Multi-instrumental detection of a fireball during Leonids of 2019
- 1Institute of Earth Physics and Space Science (ELKH EPSS), Sopron, Hungary
- 2Doctoral School of Earth Sciences, ELTE Eötvös Loránd University, Budapest, Hungary
- 3Department of Ionosphere and Aeronomy, Institute of Atmospheric Physics, Czech Academy of Sciences, Prague, Czechia
During the 2019 Leonid meteor shower, the European Fireball Network recorded a bright fireball (meteor code: EN171119_041459) at 04:15:0.2 (UT) on 17 November 2019. The fireball appeared at coordinates 49.95°N 15.56°E at the height of 134.46 km, and disappeared at coordinates 50.23°N 15.26°E and at the height of 71.81 km. The ionization effect caused by the fireball appeared in the digisonde’s campaign measurements taken with a 2 ionogram/min time resolution at Průhonice station (50.00°N, 14.60°E). The trace appeared on the ionograms as a faint sporadic E-like layer, and the maximum ionization reached the upper limit of the measurement, 17 MHz. The trace persisted for 20 min on the ionograms, first appearing at 04:15:40 (UT) and finally disappearing at 04:35:40 (UT). The virtual height of the trace according to the ionograms appeared between 114 and 142 km, first it descended and then it ascended. Drift measurements were also taken with the digisonde every minute. Between 04:19:20 and 04:35:20 (UT), between altitudes of 122–142 km, 1-5 reflections were recorded on most SkyMaps. In addition, the Continuous Doppler Sounding developed by the Institute of Atmospheric Physics CAS also recorded the ionization signature of the fireball between 04:18 and 04:30 (UT) on 2 of the 3 sounding paths operating at 4.65 MHz. This is the first evidence that the plasma trail of a documented fireball can be detected by a DPS-4D digisonde (not only on ionograms, but also by drift measurements) and by the Continuous Doppler Sounding system.
1 Introduction
The Leonid meteor swarm is one of the best-known meteor falls, associated with the comet 55P/Temple-Tuttle (Yeomans et al., 1996). It occurs between 6 November and 30 November and usually peaks on 17 November. The Leonids are a fast-moving swarm that reaches and enters the Earth’s atmosphere at an average geocentric speed of 71 km/s (Beech, 1998) and have an average size of 10 mm (Höffner et al., 1999). Leonid meteors which are visible to the naked eye have an average mass of 0.5 g and can usually generate an average brightness of −1.5 apparent magnitude (Hawkes, 2007).
A meteoric fireball or bolide, as defined by the International Astronomical Union (IAU) in 2017, is a meteor with a brightness greater than −4 absolute visual magnitude. The difference between a fireball and a bolide is that the bolide is the special fireball that explodes in a terminal flash and often visual fragmentation can be observed. On average every thousandth meteor entering the Earth’s atmosphere is a fireball. Above −17 absolute visual magnitude these bodies are called superbolides (Ceplecha et al., 1999; Di Martino and Cellino, 2004).
When meteors enter the Earth’s atmosphere, the vast majority of them burn up due to the friction and compression, and spread their material over an altitude of 70–110 km (Kozlovsky et al., 2020). Spectral analyses show that in the case of the Leonids, the meteors’ material is composed of atomic O and N, as well as Mg, Fe, Na and Ca, which are ionized at high temperatures (Borovička et al., 1999).
This meteoric dust and metallic material deposited in the lower atmosphere can form thin layers of ionization by vertical ion convergence. That trapping mechanism is created by the combined effect of the vertical shear of the zonal winds, the meridional winds and the Lorentz force controlling the movement of ions due to the local magnetic field. This ion convergence creates the phenomenon known as the sporadic E layer (Whitehead, 1961; Axford, 1963; Haldoupis, 2011). At middle latitudes this is the accepted theory of formation of sporadic E layers so far and is usually referred to as the wind shear theory.
The most commonly used meteor detection methods are optical observations (Koten et al., 2019) and meteor radars (Fukao and Hamazu, 2014; Chen et al., 2020), but during major meteor falls, echo traces were often observed on ionograms, which were classified into separate categories (Ellyett and Goldsbrough, 1976). However, this classification led to controversy, which was finally resolved by Maruyama et al. (2003). In the study, these echo traces are attributed to Fresnel scattering by increased plasma density in the meteor’s trail supported by extensive analysis and visual observations.
In summary, this means that it is possible to detect meteors with ionosondes (Kereszturi et al., 2021) because of the ionization they cause. These traces have a generally short lifetime and usually have an ionization (maximum frequency on the ionograms) exceeding that of regular sporadic E layers (in the Maruyama et al. (2003) the traces accepted as meteors are those above 5 MHz), and also different in height (Maruyama et al., 2003). They often show a distinct direction on ionograms made by Digisonde DPS-4D type of ionosonde (usually referred as digisonde), unlike the vertical reflection of the regular sporadic E layers.
The first observation of the ionization effect of a fireball event was on 28 January 1976 (Rajaram and Chandra, 1991). The Dhajala fireball passed close to Ahmedabad ionosonde station (23.2°N, 72.30°E) at 20:40 (IST–India Standard Time, UTC+5:30 h). The trace persisted for 1 h and showed an ionization of 3.4 MHz. Also mentioned in the article is that the trace breaks up into 2–3 distinct layers at altitudes of 120–140 km, separated by 6 km.
During the 2001 Leonid meteor shower on 17 November 2001, a trace appeared over Kokubunji/Tokyo (35.71°N, 139.49°E) that persisted for nearly 40 min (20:25 UTC first appearance, 21:00 UTC last appearance, Maruyama et al., 2003) and reached a top frequency of nearly 30 MHz, while its height steadily decreased over time. In the article it was called a long-duration meteor event (LDME). In 2002, during the Perseids, on 11 August at 01:50 (JST–Japanese Standard Time, UTC+9 h), another so called LDME appeared over Yamagawa (31.20° N, 130.62° E) at an apparent height of 120 km (Maruyama et al., 2008), with a top frequency of 27 MHz. It disappeared permanently at 02:47 (JST). However, its height-changes were different from the 2001 Leonids event–first decreasing and then increasing in apparent height.
On 4 December 2020 at 13:30 (UTC), a fireball was detected by several different instruments (including two cameras and an ionosonde) over northern Sweden, which has been named the Pajala fireball and is believed to have originated from the Northern Taurids. On the ionogram (captured by Sodankylä Geophysical Observatory, 67.36°N, 26.63°E), the trace appears at 13:31:07 (UTC) and persists for about 30 min as a sporadic E-like pattern that reached its maximum measurement frequency of 16 MHz at the time of its appearance and the frequency did not drop below 10 MHz until about 10 min after the trace appeared (Vierinen et al., 2022). And finally, on 17 January 2009, a fireball named Maribo was detected over Juliusruh, captured by a meteor radar operating at 32.5 MHz (Schult et al., 2015).
Due to the 2019 Leonids meteor shower, 2 ionograms/minute digisonde campaign measurements took place in both Průhonice and Sopron stations on 16, 17 and 18 November, starting after sunset and ending before sunrise. During the campaign measurement, on 17 November 2019 at 04:15:40, a trace was recorded by the digisonde of Průhonice (50.00°N, 14.60°E) that persisted for 20 min and showed high ionization levels. Based on previous studies, it was assumed that this was the trace of a fireball or a bolide. It being a fireball was confirmed by the Department of Interplanetary Matter of Astronomical Institute of Czech Academy of Sciences.
The group of Meteor physics at Department of Interplanetary Matter of Astronomical Institute of Czech Academy of Sciences observes meteors and performs theoretical interpretations of the observations. The Czech Fireball Network belongs to the European Fireball Network. The main instrument on every Czech station is the Digital Autonomous Fireball Observatory (Spurný et al., 2017; Borovička et al., 2019). The observational data are used to study physical processes during the penetration of meteoroids into Earth’s atmosphere, including radiation and ionization. The meteorite’s fall was observed in detail by Czech Fireball Network sensitive fireball cameras. The basic observational system is the European Fireball Network (Borovička et al., 2022).
The main purpose of this article is to describe and study the fireball which appeared near Prague, as detected by the Czech Fireball Network’s fireball cameras, the digisonde of Průhonice and the Continuous Doppler Sounding system. Measurements from the digisonde of Sopron were used as a reference. The secondary purpose of this article is to assess the potential of the digisonde in these kind of studies. Meteor radars usually operate at frequencies between 30 and 40 MHz, while the digisonde measures between 1 and 17 MHz. Can this be used for meteor detection? European digisonde stations measure with a time resolution of 5, 10 or 15 min. The most commonly used 5-min time resolution may be suitable for statistical studies, but is it possible to detect more meteors with high cadence campaign measurements? Can it be used to determine the geographic directions of meteorite trails? Do the Skymaps derived from the drift measurements of the digisonde show traces of meteors? These questions have never been studied, yet.
In this paper, we will first discuss the data collection methods, possibly the settings of these instruments, summarize the observations, and then compare it with a regional phenomenon before the discussion and the conclusions.
2 Methods and data
2.1 Astronomic detection methods
The main instrument on every Czech station belongs to the European Fireball Network is the Digital Autonomous Fireball Observatory (Spurný et al., 2017; Borovička et al., 2019). There are cameras providing very accurate fireball recordings. These observations are only made at night and also during the darker parts of dusk and dawn.
