Influence of Martian Radiation-like Conditions on the Growth of Secale cereale and Lepidium sativum
- 1Reactor Institute Delft (RID), Delft University of Technology and Wageningen University & Research, Delft, Netherlands
- 2Wageningen Environmental Research, Wageningen University & Research, Wageningen, Netherlands
- 3Reactor Institute Delft (RID), Delft University of Technology, Delft, Netherlands
- 4Plant Sciences Group, Laboratory of Plant Physiology, Wageningen, Netherlands
- 5Biometris, Wageningen, Netherlands
The Martian surface is constantly exposed to a high dose of cosmic radiation consisting of highly energetic particles and multiple types of ionizing radiation. The dose can increase temporarily by a factor of 50 through the occurrence of highly energetic solar flares. This may affect crop growth in greenhouses on the Martian surface possibly making settlement of humans more complicated. Shielding crops from radiation might be done at the expense of lighting efficiency. However, the most energy-efficient cultivation may be achieved through the use of natural daylight with the addition of LED lights. The goal of our research was to investigate whether Martian radiation, both the constant and the solar flares events, affects plant growth of two crop species, rye and garden cress. The levels of radiation received on the surface of Mars, simulated with an equivalent dose of 60Co γ-photons, had a significant negative effect on the growth of the two crop species. Although germination percentages were not affected by radiation, biomass growth was significantly decreased by 32% for cress and 48% for rye during the first 4 weeks after germination. Part of the biomass differences may be due to differences in temperature between radiation and control treatment, however it cannot explain the whole difference between the treatment and control. Coloring of leaves, necrosis and brown parts, was observed as well. Temporary increases in ionizing radiation dose at different development stages of the plants did not significantly influence the final dry weight of the crops.
Introduction
The intention of the National Aeronautics and Space Administration (NASA) to send the first humans to Mars in the 2030’s means that (semi-) permanent space communities will be set up at the Martian surface. An important task will be crop production, which is needed to provide the astronauts with sufficient fresh food (Horneck et al., 2006; Wamelink et al., 2014). There are several options to grow food on Mars. The first option is hydroponics. A second option is aeroponics (Maggi & Pallud, 2010). A third option is to use the regolith on Mars or the Moon for crop growth, the option used in this research. Wamelink et al. (2014) showed that different crop species are able to germinate and grow on Mars regolith simulant JSC 1A. However, crop production on Mars will be more difficult compared to Earth due to the many adverse conditions present. One of those adverse conditions is the permanent higher ionizing radiation (IoR) on the Martian surface (Guo et al., 2017).
The source of IOR on the Martian surface is bipartite. The permanent part consists of galactic cosmic rays (GCRs) at a relatively low, constant flux (ICRP, 2013). Their composition varies slightly over time but in general 85–90% consist of protons (р), 10–13% of He-ions (α), 1% of electrons (е) and 1% of heavier nuclei (Hassler et al., 2013). Measurements done by the Mars Curiosity Rover in 2012 showed that the total amount of radiation received at the surface is 233 ± 12 μGy/d (Matthiä et al., 2017). This is approximately 17 times higher than the highest natural absorbed dose measured on the Earth’s surface (UNSCEAR, 2008). Measurements in Skylab showed mean dose rates up to 860 μGy/day and Apollo 14 even up to 1,270 μGy/day (Benton & Benton, 2001). This absorbed dose (further referred to as IoR dose) is easily increased with a factor of 50 (Zeitlin et al., 2004) by the second, variable source of IoR, the solar energetic particles (SEPs). SEPs are primarily protons which are accelerated up to hundreds of MeV by sun flares and coronal mass ejections and their corresponding chocks (Hassler et al., 2013). SEP fluxes are relatively sporadic in nature and depend on the reversal of the Sun’s magnetic field each one-half of the 22 years Hall cycle. SEPs have a mean duration of 4.13 days (Jun et al., 2007), but the duration of an individual SEP can vary from a few hours up to a week.
The SEPs and the GCRs together form a complex radiation environment which differ significantly from the radiation environment present at the Earth’s surface. On Earth, radiation is a less influential factor for crop growth since the Earth’s thick atmosphere and its strong magnetic field protects crops against high doses and UV-radiation (Jakosky & Phillips, 2001; Nicholson et al.,2005). Nevertheless, crops generally possess a high degree of resistance to single, short-term high IoR doses which are not present under natural circumstances; in the case of barley (Hordeum vulgare) seeds, a dose of X-rays of 500 Gy was lethal (Caldecott, 1955). Curiosity measurements on Mars showed a dose of 233 ± 12 μGy/d (Matthiä et al., 2017), which is magnitudes lower than the experiment by Caldecott.
Other experiments have shown that a single exposure to 500 Gy of γ-photons still resulted in 20% seedling survival by different Poaceae species (Kianian et al., 2016). Experiments with Arabidopsis thaliana showed that seeds displayed 100% survival under normal radiation conditions after exposure to γ-photons with a total dose of ±1800 Gy (Hase et al., 2017). Survival rates for doses obtained by radiation with ionizing particles was much lower. Single exposures to a dose of ±100 Gy obtained by using 15.8 MeV u−1 neon ions resulted in only 40% survival. The survival of seeds exposed to 17.3 MeV u−1 carbon ions with a total dose of 300 Gy was 17% (Hase et al., 2017).
Experiments assessing the effects of long-term IoR exposure are scarce and show that plants are able to deal with chronic doses which are factors higher than the Martian radiation dose of 233 μGy day−1 (Van Hoeck et al., 2017). Therefore, it is assumed that the Martian IoR doses will not influence plant growth and development. However, as far as we know, this has never been tested. In particular, sudden dose increases, comparable to SEP events, have not been investigated yet.
