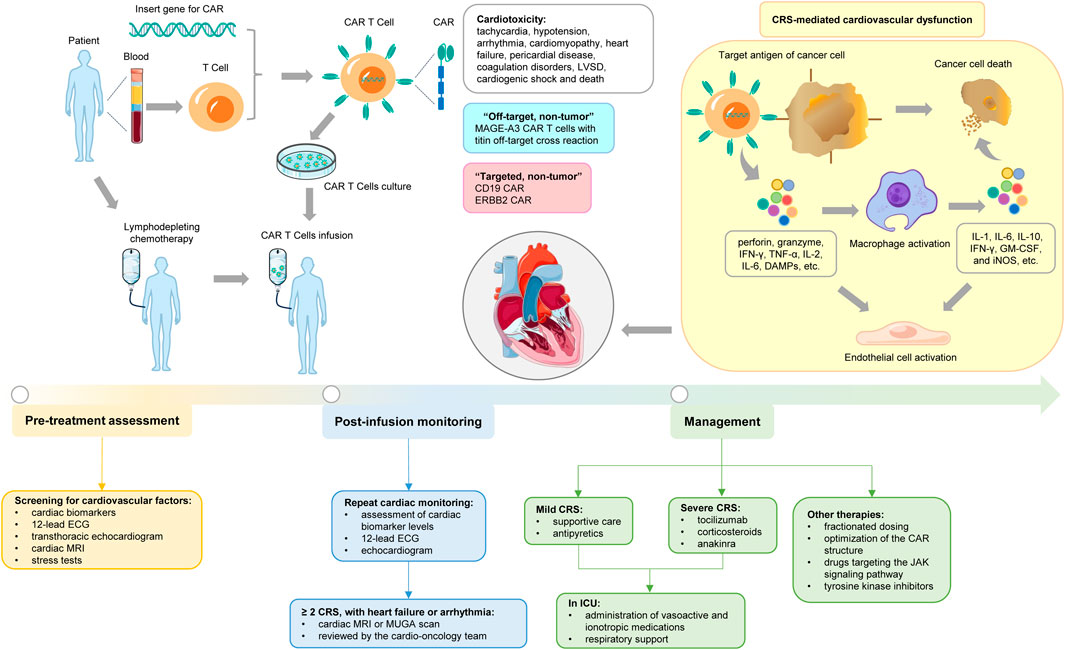
Figure 1. Cardiovascular toxicities associated with CAR T-cell therapy. The figure illustrates the general CAR T-cell therapy and the specific mechanisms of induced cardiotoxicity, as well as the associated assessment, monitoring, and management.
CAR is a synthetic transmembrane protein expressed on the surface of immune effector cells and consists of 4 domains: extracellular tumor antigen recognition domains, a hinge point connecting extracellular components to cytoplasmic elements, transmembrane domains, and intracellular domains derived from T cell receptor (TCR) that trigger signaling mechanisms leading to T cell activation (CD3ζ) or co-stimulatory domains (e.g., CD28, 4-1BB and OX-40) (Maher et al., 2002; Sadelain et al., 2013). Single-chain variable fragments of immunoglobulins are often used to recognize target tumor antigens due to their high binding specificity, subsequently leading to engineered T cell activation independent of the MHC (Dai et al., 2016; Mohanty et al., 2019). In addition to their immediate anti-tumor benefits, CAR T cells also provide long-term benefits as they promote immune surveillance to prevent tumor recurrence by continuously targeting malignant cells and assisting in the activation of tumor-infiltrating lymphocytes (June and Sadelain, 2018). It is important to note that the activity of CAR proteins can vary depending on the overall build design, and predicting the effectiveness of CAR T-cell therapy infusion is difficult because each CAR construct may have a different infusion effect (Letscher and Reddy, 2024). But the ideal target, as well as amplification to achieve sufficient numbers of CAR T, will promote targeting of rapidly proliferating malignancies (Jadlowsky et al., 2025).
In clinical trials, CAR T-cell therapy has shown significant efficacy in the treatment of a variety of highly relapsed/refractory hematological malignancies (Goyco Vera et al., 2024). In 2017, the FDA approved 2 CAR T-cell therapies: tisagenlecleucel and axicabtagene ciloleucel (U.S. Food and Drug Administration, 2017; U.S. Food and Drug Administration, 2017), which are CAR T cells targeting the B-cell receptor CD19, respectively for the treatment of pediatric and young adults with relapsed/refractory B-ALL and diffuse large B-cell lymphoma (DLBCL). Then, in July 2020, the FDA approved the CAR T-cell therapy brexucabtagene autoleucel for the treatment of adult relapsed/refractory mantle cell lymphoma (MCL) (U.S. Food and Drug Administration, 2020). Lisocabtagene maraleucel was approved in February 2021 for the treatment of adult relapsed/refractory large B-cell lymphoma (LBCL) (U.S. Food and Drug Administration, 2021). The first approved non-CD19 cell therapy was ldecabtagene vicleucel, created against B cell maturation antigen (BCMA), which has shown effectiveness in treating multiple myeloma (Ali et al., 2016; Brudno et al., 2018). This treatment fundamentally changes the paradigm of cancer treatment. Data from the World Health Organization’s International Agency for Research on Cancer (IARC) for 2023 show that five-year survival rates for myeloma and non-Hodgkin lymphoma improved from 32% and 56% in 1995–1997 to 58% and 74% in 2012–2018, marking significant clinical achievements (Siegel et al., 2023). As a result of these successes, CAR T cell immunotherapy is receiving increasing attention from scientists, clinicians, the pharmaceutical industry, and the general public, and several new clinical trials have been initiated to expand the clinical applicability of CAR T-cell therapy for cases such as chronic lymphocytic leukemia (CLL) and other solid malignancies, for example hepatocellular carcinoma, breast cancer, or pancreatic cancer (Mohanty et al., 2019). In addition, the success of hematological malignancies has stimulated research on CAR T-cell therapy for the treatment of autoimmune diseases, HIV and other infections, and transplant rejection (Maldini et al., 2018).
Cardiotoxicity associated with CAR T-cell therapy
While CAR T-cell therapy has shown strong clinical efficacy in relapsed/refractory hematological malignancies, with a response rate of up to 90%, it is limited by the incidence of severe life-threatening cardiotoxicity of up to 26%, as most patients considered for this treatment have cardiovascular comorbidities, and had previously received high doses of cardiotoxic chemotherapy and radiation (Neelapu, 2019). Manifestations may include tachycardia, hypotension, arrhythmia, cardiomyopathy, heart failure, pericardial disease, coagulation disorders, left ventricular systolic dysfunction (LVSD), cardiogenic shock and death, and may occur at various time points after CAR T-cell therapy has begun (Lyon et al., 2022). Up to now, several prospective and retrospective reports have highlighted the association between CAR T-cell therapy and adverse cardiovascular events in children and adults, significantly affecting morbidity and mortality (Burstein et al., 2018; Alvi et al., 2019; Ganatra et al., 2020; Lefebvre et al., 2020).
In pediatric and young adult patients
In 2018, Burstein et al. reported the first study on cardiotoxicity following CAR T-cell therapy, a retrospective analysis of 98 pediatric and young adult patients receiving tisagenlecleucel for relapsed/refractory ALL. 24 patients developed hypotension requiring vasopressor support, with a mean duration of onset of 4.6 days, and all had grade 3–4 CRS. Of these, 21 patients had life-threatening symptoms requiring tozzizumab, and 10 patients had LVSD revealed by echocardiography. Among these, 7 patients had persistent LVSD at the time of discharge, but during follow-up, the disorder had gradually resolved in 6 patients without any cardiac events leading to death (Burstein et al., 2018).