The highest meteors can be captured by the all-sky camera at a height of 199 km. Special behavior of fireballs can be observed from an initial height above 130 km (Koten et al., 2006; Table 1.)

TABLE 1. Initial altitudes and numbers of meteor fireballs with an initial high-altitude above 130 km in the period of years 1999–2001, (Koten et al., 2006). The Leonid meteor swarm reaches its maximum number of meteors about every 33 years, often referred to as an “outbreak”. The last one was in 2002, but 2001 also produced high numbers as shown in the table (Molau et al., 2002).
As mentioned in Spurný et al. (2000) when these meteors reach an altitude of about 130 km, their shape quickly changes to a typically drop-like form. Leonid meteor showers are typified by production meteors with a higher beginning height. The high-altitude fireballs were observed during several observations of the Leonids Multiinstrument Aircraft Campaign (MAC) in the years 1998–2001. Table 1 shows the number of observed Leonid fireballs in this period by individual height, by (Koten et al., 2006).
The observation of radio reflections from meteor trails is not rare. There is no reliable data available for the mesospheric conditions for the observed period. Therefore, in this case it is rather difficult to infer a distortion of the ionized meteor trail. This is precisely why it is very useful to track these reflections multi-instrumentally.
2.2 Digisonde (ionogram and SkyMap)
Using vertical sounding, the ionosphere reflects electromagnetic waves at a particular height at which the plasma frequency corresponds to the transmitted signal. The plasma frequency is proportional to the electron density (Eq. (1).) (Davies, 1990). An ionosonde emits electromagnetic pulses and measures time of flight of the reflected electromagnetic waves. The stratification, electron content and virtual height of the ionospheric layers can be scanned when the frequency of the sounding signal reaches the plasma frequency.
Typically, frequencies between 1 and 20 MHz are used by the ionosondes, which allows the observation of the E and F layers. A given layer will reflect the electromagnetic signals back till the critical plasma frequency of that layer (Eq. (1)). The critical frequency is the frequency at which the sounding frequency reaches the maximum plasma frequency within the layer–here, depending on the sentiment, it can be considered that the energy of the electromagnetic wave is absorbed or that the wave’s travel time becomes infinite. Signals above this frequency are reflected from a layer with higher electron density situated above that or pass through the ionosphere without reflection.
The equation for the plasma frequency is
where ωpe is the electron plasma frequency (Hz), ne is electron density (number of electrons/m3), e is the electron charge (1.609•10–19 C), m* is effective mass of the electron (9.11•10–31 kg) and ε0 is the vacuum permittivity (8.85•10–12 F/m).
The result of such a measurement is the ionogram–on which the height-frequency values are plotted, and from which electron density profiles can be constructed. It should be noted that the height of the reflection derived directly from measurement corresponds to the situation when the electromagnetic wave travels through vacuum without any delay caused by local conditions. In case of the E and Es layer, the delay in time of flight is small and usually neglected, higher within the F layer the profile inversion is necessary.
A Digisonde DPS-4D station is installed at Průhonice (50.00°N, 14.60°E) and at Sopron (47.63°N, 16.72°E) (Figure 1.) and has been providing measurements since January 2004 in Průhonice and since June 2018 in Sopron as a part of the GIRO Network (Reinisch and Galkin, 2011). This type of ionosonde is able to sound not only vertically but also obliquely, which is made possible by the four additional receiver antennas surrounding the central vertical transmitter antenna (Reinisch, 1996; Reinisch et al., 2005; Reinisch et al., 2008). This arrangement allows the direction determination based on the phase-difference of the reflected signals observed at the different receiver antennas. After collecting and processing the raw data, the ionogram is produced, where the direction of the signals is indicated by the color code.
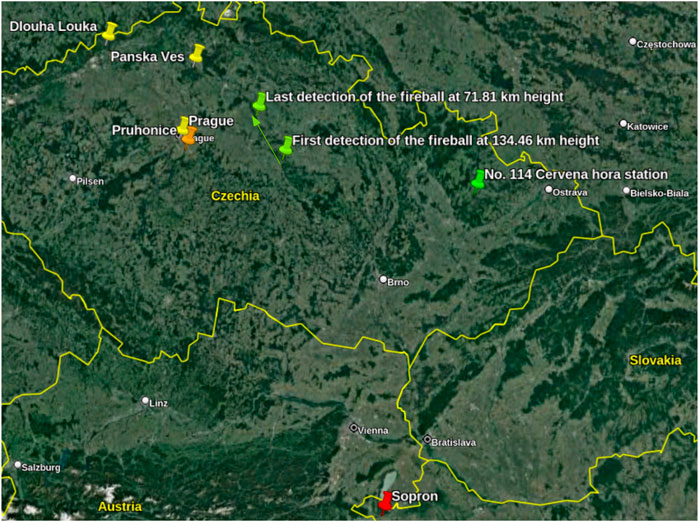
FIGURE 1. The locations of measurements, instruments and stations. The first and last detections of the fireball are marked lime green and an arrow showing projection of the recorded luminous trajectory of the fireball onto the Earth’s surface. The No. 114 Červená hora station which registered the fireball is marked bright green. The digisondes (Průhonice, Sopron) are marked orange and red respectively. The CDS transmitters (Průhonice, Panská Ves, Dlouhá Louka) and the CDS receiver (Prague) are marked with yellow. Since the digisonde and the CDS transmitter are located in Průhonice, the station is marked with orange.
The DPS-4D digisonde can also be used to perform drift measurements, which are usually timed after the measurements required for the ionogram, because the automatically scaled ionograms can provide the necessary parameters for the setup of the drift measurement (e.g., critical frequency for the E (foE) or F (foF2) layer). If the medium is moving, a Doppler-effect will occur in the signal propagation. The estimation of the drift velocity vector is made possible by determining the location of reflection points in the ionosphere (enabled by the antenna layout) and the Doppler frequency shift. The digisonde allows for automatically processed, real-time drift measurements. The final product of the measurements is three velocity vector components: vertical (vz), north (vn), and east (ve) directions. These are represented graphically in color-coded east-west and north-south planes by the so-called SkyMap.
2.3 Continuous Doppler Sounding
The Continuous Doppler Sounding (CDS) system was developed by the Institute of Atmospheric Physics CAS to study short time-scale ionospheric fluctuations (Laštovička and Chum, 2017; Chum et al., 2018; Kouba and Chum, 2018; Chum et al., 2021). The CDS instrument is suitable for fast identification of the wave-like phenomena within the ionosphere. It works on the principle of the electromagnetic wave reflection from the plasma with corresponding plasma frequency. For instance, up and down motion of the reflecting layer due to the atmospheric gravity waves causes Doppler-changes in the reflected signal. However, it does not provide data from which electron-density profiles can be derived and should be therefore completed by independent measurement.
It is worth noting that Doppler-shifts may not only be caused by the movement of the layer from which the radio waves reflect, but also, for example, by density changes caused by pressure waves (long-period infrasound) or solar flares (Chum et al., 2016; Chum et al., 2018).
The system consists of transmitters distributed across the western half of the Czech Republic and a receiver set up in Prague (Figure 1). Currently, the operation is on frequencies 3.59, 4.65 and 7.0 MHz. One transmitter is located directly next to the digisonde and is separated from the receiver by 7 km (Kouba and Chum, 2018).
3 Observations and results
3.1 Astronomic observations
A bright fireball was recorded by the European Fireball Network on 17 November 2019 after 04:15:0.2 UT (±0.10 s). The meteor code of this Leonid fireball is EN171119_041459 (Figure 2).
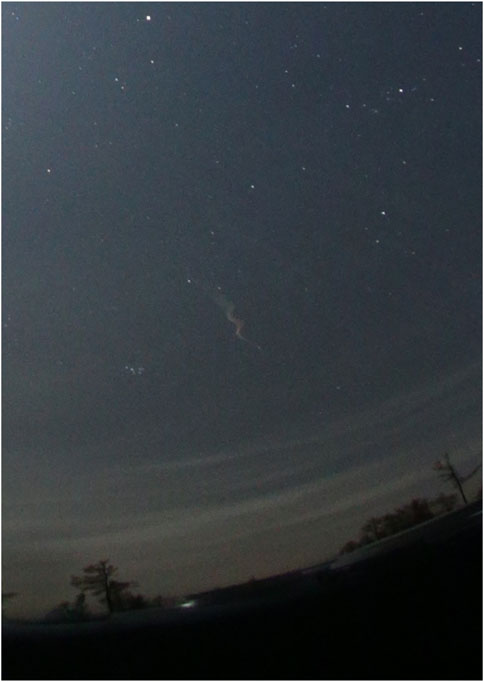
FIGURE 2. Optical image of the trail left by the fireball detected at 04:15:0.2 on 17 November 2019. The picture was taken at Červená hora station by the Czech Fireball Network betweeen 04:15:10 - 04:15:45 UT, the exposition time was 35 s.