In this study we investigated how non-ionizing radiations on the surface of Mars may affect the growth of rye (Secale cereale) and garden cress (Lepidium sativum). The goal of the experiment was to investigate whether or not germination and subsequent growth and development was influenced by the presence of a simulated Martian radiation environment. Both crops were sown in Mars regolith simulant and exposed to a constant Martian radiation dose during the course of the experiment. SEP-events were simulated on plants during different development stages to determine whether radiation changes over time affect plants.
Materials and Methods
Plant Material
The experiment was carried out with two crop species Secale cereale and Lepidium sativum. Both species were used in earlier experiments on growing crops on Mars (and Moon) regolith simulants JSC 1A and MMS (Wamelink et al., 2014, 2019). Both plant species germinate quickly (normally within 24 h) have no seed dormancy, rapid growth and grew well on Mars regolith simulant JSC 1A (enriched with organic material) if nutrient solution was supplied (Wamelink et al., 2019). Seeds of both species were sown in pots located in six rectangular trays of 52 by 32 cm (Figure 1A). Each tray consisted of 84, 4 × 4 × 6 cm (l x w x d), pots located in seven columns and twelve rows. On the bottom of a pot a piece of paper was put to prevent leaking of soil/simulant. Trays with pots were placed in trays to collect leakage water.
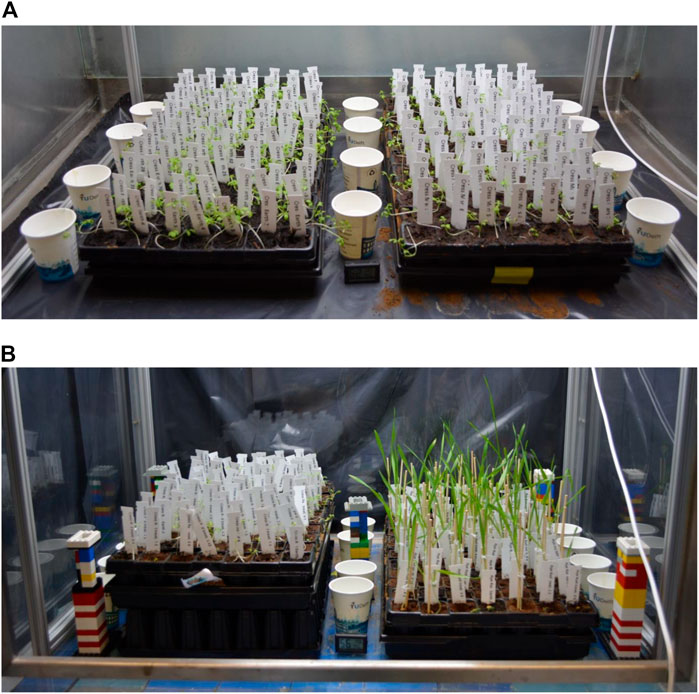
FIGURE 1. (A) sowing the cress control Earth (CE, left) and control Mars (CM, right). Shown are the pots with trays, the water cups and the temperature and humidity meter located in between the two trays. (B) is showing the radiation treatments in the radiation fume hood surrounded by lead walls. Treatments of cress (left) and rye (right) are indicated with white labels. Lego towers hold thermoluminescent dosimetry (TLD) cups at the top. Pictures were taken on the morning of harvest.
Rye seeds were sown in the center of the 84 pots filled with potting soil (composition: Welkoop, 2017; www.welkoop.nl; Control Earth; CE rye) and in 84 pots filled with Mars regolith simulant (Mars regolith simulant JSC-1A; Control Mars; CM rye). Cress seeds were sown in two other trays in the same way (CE Cress & CM Cress), with two seeds per pot in opposing corners of each pot. All seeds were pushed gently into the soil/simulant without covering the seeds with the substrate. The CE and CM trays were placed in two similar fume hoods (90 × 70 × 95 cm) without radiation and functioned as the control experiments. Note that each of the two fume hoods only contained one plant species.
In the remaining two trays, consisting of 72 pots filled with Mars regolith simulant and 12 pots filled with potting soil, seeds were sown in the same way as in the control treatments, with each species in its own tray (Figure 1B). Each pot was randomly allocated to one Solar Energetic Particle (SEP) treatment. SEP treatment was applied to part of the seeds or germinated plants (see 2.3). Seeds sown in the pots with potting soil were marked with Earth (E) and were not imposed to a SEP event. Subsequently, the two trays were put in a fume hood containing a radiation field.
To sum up, we had per plant species:
- Control: 84 pots in potting soil and 84 pots in Mars regolith simulant.
- Radiation field: 72 pots in Mars regolith simulant treated with a SEP event (12 per treatment) and 12 pots in potting soil.
Radiation Environment
A radiation environment was created in a Perspex fume hood (110 × 65 × 90 cm) surrounded by a 4.5 cm thick lead wall at three sides in order to protect the surrounding environment against the IoR used. An aluminum frame was placed in the fume hood. At 80 cm from the bottom surface of the fume hood wires were connected to the framework. To the wires five plastic capsules were attached in a five-point dice formation. The four capsules in the corners were attached at a distance of 5 cm in width and 7 cm in length from the corner edge. The fifth capsule was located exactly in the middle of the aluminum framework (Supplementary Appendix 1).
