Biocontrol Processes in Fruits and Fresh Produce, the Use of Lactic Acid Bacteria as a Sustainable Option
- Facultad de Ciencias Químicas, Universidad Autónoma de Chihuahua, Chihuahua, Mexico
After harvesting fruits and vegetables and during storage and transportation, its sensorial, nutritional and sensorial quality decreases due to its high moisture content, microbial growth, environmental factors, maturity and senescence. Considering their very short shelf life, fruits and vegetables need immediate post-harvest care to increase it. The most common conservation methods are based on the control of the transpiration rate, respiration and microbial spoiling. However, traditional methods have proven to be vulnerable. Between 25 and 40% of fruits and vegetables are lost before consumption because of inadequate post-harvest treatments. In recent years, the development of alternative methods to assure microbial safety of fresh fruits and vegetables has been an important topic of investigation. Among these new methods, biopreservation using Lactic Acid Bacteria has been gaining interest, since they are classified as “generally recognized as safe” and have shown antimicrobial capacities; additionally, it is considered an environmentally friendly method. On the other hand, microencapsulation in a biodegradable matrix protects Lactic acid bacteria against unfavorable environmental conditions, which maintains their viability for a longer period. Therefore, the use of microencapsulated Lactic acid bacteria promises to be an effective technique to guarantee safety and to extend the useful life of fruits and vegetables during post-harvest. This review describes the main methods of preservation, as well as the emerging methods used to preserve fresh fruits and vegetables, with emphasis on bio-preservation as a proposal for future research.
Introduction
After harvesting, the sensorial and nutritional quality of foods from plant origin begins to decline because of the food deterioration and microbial growth (Shafiur-Rahman, 2007). Fruits and vegetables are prone to deterioration rapidly; they have a very short shelf life due to their high moisture content. In addition, they are still living organisms that carry out transpiration, respiration and maturation after harvesting, thus their metabolism continues to increase the rate of deterioration due to maturity, senescence and unfavorable environmental factors. Therefore, since they are perishable, they need immediate post-harvest treatments to reduce the microbial load and increase their shelf life (Lal Basediya et al., 2013).
Between 25 and 40% of fruits and vegetables are lost before consumption due to deficient post-harvest management. After the fruit has been harvested, quality needs to be assured for a time period long enough, that the fruit can be transported and distributed. Post-harvest losses can be reduced by controlling the rate of transpiration and respiration, reduction in microbial contamination and providing external membrane protection, with the consequent extension in shelf life (Bisen et al., 2010).
Minimally processed fruits and vegetables attract consumers because they are fresh, nutritious, and ready for consumption. However, the use of agrochemicals during plant growth has caused increase in fungal resistance to chemical fungicides and the presence of toxic residues (Alikhani, 2014). There is a need to develop environmentally friendly techniques that can help on the reduction of spoilage and pathogenic bacteria and fungi in fresh fruits and produce, as requested by consumers (Barba et al., 2010; Boyacioglu et al., 2013). This review describes the most common preservation methods, as well as the emerging methods used to preserve fresh fruits and vegetables; emphasis is given to Lactic Acid Bacteria (LAB) as a potential sustainable option for food sanitation.
Traditional Preservation Methods
Physical Treatments
Table 1 summarizes the most common traditional methods used for the preservation of fruits and vegetables. Thermal processing is still one of the most used methods, because of its efficient reduction of the bacterial population. Thermal processing uses moist heat (121°C, 15 min) inside its final container or before packing. Foods with acid pH (≥4.6) require heating at 93°C for 15 min to ensure commercial sterility. Finally, the food product must be packaged under sterile conditions (Barrett and Lloyd, 2012). However, an increase in the thermal resistance of microorganisms due to adverse environmental conditions has been reported (Amado et al., 2014) as well as the loss of both sensorial and nutritional characteristics (Barba et al., 2010).
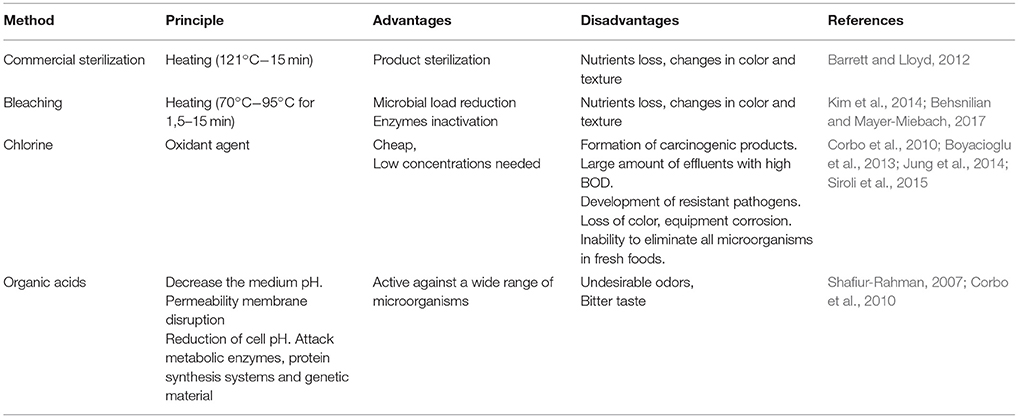
Table 1. Traditional preservation methods used to extend shelf life and food safety of fruits and vegetables.
Blanching in minimally processed fruits and vegetables is also considered as a decontamination treatment, since it reduces microbial load, but there are also unwanted effects such as nutrient loss, thermal degradation and loss of color and texture (Corbo et al., 2010; Neri et al., 2014).
Chemical Products
To reduce detrimental bacterial numbers in fruits, several chemical compounds have been used in post-harvest procedures. As potential preservative products, ethanol, mineral oils, hot or chlorinated water, chitosan and ozone have been evaluated. However, the undesirable odors and/or bitter taste conferred to the product food during storage is a major limitation for its practical applications (Sabir et al., 2010).
