- 1Department of Psychiatry, Hamad Medical Corporation, Doha, Qatar
- 2Department of Physical Medicine and Rehabilitation, Harvard Medical School, Spaulding Rehabilitation Hospital, Boston, MA, United States
- 3Neuromodulation Center and Center for Clinical Research Learning, Harvard Medical School, Spaulding Rehabilitation Hospital and Massachusetts General Hospital, Boston, MA, United States
- 4Universidad San Ignacio de Loyola, Vicerrectorado de Investigación, Unidad de Investigación para la Generación y Síntesis de Evidencias en Salud, Lima, Peru
- 5Department of Psychiatry and Mental Health, School of Medicine and Dental Health, The University of Dodoma, Dodoma, Tanzania
- 6Hospital Infantil de México Federico Gómez, Mexico City, Mexico
Depressive disorders are among the most common psychiatric conditions and contribute to significant morbidity. Even though the use of antidepressants revolutionized the management of depression and had a tremendous positive impact on the patient's outcome, a significant proportion of patients with major depressive disorder (MDD) show no or partial or response even with adequate treatment. Given the limitations of the prevailing monoamine hypothesis-based pharmacotherapy, glutamate and glutamatergic related pathways may offer an alternative and a complementary option for designing novel intervention strategies. Over the past few decades, there has been a growing interest in understanding the neurobiological underpinnings of glutamatergic dysfunctions in the pathogenesis of depressive disorders and the development of new pharmacological and non-pharmacological treatment options. There is a growing body of evidence for the efficacy of neuromodulation techniques, including transcranial magnetic stimulation, transcutaneous direct current stimulation, transcranial alternating current stimulation, and photo-biomodulation on improving connectivity and neuroplasticity associated with depression. This review attempts to revisit the role of glutamatergic neurotransmission in the etiopathogenesis of depressive disorders and review the current neuroimaging, neurophysiological and clinical evidence of these neuromodulation techniques in the pathophysiology and treatment of depression.
Introduction
Different theories were put forward to explain the etiopathogenesis of depression. This has been a major challenge for the development of treatment options, since only 30% of patients with MDD who receive an adequate treatment experience full remission (1). Some of the neurobiological factors theoretically associated with depression are the activation of the inflammatory system, hypothalamic-pituitary-adrenal axis disturbances, dysfunctional neuroanatomic circuits (particularly the default mode network), abnormal neural activity, neurotransmitter dysfunction, polymorphisms in the 5-HTT promoter region (5HTTLPR) and interactions between brain derived neurotrophic factor (BDNF); and neurotrophic tyrosine kinase receptor 2 (NTRK2) polymorphisms (2).
Theories of neurotransmitter dysfunction in depression are well established. The monoamine theory proposes that depression is caused by a decrease of the extracellular availability of noradrenaline and serotonin. Hence, the therapeutic effect of antidepressants aimed to increase their availability. Despite the multiple studies about various multimodal pharmacological and psychological treatments of depression, the evidence for the efficacy and tolerability of these treatment options remains insufficient (3).
The delayed, and in many cases poor, response to the current antidepressants may suggest that there are more neurotransmitters involved in the pathophysiology of depression than those accounted for by the monoamine theory. Prominent among them is the glutamatergic system which may contribute to the dysfunction and perceived treatment poor response (2). Given the complexity of mood disorders and the setbacks of previous monoaminergic targets, there has been growing evidence about non-invasive neuromodulating techniques that target networks such as the limbic-cortical system framework, producing both local and regional effects (4). Growing evidence from animal and human studies shows that structural pathology, network, and connectivity dysfunction related to deficits in excitatory glutamate neurons and inhibitory GABA interneurons in the cortical and limbic areas of the brain are implicated in the genesis of depressive symptoms. As current pharmacotherapeutic treatment for depression which focused on the monoamine hypothesis has significant limitations, glutamatergic related mechanisms promise to produce superior therapeutic interventions (5). This article reviews the proposed glutamatergic mechanisms of neuromodulation in the treatment of depression.
Glutamatergic System and Depression
Glutamate is the principal excitatory neurotransmitter in the brain, and glutamatergic mechanisms play significant roles in nearly all key functions affected in depressed states (6). Postmortem studies have put forward evidence linking glial cell abnormalities, whose role is synaptic glutamate removal, and the pathophysiology of mood disorders (7). Lower glutamine/glutamate levels have also been found in the cortex of patients with depression (8). The “much faster than usual” antidepressant effect of ketamine, discussed later, further solidifies the role of glutamate in treatment-resistant major depression. Ionotropic (N-methyl-D-aspartate [NMDA], α-Amino-3-Hydroxy-5-Methylisoxazole-4-Propionic Acid Receptors [AMPA], and kainate receptors [not discussed herein] and metabotropic (mGluR) glutamate receptors have been shown to be responsible for modulation of mood and associated functions that are impaired in depression (9). These observations have brought about the hypothesis that NMDA and/or AMPA receptors might be novel therapeutic targets for treating depression. Figure 1 summarizes the interplay among NMDA-R inhibition, gamma aminobutyric acid (GABA)-ergic interneuron disinhibition, and ketamine metabolites hydroxynorketamines (HNK), taking ketamine—a glutamatergic agent that has been intensively investigated over the past 20 years—as an example.
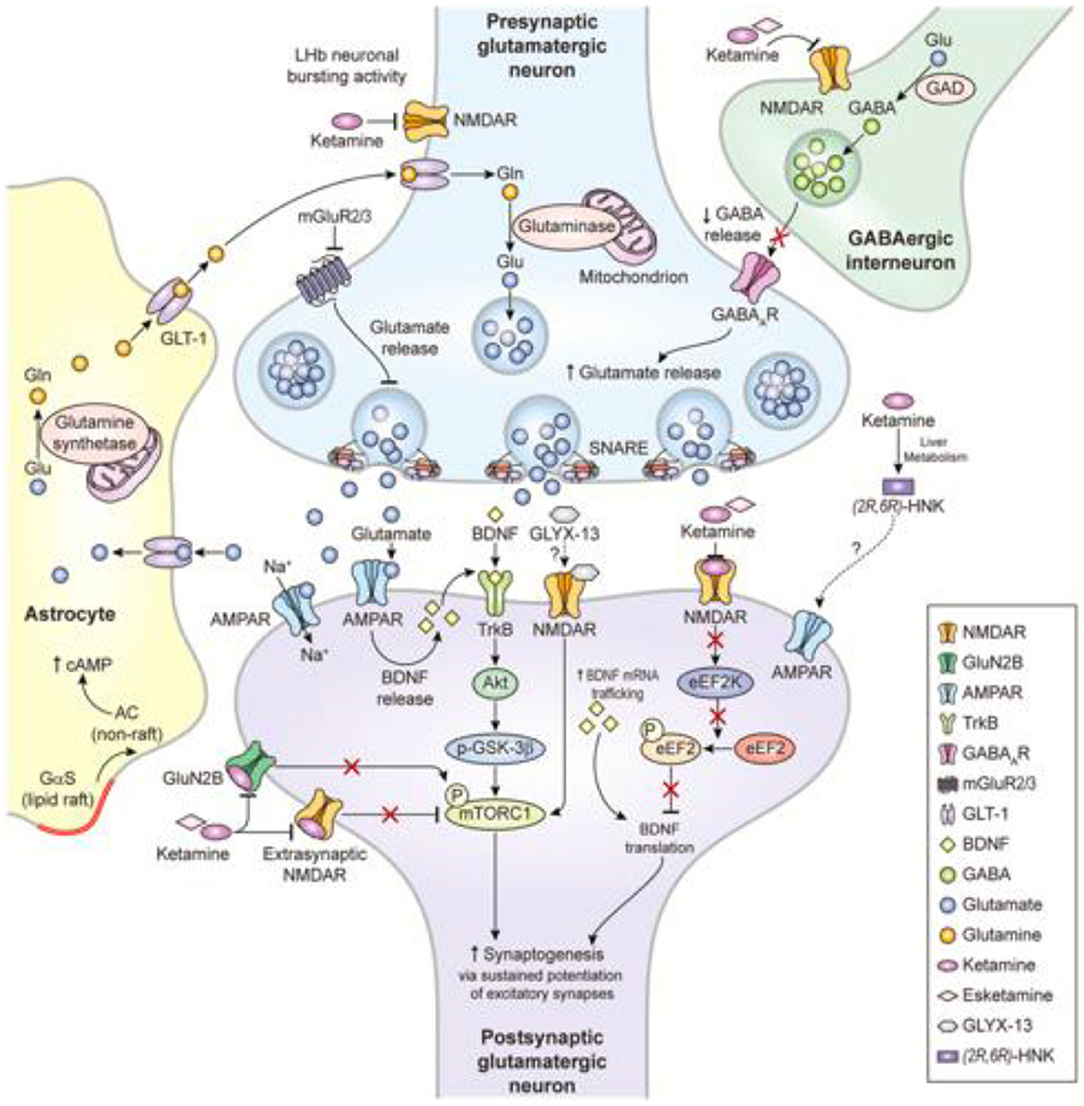
Figure 1. Proposed mechanisms of action of glutamatergic modulators and other putative rapid-acting antidepressants (10).
