Standardized metrics to quantify solar energy-land relationships: A global systematic review
- 1Department of Land, Air and Water Resources, University of California, Davis, Davis, CA, United States
- 2Wild Energy Center, Institute of the Environment and the Energy and Efficiency Institute, University of California, Davis, Davis, CA, United States
- 3Lancaster Environment Centre, Lancaster University, Lancaster, United Kingdom
- 4Energy Lancaster, Lancaster University, Lancaster, United Kingdom
- 5Department of Civil Engineering, McGill University, Montreal, QC, Canada
Ground-mounted solar energy installations, including photovoltaics (PV) and concentrating solar power (CSP), can have significant environmental, ecological, and sociocultural effects via land-use and land-cover change (LULCC). Research in disciplines ranging from engineering to environmental policy seeks to quantify solar energy-land (SE-land) interactions to better understand the comprehensive impacts of solar energy installations on society. However, increasing evidence shows that scholars across research disciplines employ disparate metrics to quantify SE-land interactions. While solar energy deployment helps to achieve progress toward sustainable development goals (SDG 7- affordable and clean energy), the inconsistent use of metrics to describe SE-land interactions may inhibit the understanding of the total environmental and ecological impacts of solar energy installations, potentially causing barriers to achieve concurrent SDG's such as life on land (SDG 15). We systematically reviewed 608 sources on SE-land relationships globally to identify and assess the most frequent metric terms and units used in published studies. In total, we identified 51 unique metric terms and 34 different units of measure describing SE-land relationships across 18 countries of author origin. We organized these findings into three distinct metric categories: (1) capacity-based (i.e., nominal), (2) generation-based, and (3) human population-based. We used the most frequently reported terms and units in each category to inform a standardized suite of metrics, which are: land-use efficiency (W/m2), annual and lifetime land transformation (m2/Wh), and solar footprint (m2/capita). This framework can facilitate greater consistency in the reporting of SE-land metrics and improved capacity for comparison and aggregations of trends, including SE-land modeling projections. Our study addresses the need for standardization while acknowledging the role for future methodological advancements. The results of our study may help guide scholars toward a common vernacular and application of metrics to inform decisions about solar energy development.
Introduction
Rapid, global development of renewable energy, especially solar energy, is increasingly playing a pivotal role in mitigating climate change and meeting Sustainable Development Goal (SDG) 7 (the equitable access to affordable, reliable, sustainable, and modern energy) of the United Nations 2030 Agenda for Sustainable Development (UN General Assembly, 2015). While transitioning from fossil fuels to renewable energy is necessary to address climate change and adhere to international climate accords, solar energy can have impacts—both positive and negative—on the environment (Stoms et al., 2013; Hernandez et al., 2014a, 2019; Walston et al., 2018; Rabaia et al., 2021). To avoid negative environmental and ecological impacts and maximize beneficial outcomes, decision makers may need to proactively manage solar energy-land (SE-land) relationships to support progress toward other SDGs (e.g., SDG 15: Life on Land) that seeks to protect terrestrial ecosystems (UN General Assembly, 2015; Fuso Nerini et al., 2019; Hernandez et al., 2020b).
Globally, solar energy is expected to outpace new fossil fuel-based generation technologies and grow six-fold between 2018 and 2030 and sustain an 8.9% annual growth rate through 2050 (IRENA, 2019; IEA, 2021). Annually, 83 gigawatt (GW) of utility-scale photovoltaic (PV) solar energy (i.e., at least 1 MW) is expected to be installed between 2022 and 2025, mainly in China, the United States, and Europe (IEA, 2020). There are currently 5.2 GW of concentrating solar power (CSP) installed or under construction globally (Murphy et al., 2019), with an expected growth rate of 0.4 GW per annum between 2022 and 2025, occurring mainly in China, Chile, the United Arab Emirates, and Morocco (IEA, 2020).
Current methods used to quantify relationships between solar energy and land often are inconsistent (Horner and Clark, 2013), creating challenges for decision makers in project review and design phases and also larger climate change mitigation strategies, such as concurrently meeting SDGs (Horner and Clark, 2013; Fritsche et al., 2017). Inconsistent measurements of the impacted area of a solar energy installation may obstruct communication of land-use requirements for solar energy development in the published literature and in the dissemination of research results to policy makers. In contrast, the use of standardized metrics to describe SE-land relationships may facilitate greater certainty, consistency, and accuracy in the quantification of solar-energy impacts on the environment (Grubert et al., 2020).
A metric, defined broadly as a standard of measurement, in the context of SE-land interactions relates a fixed area of land to a unit of energy, power generation, or energy demand (Denholm and Margolis, 2008; Fthenakis and Kim, 2009). The metric may document either the total amount of land altered from a reference state, or the land occupied by solar energy-related infrastructure. Solar energy-land metrics may also embody the duration of occupation of the solar energy-related infrastructure (Fthenakis and Kim, 2009). Further, metrics relating land-use to energy generation (watt hours [Wh]) relative to metrics relating land-use to installed capacity (watt [W]) are not directly comparable without considering other attributes influencing system performance (Horner and Clark, 2013) (e.g., system capacity factor, efficiency, climatic factors). Thus, power-land interactions and energy-land interactions require distinct metrics to avoid confusion and misrepresentation of findings (Horner and Clark, 2013).
When a range of metrics are used in science to describe similar phenomena, it is common for scientists to call for standardization to improve knowledge transfer and coordinate interpretation of results (Fraser et al., 2013; Vetter et al., 2013; Provencher et al., 2017). Furthermore, standardizing metrics by topic area may also facilitate better documentation of metadata, which can prove useful for aggregating trends in proximate and related topics (Fraser et al., 2013). In fields experiencing rapid growth, like solar energy, new metrics ought to evolve in tandem with the progression of the research (e.g., see Cagle et al., 2020; Hoffacker and Hernandez, 2020); however, standardization remains an important consideration during this phase (DeFries et al., 2015). For example, in the field of land use and agriculture, (DeFries et al., 2015) proposed a new metric to address the nutrient yield of crops in land-scarce regions—a metric that quantifies how many adults can meet their annual dietary reference intake for one year per hectare of agricultural land (DeFries et al., 2015). Shortly after, (Gustafson et al., 2016) led a consensus report and workshop to develop a standardized set of food system metrics for sustainable nutrition security. Overall, these studies demonstrate how scholars have responded to the need for new metrics and standardization as new technologies or sub-disciplines emerge and evolve, respectively.
Researchers have also identified the need to standardize metrics because of too many metrics being used in a given field of study. Grubert et al. (2020) documented the inconsistent use of terms used to describe water quantity use in the literature and recommended a mass flow-based approach to overcoming conflicts related to definitions. Boyd and Banzhaf (2007) standardized environmental accounting units within an ecosystem services framework because a large number of accounting units used throughout the literature inhibited knowledge transfer in their field. To increase knowledge transfer and common modeling efforts, Vallance et al. (2017) proposed standardized metrics for solar energy forecasting. In this case, metric standardization enabled the harmonization of data across studies and provided a model for future research (Vallance et al., 2017).
If ground-mounted installations are prioritized over solar energy installations integrated with pre-existing infrastructure (such as rooftops), land required by solar expansion may provoke a fundamental shift in the spatiotemporal ways by which society interacts with energy development (Tsoutsos et al., 2005; Sullivan et al., 2012). Approximately 70% of all new solar PV installations between 2021 and 2025 are expected to be utility-scale, ground-mounted installations, mostly in China, the United States, and Europe (IEA, 2020). In the United States alone, it is estimated that over 800,000 km2 of additional land, larger than the state of Texas, will be required for growing energy demands and the associated increase in supply of renewables by 2040 (Trainor et al., 2016). Increasing data suggest that ground-mounted solar energy is a relatively land-intensive energy technology, specifically in terms of land transformation—the area of land necessary to generate a quantity of energy over a given period of time (Fthenakis and Kim, 2009; Lovering et al., 2022). For example, Lovering et al. (2022) calculated land transformation of real-world sites across all major sources of electricity and found that ground-mounted PV has an annual land transformation of 2,000 ha/TWh. For comparison, Lovering et al. (2022) found that the annual land transformation of ground-mounted PV is significantly greater than that of coal (1,000 ha/TWh). As such, increasing rates of solar energy development may create tensions between energy and land needed for agriculture, conservation, cultural and historical preservation, religious land rights, and urban development (Mulvaney, 2013; Elborg, 2015; Capellán-Pérez et al., 2017; Mancini and Nastasi, 2020).
In natural environments, solar energy has impacts on host ecosystems and their services that humans depend on (Tsoutsos et al., 2005; Armstrong et al., 2016; Moore-O'Leary et al., 2017; Grodsky et al., 2021). Several studies have found that ground-mounted solar energy, specifically PV, can impact air temperature, photosynthetically available radiation, rate of photosynthesis (Armstrong et al., 2016; Choi et al., 2020; Guoqing et al., 2021). Additionally, solar energy can impact plant diversity, abundance, and demography (Hernandez et al., 2020a; Tanner et al., 2020), and soil moisture creating unique microclimates under and around solar arrays (Tanner et al., 2020, 2021). For example, Grodsky and Hernandez (2020) found that development decisions for a 392 megawatt (MW) concentrating solar energy power plant in the Mojave Desert reduced cover and structure of desert perennial plants and their ecosystem services and facilitated the growth of invasive grass species. In addition to effects on local ecosystems within and near solar energy installations, the continued global deployment of solar energy technologies may increase habitat loss risks in wilderness areas globally (Rehbein et al., 2020). Changes in the land-use and land-cover (LULCC) of an area impacted by solar energy development can be a driver of habitat loss and fragmentation with knock-on effects on the movement and migration of wildlife (Houghton et al., 2012; Hernandez et al., 2015; van de Ven et al., 2021). Recent research has also demonstrated that LULCC can cause significant carbon emissions due to a shift in ecosystem services and biogeochemical cycling (De Marco et al., 2014; UN General Assembly, 2015; Baruch-Mordo et al., 2019).