Chlorine
The application of chlorine in its different presentations is the most widely used decontamination method to reduce microbial loads in fruits and produce (Shafiur-Rahman, 2007; Corbo et al., 2010; Jung et al., 2014). Concentrated liquid sodium hypochlorite is the most used chlorine presentation, although granulated calcium hypochlorite or chlorine gas are also used. Since calcium hypochlorite granules must be first dissolved in water, it requires additional work; on the other hand, chlorine gas is difficult to handle. Additionally, high levels of chlorine can cause irritation in lungs and skin of workers, discoloration of food products, and corrosion of equipment, along with the formation of chlorophenols and volatile chloramines that can cause serious health problems (Shafiur-Rahman, 2007). Although chlorine treatments are the most commonly used methods to decontaminate fresh foods, its use is prohibited in some European countries in ready-to-eat products, due to the potential toxicity of halogenated compounds produced by the reaction of chlorine with organic matter. In addition, chlorine as disinfectant produces large volumes of highly contaminated effluents. Recent studies have demonstrated the inability of these products to eliminate or inactivate microorganisms in fresh foods (Corbo et al., 2010; Ge et al., 2013; Jung et al., 2014) because the concentrations used are not sufficient to eliminate all pathogenic bacterial cells (Boyacioglu et al., 2013), and can only achieve 1–2 log reduction in microbial load (Siroli et al., 2015). Free chlorine oxidative activity is affected by factors such as biofilm formation, internalization of pathogenic bacteria, organic substances released by damaged or cut surfaces of plants and hydrophobic condition of plant surfaces. In addition, there is a growing concern on the production of toxic substances for humans and the development of more resistant pathogens (Boyacioglu et al., 2013; Ge et al., 2013; Jeddi et al., 2014; Li et al., 2015; Siroli et al., 2015).
Organic Acids
The use of immersion treatments in solutions of organic acids can reduce microbial populations in fruits. Calcium lactate and propionate solutions have been successfully applied in cantaloupe and apple slices. Hydrogen peroxide solutions have also been used in melon (Corbo et al., 2010).
Organic acids such as succinic, malic, tartaric, ascorbic and citric acid are commonly found in fruits and vegetables and are used as preservatives in foods. They reduce microbial loads due to the decrease of environment pH. In addition, the presence of organic acids causes disruption of the membrane permeability; reduce cell's internal pH, affects metabolic enzymes, as well as protein synthesis. Organic acids also reduces pH of minimally processed fruits and vegetables (Shafiur-Rahman, 2007; Corbo et al., 2010).
New Preservation Technologies
Physical Treatments
New technologies for preservation include advanced technologies, which may or may not be in commercial use (Table 2). They include high-pressure processing and methods such as microwaves, pulsed electric fields and ohmic processing, which produce changes in the food while they are absorbed (Barrett and Lloyd, 2012).
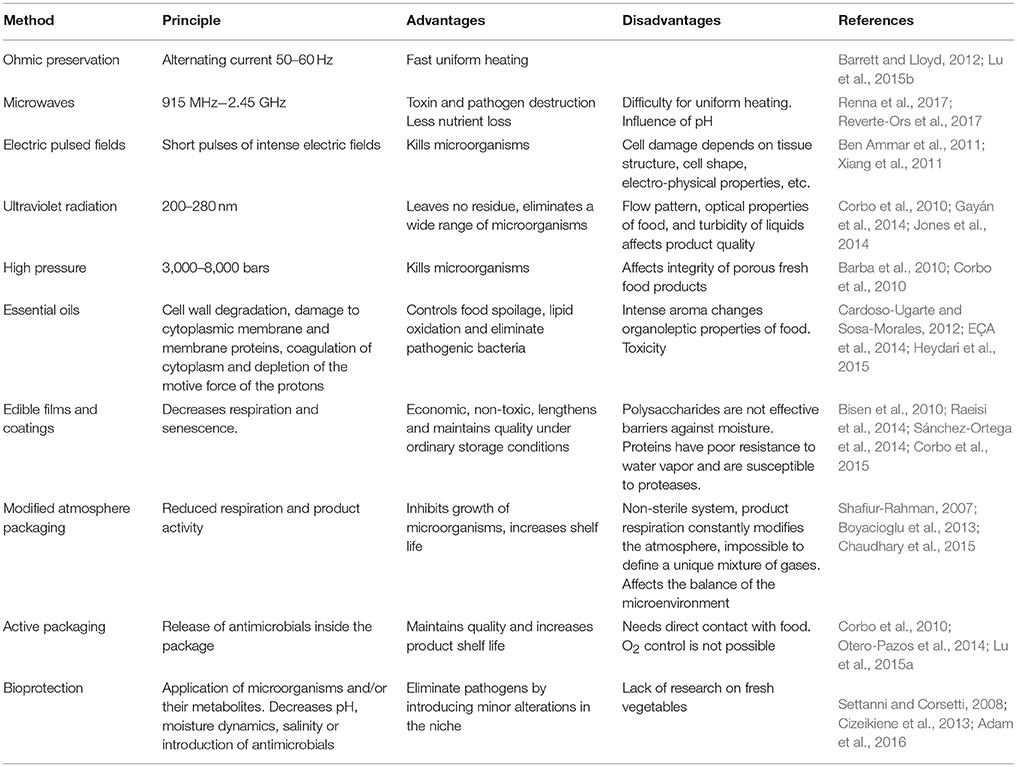
Table 2. New preservation technologies used to extend shelf life and food safety of fruits and vegetables.
Ohmic, Microwave, and Pulse Electric Fields
In comparison with traditional methods, these methods can generate a uniform application of the treatment factor over the entire product. The heat is generated rapidly and uniformly through the product, the generated steam heats adjacent areas by conduction, which produces a localized heating that causes the rupture of the microbial cells (Barrett and Lloyd, 2012).
Ohmic heating or Joule effect, is a process of heating foods by passing a low frequency electric current through them. The particles and liquid are heated at very high speeds uniformly, avoiding cold spots; then cooled by a similar technology and finally aseptically packed (Barrett and Lloyd, 2012; Lu et al., 2015b).
Although it has been demonstrated that microwave treatments can destroy toxins and pathogens (Renna et al., 2017) and food sterilized with microwaves has a lower loss of nutrients than traditional methods, if the process is not appropriate for the intended food product, quality deterioration may be greater. The challenge is to obtain a uniform heating throughout the product. In addition, food pH significantly influences the effectiveness of the process. Recently, the use of 915 MHz instead of 2.45 GHz has been studied to improve the uniformity of the electric field applied (Reverte-Ors et al., 2017).