The geographic coordinates of the captured starting point of the fireball are 49.957°N, 15.563°E, at 134.46 km height, and ending 50.237°N, 15.267°E, at 71.81 km height. Linear length of the trajectory was 73.44 km, velocity at the average trajectory point 71.18 km/s, and terminal velocity (at height 72.7 km) 70.49 km/s (Table 2). Geocentric right ascension was 153.60°, geocentric declination 21.80°, and maximum at Solar longitude 235.4°. Closest distance was 185.8 km to station No. 114 Červená hora (49.777°N, 17.542°E) with maximal convergence angle 71.7°.
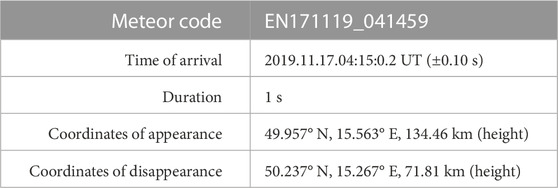
TABLE 2. A summary of the localization of the Leonid bolide above the Czech Republic, data were provided by P. Spurný (priv. comm.).
Maximum apparent magnitude of the observed fireball was −15.96 (at height 83.0 km), radiated energy 5.58•108 J, and maximum brightness point was at 50.186° N and 15.321° E, at 83.03 km height. The initial photometric mass of this meteor was primarily determined to be 1.448 kg (P. Spurný, priv. comm.).
Observation was carried out using 5 cameras from closest distance 185.8 km at the station No. 114 Červená hora (Figures 1, 2). The fireball was observed by the cameras of the European Fireball Network (Borovička et al., 2022) and the data were provided by P. Spurný (priv. comm.).
3.2 Ionogram and SkyMap
During the passage of the 2019 Leonid meteor swarm, on 16, 17, and 18 November, joint 1-min temporal resolution campaign measurements were launched in both Průhonice and Sopron digisonde stations (Figure 1) between 16:30:00 (UT) in the afternoon and 06:30:00 (UT) in the morning. 2 ionograms were taken every minute. The measurement was performed between 1.5 and 17 MHz. Furthermore, high resolution SkyMap (1/min) measurements at ∼ 2,500 kHz were also performed during the above-mentioned periods.
3.2.1 Ionograms
The first trace of the fireball appears on the ionogram taken at Průhonice station at 04:15:40 (UT) on 17 November 2019. The ionogram in front of it, taken at 04:15:00 (UT), shows no meteor traces. This agrees well with astronomical observations (Figure 2; Table 2).
The trace on the ionogram at 04:16:00 resembles the pattern of a sporadic E layer (Figure 3) similar to those described in other studies (Maruyama et al., 2003; Maruyama et al., 2008; Schult et al., 2015; Kereszturi et al., 2021; Vierinen et al., 2022). However, the trace differs in almost all respects from the regular sporadic E layer’s behavior (see Section 3.4), it was detected as a very thin layer up to high frequency range. For the months of November and December of 2019, analysis of ionograms from both Průhonice and Sopron stations shows that the median of the sporadic E frequency is 2.55 MHz for Průhonice station and 2.49 MHz for Sopron station, while the median of the height is 107.6 km for Průhonice station and 102.3 km for Sopron station. These data are based on 10406 ionograms processed for Průhonice station, with 20.2% sporadic E occurrence rate. For Sopron station, 14069 ionograms were processed, with 19.1% sporadic E occurrence rate.
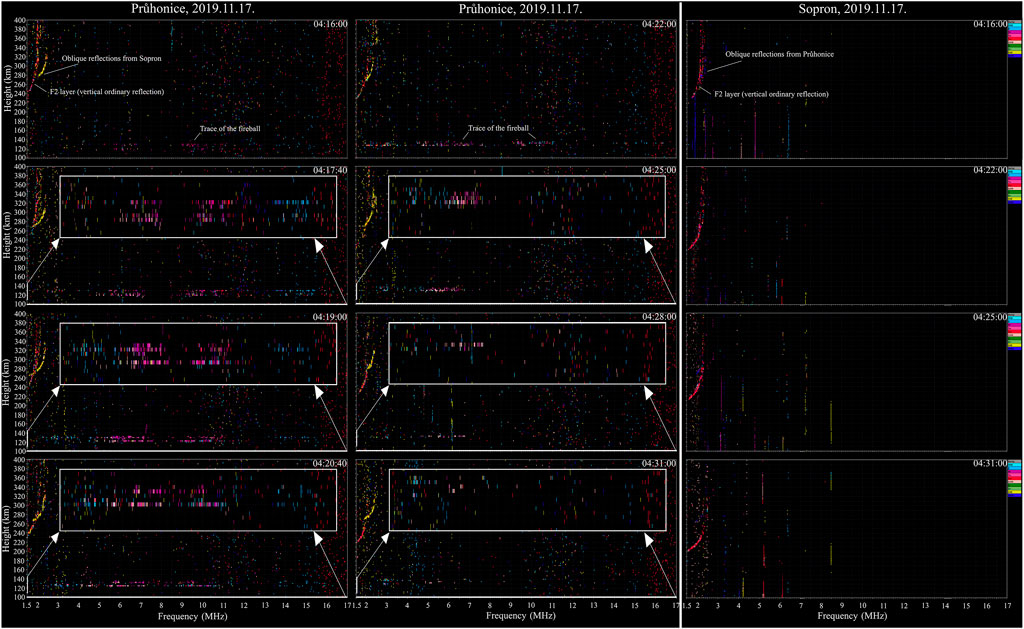
FIGURE 3. Selected ionograms from Průhonice and Sopron stations, between 1.5 and 17 MHz, at virtual heights between 100 and 400 km and at time between 04:16 - 04:31 (UT), taken at 6 dB MPA threshold. For a white background picture about the ionograms of Průhonice please see Supplementary Figure S1 and for pictures made at 4 dB MPA threshold please see Supplementary Figure S2. The ionograms from Průhonice station (left and middle column, left column between 04:16 - 04:21, middle column between 04:22 - 04:31) show the trace of the fireball-induced ionization and its evolution over time. The white rectangle shows the 110–145 km and 1.5–17 MHz zoomed-in part of the ionograms. In contrast, the ionograms of Sopron station used as reference do not show any similar phenomena, not even regular sporadic E, indicating the localisation of plasma trace related to the fireball in the near region of Průhonice station.
The maximum reflection frequency of the ionization caused by the 17 November 2019 Leonid fireball reached 17 MHz already at its first appearance on the ionograms (Table 3). It is very likely that the frequency is saturated at 17 MHz and the actual value of top frequency can be much higher. The ionization rate did not go below 10 MHz until 04:26:00 (Table 3). The last ionogram on which the fireball’s effect can still be clearly identified was taken at 04:35:40 (UT).
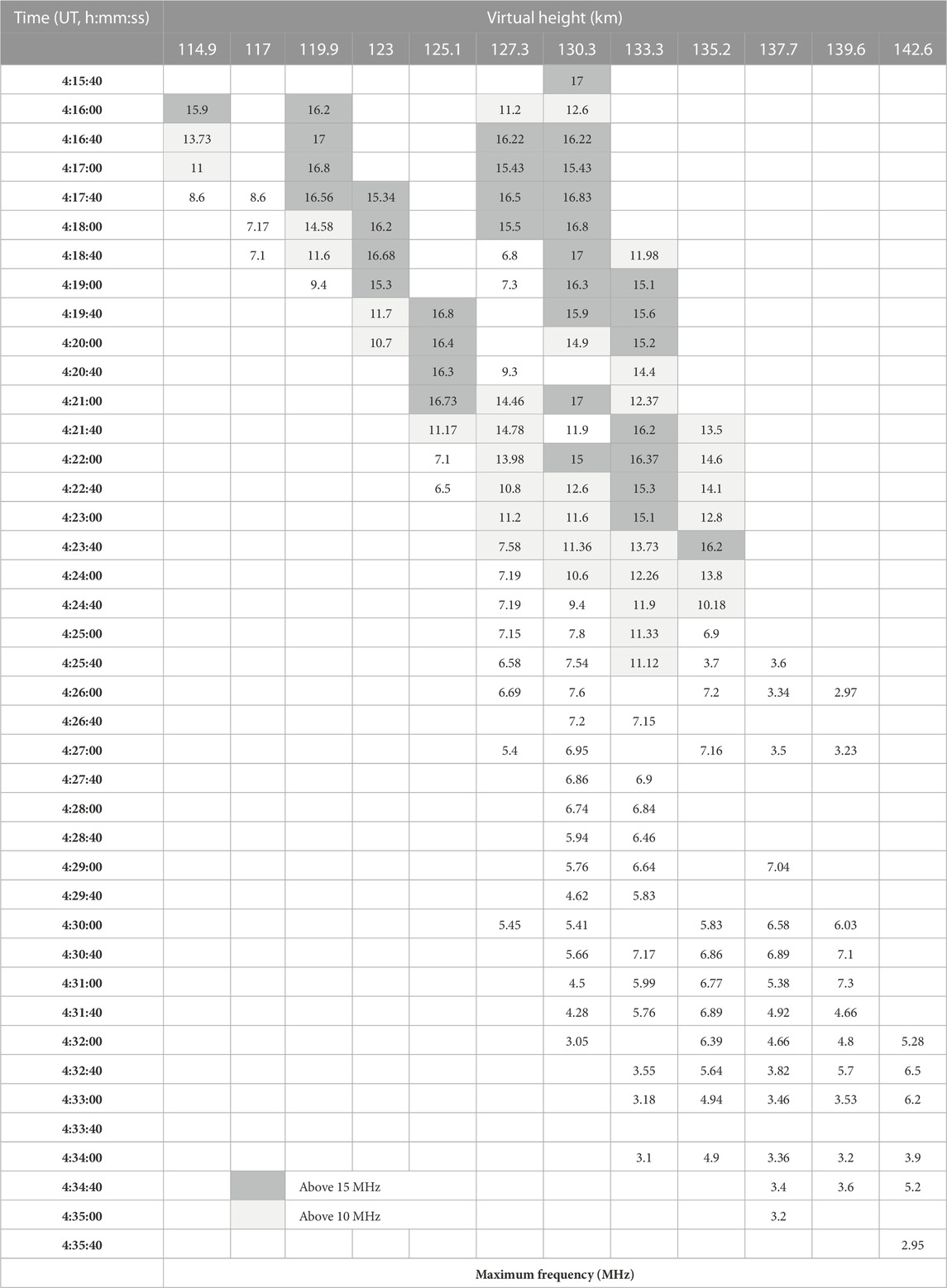
TABLE 3. The maximum frequencies reached by the fireball’s trace, according to the height of the layer, and their evolution over time. These were taken by manual reading at 4 dB MPA threshold if there were distinct reflections at 6 dB threshold of the given height. The readings are highly subjective and are included here for illustrative purposes only.