Ionotropic Receptors
These receptors are all cation-permeable, homo- and heteromeric blocks of structurally distinct subunits (11). Even though all three classes of ionotropic receptor pertain modulatory (allosteric) sites, the NMDA receptor (NMDA-R), a tetrameric heteromeric assembly of NR1 and NR2 subunits which contains glycine and glutamate recognition sites, respectively, is unique in possessing a co-agonist site and an additional site accessible to open channel blockers such as ketamine, memantine, and amantadine.
In several animal models, NMDA-R antagonists have been shown to produce antidepressant-like effects (12, 13). In humans, Crane (14, 15) was the first to report beneficial effects of high-dose D-cycloserine (DCS), an agent with NMDA-R activity initially developed to treat tuberculosis, on depressed mood, insomnia and anorexia. Although the antidepressant effects of NMDA-R blockers have not been consistent, a potent augmentation of antidepressant activity has been hypothesized when ketamine, an NMDA antagonist, was co-administered with other antidepressants to patients with depression (16, 17). NMDA antagonists are also not devoid of psychotomimetic, motor, and other adverse side effects, that might be mitigated by the use of CP-101,606, an NR2B-subunit antagonist (9).
Historically, NMDARs have received more attention as they are key players in the process of excitotoxicity, a pivotal mechanism in stroke and neurodegenerative disorders. While NMDA receptors allow entry of Ca2+, AMPA receptors heterotetramer, which consists of four glutamate receptor subunits (GluR1-4), mediate the entry of Na+ current into the neuron, thus resulting in rapid signal transmission (18). Compared with NMDA receptors in depression, AMPA receptors seem to be understimulated (19). Positive allosteric modulator Org 26576 has shown greater symptomatic improvement compared with placebo, as well as good tolerability and pharmacokinetic properties (20). Similarly, previous studies on drugs that combine serotonin uptake inhibition with positive allosteric modulation of AMPA receptors have shown that AMPA receptors potentiators (ARPs) or “AMPAkines” may augment the activity and perhaps expedite the onset of the therapeutic effects of biogenic amine and second messenger-based antidepressants (18, 21).
Ketamine: One Drug, Many Uses
Originally approved in 1970 as an anesthetic, ketamine thereafter earned notoriety as a recreational drug of abuse (“Special K”) because of its dissociative effects. Subsequently, in 2019, the Food and Drug Administration (FDA) approved the S-enantiomer of ketamine, esketamine, for patients with treatment-resistant depression. Although many monoaminergic treatments exist, at least a third of patients do not have a response after two or more trials of antidepressant drugs and are considered to have treatment-resistant depression (22). When evidence emerged about its rapid (within several hours) antidepressant effects (23), ketamine revolutionized the landscape as there was desperate need for urgent relief of suicidal crises and faster restoration of functioning, thereby providing a respite on economic and social burdens of treatment-resistant depression. Efficacy and safety studies (Studies 3002 and 3003) of esketamine in treatment-resistant depression showed its impressive and rapid onset of action on day 2 of treatment, along with its efficacy beyond 1 month in patients who had an initial response (24).
Ketamine is a mixture of two enantiomers (mirror image molecules): R- and S-ketamine. (2S,6S)- and (2R,6R)-HNK are ketamine's major metabolites (10). Esketamine's product monograph enumerates increased blood pressure, dissociation, dizziness, and nausea among others as common side effects (25). Nonetheless, Zanos et al. (26) indicated that (2R,6R)-HNK did not induce any of the side effects typically associated with ketamine.
Besides, some clinical trials have shown that by modulating rather than inhibiting NMDARs, may prevent ketamine's NMDAR inhibition-mediated side effects. For example, partial agonists at the NMDAR glycine B binding site, such as GLYX-13 (rapastinel) and D-cycloserine, produced antidepressant effects in both human and animal studies (27). Furthermore, another likely more potent and novel NMDAR enhancer, sarcosine, a glycine transporter-1 (GlyT-1) inhibitor, showed improvement in depressive behaviors and symptoms (28, 29). Another novel NMDAR enhancer which looks promising in the treatment of mood disorders is sodium benzoate, a D-amino acid oxidase (DAAO) inhibitor which prevents degradation of D-amino acid (30). A recent clinical trial demonstrated that sodium benzoate can decrease perceived stress, improve cognitive function, and enhance treatment adherence in late-life depression (31).
Metabotropic Receptors
Metabotropic glutamatergic receptors (mGluR)[homodimers], which are mainly distributed in corticolimbic areas, can be broadly divided into three categories according to their structures, ligand recognition profiles and coupling to cellular transduction systems: group I (mGluR1 and 5) receptors, group II (mGluR2 and 3), and group III (mGluR4, 6,7 and 8) (32).
Group I mGluR antagonists mimic certain properties of NMDA antagonists to some extent such as their influence on mood, as well as their neuroprotective effects (32, 33). This similarity could be explained by the indirect facilitation of NMDA receptors by presynaptic group I receptors, and thus promoting glutamate release in cortico-limbic areas such as the amygdala (34). Moreover, it has been shown that mGluR 5 antagonists have antidepressant actions in animal models of acute and chronic stress (35).
While group I receptors are positively coupled with Phospholipase C via Gq, group II and III receptors, on the other hand, are positively coupled to Gi, leading to an inhibitory effect on adenylyl cyclase (32, 36) which subsequently suppresses the release of glutamate (37, 38) and in turn modulates depressive states. As shown in Figure 1, mGluR 2/3 antagonists are thought to enhance synaptic glutamate levels, thereby boosting AMPAR transmission and firing rates and extracellular monoamine levels. A few articles have suggested that the antidepressant effects of group II antagonists is linked to their induction of glutamate release in the serotonin-rich dorsal raphe nucleus (DRN) (39, 40). The DRN has been strongly associated with mood disorders such as depression (41). Furthermore, group III agonists have been found to possess antidepressant effects in rodent models by reducing excessive glutamate release (42, 43).
Assessing the Glutamatergic System
The assessment of glutamate and glutamate receptors is of high interest across many neuropsychiatric disorders. However, compared to other neurotransmitter systems, its in-vivo assessments pose several challenges (44). The most common assessment approach is the so-called “molecular imaging” with positron emission tomography (PET) and single photon emission computed tomography (SPECT). These techniques have the advantage of being an in vivo technique and having an excellent safety profile (45). Specific tracers are available for PET/SPECT molecular imaging of subtypes of the ionotropic (NMDA, AMPA, and kainate receptors) and metabotropic glutamate receptors (mGluRs).
Due to conformational issues and the difficulty of utilizing brain-penetrating ligands for amino acid receptors, there has been little advance in developing radiotracers for ionotropic glutamate receptors, with the exception of the NMDA receptor (GluN2B subunit ligand). On the other hand, mGluR imaging, particularly for subtypes 5 and 1, has been found effective (44), and has been used in studying the pathophysiology, endophenotypes, and drug development for depression (46–48).
Moreover, 1H magnetic resonance spectroscopy (MRS) is also a non-invasive method for the assessment of levels of neurometabolites including glutamatergic ones in the brain (49). MRS takes advantage of differential protons' magnetic fields depending on their chemical environment. Using this property, it helps distinguish between different chemical compounds, and quantify their concentrations, including glutamate-related metabolites (50). The metabolites are measured in a specified volume, the voxel. MRS was widely used in depression studies showing that patients with depression had lower glutamate and glutathione (GSH) levels; however, due to overlapping signals and low concentrations, this method is prone to signal-to-noise ratio challenges. New methodological improvements are needed to boost this ratio and increase the likelihood of generating robust and reliable results in the study of depression (51, 52).
Furthermore, one can indirectly assess the glutamatergic synapsis in the cortex using transcranial magnetic stimulation (TMS) with paired pulse protocol. The intracortical facilitation (ICF) metric has been associated with glutamate tonus in the motor cortex (53, 54). In particular, pharmacological studies with paired-pulse TMS have demonstrated that both GABA-A agonist (55, 56) and NMDA antagonist reduced ICF (57, 58). Thus, ICF is assumed to be mainly associated with glutamate receptor-mediated excitatory functions in the motor cortex. Only few studies have assessed the role of ICF in depression with heterogenous results (59–61). New evidence on glutamatergic tonus from non-motor cortex (such as DLPFC) areas with combined TMS-EEG protocols is needed.
Although, none of the above methods is the perfect and reliable technique to assess glutamatergic pathways in patients with depression. The combination of clinical and multimodal neuroimaging assessments is the best approach for robust and reproducible protocols.
Modulatory Techniques That Target Glutamate Pathways
The International Neuromodulation Society defines therapeutic neuromodulation as “the alteration of nerve activity through targeted delivery of a stimulus, such as electrical stimulation or chemical agents, to specific neurological sites in the body (62).
In the last decades, several non-invasive brain stimulation techniques have been used to treat depression in humans, including rTMS or tDCS. Reduced connectivity in the prefrontal cortex and anterior cingulate gyrus and reduced neuroplasticity are found in depressive disorders and play important roles in the pathophysiology of depression (63–66).
Brain stimulation's effect on depression might be through neuroplasticity modulation. Electroconvulsive therapy (ECT) is among the oldest brain stimulation techniques that have been used to treat refractory depression. ECT impacts plasticity-associated transcripts and their proteins in the hippocampus (67). Also, several clinical studies have shown an increase in connectivity and cerebellar volume in patients with MDD (68–72) and white matter modulation in the pathways between the frontal and limbic areas after receiving ECT therapy (73). Animal models showed that ECT influences the glutamatergic system; rat models with decreased glutamate, exhibited NR2B upregulation after ECT.