Processing with pulsed electric fields is commonly applied to fruit juices to inactivate microorganisms and enzymes. The method involves the application of short pulses of intense electric fields in a flowing liquid to eliminate microorganisms; inactivation depends on the number of pulses and the intensity of the electric field (Xiang et al., 2011; Barrett and Lloyd, 2012). However, cell damage may depend on the shape of the cells, their orientation in the external field and the electro physical properties of cells among other factors (Ben Ammar et al., 2011).
Ultraviolet Radiation
Non-ionizing artificial ultraviolet radiation has many applications for disinfection of water, surfaces, food containers and food surfaces. Among the advantages of this treatment, is that the method is easy to use, does not leave residues and is effective against a wide variety of microorganisms (Corbo et al., 2010; Gayán et al., 2014). The bactericidal efficacy of the UV treatments is affected by equipment used, food properties and physicochemical characteristics of the treatment medium (Gayán et al., 2015). The use of ultraviolet radiation in liquids at industrial scale is limited by liquid turbidity, which blocks and absorbs UV light, protecting the microorganisms (Gayán et al., 2014; Jones et al., 2014).
High Pressure Preservation
This method causes the rupture of microbial membranes (Barrett and Lloyd, 2012). The use of high pressures in a range of 3,000–8,000 bars can be used to inactivate enzymes and microorganisms, while preserving food nutrients and flavor (Barba et al., 2010). Unfortunately, the high pressure treatment is not suitable for fresh porous products, since the air entrapped in the food matrix is affected by the process (Corbo et al., 2010).
Essential Oils
Chemical preservatives have caused allergic reactions in sensitive people, hence, the consumer's interest in natural antimicrobial compounds. Plants have been valued for centuries because of their antimicrobial activity. Herbs and spices or the essential oils derived from them have been used as preservatives for centuries. Essential oils are volatile odorous products of the secondary metabolism of plants, rich in a complex mixture of bioactive compounds such as esters, aldehydes, ketones, terpenes, phenolic acids (Shafiur-Rahman, 2007; Heydari et al., 2015) and other aromatic and aliphatic compounds (EÇA et al., 2014).
Essential oils can be used to control food spoilage, lipid oxidation and to eliminate pathogenic bacteria (Heydari et al., 2015). Essential oils components with proven antimicrobial activity include thymol from thyme and oregano, eugenol from cloves, cinnamaldehyde from cinnamon (Shafiur-Rahman, 2007), methyl jasmonate present in jasmine essential oil, carvacol and cinnamic acid present in cinnamon (Corbo et al., 2010). Essential oils in plants are part of the defense mechanisms against microorganisms and pests, hence their antimicrobial and antifungal capacity (Shafiur-Rahman, 2007; Cardoso-Ugarte and Sosa-Morales, 2012).
The antimicrobial activity of essential oils is not attributed to a single mechanism, since they are usually a mixture of diverse chemical compounds. Different mechanisms have been proposed such as the degradation of the cell wall, damage of the cell membrane or membrane proteins, or other internal mechanisms (Cardoso-Ugarte and Sosa-Morales, 2012).
Basil essential oil has been effective in inhibiting both Gram-positive bacteria (Micrococcus flavus, Sarcina lutea, Staphylococcus aureus, S. epidermis, and Bacillus subtilis) and Gram-negative bacteria (Escherichia coli, Pseudomonas aeuruginosa, Salmonella typhi, S. enteritidis, and Shigella sonei) although; Gram-positive bacteria have been more susceptible (Cardoso-Ugarte and Sosa-Morales, 2012). These antimicrobial compounds have the drawback that have an intense aroma that can cause changes in the sensorial characteristics of a food product; therefore they have a limited use in food preservation (Shafiur-Rahman, 2007; EÇA et al., 2014). Assessments of the antimicrobial capacity of essential oils have been carried out through in in vitro experiments, however, direct application is very limited (Cardoso-Ugarte and Sosa-Morales, 2012).
Edible Films and Coatings
Edible films and coatings are used for protection and extension of shelf life of fruits and vegetables worldwide. Among their advantages are their biocompatibility, non-toxicity, low environmental impact and low cost while maintaining quality even in ordinary storage conditions (Bisen et al., 2010; Raeisi et al., 2014). An edible coating is a thin layer of material suitable for consumption, formed by suspensions of hydrocolloids or food-grade lipids that can be applied to food surface by spraying, smearing or dipping and when the material dries, it forms a thin layer. Edible films are defined as a thin layer of edible material obtained from food-grade filmogenic suspensions, formed on the surface of the product as a coating or placed pre-formed on inert surfaces. These coatings decrease plant food respiration by providing a barrier to water vapor, oxygen and carbon dioxide, which results in a high humidity environment. Thus, they slow down oxidation reactions and protect aroma, texture and color while reducing microbial contamination. Edible films also serve as carriers of antimicrobial substances and are considered part of the final product (Sánchez-Ortega et al., 2014; Corbo et al., 2015; Heydari et al., 2015). The main difference between films and coatings is the cover thickness (Sánchez-Ortega et al., 2014).
Edible coatings are generally prepared with four types of materials: lipids, resins, polysaccharides and proteins. Polysaccharides produce transparent and homogeneous edible films with selective permeability against O2 and CO2 and resistance to fats and oils due to their ordered structural network of tightly packed hydrogen bonds. In addition, they are odorless and tasteless; however, they are not effective barriers against moisture due to their hydrophilic nature (Raeisi et al., 2014; Sánchez-Ortega et al., 2014).
Protein-based edible films provide a good barrier against oxygen and carbon dioxide and adhere well to hydrophilic surfaces. However, their application is limited by poor water vapor resistance due to the hydrophilicity of some proteins and are susceptible to proteolytic enzymes (Sánchez-Ortega et al., 2014; Corbo et al., 2015). Lipid coatings are efficient barriers against moisture and improve the appearance of surfaces (Corbo et al., 2015). The functionality of the edible coatings can be increased by adding some additives to help the preservation and quality of food, including antimicrobial compounds, antioxidants, organic acids, essential oils, etc. (Alikhani, 2014; EÇA et al., 2014; Raeisi et al., 2016). However, it is also important to evaluate the interaction of the added materials with the film or coating components (EÇA et al., 2014). For example, coatings have shown an increase in the efficiency of preservatives, but fungicides have reduced their activity (Shafiur-Rahman, 2007).