The height of the fireball’s trace (Figure 3.) started at 129.3 km virtual height at 04:15:40 (Table 3). On the next ionogram, the trace is already split into three distinct and separeted “layers”, and this remains the case in all the following ionograms. The trace reached a maximum of 5 distinct “layers”. The height of these layers according to the ionogram readings (Table 3.) first decreased, then increased slowly but steadily over time–the lowest is at 114.9 km virtual height, while the highest before the decay of the fireball’s trace is at 142.6 km virtual height. The rise in the height of the fireball’s trace is a phenomenon that has not only been observed elsewhere (Maruyama et al., 2008), but is also reproduced by numerical modeling (Zinn and Drummond, 2005; Zinn and Drummond, 2007). However, these height changes observed on the ionograms should not be interpreted as clear vertical motion only, especially that the CDS measurements indicate the presence of wind shear. The movements are probably the results of the digisonde’s setup with 3D motions of fireball’s trace, since the measurement is based on the time-of-flight of the sounding electromagnetic waves so the exact height changes cannot be determined based on that.
Meanwhile, the ionograms of Sopron station did not show any similar effect–there is no regular sporadic E layer on the ionograms (Figure 3). Both stations were taking measurements synchronously allowing for oblique measurement. With high probability, we also ruled out the presence of sporadic E layer in half of the distance between the stations as it would be observed on oblique ionograms. Therefore we deduced that the trace detected at Průhonice station is a local plasma structure restricted to a limited area close to Průhonice DPS-4D (Figure 1). Indeed, the location of the meteor trace corresponds to the sounding cone of the Průhonice’s digisonde, while it is out of the cone of Sopron’s digisonde.
It may also be worth noting that for the most often used 6 dB data presentation threshold (defined as a noise level based on the most probable amplitude (MPA)), it appears as if reflections are disappearing in bursts by frequency. However, this is not a real physical phenomenon, but is due to a filtering in the processing software and/or in the raw data save settings after the digisonde measurement (Reinisch et al., 2008; Reinisch, 2009). When the threshold is set to 4 dB or lower, these reflections can be found (Figure 4).
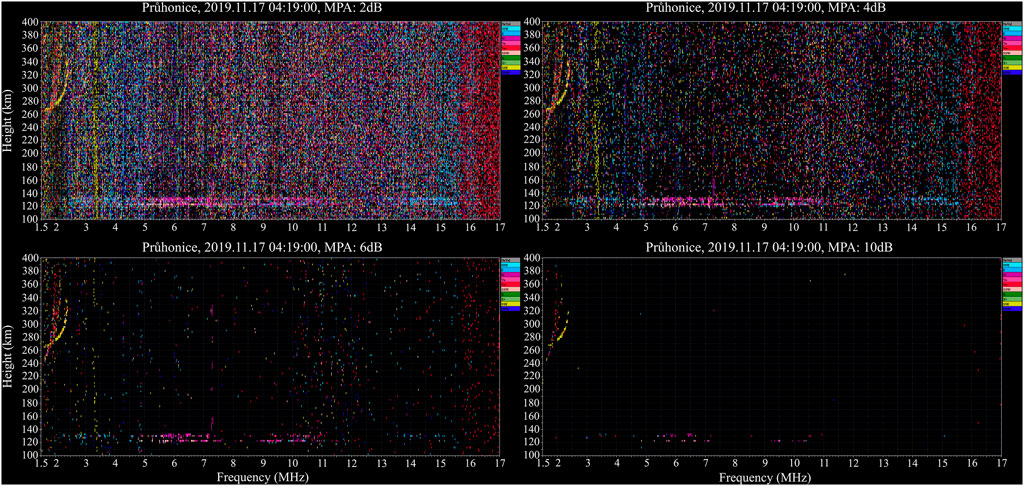
FIGURE 4. The effect of the most probable amplitude threshold settings. The lower the threshold (2 dB or 4 dB, upper row) the noisier the ionogram, but also more details of the fireball’s effect can be seen. The higher the threshold (6 dB–the default setting used for processing–or 10 dB, lower row), the lower the noise, but the more detail is lost from the fireball’s effect.
Another known measurement feature is the phase jump. Although the antenna layout allows the direction of the reflections to be determined, it is possible that due to phase ambiguity of the signal the direction of the arriving signal is misinterpreted and rotated by 180°. The ionization cloud caused by the fireball shows E, NNE, W and SSW oblique reflections (Figures 3, 4), but the W and SSW directions only occur at well-defined frequency ranges (usually between 5–13 MHz) and, given the fireball’s detection and the drift measurements, there is a strong possibility that the W and SSW directions were in reality probably E and NNE directions (Reinisch et al., 2008; Reinisch, 2009).
The ionograms were also scaled manually between 03:45:00 and 04:45:00 (UT) for both Průhonice and Sopron stations, in order to see if the fireball had any local effect on the heights of the ionospheric layers because bolides and superbolides are capable of generating traveling ionospheric disturbance-like phenomena (Chernogor, 2015). Based on the ionograms, there was only the F2 layer at this time of that day beside the thin sporadic E layer caused by the fireball at Průhonice station. It is worth noting that the limited quality of the ionograms (because of the 1 min cadence) and the presence of a strong spread F activity (Wang et al., 2018) made the manual evaluation difficult and subject to bias.
According to the height analysis (see Supplementary Figure S3), there was a spike in the F2 layer’s virtual height at 04:13:40 (UT) at Sopron station and at 04:14:40 (UT) at Průhonice station. Relatively close to the appearance of the fireball’s trace–which happened at 04:15:0.2 (UT) according to the astronomical observations (Figure 2; Table 2) and 04:15:40 (UT) according to the ionograms–it would be easy to attribute this phenomenon as the fireball’s effect, but the ionograms of Sopron station also showed this spike in height almost a minute earlier than Průhonice station. This suggested that this sudden increase in height was probably a regional, disturbance-like phenomenon since it was unlikely that a fireball with a linear trajectory length of 73.44 km and a duration of 1 s could have taken a perceivable effect at Sopron station almost 2 min before the event itself. This means that, presumably, the fireball had no effect on the height of the F2 layer, which is not surprising given that it is a fireball and not a bolide or superbolide.
3.2.2 SkyMaps
During the campaign measurements, drift measurements were also taken every minute. The measurement settings were chosen based on the automatic scaling of the ionograms. Between 04:19:20 (UT) and 04:35:20 (UT)—with the exception of 04:21:20, 04:27:40 and 04:34:20 (UT) SkyMaps –,1 to 5 reflection points were displayed on each SkyMap (Figure 5). These are not sufficient to determine a plasma drift velocity, but can serve to estimate the height of the reflection points which varies between 122 and 140 km, suggesting that they are reflected from the fireball’s trace (Table 3). Before 04:19:20 and after 04:35:20 the SkyMaps are completely empty for a long time. Presence of only a few reflection points on the SkyMaps indicate that in general the ionosphere is nicely stratified with only a small and very localized distortions of reflection planes due to the fireball’s passage and follow-up processes of sporadic E layer formation.