TMS is a non-invasive technique of brain stimulation that uses the principles of electromagnetic induction to produce an electrical current in the brain surface. A magnetic field induces a secondary electrical current. There are different types of TMS including rTMS which generates repetitive magnetic pulses from seconds to minutes. rTMS is a Food and Drug Administration (FDA) approved treatment for patients with MDD who are resistant to antidepressant treatment. rTMS might stimulate neurogenesis like ECT, and also modulate brain activity and neurotransmitters including dopamine and serotonin. Cortical excitability modulation might be impaired in patients with depression, and TMS might improve cortical modulation (74), and modulate functional connectivity between the central executive work and default mode network (75).
Transcutaneous Direct Current Stimulation TDCS
tDCS is another noninvasive brain stimulation technique for the treatment of depressive disorders. The device of tDCS has two electrodes, anode, and cathode. It applies a constant low current (0.5–2mA) via electrodes on the scalp and changes the cortical excitability.
At a neuronal level, tDCS modulates the resting membrane potential in a polarity-dependent fashion: anodal stimulation increases cortical excitability in the stimulated region while cathodal decreases it.
A recent meta-analysis that included 27 randomized controlled trials concluded that tDCS was effective in the treatment of depression when compared to sham (76). Also, some authors consider that tDCS should be considered as “definitely effective” (Level A) given the current evidence.
Transcranial Alternating Current Stimulation (TACS)
Another stimulation approach used to modulate endogenous brain activity is tACS, which applies a weak bidirectional and biphasic current with a sine-wave pattern to the scalp for a duration of 2–5 min (lower than the typical time for stimulation for tDCS which is 20 min) (77). Three recent articles have showcased the potential therapeutic effects of tACS for the treatment of MDD and cognitive impairment: one well-structured double-blind randomized clinical trial (78) and two case reports (79, 80). In a nutshell, these three papers seem to indicate that tACS is a safe, even during pregnancy, and efficacious long-term tool in MDD treatment research. Proposed mechanisms of action for tACS have been changes in cortical excitability, in brain electrical activity, and biochemical changes including neurotransmitter release (77). This non-invasive brain stimulation paradigm deserves further investigation in larger randomized trials and with various neurophysiologic assessments, especially that the mechanism of tACS, in association with the glutamatergic pathway, has been understudied.
Photo-Biomodulation
Light has a wide range of effects on physiological and behavioral functions, including circadian rhythm, mood, and cognition (81, 82).
Recent research studies support antidepressant effects of light therapy, while light deprivation can induce depressive-like behaviors, which further indicates that light signals are a powerful modulator of mood-related behaviors.
Photo-biomodulation therapy (PBMT) is a novel and non-invasive therapy based on delivering photons in the range of red to near-infrared (NIR) spectra (600–1,100 nm) inside tissues (83, 84). PBMT can efficiently penetrate biological tissues including the CNS and produce beneficial photo-biomodulation effects; some of the proposed mechanisms of action are the increase in ATP synthesis, neurogenesis stimulation, increase on brain perfusion, and decrease of inflammation (85).
Recently, several animal and clinical studies on MDD have shown that PBMT can induce antidepressant-like effects when the prefrontal cortex (PFC) is targeted (86, 87). However, the mechanism by which PBMT ameliorates glutamatergic dysfunction to display the antidepressant phenotype is unclear.
Zhan et al. found in an animal model, that tPBMT decreased extracellular glutamate levels via upregulation of glutamate transporter-1 (GLT-1), alleviated dendritic atrophy and upregulated the expression of AMPA receptors on the postsynaptic membrane. This was associated with behaviorally significant antidepressant effects in mice exposed to chronic unpredictable mild stress (88).
Vagal Nerve Stimulation (VNS)
VNS, initially developed for seizure disorders, came into the spotlight when patients reported improvements in mood after being treated for epilepsy. VNS involves surgically implanting a vagal nerve stimulator in the upper left area of the chest wall which then provides electrical stimulation to the vagal (tenth cranial) nerve. The stimulation lasts for 30 s, and can be turned on or off by the patient by holding a magnet over the device. The largest VNS study targeting treatment-resistant MDD (failure to respond to medications and ECT) consisted of 235 patients who received either VNS or a placebo/sham treatment (89). The duration of treatment was over 12 weeks and there was only a 15% positive response rate and 10% remission rate. However, positive response increased to 34% over time (90). This finding suggests that VNS would not be recommended for an acute debilitating depressive episode or high-risk clinical situations of harm to self or others. Nonetheless, this treatment is worth considering as an adjunct to medication management. One theory behind the antidepressant effects of VNS is the decrease of glutamate concentration in the rostral anterior cingulate cortex (91).
Current Neurophysiological and Clinical Evidence
Non-invasive brain stimulation offers a potential alternative, non-pharmacologic approach to treat unipolar depression and treatment resistant depression (TRD), even though there is some evidence about the efficacy and safety of non-invasive neuromodulation techniques to treat unipolar depression, especially in the case of ECT and rTMS (92).
The rationale for neuromodulation for depression is based on a neural network theory that posits a specific set of structurally and functionally connected brain regions that work together to maintain normal mood regulation (93, 94), however, little is known about the specific mechanisms.
The putative cellular mechanisms of action to this group of therapies involve the action over the glutamatergic neuronal firing, depending on the technique and polarity used, TMS and ECT will depolarize the cell membrane and generate an action potential while tDCS have an impact on the action potential depending on the polarity, cathodal stimulation will decrease, and anodal stimulation will increase the action potential. The after-effects are mediated by “long-term potentiation (LTP)-like” mechanisms where the upregulation of neurotransmitter release facilitates the opening of AMPARs and indirectly that of NMDARs (95). The mechanism of action of tDCS in psychiatric disorders has been attributed to the effects of anodal stimulation (96). Studies often report the anode placement over the frontal cortex while the cathodal electrode placement reports are more inconsistent, however, there are reports of inflammation reduction and neuroprotective effects by cathodal stimulation (97).
In this review, we looked for the possible clinical impact of different neuromodulation techniques over glutamate pathways and we found three rTMS studies (Table 1): 2 with TRD patients (98, 99) and another with MDD patients (100) that were not taking any pharmacological treatment. MRI spectroscopy was performed before and after the intervention to assess the glutamate/GABA ratio (Table 1). The studies found modifications on the glutamatergic and GABAergic neurotransmission after high-frequency rTMS treatment on DLPFC. Specifically, an increase of the glutamine/creatine ratio (100) and GABA concentrations (98, 99) on the left DLPFC after ~20–25 daily sessions. Indeed, this justify further research on glutamate biomarkers for the optimization of rTMS protocols in depression.
Given that deficits in glutamate in cortical and limbic regions are implicated in MDD and causally related to depressive-like behaviors; treatment that reverses or normalizes glutamate can improve depressive symptoms. Glutamate receptors, specifically the metabotropic subgroup II receptors of mGluRs are targets of interest in developing novel antidepressants (103). Preclinical studies have demonstrated that the antagonism and negative allosteric modulation of mGlu2/3 receptor has antidepressant properties (104, 105). Indeed, the expression mGluR2/3 receptors reduction is observed in the anterior cingulate cortex among patients with MDD (106, 107). Furthermore, significant increase in CSF levels of glutamate and glutamine among MDD patients compared to healthy control (108) has been observed and linked to hyperactivation of NMDA receptors (109), inflammatory process (110, 111) and serotonergic signaling (111). Similarly, a systematic review and meta-analysis evidence shows that even peripheral blood glutamate levels were significantly increased in MDD patients compared to the controls (112).
While pharmacological techniques have targeted the glutamatergic receptors as a therapeutic target for treating depressive disorders, neuromodulation techniques offer alternative or augmentative treatment for the same. Non-invasive brain stimulation techniques including the non-convulsive TMS and tDCS and convulsive ECT and MST provide safe options targeting specific areas in dorsolateral prefrontal cortex (DLPFC) which is a key site for the frontoparietal network (FPN). In hypoactive state, (FPN) is associated with hyperactivity of the default mode network (DMN) that may promote depressive behavior and cognitions such as self-referential processing, depressive rumination and negative bias observed in MDD (113–115).
Provided at high frequency (HF-TMS), low frequency (LF-TMS), theta-bursts (TBS) and deep (dTMS) (116, 117); clinical trials have demonstrated the efficacy and safety of TMS treatment. HF-TMS over the left DLPFC is shown to have a superior effectiveness compared to sham treatment in individuals with depression even when used without concomitant antidepressant (118, 119), and has the benefit of accelerating clinical response (120) and also effective as a monotherapy for both unipolar or bipolar depression (121). In case of poor response to HF-rTMS, LF-TMS can be especially advantageous and safe when there is high risk of seizures and poor tolerability to pain (117, 122).
Similar to TMS techniques, tDCS targets left DLPFC primarily aiming to counterbalance the hyperactivity of the DMN secondary to hypoactivity of the frontoparietal network of the left DLPFC (123). tDCS combined with antidepressant produce faster and greater response compared to the antidepressant alone or tDCS alone (119). In a non-inferiority trial, tDCS failed to show its non-inferiority to escitalopram, but was still superior to placebo (124).