Modified Atmosphere Packing
Packaging in modified atmospheres (MAP) can minimize microbial deterioration of fresh food products by providing an atmosphere with different composition (Shafiur-Rahman, 2007; Corbo et al., 2015). During transportation of products, the storage temperature and the concentration of gases are controlled by limiting the supply of O2 (2–3%) and applying a high level of CO2 (5–20%). This reduces respiration rate and microbial growth (Boyacioglu et al., 2013; Chaudhary et al., 2015). The required basic level of metabolism is variable with the different food products, and is influenced by maturity, composition, constituents, natural microbiota, water activity and pH. It also depends strongly on storage temperature and the degree of processing previously applied, such as cube cutting or slicing (Corbo et al., 2015; Barbosa et al., 2016). The food product has a significant respiratory activity that constantly modifies the gaseous atmosphere inside the packaging material during the storage period, making it impossible to define a single gas mixture. It is necessary an expert knowledge to design the packaging of a product, and for each fruit type the appropriate packaging in a modified atmosphere should be studied (Shafiur-Rahman, 2007; Corbo et al., 2010). Conservation in MAP is a non-sterile preservation system by design. Fruits and vegetables are characterized by a normal microbiota that includes bacteria and fungi; many of them are also involved in food deterioration. Foodborne pathogens must compete with the microbiota present, but changes in the food microenvironment can promote its otherwise unusual growth (Shafiur-Rahman, 2007). Aerobic spoilage bacteria will be inhibited in environments with low oxygen concentration, but this condition can stimulate the growth of other microbial groups (Hempel et al., 2013). In this way, the microbiota of the final product will be influenced by the quality of the raw material, processing, packaging conditions and storage temperature (Caldera and Franzetti, 2014).
Active Packing
Food active packaging is designed to interact actively with the product or its environment to maintain quality and increase its shelf life (Otero-Pazos et al., 2014; Lu et al., 2015a). The first active packaging marketed was a device of sustained release placed in the headspace in the form of sachets inside the package containing the antimicrobial material, including organic acids and essential oils (Corbo et al., 2010; Otero-Pazos et al., 2014). The antimicrobial compound can also be embedded in a polymeric substance that migrated to interact with the microorganisms. Polymers used include peptides, organic acids and polyamides. The main drawback is that direct contact between the food and the polymer is needed (Corbo et al., 2010).
In active packaging, materials that can exchange compounds to the gas phase are also used, in order to maintain a specific concentration of CO2, O2, water vapor or ethylene (Lu et al., 2015a). The amount of active compounds needed will depend on the rate of gas production, the concentrations to be reached and the time in which the packing must be functional (Shafiur-Rahman, 2007).
Bioprotection
Bioprotection can be included in the natural strategies for food protection that can assure food safety and extend shelf life. Bioprotection is based on the use of beneficial microorganisms that can control the presence of other microorganisms by the production of specific metabolites. The competence of bioprotective microorganisms in the microbial ecosystem formed in food products, is part of the natural equilibrium in the system. The characteristics of these microorganisms can be used to propose preservation methods than will assure food safety, while maintaining the food nutritional and sensorial properties (Settanni and Corsetti, 2008; Cizeikiene et al., 2013; Elsser-Gravesen and Elsser-Gravesen, 2014).
Pathogens present in food systems can cause alterations that can affect the beneficial functions of the native microbial community. However, pathogens can be eliminated selectively by introducing minor alterations in the niche, such as the use of bacteria that modify pH, moisture dynamics or salinity (Adam et al., 2016).
Growth capacities of beneficial microorganisms in a specific food product, will determine its potential use as biocontrol culture. (Reina et al., 2005). The gap between the laboratory results and efficacy in the field for bioprotective cultures can be shortened by understanding their mode of action (Parnell et al., 2016). Among the most important biocontrol mechanisms are the liberation of antimicrobial compounds, as well as competition for nutrients and space (Trias et al., 2008a; Corbo et al., 2015). The success does not depend on a single attribute, but on the combination of several characteristics of the biocontrol culture, including its technical effectiveness, practicality of their use, persistence and commercial viability. The formulation of biocontrol products is a crucial issue, but there is still few information reported on this regard (Parnell et al., 2016).
Lactic Acid Bacteria
Among the methods proposed to inhibit the colonization of pathogenic bacteria in food products is the use of Lactic Acid Bacteria (LAB). LAB have an antagonistic capacity against pathogenic bacteria and are generally recognized as safe (GRAS) by the Food and Drug Administration (FDA) of the United States, which makes them ideal for developing bioprotective agents in fresh fruits and vegetables (Trias et al., 2008a; Jeddi et al., 2014; Li et al., 2015). Siroli et al. (2015), highlighted the importance of isolating and selecting biocontrol agents from the same environment where they will be reintroduced, since it was determined that the highest antagonistic capacity of the LAB strains tested was against pathogenic and deteriorative microorganisms present; this can be attributed to suitable strain colonization and competition for nutrients and space. LAB metabolites with antimicrobial capacity includes lactic acid, ethanol and bacteriocins among others (Abedi et al., 2013; Ghanbari et al., 2013; Menconi et al., 2014; Li et al., 2015). Moreover, LAB can survive under cold storage temperatures and can be directly applied as food additives, or their fermentation products or purified metabolites can be used instead (Snyder and Worobo, 2013; Olvera-García et al., 2015).
In agriculture, the interest in the use of LAB as an alternative for the control of enteric pathogens has been increasing (Menconi et al., 2014; Nguyen et al., 2015). Therefore, the use of LAB in fresh foods has prevented the growth of pathogenic microorganisms (Russo et al., 2014; Li et al., 2015). There is also possible to obtain LAB from non-dairy sources for use as bioprotection cultures, including cereals, fruits and vegetable samples (Swain et al., 2014).