In July 2020, Shalabi et al. reported a second retrospective study of the effects of CD19 CAR T-cell therapy on cardiac function in 52 pediatric and young adult patients with relapsed/refractory B-ALL or non-Hodgkin lymphoma. All patients were previously exposed to anthracycline chemotherapy. 37 patients developed CRS, 9 had hypotension requiring vasopressor support, and 7 received tozizumab or steroids. Of the patients who developed CRS, 6 had post-infusion echocardiography showing cardiac insufficiency, of which 4 had grade 3–4 CRS. Elevated troponin was observed in 4 of 13 patients with systolic dysfunction, and the left ventricular ejection fraction (LVEF) decreased from 64% to 20%. 4 of the 6 patients with cardiac insufficiency had resolved by day 28 after CAR T cell infusion, and the remaining 2 patients showed complete cardiac recovery at 3 months follow-up. Interestingly, these 2 patients had the highest exposure to anthracyclines prior to treatment (Sadelain et al., 2013; Shalabi et al., 2020).
Thus, clinical data in pediatric and young adults suggest that cardiotoxicity following CAR T-cell therapy, though severe in some cases, is generally reversible and does not have lasting effects.
In adult patients
In June 2020, Lefebvre et al. reported a retrospective study of the effects of CD19 CAR T-cell therapy on cardiac function in 145 adult patients with DLBCL (30%), ALL (25%), or CLL (45%). In the study, 60% of patients were exposed to anthracyclines. Patients received commercially available (axicabtagene ciloleucel and tisagenlecleucel) or non-commercially available CAR T cells. The researchers identified 41 cases of major cardiac adverse events (MACE) that developed 11 days after CAR T cell infusion, with grade 3–4 CRS having a higher risk. 22 patients had heart failure events, 12 episodes of atrial fibrillation, 2 other arrhythmia events, 2 episodes of acute coronary syndrome, and 2 cardiogenic deaths. In addition, MACE was associated with statins, aspirin, insulin, and patients with prior atrial fibrillation and elevated creatinine at baseline (Lefebvre et al., 2020). Therefore, these results suggest that patients with high-risk cardiovascular characteristics are more likely to develop MACE.
In October 2020, Ganatra et al. published a retrospective review of 187 adult patients treated with CD19 CAR T cells for non-Hodgkin lymphoma to detect the incidence of cardiomyopathy. 97 percent of the patients received axicabtagene ciloleucel. A total of 155 patients had CRS, of which more than 50% had grade ≥2 CRS. Of the 116 patients who underwent continuous echocardiography, 12 developed new or worsening cardiomyopathy, manifested by a mean decrease in LVEF from 58% to 37% at 12.5 days after CAR T cell infusion. In these 12 patients with cardiomyopathy, 6 LVEF recovered to normal, 3 partially recovered, and 3 cardiogenic deaths. Similar to previous studies, most patients with cardiomyopathy had ≥ grade 2 CRS. In addition, the researchers note that older patients with hyperlipidemia, coronary artery disease, and the use of renin-angiotensin inhibitors and β blockers are at increased risk for cardiomyopathy. Interestingly, there was no significant difference in exposure to anthracyclines or radiotherapy between patients with cardiomyopathy and those without (Ganatra et al., 2020).
Thus, clinical data in adults, compared to pediatrics and young adults, suggest that cardiotoxicity in cases of grade 2 or higher CRS is not always reversible, and that patients with increased cardiovascular risk factors are at greater risk of fatal cardiac events.
CRS-mediated cardiovascular dysfunction
Cardiotoxicity may be due to the direct or indirect effects of the infusion of CAR T cells, and the mechanism revolves around three pathways: “targeted, non-tumor” toxicity, “off-target, non-tumor” toxicity, and CRS-mediated cardiovascular dysfunction (Joseph et al., 2025). In “targeted, non-tumor” toxicity, T cells target normal tissue cells that express tumor-associated antigens, such as B-cell aplastic anemia observed with CD19 CAR, and pulmonary edema caused by ERBB2 CAR (Chen et al., 2022). In “off-target, non-tumor” toxicity, CAR T cells may also attack the heart by cross-reacting with myocardial antigens from normal tissue (Nunes et al., 2024). Linette et al. reported that 2 patients receiving CAR T-cell therapy for melanoma targeting melanoma-associated antigen 3 (MAGE-A3) developed fatal myocarditis and cardiogenic shock. This is because CAR T cells exhibit an off-target cross-reaction to titin, a sarcomere protein expressed in the myocardium, which contains an epitope similar to that of MAGE-A3. Notably, MAGE-A3 is widely expressed in melanoma (Linette et al., 2013).
The key driver of cardiotoxicity associated with CAR T-cell therapy is thought to be primarily CRS resulting from over-activation of the immune system (Asnani, 2018; Zhao et al., 2018). In retrospective analyses of patients receiving CAR T treatment, the severity of CRS is typically closely associated with the occurrence of cardiotoxicity (Lee et al., 2014; Lefebvre et al., 2020). Cardiac events are mainly observed in patients with CRS of grade ≥2 and are usually caused by elevated troponin levels (Ghosh et al., 2020). Potential disease burden, tumor load, the type of CAR T construct, the infused dose of CAR T cells, and the addition of fludarabine in the lymphodepletion regimen are key risk factors for the severity of CRS (Maude et al., 2014).
In 2018, the American Society for Transplantation and Cellular Therapy (ASTCT) released a consensus system for the clinical grading of the severity of CRS, which is mainly based on fever, hypoxia, and hypotension (Table 1). CRS is defined as any hyper-physiological reaction caused by the activation or involvement of endogenous or infused T cells and/or other immune effector cells after any immunotherapy (Lee et al., 2019). Clinically, the symptoms of CRS range from mild to severe and can rapidly progress from fever, weakness, headache, rash, diarrhea, and musculoskeletal pain to hypoxia, hypotension, tachycardia, left ventricular systolic dysfunction, arrhythmia, elevated troponin, coagulation disorders, pericardial disease, decompensated heart failure, cardiogenic shock, and CRS may develop into a life-threatening condition (Davila et al., 2014; Maude et al., 2014; Lee et al., 2015; Ali et al., 2016).
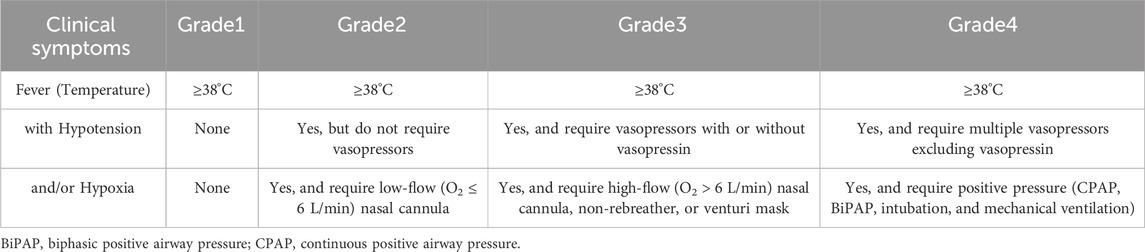
Table 1. Consensus cytokine release syndrome grading scale-the American society for transplantation and cellular therapy.
CRS typically begins within the first week after CAR T cell administration and can persist for up to 10–14 days, although symptoms may be delayed (Santomasso et al., 2019). Clinical data indicate that the incidence of CRS in patients receiving CAR T treatment ranges from 35% to 94%, with the incidence of severe CRS (≥ grade 3) ranging from 10% to 30% (Grigor et al., 2019). In fact, every patient receiving CAR T-cell therapy is expected to, and indeed hopes to, develop some degree of CRS, as grade 1 disease indicates a response to the treatment.