In 17 plastic High-Density Polyethylene (HDPE) type E cups, 25 mg of 59Co powder was placed. The cups were piled and put into a plastic irradiation capsule. The capsule was irradiated with a thermal neutron flux of 3.7*1012 neutrons cm−2 s−1 for 5 h in the Befa-08 irradiation facility of the Reactor Institute Delft (RID): Delft, Netherlands (www.rid.tudelft.nl). This resulted in 60Co, and some other radionuclides as by-products. The yield of these radionuclides is very limited (<< 0.1%) and/or their half-life is much shorter than that of the 60Co and therefore did not influence our experiment. After one day of cooling, individual cups were transferred to five source holders. The four source holders in the corner received three irradiated cups each and the remaining five cups were put in the central source holder to create an even field. The total dose rate of the radiation environment at the growing tips of the plants was equal to 270.50 Gy d−1 as measured by thermoluminescence dosimetry at five different locations in the radiation fume hood (Supplementary Appendix 2). The meters were placed in the fume hood below the five 60Co emitters and raised during the experiment to match the top of the plants (Figure 1B, the small ‘Lego’ towers). The exposure to the roots was not measured. The dose rate of 270.50 ± 25.31 μGy d−1 was higher compared to the dose rate measured at the Martian surface of 233 ± 12 μGy/d (Matthiä et al., 2017), but close enough for our purpose. All irradiated plants were placed in the led walled fume hood.
Solar Energetic Particle Events
SEP-events were simulated by imposing seeds, seedlings and plants to the beam of the 60Co source 8,290 of the RID with an equivalent calculated dose rate of on average 1,333 μGy h−1. The time plants were exposed to the 60Co source slightly increased over the experiment due to decreasing activity of the 60Co source but equaled on average 22.51 h. The duration of each SEP-treatment was calculated by dividing the intended total dose of 30 mGy by the absorbed dose rate of the 60Co source at the moment of radiation. The total dose of 30 mGy was chosen based on the records of a real SEP-event on Mars (Zeitlin et al., 2004).
In our experiment one SEP-event consisted of a dose of on average 27.29 ± 1.17 mGy (Supplementary Appendix 3). The distance between the 60Co source and the plant material was 300 cm during each treatment to get the correct absorbed dose (D). 5 days before sowing, twelve seeds of both species (T1) were exposed to a simulated SEP-event. Subsequently, every week a new set of twelve plants (whole plants including the roots in the soil/simulant) of each species grown in the radiation environment was exposed to a simulated SEP-event, treatments T2-T5 (Table 1). Another twelve pots were marked with No-SEP (NSEP) and similar to the Earth (E) treatments they were never exposed to a simulated SEP-event. So, for every treatment we had twelve plants giving twelve independent responses per treatment.

TABLE 1. Overview of imposed simulated Solar Energetic Particle (SEP) events per plant species (n = 12 per treatment). A SEP-event was simulated by exposing the plant material 22.51 h to a 60Co-source with a dose rate of 1,333 μGy h−1. Days are counted from the start of the experiment.
During each SEP-event the total absorbed dose was measured by thermoluminescence dosimetry in quadruple. At the end of each SEP-event TLD cups were collected and stored till the end of the experiment. The final radiation dose was calculated by multiplying the amount of counts with a 60Co-conversion factor of 2.29 μGy count−1. After exposure to a SEP-event, all plants were put back in the fume hood with the constant radiation environment at places similar to the places they stood before.
Growth Conditions
All treatments were placed in fume hoods. This was not ideal for plant growth, but a necessity because of safety precautions related to the radiation treatment. In the two fume hoods used for the control treatments ventilation was shut down to improve environmental conditions regarding temperature and moisture content, which would be too low with the ventilation on. In the radiation fume hood ventilation was kept on, with an air speed of 0.25–0.45 m/s at the opening (according to NEN standard), because of radiation safety regulations.
The glass or Perspex windows of all fume hoods were covered with light-impermeable black garbage bags, to prevent light coming from the outside to influence the experiment. Lighting was done by PAN-KW-60–60 LED-lights (40W, white, 5000 K, 5630 SMD Samsung) with dimensions of 60 × 60 × 11 cm. The lights were adjusted to the aluminum frame at 85 cm above the pots, yielding around 2,200 Lux (or around 42 µmol/m2/s) at pot level. A time switch was used to switch the lights on at seven AM and off at seven PM resulting in a 12 h photoperiod.
Water was supplied as normal tap water. Once a week instead of water a standard nutrient solution was supplied (with EC 1.45 mS, pH 5.7). The solution was made by adding 25.2 L Zwakal, 44.2 L BFK, 14.4 L Baskal, 13.8 L Amnitra, 10.4 L Magnitra and 64 L Calsal to 100,000 L of water (standard nutrient solution at WUR, see also Wamelink et al., 2019). Plants were watered daily with paper cups to keep the soil/simulant moist, excessive water was stored in a second tray underneath the tray with the pots. Spraying was used to increase humidity. Average air humidity was 50 ± 8% for control experiments and 47 ± 3% for the radiation experiment and do not differ significantly overall (Supplementary Appendix 4). Once a week instead of water a standard nutrient solution was supplied (with EC 1.45 mS, pH 5.7). The solution was made by adding 25.2 L Zwakal, 44.2 L BFK, 14.4 L Baskal, 13.8 L Amnitra, 10.4 L Magnitra and 64 L Calsal to 100,000 L of water (standard nutrient solution at WUR, see also Wamelink et al., 2019). Average air temperature was 20.5 ± 0.4 C for the control experiments and 18.0 ± 0.8 C for the radiation experiment (Figure 2). Air temperature and humidity were measured daily in all fume hoods with a 2-in-1 humidity- and thermometer (www.woodandtools.com, ref. number WT1485393632).