The inhibitory activity of LAB has been widely reported (Table 3). LAB strains were isolated from samples of traditional pickles taken from rural and urban areas of Himachal Pradesh, and all isolates showed antimicrobial activity against Bacillus cereus, Escherichia coli, Staphylococcus aureus, and Shigella dysenteriae (Monika et al., 2017). Also, LAB with antimicrobial capacity has been isolated from a traditional fermented milk and corn product (Ahlberg et al., 2016). Lactobacillus plantarum was the strain that showed greater antifungal activity against A. flavus. LAB have been isolated also from apples in pieces and lettuce and isolates were evaluated for their ability to antagonize foodborne pathogens. No strain demonstrated the ability to produce bacteriocins, however, L. plantarum, Ln. mesenteroides, W. soli, and L. pentosus inhibited the growth of S. enteritidis, L. monocytogenes, and E. coli (Siroli et al., 2015). Li et al. (2015) isolated Lactobacillus plantarum subsp. plantarum, Pediococcus pentosaceus, Enterococcus mundtii, Weissella cibaria, and Leuconostoc pseudomesenteroides from corn stubble silage. Of 59 strains isolated, 56 showed antimicrobial activity against Salmonella enterica, Micrococcus luteus, and E. coli.
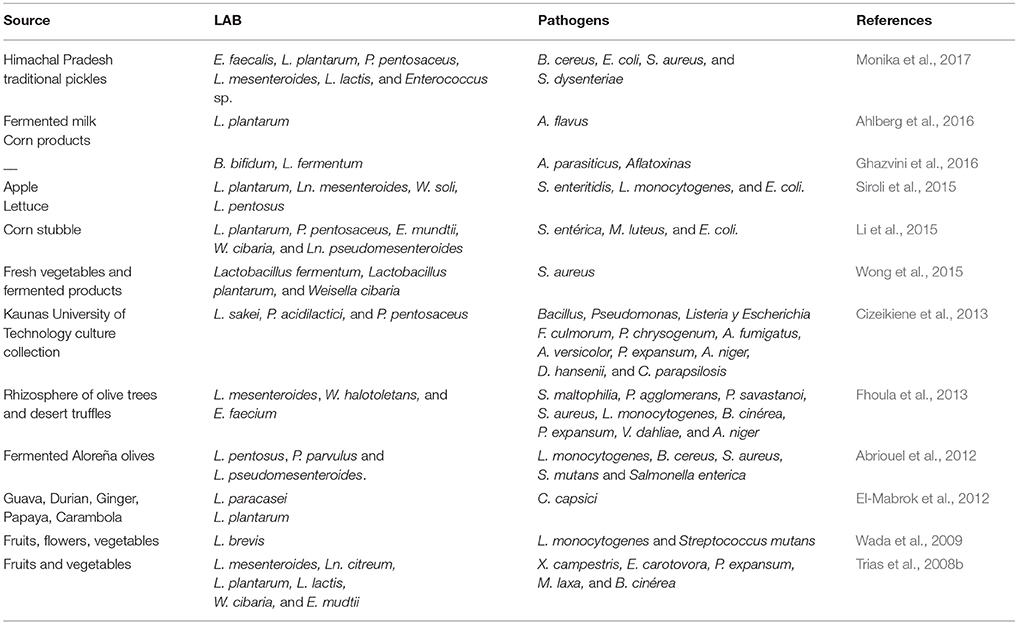
Table 3. Antimicrobial activity of Lactic Acid Bacteria (LAB) isolated from plant material and food products.
The presence of LAB significantly reduced mesophilic aerobic counts in a similar form as chlorine. LAB strains isolated from fresh vegetables and fermented products were evaluated for their antimicrobial capacity against Staphylococcus aureus; Lactobacillus fermentum, Lactobacillus plantarum, and Weisella cibaria were among the isolated with strong antimicrobial activity (Wong et al., 2015). Cizeikiene et al. (2013) evaluated the antimicrobial activity of Lactobacillus sakei, Pediococcus acidilactici, and Pediococcus pentosaceus against pathogenic bacteria, fungi and yeasts. The metabolites produced by the LAB strains inhibited the growth of Bacillus, Pseudomonas, Listeria, and Escherichia at different degrees. In addition, the metabolites showed fungicidal and fungistatic activity.
Leuconostoc mesenteroides, Weisella halotoletans, and Enterococcus faecium isolated from the rhizosphere of olive trees and desert truffles showed a strong antimicrobial capacity against bacterial and fungal plant pathogens, as well as against foodborne pathogens such as Staphylococcus aureus and Listeria monocytogenes (Fhoula et al., 2013). LAB strains isolated from fermented Aloreña olives produces antimicrobial metabolites against Listeria monocytogenes, Bacillus cereus, Staphylococcus aureus, Streptococcus mutans, and Salmonella (Abriouel et al., 2012). In another report, LAB were isolated from fruits, flowers, vegetables and fermented products, and one of the isolated strains was identified as L. brevis that produced brevicin (Wada et al., 2009). The ability to inhibit bacterial growth in apple lesions and cut Iceberg lettuce leaves was also evaluated to LAB strains isolated from fruits and vegetables (Trias et al., 2008a). The strains grew in the substrate without causing negative effects in the tissues of apple slices and lettuce leaves and were able to reduce the counts of S. typhimurium and E. coli while the growth of L. monocytogenes was completely inhibited.
Antifungal capacities and inhibition of aflatoxin production has also been reported by Bifidobacterium bifidum and Lactobacillus fermentum strains tested against Aspergillus parasiticus; LAB metabolites reduced aflatoxin levels in 88.8–99.8% (Ghazvini et al., 2016). El-Mabrok et al. (2012) reported the effectiveness of L. paracasei and L. pantarum isolated from fruits and vegetables as biocontrol agents against Colletotrichum capsici, a fungus that causes anthracnose in chili peppers (Capsicum annum). Seven strains of LAB showed good inhibitory activity while their supernatants showed strong inhibitory activity.
Antimicrobial Peptides
LAB can produce other antimicrobial compounds in addition to the well-known organic acids, hydrogen peroxide and diacetyl compounds (Olvera-García et al., 2015) of protein or peptide nature (Coda et al., 2011; Pessione, 2012; Muhialdin et al., 2016).