CRS is a systemic disease induced by the over-activation of immune effector cells and the hyper-physiological levels of various pro-inflammatory cytokines (Lee et al., 2019; Cobb and Lee, 2021). It is characterized by a pro-inflammatory cytokine storm following cell infusion and its interaction with the tumor microenvironment (Hughes et al., 2024). After recognizing tumor antigens, CAR T cells are activated and secrete large amounts of perforin, granzyme, and inflammatory cytokines such as IFN-γ, tumor necrosis factor-α (TNF-α), IL-2, IL-6, etc., to induce pyroptosis of tumor cells and release a large number of damage-associated molecular patterns (DAMPs) (Giavridis et al., 2018; Shimabukuro-Vornhagen et al., 2018). Meanwhile, they can also directly activate the other immune or non-immune cells, such as the monocyte/macrophage system, through inflammatory cytokines, perpetuating the vicious cycle of inflammation (Mosser and Edwards, 2008). In CRS, macrophages are considered the primary source of pro-inflammatory cytokines, such as IL-1, IL-6, IL-10, IFN-γ, granulocyte-macrophage colony-stimulating factor (GM-CSF), and inducible nitric oxide synthase (iNOS), among others (Hao et al., 2020). Additionally, macrophage pyroptosis and further DAMPs leakage amplify the inflammatory cascade, and secreted catecholamines can also promote the release of other cytokines, thus causing a distortion of the cytokine network (Johnson et al., 2005; Flierl et al., 2007; Shaked et al., 2015). These cytokines may lead to endothelial cell activation by regulating the angiopoietin-TIE2 axis and releasing NO, subsequently resulting in hemodynamic instability, capillary leakage, edema, organ hypoperfusion, cardiovascular dysfunction, and consumptive coagulopathy, and promoting cytokine production, thereby exacerbating CRS (Gust et al., 2017; Hay et al., 2017; Chou and Turtle, 2019). In cancer survivors treated with CAR T-cell therapy, persistent endothelial activation leads to endothelial damage, driving chronic inflammation, hypertension, and a prothrombotic state that accelerates the development of atherosclerosis (Choi et al., 2025).
Among the cytokines involved, IL-6 is the most crucial cytokine mediating CRS toxicity (Lee et al., 2014). Its level is positively correlated with the severity of CRS, and it is a major driver of the inflammatory activation cascade (Shimabukuro-Vornhagen et al., 2018; Chen et al., 2022). IL-6 mainly interacts with another membrane protein, gp130, either through “cis-signaling” of the membrane-bound IL-6 receptor (mIL-6R) or through “trans-signaling” of the soluble IL-6 receptor (sIL-6R), activating the janus kinase/signal transducer and activator of transcription 3 (JAK/STAT3) signaling pathway (Neelapu et al., 2018). This leads to abnormal myocardial electrical activity and a tendency towards arrhythmia, thus affecting normal cardiac electrical activity (Alí et al., 2018). It has been shown that IL-6 gene knockout significantly reduced cardiomyocyte apoptosis in a mouse model of dilated cardiomyopathy (Li Q. et al., 2019). In addition, in sepsis-induced myocardial dysfunction model, IL-6/STAT3 pathway interferes with endoplasmic reticulum (ER) and mitochondrial function by regulating the expression of proteins located in mitochondria-associated ER membranes, increasing intracellular calcium level and leading to myocardial apoptosis (Jiang et al., 2021). At the same time, IL-6/STAT3 pathway may also activate PERK pathway, leading to imbalance of intracellular calcium homeostasis and aggravation of myocardial dysfunction (Men et al., 2023). Blockade of the IL-6 signaling pathway results in the downregulation of pro-inflammatory cytokine secretion and the resolution of most clinical manifestations of CRS (Li Y. et al., 2019; Sterner et al., 2019). TNF-α can also induce the expression of adhesion molecules in vascular endothelial cells by activating the NF-κB signaling pathway, increase ROS production, and promote inflammatory cell infiltration, leading to endothelial injury (Zhou et al., 2017). In sepsis, IL-1β acts synergistically with TNF-α to aggravate myocardial injury by disrupting the blood-brain barrier and increasing mitochondrial membrane permeability, mitochondrial dysfunction and energy metabolism disorders, which aggravate inflammation and oxidative stress (Liu et al., 2025).
Pre-treatment assessment and post-infusion monitoring for cardiotoxicity
In real-world practice, factors such as advanced age, high tumor burden, and baseline heart conditions may increase the risk of cardiotoxicity due to reduced cardiac functional reserve or an additive effect of CAR T toxicity on pre-damaged myocardium, but these patients still routinely receive CAR T-cell therapy (Costanzo et al., 2024). For example, one study found that patients with underlying cardiac conditions had a higher rate of cardiovascular events after CAR T-cell therapy that correlated with the severity of CRS (Lefebvre et al., 2020). Therefore, pre-treatment assessment of cardiovascular health and risk is crucial for patients who require more intensive monitoring. The clinical practice guidelines issued by the American Society of Clinical Oncology (ASCO) outline evidence-based recommendations for the prevention and monitoring of cancer treatment-related cardiotoxicity (Slivnick et al., 2020). These recommendations commence with a detailed history of cardiovascular symptoms and physical examination, especially screening for cardiovascular risk factors prior to the initiation of treatment (Armenian et al., 2017). All patients scheduled to receive CAR T-cell therapy should undergo a comprehensive baseline cardiac screening using cardiac biomarkers (cardiac troponin and natriuretic peptides), 12-lead electrocardiogram (ECG), transthoracic echocardiogram, cardiac magnetic resonance imaging (MRI), stress tests, etc. (Dalal et al., 2022; Ganatra et al., 2022; Lyon et al., 2022; Camilli et al., 2023a). Collectively, these may provide additional key insights into the ability to tolerate hemodynamic changes during treatment.
After treatment infusion, it is recommended to repeat cardiac monitoring at 7 days and 3 months post-treatment, including assessment of cardiac biomarker levels, 12-lead ECG, and echocardiogram (Cho et al., 2024). Higher peak levels of inflammatory factors such as IL-6 and IL-1, C-reactive protein, ferritin, and troponin, together with CRS, may serve as prognostic markers of cardiotoxicity in patients receiving CAR T-cell therapy (Mahmood et al., 2023). Patients can be divided into low-risk, intermediate-risk, and high-risk groups based on their baseline N-terminal pro brain natriuretic peptide (NT-proBNP) and troponin levels. In high-risk patients, weekly echocardiography is recommended for 1 month and then every 3 months for 1 year. Patients at intermediate risk can be checked every 3 months; Low-risk patients can be tested every 6 months. At the same time, cardiac oncology clinics can consider equipping patients with wearable ECG monitors to detect arrhythmia in time and capture paroxysmal atrial fibrillation or premature ventricular contractions during CRS recovery, so as to take targeted interventions, but manual review is required simultaneously (Cardiovascular Expert Committee of Chinese Medical Doctor Association of Laboratory Medicine, 2024). Patients in whom CRS of grade ≥2 is detected, as well as those with new-onset or deteriorating heart failure or arrhythmia, should be hospitalized for cardiac MRI or multiple-gated acquisition (MUGA) scan, and the case should be reviewed by the cardio-oncology team (Avelar et al., 2017; Jeong et al., 2019). Subclinical fibrosis, as detected by cardiac MRI with measures such as late gadolinium enhancement and extracellular volume fraction, can be a useful tool for predicting late-onset arrhythmias. Studies have shown that the degree of myocardial fibrosis correlates with arrhythmic burden and that fibrosis predicts arrhythmic events even in patients with higher LVEF (Gulati et al., 2013; Bourke et al., 2024). Additionally, the ASCO guidelines recommend that for asymptomatic high-risk patients, echocardiography monitoring should be repeated 6–12 months after the completion of treatment (Armenian et al., 2017).