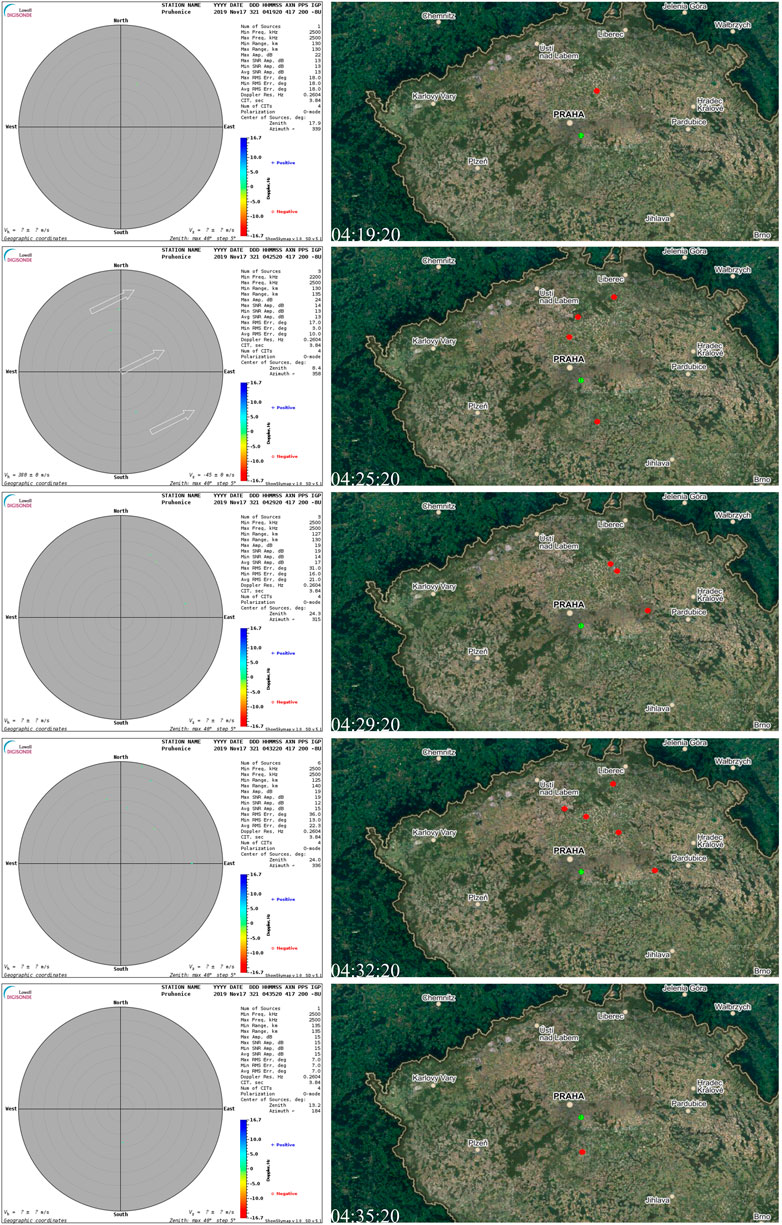
FIGURE 5. Selected SkyMaps, displaying the drift measurement (left column), the geographical location of the reflection (right column). Průhonice station is marked with a green dot. The reflections detected by the digisonde are marked with red dots. Since the measurements were not enough to reliably determine a drift velocity, the red dots of the right column are only representative and do not indicate any physical quantity.
Based on the drift measurements, the approximate location of the sporadic E layer-like ionization cloud caused by the fireball can be determined and it agrees well with the trajectory estimated by the astronomical observations (Figure 1). Furthermore, it is consistent with what can be seen in the spectrograms of the Continuous Doppler Sounding, where the trace appears on the Panská Ves-Prague and the Průhonice-Prague sounding paths, but not on the Dlouhá Louka-Prague path (see Section 3.3).
3.3 CDS
Continuous Doppler Sounding measurements were taken at 4.65 MHz and were plotted on the Doppler-shift spectrogram for the time period of 04:00-05:00 UT on 17 November in Figure 6. The fireball trace appeared as a diffuse blip on 2 of the 3 sounding paths from about 04:18 UT to 04:30 UT, which roughly coincides with the observations on the ionograms (Table 3). The fact that it appeared on only 2 sounding lines (on the Průhonice-Prague and Panská-Ves-Prague lines, but not on the Dlouhá Louka-Prague line) may also suggest that this is a relatively small-scale localized phenomenon (Figure 1).
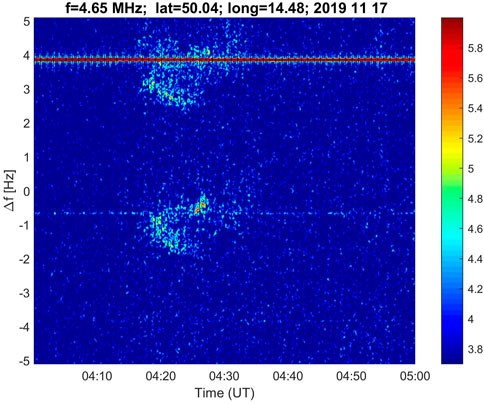
FIGURE 6. Doppler shift spectrogram taken on 17 November 2019 between 4:00 and 5:00 a.m. The fireball’s trace is visible between 4:18 and 4:30 on 2 of the 3 paths (Figure 1).
In addition to the relatively broad and diffuse spectrum (blip) observed on these two sounding tracks, two peaks in the spectral densities can be seen. One in the vicinity of zero Doppler shift (indicated by the ground wave–horizontal line in the Doppler-shift spectrogram) and the other with a negative Doppler-shift; the negative Doppler-shift decreases with time (absolute Doppler shift increases). This means that there are two subregions with different radial velocities relative to the midpoints between transmitters and receiver from which radio waves predominantly reflect, which might indicate a wind shear in the region of reflection (meteoric trail). The subregion with the negative Doppler shifts moves away. Interestingly, the signal strength of the subregion corresponding to the approximately zero Doppler-shifts increases just before the signal disappears, probably due to some dynamic changes in the electron densities.
This event is very different from how a descending sporadic E usually appears on spectrograms (see Section 3.4.2). It should be noted here that sporadic E layer is rather a summer phenomenon, however it could be observed through the year as there are many physical processes contributing to the layer formation (Whitehead, 1961; Whitehead, 1989; Mathews, 1998; Haldoupis, 2012; Arras and Wickert, 2018 among others).
3.4 Comparison with a strong sporadic E
It may be useful to compare these traces left by the fireball with cases when a sporadic E layer is present at the ionograms and also in the Doppler-shift spectrogram. For comparison, we have taken a case from 1 January 2020, showing a southward drifting sporadic E with relatively high ionization level (∼8 MHz critical frequency (foEs) in certain times) which is also nicely visible in the CDS measurements.
The geomagnetic conditions show a quiet day (based on the OmniWeb: Kp ∼ 1, Dst ∼ −5 nT, see Supplementary Figure S4) and according to the satellite data the tropospheric conditions were calm, too (see Supplementary Figure S5). The ionograms (Figure 7.) and the Continuous Doppler Sounding (Figure 8.) show a clear difference compared to the previously shown fireball-induced changes. Unfortunately, the drift measurements were primed to observed motions at the F2 layer at both stations and could not be used here (see Supplementary Figure S6).
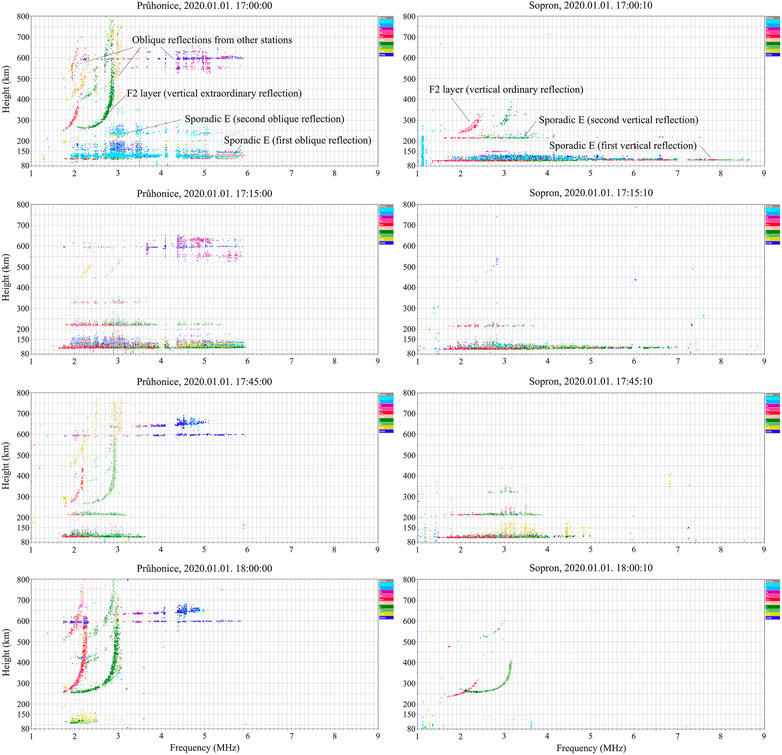
FIGURE 7. Ionograms recorded at 17:00, 17:15, 17:45 and 18:00 (UT) at Průhonice (left column) and at Sopron (right column) stations. They show the temporal evolution of a strong, regional sporadic E layer.
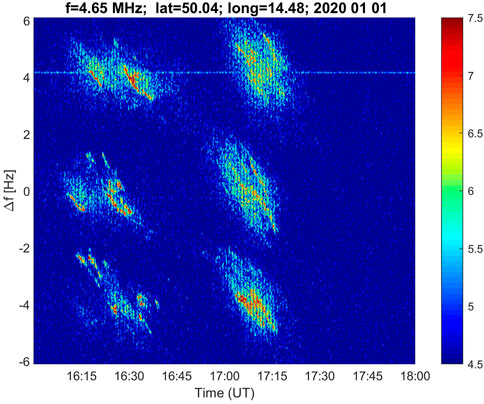
FIGURE 8. A regional sporadic E layer drifting toward south on 4.65 MHz Doppler-shift spectrogram made on 1 January 2020.