A high antimicrobial activity has been attributed to LAB-derived peptides against many pathogens, including some that are resistant to different antimicrobials (Sharma et al., 2011; Ghanbari et al., 2013). Although there are many antimicrobial peptides successfully identified, there are still many antimicrobial peptide-producing bacteria and peptides to be discovered (Hu et al., 2013). Bioprospecting of microorganisms isolated from natural environments is a usual strategy to obtain strains of industrial importance (Grosu-Tudor et al., 2014); continuous research aimed at the discovery of new antimicrobial peptides will lead to their commercialization for use in food and agriculture (Snyder and Worobo, 2013).
Antimicrobial peptides are multifaceted substances with complex mechanisms of action, similar to animal hormones, which are related to interactions with pathogenic cells through its membrane, or by interaction with internal targets related to DNA, RNA or proteins synthesis. They are resistant to heat, acidity and low aw, so they can be used as preservatives in food, replacing chemical additives (Sharma et al., 2011; Hernández Saldaña et al., 2016).
Several models of mechanisms of action have been proposed for antimicrobial peptides derived from LAB metabolism. The initial interaction between the antimicrobial peptides and the microbial cell is by attraction between the cationic peptide and the negatively charged bacterial envelope (Snyder and Worobo, 2013). The antibacterial activity can result from membrane pore formation, with the consequent loss of transmembrane potential and leakage of vital solutes (Sánchez-Ortega et al., 2014). The amphipathic nature of many antimicrobial peptides allow them to bind to molecules in the cell wall of Gram positive and Gram negative bacteria, but they can also be transported into the cell cytoplasm. Many antimicrobial peptides act as perturbing molecules of the proton gradient causing depolarization of the membrane (such as polymyxin B or colistin). Complex mechanisms have also been reported including the inhibition of macromolecule synthesis, as well as a synergistic action with the host's innate immune mechanisms (Sharma et al., 2011). In nature, antimicrobial compounds rarely work isolated; therefore, multifactorial strategies, as proposed in hurdle technologies, will be more effective in food preservation (Elsser-Gravesen and Elsser-Gravesen, 2014).
Table 4 presents a summary of peptides produced by LAB and its antimicrobial capacity. Examples include the identification of plantaricyclin A from Lactobacillus plantarum NI326 isolated from olives (Borrero et al., 2017), as well as eight peptide fractions produced by L. plantarum, that were effective against Aspergillus flavus, Penicillium roqueforti, and Eurotium rubrum (Muhialdin et al., 2016). In addition, a L. plantarum isolated from Kimchi produced two cyclic dipeptides, cis-cycle (L-Val-L-Pro) with antimicrobial activity against Ganoderma boninense and C. albicans and cis-cyclo (L-Phe-L-Pro) effective only against C. albicans (Kwak et al., 2014). Lactobacillus plantarum isolated from fermented cabbage, produced a bacteriocin called plantaricin 163. The bacteriocin was highly thermostable (20 min, 121°C), stable at a wide range of pH (2–10), sensitive to proteases and with broad antimicrobial spectrum (Hu et al., 2013). On another report, the antifungal activity of Lactobacillus plantarum during the fermentation of sour dough was evaluated, and several new antifungal peptides were identified. Fractions with low molecular weight (< 10 KDa) were able to inhibit the germination of P. roqueforti (Coda et al., 2011).
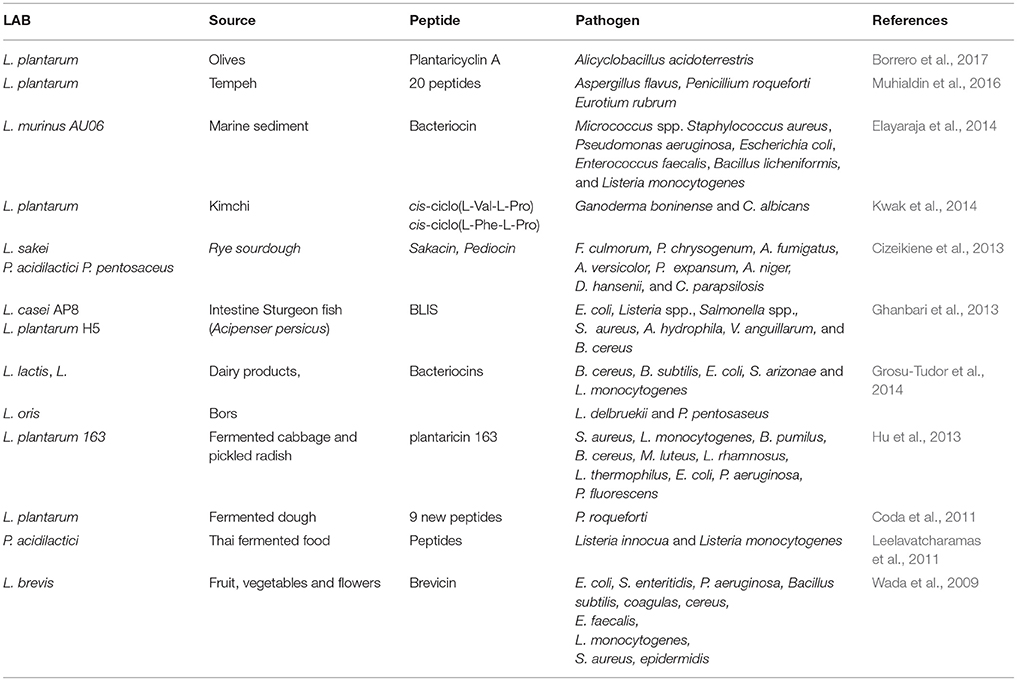
Table 4. Antimicrobial peptides obtained from Lactic Acid Bacteria (LAB) and their activity against plant and animal pathogenic microorganisms.