Therapeutic management measures for cardiotoxicity
Given the close association between CRS and CAR T cell related cardiotoxicity, mitigating the progression of CRS to more severe grades is of utmost importance in reducing the risk of cardiotoxicity (Alvi et al., 2019; Lefebvre et al., 2020). The grading of CRS is helpful in guiding treatment. Supportive care and antipyretics are commonly used to manage mild CRS, including inotropic support with dobutamine or milrinone when necessary (Faqihi et al., 2020; Ashkar et al., 2025). The IL-6 receptor antagonist tocilizumab was approved by the FDA for the treatment of CRS in 2017 and is currently considered as the first-line treatment for severe CRS secondary to CAR T-cell therapy (Le et al., 2018). Corticosteroids such as dexamethasone and methylprednisolone are regarded as the second line treatment for CRS refractory to anti-IL-6R therapy (Lakomy et al., 2023; Mulvey et al., 2025). Ongoing clinical trials of the IL-1 antagonist anakinra aim to demonstrate its role as an emergency treatment option (Le et al., 2018; Norelli et al., 2018; Camilli et al., 2023b). Typically, CRS requires treatment in an intensive care unit (ICU) for the administration of vasoactive and ionotropic medications, along with aggressive respiratory support (Bellal et al., 2024). In addition, strategies such as fractionated dosing, optimization of the CAR structure, drugs targeting the JAK signaling pathway (such as ruxolitinib and itacitinib), tyrosine kinase inhibitors (such as dasatinib and ibrutinib), and other rational therapies (such as the use of catecholamines or atrial natriuretic peptide, blocking TNF-α, etc.) can also be used for management (Figure 1) (Parampalli Yajnanarayana et al., 2015; Mestermann et al., 2019; Huarte et al., 2020; Huarte et al., 2021). When formulating management strategies, factors such as the pre-treatment tumor burden and tumor type should be taken into account. This is to alleviate symptoms without compromising the therapeutic benefits of this potentially curative approach (Cobb and Lee, 2021).
Conclusion and perspectives
In summary, with the expanded use of CAR T-cell therapy, the survival rate of cancer patients has increased. However, the treatment is limited by life-threatening CRS and associated cardiotoxicity. Understanding the mechanisms underlying cardiac injury and managing the cardiovascular toxicity of treatment approaches will become increasingly important, as this will enable monitoring, early intervention, and potential prevention of cardiotoxicity (Simela et al., 2025). Novel cardiac biomarkers and specific monitoring parameters not only help to detect the early signs of toxicity and its severity, but also contribute to enhancing clinical management and providing personalized medical care to patients (Christenson et al., 2015; Singh et al., 2015; Henri et al., 2016). Incorporating cardiovascular toxicity monitoring into clinical trials will play a crucial role in improving the safety of CAR T-cell therapy for cancer patients and fully realizing its therapeutic potential (Cho et al., 2024). However, the assessment of cardiotoxic outcomes may be biased by the selection of patients in early-phase clinical trials and selective reporting in retrospective studies. In recent years, various authorities are trying to establish the standardization of cardiotoxicity monitoring of CAR T-cell therapy, for example, the European Society of Cardiology (ESC), American Heart Association (AHA) and so on. Better preventive and treatment methods can be developed through prospective studies of patients and multidisciplinary integration among oncologists, oncohematologists, cardiologists, and other specialists, so as to promote wider application of this new therapy (Zhao et al., 2024).
Author contributions
J-HL: Writing – original draft, Writing – review and editing, Conceptualization, Data curation, Formal Analysis, Visualization. K-YL: Writing – review and editing. XaZ: Writing – review and editing. XnZ: Conceptualization, Writing – review and editing, Supervision, Funding acquisition. YJ: Conceptualization, Writing – review and editing, Supervision, Resources, Funding acquisition.
Funding
The author(s) declare that financial support was received for the research and/or publication of this article. YJ was funded by the Traditional Chinese Medicine Science and Technology Program of Jilin Province (2023065). XnZ was supported by grants from the National Natural Science Foundation of China (82002962 and 82372819), the Department of Science and Technology of Jilin Province (20210401076YY), and the Norman Bethune Program (2023B06).
Conflict of interest
The authors declare that the research was conducted in the absence of any commercial or financial relationships that could be construed as a potential conflict of interest.
Generative AI statement
The authors declare that no Generative AI was used in the creation of this manuscript.
Publisher’s note
All claims expressed in this article are solely those of the authors and do not necessarily represent those of their affiliated organizations, or those of the publisher, the editors and the reviewers. Any product that may be evaluated in this article, or claim that may be made by its manufacturer, is not guaranteed or endorsed by the publisher.
References
Alí, A., Boutjdir, M., and Aromolaran, A. S. (2018). Cardiolipotoxicity, inflammation, and arrhythmias: role for interleukin-6 molecular mechanisms. Front. Physiol. 9, 1866. doi:10.3389/fphys.2018.01866
Ali, S. A., Shi, V., Maric, I., Wang, M., Stroncek, D. F., Rose, J. J., et al. (2016). T cells expressing an anti-B-cell maturation antigen chimeric antigen receptor cause remissions of multiple myeloma. Blood 128 (13), 1688–1700. doi:10.1182/blood-2016-04-711903
Alvi, R. M., Frigault, M. J., Fradley, M. G., Jain, M. D., Mahmood, S. S., Awadalla, M., et al. (2019). Cardiovascular events among adults treated with chimeric antigen receptor T-cells (CAR-T). J. Am. Coll. Cardiol. 74 (25), 3099–3108. doi:10.1016/j.jacc.2019.10.038
Armenian, S. H., Lacchetti, C., Barac, A., Carver, J., Constine, L. S., Denduluri, N., et al. (2017). Prevention and monitoring of cardiac dysfunction in survivors of adult cancers: American society of clinical oncology clinical practice guideline. J. Clin. Oncol. 35 (8), 893–911. doi:10.1200/jco.2016.70.5400
Ashkar, H., Adnan, G., Patel, P., and Makaryus, A. N. (2025). Dobutamine, in StatPearls (Treasure Island (FL): StatPearls Publishing).