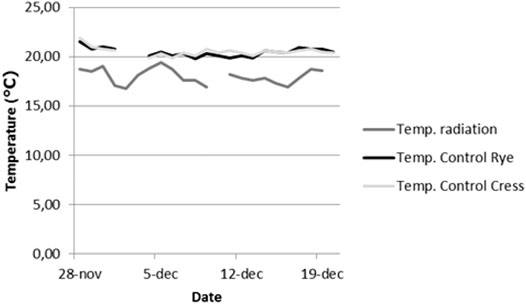
FIGURE 2. Temperature changes over the course of the experiment for control and radiation fume hoods. Gaps in lines indicate missing data. Temperatures were obtained with a 2-in-1 humidity- and temperature measurement device (see also Supplementary Appendix 4).
Data
The germination rate was determined daily during the experiment and final germination percentages were calculated. Differences between germination rates for the various treatments were pairwise tested by means of t-test. After 28 days, plants were harvested and dry weight (DW) was determined after two days of oven (Marius Nieuwegein. 380 v, 8 KW) drying at 70 C. DW was statistically analyzed by means of regression from which p-values for pairwise differences were obtained using a Fisher exact test by calculations in Genstat (VSN International, 2020). Differences were assumed significant when p < 0.01 in all tests performed.
Results
Germination
For both rye and cress, the highest germination percentage (98.8%) was observed in the CE treatments. Lowest germination percentage for rye was found for the T5 treatment (Table 1, 75.0%). For cress, the lowest germination percentage (91.7%) was found for T1, T3, T4 and E (Supplementary Appendix 5). Pairwise comparisons of germination percentages proved no significant differences among treatments (n = 12 per treatment; 12 Earth control and six SEP events on Mars regolith simulant of 12 replicas, p < 0.01; Supplementary Appendix 6). There is only an indication for a difference in rye between T5 and CE (p = 0.011; Supplementary Appendix 6).
Germination under a Martian radiation dose did not significantly differ from germination in a non-radiation environment for both rye and cress. The Control and Martian germination percentages were all above 96%. Only for some specific SEP radiation treatments germination of rye dropped below 90%. These were not statistically different from other treatments, also due to the small number of seeds (n = 12, p = 0.01) used for the SEP treatments. Experiments with more seeds are required to detect germination differences.
Visible Coloring and Malformation of Leaves
For both rye and cress, remarkable malformations and coloring of leaves were observed for irradiated plants. Malformation included divergent leaf form (Figure 3A) and lagging of length growth without malformation of leaves (Figure 3B). Coloring was mainly observed at leaf tips for both crops (Figures 3C,D). Colors varied from light green to yellow or brown and necrosis varied from 1 mm to approximately 2 cm. Coloring and malformation occurred for all radiated plants on both Earth potting soil and Mars regolith simulant. No malformations or colorings were observed in the non-irradiated pots.
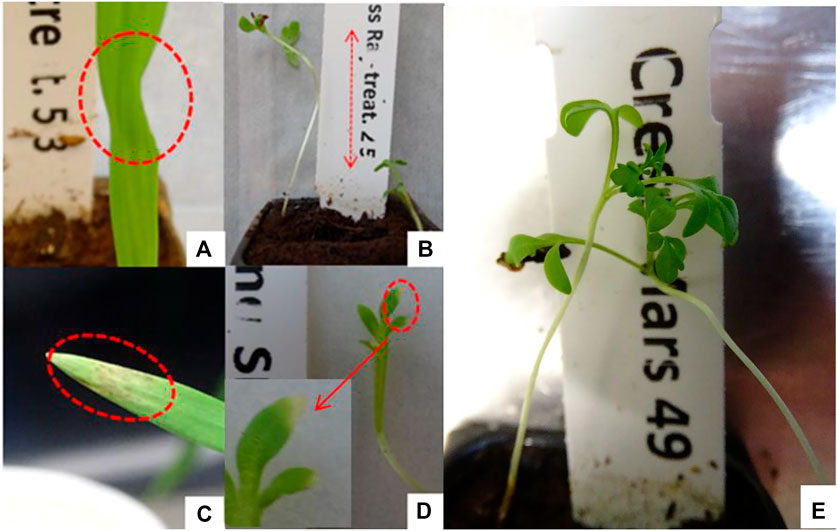
FIGURE 3. Pictures of individual plants showing the observed malformations in rye (A) and cress (B) and the abnormal coloring of leaves for both rye (C) and cress (D). (E) gives ‘normal’ non-irradiated cress plants on Mars regolith simulant.
Dry Weight
For rye, the highest mean DW was found for the Control Earth (CE) treatment, 0.0414 g (n = 12, Figure 4A and Supplementary Appendix 7A). This mean differed significantly (p = 0.000) from the Control Mars (CM) treatment mean of 0.0215 g. DW of both CE and CM differed significantly with treatments T1-T5 and NSEP (Supplementary Appendix 7A). Treatment Earth (CE) differed significantly with all other treatments except CM. The treatments T1-T5 and NSEP did not differ significantly among each other.
