- 1Laboratory for Atmospheric and Space Physics (LASP), University of Colorado Boulder, Boulder, CO, United States
- 2University of California, Los Angeles, Los Angeles, CA, United States
- 3University of Michigan, Ann Arbor, MI, United States
- 4SSL, University of California, Berkeley, Berkeley, CA, United States
- 5University of Iowa, Iowa City, IA, United States
- 6University of Oregon, Eugene, OR, United States
- 7Los Alamos National Laboratory, Los Alamos, NM, United States
- 8Boston University, Boston, MA, United States
- 9APL, The Johns Hopkins University, Baltimore, MD, United States
- 10University of Alaska Fairbanks, Fairbanks, AK, United States
- 11Jet Propulsion Laboratory, California Institute of Technology, Pasadena, CA, United States
- 12NASA/GSFC, Washington, DC, United States
- 13Ludwig Maximilian University of Munich, Ingolstadt, Germany
- 14LESIA, Observatoire de Paris, Université PSL, CNRS, Sorbonne Université, Université de Paris, Meudon, France
- 15Aix Marseille Université, CNRS, CNES, LAM, Marseille, France
- 16National Center for Atmospheric Research, Boulder, CO, United States
- 17Embry–Riddle Aeronautical University, Daytona Beach, FL, United States
- 18Astrophysical and Planetary Sciences Department, University of Colorado Boulder, Boulder, CO, United States
- 19Swedish Institute of Space Physics, Kiruna, Sweden
- 20Freie Universitaet Berlin, Berlin, Germany
- 21SETI Institute, Mountain View, CA, United States
- 22University of Texas at Austin, Austin, TX, United States
- 23Japan Aerospace Exploration Agency, Tokyo, Japan
- 24Technische Universitaet Braunschweig, Braunschweig, Germany
The magnetospheric systems of ice giants, as the ideal and the unique template of a typical class of exoplanets, have not been sufficiently studied in the past decade. The complexity of these asymmetric and extremely dynamic magnetospheres provides us a great chance to systematically investigate the general mechanism of driving the magnetospheres of such common exoplanets in the Universe, and the key factors of influencing the global and local magnetospheric structures of this type of planets. In this paper, we discuss the science return of probing magnetospheric systems of ice giants for the future missions, throughout different magnetospheric regions, across from the interaction with upstream solar wind to the downstream region of the magnetotail. We emphasize the importance of detecting the magnetospheric systems of ice giants in the next decades, which enables us to deeply understand the space enviroNMent and habitability of not only the ice giants themselves but also the analogous exoplanets which are widely distributed in the Universe.
1 Introduction
The scientific investigation and the community’s attention paid to the magnetospheric systems of Uranus and Neptune have been underestimated in the past decade, compared to other terrestrial and gas giant planets. Previous studies intensively discussed the significance of investigating Uranus system, e.g., Arridge et al. (2014); Bocanegra-Bahamón et al. (2015) and Blanc et al. (2021). With the proposed top-priority Flagship mission to Uranus by Planetary Science and Astrobiology Decadal Survey 2023–2032, we suppose that it is essential to identify and emphasize the scientific goals in the community of heliophysics science to investigate the unique magnetospheric systems of the ice giants which needs to be addressed in the future mission.
Both Uranus and Neptune have very special magnetic and rotational configurations among the other planets in our Solar System, as Figure 1 shows. The resultant magnetospheric structure and dynamics provide us an ideal and unique space laboratory to study this type of planets, and a template to study the habitability of analogous exoplanets, since Kepler Space Telescope’s observation confirmed that the largest population of them are near-Uranus/Neptune-size [See Figure 1 in Atreya et al. (2020); Batalha et al. (2013); Zhu and Dong (2021)].
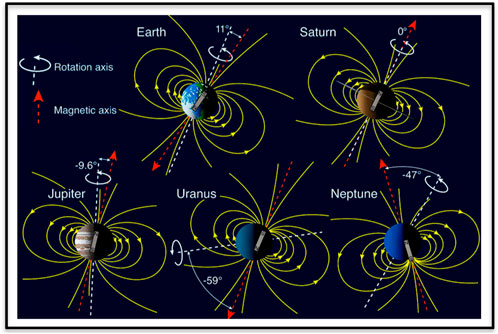
FIGURE 1. The comparison of the rotational (white dashed lines) and magnetic axes (orange dashed lines) between the Earth, Saturn, Jupiter, Uranus and Neptune. The yellow curves represent the magnetic field lines. Modified from (Kivelson and Bagenal, 2014 and also credit: Fran Bagenal & Steve Bartlett, see LASP’s MOP website: https://lasp.colorado.edu/mop/resources/graphics/).