Asnani, A. (2018). Cardiotoxicity of immunotherapy: incidence, diagnosis, and management. Curr. Oncol. Rep. 20 (6), 44. doi:10.1007/s11912-018-0690-1
Avelar, E., Strickland, C. R., and Rosito, G. (2017). Role of imaging in cardio-oncology. Curr. Treat. Opt. Cardiovasc Med. 19 (6), 46. doi:10.1007/s11936-017-0546-2
Bachier, C. R., Palomba, M. L., Abramson, J. S., Andreadis, C., Sehgal, A. R., Godwin, J., et al. (2019). Outpatient treatment with lisocabtagene maraleucel (liso-cel) in three ongoing clinical studies in relapsed/refractory (R/R) B cell non-hodgkin lymphoma (NHL), including second-line transplant ineligible patients: transcend NHL 001, outreach, and PILOT. Blood 134 (Suppl. 1), 2868. doi:10.1182/blood-2019-127566
Baskar, R., Lee, K. A., Yeo, R., and Yeoh, K. W. (2012). Cancer and radiation therapy: current advances and future directions. Int. J. Med. Sci. 9 (3), 193–199. doi:10.7150/ijms.3635
Bellal, M., Malherbe, J., Damaj, G., and Du Cheyron, D. (2024). Toxicities, intensive care management, and outcome of chimeric antigen receptor T cells in adults: an update. Crit. Care 28 (1), 69. doi:10.1186/s13054-024-04851-0
Bourke, J., Tynan, M., Stevenson, H., Bremner, L., Gonzalez-Fernandez, O., and McDiarmid, A. K. (2024). Arrhythmias and cardiac MRI associations in patients with established cardiac dystrophinopathy. Open Heart 11 (1), e002590. doi:10.1136/openhrt-2023-002590
Brudno, J. N., and Kochenderfer, J. N. (2019). Recent advances in CAR T-cell toxicity: mechanisms, manifestations and management. Blood Rev. 34, 45–55. doi:10.1016/j.blre.2018.11.002
Brudno, J. N., and Kochenderfer, J. N. (2024). Current understanding and management of CAR T cell-associated toxicities. Nat. Rev. Clin. Oncol. 21 (7), 501–521. doi:10.1038/s41571-024-00903-0
Brudno, J. N., Maric, I., Hartman, S. D., Rose, J. J., Wang, M., Lam, N., et al. (2018). T cells genetically modified to express an anti-B-cell maturation antigen chimeric antigen receptor cause remissions of poor-prognosis relapsed multiple myeloma. J. Clin. Oncol. 36 (22), 2267–2280. doi:10.1200/jco.2018.77.8084
Burstein, D. S., Maude, S., Grupp, S., Griffis, H., Rossano, J., and Lin, K. (2018). Cardiac profile of chimeric antigen receptor T cell therapy in children: a single-institution experience. Biol. Blood Marrow Transpl. 24 (8), 1590–1595. doi:10.1016/j.bbmt.2018.05.014
Camilli, M., Maggio, L., Tinti, L., Lamendola, P., Lanza, G. A., Crea, F., et al. (2023a). Chimeric antigen receptor-T cell therapy-related cardiotoxicity in adults and children cancer patients: a clinical appraisal. Front. Cardiovasc Med. 10, 1090103. doi:10.3389/fcvm.2023.1090103
Camilli, M., Viscovo, M., Hohaus, S., Lamendola, P., Verrecchia, E., Gerardino, L., et al. (2023b). Incessant pericarditis successfully treated with anakinra in a patient on active treatment for mediastinal lymphoma. Can. J. Cardiol. 39 (8), 1154–1157. doi:10.1016/j.cjca.2022.10.027
Cardiovascular Expert Committee of Chinese Medical Doctor Association of Laboratory Medicine (2024). Chinese expert consensus on cardiac biomarkers for monitoring and management of cardiovascular toxicity in cancer therapy (2024 edition). Zhonghua Yi Xue Za Zhi 104 (36), 3371–3385. doi:10.3760/cma.j.cn112137-20240510-01091
Chen, L. R., Li, Y. J., Zhang, Z., Wang, P., Zhou, T., Qian, K., et al. (2022). Cardiovascular effects associated with chimeric antigen receptor T cell therapy in cancer patients: a meta-analysis. Front. Oncol. 12, 924208. doi:10.3389/fonc.2022.924208
Cho, I., You, S.-C., Cha, M.-J., Hwang, H.-J., Cho, E. J., Kim, H. J., et al. (2024). Cancer therapy-related cardiac dysfunction and the role of cardiovascular imaging: systemic review and opinion paper from the working group on cardio-oncology of the Korean society of cardiology. J. Cardiovasc. Imaging 32 (1), 13. doi:10.1186/s44348-024-00014-5
Choi, A., Kim, S., Kim, S., Cho, I., Cha, M. J., and You, S. C. (2025). Atherosclerotic cardiovascular disease in cancer survivors: current evidence, risk prediction, prevention, and management. J. Lipid Atheroscler. 14 (1), 30–39. doi:10.12997/jla.2025.14.1.30
Chou, C. K., and Turtle, C. J. (2019). Insight into mechanisms associated with cytokine release syndrome and neurotoxicity after CD19 CAR-T cell immunotherapy. Bone Marrow Transpl. 54 (Suppl. 2), 780–784. doi:10.1038/s41409-019-0602-5
Christenson, E. S., James, T., Agrawal, V., and Park, B. H. (2015). Use of biomarkers for the assessment of chemotherapy-induced cardiac toxicity. Clin. Biochem. 48 (4-5), 223–235. doi:10.1016/j.clinbiochem.2014.10.013
Cobb, D. A., and Lee, D. W. (2021). Cytokine release syndrome biology and management. Cancer J. 27 (2), 119–125. doi:10.1097/ppo.0000000000000515
Costanzo, V., Ratre, Y. K., Andretta, E., Acharya, R., Bhaskar, L., and Verma, H. K. (2024). A comprehensive review of cancer drug-induced cardiotoxicity in blood cancer patients: current perspectives and therapeutic strategies. Curr. Treat. Opt. Oncol. 25 (4), 465–495. doi:10.1007/s11864-023-01175-z
Crees, Z. D., and Ghobadi, A. (2021). Cellular therapy updates in B-cell lymphoma: the state of the CAR-T. Cancers 13 (20), 5181. doi:10.3390/cancers13205181
Dai, H., Wang, Y., Lu, X., and Han, W. (2016). Chimeric antigen receptors modified T-cells for cancer therapy. J. Natl. Cancer Inst. 108 (7), djv439. doi:10.1093/jnci/djv439
Dalal, P. J., Patel, N. P., Feinstein, M. J., and Akhter, N. (2022). Adverse cardiac effects of CAR T-cell therapy: characteristics, surveillance, management, and future research directions. Technol. Cancer Res. Treat. 21, 15330338221132927. doi:10.1177/15330338221132927
Darragh, L. B., and Karam, S. D. (2022). Amateur antigen-presenting cells in the tumor microenvironment. Mol. Carcinog. 61 (2), 153–164. doi:10.1002/mc.23354
Davila, M. L., Riviere, I., Wang, X., Bartido, S., Park, J., Curran, K., et al. (2014). Efficacy and toxicity management of 19-28z CAR T cell therapy in B cell acute lymphoblastic leukemia. Sci. Transl. Med. 6 (224), 224ra25. doi:10.1126/scitranslmed.3008226
Faqihi, F., Alharthy, A., Alshaya, R., Papanikolaou, J., Kutsogiannis, D. J., Brindley, P. G., et al. (2020). Reverse takotsubo cardiomyopathy in fulminant COVID-19 associated with cytokine release syndrome and resolution following therapeutic plasma exchange: a case-report. BMC Cardiovasc Disord. 20 (1), 389. doi:10.1186/s12872-020-01665-0