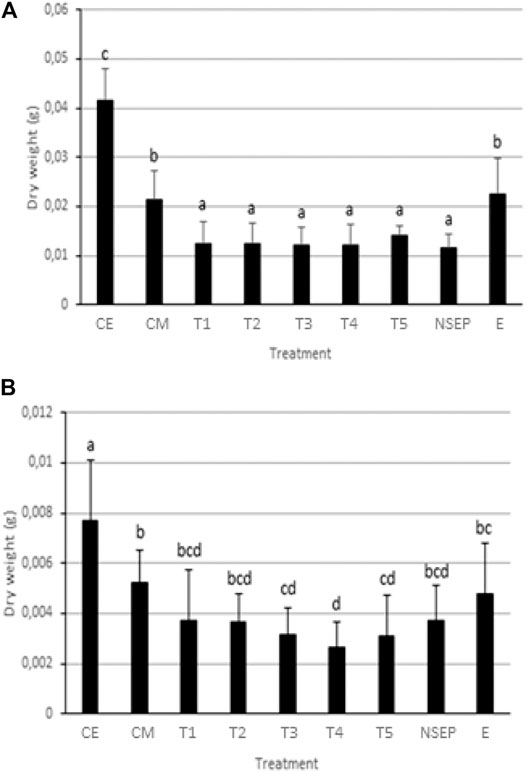
FIGURE 4. Mean dry weight (DW) of Secale cereale (rye) (A) and Lepidium sativum (cress) (B) in different treatments, with n = 12 per treatment. CE = Control Earth (Potting soil), CM = Control Mars regolith simulant, T1 = SEP 5 days before sowing, T2 = 4 days after sowing (a.s.), T3 = 7 days a. s., T4 = 14 days a. s., T5 = 21 days a. s., NS = No SEP, E = Earth (Potting soil), No SEP. Significant differences (p < 0.01) between treatments are indicated by different letters plotted above the bars. Error bars represent positive standard errors of the treatment mean.
For cress, the mean DWs of CE (0.00767 g) and CM (0.00523 g) differed significantly (n = 12 per treatment, p < 0.01, Figure 4B and Supplementary Appendix 7B). All radiation treatments differed significantly from the CE treatment, but only T3, T4 and T5 did differ significantly from the CM treatment. The lowest treatment mean, 0.00264 g, was found in the T4 treatment and differed significantly from CE, CM and E. No significant difference was found among E, CM and the radiation treatments (Supplementary Appendices 7B, 8).
Discussion
Germination
The results we obtained are in agreement with germination experiments of Hui et al. (2017). They showed that wheat seeds (Triticum aestivum L.) irradiated with doses varying from 646 to 1,060 μGy s−1, displayed germination percentages varying from 96 to 99%. An explanation for this high germination percentage can be found in the upregulation of the reactive oxygen species (ROS) scavenging processes. Under natural conditions, these processes are needed to compensate for the negative effects of the increased ROS production, inherent to the reactivation of the metabolic system during germination (Bailly, 2004). The ROS formed due to IoR can be scavenged by these processes as well, limiting the negative effects of IoR on plants. For Arabidopsis thaliana and Raphanus sativus seeds it was shown that for IoR up to 1 kGy almost all seeds germinated. Above this dose scavenging of ROS was not efficient enough to protect the seeds against the high concentrations of ROS (Kumagai et al., 2000). Since the maximum total dose obtained by seeds in our experiment was ±35 mGy, our results are in agreement with these observations.
Physiological and Morphological Reactions
The continues low dose radiation showed leaf malformation, leaf coloring and dwarf growth for irradiated individual plants. Tobacco plants exposed to relative low doses showed that malformation was more severe for the lowest doses compared to higher doses (Koeppe et al., 1970). At higher doses cessation of growth was observed, explaining the lack of new malformed leaves. Low chronic doses of gamma-photons also influenced leaf shape and coloring in Curcuma alismatifolia. A possible explanation may be that the accumulation of ROS induces more double strand breakage, leading to genetic chimerism in the plant (Taheri et al., 2016). In future experiments, chronic low dose radiation experiments should therefore include ROS-concentration measurements.
Leaf coloring in both rye and cress may have been caused by IoR damaging the photosynthetic system. Rice leaves exposed to a very low dose of 5.34 μGy d−1 for 3 or 4 days already showed a decrease of greenness of the leaves (Rakwal et al., 2009). Moreover, stress-related accumulation of two major rice phytoalexins, sakuranetin and momilactone A, was shown by Rakwal et al. (2009). Those phytoalexins are involved in the regulation of the ROS concentration in leaves and may protect the plant against the negative consequences of ROS. Related phytoalexins are, as well, involved in the initiation process of apoptosis (Pervaiz, 2004), which may explain the brown necrosis observed in our experiment. ROS mostly occurs at higher doses of radiation, in our case only for the SEP events. A low dose of ionizing radiation normally produces too few reactive oxygen particles to directly affect antioxidant concentrations in cells (Smith et al., 2012).
Dry Weight
The significant decrease of DW for both crops under IoR conditions is unexpected since earlier experiments did not show detrimental effects on plant growth and development with doses even higher than the doses in our experiment (part of the difference could be due to the set-up, giving differences in temperature, see below). Sheppard et al. (1982) showed that chronic exposure of Pinus sylvestris L. did not influence total DW of seedlings at doses till 0.7 mGy h−1. Severe impairment of growth and total biomass was only observed at a dose rate of 7.0 mGy h−1, which is more than 600 times higher compared to the dose rate of 0.011 mGy h−1 in our experiment. However, results comparable with our experiment were reported by Chandorkar and Clark, (1986). They showed that photosynthesis of Pinus strobus L. and Pinus sylvestris L. seedlings exposed for 150 days to gamma dose rate of 0.1015 mGy h−1 was reduced by 16–19%. Also, the respiration rate was lowered by about 14–23% and the amount of 80%-ethanol soluble sugar content decreased with 14–25%. Overall results included smaller seedlings with a decreased weight and a more compact phenotype (i.e., plants were more compact). Both were also observed in our experiment.