The rotational and magnetic characteristics of ice giants are extraordinary and unique. Uranus has a very high obliquity (∼97.9°), compared to that of Neptune (∼29.6°). Both of them have extraordinarily large tilted angles between their spin axis and magnetic axis (Uranus: ∼59° and Neptune: ∼47°) (Ness et al., 1986; 1989). Besides, they have rapid rotation speed which can drive the plasma dynamics actively in the magnetosphere. Furthermore, both ice giants hold a significantly off-centered dipole moment.
This paper will systematically discuss critical scientific returns based on the magnetospheric structure and the potential measurement regions for the future mission, across from the interaction with upstream solar wind to the downstream region of the magnetotail.
2 The interaction with solar wind
The magnetospheric structure of ice giants are essentially formed by the interaction between the planetary intrinsic magnetic field and the high-speed solar wind. The magnetosphere of Uranus was believed to be driven by the solar wind based on the theoretic calculation (Vasyliunas, 1986), and the magnetosphere of Neptune might be internally driven due to the potential plasma source generated from its moon Triton (Mauk et al., 1991). In general, both magnetospheres are dominated or, at least, largely affected by the upstream solar wind, since the solar wind keeps exchanging the mass, momentum and energy with the resultant magnetosphere. Compared to the other planets in our Solar System, the ice giants’ interaction with the solar wind is way more complicated, because of their unique rotational and intrinsic magnetic characteristics described above, which directly determine the structure of planetary magnetospheres. Besides, based on the ideal Parker’s solar wind model (Parker, 1958), the IMF is almost perpendicular to the planet-Sun line at such a far distance from the Sun.
The first measurement of Uranus’ bow shock was made by the Voyager 2 spacecraft, and the detected bow shock location is about 23.7 RU (Uranus radii) upstream from the planet. The magnetopause boundary was located about 18 RU near the subsolar point at solstice (Ness et al., 1986; Masters, 2014). The bow shock and magnetopause locations at Neptune are respectively at 34.9 and 26.5 RN (Neptune radii) upstream, based on the Voyager 2’s fly-by measurement in 1989. A single fluid MHD model reproduced the approximate magnetopause and bow shock distance from Uranus for the crossing period at solstice in 1986 (Tóth et al., 2004). Another numerical simulation computed the magnetopause and bow shock profile when Voyager 2 flew by Neptune (Mejnertsen et al., 2016). However, it is still unknown how the structure of such ice giants’ magnetopause boundary would vary with the orbital season and planetary rotation, since Voyager 2 had been limited to only one fly-by measurement. A recent work quantitatively studied the dynamic variation of Uranian magnetopause boundary with the planetary rotation under different IMF orientation for the first time, which revealed that the magnetopause configuration (e.g., standoff distance, flaring parameter and cusp indentation) of Uranus is mainly dominated by the planetary rotation, but also modulated by the IMF’s orientation (Cao and Paty, 2021), as Figure 2 shows. The result of that paper appeals for an orbiter mission to Uranus in order to validate the extremely dynamic diurnal variation of the asymmetric magnetopause boundary and its dependence on the different IMF and solar wind conditions. The complicatedly varying boundary layers would result in much higher complexities for related physical phenomena, such as reconnections, flux transfer events, Kelvin-Helmholtz instabilities, surface waves, etc. In this paper, we also propose that the future orbital mission to Uranus and/or Neptune with a magnetometer on board should design orbits crossing the magnetopause boundary multiple times for further detection. Such a detection can help us deeply understand the interaction between the magnetospheres of ice giants and the solar wind, and understand how this interaction impacts the magnetopause boundary in the upstream solar wind.
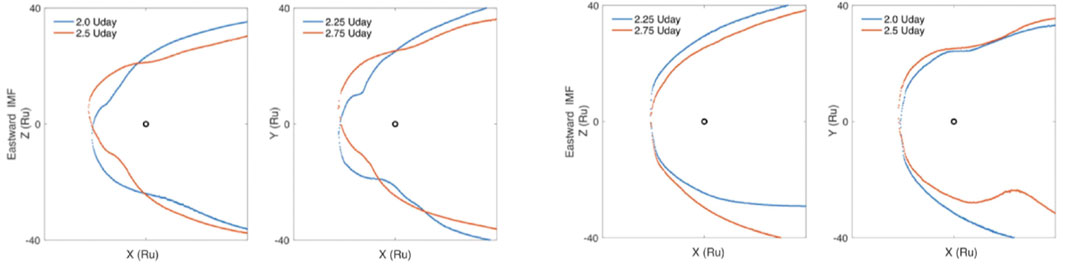
FIGURE 2. The diurnal variation of Uranian magnetopause at solstice, based on a multi-fluid MHD model. The magnetopause boundaries in the XZ (meridian) and XY (ecliptic) plane are respectively shown in each panel. The figure shows the variations of the shape of Uranus’ magnetopause during the planetary rotation. Uday in the figure represents Uranus day or Uranus’ rotational period. See more details under different IMF orientations in Cao and Paty (2021).