Land-use decision makers, practitioners, and developers may benefit from a uniform and standardized understanding of the spatial consequences of solar energy development such that the land-sparing outcomes of non-conventional recipient environments, such as the built environment and contaminated lands are fully understood (Hoffacker et al., 2017). Additionally, the strategic colocation of solar energy with pre-existing land uses, such as agriculture and human-made water bodies, can add to the sustainability of solar energy by supporting additional ecosystem services (Hernandez et al., 2019; Cagle et al., 2020; Exley et al., 2021). Strategically engineering solar energy technologies to collocate with preexisting land uses and functions can increase the sustainability of a solar energy system and add benefits in addition to energy production (Hernandez et al., 2019). Additionally, to attain a comprehensive understanding of an energy generation technology's full life cycle impact, specific land-energy metrics must be employed by researchers (Jordaan et al., 2017). Greater metric specificity than simply land area impacted is necessary to understand the larger impact of energy generation on a given landscape (Dorning et al., 2019).
In the field of life cycle assessment (LCA), the terms land transformation and land occupation are increasingly documented as representing two distinct properties involved with alterations to a given area of land. For example, land transformation has been defined as the area of land modified to meet an anthropogenic use and does not always include a temporal scale associated with the impact. In contrast, land occupation, while also defined as the area of land modified for an anthropogenic purpose, commonly includes a temporal element (Koellner et al., 2013). In LCA, both terms are normalized over the output over the life of a facility, for example, the lifetime electricity generated for a solar project. Despite efforts to standardize these terms and their associated metrics, they have not been used consistently in describing SE-land interactions (Horner and Clark, 2013). While time is explicitly considered in LCA—with occupation metrics and the lifetime of the facility as a required input—the use and definition of the temporal aspect of land use impacts associated with solar energy are often tacit in similar fields of research when they should be explicit.
Useful scientific metrics enable practitioners to structure and use data functionally across fields of study and with the foundational capacity to inform science policy (Lane et al., 2014). To address the uncertainty of standardized metrics used to describe SE-land relationships, we performed a systematic literature review. Systematic literature reviews are increasingly used across environmental and ecological disciplines to increase the comparability of findings across studies and disseminate findings more consistently to a broader audience (Pullin and Stewart, 2006). In this study, we sought to (i) identify the metrics, including associated terms and units, used to describe SE-land relationships in the literature; (ii) determine the frequency of metric use across time, the geographic distribution of term use, and which research subject areas report SE-land metrics; (iii) identify distinct categories of SE-land metrics; and lastly, (iv) use these results to inform a set of standardized metrics to increase the overall quality of dissemination of SE-land interactions in future scientific publications and communications broadly. To the best of our knowledge, this is the first large-n assessment of metrics used for solar energy-land relationships.
Methods
We conducted a systematic literature review of peer-reviewed journal articles, published conference proceedings, book and book sections, and technical reports (hereafter “articles”) containing text and/or data on land-related properties of PV and CSP solar energy generation technologies. We conducted the literature search in Elsevier's title and abstract database, SCOPUS, on December 20, 2019 and the corpus search continued until March 15, 2020. We designed the literature search to include the entire SCOPUS corpus, which has material ranging from 1788-present and cited material ranging from 1970-present (Baas et al., 2020). Additionally, we evaluated article references and metric citations from articles identified in the systematic review for consideration in our corpus if they were in accordance with inclusion and exclusion criteria (see below). Among these additional articles, we specifically identified technical reports, books, and book chapters.
Criteria for inclusion in the study included the following: (i) all sources be in English, (ii) documents must document a relationship between solar energy and land area using a metric, and (iii) all sources selected describe solar energy ultimately for electricity production and not heat generation (e.g., no residential solar thermal water heaters). We defined a metric as a standard of measurement using a term and unit combination to describe the interaction between energy production and land use. Similarly, we defined unique metrics as a metric that varies in either metric term (e.g., land-use efficiency), unit (e.g., Wh), or term-unit combination from other identified metrics. We did not include volumetric power densities, such as W/m3 or btu/ft3, in this analysis because they refer more commonly to combustible fuel sources and are not applicable to the two-dimensional plane of SE-land relationships.
We searched both solar energy-related (e.g., solar PV, photovoltaics, and solar energy) and land-related terms (e.g., land-use intensity, land use, and land-use efficiency) simultaneously in a query sequence (Appendix A). As we screened articles for inclusion in the final corpus (first by reading the title and abstract, then the full-text), if a given article cited metrics from an article not identified in the initial SCOPUS review, the cited article was evaluated for inclusion within the study based on the same inclusion criteria. The systematic literature review in this study conforms to guidelines proposed by the PRISMA (Preferred Reporting Items for Systematic reviews and Meta-Analyses) statement. The PRISMA statement is a 27-item checklist coupled with a 4-phase flow diagram aimed at standardizing the methodology and reporting process of systematic reviews and meta-analyses (Figure 1) (Moher et al., 2009). Following the PRISMA flow diagram, we conducted an in-depth analysis of the search results generated via the SCOPUS database.
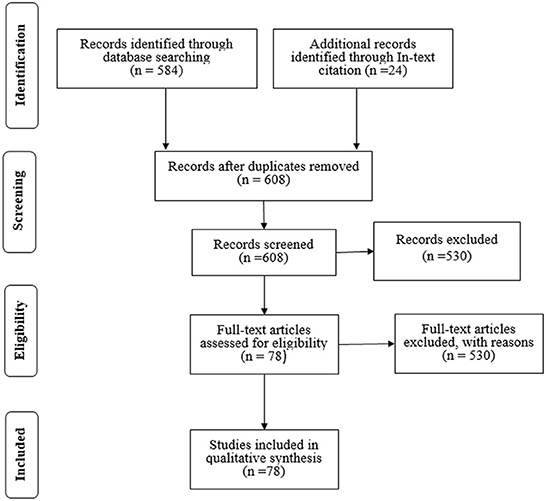
Figure 1. Flow diagram illustrating the PRISMA systematic review exclusion process and the number of sources identified, included, or excluded at each stage of the analysis (Moher et al., 2009).
Once we excluded all irrelevant articles, we compiled a database that included the following information for articles that met criteria for inclusion: publication year, metric(s) reported (term and unit), journal of article (or publishing body), country or countries of study origin, subject area as defined by SCOPUS, and whether articles were identified in the systematic search or cited information (Appendix B). We gathered information on the country of study origin by reviewing the listed locations of all the authors for each article. Because the country of study origin was based on author address listings, articles often were associated with more than one country of origin. We mapped the study origin at the country-level
using the map chart program within Microsoft Excel® (version 2109). Data on the articles' publication category (e.g., energy, engineering, environmental science) and publication type (e.g., review, conference paper, or article) was also collected via SCOPUS and represented visually using Tableau® Public (ver. 2021.3) software.
The metrics identified in this study were classified into three distinct categories based on the chosen metric unit for analysis: capacity-based (nominal), generation-based, and human population-based. Capacity-based metrics refer to any SE-land metric relating a unit of land to a given unit of electrical capacity from a solar energy installation (e.g., W/m2). Generation-based metrics convey the actual or theoretical electrical generation per unit of land for a given solar energy installation (e.g., m2/Wh). Finally, human population-based metrics refer to metrics that convey the amount of land necessary to supply power to a defined group of human individuals in a given area based on localized energy demand (e.g., m2/capita).
To determine which metric terms and units to suggest as the standard metrics for different categories, we assessed the metric term and unit use based on the frequency of occurrence within the full-text of each article. We counted unique metric terms and units a maximum of once per article and categorized them into one of the three SE-land metric types. If one metric was described with the term “PV Energy Density” and another metric described a similar term, such as “Energy Density”, these terms were counted as the same term. Additionally, the units of metrics were combined if the unit was described with directly proportional measures. For example, the units of m2/MW, m2/kW, and m2/W were all counted under the unit m2/W. The term with the most frequent use across the entire corpus of articles was selected as the standardized term for each category. Similarly, the metric unit of measurement with the most frequent use was selected as the standardized unit for each category.
Results
Out of the 608 articles returned from the initial gathering phase of the systematic literature review, we identified 80 articles that met inclusion criteria, with 55 articles coming from the systematic literature review in SCOPUS and 23 additional articles that were cited by articles in the initial corpus (Figure 1). We identified articles in 18 distinct research disciplines, including environmental science, business, management and accounting, and engineering (Figure 2A). Out of the 80 articles, we found that the majority of the articles (n = 66) were peer-reviewed journal publications, six technical reports, three books or book chapters, and five conference proceedings (Figure 2B). The earliest explicit mention of land and solar energy interactions was in 1977 when (Pohl, 1977) compared the average electricity production of solar energy with a light water nuclear reactor technology. We found a large gap in the literature from 1980 to 2002; we identified only three articles that met the criteria for our analysis (Figure 2C). Publications documenting SE-land relationships began to increase in frequency in 2002; however, we noticed a rise in articles from 2008 onward, with the greatest number of articles published in 2017 (Figure 2C).