3.4.1 Ionograms during a strong sporadic E activity
Průhonice station made an ionogram every 15 min at this time, while Sopron station made one every 5 min. The measurements of the two stations were asynchronous at this time, with the ionograms of Sopron station being taken 10 s later than the ionograms of Průhonice station.
A strong, patchy sporadic E layer was detected at both stations during the evening hours (16:00 - 18:30 UT) on 01.01.2020. Figure 7 displays the temporal evolution of this regional sporadic E layer, with strong ionization and spread. Both stations show northward reflections at the beginning and southward reflections before the disappearance of the sporadic E layer, which may suggest a southward direction of plasma motion. Despite the proximity of the stations, the temporal behavior is different, with sporadic E appearing and disappearing earlier in Sopron than in Průhonice.
Some features are also worth noting. The critical frequency of sporadic E layer (foEs) over Sopron station almost reaches 8 MHz at 17:00:10 (UT). The ionograms show a second reflection at 17:15:10 (UT) and at 17:45:10 (UT) a third reflection, too. For Sopron station, between 17:05:10 and 17:55:10 (UT) the F2 layer is completely blanketed.
Similar features can be observed for Průhonice station, although the effects are somewhat weaker and the durations are not the same. The maximum critical frequency (foEs) of 5.83 MHz is reached at 17:15:00 and the sporadic E layer on this ionogram produces a third reflection. The F2 layer is also completely blanketed but only on this ionogram. On the 17:45:00 ionogram there is still some blanketing, but at 18:00:00 the sporadic E layer has disappeared in the ordinary component, but is still detectable in the extraordinary component, unlike in Sopron station.
All of this is in sharp contrast to what can be seen with the fireball’s effect–despite the much higher ionization (reaching 17 MHz), there is no second reflection and no blanketing (Figure 3), also, the effect was local and not regional since nothing can be seen on the ionograms of Sopron.
3.4.2 CDS measurement during a strong sporadic E activity
There is a sequence of two sporadic E layers seen as oblique spread structures in the Doppler shift spectrogram recorded between 16:00 and 18:00 UT (Figure 8). Sporadic E was moving at velocity of approximately 100–110 m/s in a roughly south-westerly direction (azimuth approximately 210°–220°), which can be inferred from the time lags between occurrences of the structure along the various sounding paths (transmitter-receiver pairs). The trace of a distinct regional inhomogeneous sporadic E layer usually appears as such a massive signal (oblique spread structure), in contrast to the faint, diffuse and dispersed signal of the fireball (Figure 6).
4 Discussion
Compared to ionospheric scales, the effect of the fireball was highly localized. This can be observed in the fact that while the Průhonice station ionograms showed a clear trace, the measurements of Sopron station showed no reflection originating from the Es region. It has been shown that the sporadic E layers are driven by modulation of large-scale tidal waves by planetary waves (Pancheva et al., 2003; Haldoupis et al., 2006; Šauli and Bourdillon, 2008; Mošna et al., 2015) which means that the presence of sporadic E layers at two close locations as Průhonice and Sopron is typically driven by the same forcing and therefore highly correlated. Furthermore, in the case of Continuous Doppler Sounding, which measures 3 sounding paths at 4.65 MHz, the effect was only visible on 2 of the 3 paths, Panská Ves-Prague and Průhonice-Prague, but not on the Dlouhá Louka-Prague path. This can also be seen in the drift measurements of Průhonice station because there is an average of 1–3 reflections per SkyMap from 122 to 142 km height over the duration of the event, and although these points are scattered, they still fall within a well-defined area, which is consistent with what is observed in Continuous Doppler Sounding and are within reasonable distance from the original trajectory of the fireball.
The movement of the fireball’s ionization trace was within expectations. The manual readings of the heights (Table 3) showed that the height of the layer decreased immediately after its appearance, and then increased shortly afterwards. This is consistent with what was described by Maruyama et al. (2008) and reproduced by numerical modeling in Zinn and Drummond 2005 and Zinn and Drummond 2007. But these motions observed on the ionograms should not be interpreted as clear vertical motion, especially that the CDS measurements indicate the presence of wind shear. The movements were probably the results of the digisonde’s setup with 3D motions of fireball’s trace.
Regarding the layers or stratification of the trace (it has broken up into 3–5 distinct layers, usually separated by 3–6 km) a similar behavior has been observed before in the case of the Dhajala fireball (3 layer, separated by 6 km, Rajaram and Chandra, 1991).
The lifetime of the ionized layer was 12 min according to the Continuous Doppler Sounding measurements and 20 min according to the digisonde measurements. This was slightly below what has been observed so far. Maruyama et al. (2003) and Maruyama et al. (2008) reported lifetimes of 40 min although the exact origin of their phenomena is unclear. Vierinen et al. (2022) reported a lifetime of 30 min for the Pajala fireball. The Dhajala fireball’s trace lasted for an hour (Rajaram and Chandra, 1991). The lifetime of the observed faint sporadic E layer probably depends on parameters of the particular observed fireballs like mass (Dhajala fireball was estimated to be above 10 kg, the Pajala fireball’s dynamic mass was estimated between 0.6 and 1.7 kg, the fireball of this study had a photometric mass of 1.45 kg), velocity, local atmospheric and ionospheric conditions including the wind conditions, geomagnetic location, and lower atmosphere situation due to coupling within the neutral and ionized atmosphere.
Discussing the extent of ionization is somewhat complicated by the fact that the upper limit of the digisonde measurement was 17 MHz. In several cases, the ionograms clearly showed that saturation occurs at this frequency, i.e., the maximum was above this value. This situation was similar to that observed for the Pajala fireball, where the measurement limit was 16 MHz. Since Schult et al. (2015) detected a fireball at 32.5 MHz with meteor radar; the ionosonde measurements of Maruyama et al. (2003) reached a top frequency of 30 MHz and Maruyama et al. (2008) reached 27 MHz, it is in agreement with our assumption that the top frequency of the fireball exceeded 17 MHz. In addition, the phenomenon observed for the Pajala fireball, where the top frequency dropped below 10 MHz only after 10 min, was also produced by this fireball.
The trace caused by a fireball (which resembles a faint sporadic E) compared to a strong sporadic E shows substantial differences. For this purpose, a case was chosen when both the geomagnetic and tropospheric conditions were quiescent. The sporadic E layer of 1 January 2020 was a southward moving (based on CDS measurements), probably tilted layer, showing a strong spread and ionization reaching 6–8 MHz on the ionograms. The explanation of the large spread feature is unknown since it happened during tropospherically and geomagnetically quiet conditions. The important characteristics of the fireball induced sporadic E layer was its thickness. Sporadic E layers are often observed as electromagnetically thick layers reflecting almost all the sounding signals, while the structures observed in connection with meteor occurrence we observe thin and localized structures. In comparison it may worth noting that, unlike the behavior of this stronger sporadic E layer, no second reflection or blanketing occurred with the trace of the fireball was observed, suggesting that despite the high plasma frequency of the trace its ionization was not strong enough to cause blanketing for the upper layers and/or the trace occurred only in a very small area near the ionosonde.
Manual scaling was used to investigate whether the fireball had any effect on the height of the F2 region. For the Průhonice station, there was an increase in the height of the F2 layer at 04:14:40 (UT), followed by a rapid decrease in height. The same features can be observed in the data of Sopron station used as a reference, but the peak occured at 04:13:40 (UT). While the timing of these spikes were highly convenient, especially considering the trajectory of the fireball, is most likely not the result of the fireball, but rather of a regional small-scale traveling ionospheric disturbance (TID) or some similar atmospheric phenomenon. Since the investigated phenomena was classified as a fireball, and not as a bolide or superbolide, this is within expectations.
It may be of interest to note that the fireball effect is first detected by the CDS measurements at 04:18 (UT), while the drift measurements detected it at 04:19:20 (UT), i.e. 3 and 4 min after the fireball’s appearance. Furthermore, although the drift measurements were detecting it until the disappearance of the trace at 04:35:40 (UT), the diffuse blip on CDS measurements disappeared 5 min earlier, as early as 04:30. Since the ionosonde measurements show that there was still ionization above 4.65 MHz after 04:30, but the ionosonde covers a larger area, it is conceivable that there was not enough of the trace left in the sounding paths for the CDS to detect it.
Unfortunately, there was no reliable high-altitude wind data. The impact of the wind seems to appear on the optical observation of the fireball itself (Figure 2). The fact that the trace on the ionograms splits into 3 distinct layers immediately after its first appearance like the Dhajala-fireball, and that this number goes up to 5 over time (Figure 3), and that the trace also occurs between altitudes 114.9 and 142.6 km (Table 3) during its 20 min lifetime, suggests that there was some kind of wind activity above altitude 100 km and that possibly some wind shear has occurred. Another clue to this might be the spatial distribution of the trajectory marked in Figure 1 and the incoming reflections on the SkyMaps shown in Figure 5, which shows a good correlation, but the points reflected from the ionosphere are coming from a wider area, which could be due to wind effects. Further evidence of this may be the strong frequency spread F activity on the ionograms, a known nighttime phenomenon which has roots in the atmospheric conditions (Wang et al., 2018).