3 Inner magnetosphere and the internal magnetic field
The closest approach to Uranus is about 4 RU when the Voyager 2 spacecraft flew by (Ness et al., 1986). An offset titled dipole (OTD) field model well represents the inner magnetosphere of Uranus even if the spacecraft arrived at the closest regions from the planet in 1986. Specifically, the OTD well represents the magnetic field between 4 and 15 RN (Ness et al., 1989). However, the inner magnetospheric region within 4 planetary radii is not clearly known at both ice giants. Such a close region enables higher order magnetic multipoles to dominate the ice giants’ magnetospheric environment, which has not been sufficiently studied from the measurement by Voyager 2. This near-planet space links the ionosphere to the distant magnetospheric regions, and plays a crucial role in the magnetosphere-ionosphere coupling process, e.g., providing a field topology for planetward flows, outflows, and reconstruct a completely different substorm current wedge (SCW) structure from that of the Earth. The trapped energetic particles in the magnetosphere of Uranus are also affected by the presence of its moons (Krimigis et al., 1986; Mauk et al., 1987). In addition, the potential radiation belts at Uranus may have different signatures and dynamics from that at the Earth (Masters et al., 2022). Meanwhile, how the offset tilted magnetic field is driven by the planetary internal fluid system lacks sufficient direct exploration. Furthermore, the surface magnetic fields of both ice giants are dominated by the multipole moments rather than a dipole moment (Soderlund and Stanley, 2020). Measurement of the magnetic field at different locations will provide details about the high-order magnetic topology. Especially, a combination of empirical model and numerical simulation will reveal how the magnetic field is generated, similar to how Ganymede’s magnetic field’s generation mechanisms were investigated (Bland et al., 2008). This may also provide information about the internal structure of ice giants.
Therefore, we propose that it would return us significant scientific merits if the designed orbits of the future ice giant mission include the near-planet space, e.g., within 4 planetary radii (across different longitudes and latitudes from the planet) and include the potential radiation belt region to investigate such an important and representative magnetospheric region.
4 Moon-magnetosphere interaction
The moon-magnetosphere interaction is an important part in the planetary systems of the ice giants. Some of the moons can not only provide an internal plasma source to the magnetosphere impacting the magnetospheric dynamics, but also create additional linkages to the upper atmosphere/ionosphere of the planets affecting the magnetosphere-ionosphere coupling.
With respect to Uranus, the detection of potential subsurface oceans on the icy moons of Uranus is important for assessing their potential habitability, as discussed in Arridge and Eggington (2021). This understanding is vital for the broader study of celestial bodies that could support life. Regarding Neptune system, the magnetic and plasma environment at its moon Triton may be even more complicated. Therefore, without loss of generality, we focus on discussion on the Triton in this section. However, it is noteworthy that the moons of Uranus hold equivalent scientific importance. Figure 3 reveals that Triton’s orbit is highly titled from Neptune’s equatorial plane. Triton’s orbit is highly titled from Neptune’s equatorial plane. Considering Neptune’s large tilted magnetic axis, Triton crosses regions from low magnetic latitude to high magnetic latitude during every orbital period. Combined with Neptune’s non-negligible off-centered dipole moment, the temporal variation of the surrounding magnetic fields Triton experiences become asymmetric over each orbit.
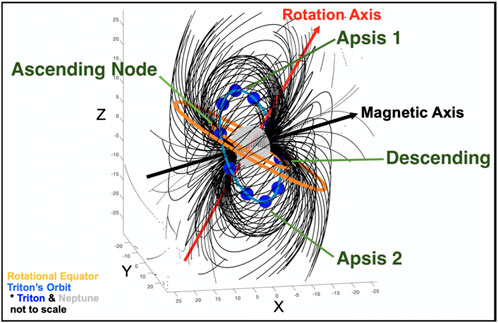
FIGURE 3. The Neptune-Triton System. The relative locations of Neptune, Triton and rings are shown in the figure. Note that the Triton’s orbit is not on the equatorial plane of Neptune.