Flierl, M. A., Rittirsch, D., Nadeau, B. A., Chen, A. J., Sarma, J. V., Zetoune, F. S., et al. (2007). Phagocyte-derived catecholamines enhance acute inflammatory injury. Nature 449 (7163), 721–725. doi:10.1038/nature06185
Freyer, C. W., and Porter, D. L. (2020). Advances in CAR T therapy for hematologic malignancies. Pharmacotherapy 40 (8), 741–755. doi:10.1002/phar.2414
Ganatra, S., Dani, S. S., Yang, E. H., Zaha, V. G., and Nohria, A. (2022). Cardiotoxicity of T-cell antineoplastic therapies: JACC: CardioOncology primer. JACC CardioOncol 4 (5), 616–623. doi:10.1016/j.jaccao.2022.07.014
Ganatra, S., Redd, R., Hayek, S. S., Parikh, R., Azam, T., Yanik, G. A., et al. (2020). Chimeric antigen receptor T-cell therapy-associated cardiomyopathy in patients with refractory or relapsed non-hodgkin lymphoma. Circulation 142 (17), 1687–1690. doi:10.1161/circulationaha.120.048100
Geiger, T. L., and Howard, S. C. (2007). Acetaminophen and diphenhydramine premedication for allergic and febrile nonhemolytic transfusion reactions: good prophylaxis or bad practice? Transfus. Med. Rev. 21 (1), 1–12. doi:10.1016/j.tmrv.2006.09.001
Ghosh, A. K., Chen, D. H., Guha, A., Mackenzie, S., Walker, J. M., and Roddie, C. (2020). CAR T cell therapy–related cardiovascular outcomes and management: systemic disease or direct cardiotoxicity? JACC CardioOncology 2 (1), 97–109. doi:10.1016/j.jaccao.2020.02.011
Giavridis, T., van der Stegen, S. J. C., Eyquem, J., Hamieh, M., Piersigilli, A., and Sadelain, M. (2018). CAR T cell-induced cytokine release syndrome is mediated by macrophages and abated by IL-1 blockade. Nat. Med. 24 (6), 731–738. doi:10.1038/s41591-018-0041-7
Gill, J. (2023). Cardiovascular toxicities with chimeric antigen receptor T-cell therapy. Curr. Cardiol. Rev. 19 (1), e230622206353. doi:10.2174/1573403x18666220623152350
Goyco Vera, D., Waghela, H., Nuh, M., Pan, J., and Lulla, P. (2024). Approved CAR-T therapies have reproducible efficacy and safety in clinical practice. Hum. Vaccin Immunother. 20 (1), 2378543. doi:10.1080/21645515.2024.2378543
Gray, J. C., Johnson, P. W. M., and Glennie, M. J. (2006). Therapeutic potential of immunostimulatory monoclonal antibodies. Clin. Sci. 111 (2), 93–106. doi:10.1042/cs20060024
Grigor, E. J. M., Fergusson, D., Kekre, N., Montroy, J., Atkins, H., Seftel, M. D., et al. (2019). Risks and benefits of chimeric antigen receptor T-cell (CAR-T) therapy in cancer: a systematic review and meta-analysis. Transfus. Med. Rev. 33 (2), 98–110. doi:10.1016/j.tmrv.2019.01.005
Gulati, A., Jabbour, A., Ismail, T. F., Guha, K., Khwaja, J., Raza, S., et al. (2013). Association of fibrosis with mortality and sudden cardiac death in patients with nonischemic dilated cardiomyopathy. JAMA 309 (9), 896–908. doi:10.1001/jama.2013.1363
Gust, J., Hay, K. A., Hanafi, L. A., Li, D., Myerson, D., Gonzalez-Cuyar, L. F., et al. (2017). Endothelial activation and blood-brain barrier disruption in neurotoxicity after adoptive immunotherapy with CD19 CAR-T cells. Cancer Discov. 7 (12), 1404–1419. doi:10.1158/2159-8290.Cd-17-0698
Hafeez, U., Parakh, S., Gan, H. K., and Scott, A. M. (2020). Antibody-drug conjugates for cancer therapy. Molecules 25 (20), 4764. doi:10.3390/molecules25204764
Hao, Z., Li, R., Meng, L., Han, Z., and Hong, Z. (2020). Macrophage, the potential key mediator in CAR-T related CRS. Exp. Hematol. Oncol. 9, 15. doi:10.1186/s40164-020-00171-5
Hay, K. A., Hanafi, L. A., Li, D., Gust, J., Liles, W. C., Wurfel, M. M., et al. (2017). Kinetics and biomarkers of severe cytokine release syndrome after CD19 chimeric antigen receptor-modified T-cell therapy. Blood 130 (21), 2295–2306. doi:10.1182/blood-2017-06-793141
Hay, Z. L. Z., and Slansky, J. E. (2022). Granzymes: the molecular executors of immune-mediated cytotoxicity. Int. J. Mol. Sci. 23 (3), 1833. doi:10.3390/ijms23031833
Henri, C., Heinonen, T., and Tardif, J. C. (2016). The role of biomarkers in decreasing risk of cardiac toxicity after cancer therapy. Biomark. Cancer 8 (Suppl. 2), 39–45. doi:10.4137/bic.S31798
Herrero-Beaumont, G., Martinez Calatrava, M. J., and Castaneda, S. (2012). Abatacept mechanism of action: concordance with its clinical profile. Reumatol. Clin. 8 (2), 78–83. doi:10.1016/j.reuma.2011.08.002
Huang, H., Yu, L., Weng, H., Zhang, W., Wang, Z., Wang, L., et al. (2024). Advances in CAR-T cell therapy for hematologic and solid malignancies: latest updates from 2024 ESMO Congress. J. Hematol. Oncol. 17 (1), 120. doi:10.1186/s13045-024-01639-1
Huarte, E., O'Connor, R. S., Peel, M. T., Nunez-Cruz, S., Leferovich, J., Juvekar, A., et al. (2020). Itacitinib (INCB039110), a JAK1 inhibitor, reduces cytokines associated with cytokine release syndrome induced by CAR T-cell therapy. Clin. Cancer Res. 26 (23), 6299–6309. doi:10.1158/1078-0432.Ccr-20-1739
Huarte, E., Peel, M. T., Verbist, K., Fay, B. L., Bassett, R., Albeituni, S., et al. (2021). Ruxolitinib, a JAK1/2 inhibitor, ameliorates cytokine storm in experimental models of hyperinflammation syndrome. Front. Pharmacol. 12, 650295. doi:10.3389/fphar.2021.650295
Hughes, A. D., Teachey, D. T., and Diorio, C. (2024). Riding the storm: managing cytokine-related toxicities in CAR-T cell therapy. Seminars Immunopathol. 46 (3), 5. doi:10.1007/s00281-024-01013-w
Jadlowsky, J. K., Hexner, E. O., Marshall, A., Grupp, S. A., Frey, N. V., Riley, J. L., et al. (2025). Long-term safety of lentiviral or gammaretroviral gene-modified T cell therapies. Nat. Medicine. doi:10.1038/s41591-024-03478-6
Jeong, D., Gladish, G., Chitiboi, T., Fradley, M. G., Gage, K. L., and Schiebler, M. L. (2019). MRI in cardio-oncology: a review of cardiac complications in oncologic care. J. Magn. Reson Imaging 50 (5), 1349–1366. doi:10.1002/jmri.26895
Jiang, T., Peng, D., Shi, W., Guo, J., Huo, S., Men, L., et al. (2021). IL-6/STAT3 signaling promotes cardiac dysfunction by upregulating FUNDC1-dependent mitochondria-associated endoplasmic reticulum membranes formation in sepsis mice. Front. Cardiovasc Med. 8, 790612. doi:10.3389/fcvm.2021.790612
Johnson, J. D., Campisi, J., Sharkey, C. M., Kennedy, S. L., Nickerson, M., Greenwood, B. N., et al. (2005). Catecholamines mediate stress-induced increases in peripheral and central inflammatory cytokines. Neuroscience 135 (4), 1295–1307. doi:10.1016/j.neuroscience.2005.06.090