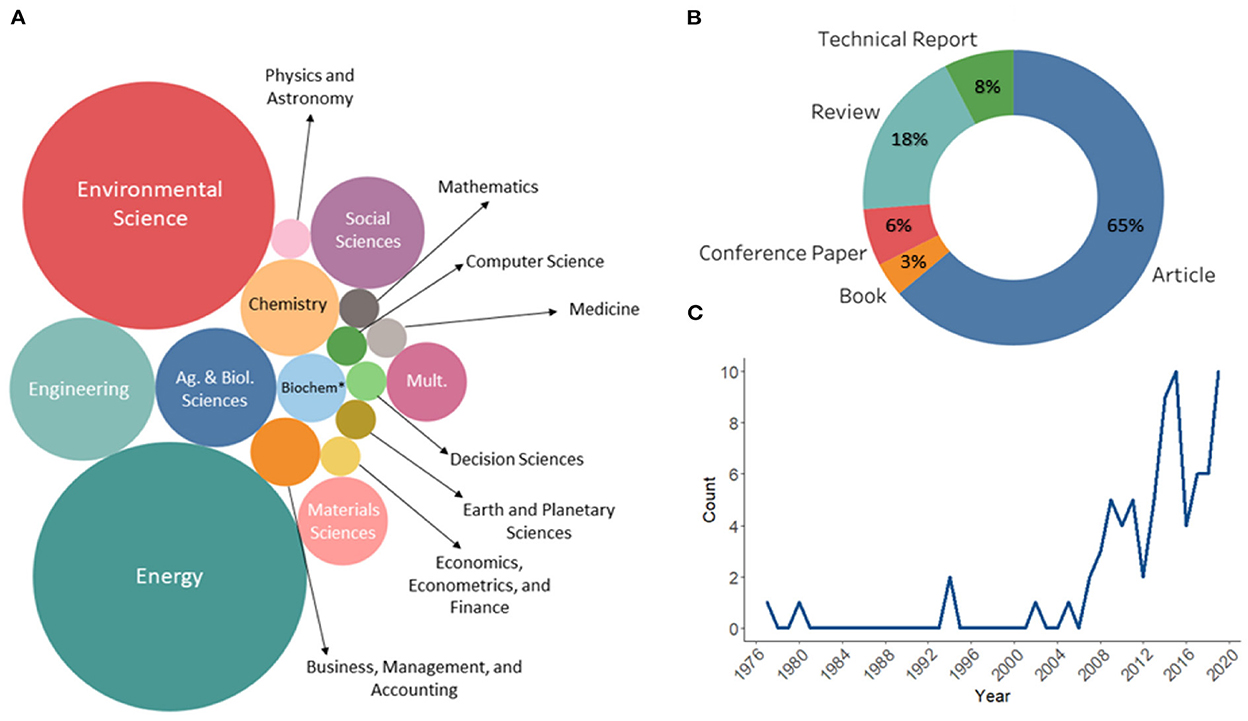
Figure 2. Bibliometric attributes of articles using solar energy-land (SE-land) metrics, including: (A) subject areas of the articles as categorized by the SCOPUS database, where “mult.” represents multidisciplinary sciences and “biochem*” represents biochemistry; size of circle is relative to the frequency of use of SE-land metrics in a category; (B) percentage of studies by type of publication; and (C) publication frequency of SE-land metrics across time.
We recorded 75 unique metrics across a total of 101 documented uses. Metrics consisted of 51 distinct terms and 43 units, which could each be classified into one of three categories: generation-based, capacity-based, and human population-based metrics (Tables 1, 2). The 51 terms reflect a combination of analogous terms identified in the review. In addition to a range of metrics used, we found multiple instances of metrics describing SE-land interactions across more than one category. Specifically, nine of the 51 terms were used in more than one of the three categories (Table 3).
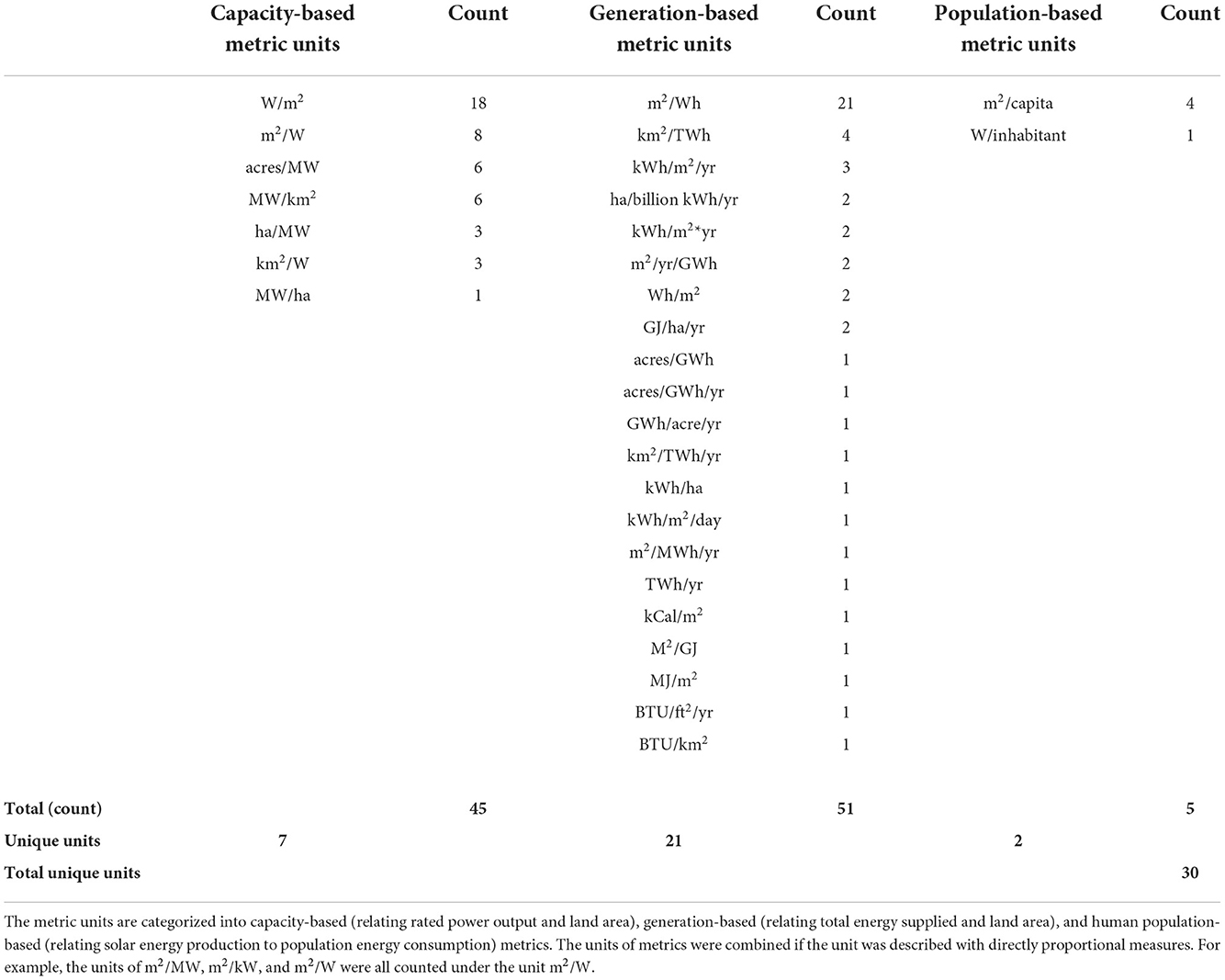
Table 1. Classification of metric units documented in the literature on solar energy-land relationships.
We found that country of study origin was highly skewed among articles, with most articles (n = 51) published by scholars and institutions in the United States. In total, we found 18 different countries of study origin and none were from South America or China; only one article had a study origin from the continent of Africa (Figure 3). Of the articles published in academic, peer-reviewed journals, we identified articles in 22 different academic journals (Figure 4). More articles (n = 13) were published in Renewable and Sustainable Energy Reviews than any other journal. Journals ranged from land-use and policy-centric journals, such as Land Use Policy, to applied engineering journals, such as Environmental Research, Engineering and Management (Figure 4). We found that articles in the corpus presenting human population-based metrics are mainly found in policy-oriented journals (e.g., Energy Policy). We also found that most metrics, both terms and units, represent less than five percent of the overall metric use in the SE-land corpus (Table 3).
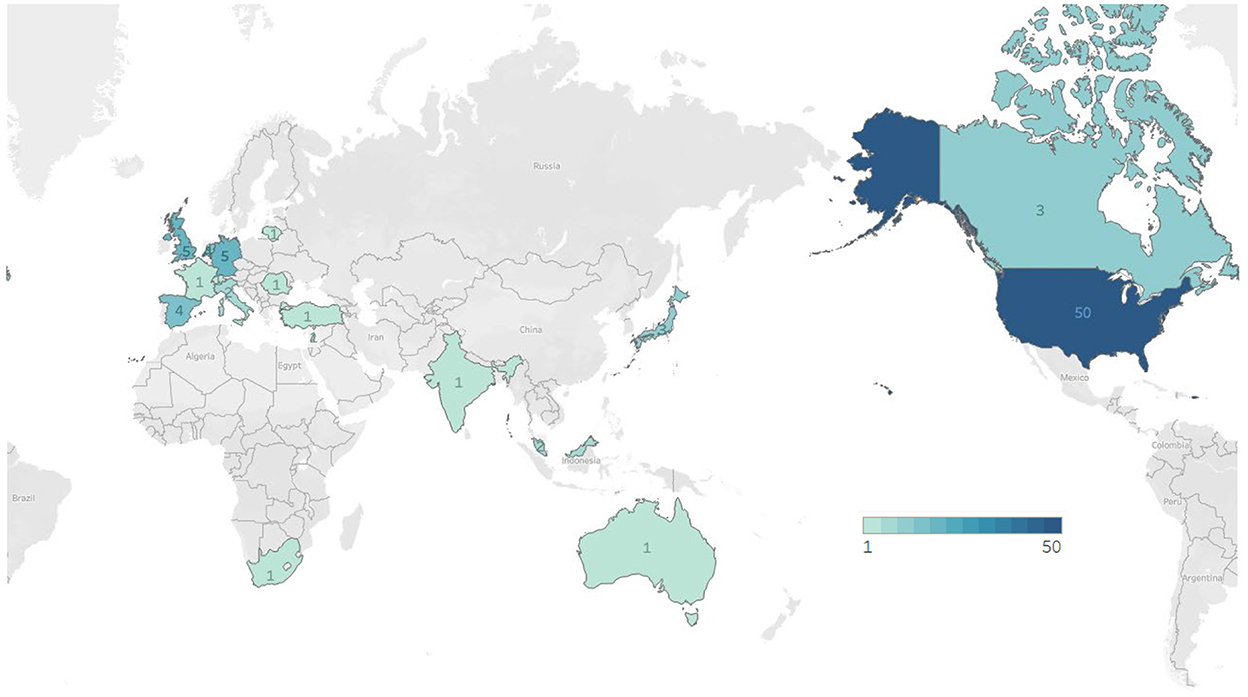
Figure 3. Number of articles reporting a metric, specifically a term and unit, to describe solar energy-land relationships by country of corresponding author origin (n = 18) in the SCOPUS database from 1977 to 2019.