5 Conclusion
A bright fireball was recorded between 49.957°N, 15.563°E, at 136.46 km height and 50.237°N 15.267°E at 71.81 km height on 17 November 2019 04:15:0.2 (UT). Once in the atmosphere, the fireball caused strong ionization. Due to its proximity to the Průhonice digisonde station and the Continuous Doppler Sounding network developed by the Institute of Atmospheric Physics CAS, the event allowed for observation of the ionospheric effects.
It was the first evidence, to our knowledge, that a digisonde (DPS-4D type) detected a documented fireball. A major advantage of the digisonde over other “classical” ionosondes is its ability to determine direction. Furthermore, this is the first time that drift measurements made by the digisonde have shown reflections attributable to the fireball’s effect. In the ionograms recorded by the digisonde, the trace of the fireball appeared as a faint sporadic E layer. The ionization reached 17 MHz which was the measurement’s limit, the trace was split into several layers, the height of which moved downwards and then upwards. This cannot be assessed as a purely vertical movement due to the lack of wind data and was probably the result of complex 3D movements which is also indicated by the CDS measurement. There were also synchronized measurements at Sopron station. In these ionograms there was no trace of sporadic E activity (neither vertical nor oblique), suggesting that the observed effect at Průhonice station was a local one.
It was the first time that Continuous Doppler Sounding had detected a trace of a fireball. It appeared as a relatively broad and diffuse spectrum (blip) observed on 2 of the 3 sounding paths, indicating a relative localization. The measurements displayed two subregions with different radial velocities relative to the midpoints between transmitters and receiver from which radio waves predominantly reflect, which might indicate a wind shear in the region of reflection.
Since many properties observed in this paper (height changes, stratification, high top frequency, duration, etc.) are scattered but have been reported in previous literatures (Rajaram and Chandra, 1991; Maruyama et al., 2003; Maruyama et al., 2008; Schult et al., 2015; Vierinen et al., 2022), we compared this with the behavior of a strong sporadic E layer. Clear differences were observed between the fireball’s trace and the “average” sporadic E layer (e.g., blanketing, secondary reflections).
As for the digisonde itself and its capabilities, this example illustrates that this instrument can be used to detect bodies of meteoric origin. The range of sizes over which it operates will need to be further investigated. These measurements can be carried out regardless of the time of day and metrological conditions, however, the measurements are limited to a roughly 100 km radius of the digisonde. A trace of a fireball can be expected to persist for 20–60 min, based on the evidence so far. The most commonly used 5 min time resolution measurements may be suitable for detecting such an object, but for detecting smaller bodies it may be more appropriate to use a higher time resolution. The digisonde direction determination may contain an error of 180°. It is necessary to take this into account and the development of this feature would be desirable in the future. The drift measurements and Skymaps clearly showed reflections associated with the fireball. In this case, complex 3D movements and the extent of the affected area can be inferred.
Detection of meteors by means of high sampling rate digisonde measurement represents useful complementary observation techniques. This well documented example for the plasma trace detection of a fireball by digisonde measurements can open the door for further investigations of ionospheric impacts of individual meteors.
Data availability statement
The SAOExplorer (used for manual scaling) is available at https://ulcar.uml.edu/SAO-X/SAO-X.html NASA/GSFC’s OMNI data set through OMNIWeb https://omniweb.gsfc.nasa.gov/ The astronomical data is the property of Czech Fireball Network and cannot be distributed freely. The Doppler data in the form of spectrograms are available at http://datacenter.ufa.cas.cz/ under the link to Spectrogram archive. The datasets of digisonde measurements (ionograms, SkyMaps) presented in this study can be found in online repositories. The names of the repository/repositories and accession number(s) can be found below: Global Ionospheric Radio Observatory http://giro.uml.edu The SAT24 meteorological satellite pictures are available at: https://www.sat24.com/history.aspx further inquiries can be directed to the corresponding author.
Author contributions
VB was conceptual author of the digisonde campaign measurements leading to this article and helped with extensive knowledge regarding various ionospheric phenomena. CsSz found the fireball’s trace on the ionograms, pre-wrote the manuscript, analyzed the movements of the trace and scaled ionograms by hand. JCh found the trace of the fireball in the CDS, analyzed the CDS measurements and chose the sporadic E used for the comparison, also helped with extensive knowledge regarding various ionospheric phenomena. PKk helped with finding and linking the separate data (astronomical, ionospheric) on which the article is based on, helped with the height contour analysis and helped with extensive knowledge regarding various ionospheric phenomena. ZM analyzed the general behavior of the sporadic E in 2019 November and December (manually processing 20000+ ionograms) and helped with extensive knowledge regarding various ionospheric phenomena. KP prepared, analyzed and calculated everything related to astronomical data. DK analyzed the drift measurements and made the plots about the geographical representation of the reflections. All authors contributed to the article and approved the submitted version.
Funding
The work was supported also by the GINOP-2.3.2-15-2016-00003 (titled “Kozmikus hatások és kockázatok”) Hungarian national project. This work was made possible by the “Multiinstrumental investigation of the midlatitude ionospheric variability” bilateral project of the Czech Academy of Sciences and Hungarian Academy of Sciences (MTA-19-03 and NKM 2018-28). The support under the grant GA21-03295S by the Czech Science Foundation is acknowledged. The contribution of VB was partially supported by Bolyai Fellowship (GD, no. BO/00461/21).
Acknowledgments
This fireball was observed by the cameras of the European Fireball Network (Borovička et al., 2022) and the data were provided by P. Spurný (priv. comm.). We also thank Czech Fireball Network, especially J. Borovička and P. Spurný for very kindly providing the data.
Conflict of interest
The authors declare that the research was conducted in the absence of any commercial or financial relationships that could be construed as a potential conflict of interest.
Publisher’s note
All claims expressed in this article are solely those of the authors and do not necessarily represent those of their affiliated organizations, or those of the publisher, the editors and the reviewers. Any product that may be evaluated in this article, or claim that may be made by its manufacturer, is not guaranteed or endorsed by the publisher.
Supplementary material
The Supplementary Material for this article can be found online at: https://www.frontiersin.org/articles/10.3389/fspas.2023.1197832/full#supplementary-material
References
Arras, C., and Wickert, J. (2018). Estimation of ionospheric sporadic E intensities from GPS radio occultation measurements. J. Atmos. Sol. Terr. Phys.171, 60–63. doi:10.1016/j.jastp.2017.08.006
Axford, W. I. (1963). The formation and vertical movement of dense ionized layers in the ionosphere due to neutral wind shears. J. Geophys Res.68, 769–779. doi:10.1029/JZ068i003p00769
Beech, M. (1998). Large-body meteoroids in the Leonid stream. Astron J.116, 499–502. doi:10.1086/300435
Borovička, J., Spurný, P., and Shrbený, L. (2019). “New spectroscopic program of the European fireball network,” in International meteor conference, pezinok-modra, Slovakia. Editors R. Rudawska, J. Rendtel, C. Powell, R. Lunsford, and C. Verbeeck (Knofel), 28–32.
Borovička, J., Spurný, P., Shrbený, L., Štork, R., Kotková, L., Fuchs, J., et al. (2022). Data on 824 fireballs observed by the digital cameras of the European Fireball Network in 2017–2018. Astron Astrophys.667, A157. doi:10.1051/0004-6361/202244184
Borovička, J., Štork, R., and Bocek, J. (1999). First results from video spectroscopy of 1998 Leonid meteors. Meteorit. Planet Sci.34, 987–994. doi:10.1111/j.1945-5100.1999.tb01418.x
Ceplecha, Z., Spalding, E. R., Jacobs, C., Revelle, D. O., Tagliaferri, E., and Brown, P. (1999). “Superbolides,” in Meteroids 1998. Editors W. J. Baggaley, and V. Porubcan, 37.
Chen, J. S., Wang, C. Y., Su, C. L., and Chu, Y.-H. (2020). Meteor observations using radar imaging techniques and norm-constrained Capon method. Planet Space Sci.184, 104884. doi:10.1016/j.pss.2020.104884
Chernogor, L. F. (2015). Ionospheric effects of the Chelyabinsk meteoroid. Geomagnetism Aeronomy55, 353–368. doi:10.1134/S0016793215030044
Chum, J., Liu, J. Y., Laštovička, J., Fišer, J., Mošna, Z., Baše, J., et al. (2016). Ionospheric signatures of the April 25, 2015 Nepal earthquake and the relative role of compression and advection for Doppler sounding of infrasound in the ionosphere. Earth, Planets Space68, 24. doi:10.1186/s40623-016-0401-9
Chum, J., Podolská, K., Rusz, J., Baše, J., and Tedoradze, N. (2021). Statistical investigation of gravity wave characteristics in the ionosphere. Earth, Planets Space73, 60. doi:10.1186/s40623-021-01379-3
Chum, J., Urbář, J., Laštovička, J., Cabrera, M. A., Liu, J.-Y., Bonomi, F. A. M., et al. (2018). Continuous Doppler sounding of the ionosphere during solar flares. Earth, Planets Space70, 198. doi:10.1186/s40623-018-0976-4
Di Martino, M., and Cellino, A. (2004). “Physical properties of comets and asteroids inferred from fireball observations,” in Mitigation of hazardous comets and asteroids, 156.