As for convulsive modalities, ECT has been used for decades as second line treatment for MDD and in some cases such as acute suicidal or psychotic features, it can be used as a first line. ECT is now considered safe, effective and tolerable with increased efficacy with increased in association with medications, when combined with nortriptyline and lithium and venlafaxine and lithium (125, 126). MST is a TMS variant with a more focused superficial field and minimal stimulation of inner brain structures such as hippocampus (127), thus having lower incidences of cognitive deficits unlike ECT (128) but with antidepressant effect similar to RUL ECT (129, 130). Animal models reveal a better understanding of the antidepressant mechanism of neuromodulatory techniques such as deep brain stimulation (DBS), which is observed to work through activating brain derived neurotrophic factor (BDNF) related pathways. DBS targeting the ventral medial prefrontal cortex is thought to exert its antidepressant-like and cognitive enhancement effect by increasing expression of BDNF, Akt, and mammalian target of rapamycin (mTOR), and observed to restore stress-induced synaptic loss in the hippocampus (131).
While abnormal projection of excitatory glutamate neurons in the brain regions could contribute to structural alteration, emotional stress and depression alter GABAergic function that impairs the fine-tune and control glutamate related excitatory function leading to dysfunction in these neural circuits (132, 133). Brain imaging studies have shown structural and functional alterations in depression, particularly the decreased volume of the hippocampus and the subgenual and anterior cingulate cortex of the prefrontal cortex (134, 135). Functional brain imaging shows dysfunction in three major networks related to depression, including default mode network (DMN) responsible for resting taste introspection and ruminations, the salience network (SAL) processing salient information from external sources and the central network (CEN) responsible for working memory and attention (136). Unlike the monoamine system with the main role of providing extrinsic inputs to the cerebral cortex, Glutamate provides both excitatory and inhibitory control of information flow in the brain (132, 137, 138).
The alteration of glutamate in CSF, blood and brain tissue related to synthesis, metabolism and reuptake into neuronal or glial cells is linked to decreased glutamate in the subgenual of anterior cingulate cortex associated with reduced connectivity with insula and decreased BOLD response to emotional stimuli in MDD patients (139). Alteration of the glutamatergic pathway could be linked to acute and chronic stress affecting specific circuits relevant to increased or decreased connectivity observed in individuals with MDD. Indeed, chronic stress is thought to cause atrophy of glutamate neurons contributing in volume reduction in cortical and limbic structures implicated in depression (63, 140). The alteration of glutamatergic neurons secondary to stress could also be attributed to neuroendocrine mechanism related to HPA and elevation of adrenal glucocorticoids which are implicated in the etiopathogenesis of depression. While administration of glucocorticoids may lead to dendritic atrophy and reduction in number of synapsis in the hippocampus and PFC, survival of neurons may be enhanced by downstream regulation of glucocorticoids by major neurotrophic factors such as (BDNF) which plays a role in regulation and function of neurons (141–145).
Conclusion
Non-invasive brain stimulation offers a potential alternative, non-pharmacological approach to treat unipolar depression and TRD. While pharmacological techniques have targeted the glutamatergic receptors as a therapeutic target for treating depressive disorders, neuromodulation techniques offer alternative or augmentative treatment for the same. Non-invasive brain stimulation techniques including the non-convulsive TMS and tDCS and convulsive ECT and MST provide safe options targeting specific areas in DLPFC which is a key site for the frontoparietal network (FPN). The rationale for neuromodulation for depression is based on a neural network theory that posits a specific set of structurally and functionally connected brain regions that work together to maintain normal mood regulation. However, little is known about the specific mechanisms and further research is needed to investigate the considerable potential of ionotropic and metabotropic receptors in relation to multi-target treatment of depressive disorders.
Author Contributions
ME-G and KP-B conceptualized the idea. MK coordinated research activity planning and execution. GC-K acquired funding and worked on references. MK and SO reviewed and edited the manuscript. All authors approved the final version of the manuscript. All authors participated in writing the first draft of the manuscript.
Funding
The publication of this article was funded by Children's Hospital of Mexico Federico Gomez.
Conflict of Interest
The authors declare that the research was conducted in the absence of any commercial or financial relationships that could be construed as a potential conflict of interest.
Publisher's Note
All claims expressed in this article are solely those of the authors and do not necessarily represent those of their affiliated organizations, or those of the publisher, the editors and the reviewers. Any product that may be evaluated in this article, or claim that may be made by its manufacturer, is not guaranteed or endorsed by the publisher.
Acknowledgments
We would like to thank Dr. Mustafa Nissar Bankur from the Neurosciences Department, King's College Hospital, for language and editing support.
References
1. Gaynes BN, Lux L, Gartlehner G, Asher G, Forman-Hoffman V, Green J, et al. Defining treatment-resistant depression. Depression and Anxiety. (2020) 37:134–45. doi: 10.1002/da.22968
2. Murphy JA, Sarris J, Byrne GJ. A review of the conceptualisation and risk factors associated with treatment-resistant depression. Depression Research and Treatment. (2017) 2017:4176825. doi: 10.1155/2017/4176825
3. Singh MK, Gotlib IH. The neuroscience of depression: Implications for assessment and intervention. Behav Res Ther. (2014) 62:60–73. doi: 10.1016/j.brat.2014.08.008
4. Mayberg HS, Lozano AM, Voon V, McNeely HE, Seminowicz D, Hamani C, et al. Deep brain stimulation for treatment-resistant depression. Neuron. (2005) 45:651–60 doi: 10.1016/j.neuron.2005.02.014
5. Duman RS, Sanacora G, Krystal JH. Altered connectivity in depression: GABA and glutamate neurotransmitter deficits and reversal by novel treatments. Neuron. (2019) 102:75–90. doi: 10.1016/j.neuron.2019.03.013
6. Paul IA, Skolnick P. Glutamate and depression: clinical and preclinical studies. Ann N Y Acad Sci. (2003) 1003:250–72 doi: 10.1196/annals.1300.016
7. Kugaya A, Sanacora G. Beyond monoamines: glutamatergic function in mood disorders. CNS Spectr. (2005) 10:808–19. doi: 10.1017/S1092852900010403
8. Yildiz-Yesiloglu A, Ankerst DP. Review of 1H magnetic resonance spectroscopy findings in major depressive disorder: a meta-analysis. Psychiatry Res Neuroimaging. (2006) 147:1–25. doi: 10.1016/j.pscychresns.2005.12.004
9. Millan MJ. Multi-target strategies for the improved treatment of depressive states: conceptual foundations and neuronal substrates, drug discovery and therapeutic application. Pharmacol Ther. (2006) 110:135–370. doi: 10.1016/j.pharmthera.2005.11.006
10. Kadriu B, Musazzi L, Henter ID, Graves M, Popoli M, Zarate CA. Glutamatergic neurotransmission: pathway to developing novel rapid-acting antidepressant treatments. Int J Neuropsychopharmacol Oxford Acad. (2019) 22:119–35. doi: 10.1093/ijnp/pyy094
11. Kew JNC, Kemp JA. Ionotropic and metabotropic glutamate receptor structure and pharmacology. Psychopharmacology. (2005) 179:4–29. doi: 10.1007/s00213-005-2200-z
12. Skolnick P. Antidepressants for the new millennium. Eur J Pharmacol. (1999) 375:31–40. doi: 10.1016/S0014-2999(99)00330-1
13. Skolnick P, Legutko B, Li X, Bymaster FP. Current perspectives on the development of non-biogenic amine-based antidepressants. Pharmacol Res. (2001) 43:411–22. doi: 10.1006/phrs.2000.0806
14. CRANE GE. Cyloserine as an antidepressant agent. Am J Psychiatry. (1959) 115:1025–6. doi: 10.1176/ajp.115.11.1025
15. Crane GE. The psychotropic effects of cycloserine: a new use for an antibiotic. Compr Psychiatry. (1961) 2:51–9. doi: 10.1016/S0010-440X(61)80007-2
16. Zarate CA, Singh JB, Quiroz JA, De Jesus G, Denicoff KK, Luckenbaugh DA, et al. A double-blind, placebo-controlled study of memantine in the treatment of major depression. Am J Psychiatry. (2006) 163:153–5. doi: 10.1176/appi.ajp.163.1.153
17. Zarate CA, Singh JB, Carlson PJ, Brutsche NE, Ameli R, Luckenbaugh DA, et al. A randomized trial of an N-methyl-D-aspartate antagonist in treatment-resistant major depression. Arch Gen Psychiatry. (2006) 63:856–64. doi: 10.1001/archpsyc.63.8.856
18. Marenco S, Weinberger DR. Therapeutic potential of positive AMPA receptor modulators in the treatment of neuropsychiatric disorders. CNS Drugs. (2006) 20:173–85. doi: 10.2165/00023210-200620030-00001
19. Maeng S, Zarate CA, Du J, Schloesser RJ, McCammon J, Chen G, et al. Cellular mechanisms underlying the antidepressant effects of ketamine: role of α-amino-3-hydroxy-5-methylisoxazole-4-propionic acid receptors. Biol Psychiatry. (2008) 63:349–52. doi: 10.1016/j.biopsych.2007.05.028
20. Nations KR, Dogterom P, Bursi R, Schipper J, Greenwald S, Zraket D, et al. Examination of Org 26576, an AMPA receptor positive allosteric modulator, in patients diagnosed with major depressive disorder: an exploratory, randomized, double-blind, placebo-controlled trial. J Psychopharmacol. (2012) 26:1525–39. doi: 10.1177/0269881112458728
21. Li X, Witkin JM, Need AB, Skolnick P. Enhancement of antidepressant potency by a potentiator of AMPA receptors. Cell Mol Neurobiol. (2003) 23:419–30. doi: 10.1023/A:1023648923447
22. Rush AJ, Trivedi MH, Wisniewski SR, Nierenberg AA, Stewart JW, Warden D, et al. Acute and longer-term outcomes in depressed outpatients requiring one or several treatment steps: A STAR*D report. Am J Psychiatry. (2006) 163:1905–17. doi: 10.1176/ajp.2006.163.11.1905
23. Kavalali ET, Monteggia LM. Synaptic mechanisms underlying rapid antidepressant action of ketamine. Am J Psychiatry. (2012) 169:1150–6. doi: 10.1176/appi.ajp.2012.12040531
24. Kim J, Farchione T, Potter A, Chen Q, Temple R. Esketamine for treatment-resistant depression — first FDA-approved antidepressant in a new class. N Engl J Med. (2019) 381:1–4. doi: 10.1056/NEJMp1903305
25. Janssen Inc. Product Monograph Including Patient Medication Information Nspravato® Esketamine Nasal Spray Solution, 28 mg esketamine (as esketamine hydrochloride), nasal Antidepressant ATC Code: N06AX27 (2020).