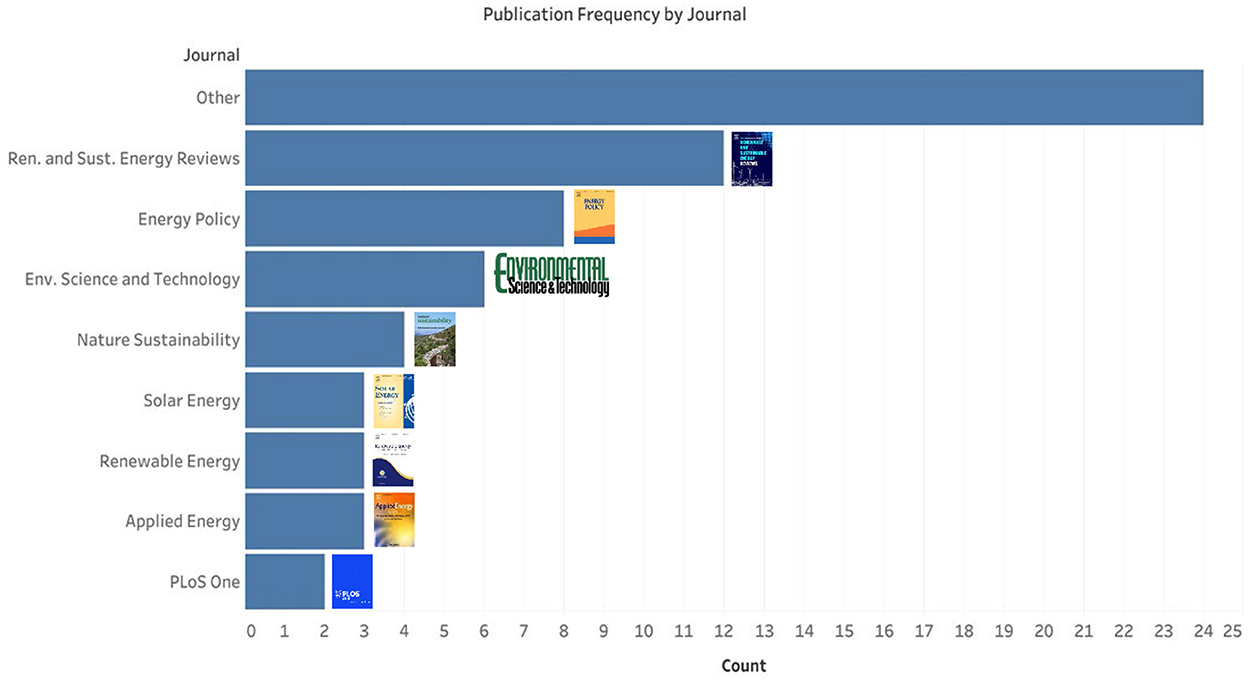
Figure 4. Number of articles (n = 66) published in journals (total unique journals = 33) identified in the SCOPUS database as of February 26th, 2020 that met inclusion criteria for this study. The category of “Other” represents number of individual journals in which one journal article reported solar energy-land relationship metrics, specifically a term and a unit, in an article.
We found the most common metric term and unit used to describe capacity-based SE-land relationships is land-use efficiency measured in W/m2 (n = 6). The most common metric term and unit used to describe generation-based SE-land relationships is land transformation measured in m2/Wh (n = 9) (Figure 5). Lastly, the most common metric describing human population-based SE-land relationships is solar footprint measured in m2/capita; however, the sample size was low (n = 2) (Figure 5). We propose these three metrics as the standardized metrics for each of the three respective categories for all future analyses describing SE-land relationships (Figure 5).
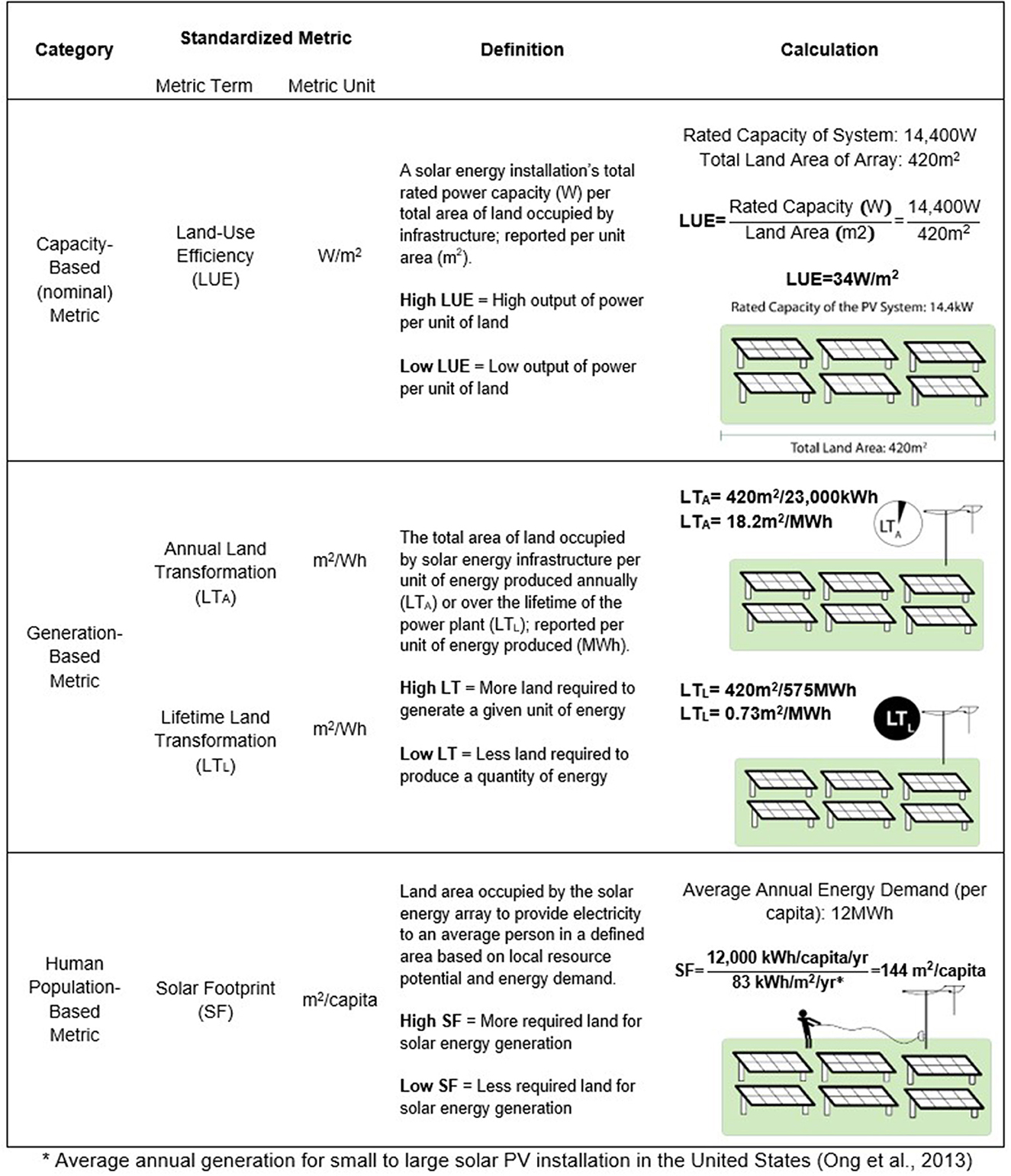
Figure 5. Standardized solar energy-land metrics for capacity-based, generation-based, and human population-based applications, including their definition and characteristics.
Discussion
We systematically evaluated the metrics employed by scholars globally to describe SE-land relationships and found not only an extraordinary number of documented terms and units but also a lack of consistency in their use. The inconsistent use of solar energy-land metrics is likely not limited to this single technology but may also characterize metric use in other emerging sustainable technologies. For example, Ahi et al. (2016) performed a bibliometric analysis of energy-related metrics in sustainable supply chains, and after identifying over 100 metrics, they found that most were used only once or twice and only three metrics were used 10 times or more. Similarly, we found that most metrics, both terms and units, represent less than five percent of the total metric use documented in the literature (Table 3). Our analysis may serve as a model to assess energy-land interaction metrics more broadly and how we, as scholars, can use a corpus of representative articles to standardize metrics in a systematic way.
An increasing concern among scholars is that the relatively large land footprint associated with a renewable energy transition seeking to meet the United Nations's SDG 7 (the equitable access to affordable, reliable, sustainable, and modern energy) may interfere with progress toward SDG 15 (the protection, restoration, and promotion of terrestrial ecosystems) (UN General Assembly, 2015). Indicators for SDG 7, such as 7.b.1 (installed renewable energy-generating capacity in developing countries), assess progress as a function of installed watts per capita, potentially overlooking the associated land footprint necessary for the deployment of a given capacity. In land-scarce nations, reaching a high level of renewable generation may require increasing amounts of forested land or areas of high biodiversity, impacting progress toward SDG 15 (Obane et al., 2020). Indicators for SDG 15 include 15.1.1 (forest area as a proportion of total land area) and 15.3.1 (proportion of degraded over total land area), both of which may be affected by large, ground-mounted solar energy development. In summary, standardized metrics that contextualize solar energy development as a function of land area are necessary to understand and anticipate reciprocity between SDG 7 and SDG 15.