Previous studies showed that there exists a “trans-Triton” heavy ion population corotating with Neptune, the pitch angle distribution of which implied a likely interaction with a neutral torus; The mechanism of how the nearby hot plasma and energetic particles were controlled by the presence of Triton and the sourced neutral particles is still unknown (Mauk et al., 1991). Furthermore, how largely those internal sources influence the global magnetosphere demands for further direct measurement.
The moon with self-sourced plasma in the planetary magnetosphere usually generates an Alfven wing or a mass loading structure. Such a physical structure has been observed at some moons such as Io (Neubauer, 1980), Enceladus (Jia et al., 2010), Titan (Sillanpää and Johnson, 2015) and Earth’s moon (Cao, et al., 2020). The aurora emission features on Jupiter have been observed to be associated with its moon via the Alfven wing structure (Connerney et al., 1993; Mura et al., 2018). The Alfven wing structure also likely exists at Triton, based on a recent numerical simulation (Liuzzo et al., 2021). But the difference of the moon-magnetosphere interaction of Triton from that of the other planetary moons is still lacking direct measurement of fields and particles in the moon’s ambience. Meanwhile, the moons in the inner magnetospheric region can absorb high energy particles and leave significant signatures in the radiation belts. Based on the reasons above, it would provide us a more complete portrait about the moon-magnetosphere interaction and/or moon-magnetosphere-ionosphere coupling if a measurement of fields and particles to at least one or more moons of ice giants is included in future missions.
5 Magnetotail
Based on the measurement made by Voyager 2 spacecraft, the magnetotail was rotating almost about the Uranus-Sun line during the solstice season (Ness et al., 1986). Such a rotation results in a twisted magnetotail configuration at Uranus (Behannon et al., 1987; Lepping, 1994; Tóth et al., 2004) with significant curvature of plasma sheet (Tóth et al., 2004; Cao and Paty, 2017), which have never been observed in the magnetospheric systems of other planets in our Solar System. Figure 4 shows a snapshot of the Uranus twisted magnetotail configuration at solstice. The twist of tail field-lines at Neptune was small, compared to that of Uranus, but the field lines’ pitch angle should oscillate with the planetary rotation (Lepping, 1994). The magnetotails of both Uranus and Neptune are expected to have a seasonal variation with different tail configurations during different seasons. However, how their unique magnetotail structure transitions between different seasons, and how the structure responds to the variation of upstream solar wind conditions has not been directly measured yet. Therefore, we suggest that significant scientific benefits could be gained if future missions were to observe the magnetotail regions at various distances from the planets over multiple planetary rotations. The designed trajectories should cover the near-planet, mid- and distant-tail, the tail lobes and tail plasma sheet. Such a detection will provide us more details about the diurnal variation of ice giants’ magnetotail configuration during a specific season or a certain period between solstice and equinox.
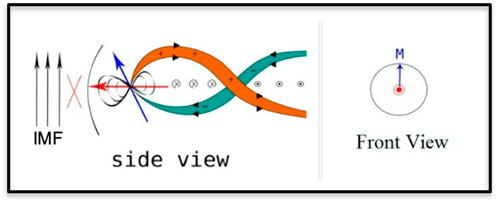
FIGURE 4. The expected twisted magnetotail structure of Uranus during solstice when Voyager 2 flew by. The red arrow and blue arrow respectively represent the rotation axis and magnetic axis of Uranus. The red cross symbol indicates the dayside reconnection when the IMF is northward oriented. See the similar magnetotail structure with the simplified assumption of zero IMF at (Tóth et al., 2004).
The dominant protons and electrons of the plasma sheet in the tail region at Uranus cross from a few to a few thousand electron volts (Behannon et al., 1987). And streaming ions and electrons towards Neptune were also observed in the tail plasma sheet (Mauk et al., 1991), which indicates a possible tail reconnection occurrence during the Voyager 2’s fly-by. How the extremely dynamic magnetotail of the ice giants differs from those of the other planets is still unclear. A recent study has reported the presence of a tailward-traveling plasmoid with trapped plasma inside the loop-like structure at Uranus (DiBraccio and Gershman, 2019). The plasmoids may serve as a major transport for mass loss through the magnetotail. The role of magnetic reconnection in the magnetotail of Uranus and/or Neptune needs further exploration.