Lactobacillus murinus AU06, produced a bacteriocin with a molecular weight of 21 kDa, sensitive to proteases and with high antimicrobial activity against fish pathogens (Elayaraja et al., 2014). Bacteriocin-producing LAB were isolated from fermented dairy products and fermented cereals (Bors). The bacteriocin of L. lactis from fermented dairy products was a thermostable polypeptide of low molecular weight, tolerant to alkaline pH, with broad inhibitory spectrum that inhibited the growth of B. cereus, B. subtilis, E. coli, S. arizonae, and L. monocytogenes. The bacteriocin of L. oris (isolated from fermented cereal) was a thermolabile protein of high molecular weight with a narrow inhibitory spectrum (L. delbruekii and P. pentosaseus) (Grosu-Tudor et al., 2014). Pediococcus acidilactici isolated from Thai fermented foods, produced bacteriocins sensitive to protease K and thermostable (100–121°C) capable of inhibiting the growth of Listeria innocua and Listeria monocytogenes (Leelavatcharamas et al., 2011).
Although the antimicrobial action of bacteriocins are well known, many antimicrobial peptides does not comply with the characteristics traditionally described for bacteriocins; yet, they show antimicrobial action. These substances are described ad bacteriocin-like inhibitory substances (BLIS). Cizeikiene et al. (2013) reported BLIS production by L. sakei (sakacin), P. acidilactici, and P. pentosaceus (Pediocin) from a fermented dough from Lithuania. These antimicrobial peptides had activity against phytopathogenic yeasts and molds. BLIS production has also been reported by L. casei AP8 and L. plantarum H5 strains isolated from the intestinal microbiota of Sturgeon (Acipenser persicus). The peptides inhibited the growth of E. coli, Listeria spp. Salmonella spp., S. aureus, A. hydrophila, V. anguillarum, and B. cereus.
It is very likely that the antimicrobial activity is a complex matrix of synergism between the LAB strain and the production of metabolites produced under the conditions tested, including temperature and humidity, as well as the nature of the pathogenic bacterial or fungal strain analyzed (El-Mabrok et al., 2012; Ahlberg et al., 2016; Muhialdin et al., 2016).
LAB can be applied directly to food products as a protective culture, considering that the food product will promote LAB growth, and competence with the target microorganisms will occur (Shafiur-Rahman, 2007). The use of LAB seek the following benefits: improved food durability due to the action of the antimicrobial metabolites and the decrease of water activity (salting/drying); increase in food safety due to the reduction of pathogenic microorganisms and their toxins; improved nutritional value and sensorial characteristics.
Microencapsulation
Since the viability of LAB is essential for their role as bioprotective cultures, there has been several methods suggested to maintain cell viability. Microencapsulation is still recognized as the most useful method (Chun et al., 2014), since immobilization of LAB cells in a hydrocolloid droplet matrix results in improved protection against environmental conditions (Özer et al., 2009). Microencapsulation is defined as the process of enclosing micrometrized particles of solids, droplets of liquids or gases in an inert capsule (Alikhani, 2014). Due to the formation of a continuous thin envelope around an encapsulant (Shafiur-Rahman, 2007), it gives the ability to protect against stress caused by environmental conditions (heat, air, light, humidity), as well as against competitors and predators even in non-refrigerated environments (Crittenden et al., 2006; Parra, 2010a; Solanki et al., 2013; Alikhani, 2014). In addition, the encapsulated material is released gradually ensuring the presence of the bacteria by a longer time (Parra, 2010a; Solanki et al., 2013). The most important considerations for the LAB to exert their biological effect are viability and activity (Chun et al., 2014), and although this is of importance for probiotic bacteria that will cross the digestive tract before settling in the colon, the same stands for extended survival and distribution of BAL as bioprotective cultures in post-harvest treatments of fruits and vegetables.
Microencapsulation Methods
LAB encapsulation methods usually include a material that can produce a gel where bacterial cells can be trapped. Polyionic polymers, including alginate, carrageenan and gellan gum are usually used to form hydrocolloid beads (Chun et al., 2014). The effectiveness of microencapsulation depends on the method and materials used, as well as on the presence or lack of a cover factor that affect the size, porosity and texture of the capsules and consequently the protection of microorganisms (Albadran et al., 2015). Once formed, microcapsules will show specific properties depending on their chemical components, degree of crosslinking and size. Microbial survival within the matrix depends on permeability for nutrient supply and metabolite removal (Gasperini et al., 2014). The microencapsulation technique is classified depending on the method used to form the microcapsules: extrusion (drop method) or external ionic gelation and emulsion (two-phase system) or internal ionic gelation (Özer et al., 2009; Chun et al., 2014).
Extrusion
The method consists of extruding a suspension of hydrogel and microbial cells by passing the solution across a small tube or needle over a suitable hardening bath. The diameter of the microcapsule depends on the solution density as well as the diameter of the tube or needle used. This method usually produces capsules above one millimeter in diameter and are not always spherical (Gasperini et al., 2014). The extrusion method faces many challenges to bring the process to an industrial scale due to its low production capacity, reduction size limitation (up to 1 mm) and uneven capsule formation (Chun et al., 2014; Gasperini et al., 2014).
Emulsion
Emulsion is one of the best techniques for microencapsulation of LAB since it is simple, economical and can be used in large-scale production (Ruíz-Martínez et al., 2009). Emulsion encapsulation is usually achieved by dispersing a hydrogel precursor in an immiscible phase that can be stabilized using surfactants (Gasperini et al., 2014). The emulsification method is limited to batch production and it usually requires additional steps to remove the surfactant added (Chun et al., 2014).
Microfluidic
Microfluidic is related to carrying fluids through microchannels, generally in a laminal flow that allows the control of the microdroplets that can be generated; this formation usually involves the generation of emulsions of biopolymer droplets in a continuous immiscible phase (Gasperini et al., 2014). Spray techniques are useful because they can produce smaller microcapsules at higher speeds (Chun et al., 2014).
Coating Material
The coating material is a key factor in the development of successful microcapsules (Chun et al., 2014) hence, it must accomplish conditions like chemical compatibility, must not react with the encapsulating component and must afford the characteristics required for the capsules like strength, flexibility, impermeability and stability (Castro-Rosas et al., 2017).