When several metrics are used to describe the same phenomena in a field, standardization can be a means to reduce the vague and inconsistent presentation of research in the future and to align future research directives (Vetter et al., 2013). For example, we found that capacity-based units (m2/MW) and generation-based units (m2/MWh) were interchangeably used to define the term ‘land-use energy intensity' (Table 3). However, the two units are not easily interchangeable without additional information such as a performance-calculated capacity factor and local irradiance conditions. Using capacity-based and generation-based metric interchangeably can also lead to inaccurate results as there may not be a direct correlation between the land-use efficiency (capacity-based) and land transformation (generation-based) of all operational PV installations (Figure 6). Specifically, the energy generated from a given solar energy installation is dependent on a combination of design considerations and local climatic and irradiance conditions. Generalizing results solely from peak capacity-based ratings may under- or overestimate land transformation values (Dabou et al., 2016). As seen in Figure 6, out of six randomly selected PV installations chosen in California, the facility (CALRENEW) that generates the most energy per square meter on an annual basis also has the least efficient land footprint on a rated capacity basis. While this can be explained by the greater annual irradiance received at the CALRENEW site, it demonstrates the importance of understanding the definition of capacity-based and generation-based metrics and the potential implications that may arise from conflating the two categories of metrics.
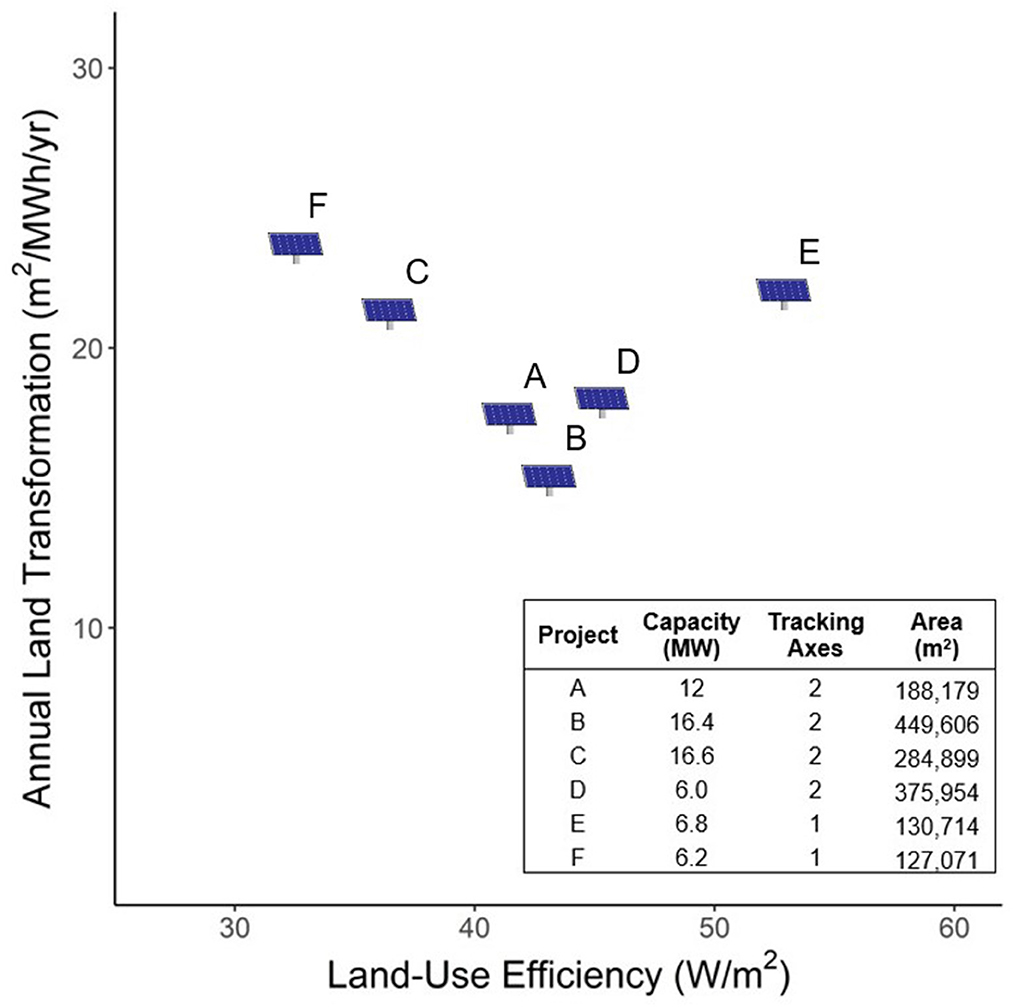
Figure 6. The land-use efficiency and land transformation of six randomly-chosen, operational large-scale solar PV installations within the state of California, United States. Additional site information and installation details can be found in Appendix C.
Along with the conflation of metric units with the same metric terms in the literature, we also identified the inconsistent transfer of metric terms themselves across the literature. Specifically, we found publications using SE-land relationship findings from previous works that define the same metrics with unique variations of the metric term from the original publication. For example, the findings of (Ong et al., 2013), a technical report demonstrating average land-use patterns for various-sized PV and CSP installations, have been cited by multiple publications that use various different metric terms to describe the original SE-land metric used in the report (capacity-weighted and generation-weighted average area requirements) (Ong et al., 2013; Calvert and Mabee, 2015; Capellán-Pérez et al., 2017). By not using consistent metric terms as stated by the original article, unnecessary ambiguity is immediately introduced to the literary corpus as the same findings have now been associated with disparate terminology.
In this study, we demonstrate the urgent need to differentiate between metrics used to describe SE-land relationships and present three unique metric categories: capacity-based, generation-based, and human population-based metrics. Explicitly differentiating between these categories and assigning a standard metric within each category allows for increased compatibility of findings across studies and avoidance of previously expressed difficulties in aggregating metadata across the literature (Horner and Clark, 2013; Hernandez et al., 2014b). Through this category-specific separation of SE-land metrics, the demonstrated benefits and limitations of the three categories of analysis may be better understood and employed across the field of study.
Capacity-based metrics
Capacity-based metrics used to describe SE-land relationships allow for comparisons of maximum theoretical (nameplate) power output over a given unit of area, thus enabling direct comparison of multiple generation technology scenarios and different deployment scenarios of the same technology (Hernandez et al., 2014b). For example, fixed-tilt solar PV arrays with different packing factors (area of solar panels relative to direct land area) can have drastically different land-use efficiencies even if the array has the same number of panels (Pasqualetti and Miller, 1984; Ong et al., 2013; van de Ven et al., 2021). Additionally, capacity-based metrics are helpful in developing efficiency targets for new and proposed projects (Ong et al., 2013). The capacity of a proposed solar energy system tends to determine the overall cost of an installation, typically in the unit of $/WDC (Feldman et al., 2015). A consistently quantified capacity-based SE-land metric can thus assist in estimating system cost and necessary land acquisition for projects during the development stage.
We found the most common metric used to describe capacity-based SE-land relationships to be land use efficiency measured in W/m2 (Figure 5). The use of the term land-use efficiency separates capacity-based metrics specifically related to SE-land interactions from similar metrics used in previous literature with various meanings, namely the power density metric. Historically, the power density metric (typically in W/m2) is a performance measure for the gravimetric density of energy converters, the installed capacity per rotor-swept area of wind turbines, the electrical output per unit area of a particular solar PV cell, and various other electrical engineering applications (Smil, 2015; Mittapally et al., 2021). Specifically, the power density metric, in the context of solar PV literature, regularly refers to the surface area of the cell converter surface itself, not the additional spatial considerations in a solar energy installation, such as panel (or heliostat) row spacing, balance-of-system equipment, and maintenance roads (Smil, 2010; Ann et al., 2020). Creating a capacity-based metric specifically for quantifying SE-land interactions reduces the risk of confusion between one of the multiple applications of the power density metric, such as the land-use considerations of solar energy and the converter-level efficiency of certain solar technologies.
Generation-based metrics
In addition to capacity-based metrics, generation-based metrics can be used for comparisons of multiple land use impact categorizations, specifically by relating the required amount of land for a unit of produced energy from a specific energy generation source (Fthenakis and Kim, 2009). For generation-based metrics, we propose the metric land transformation with units m2/Wh as it was the most frequently used metric term and unit identified in the literary corpus. Generation-based metrics, unlike capacity-based metrics, can describe actual energy generation from a solar energy installation from a given unit of land as opposed to just a theoretical maximum power output. Generation-based metrics can help to compare the actual land transformation of a project in operation to land transformation estimates during the planning, siting, and permitting phases of other projects. Utilizing an energy generation project's actual performance can help to provide more detailed and accurate insights when comparing across technologies, assessing environmental impacts, and informing future developments (Huso et al., 2021; Jordaan et al., 2021).
Factors impacting solar performance, both spatial and climatic, demonstrate the important difference between capacity-based and generation-based SE-land metrics. For example, the solar technologies evaluated in this study have lower land-use efficiencies (capacity-based measurement) than conventional fossil-fuel energy generation technologies, thus requiring more land to produce the same amount of energy (van Zalk and Behrens, 2018). Understanding the factors that affect a power plant's land-use efficiency (W/m2) and land transformation (m2/Wh) is critical to translate SE-land interactions accurately and arrive at a well-informed decision when comparing across technology types. Specifically, when discussing solar energy, the capacity factor (CF) of the solar technology deployed can have a significant impact on the actualized energy generation of the installation. Without actual generation data from a given solar installation, the generation-based land use or land transformation can be calculated by multiplying the theoretical capacity by a given capacity factor (Equation 1).
Capacity factor refers to the actualized energy generation over a given year (kWhAC) versus the theoretical maximum energy the facility could generate over the same amount of time as a function of rated capacity. Understanding the importance of a CF and the variation of capacity factors across geographic areas and technology type is an essential consideration when assessing the overall system's energy output as a function of its land-use efficiency. A fixed-tilt solar PV system may have a greater land-use efficiency (45.4 W/m2) when compared to a single-axis tracking solar PV system (40 W/m2); however, due to the increased CF of a single-axis tracking systems, the single-axis system can typically generate 25% more energy per installed capacity than the fixed-tilt system, depending on local climatic and irradiance conditions (Ong et al., 2013; Martín-Chivelet, 2016; Cossu et al., 2021). Understanding the differences between capacity-based and generation-based SE-land metrics and the application of each category for different analyses can help to reduce the conflation of metric terms with units of measurement in different categories.