26. Zanos P, Moaddel R, Morris PJ, Riggs LM, Highland JN, Georgiou P, et al. Ketamine and ketamine metabolite pharmacology: Insights into therapeutic mechanisms. Pharmacol Rev. (2018) 70:621–60. doi: 10.1124/pr.117.015198
27. Zanos P, Gould TD. Mechanisms of ketamine action as an antidepressant. Mol Psychiatry. (2018) 23:801–11. doi: 10.1038/mp.2017.255
28. Huang CC, Wei IH, Huang CL, Chen KT, Tsai MH, Tsai P, et al. Inhibition of glycine transporter-I as a novel mechanism for the treatment of depression. Biol Psychiatry. (2013) 74:734–41. doi: 10.1016/j.biopsych.2013.02.020
29. Chen KT, Wu CH, Tsai MH, Wu YC, Jou MJ, Huang CC, et al. Antidepressant-like effects of long-term sarcosine treatment in rats with or without chronic unpredictable stress. Behav Brain Res. (2017) 316:1–10. doi: 10.1016/j.bbr.2016.06.004
30. Lai CH, Lane HY, Tsai GE. Clinical and cerebral volumetric effects of sodium benzoate, a d-amino acid oxidase inhibitor, in a drug-nave patient with major depression. Biol Psychiatry. (2012) 71:e9–10. doi: 10.1016/j.biopsych.2011.10.034
31. Lin C-H, Wang S-H, Lane H-Y. Effects of sodium benzoate, a D-amino acid oxidase inhibitor, on perceived stress and cognitive function among patients with late-life depression: a randomized, double-blind, sertraline- and placebo-controlled trial. Int J Neuropsychopharmacol. (2022) 1–11. doi: 10.1093/ijnp/pyac006
32. Pin JP, Duvoisin R. The metabotropic glutamate receptors: structure and functions. Neuropharmacol Pergamon. (1995) 34:1–26. doi: 10.1016/0028-3908(94)00129-G
33. Lea IV PM, Faden AI. Modulation of metabotropic glutamate receptors as potential treatment for acute and chronic neurodegenerative disorders. Drug News and Perspectives. (2003) 16:513–22. doi: 10.1358/dnp.2003.16.8.829350
34. Rodrigues SM, Bauer EP, Farb CR, Schafe GE, LeDoux JE. The Group I metabotropic glutamate receptor mGluR5 is required for fear memory formation and long-term potentiation in the lateral amygdala. J Neurosci. (2002) 22:5219–29. doi: 10.1523/JNEUROSCI.22-12-05219.2002
35. Peterlik DJ, Flor P, Uschold-Schmidt N. The emerging role of metabotropic glutamate receptors in the pathophysiology of chronic stress-related disorders. Curr Neuropharmacol. (2015) 14:514–39. doi: 10.2174/1570159X13666150515234920
36. Pin JP, Acher F. The metabotropic glutamate receptors: structure, activation mechanism and pharmacology. Curr Drug Targets CNS Neurol Disord. (2002) 1:297–317. doi: 10.2174/1568007023339328
37. Cartmell J, Schoepp DD. Regulation of neurotransmitter release by metabotropic glutamate receptors. J Neurochem. (2000) 75:889–907. doi: 10.1046/j.1471-4159.2000.0750889.x
38. Schoepp DD. Unveiling the functions of presynaptic metabotropic glutamate receptors in the central nervous system. J Pharmacol Exp Therap. (2001) 299:12–20.
39. Kawashima N, Karasawa JI, Shimazaki T, Chaki S, Okuyama S, Yasuhara A, et al. Neuropharmacological profiles of antagonists of group II metabotropic glutamate receptors. Neurosci Lett. (2005) 378:131–4. doi: 10.1016/j.neulet.2004.12.021
40. Matrisciano F, Scaccianoce S, Del Bianco P, Panaccione I, Canudas AM, Battaglia G, et al. Metabotropic glutamate receptors and neuroadaptation to antidepressants: Imipramine-induced down-regulation of β-adrenergic receptors in mice treated with metabotropic glutamate 2/3 receptor ligands. J Neurochem. (2005) 93:1345–52. doi: 10.1111/j.1471-4159.2005.03141.x
41. Michelsen KA, Prickaerts J, Steinbusch HWM. The dorsal raphe nucleus and serotonin: implications for neuroplasticity linked to major depression and Alzheimer's disease. Progr Brain Res Elsevier. (2008) 172:233–64. doi: 10.1016/S0079-6123(08)00912-6
42. Pałucha A, Tatarczyńska E, Brański P, Szewczyk B, Wierońska JM, Kłak K, et al. Group III mGlu receptor agonists produce anxiolytic- and antidepressant-like effects after central administration in rats. Neuropharmacology. (2004) 46:151–9. doi: 10.1016/j.neuropharm.2003.09.006
43. Tatarczyńska E, Pałucha A, Szewczyk B, Chojnacka-Wójcik E, Wierońska J, Pilc A. Anxiolytic- and antidepressant-like effects of group III metabotropic glutamate agonist (1S,3R,4S)-1-aminocyclopentane-1,3,4-tricarboxylic acid (ACPT-I) in rats. Pol J Pharmacol. (2002) 54:707–10.
44. Kim JH, Marton J, Ametamey SM, Cumming P. A review of molecular imaging of glutamate receptors. Molecules. (2020) 25:4749. doi: 10.3390/molecules25204749
45. Majo VJ, Prabhakaran J, Mann JJ, Kumar JSD. PET and SPECT tracers for glutamate receptors. Drug Discov Today. (2013) 18:173–84. doi: 10.1016/j.drudis.2012.10.004
46. Hasler G, Northoff G. Discovering imaging endophenotypes for major depression. Mol Psychiatry. (2011) 16:604–19. doi: 10.1038/mp.2011.23
47. McCluskey SP, Plisson C, Rabiner EA, Howes O. Advances in CNS PET: the state-of-the-art for new imaging targets for pathophysiology and drug development. Eur J Nucl Med Mol Imaging. (2020) 47:451–89. doi: 10.1007/s00259-019-04488-0
48. Mecca AP, Rogers K, Jacobs Z, McDonald JW, Michalak HR, DellaGioia N, et al. Effect of age on brain metabotropic glutamate receptor subtype 5 measured with (18F)FPEB PET. Neuroimage. (2021) 238:118217. doi: 10.1016/j.neuroimage.2021.118217
49. Bertholdo D, Watcharakorn A, Castillo M. Brain Proton Magnetic Resonance Spectroscopy: Introduction and Overview. Neuroimaging Clin N Am. (2013) 23:359–80. doi: 10.1016/j.nic.2012.10.002
50. Erchinger VJ, Ersland L, Aukland SM, Abbott CC, Oltedal L. Magnetic resonance spectroscopy in depressed subjects treated with electroconvulsive therapy—a systematic review of literature. Front Psychiatry. (2021) 12:12. doi: 10.3389/fpsyt.2021.608857
51. Godlewska BR, Near J, Cowen PJ. Neurochemistry of major depression: a study using magnetic resonance spectroscopy. Psychopharmacology (Berl). (2015) 232:501–7. doi: 10.1007/s00213-014-3687-y
52. Roddy DW, Kelly JR, Drago T, Raajakesary K, Haines M, O'Hanlon E. Neurobiochemistry alterations associated with major depression: a review of translational magnetic resonance spectroscopic studies. Transl Res Methods Major Depress Disord. (2022) 2022:265–309. doi: 10.1007/978-1-0716-2083-0_13
53. Pacheco-Barrios K, Pinto CB, Saleh Velez FG, Duarte D, Gunduz ME, Simis M, et al. Structural and functional motor cortex asymmetry in unilateral lower limb amputation with phantom limb pain. Clin Neurophysiol. (2020) 131:2375–82. doi: 10.1016/j.clinph.2020.06.024
54. Gunduz ME, Pinto CB, Velez FGS, Duarte D, Pacheco-Barrios K, Lopes F, et al. Motor cortex reorganization in limb amputation: a systematic review of TMS motor mapping studies. Front Neurosci. (2020) 14:314. doi: 10.3389/fnins.2020.00314
55. Ziemann U, Lönnecker S, Steinhoff BJ, Paulus W. The effect of lorazepam on the motor cortical excitability in man. Exp Brain Res. (1996) 109:127–35. doi: 10.1007/BF00228633
56. Ziemann U, Lönnecker S, Paulus W. Inhibition of human motor cortex by ethanol a transcranial magnetic stimulation study. Brain. (1995) 118:1437–46. doi: 10.1093/brain/118.6.1437
57. Ziemann U, Chen R, Cohen LG, Hallett M. Dextromethorphan decreases the excitability of the human motor cortex. Neurology. (1998) 51:1320–4. doi: 10.1212/WNL.51.5.1320
58. Schwenkreis P, Witscher K, Janssen F, Addo A, Dertwinkel R, Zenz M, et al. Influence of the N-methyl-D-aspartate antagonist memantine on human motor cortex excitability. Neurosci Lett. (1999) 270:137–40. doi: 10.1016/S0304-3940(99)00492-9
59. Veronezi BP Moffa AH Carvalho AF Galhardoni R Simis M Benseñor IM . Evidence for increased motor cortical facilitation and decreased inhibition in atypical depression. Acta Psychiatr Scand. (2016) 134:172–82. doi: 10.1111/acps.12565
60. Croarkin PE, Nakonezny PA, Husain MM, Melton T, Buyukdura JS, Kennard BD, et al. Evidence for increased glutamatergic cortical facilitation in children and adolescents with major depressive disorder. JAMA Psychiatry. (2013) 70:291–9. doi: 10.1001/2013.jamapsychiatry.24
61. Bajbouj M, Lisanby SH, Lang UE, Danker-Hopfe H, Heuser I, Neu P. Evidence for impaired cortical inhibition in patients with unipolar major depression. Biol Psychiatry. (2006) 59:395–400. doi: 10.1016/j.biopsych.2005.07.036