6 Aurora emissions and magnetosphere-ionosphere coupling
The aurora on Uranus was first unambiguously observed in the H2 Lyman and Werner bands around both magnetic poles by Voyager 2/UVS at solstice (Broadfoot et al., 1986; Herbert and Sandel, 1994) and more recently imaged from Earth by HST past equinox (Lamy et al., 2012; Lamy et al., 2017). Due to the unusual configuration of Uranus, with a highly tilted rotation axis, and an almost 60 degrees offset of the magnetic poles, Uranus’ auroras are generated at unusual locations compared to other planets. Overall, the auroras do not show a complete oval as other planets, but patchy emissions clustered near the magnetic poles. Furthermore, the aurora shows strong hemispheric asymmetry that it is stronger and more extended around the northern magnetic pole where the magnetic field is weak. Since the aurora is excited due to the collision of the atmosphere and the precipitating energetic particles from the magnetosphere, the evolution of Uranus’ aurora provides fundamental planetary properties of the Uranus’ magnetospheric dynamics (e.g., rotation characteristics, solar wind and magnetosphere interaction, magnetosphere-ionosphere coupling). For example, Uranus’ aurora maps to near magnetotail, which have strong indications regarding its driving mechanisms (Herbert and Sandel, 1999). In addition, the shape of the patchy aurora, when mapped to the magnetotail, provides critical information about its driving region. Analogously, the aurora emission at Neptune also needs further direct measurement for high-accuracy observations (Lamy, 2020). Besides, the limited signal-to-noise ratio of Earth-based observations appeals for direct measurements to Uranus in order to investigate the detailed morphology variability of its auroras with high-quality imaging. Therefore, we propose that the aurora emission should be one of the potential observation goals by UV and/or IR imagers in future missions, which provides important information regarding the magnetospheric dynamics of Uranus and/or Neptune systems, as well as their underexplored solar wind-magnetosphere-ionosphere coupling process.
7 Global configuration and dynamics of these magnetospheres
A recent study reveals that Uranus has a very special global magnetospheric configuration: the “switch-like” magnetosphere. The magnetosphere of Uranus switches between an open and a closed global structure on a daily basis, due to its periodic magnetic reconnection with the upstream IMF (Cao and Paty, 2014; 2017), as Figure 5 shows. A similar structure was also expected at Neptune’s magnetosphere (Mejnertsen et al., 2016). Such a drastic diurnal variability of the unique global structure has never been observed at other planets in our Solar System, during the periods when the upstream IMF orientation keeps quasi-stable.
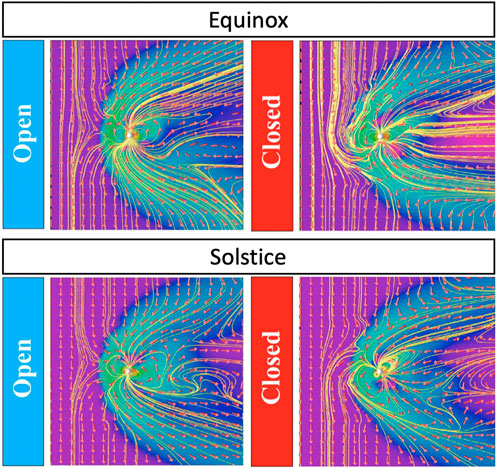
FIGURE 5. The “switch-like” magnetosphere of Uranus, based on a multi-fluid MHD simulation (Cao and Paty, 2017). The magnetosphere of Uranus is expected to switch between an open and a closed global configuration during one Uranian day, via the interaction with the upstream solar wind. The upper panels show the open and closed magnetospheric structures at equinox and the bottom show those at solstice season in the XZ plane.
Since the dayside reconnection plays an important role in exchanging mass, momentum and energy with the surrounding space environment, and determines the driving mechanism of the magnetospheric dynamics, here we propose that dayside reconnection regions should be included as one of the potential scientific targets for the future mission to Uranus and/or Neptune. The particle and field instruments (e.g., ion and electron analyzers, magnetometer and electric field instrument) on board are suggested to measure the plasma acceleration and the field topology where the reconnections and other physical processes are expected to occur. Such a measurement can return us significant science merits for comparatively studying the driving process of planetary magnetospheres between the Earth and Ice Giants.
Voyager 2 measured significant temporal variations of the fields and plasma in the magnetosphere of Uranus, which might be related to a potential substorm (Behannon et al., 1987). The investigation of how the substorm events behave in the ice giants’ magnetospheric systems, and what differences and similarities such a magnetospheric activity at ice giants are present, compared to those at the Earth, Jupiter or Saturn, is of key importance to deeply understand the driving mechanism of the magnetospheric dynamics in different planetary environments.