A significant difference between capacity-based and generation-based metrics is the inherent temporal aspect of generation-based metrics that relate the energy generation time period to the amount of land transformed. However, we found that the methods of articles evaluating SE-land interactions do not consistently present the time horizon for measuring land transformation. For solar energy installations, the energy generation timeline can significantly impact the associated land transformation. For example, the annual land transformation from a solar energy installation that has an expected lifetime of 30 years can mislead the reader by orders of magnitude if conflated with the lifetime land transformation of that same solar installation. Due to the importance of the temporal aspect of generation-based SE-land metrics, we propose two standardized metrics in the generation-based category: annual land transformation and lifetime land transformation (Equations 2 and 3).
Annual land transformation
The metric of annual land transformation with regards to SE-land interactions relates the actual or predicted energy generation of a given solar generation facility over the course of one year (Equation 2). The annual land transformation of a given facility may be helpful when determining land requirements for a solar facility in a given area as it demonstrates the actual output of given solar technologies in that geographical area (Trainor et al., 2016). Additionally, annual land transformation metrics may be helpful when comparing solar energy technologies to conventional fossil fuel technologies as annual energy generation is a common method of reporting the performance of an energy-generating facility (Jordaan et al., 2021). Annual land transformation also uniquely demonstrates the relatively constant land-use for equivalent annual solar energy generation year after year, as opposed to the cumulative annual land use required for extractive energy sources, such as coal and natural gas (Trainor et al., 2016).
Lifetime land transformation
Our findings are relevant to the field of LCA, where SE-land interactions are examined over the life of a particular project. The lifetime land transformation of a solar energy installation is different from the annual land transformation in that it represents the land transformed divided by the cumulative energy generation over the lifetime of a solar energy installation (Equation 3). A lifetime land transformation metric also allows for the consideration of repowering the same land parcel with updated technologies at the end of a previous solar installation's useful life. As opposed to the annual land transformation, which provides SE-land interaction information on a specific annual timestep of a solar energy installation's generation as it compares to affected land area, the lifetime land transformation metric relates the entirety of energy generation of a given solar energy installation to its land area.
The lifetime land transformation metric may allow for fairer and more consistent comparisons of solar energy installations and other energy generation technologies on strictly a land-energy basis. Fossil fuel-fired power requires the conversion of new land for fuel extraction over the lifetime of the power plants. Fuel extraction sites—particularly natural gas wells—often remain unreclaimed (Haden Chomphosy et al., 2021). Shorter timescales thus discount the land benefits of solar power, which may not require new land transformation over time. Lifetime results may better inform stakeholders and decision-makers on actual land area requirements for a given project and their long-term effects.
Human population-based metrics
Human population-based metrics are used far less frequently than metrics in the capacity-based and generation-based categories. The most frequently used metric is utility-scale solar footprint with the unit m2/capita (Table 1, Figure 5). Most human population-based metrics that we identified in this study consider local energy demand, local irradiance and climatic conditions, and annual solar energy generation as a function of land area (Denholm and Margolis, 2008). By doing so, the land-related attributes of a solar energy installation can be related to the human population of a given area. Human population-based metrics may help address the need for an increase in social science engagement with solar energy development issues, including the fields human geography and land-use planning (Walker and Cass, 2007; Sovacool, 2014). Human population-based metrics may also be more relatable to broader audiences outside of engineering, helping the layperson to connect the inextricable relationship between solar energy and land. More research is needed to both quantitatively and qualitatively explore relationships between people with energy production across all disciplines.
Challenges and opportunities for the future of solar energy-land metrics
Since 2000, there has been significant progress toward incorporating accurate land calculations in the field of, specifically around energy-land interactions. Particularly, LCA analyses incorporating land-related relationships are commonly associated with the terms: land transformation and land occupation (Jordaan et al., 2021). We did identify land transformation as the most frequently used generation-based metric, but we explicitly distinguish two forms of the term: annual and lifetime. The metric land occupation, which we identified throughout our corpus but was not identified as the most used, also accounts for temporal aspects of land use. We acknowledge that these two terms, land transformation and land occupation, are likely proportionally more frequently used within the field of LCA than the metrics we identified across the entire interdisciplinary corpus.
While we recommend the use of the terms land-use efficiency and land transformation, it is critical to differentiate these types of intensity-based metrics—those quantified by directly relating land area to capacity or generation, respectively—with those that focus solely on the absolute amount of land area converted for an installation (e.g., footprint). While intensity-based metrics are immensely valuable, the absolute area of land converted to energy–or any human-dominated land-use–is also useful, especially for conservation science, in which area-based metrics are considered highly effective and are commonly employed.
Lastly, the rise of dual-use SE-land occupation, such as the co-location of PV with agriculture or livestock grazing (known as agrivoltaics and rangevoltaics, respectively), presents a unique challenge for SE-land metrics (Amaducci et al., 2018). While our metrics, as is, do not fully encapsulate the impacts (and benefits) of co-location, our metric system serves as a foundation on which future metrics can be derived and modified to meet the rapidly evolving needs of this opportunity.
Conclusion
The global increase in solar energy, and the area of land associated with its development, has stimulated increased interest and research in the field of SE-land interactions. As the field of research on SE-land interactions continues to grow expeditiously, we identified an obvious need for common, standardized metrics to assess these interactions. We also provide clear evidence that the study of SE-land interactions is an interdisciplinary pursuit. Thus, the need for standardization is necessary for the dissemination of findings across the various disciplines contributing to this field.
Standardized metrics and language to describe SE-land relationships may unite studies across a wide range of disciplines and allow for transparent dissemination of both previous and future findings. Following a systematic literature review of the current use of SE-land relationship metrics, we classified SE-land relationship metrics into capacity-based, generation-based, and human population-based metric categories and propose standard metrics, both metric term and unit, for each category. Specifically, we propose land-use efficiency (W/m2) for capacity-based, both annual and lifetime land transformation (m2/Wh) for generation-based, and solar footprint (m2/capita) for human population-based metrics. The proposed standardized metrics offer a uniting solution across all disciplines researching and reporting on SE-land relationships. Overall, the metrics proposed in this study may help to provide greater insight and understanding toward the various categories of SE-land interactions and the difference in utility provided by each category of SE-land relationships. Future research should utilize and expand on these standardized metrics to improve the accuracy of assessments and analyses describing regional, national, and global SE-land relationships.
Data availability statement
The original contributions presented in the study are included in the article/Supplementary material, further inquiries can be directed to the corresponding author.
Author contributions
AC and RH conceived of the concept for the research. AC contributed to research, data analysis, and manuscript development. MS contributed to systematic literature review, research, and manuscript development. SG contributed to project scope, methods, and review. AA contributed to concept development and manuscript review. SJ contributed to manuscript development and review. RH contributed to project conceptualization, methods, manuscript conceptualization, and manuscript review. All authors contributed to the article and approved the submitted version.
Funding
This work was supported by: AC was funded Enel Green Power, North America (A22-1395-0), the U.S. Department of Energy (DE-EE0008746), Institute of the Environment Graduate Student Fellowship, including funds from the Steindler Endowment. RH was funded Enel Green Power, North America (A22-1395-0), the U.S. Department of Energy (DE-EE0008746), Department of Land, Air and Water Resources at the University of California, Davis, and the UC Davis Agricultural Experiment Station Hatch projects (CA-R-A-6689; CA-D-LAW-2352-H). SG was funded by the Bureau of Land Management (L19AC00279). AA was funded by a NERC Industrial Innovation Fellowship (grant number: NE/R013489/1). SJ was supported by an Alfred P. Sloan grant (G-2019-12470).
Conflict of interest
The authors declare that the research was conducted in the absence of any commercial or financial relationships that could be construed as a potential conflict of interest.
Publisher's note
All claims expressed in this article are solely those of the authors and do not necessarily represent those of their affiliated organizations, or those of the publisher, the editors and the reviewers. Any product that may be evaluated in this article, or claim that may be made by its manufacturer, is not guaranteed or endorsed by the publisher.