Temperature, Humidity and Light
Due to radiation safety regulations the control was placed in a separate room. This resulted in temperature differences between the control experiments and the radiation experiment. Overall differences in humidity were not significant (50 ± 8% and 47 ± 3%). Differences for temperature were significant (20.5 ± 0.4°C and 18.0 ± 0.8°C, p < 0.001). The temperature differences may significantly influence the total DW accumulated by plants due to the increased metabolic speed at higher temperatures (Berry & Björkman, 1980). Optimum growth temperature for rye, as given by the FAO (Acevedo et al., 2002), is between 12 and 25 C. According to experiments carried out by Yamori et al. (2006) a deviation of 2.5 C causes a decrease of photosynthesis of 11.6% at most.
There may be an effect of temperature on the growth in our experiment resulting in lower DW. But it is uncertain if it can explain the differences found in this experiment. The effect of temperature and air flow in the fume hoods may also influence moisture content of the soil/simulant and thus the growth. In a later conducted experiment under the same circumstances, it showed that there was almost no difference in moist content of the soil and simulant for both fume hoods (see further Pouwels, 2019). Air flow may also significantly influence the photosynthesis rate (Kitaya et al., 2012), the effects are not as such monitored in this project.
The used LED-lights yielded 42 µmol/m2/s at the pot level. This is rather low for the standards in 2021. The panels were chosen also because they had to be fit in the lead walls to contain the radiation. This was at the moment of the experiment (2017) the optimal LED panel. Nowadays smaller and more powerful LED lights are available and that would be our choice. Nevertheless, the less powerful lights may have influenced the growth of the plants and looks to be visible in the elongated growth of the garden cress. All treatments had the same light conditions and most likely the results of the experiment will not be influenced. But, an interaction between treatment and light intensity can not be excluded.
Radiation Conditions
The intended daily radiation was to 233 ± 12 μGy d−1, as measured by the Mars Curiosity Rover between 15 November and 15 January in the Gale crater (Matthiä et al., 2017). The thermoluminescence dosimeters (TLDs) showed that the average dose in our experiment was 270.5 μGy d−1. This implies that during the 26 experimental days plants received ±975 μGy more than on average on Mars.
Moreover, the dose to which our plants were exposed was obtained by employing high-energetic 60Co γ-photons. This neglects the composition of the Martian radiation spectrum, consisting of 85–90% protons, 10–13% of He-ions, 1% of electrons and 1% of heavier nuclei (Hassler et al., 2013). The lack of high energetic particle radiation may have caused the damage observed in the plants to be less severe than can be expected at the Martian surface. Especially, since plants appear to be less resistant to particle radiation compared to photon radiation, due to local energy disposure (Hase et al., 2017). Plants may partly be protected against those particles by using polymers rich in low Z-materials, such as hydrogen (Nambiar & Yeow, 2012). However, such materials are not sufficient to protect against the highly energetic γ-photon rays present, which may cause a significant decrease in DW, as shown in our experiment.
The DW decrease we showed as a result of the Martian radiation dose is a major concern for future civilization on Mars, since this indicates that crop growth at the Martian surface, indoors in a greenhouse, may not be very successful. Yield will be limited resulting in the need for large-area cultivation, which is economically and technically difficult, due to the likely scarce resources, e.g., construction materials and essential plant resources. Therefore, crop cultivation is most likely to succeed at locations under the Martian surface where the Martian regolith will provide protection against the galactic cosmic rays (GCR) and the SEP-events.
Conclusion
Crops grown at the Martian surface will constantly be exposed to a radiation dose 17 times higher compared to Earth. This dose of Martian radiation, simulated with 60Co γ-photons, could have a significant negative effect on two crop species, rye and garden cress. During the first 4 weeks after germination biomass production was almost halved for both cress and rye and visible coloring of the leaves for both crops was observed.
Germination was not significantly influenced by long-term exposure to ionizing radiation. A temporary increase in the dose of radiation, in order to simulate Martian SEP-events, at different developmental stages of the young plants did not significantly influence the final dry weight of the crops.
Data Availability Statement
The original contributions presented in the study are included in the article/Supplementary Material, further inquiries can be directed to the corresponding authors.
Author Contributions
NT did day to day work and wrote the MS. GW, AD, MS, HH and HW overviewed the experiment and reviewed the MS. GW and MS were direct supervisors. GW designed the original set up and came up with the idea. PG carried out the statistics.
Conflict of Interest
The authors declare that the research was conducted in the absence of any commercial or financial relationships that could be construed as a potential conflict of interest.
Publisher’s Note
All claims expressed in this article are solely those of the authors and do not necessarily represent those of their affiliated organizations, or those of the publisher, the editors and the reviewers. Any product that may be evaluated in this article, or claim that may be made by its manufacturer, is not guaranteed or endorsed by the publisher.
Acknowledgments
The authors want to express their special thanks to the staff of the Reactor Institute Delft (RID) for their supporting activities over the course of the experiment. Special thanks are given to the Education and Training Centre of NCSV and the section of Applied Radiation and Isotopes (ARI), with attention to F.D.P. Geurink, for their unconditional support and fruitful remarks. Special thanks to ing. K.A. Kamman of the Dienst Elektronische en Mechanische Ontwikkeling (DEMO) for the development of the experimental frames. Thanks to M. Blaauw for his statistical explanations on the results and to the StralingsBeschermingsDienst (SBD) for the control of the radiation sources used during the experiments. Part of this research was financed via crowdfunding. Thank you to three reviewers for improving the manuscript.