62. International Neuromodulation Society. Welcome to the International Neuromodulation Society (2016).
63. Duman RS, Aghajanian GK, Sanacora G, Krystal JH. Synaptic plasticity and depression: New insights from stress and rapid-acting antidepressants. Nat Med. (2016) 22:238–49. doi: 10.1038/nm.4050
64. Player MJ, Taylor JL, Weickert CS, Alonzo A, Sachdev P, Martin D, et al. Neuroplasticity in depressed individuals compared with healthy controls. Neuropsychopharmacology. (2013) 38:2101–8. doi: 10.1038/npp.2013.126
65. Fossati P, Radtchenko A, Boyer P. Neuroplasticity: From MRI to depressive symptoms. Eur Neuropsychopharmacol. (2004):S503–10. doi: 10.1016/j.euroneuro.2004.09.001
66. Pittenger C, Duman RS. Stress, depression, and neuroplasticity: a convergence of mechanisms. Neuropsychopharmacology. (2008) 33:88–109. doi: 10.1038/sj.npp.1301574
67. Conti B, Maier R, Barr AM, Morale MC, Lu X, Sanna PP, et al. Region-specific transcriptional changes following the three antidepressant treatments electro convulsive therapy, sleep deprivation and fluoxetine. Mol Psychiatry. (2007) 12:167–89. doi: 10.1038/sj.mp.4001897
68. Abbott CC, Jones T, Lemke NT, Gallegos P, McClintock SM, Mayer AR, et al. Hippocampal structural and functional changes associated with electroconvulsive therapy response. Transl Psychiatry. (2014) 4:e483–e483. doi: 10.1038/tp.2014.124
69. Joshi SH, Espinoza RT, Pirnia T, Shi J, Wang Y, Ayers B, et al. Structural plasticity of the hippocampus and amygdala induced by electroconvulsive therapy in major depression. Biol Psychiatry. (2016) 79:282–92. doi: 10.1016/j.biopsych.2015.02.029
70. Nordanskog P, Dahlstrand U, Larsson MR, Larsson EM, Knutsson L, Johanson A. Increase in hippocampal volume after electroconvulsive therapy in patients with depression: a volumetric magnetic resonance imaging study. J ECT. (2010) 26:62–7. doi: 10.1097/YCT.0b013e3181a95da8
71. Sartorius A, Demirakca T, Böhringer A, Clemm von Hohenberg C, Aksay SS, Bumb JM, et al. Electroconvulsive therapy increases temporal gray matter volume and cortical thickness. Eur Neuropsychopharmacol. (2016) 26:506–17. doi: 10.1016/j.euroneuro.2015.12.036
72. Tendolkar I, van Beek M, van Oostrom I, Mulder M, Janzing J, Voshaar RO, et al. Electroconvulsive therapy increases hippocampal and amygdala volume in therapy refractory depression: a longitudinal pilot study. Psychiatry Res Neuroimaging. (2013) 214:197–203. doi: 10.1016/j.pscychresns.2013.09.004
73. Lyden H, Espinoza RT, Pirnia T, Clark K, Joshi SH, Leaver AM, et al. Electroconvulsive therapy mediates neuroplasticity of white matter microstructure in major depression. Transl Psychiatry. (2014) 4:e380–e380. doi: 10.1038/tp.2014.21
74. Fidalgo TM, Morales-Quezada JL, Muzy GSC, Chiavetta NM, Mendonca ME, Santana MVB, et al. Biological markers in noninvasive brain stimulation trials in major depressive disorder: a systematic review. Journal of ECT. (2014) 30:47–61. doi: 10.1097/YCT.0b013e31828b34d8
75. Liston C, Chen AC, Zebley BD, Drysdale AT, Gordon R, Leuchter B, et al. Default mode network mechanisms of transcranial magnetic stimulation in depression. Biol Psychiatry. (2014) 76:517–26. doi: 10.1016/j.biopsych.2014.01.023
76. Zhang R, Lam CLM, Peng X, Zhang D, Zhang C, Huang R, et al. Efficacy and acceptability of transcranial direct current stimulation for treating depression: a meta-analysis of randomized controlled trials. Neurosci Biobehav Rev. (2021)126:481–90. doi: 10.1016/j.neubiorev.2021.03.026
77. Moreno-Duarte I, Gebodh N, Schestatsky P, Guleyupoglu B, Reato D, Bikson M, et al. Transcranial electrical stimulation: Transcranial Direct Current Stimulation (tDCS), Transcranial Alternating Current Stimulation (tACS), Transcranial Pulsed Current Stimulation (tPCS), and Transcranial Random Noise Stimulation (tRNS). The Stimulated Brain: Cognitive Enhancement Using Non-Invasive Brain Stimulation. (2014) 35–59. doi: 10.1016/B978-0-12-404704-4.00002-8
78. Alexander ML, Alagapan S, Lugo CE, Mellin JM, Lustenberger C, Rubinow DR, et al. Double-blind, randomized pilot clinical trial targeting alpha oscillations with transcranial alternating current stimulation (tACS) for the treatment of major depressive disorder (MDD). Transl Psychiatry. (2019) 9:106. doi: 10.1038/s41398-019-0439-0
79. Wilkening A, Kurzeck A, Dechantsreiter E, Padberg F, Palm U. Transcranial alternating current stimulation for the treatment of major depression during pregnancy. Psychiatry Res. (2019) 279:399–400. doi: 10.1016/j.psychres.2019.06.009
80. Riddle J, Rubinow DR, Frohlich F. A case study of weekly tACS for the treatment of major depressive disorder. Brain Stimul. (2020) 13:576–7. doi: 10.1016/j.brs.2019.12.016
81. Legates TA, Fernandez DC, Hattar S. Light as a central modulator of circadian rhythms, sleep and affect. Nature Reviews Neurosci. (2014) 15:443–54. doi: 10.1038/nrn3743
82. Fu Y, Liao HW, Do MTH, Yau KW. Non-image-forming ocular photoreception in vertebrates. Curr Opin Neurobiol. (2005) 15:415–22. doi: 10.1016/j.conb.2005.06.011
83. Eells JT, Henry MM, Summerfelt P, Wong-Riley MTT, Buchmann E V, Kane M, et al. Therapeutic photobiomodulation for methanol-induced retinal toxicity. Proc Natl Acad Sci USA. (2003) 100:3439–44. doi: 10.1073/pnas.0534746100
84. Rojas JC, Lee J, John JM, Gonzalez-Lima F. Neuroprotective effects of near-infrared light in an in vivo model of mitochondrial optic neuropathy. J Neurosci. (2008) 28:13511–21. doi: 10.1523/JNEUROSCI.3457-08.2008
85. De Freitas LF, Hamblin MR. Proposed mechanisms of photobiomodulation or low-level light therapy. IEEE J Sel Top Quantum Electron. (2016) 22:348–64. doi: 10.1109/JSTQE.2016.2561201
86. Naeser MA, Zafonte R, Krengel MH, Martin PI, Frazier J, Hamblin MR, et al. Significant improvements in cognitive performance post-transcranial, red/near-infrared light-emitting diode treatments in chronic, mild traumatic brain Injury: Open-protocol study. J Neurotrauma. (2014) 31:1008–17. doi: 10.1089/neu.2013.3244
87. Schiffer F, Johnston AL, Ravichandran C, Polcari A, Teicher MH, Webb RH, et al. Psychological benefits 2 and 4 weeks after a single treatment with near infrared light to the forehead: a pilot study of 10 patients with major depression and anxiety. Behav Brain Funct. (2009) 5:46. doi: 10.1186/1744-9081-5-46
88. Zhang D, Shen Q, Wu X, Xing D. Photobiomodulation therapy ameliorates glutamatergic dysfunction in mice with chronic unpredictable mild stress-induced depression. Oxid Med Cell Longev. (2021) 2021:6678276. doi: 10.1155/2021/6678276
89. Rush AJ, Marangell LB, Sackeim HA, George MS, Brannan SK, Davis SM, et al. Vagus nerve stimulation for treatment-resistant depression: a randomized, controlled acute phase trial. Biol Psychiatry. (2005) 58:347–54. doi: 10.1016/j.biopsych.2005.05.025
90. Rush AJ, Sackeim HA, Marangell LB, George MS, Brannan SK, Davis SM, et al. Effects of 12 months of vagus nerve stimulation in treatment-resistant depression: a naturalistic study. Biol Psychiatry. (2005) 58:355–63. doi: 10.1016/j.biopsych.2005.05.024
91. Li XJ, Wang L, Wang HX, Zhang L, Zhang GL, Rong PJ, et al. The effect of transcutaneous auricular vagus nerve stimulation on treatment-resistant depression monitored by resting-state fMRI and MRS: the first case report. Brain Stimul. (2019) 12:377–9. doi: 10.1016/j.brs.2018.11.013
92. Mutz J, Vipulananthan V, Carter B, Hurlemann R, Fu CHY, Young AH. Comparative efficacy and acceptability of non-surgical brain stimulation for the acute treatment of major depressive episodes in adults: systematic review and network meta-analysis. BMJ. (2019) 364:1079. doi: 10.1136/bmj.l1079
93. Mayberg HS. Modulating dysfunctional limbic-cortical circuits in depression: towards development of brain-based algorithms for diagnosis and optimised treatment. Br Med Bull. (2003) 65:193–207. doi: 10.1093/bmb/65.1.193
94. Drevets WC, Raichle ME. Neuroanatomical circuits in depression: Implications for treatment mechanisms. Psychopharmacol Bull. (1992). 28:261–74.