Supplementary material
The Supplementary Material for this article can be found online at: https://www.frontiersin.org/articles/10.3389/frsus.2022.1035705/full#supplementary-material
References
Ahi, P., Searcy, C., and Jaber, M.Y. (2016). Energy-related performance measures employed in sustainable supply chains: A bibliometric analysis. Sustain. Prod. Consum. 7, 1–15. doi: 10.1016/j.spc.2016.02.001
Ahiablame, L. M., Engel, B. A., and Chaubey, I. (2012). Effectiveness of low impact development practices: Literature review and suggestions for future research. Water, Air, Soil Pollut. 223, 4253–4273. doi: 10.1007/s11270-012-1189-2
Amaducci, S., Yin, X., and Colauzzi, M. (2018). Agrivoltaic systems to optimise land use for electric energy production. Appl. Ener. 220, 545–561. doi: 10.1016/j.apenergy.2018.03.081
Ann, M. H., Kim, J., Kim, M., Alosaimi, G., Kim, D., Ha, N. Y., et al. (2020). Device design rules and operation principles of high-power perovskite solar cells for indoor applications. Nano Ener. 68, 104321. doi: 10.1016/j.nanoen.2019.104321
Armstrong, A., Ostle, N. J., and Whitaker, J. (2016). Solar Park microclimate and vegetation management effects on grassland carbon cycling. Environ. Res. Lett. 11. doi: 10.1088/1748-9326/11/7/074016
Baas, J., Schotten, M., Plume, A., Côté, G., and Karimi, R. (2020). Scopus as a curated, high-quality bibliometric data source for academic research in quantitative science studies. Quant. Sci. Stud. 1, 377–386. doi: 10.1162/qss_a_00019
Baruch-Mordo, S., Kiesecker, J. M., Kennedy, C. M., Oakleaf, J. R., and Opperman, J. J. (2019). From Paris to practice: sustainable implementation of renewable energy goals. Environ. Res. Lett. 14, 024013. doi: 10.1088/1748-9326/aaf6e0
Boyd, J., and Banzhaf, S. (2007). What are ecosystem services? The need for standardized environmental accounting units. Ecol. Econ. 63, 616–626. doi: 10.1016/j.ecolecon.2007.01.002
Cagle, A. E., Armstrong, A., Exley, G., Grodsky, S. M., Macknick, J., Sherwin, J., et al. (2020). The land sparing, water surface use efficiency, and water surface transformation of floating photovoltaic solar energy installations. Sustainability. 12, 8154. doi: 10.3390/su12198154
Calvert, K., and Mabee, W. (2015). More solar farms or more bioenergy crops? Mapping and assessing potential land-use conflicts among renewable energy technologies in eastern Ontario, Canada. Appl. Geog. 56, 209–221. doi: 10.1016/j.apgeog.2014.11.028
Capellán-Pérez, I., de Castro, C., and Arto, I. (2017). Assessing vulnerabilities and limits in the transition to renewable energies: land requirements under 100% solar energy scenarios. Renew. Sust. Energ. Rev. 77, 760–782. doi: 10.1016/j.rser.2017.03.137
Choi, C. S., Cagle, A. E., Macknick, J., Bloom, D. E., Caplan, J. S., and Ravi, S. (2020). Effects of revegetation on soil physical and chemical properties in solar photovoltaic infrastructure. Front. Environ. Sci. 8. doi: 10.3389/fenvs.2020.00140
Cossu, S., Baccoli, R., and Ghiani, E. (2021). Utility scale ground mounted photovoltaic plants with gable structure and inverter oversizing for land-use optimization. Energies. 14, 3084. doi: 10.3390/en14113084
Dabou, R., Bouchafaa, F., Hadj Arab, A., Bouraiou, A., Djamel Draou, M., Neçaibia, A., et al. (2016). Monitoring and performance analysis of grid connected photovoltaic under different climatic conditions in south Algeria. Energ. Convers. Manage. 130, 200–206. doi: 10.1016/j.enconman.2016.10.058
De Marco, A., Petrosillo, I., Semeraro, T., Pasimeni, M. R., Aretano, R., and Zurlini, G. (2014). The contribution of Utility-Scale Solar Energy to the global climate regulation and its effects on local ecosystem services. Glob. Ecol. Conserv. 2, 324–337. doi: 10.1016/j.gecco.2014.10.010
DeFries, R., Fanzo, J., Remans, R., Palm, C., Wood, S., and Anderman, T. L. (2015). Metrics for land-scarce agriculture. Science. 349, 238–40. doi: 10.1126/science.aaa5766
Denholm, P., and Margolis, R. M. (2008). Land-use requirements and the per-capita solar footprint for photovoltaic generation in the United States. Energy Policy. 36, 3531–3543. doi: 10.1016/j.enpol.2008.05.035
Dorning, M. A., Diffendorfer, J. E., Loss, S. R., and Bagstad, K. J. (2019). Review of indicators for comparing environmental effects across energy sources. Environ. Res. Lett. 14, 103002. doi: 10.1088/1748-9326/ab402d
Elborg, M. (2015). Reducing land competition for agriculture and photovoltaic energy generation-a comparison of two agro-photovoltaic plants in Japan. Int. J. Sci. Res. 39, 35.
EPRI (2019). Environmental Aspects of Renewables Workshop: 2019 Workshop Summary and Research Needs.
Exley, G., Hernandez, R. R., Page, T., Chipps, M., Gambro, S., Hersey, M., et al. (2021). Scientific and stakeholder evidence-based assessment: Ecosystem response to floating solar photovoltaics and implications for sustainability. Renew. Sust. Energ. Rev. 152, 111639. doi: 10.1016/j.rser.2021.111639
Feldman, D., Barbose, G., Margolis, R., Bolinger, M., Chung, D., Fu, R., et al. (2015). Photovoltaic System Pricing Trends. Historical, Recent, and Near-Term Projections, 2015 Edition. Golden, CO, United States. doi: 10.2172/1225306
Fraser, L. H., Henry, H. A. L., Carlyle, C. N., White, S. R., Beierkuhnlein, C., Cahill, J. F., et al. (2013). Coordinated distributed experiments: an emerging tool for testing global hypotheses in ecology and environmental science. Front. Ecol. Environ. 11, 147–155. doi: 10.1890/110279
Fritsche, U. R., Berndes, G., Cowie, A. L., Dale., V. H, Kline, K. L., Johnson, F. X., et al. (2017). Global Land Outlook: Energy and Land Use. International Renewable Energy Agency (IRENA)
Fthenakis, V., and Kim, H. C. (2009). Land use and electricity generation: a life-cycle analysis. Renew. Sust. Energ. Rev. 13, 1465–1474. doi: 10.1016/j.rser.2008.09.017
Fuso Nerini, F., Sovacool, B., Hughes, N., Cozzi, L., Cosgrave, E., Howells, M., et al. (2019). Connecting climate action with other sustainable development goals. Nat. Sustain. 2, 674–680. doi: 10.1038/s41893-019-0334-y
Grodsky, S. M., Campbell, J. W., and Hernandez, R. R. (2021). Solar energy development impacts flower-visiting beetles and flies in the Mojave Desert. Biol. Conserv. 263, 109336. doi: 10.1016/j.biocon.2021.109336
Grodsky, S. M., and Hernandez, R. R. (2020). Reduced ecosystem services of desert plants from ground-mounted solar energy development. Nat. Sustain. 3, 1036–1043. doi: 10.1038/s41893-020-0574-x
Grubert, E., Rogers, E., and Sanders, K. T. (2020). Consistent terminology and reporting are needed to describe water quantity use. J. Water Resour. Plan. Manag. 146, 04020064. doi: 10.1061/(ASCE)WR.1943-5452.0001241
Guoqing, L., Hernandez, R. R., Blackburn, G. A., Davies, G., Hunt, M., Whyatt, J. D., et al. (2021). Ground-mounted photovoltaic solar parks promote land surface cool islands in arid ecosystems. Renew. Sust. Energ. Trans. 1, 100008. doi: 10.1016/j.rset.2021.100008
Gustafson, D., Gutman, A., Leet, W., Drewnowski, A., Fanzo, J., and Ingram, J. (2016). Seven food system metrics of sustainable nutrition security. Sustainability 8, 196. doi: 10.3390/su8030196
Haden Chomphosy, W., Varriano, S., Lefler, L. H., Nallur, V., McClung, M. R., and Moran, M. D. (2021). Ecosystem services benefits from the restoration of non-producing US oil and gas lands. Nat. Sustain. 4, 547–554. doi: 10.1038/s41893-021-00689-4
Hernandez, R. R., Armstrong, A., Burney, J., Ryan, G., Moore-O'Leary, K., Diédhiou, I., et al. (2019). Techno–ecological synergies of solar energy for global sustainability. Nat. Sustain. 2, 560–568. doi: 10.1038/s41893-019-0309-z
Hernandez, R. R., Easter, S. B., Murphy-Mariscal, M. L., Maestre, F. T., Tavassoli, M., Allen, E. B., et al. (2014a). Environmental impacts of utility-scale solar energy. Renew. Sust. Energ. Rev. 29, 766–779. doi: 10.1016/j.rser.2013.08.041
Hernandez, R. R., Hoffacker, M. K., and Field, C. B. (2014b). Land-use efficiency of big solar. Environ. Sci. Technol. 48, 1315–1323. doi: 10.1021/es4043726
Hernandez, R. R., Hoffacker, M. K., Murphy-Mariscal, M. L., Wu, G. C., and Allen, M. F. (2015). Solar energy development impacts on land cover change and protected areas. Proc Natl Acad Sci U S A. 112, 13579–13584. doi: 10.1073/pnas.1517656112
Hernandez, R. R., Jordaan, S. M., Kaldunski, B., and Kumar, N. (2020b). Aligning climate change and sustainable development goals with an innovation systems roadmap for renewable power. Front. Sustain. 1. doi: 10.3389/frsus.2020.583090
Hernandez, R. R., Tanner, K. E., Haji, S., Parker, I. M., Pavlik, B. M., and Moore-O'Leary, K. A. (2020a). Simulated photovoltaic solar panels alter the seed bank survival of two desert annual plant species. Plants. 9, 1125. doi: 10.3390/plants9091125
Hoffacker, M. K., Allen, M. F., and Hernandez, R. R. (2017). Land-sparing opportunities for solar energy development in agricultural landscapes: a case study of the Great Central Valley, CA, United States. Environ. Sci. Technol. 51, 14472–14482. doi: 10.1021/acs.est.7b05110
Hoffacker, M. K., and Hernandez, R. R. (2020). Local energy: spatial proximity of energy providers to their power resources. Front. Sustain. 0, 7. doi: 10.3389/frsus.2020.585110
Horner, R. M., and Clark, C. E. (2013). Characterizing variability and reducing uncertainty in estimates of solar land use energy intensity. Renew. Sust. Energ. Rev. doi: 10.1016/j.rser.2013.01.014
Houghton, R. A., House, J. I., Pongratz, J., Van Der Werf, G. R., Defries, R. S., Hansen, M. C., et al. (2012). Carbon emissions from land use and land-cover change. Biogeosciences. 9, 5125–5142. doi: 10.5194/bg-9-5125-2012
Huso, M., Conkling, T., Dalthorp, D., Davis, M., Smith, H., Fesnock, A., et al. (2021). Relative energy production determines effect of repowering on wildlife mortality at wind energy facilities. J. Appl. Ecol. 58, 1284–1290. doi: 10.1111/1365-2664.13853
IEA (2020). Solar PV – Renewables (2020). [WWW Document]. Available online at: https://www.iea.org/reports/renewables-2020/solar-pv (accessed December 02, 2021).