8 Conclusion
The epic space mission Voyager 2, has made exceptional contributions to our understanding of the ice giants, via its fly-by measurement. However, further more important scientific questions have been raised and remain unanswered, as discussed above. It is noteworthy that 1) both ice giants have distinct rotational and magnetic geometries from those of other planets in the Solar System, and 2) according to the observations by Kepler Telescope, the largest population of exoplanets is close to Uranus and Neptune’s size. Unlike the traditional viewpoint of usually taking the Earth and other planets as a template to study exoplanets, these facts inspire us to think, perhaps what we have seen on Uranus and Neptune is just the more general norm for planets in the Universe instead: very unique magnetospheres with highly obliquities and less-aligned magnetic fields. Understanding how these complex magnetospheres shield exoplanets from energetic stellar wind is of key importance for studying the habitability of these newly discovered worlds. Therefore, it is essential to utilize an orbital probe to comprehensively investigate ice giants’ complicated and unique magnetospheric systems in the next decades, with high-accuracy instruments on board, throughout the space regions of interest as discussed in the previous sections. The science return of detecting the ice giants’ magnetospheric systems will reward us for deeply understanding the space environment and habitability not only of the ice giants themselves but also of the analogous exoplanets which are widely distributed in the Universe.
Author contributions
The first author XC leaded the manuscript, and the co-authors reviewed, commented and edited the manuscript. All authors contributed to the article and approved the submitted version.
Acknowledgments
XC thanks the editor and reviewers for their constructive comments and their efforts to help this paper improved. LL thanks the support from CNES and from CNRS/INSU national programs of planetology and heliophysics.
Conflict of interest
The authors declare that the research was conducted in the absence of any commercial or financial relationships that could be construed as a potential conflict of interest.
Publisher’s note
All claims expressed in this article are solely those of the authors and do not necessarily represent those of their affiliated organizations, or those of the publisher, the editors and the reviewers. Any product that may be evaluated in this article, or claim that may be made by its manufacturer, is not guaranteed or endorsed by the publisher.
References
Arridge, C. S., Achilleos, N., Agarwal, J., Agnor, C. B., Ambrosi, R., André, N., et al. (2014). The science case for an orbital mission to Uranus: exploring the origins and evolution of ice giant planets. Planet. Space Sci. 104, 122–140. doi:10.1016/j.pss.2014.08.009
Arridge, C. S., and Eggington, J. W. (2021). Electromagnetic induction in the icy satellites of Uranus. Icarus 367, 114562. doi:10.1016/j.icarus.2021.114562
Atreya, S. K., Hofstadter, M. H., Mousis, O., Reh, K., and Wong, M. H. (2020). Deep atmosphere composition, structure, origin, and exploration, with particular focus on critical in situ science at the icy giants. Space Sci. Rev. 216, 18. doi:10.1007/s11214-020-0640-8
Batalha, N. M., Rowe, J. F., Bryson, S. T., Barclay, T., Burke, C. J., Caldwell, D. A., et al. (2013). Planetary candidates observed by Kepler. III. Analysis of the first 16 months of data. Astrophysical J. Suppl. Ser. 204 (2), 24. doi:10.1088/0067-0049/204/2/24
Behannon, K. W., Lepping, R. P., Sittler, E. C., Ness, N. F., Mauk, B. H., Krimigis, S. M., et al. (1987). The magnetotail of Uranus. J. Geophys. Res. Space Phys. 92 (A13), 15354–15366. doi:10.1029/JA092iA13p15354
Blanc, M., Mandt, K., Mousis, O., André, N., Bouquet, A., Charnoz, S., et al. (2021). Science goals and mission objectives for the future exploration of ice giants systems: a horizon 2061 perspective. Space Sci. Rev. 217, 3–59. doi:10.1007/s11214-020-00769-5
Bland, M. T., Showman, A. P., and Tobie, G. (2008). The production of Ganymede's magnetic field. Icarus 198 (2), 384–399. doi:10.1016/j.icarus.2008.07.011
Bocanegra-Bahamón, T., Bracken, C., Sitjà, M. C., Dirkx, D., Gerth, I., Konstantinidis, K., et al. (2015). MUSE–Mission to the Uranian system: unveiling the evolution and formation of ice giants. Adv. Space Res. 55 (9), 2190–2216. doi:10.1016/j.asr.2015.01.037
Broadfoot, A. L., Herbert, F., Holberg, J. B., Hunten, D. M., Kumar, S., Sandel, B. R., et al. (1986). Ultraviolet spectrometer observations of Uranus. Science 233, 74–79. doi:10.1126/science.233.4759.74
Cao, X., Halekas, J., Poppe, A., Chu, F., and Glassmeier, K. H. (2020). The acceleration of lunar ions by magnetic forces in the terrestrial magnetotail lobes. J. Geophys. Res. Space Phys. 125 (6), e2020JA027829. doi:10.1029/2020JA027829
Cao, X., and Paty, C. (2017). Diurnal and seasonal variability of Uranus's magnetosphere. J. Geophys. Res. Space Phys. 122 (6), 6318–6331. doi:10.1002/2017JA024063
Cao, X., and Paty, C. (2021). Asymmetric structure of Uranus' magnetopause controlled by IMF and planetary rotation. Geophys. Res. Lett. 48 (4), e2020GL091273. doi:10.1029/2020GL091273
Cao, X., and Paty, C. S. (2014). A seasonal study of Uranus' magnetosphere. AGU Fall Meet. Abstr. 2014, SM51E–4283.