Ellyett, C. D., and Goldsbrough, P. F. (1976). Relationship of meteors to sporadic E, 1. A sorting of facts. J. Geophys Res.81, 6131–6134. doi:10.1029/JA081i034p06131
Fukao, S., and Hamazu, K. (2014). “Observations by atmospheric radar,” in Radar for meteorological and atmospheric observations (Tokyo: Springer Japan), 435–485. doi:10.1007/978-4-431-54334-3_12
Haldoupis, C. (2011). “A tutorial review on sporadic E layers,” in Aeronomy of the Earth’s atmosphere and ionosphere (Dordrecht: Springer Netherlands), 381–394. doi:10.1007/978-94-007-0326-1_29
Haldoupis, C., Meek, C., Christakis, N., Pancheva, D., and Bourdillon, A. (2006). Ionogram height–time–intensity observations of descending sporadic E layers at mid-latitude. J. Atmos. Sol. Terr. Phys.68, 539–557. doi:10.1016/j.jastp.2005.03.020
Haldoupis, C. (2012). Midlatitude sporadic E. A typical paradigm of atmosphere-ionosphere coupling. Space Sci. Rev.168, 441–461. doi:10.1007/s11214-011-9786-8
Hawkes, R. L. (2007). Peter jenniskens, meteor showers and their parent comets. Earth Moon Planets101, 93–95. doi:10.1007/s11038-007-9145-7
Höffner, J., von Zahn, U., McNeil, W. J., and Murad, E. (1999). The 1996 Leonid shower as studied with a potassium lidar: Observations and inferred meteoroid sizes. J. Geophys Res. Space Phys.104, 2633–2643. doi:10.1029/1998JA900063
Kereszturi, Á., Barta, V., Bondár, I., Czanik, C., Igaz, A., Mónus, P., et al. (2021). Review of synergic meteor observations: Linking the results from cameras, ionosondes, infrasound and seismic detectors. Mon. Not. R. Astron Soc.506, 3629–3640. doi:10.1093/mnras/stab1918
Koten, P., Rendtel, J., Shrbený, L., Gural, P., Borovička, J., and Kozak, P. (2019). “Meteors and meteor showers as observed by optical techniques,” in Meteoroids: Sources of meteors on Earth and beyond. Editors G. O. Ryabova, D. J. Asher, and M. J. Campbell-Brown, 90.
Koten, P., Spurný, P., Borovička, J., Evans, S., Elliott, A., Betlem, H., et al. (2006). The beginning heights and light curves of high-altitude meteors. Meteorit. Planet Sci.41, 1305–1320. doi:10.1111/j.1945-5100.2006.tb00523.x
Kouba, D., and Chum, J. (2018). Ground-based measurements of ionospheric dynamics. J. Space Weather Space Clim.8, A29. doi:10.1051/swsc/2018018
Kozlovsky, A., Lukianova, R., and Lester, M. (2020). Occurrence and altitude of the long-lived nonspecular meteor trails during meteor showers at high latitudes. J. Geophys Res. Space Phys.125. doi:10.1029/2019JA027746
Laštovička, J., and Chum, J. (2017). A review of results of the international ionospheric Doppler sounder network. Adv. Space Res.60, 1629–1643. doi:10.1016/j.asr.2017.01.032
Maruyama, T., Kato, H., and Nakamura, M. (2003). Ionospheric effects of the Leonid meteor shower in November 2001 as observed by rapid run ionosondes. J. Geophys Res.108 (1), 4–13. doi:10.1029/2003JA009831
Maruyama, T., Kato, H., and Nakamura, M. (2008). Meteor-induced transient sporadic E as inferred from rapid-run ionosonde observations at midlatitudes. J. Geophys Res. Space Phys.113. n/a-n/a. doi:10.1029/2008JA013362
Mathews, J. D. (1998). Sporadic E: Current views and recent progress. J. Atmos. Sol. Terr. Phys.60, 413–435. doi:10.1016/S1364-6826(97)00043-6
Molau, S., Gural, P. S., and Okamura, O. (2002). Comparison of the ``American’’ and the ``Asian’’ 2001 Leonid meteor storm. WGN, J. Int. Meteor Organ.30, 3–21.
Mošna, Z., Knížová, P. K., and Potužníková, K. (2015). Coherent structures in the Es layer and neutral middle atmosphere. J. Atmos. Sol. Terr. Phys.136, 155–162. doi:10.1016/j.jastp.2015.06.007
Pancheva, D., Haldoupis, C., Meek, C. E., Manson, A. H., and Mitchell, N. J. (2003). Evidence of a role for modulated atmospheric tides in the dependence of sporadic E layers on planetary waves. J. Geophys Res. Space Phys.108. doi:10.1029/2002JA009788
Rajaram, G., and Chandra, H. (1991). Sporadic E ionization associated with meteor events. Proc. Indian Acad. Sciences-Earth Planet. Sci.100, 255–265. doi:10.1007/bf02895986
Reinisch, B. W., and Galkin, I. A. (2011). Global ionospheric radio observatory (GIRO). Earth, Planets Space63, 377–381. doi:10.5047/eps.2011.03.001
Reinisch, B. W., Galkin, I. A., Khmyrov, G. M., Kozlov, A. V., Lisysyan, I. A., Bibl, K., et al. (2008). Advancing digisonde technology: The DPS4. In. Radio Sound. Plasma Phys. AIP Conf. Proc.974, 127–143.
Reinisch, B. W., Huang, X., Galkin, I. A., Paznukhov, V., and Kozlov, A. (2005). Recent advances in real-time analysis of ionograms and ionospheric drift measurements with digisondes. J. Atmos. Sol. Terr. Phys.67, 1054–1062. doi:10.1016/j.jastp.2005.01.009
Šauli, P., and Bourdillon, A. (2008). Height and critical frequency variations of the sporadic-E layer at midlatitudes. J. Atmos. Sol. Terr. Phys.70, 1904–1910. doi:10.1016/j.jastp.2008.03.016
Schult, C., Stober, G., Keuer, D., and Singer, W. (2015). Radar observations of the Maribo fireball over Juliusruh: Revised trajectory and meteoroid mass estimation. Mon. Not. R. Astron Soc.450, 1460–1464. doi:10.1093/mnras/stv614
Spurný, P., Betlem, H., Jobse, K., Koten, P., and Levenvan’t, J. (2000). New type of radiation of bright Leonid meteors above 130 km. Meteorit. Planet Sci.35, 1109–1115. doi:10.1111/j.1945-5100.2000.tb01497.x
Spurný, P., Borovička, J., Mucke, H., and Svoreň, J. (2017). Discovery of a new branch of the Taurid meteoroid stream as a real source of potentially hazardous bodies. Astron Astrophys.605, A68. doi:10.1051/0004-6361/201730787
Vierinen, J., Aslaksen, T., Chau, J. L., Gritsevich, M., Gustavsson, B., Kastinen, D., et al. (2022). Multi-instrument observations of the Pajala fireball: Origin, characteristics, and atmospheric implications. Front. Astronomy Space Sci.9. doi:10.3389/fspas.2022.1027750
Wang, N., Guo, L., Zhao, Z., Ding, Z., and Lin, L. (2018). Spread-F occurrences and relationships with foF2 and h′F at low- and mid-latitudes in China. Earth, Planets Space70, 59. doi:10.1186/s40623-018-0821-9
Whitehead, J. D. (1989). Recent work on mid-latitude and equatorial sporadic-E. J. Atmos. Terr. Phys.51, 401–424. doi:10.1016/0021-9169(89)90122-0
Whitehead, J. D. (1961). The formation of the sporadic-E layer in the temperate zones. J. Atmos. Terr. Phys.20, 49–58. doi:10.1016/0021-9169(61)90097-6
Yeomans, D. K., Yau, K. K., and Weissman, P. R. (1996). The impending appearance of comet tempel–tuttle and the Leonid meteors. Icarus124, 407–413. doi:10.1006/icar.1996.0218
Zinn, J., and Drummond, J. (2007). Formation of parallel meteor trail pairs as associated with their buoyant rise. Adv. Space Res.39, 555–561. doi:10.1016/j.asr.2006.12.007
Keywords: european fireball network, digital autonomous fireball observatory (DAFO), GIRO network, digisonde, drift measurements, continuous Doppler sounding, sporadic E, leonid fireball
Citation: Szárnya C, Chum J, Podolská K, Kouba D, Koucká Knížová P, Mošna Z and Barta V (2023) Multi-instrumental detection of a fireball during Leonids of 2019. Front. Astron. Space Sci. 10:1197832. doi: 10.3389/fspas.2023.1197832
Received: 31 March 2023; Accepted: 19 May 2023;
Published: 14 June 2023.
Edited by:
Olga V. Khabarova, Tel Aviv University, IsraelReviewed by:
Albino Carbognani, National Institute of Astrophysics (INAF), ItalyAdam Popowicz, Silesian University of Technology, Poland
Copyright © 2023 Szárnya, Chum, Podolská, Kouba, Koucká Knížová, Mošna and Barta. This is an open-access article distributed under the terms of the Creative Commons Attribution License (CC BY). The use, distribution or reproduction in other forums is permitted, provided the original author(s) and the copyright owner(s) are credited and that the original publication in this journal is cited, in accordance with accepted academic practice. No use, distribution or reproduction is permitted which does not comply with these terms.
*Correspondence: Cs. Szárnya, szarnya.csilla@epss.hu