IEA (2021). World Energy Outlook 2021. Available online at: https://www.iea.org/reports/world-energy-outlook-2021
IRENA (2019). Future of Solar Photovoltaic: Deployment, Investment, Technology, Grid Integration and Socio-Economic Aspects. Abu Dhabi.
Jordaan, S. M., Heath, G. A., Macknick, J., Bush, B. W., Mohammadi, E., Ben-Horin, D., et al. (2017). Understanding the life cycle surface land requirements of natural gas-fired electricity. Nat. Energy. 2, 804–812. doi: 10.1038/s41560-017-0004-0
Jordaan, S. M., Lee, J., McClung, M. R., and Moran, M. D. (2021). Quantifying the ecosystem services values of electricity generation in the US Chihuahuan Desert: a life cycle perspective. J. Ind. Ecol. 25, 1089–1101. doi: 10.1111/jiec.13111
Koellner, T., de Baan, L., Beck, T., Brandão, M., Civit, B., Margni, M., et al. (2013). UNEP-SETAC guideline on global land use impact assessment on biodiversity and ecosystem services in LCA. Int. J. Life Cycle Assess. 18, 1188–1202. doi: 10.1007/s11367-013-0579-z
Lane, J., Largent, M., and Rosen, R. (2014). “Science Metrics and Science Policy”, in Beyond Bibliometrics: Harnessing Multidimensional Indicators of Scholarly Impact, Cronin, B., and Sugimoto, C. R. (Eds.). Cambridge, Massachusetts: The MIT Press.
Lovering, J., Swain, M., Blomqvist, L., and Hernandez, R. R. (2022) Land-use intensity of electricity production tomorrow's energy landscape. PLoS ONE 17, e0270155. doi: 10.1371/journal.pone.0270155
Mancini, F., and Nastasi, B. (2020). Solar energy data analytics: PV deployment and land use. Energies. 13, 417. doi: 10.3390/en13020417
Martín-Chivelet, N. (2016). Photovoltaic potential and land-use estimation methodology. Energy. 94, 233–242. doi: 10.1016/j.energy.2015.10.108
Mittapally, R., Lee, B., Zhu, L., Reihani, A., Lim, J. W., Fan, D., et al. (2021). Near-field thermophotovoltaics for efficient heat to electricity conversion at high power density. Nat. Commun. 12, 1–8. doi: 10.1038/s41467-021-24587-7
Moher, D., Liberati, A., Tetzlaff, J., and Altman, D. G. (2009). Preferred reporting items for systematic reviews and meta-analyses: the PRISMA statement. PLoS Med. 6, e1000097. doi: 10.1371/journal.pmed.1000097
Moore-O'Leary, K. A., Hernandez, R. R., Johnston, D. S., Abella, S. R., Tanner, K. E., Swanson, A. C., et al. (2017). Sustainability of utility-scale solar energy - critical ecological concepts. Front. Ecol. Environ. 15, 385–394. doi: 10.1002/fee.1517
Mulvaney, D. (2013). Opening the black box of solar energy technologies: exploring tensions between innovation and environmental justice. Sci. Culture. 22, 230–237. doi: 10.1080/09505431.2013.786995
Murphy, C., Sun, Y., Cole, W. J., Maclaurin, G. J., Mehos, M. S., and Turchi, C. S. (2019). The potential role of concentrating solar power within the context of DOE's 2030 solar cost targets (No. NREL/TP-6A20-71912). Golden, CO: National Renewable Energy Lab (NREL). doi: 10.2172/1491726
Obane, H., Nagai, Y., and Asano, K. (2020). Assessing land use and potential conflict in solar and onshore wind energy in Japan. Renew. Ener. 160, 842–851. doi: 10.1016/j.renene.2020.06.018
Ong, S., Campbell, C., Denholm, P., Margolis, R., and Heath, G. (2013). Land-Use Requirements for Solar Power Plants in the United States. (No. NREL/TP-6A20-56290). National Renewable Energy Lab (NREL), Golden, CO, United States. doi: 10.2172/1086349
Pasqualetti, M. J., and Miller, B. A. (1984). Land requirements for the solar and coal options. Geogr. 150, 192. doi: 10.2307/634998
Pohl, R. O. (1977). Land-use for nuclear and for solar energy. Environ. Conserv. 4, 289–290. doi: 10.1017/S0376892900026217
Provencher, J. F., Bond, A. L., Avery-Gomm, S., Borrelle, S. B., Bravo Rebolledo, E. L., Hammer, S., et al. (2017). Quantifying ingested debris in marine megafauna: a review and recommendations for standardization. Anal. Methods. 9, 1454–1469. doi: 10.1039/C6AY02419J
Pullin, A. S., and Stewart, G. B. (2006). Guidelines for systematic review in conservation and environmental management. Conserv. Biol. 20, 1647–1656. doi: 10.1111/j.1523-1739.2006.00485.x
Rabaia, M. K. H., Abdelkareem, M. A., Sayed, E. T., Elsaid, K., Chae, K. J., Wilberforce, T., et al. (2021). Environmental impacts of solar energy systems: a review. Sci. Total Environ. 754, 141989. doi: 10.1016/j.scitotenv.2020.141989
Rehbein, J. A., Watson, J. E. M., Lane, J. L., Sonter, L. J., Venter, O., Atkinson, S. C., et al. (2020). Renewable energy development threatens many globally important biodiversity areas. Glob. Change Biol. 26, 3040–3051. doi: 10.1111/gcb.15067
Smil, V. (2010). Power Density Primer: Understanding the Spatial Dimension of the Unfolding Transition to Renewable Electricity Generation (Part I-Definitions).
Smil, V. (2015). Power Density: A Key to Understanding Energy Sources and Uses. MIT Press. doi: 10.7551/mitpress/10046.001.0001
Sovacool, B. K. (2014). What are we doing here? Analyzing fifteen years of energy scholarship and proposing a social science research agenda. Energy Res. Soc. Sci. 1, 1–29. doi: 10.1016/j.erss.2014.02.003
Stoms, D. M., Dashiell, S. L., and Davis, F. W. (2013). Siting solar energy development to minimize biological impacts. Renew. Energy. 57, 289–298. doi: 10.1016/j.renene.2013.01.055
Sullivan, R. G., Kirchler, L. B., Mccarty, J., Beckman, K., and Richmond, P. (2012). “Visual Impacts of Utility-scale Solar Energy Facilities on Southwestern Desert Landscapes,” in The National Association of Environmental Professionals 37th Annual Conference. p. 21–24.
Tanner, K. E., Moore-O'Leary, K. A., Parker, I. M., Pavlik, B. M., Haji, S., and Hernandez, R. R. (2021). Microhabitats associated with solar energy development alter demography of two desert annuals. Ecol. Appl. 31, e02349. doi: 10.1002/eap.2349
Tanner, K. E., Moore-O'Leary, K. A., Parker, I. M., Pavlik, B. M., and Hernandez, R. R. (2020). Simulated solar panels create altered microhabitats in desert landforms. Ecosphere. 11, e03089. doi: 10.1002/ecs2.3089
Trainor, A. M., Mcdonald, R. I., and Fargione, J. (2016). Energy sprawl is the largest driver of land use change in United States. PLoS ONE. 11, e0162269. doi: 10.1371/journal.pone.0162269
Tsoutsos, T., Frantzeskaki, N., and Gekas, V. (2005). Environmental impacts from the solar energy technologies. Energy Policy. 33, 289–296. doi: 10.1016/S0301-4215(03)00241-6
Vallance, L., Charbonnier, B., Paul, N., Dubost, S., and Blanc, P. (2017). Toward a standardized procedure to assess solar forecast accuracy: a new ramp and time alignment metric. Sol. Energy. 150, 408–422. doi: 10.1016/j.solener.2017.04.064
van de Ven, D. J., Capellan-Peréz, I., Arto, I., Cazcarro, I., de Castro, C., Patel, P., et al. (2021). The potential land requirements and related land use change emissions of solar energy. Sci. Reports. 11, 2907. doi: 10.1038/s41598-021-82042-5
van Zalk, J., and Behrens, P. (2018). The spatial extent of renewable and non-renewable power generation: a review and meta-analysis of power densities and their application in the U.S. Energy Policy. 123, 83–91. doi: 10.1016/j.enpol.2018.08.023
Vetter, D., Rücker, G., and Storch, I. (2013). Meta-analysis: a need for well-defined usage in ecology and conservation biology. Ecosphere. 4, 1–24. doi: 10.1890/ES13-00062.1
Walker, G., and Cass, N. (2007). Carbon reduction, ‘the public' and renewable energy: engaging with socio-technical configurations. Area. 39, 458–469. doi: 10.1111/j.1475-4762.2007.00772.x
Keywords: land-use and land-cover change, renewable energy, electricity, energy transition, solar energy, metrics
Citation: Cagle AE, Shepherd M, Grodsky SM, Armstrong A, Jordaan SM and Hernandez RR (2023) Standardized metrics to quantify solar energy-land relationships: A global systematic review. Front. Sustain. 3:1035705. doi: 10.3389/frsus.2022.1035705
Received: 03 September 2022; Accepted: 31 October 2022;
Published: 27 February 2023.
Edited by:
Mikołaj Owsianiak, Technical University of Denmark, DenmarkReviewed by:
Afreen Siddiqi, Massachusetts Institute of Technology, United StatesYang Yu, Beijing Forestry University, China
Copyright © 2023 Cagle, Shepherd, Grodsky, Armstrong, Jordaan and Hernandez. This is an open-access article distributed under the terms of the Creative Commons Attribution License (CC BY). The use, distribution or reproduction in other forums is permitted, provided the original author(s) and the copyright owner(s) are credited and that the original publication in this journal is cited, in accordance with accepted academic practice. No use, distribution or reproduction is permitted which does not comply with these terms.
*Correspondence: Alexander E. Cagle, aecagle@ucdavis.edu
†Present address: Steven M. Grodsky, U.S. Geological Survey, New York Cooperative Fish and Wildlife Research Unit, Department of Natural Resources and the Environment, Cornell University, Ithaca, NY, United States