Connerney, J. E. P., Baron, R., Satoh, T., and Owen, T. (1993). Images of excited H3+ at the foot of the Io flux tube in Jupiter's atmosphere. Science 262 (5136), 1035–1038. doi:10.1126/science.262.5136.1035
DiBraccio, G. A., and Gershman, D. J. (2019). Voyager 2 constraints on plasmoid-based transport at Uranus. Geophys. Res. Lett. 46, 10710–10718. doi:10.1029/2019GL083909
Herbert, F., and Sandel, B. R. (1994). The Uranian aurora and its relationship to the magnetosphere. J. Geophys. Res. 99, 4143–4160. doi:10.1029/93JA02673
Herbert, F., and Sandel, B. R. (1999). Ultraviolet observations of Uranus and Neptune. Planet Space Sci. 47, 1119–1139. doi:10.1016/S0032-0633(98)00142-1
Jia, Y. D., Russell, C. T., Khurana, K. K., Toth, G., Leisner, J. S., and Gombosi, T. I. (2010). Interaction of Saturn's magnetosphere and its moons: 1. Interaction between corotating plasma and standard obstacles. J. Geophys. Res. Space Phys. 115 (4). doi:10.1029/2009JA014630
Kivelson, M. G., and Bagenal, F. (2014). “Planetary magnetospheres,” in Encyclopedia of the solar system (Elsevier), 137–157.
Krimigis, S. M., Armstrong, T. P., Axford, W. I., Cheng, A. F., Gloeckler, G., Hamilton, D. C., et al. (1986). The magnetosphere of Uranus: hot plasma and radiation environment. Science 233 (4759), 97–102. doi:10.1126/science.233.4759.97
Lamy, L. (2020). Auroral emissions from Uranus and Neptune. Philosophical Trans. R. Soc. A 378 (2187), 20190481. doi:10.1098/rsta.2019.0481
Lamy, L., Prangé, R., Hansen, K. C., Clarke, J. T., Zarka, P., Cecconi, B., et al. (2012). Earth-based detection of Uranus’ aurorae. Geophys. Res. Lett. 39 (7).
Lamy, L., Prangé, R., Hansen, K. C., Tao, C., Cowley, S. W. H., Stallard, T. S., et al. (2017). The aurorae of Uranus past equinox. J. Geophys. Res. Space Phys. 122 (4), 3997–4008.
Lepping, R. P. (1994). Comparisons of the field configurations of the magnetotails of Uranus and Neptune. Planet. Space Sci. 42 (10), 847–857. doi:10.1016/0032-0633(94)90065-5
Liuzzo, L., Paty, C., Cochrane, C., Nordheim, T., Luspay-Kuti, A., Castillo-Rogez, J., et al. (2021). Triton’s variable interaction with Neptune’s magnetospheric plasma. J. Geophys. Res. Space Phys. 126 (11), e2021JA029740. doi:10.1029/2021JA029740
Masters, A. (2014). Magnetic reconnection at Uranus' magnetopause. J. Geophys. Res. Space Phys. 119 (7), 5520–5538. doi:10.1002/2014JA020077
Masters, A., Ioannou, C., and Rayns, N. (2022). Does Uranus' asymmetric magnetic field produce a relatively weak proton radiation belt? Geophys. Res. Lett. 49, e2022GL100921. doi:10.1029/2022GL100921
Mauk, B. H., Keath, E. P., Kane, M., Krimigis, S. M., Cheng, A. F., Acuna, M. H., et al. (1991). The magnetosphere of Neptune: hot plasmas and energetic particles. J. Geophys. Res. Space Phys. 96 (S01), 19061–19084. doi:10.1029/91JA01820
Mauk, B. H., Krimigis, S. M., Keath, E. P., Cheng, A. F., Armstrong, T. P., Lanzerotti, L. J., et al. (1987). The hot plasma and radiation environment of the Uranian magnetosphere. J. Geophys. Res. Space Phys. 92 (A13), 15283–15308. doi:10.1029/JA092iA13p15283
Mejnertsen, L., Eastwood, J. P., Chittenden, J. P., and Masters, A. (2016). Global MHD simulations of Neptune's magnetosphere. J. Geophys. Res. Space Phys. 121 (8), 7497–7513. doi:10.1002/2015JA022272
Mura, A., Adriani, A., Connerney, J. E. P., Bolton, S., Altieri, F., Bagenal, F., et al. (2018). Juno observations of spot structures and a split tail in Io-induced aurorae on Jupiter. Science 361 (6404), 774–777. doi:10.1126/science.aat1450