Joseph, T., Sanchez, J., Abbasi, A., Zhang, L., Sica, R. A., and Duong, T. Q. (2025). Cardiotoxic effects following CAR-T cell therapy: a literature review. Curr. Oncol. Rep. 27, 135–147. doi:10.1007/s11912-024-01634-2
June, C. H., and Sadelain, M. (2018). Chimeric antigen receptor therapy. N. Engl. J. Med. 379 (1), 64–73. doi:10.1056/NEJMra1706169
Lakomy, T., Akhoundova, D., Nilius, H., Kronig, M. N., Novak, U., Daskalakis, M., et al. (2023). Early use of corticosteroids following CAR T-cell therapy correlates with reduced risk of high-grade CRS without negative impact on neurotoxicity or treatment outcome. Biomolecules 13 (2), 382. doi:10.3390/biom13020382
Le, R. Q., Li, L., Yuan, W., Shord, S. S., Nie, L., Habtemariam, B. A., et al. (2018). FDA approval summary: tocilizumab for treatment of chimeric antigen receptor T cell-induced severe or life-threatening cytokine release syndrome. Oncologist 23 (8), 943–947. doi:10.1634/theoncologist.2018-0028
Lee, D. W., Gardner, R., Porter, D. L., Louis, C. U., Ahmed, N., Jensen, M., et al. (2014). Current concepts in the diagnosis and management of cytokine release syndrome. Blood 124 (2), 188–195. doi:10.1182/blood-2014-05-552729
Lee, D. W., Kochenderfer, J. N., Stetler-Stevenson, M., Cui, Y. K., Delbrook, C., Feldman, S. A., et al. (2015). T cells expressing CD19 chimeric antigen receptors for acute lymphoblastic leukaemia in children and young adults: a phase 1 dose-escalation trial. Lancet 385 (9967), 517–528. doi:10.1016/s0140-6736(14)61403-3
Lee, D. W., Santomasso, B. D., Locke, F. L., Ghobadi, A., Turtle, C. J., Brudno, J. N., et al. (2019). ASTCT consensus grading for cytokine release syndrome and neurologic toxicity associated with immune effector cells. Biol. Blood Marrow Transpl. 25 (4), 625–638. doi:10.1016/j.bbmt.2018.12.758
Lefebvre, B., Kang, Y., Smith, A. M., Frey, N. V., Carver, J. R., and Scherrer-Crosbie, M. (2020). Cardiovascular effects of CAR T cell therapy: a retrospective study. JACC CardioOncol 2 (2), 193–203. doi:10.1016/j.jaccao.2020.04.012
Letscher, K. P., and Reddy, S. T. (2024). Multidimensional analysis reveals predictive markers for CAR-T efficacy. Nat. Cancer 5 (7), 960–961. doi:10.1038/s43018-024-00785-2
Li, Q., Ye, W. X., Huang, Z. J., Zhang, Q., and He, Y. F. (2019a). Effect of IL-6-mediated STAT3 signaling pathway on myocardial apoptosis in mice with dilated cardiomyopathy. Eur. Rev. Med. Pharmacol. Sci. 23 (7), 3042–3050. doi:10.26355/eurrev_201904_17586
Li, Y., He, Y., Butler, W., Xu, L., Chang, Y., Lei, K., et al. (2019b). Targeting cellular heterogeneity with CXCR2 blockade for the treatment of therapy-resistant prostate cancer. Sci. Transl. Med. 11 (521), eaax0428. doi:10.1126/scitranslmed.aax0428
Linette, G. P., Stadtmauer, E. A., Maus, M. V., Rapoport, A. P., Levine, B. L., Emery, L., et al. (2013). Cardiovascular toxicity and titin cross-reactivity of affinity-enhanced T cells in myeloma and melanoma. Blood 122 (6), 863–871. doi:10.1182/blood-2013-03-490565
Liu, H., Zhang, T., Zhang, L., and Zhong, Y. (2025). Neuroinflammatory mechanisms of adult sepsis-associated encephalopathy: implications for blood–brain barrier disruption and oxidative stress. Diagnostics 15 (7), 873. doi:10.3390/diagnostics15070873
Lyon, A. R., López-Fernández, T., Couch, L. S., Asteggiano, R., Aznar, M. C., Bergler-Klein, J., et al. (2022). 2022 ESC guidelines on cardio-oncology developed in collaboration with the European hematology association (EHA), the European society for therapeutic radiology and oncology (ESTRO) and the international cardio-oncology society (IC-OS). Eur. Heart J. 43 (41), 4229–4361. doi:10.1093/eurheartj/ehac244
Maher, J., Brentjens, R. J., Gunset, G., Rivière, I., and Sadelain, M. (2002). Human T-lymphocyte cytotoxicity and proliferation directed by a single chimeric TCRzeta/CD28 receptor. Nat. Biotechnol. 20 (1), 70–75. doi:10.1038/nbt0102-70
Mahmood, S. S., Riedell, P. A., Feldman, S., George, G., Sansoterra, S. A., Althaus, T., et al. (2023). Biomarkers and cardiovascular outcomes in chimeric antigen receptor T-cell therapy recipients. Eur. Heart J. 44 (22), 2029–2042. doi:10.1093/eurheartj/ehad117
Maldini, C. R., Ellis, G. I., and Riley, J. L. (2018). CAR T cells for infection, autoimmunity and allotransplantation. Nat. Rev. Immunol. 18 (10), 605–616. doi:10.1038/s41577-018-0042-2
Maude, S. L., Frey, N., Shaw, P. A., Aplenc, R., Barrett, D. M., Bunin, N. J., et al. (2014). Chimeric antigen receptor T cells for sustained remissions in leukemia. N. Engl. J. Med. 371 (16), 1507–1517. doi:10.1056/NEJMoa1407222
Men, L., Guo, J., Cao, Y., Huang, B., Wang, Q., Huo, S., et al. (2023). IL-6/gp130/STAT3 signaling contributed to the activation of the PERK arm of the unfolded protein response in response to chronic β-adrenergic stimulation. Free Radic. Biol. Med. 205, 163–174. doi:10.1016/j.freeradbiomed.2023.06.005
Mestermann, K., Giavridis, T., Weber, J., Rydzek, J., Frenz, S., Nerreter, T., et al. (2019). The tyrosine kinase inhibitor dasatinib acts as a pharmacologic on/off switch for CAR T cells. Sci. Transl. Med. 11 (499), eaau5907. doi:10.1126/scitranslmed.aau5907
Mohanty, R., Chowdhury, C. R., Arega, S., Sen, P., Ganguly, P., and Ganguly, N. (2019). CAR T cell therapy: a new era for cancer treatment (Review). Oncol. Rep. 42 (6), 2183–2195. doi:10.3892/or.2019.7335
Mosser, D. M., and Edwards, J. P. (2008). Exploring the full spectrum of macrophage activation. Nat. Rev. Immunol. 8 (12), 958–969. doi:10.1038/nri2448
Mulvey, A., Trueb, L., Coukos, G., and Arber, C. (2025). Novel strategies to manage CAR-T cell toxicity. Nat. Rev. Drug Discovery. doi:10.1038/s41573-024-01100-5
Neelapu, S. S. (2019). Managing the toxicities of CAR T-cell therapy. Hematol. Oncol. 37 (Suppl. 1), 48–52. doi:10.1002/hon.2595
Neelapu, S. S., Tummala, S., Kebriaei, P., Wierda, W., Gutierrez, C., Locke, F. L., et al. (2018). Chimeric antigen receptor T-cell therapy - assessment and management of toxicities. Nat. Rev. Clin. Oncol. 15 (1), 47–62. doi:10.1038/nrclinonc.2017.148
Norelli, M., Camisa, B., Barbiera, G., Falcone, L., Purevdorj, A., Genua, M., et al. (2018). Monocyte-derived IL-1 and IL-6 are differentially required for cytokine-release syndrome and neurotoxicity due to CAR T cells. Nat. Med. 24 (6), 739–748. doi:10.1038/s41591-018-0036-4