95. Müller-Dahlhaus F, Vlachos A. Unraveling the cellular and molecular mechanisms of repetitive magnetic stimulation. Front Mol Neurosci. (2013) 6:50. doi: 10.3389/fnmol.2013.00050
96. Fregni F, El-Hagrassy MM, Pacheco-Barrios K, Carvalho S, Leite J, Simis M, et al. Evidence-based guidelines and secondary meta-analysis for the use of transcranial direct current stimulation in neurological and psychiatric disorders. Int J Neuropsychopharmacol. (2021) 24:256–313. doi: 10.1093/ijnp/pyaa051
97. Yamada Y, Sumiyoshi T. Preclinical evidence for the mechanisms of transcranial direct current stimulation in the treatment of psychiatric disorders: a systematic review. Clin EEG Neurosci. (2021) 13:15500594211066151. doi: 10.1177/15500594211066151
98. Baeken C, Lefaucheur JP, Van Schuerbeek P. The impact of accelerated high frequency rTMS on brain neurochemicals in treatment-resistant depression: insights from 1H MR spectroscopy. Clin Neurophysiol. (2017) 128:1664–72. doi: 10.1016/j.clinph.2017.06.243
99. Dubin MJ, Mao X, Banerjee S, Goodman Z, Lapidus KAB, Kang G, et al. Elevated prefrontal cortex GABA in patients with major depressive disorder after TMS treatment measured with proton magnetic resonance spectroscopy. J Psychiatry Neurosci. (2016) 41:E37–45. doi: 10.1503/jpn.150223
100. Erbay MF, Zayman EP, Erbay LG, Ünal S. Evaluation of transcranial magnetic stimulation efficiency in major depressive disorder patients: a magnetic resonance spectroscopy study. Psychiatry Investig. (2019) 16:745–50. doi: 10.30773/pi.2019.07.17.3
101. Filmer HL, Ehrhardt SE, Bollmann S, Mattingley JB, Dux PE. Accounting for individual differences in the response to tDCS with baseline levels of neurochemical excitability. Cortex. (2019) 115:324–34. doi: 10.1016/j.cortex.2019.02.012
102. Mezger E, Rauchmann BS, Brunoni AR, Bulubas L, Thielscher A, Werle J, et al. Effects of bifrontal transcranial direct current stimulation on brain glutamate levels and resting state connectivity: multimodal MRI data for the cathodal stimulation site. Eur Arch Psychiatry Clin Neurosci. (2021) 271:111–22. doi: 10.1007/s00406-020-01177-0
103. Wright RA, Johnson BG, Zhang C, Salhoff C, Kingston AE, Calligaro DO, et al. CNS distribution of metabotropic glutamate 2 and 3 receptors: Transgenic mice and (3H)LY459477 autoradiography. Neuropharmacology. (2013) 66:89–98. doi: 10.1016/j.neuropharm.2012.01.019
104. Chaki S. mGlu2/3 Receptor antagonists as novel antidepressants. Trends Pharmacol Sci. (2017) 38:569–80. doi: 10.1016/j.tips.2017.03.008
105. Musazzi L. Targeting metabotropic glutamate receptors for rapid-acting antidepressant drug discovery. Expert Opin Drug Discov. (2021) 16:147–57. doi: 10.1080/17460441.2020.1822814
106. Li CT, Yang KC, Lin WC. Glutamatergic dysfunction and glutamatergic compounds for major psychiatric disorders: evidence from clinical neuroimaging studies. Front Psychiatry. (2019) 10.
107. Mcomish CE, Pavey G, Gibbons A, Hopper S, Udawela M, Scarr E, et al. Lower (3H)LY341495 binding to mGlu2/3 receptors in the anterior cingulate of subjects with major depressive disorder but not bipolar disorder or schizophrenia. J Affect Disord. (2016) 190:241–8. doi: 10.1016/j.jad.2015.10.004
108. Reis T, Laks J, Panizzutti R, Ferreira ST. Elevated glutamate and glutamine levels in the cerebrospinal fluid of patients with probable Alzheimer's disease and depression caroline madeira, charles vargas-lopes, I Carlos Otávio Brandão2,6 < . Front Psychiatry. (2018) 9:561. doi: 10.3389/fpsyt.2018.00561
109. Hashimoto K, Malchow B, Falkai P, Schmitt A. Glutamate modulators as potential therapeutic drugs in schizophrenia and affective disorders. Eur Arch Psychiatry Clin Neurosci. (2013) 263:367–77. doi: 10.1007/s00406-013-0399-y
110. Ledo JH, Azevedo EP, Beckman D, Ribeiro FC, Santos LE, Razolli DS, et al. Cross talk between brain innate immunity and serotonin signaling underlies depressive-like behavior induced by Alzheimer's amyloid-β oligomers in mice. J Neurosci. (2016) 36:12106–16. doi: 10.1523/JNEUROSCI.1269-16.2016
111. Ledo JH, Azevedo EP, Clarke JR, Ribeiro FC, Figueiredo CP, Foguel D, et al. Correction: Amyloid-β oligomers link depressive-like behavior and cognitive deficits in mice. Mol Psychiatry. (2021) 26:6100–1. doi: 10.1038/mp.2012.168
112. Inoshita M, Umehara H, Watanabe SY, Nakataki M, Kinoshita M, Tomioka Y, et al. Elevated peripheral blood glutamate levels in major depressive disorder. Neuropsychiatr Dis Treat. (2018) 14:945–53. doi: 10.2147/NDT.S159855
113. Kaiser RH, Andrews-Hanna JR, Wager TD, Pizzagalli DA. Large-scale network dysfunction in major depressive disorder: a meta-analysis of resting-state functional connectivity. JAMA Psychiatry. (2015) 72:603–11. doi: 10.1001/jamapsychiatry.2015.0071
114. Padmanabhan JL, Cooke D, Joutsa J, Siddiqi SH, Ferguson M, Darby RR, et al. A human depression circuit derived from focal brain lesions. Biol Psychiatry. (2019) 86:749–58. doi: 10.1016/j.biopsych.2019.07.023
115. Williams LM. Precision psychiatry: a neural circuit taxonomy for depression and anxiety. Lancet Psychiatry. (2016) 3:472–80. doi: 10.1016/S2215-0366(15)00579-9
116. Thielscher A, Antunes A, Saturnino GB. Field modeling for transcranial magnetic stimulation: A useful tool to understand the physiological effects of TMS? Annu Int Conf IEEE Eng Med Biol Soc. (2015) 2015:222–5. doi: 10.1109/EMBC.2015.7318340
117. McClintock SM, Reti IM, Carpenter LL, McDonald WM, Dubin M, Taylor SF, et al. Consensus recommendations for the clinical application of repetitive transcranial magnetic stimulation (rTMS) in the treatment of depression. J Clin Psychiatry. (2018) 79:35–48. doi: 10.4088/JCP.16cs10905
118. Lisanby SH, Husain MM, Rosenquist PB, Maixner D, Gutierrez R, Krystal A, et al. Daily left prefrontal repetitive transcranial magnetic stimulation in the acute treatment of major depression: clinical predictors of outcome in a multisite, randomized controlled clinical trial. Neuropsychopharmacology. (2009) 34:522–34. doi: 10.1038/npp.2008.118
119. O'Reardon JP, Solvason HB, Janicak PG, Sampson S, Isenberg KE, Nahas Z, et al. Efficacy and safety of transcranial magnetic stimulation in the acute treatment of major depression: a multisite randomized controlled trial. Biol Psychiatry. (2007) 62:1208–16. doi: 10.1016/j.biopsych.2007.01.018
120. Berlim MT, Van Den Eynde F, Daskalakis ZJ. High-frequency repetitive transcranial magnetic stimulation accelerates and enhances the clinical response to antidepressants in major depression: A meta-analysis of randomized, double-blind, and sham-controlled trials. J Clin Psychiatry. (2013) 74:e122–9. doi: 10.4088/JCP.12r07996