Supplementary Material
The Supplementary Material for this article can be found online at: https://www.frontiersin.org/articles/10.3389/fspas.2021.665649/full#supplementary-material
References
Acevedo, E., Silva, P., and Silva, H. (2002). Wheat growth and physiology. (last visited on 15-6-2021). http://www.fao.org/3/y4011e/y4011e06.htm.
Bailly, C. (2004). Active oxygen species and antioxidants in seed biology. Seed Sci. Res. 14, 93–107. doi:10.1079/SSR2004159
Benton, E. R., and Benton, E. V. (2001). Space radiation dosimetry in low-Earth orbit and beyond. Nucl. Instr. Methods Phys. Res. Section B: Beam Interactions Mater. Atoms 184, 255–294. doi:10.1016/s0168-583x(01)00748-0
Berry, J., and Björkman, O. (1980). Photosynthetic response and adaptation to temperature in higher plants. Annu. Rev. Plant Physiol. 31, 491–543. doi:10.1146/annurev.pp.31.060180.002423 Site: www.annualreviews.org.
Caldecott, R. S. (1955). The Effects of X-rays, 2-Mev Electrons, Thermal Neutrons, and Fast Neutrons on Dormant Seeds of Barley. Ann. N.Y Acad. Sci. 59, 514–535. doi:10.1111/j.1749-6632.1955.tb45967.x
Chandorkar, K. R., and Clark, G. M. (1986). Physiological and morphological responses of Pinus Strobus L. and Pinus Sylvestris L. seedlings subjected to low-level continuous gamma irradiation at a radioactive waste disposal area. Environ. Exp. Bot., 26, 259–270. doi:10.1016/0098-8472(86)90038-9
Guo, J., Slaba, T. C., Zeitlin, C., Wimmer-Schweingruber, R. F., Badavi, F. F., Böhm, E., et al. (2017). Dependence of the Martian radiation environment on atmospheric depth: Modeling and measurement. J. Geophys. Res. Planets 122, 329–341. doi:10.1002/2016JE005206
Hase, Y., Nozawa, S., Narumi, I., and Oono, Y. (2017, Effects of ion beam irradiation on size of mutant sector and genetic damage in Arabidopsis, Nucl. Instr. Methods Phys. Res. Section B: Beam Interactions Mater. Atoms). Nuclear Instruments and Methods in Physics Research Section B: Beam Interactions with Materials and Atoms. Elsevier, 391, 14–19. doi:10.1016/j.nimb.2016.11.023
Hassler, D. M., Zeitlin, C., Wimmer-Schweingruber, R. F., Ehresmann, B., Rafkin, S., Eigenbrode, J. L., et al. the MSL Science Team (2013). Mars' surface radiation environment measured with the Mars Science Laboratory's Curiosity rover. Science 343, 1244797. doi:10.1126/science.1244797
Horneck, G., Facius, R., Reichert, M., Rettberg, P., Seboldt, W., Manzey, D., et al. (2006). HUMEX, a study on the survivability and adaptation of humans to long-duration exploratory missions, part II: Missions to Mars. Adv. Space Res., 38, 752–759. doi:10.1016/j.asr.2005.06.072
Hui, L., Dawei, H., Chen, D., Yuming, F., uanghui, L., Youcai, Q., et al. (2017). Low-dose ionizing radiation limitations to seed germination: Results from a model linking physiological characteristics and developmental-dynamics simulation strategy. J. Theor. Biol., 427, 10–16. doi:10.1016/j.jtbi.2017.05.024
ICRP (2013). Assessment of Radiation Exposure of Astronauts in Space: Annals of the ICRP. London: ICRP Publication. 123, 42, pp. 33–48.
Jakosky, B. M., and Phillips, R. J. (2001). Mars' volatile and climate history. Nature 412, 237–244. doi:10.1038/35084184
Jun, I., Swimm, R. T., Ruzmaikin, A., Feynman, J., Tylka, A. J., and Dietrich, W. F. (2007). Statistics of solar energetic particle events: Fluences, durations, and time intervals. Adv. Space Res., 40, 304–312. doi:10.1016/j.asr.2006.12.019
Kianian, P. M. A., Liberatore, K. L., Miller, M. E., Hegstad, J. B., and Kianian, S. F. (2016). “Dissecting Plant Chromosomes by the Use of IR,” in Plant Cytogenetics. Methods In Molecular Biology, 1429. Editors S. Kianian, and P. Kianian (New York, NY: Humana Press).