Ness, N. F., Acuna, M. H., Behannon, K. W., Burlaga, L. F., Connerney, J. E., Lepping, R. P., et al. (1986). Magnetic fields at Uranus. Science 233 (4759), 85–89. doi:10.1126/science.233.4759.85
Ness, N. F., Acuna, M. H., Burlaga, L. F., Connerney, J. E., Lepping, R. P., and Neubauer, F. M. (1989). Magnetic fields at Neptune. Science 246 (4936), 1473–1478. doi:10.1126/science.246.4936.1473
Neubauer, F. M. (1980). Nonlinear standing Alfvén wave current system at Io: theory. J. Geophys. Res. Space Phys. 85 (A3), 1171–1178. doi:10.1029/JA085iA03p01171
Parker, E. N. (1958). Dynamics of the interplanetary gas and magnetic fields. Astrophysical J. 128, 664. doi:10.1086/146579
Sillanpää, I., and Johnson, R. E. (2015). The role of ion-neutral collisions in Titan’s magnetospheric interaction. Planet. Space Sci. 108, 73–86. doi:10.1016/j.pss.2015.01.007
Soderlund, K. M., and Stanley, S. (2020). The underexplored frontier of ice giant dynamos. Philosophical Trans. R. Soc. A 378 (2187), 20190479. doi:10.1098/rsta.2019.0479
Tóth, G., Kovács, D., Hansen, K. C., and Gombosi, T. I. (2004). Three-dimensional MHD simulations of the magnetosphere of Uranus. J. Geophys. Res. Space Phys. 109 (A11). doi:10.1029/2004JA010406
Turrini, D., Politi, R., Peron, R., Grassi, D., Plainaki, C., Barbieri, M., et al. (2014). The comparative exploration of the ice giant planets with twin spacecraft: unveiling the history of our Solar System. Planet. Space Sci. 104, 93–107. doi:10.1016/j.pss.2014.09.005
Vasyliunas, V. M. (1986). The convection-dominated magnetosphere of Uranus. Geophys. Res. Lett. 13 (7), 621–623. doi:10.1029/GL013i007p00621
Keywords: ice giant magnetospheres, future mission, Uranus magnetosphere, Neptune magnetosphere, Uranus, Neptune, ice giants, planetary magnetosphere
Citation: Cao X, Chu X, Hsu H-W, Cao H, Sun W, Liuzzo L, Halekas J, Paty C, Chu F, Agiwal O, Blum L, Crary F, Cohen IJ, Delamere P, Hofstadter M, Hospodarsky G, John C, Kollmann P, Kronberg E, Kurth W, Lamy L, Lin D, Li W, Ma X, Malaspina D, Morooka M, Nordheim T, Postberg F, Poppe A, Richard C, Ruhunusiri S, Soderlund K, O'Donoghue J and Plaschke F (2024) Science return of probing magnetospheric systems of ice giants. Front. Astron. Space Sci. 11:1203705. doi: 10.3389/fspas.2024.1203705
Received: 11 April 2023; Accepted: 22 January 2024;
Published: 13 March 2024.
Edited by:
Sabrina Savage, National Aeronautics and Space Administration, United StatesReviewed by:
Anna Milillo, Institute for Space Astrophysics and Planetology (INAF), ItalyBertrand Bonfond, University of Liège, Belgium
Copyright © 2024 Cao, Chu, Hsu, Cao, Sun, Liuzzo, Halekas, Paty, Chu, Agiwal, Blum, Crary, Cohen, Delamere, Hofstadter, Hospodarsky, John, Kollmann, Kronberg, Kurth, Lamy, Lin, Li, Ma, Malaspina, Morooka, Nordheim, Postberg, Poppe, Richard, Ruhunusiri, Soderlund, O'Donoghue and Plaschke. This is an open-access article distributed under the terms of the Creative Commons Attribution License (CC BY). The use, distribution or reproduction in other forums is permitted, provided the original author(s) and the copyright owner(s) are credited and that the original publication in this journal is cited, in accordance with accepted academic practice. No use, distribution or reproduction is permitted which does not comply with these terms.
*Correspondence: Xin Cao, WGluLkNhb0BsYXNwLmNvbG9yYWRvLmVkdQ==