121. Berlim MT, Van Den Eynde F, Tovar-Perdomo S, Daskalakis ZJ. Response, remission and drop-out rates following high-frequency repetitive transcranial magnetic stimulation (rTMS) for treating major depression: a systematic review and meta-analysis of randomized, double-blind and sham-controlled trials. Psychol Med. (2014) 44:225–39. doi: 10.1017/S0033291713000512
122. Berlim MT, Van Den Eynde F, Jeff Daskalakis Z. Clinically meaningful efficacy and acceptability of low-frequency repetitive transcranial magnetic stimulation (rTMS) for treating primary major depression: a meta-analysis of randomized, double-blind and sham-controlled trials. Neuropsychopharmacology. (2013) 38:543–51. doi: 10.1038/npp.2012.237
123. Borrione L, Moffa AH, Martin D, Loo CK, Brunoni AR. Transcranial direct current stimulation in the acute depressive episode: a systematic review of current knowledge. J ECT. (2018) 34:153–63. doi: 10.1097/YCT.0000000000000512
124. Brunoni AR, Moffa AH, Sampaio-Junior B, Borrione L, Moreno ML, Fernandes RA, et al. Trial of electrical direct-current therapy versus escitalopram for depression. N Engl J Med. (2017) 376:2523–33. doi: 10.1056/NEJMoa1612999
125. Dunne RA, McLoughlin DM. Systematic review and meta-analysis of bifrontal electroconvulsive therapy versus bilateral and unilateral electroconvulsive therapy in depression. World J Biol Psychiatry. (2012) 13:248–58. doi: 10.3109/15622975.2011.615863
126. Prudic J, Haskett RF, McCall WV, Isenberg K, Cooper T, Rosenquist PB, et al. Pharmacological strategies in the prevention of relapse after electroconvulsive therapy. J ECT. (2013) 29:3–12. doi: 10.1097/YCT.0b013e31826ea8c4
127. Deng Z De, Lisanby SH, Peterchev A V. Electric field strength and focality in electroconvulsive therapy and magnetic seizure therapy: a finite element simulation study. J Neural Eng. (2011) 8:016007. doi: 10.1088/1741-2560/8/1/016007
128. Zhang XY, Chen H Di, Liang WN, Yang XH, Cai D Bin, Huang X, et al. Adjunctive magnetic seizure therapy for schizophrenia: a systematic review. Front Psychiatry. (2022) 12:813590. doi: 10.3389/fpsyt.2021.813590
129. Kloß M, Wagner S, Engel A, Kayser S. Magnetic seizure therapy in severe depression. Nervenheilkunde. (2015) 34:1012–5. doi: 10.1055/s-0038-1627661
130. Fitzgerald PB, Hoy KE, Elliot D, McQueen S, Wambeek LE, Chen L, et al. A pilot study of the comparative efficacy of 100 Hz magnetic seizure therapy and electroconvulsive therapy in persistent depression. Depress Anxiety. (2018) 35:393–401. doi: 10.1002/da.22715
131. Sun Z, Jia L, Shi D, He Y, Ren Y, Yang J, et al. Deep brain stimulation improved depressive-like behaviors and hippocampal synapse deficits by activating the BDNF/mTOR signaling pathway. Behav Brain Res. (2022) 419:113709. doi: 10.1016/j.bbr.2021.113709
132. Fee C, Banasr M, Sibille E. Somatostatin-positive gamma-aminobutyric acid interneuron deficits in depression: cortical microcircuit and therapeutic perspectives. Biol Psychiatry. (2017) 82:549–59. doi: 10.1016/j.biopsych.2017.05.024
133. Ghosal S, Hare BD, Duman RS. Prefrontal cortex GABAergic deficits and circuit dysfunction in the pathophysiology and treatment of chronic stress and depression. Curr Opin Behav Sci. (2017) 14:1–8. doi: 10.1016/j.cobeha.2016.09.012
134. Frodl T, Schüle C, Schmitt G, Born C, Baghai T, Zill P, et al. Association of the brain-derived neurotrophic factor Val66Met polymorphism with reduced hippocampal volumes in major depression. Arch Gen Psychiatry. (2007) 64:410–6. doi: 10.1001/archpsyc.64.4.410
135. Evans JW, Szczepanik J, Brutsché N, Park LT, Nugent AC, Zarate CA. Default mode connectivity in major depressive disorder measured up to 10 days after ketamine administration. Biol Psychiatry. (2018) 84:582–90. doi: 10.1016/j.biopsych.2018.01.027
136. Menon V. Large-scale brain networks and psychopathology: a unifying triple network model. Trends Cogn Sci. (2011) 15:483–506. doi: 10.1016/j.tics.2011.08.003
137. Abdallah CG, Jiang L, De Feyter HM, Fasula M, Krystal JH, Rothman DL, et al. Glutamate metabolism in major depressive disorder. Am J Psychiatry. (2014) 171:1320–7. doi: 10.1176/appi.ajp.2014.14010067
138. Sanacora G, Treccani G, Popoli M. Towards a glutamate hypothesis of depression: an emerging frontier of neuropsychopharmacology for mood disorders. Neuropharmacology. (2012) 62:63–77. doi: 10.1016/j.neuropharm.2011.07.036
139. Lener MS, Niciu MJ, Ballard ED, Park M, Park LT, Nugent AC, et al. Glutamate and gamma-aminobutyric acid systems in the pathophysiology of major depression and antidepressant response to ketamine. Biol Psychiatry. (2017) 81:886–97. doi: 10.1016/j.biopsych.2016.05.005
140. McEwen BS, Bowles NP, Gray JD, Hill MN, Hunter RG, Karatsoreos IN, et al. Mechanisms of stress in the brain. Nat Neurosci. (2015) 18:1353–63. doi: 10.1038/nn.4086
141. Marrocco J, McEwen BS. Sex in the brain: Hormones and sex differences. Dialogues Clin Neurosci. (2016) 18:373–83. doi: 10.31887/DCNS.2016.18.4/jmarrocco
142. McEwen BS, Nasca C, Gray JD. Stress Effects on Neuronal Structure: Hippocampus, Amygdala, and Prefrontal Cortex. Neuropsychopharmacology. (2016) 41:3–23. doi: 10.1038/npp.2015.171
143. Hedrick NG, Harward SC, Hall CE, Murakoshi H, McNamara JO, Yasuda R. Rho GTPase complementation underlies BDNF-dependent homo- and heterosynaptic plasticity. Nature. (2016) 538:104–8. doi: 10.1038/nature19784
144. Martinowich K, Manji H, Lu B. New insights into BDNF function in depression and anxiety. Nat Neurosci. (2007) 10:1089–93. doi: 10.1038/nn1971
Keywords: glutamate, neuromodulation, TMS (repetitive transcranial magnetic stimulation), direct current stimulation (tDCS), photo-biomodulation therapy, treatment-resistant depression, depression, mini review
Citation: Khoodoruth MAS, Estudillo-Guerra MA, Pacheco-Barrios K, Nyundo A, Chapa-Koloffon G and Ouanes S (2022) Glutamatergic System in Depression and Its Role in Neuromodulatory Techniques Optimization. Front. Psychiatry 13:886918. doi: 10.3389/fpsyt.2022.886918
Received: 01 March 2022; Accepted: 28 March 2022;
Published: 14 April 2022.
Edited by:
Hsien-Yuan Lane, China Medical University, TaiwanReviewed by:
Tomiki Sumiyoshi, National Center of Neurology and Psychiatry, JapanChieh-Hsin Lin, Kaohsiung Chang Gung Memorial Hospital, Taiwan
Kenji Hashimoto, Chiba University, Japan
Copyright © 2022 Khoodoruth, Estudillo-Guerra, Pacheco-Barrios, Nyundo, Chapa-Koloffon and Ouanes. This is an open-access article distributed under the terms of the Creative Commons Attribution License (CC BY). The use, distribution or reproduction in other forums is permitted, provided the original author(s) and the copyright owner(s) are credited and that the original publication in this journal is cited, in accordance with accepted academic practice. No use, distribution or reproduction is permitted which does not comply with these terms.
*Correspondence: Gina Chapa-Koloffon, Z2luYWNoYXBha0BnbWFpbC5jb20=
†These authors have contributed equally to this work and share first authorship