Kitaya, Y., Hirai, H., and Shibuya, T. (2010). Important Role of Air Convection for Plant Production in Space Farming. Biol. Sci. Space 24, 121–128. doi:10.2187/bss.24.121
Koeppe, D. E., Rohrbaugh, L. M., Rice, E. L., and Wender, S. H. (1970). The effect of X-radiation on the concentration of scopolin and caffeoylquinic acids in tobacco. Radiat. Bot., 10, 261–265. doi:10.1016/S0033-7560(70)80019-9
Kumagai, J., Katoh, H., Kumada, T., Tanaka, A., Tano, S., and Miyazaki, T. (2000). Strong resistance of Arabidopsis thaliana and Raphanus sativus seeds for ionizing radiation as studied by ESR, ENDOR, ESE spectroscopy and germination measurement: Effect of long-lived and super-long-lived radicals. Radiat. Phys. Chem., 57, 75–83. doi:10.1016/S0969-806X(99)00306-0
Maggi, F., and Pallud, C. (2010). Space agriculture in micro- and hypo-gravity: A comparative study of soil hydraulics and biogeochemistry in a cropping unit on Earth, Mars, the Moon and the space station. Planet. Space Sci. 58, 1996–2007. doi:10.1016/j.pss.2010.09.025
Matthiä, D., Hassler, D. M., de Wet, W., Ehresmann, B., Firan, A., Flores-McLaughlin, J., et al. (2017). The radiation environment on the surface of Mars - Summary of model calculations and comparison to RAD data. Life Sci. Space Res., 14, 18–28. doi:10.1016/j.lssr.2017.06.003
Nambiar, S., and Yeow, J. T. W. (2012). Polymer-Composite Materials for Radiation Protection. ACS Appl. Mater. Inter. 4, 5717–5726. doi:10.1021/am300783d
Nicholson, W. L., Schuerger, A. C., and Setlow, P. (2005). The solar UV environment and bacterial spore UV resistance: considerations for Earth-to-Mars transport by natural processes and human spaceflight. Mutat. Research/Fundamental Mol. Mech. Mutagenesis, 571, 249–264. doi:10.1016/j.mrfmmm.2004.10.012
Pervaiz, S. (2004). Chemotherapeutic potential of the chemopreventive phytoalexin resveratrol. Drug Resist. Updates, 7, 333–344. doi:10.1016/j.drup.2004.11.001
Pouwels, C. (2019). A radiation research on Co-60 irradiated cress on Mars simulant soil. Netherlands: Reactor Institute Delft. Thesis report. Wageningen Environmental Research.
Rakwal, R., Agrawal, G. K., Shibato, J., Imanaka, T., Fukutani, S., Tamogami, S., et al. (2009). Ultra Low-Dose Radiation: Stress Responses and Impacts Using Rice as a Grass Model. Ijms 10, 1215–1225. doi:10.3390/ijms10031215
Sheppard, M., Thibault, D., and Guthrie, J. (1982). Radiosensitivity and the role of Pinus sylvestris L. in the revegetation of radioactive disposal areas. Environ. Exp. Bot., 22, 193–198. doi:10.1016/0098-8472(82)90038-7
Smith, J. T., Willey, N. J., and Hancock, J. T. (2012). Low dose ionizing radiation produces too few reactive oxygen species to directly affect antioxidant concentrations in cells. Biol. Lett. 8, 594–597. doi:10.1098/rsbl.2012.0150
Taheri, S., Abdullah, T. L., Abdullah, T. L., Ahmad, Z., Sahebi, M., and Azizi, P. 2016). Phenotypic and molecular effects of chronic gamma irradiation on Curcuma alismatifolia. Europ.J.Hortic.Sci., 81, 137–147. doi:10.17660/eJHS.2016/81.3.1
UNSCEAR (2008). Sources and effects of IR (V.16-02682 (E)). United Nations Scientific Committee on the Effects of Atomic Radiation. New York, NY: United Nations.
Van Hoeck, A., Horemans, N., Nauts, R., Van Hees, M., Vandenhove, H., and Blust, R. (2017). Lemna minor plants chronically exposed to ionising radiation: RNA-seq analysis indicates a dose rate dependent shift from acclimation to survival strategies. Plant Sci., 257, 84–95. doi:10.1016/j.plantsci.2017.01.010
VSN International (2020). Genstat for Windows. 21st Edition. Hemel Hempstead, UK: VSN International. Genstat.co.uk.
Wamelink, G. W. W., Frissel, J. Y., Krijnen, W. H. J., and Verwoert, M. R. (2019). Crop growth and viability of seeds on Mars and Moon soil simulants. Open Agric. 4, 509–516. doi:10.1515/opag-2019-0051
Wamelink, G. W. W., Frissel, J. Y., Krijnen, W. H. J., Verwoert, M. R., and Goedhart, P. W. (2014). Can Plants Grow on Mars and the Moon: A Growth Experiment on Mars and Moon Soil Simulants. Plos One, 9, e103138. doi:10.1371/journal.pone.0103138
Yamori, W., Suzuki, K., Noguchi, K., Nakai, M., and Terashima, I. (2006). Effects of Rubisco kinetics and Rubisco activation state on the temperature dependence of the photosynthetic rate in spinach leaves from contrasting growth temperatures. Plant Cel Environ 29, 1659–1670. doi:10.1111/j.1365-3040.2006.01550.x
Keywords: radiation, Mars, Secale cereale, rye, Lepidium sativum, garden cress, regolith, food
Citation: Tack N, Wamelink G, Denkova A, Schouwenburg M, Hilhorst H, Wolterbeek H and Goedhart P (2021) Influence of Martian Radiation-like Conditions on the Growth of Secale cereale and Lepidium sativum. Front. Astron. Space Sci. 8:665649. doi: 10.3389/fspas.2021.665649
Received: 08 February 2021; Accepted: 13 July 2021;
Published: 02 August 2021.
Edited by:
Cyprien Verseux, University of Bremen, GermanyReviewed by:
Ricardo Amils, Autonomous University of Madrid, SpainLucie Poulet, NASA Postdoctoral Program, United States
Copyright © 2021 Tack, Wamelink, Denkova, Schouwenburg, Hilhorst, Wolterbeek and Goedhart. This is an open-access article distributed under the terms of the Creative Commons Attribution License (CC BY). The use, distribution or reproduction in other forums is permitted, provided the original author(s) and the copyright owner(s) are credited and that the original publication in this journal is cited, in accordance with accepted academic practice. No use, distribution or reproduction is permitted which does not comply with these terms.
*Correspondence: G.W.W. Wamelink, wieger.wamelink@wur.nl; A.G. Denkova, A.G.Denkova@tudelft.nl