Nunes, F., de Gusmão, B. M., Wiginesk, F. B., Manenti, E., Soares, J., Freitas, M. G., et al. (2024). From the mechanism of action to clinical management: a review of cardiovascular toxicity in adult treated with CAR-T therapy. Hematol. Transfus. Cell Ther. 47, 103693. doi:10.1016/j.htct.2024.06.008
Padmanabhan, A. (2018). Cellular collection by apheresis. Transfusion 58, 598–604. doi:10.1111/trf.14502
Parampalli Yajnanarayana, S., Stübig, T., Cornez, I., Alchalby, H., Schönberg, K., Rudolph, J., et al. (2015). JAK1/2 inhibition impairs T cell function in vitro and in patients with myeloproliferative neoplasms. Br. J. Haematol. 169 (6), 824–833. doi:10.1111/bjh.13373
Perry, C., and Rayat, A. (2021). Lentiviral vector bioprocessing. Viruses-Basel 13 (2), 268. doi:10.3390/v13020268
Sadelain, M., Brentjens, R., and Rivière, I. (2013). The basic principles of chimeric antigen receptor design. Cancer Discov. 3 (4), 388–398. doi:10.1158/2159-8290.Cd-12-0548
Santomasso, B., Bachier, C., Westin, J., Rezvani, K., and Shpall, E. J. (2019). The other side of CAR T-cell therapy: cytokine release syndrome, neurologic toxicity, and financial burden. Am. Soc. Clin. Oncol. Educ. Book 39, 433–444. doi:10.1200/edbk_238691
Schmid, D., Dengjel, J., Schoor, O., Stevanovic, S., and Münz, C. (2006). Autophagy in innate and adaptive immunity against intracellular pathogens. J. Mol. Medicine-Jmm 84 (3), 194–202. doi:10.1007/s00109-005-0014-4
Shaked, I., Hanna, R. N., Shaked, H., Chodaczek, G., Nowyhed, H. N., Tweet, G., et al. (2015). Transcription factor Nr4a1 couples sympathetic and inflammatory cues in CNS-recruited macrophages to limit neuroinflammation. Nat. Immunol. 16 (12), 1228–1234. doi:10.1038/ni.3321
Shalabi, H., Sachdev, V., Kulshreshtha, A., Cohen, J. W., Yates, B., Rosing, D. R., et al. (2020). Impact of cytokine release syndrome on cardiac function following CD19 CAR-T cell therapy in children and young adults with hematological malignancies. J. Immunother. Cancer 8 (2), e001159. doi:10.1136/jitc-2020-001159
Shank, B. R., Do, B., Sevin, A., Chen, S. E., Neelapu, S. S., and Horowitz, S. B. (2017). Chimeric antigen receptor T cells in hematologic malignancies. Pharmacotherapy 37 (3), 334–345. doi:10.1002/phar.1900
Shimabukuro-Vornhagen, A., Gödel, P., Subklewe, M., Stemmler, H. J., Schlößer, H. A., Schlaak, M., et al. (2018). Cytokine release syndrome. J. Immunother. Cancer 6 (1), 56. doi:10.1186/s40425-018-0343-9
Siegel, R. L., Miller, K. D., Wagle, N. S., and Jemal, A. (2023). Cancer statistics, 2023. CA Cancer J. Clin. 73 (1), 17–48. doi:10.3322/caac.21763
Simela, C., Walker, J. M., Ghosh, A. K., and Chen, D. H. (2025). SGLT2 inhibitors for prevention and management of cancer treatment-related cardiovascular toxicity: a review of potential mechanisms and clinical insights. Cardio-Oncology 11 (1), 15. doi:10.1186/s40959-024-00284-4
Singh, D., Thakur, A., and Tang, W. H. (2015). Utilizing cardiac biomarkers to detect and prevent chemotherapy-induced cardiomyopathy. Curr. Heart Fail Rep. 12 (3), 255–262. doi:10.1007/s11897-015-0258-4
Slivnick, J., Vallakati, A., Addison, D., Wallner, A., and Tong, M. S. (2020). Personalized approach to cancer treatment-related cardiomyopathy. Curr. Heart Fail Rep. 17 (2), 43–55. doi:10.1007/s11897-020-00453-3
Sterner, R. M., Sakemura, R., Cox, M. J., Yang, N., Khadka, R. H., Forsman, C. L., et al. (2019). GM-CSF inhibition reduces cytokine release syndrome and neuroinflammation but enhances CAR-T cell function in xenografts. Blood 133 (7), 697–709. doi:10.1182/blood-2018-10-881722
U.S. Food and Drug Administration (2017a). Kymriah. Available online at: https://www.fda.gov/vaccines-blood-biologics/cellular-gene-therapy-products/kymriah-tisagenlecleucel (Accessed January 15, 2025).
U.S. Food and Drug Administration (2017b). Yescarta. Available online at: https://www.fda.gov/vaccines-blood-biologics/cellular-gene-therapy-products/yescarta-axicabtagene-ciloleucel (Accessed January 15, 2025).
U.S. Food and Drug Administration (2020). Tecartus. Available online at: https://www.fda.gov/vaccines-blood-biologics/cellular-gene-therapy-products/tecartus-brexucabtagene-autoleucel (Accessed January 15, 2025).
U.S. Food and Drug Administration (2021). Breyanzi. Available online at: https://www.fda.gov/vaccines-blood-biologics/cellular-gene-therapy-products/breyanzi-lisocabtagene-maraleucel (Accessed January 15, 2025).
Yoshikai, Y., and Nishimura, H. (2000). The role of interleukin 15 in mounting an immune response against microbial infections. Microbes Infect. 2 (4), 381–389. doi:10.1016/s1286-4579(00)00329-4
Zhao, W. H., Liu, J., Wang, B. Y., Chen, Y. X., Cao, X. M., Yang, Y., et al. (2018). A phase 1, open-label study of LCAR-B38M, a chimeric antigen receptor T cell therapy directed against B cell maturation antigen, in patients with relapsed or refractory multiple myeloma. J. Hematol. Oncol. 11 (1), 141. doi:10.1186/s13045-018-0681-6
Zhao, Y., Bai, X., Guo, S., Zhang, X., Liu, J., Zhao, M., et al. (2024). Efficacy and safety of CAR-T therapy targeting CLL1 in patients with extramedullary diseases of acute myeloid leukemia. J. Transl. Med. 22 (1), 888. doi:10.1186/s12967-024-05705-7
Keywords: CAR T cell, cardio-oncology, cytokine release syndrome, cardiotoxicity, chimeric antigen receptor
Citation: Liu J-H, Liu K-Y, Zhao X, Zhou X and Jiang Y (2025) Cardiovascular toxicities associated with chimeric antigen receptor T-cell therapy. Front. Pharmacol. 16:1578157. doi: 10.3389/fphar.2025.1578157
Received: 17 February 2025; Accepted: 08 April 2025;
Published: 08 May 2025.
Edited by:
Lin Zhao, Capital Medical University, ChinaCopyright © 2025 Liu, Liu, Zhao, Zhou and Jiang. This is an open-access article distributed under the terms of the Creative Commons Attribution License (CC BY). The use, distribution or reproduction in other forums is permitted, provided the original author(s) and the copyright owner(s) are credited and that the original publication in this journal is cited, in accordance with accepted academic practice. No use, distribution or reproduction is permitted which does not comply with these terms.
*Correspondence: Xin Zhou, eGluemhvdUBqbHUuZWR1LmNu; Yichuan Jiang, ZmlnYXJvQGpsdS5lZHUuY24=