The matrix most used for microencapsulation of LAB is alginate. It is a non-toxic, GRAS substance, economical, biocompatible, reproducible, requires mild gelation conditions, presents low immunogenicity, has the property of ionotropic gelation and can be a good carrier of bioactive natural compounds (Islam et al., 2010; Chun et al., 2014; Heydari et al., 2015; Raeisi et al., 2016). In addition, alginate is easy to use and has been shown to increase the survival of bacteria (Ruíz-Martínez et al., 2009; Parra, 2010b). Increasing the concentration of alginate leads to an increase in colony counts of Bifidobacterium longum (Özer et al., 2009). Alginate microparticles are not chemically stable to protect the encapsulable content due to the presence of non-gelling cations such as sodium or magnesium and chelating agents such as phosphate or citrate (Islam et al., 2010). The effectiveness of encapsulation with alginate matrix can be improved by co-encapsulating other compounds or by covering the capsules with cationic polymers (Chun et al., 2014). The stability of the microparticles was increased and less encapsulated material was lost (Islam et al., 2010).
LAB encapsulation has been suggested for preservation of functionality and controlled release of metabolites; most of the research has focused on encapsulation of probiotic strains to be protected while consumed. An extensive review on the methods used for encapsulation, as well as the coating material used was prepared by de Vos et al. (2010). There are few reports on the use of encapsulated LAB as bioprotective cultures in post-harvest processes, a novel application we consider can be used in fresh cut fruits and vegetables. Although the applications could be different, it is important to describe the success in LAB encapsulation reported in recent years, as summarized in Table 5. In all cases, LAB survival and functionality were the goals of encapsulation. Ilango et al. (2016) encapsulated E. hirae, E. faecalis, B. amyloliquefaciens, and L. plantarum in skimmed milk powder by applying lyophilization and spray drying. Storage for 16 weeks at room temperature reduced the LAB concentration in two logarithms, while at 4°C only one logarithm was reduced. The survival of L. plantarum in an alginate matrix coated with chitosan using lyophilization and fluid bed dryer methods at different water activities and temperatures was evaluated. Microencapsulated cells by fluid bed drying remained viable for more than one log difference for 45 days (Albadran et al., 2015). Immobilized cells of Lactobacillus curvatus by extrusion in a matrix of calcium alginate, were tested for survival and antimicrobial capacity against L. monocytogenes inoculated in salami. Microencapsulation did not affect the production of bacteriocins; although there was no difference in the inhibition of pathogens between encapsulated and non-encapsulated cells, there was a better survival of the encapsulated cells (Barbosa et al., 2015).
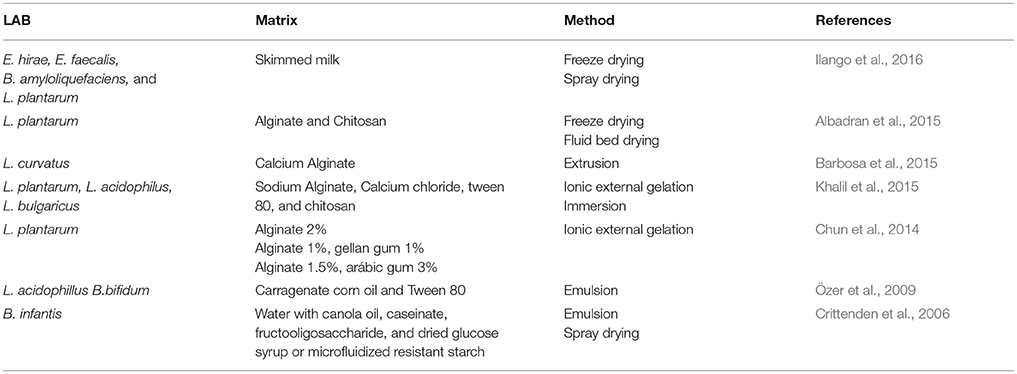
Table 5. Examples of microencapsulation of Lactic Acid Bacteria (LAB) for use in food systems as biopreservatives.
Lyophilized LAB strains were microencapsulated with sodium alginate, calcium chloride, tween 80 and chitosan using a two-step method, first external ionic gelation and then coating the microcapsules by immersion. The survival of microencapsulated cells was significantly higher than the survival of the non-encapsulated cells when they were subjected to a simulation of the gastrointestinal tract (Khalil et al., 2015). Chun et al. (2014) also applied the external ionic gelation method using a spray device to microencapsulate Lactobacillus plantarum. The bacterial viability in an environment with high acidity and bile presence was evaluated. Three formula were used, 2% alginate; 1% alginate and 1% gellan gum; and 1.5% alginate and 3% Arabic gum; the later showed the highest microencapsulation performance (98.11%) and the larger LAB survival.
In another report, Lactobacillus acidophillus and Bifidobacterium bifidum were microencapsulated using emulsion techniques (carrageenan, corn oil and Tween 80) and extrusion (alginate). Both bacteria decreased their counts by only one logarithm when they were added to Turkish cheese brined, while free cells lowered their counts in three logarithms (Özer et al., 2009). Crittenden et al. (2006) microencapsulated a strain of Bifidobacterium infantis in a water emulsion with canola oil, caseinate, fructooligosaccharide as a prebiotic and dried glucose syrup or microfluidized resistant starch. The emulsion was spray dried to form the particles and dry microcapsules of 15–20 μm were obtained. The method significantly protected the viability of bacteria stored in an open container at 25°C and a relative humidity of 50%.
Trabelsi et al. (2013) determined an enhancement of the viability and antibacterial activity of microencapsulated Lactobacillus plantarum TN9 in a matrix of alginate by extrusion techniques and coated with chitosan in comparison with alginate with gelatin as a coating material, alginate without coatings and free cells. Concentration of L. plantarum TN9 microencapsulated in alginate coated with chitosan diminished from 7.9 to 5.8 Log CFU/mL after 35 days at 4°C, while concentration the microorganism in microcapsules without coating was reduced to 1.2 Log CFU/mL and no survival was observed for the free cells and cells microencapsulated in alginate with gelatin coating. Therefore, these researches suggest that improvement of the potential of LAB as bioprotective agents in fresh produce may be fostered by microencapsulation techniques.
Conclusions
The need to preserve fruit and produce long enough to distribute and consume them, protecting their nutrient content and sensorial properties, is a technological challenge. Novel disinfection methods to be used in fruit and produce post-harvest technologies can help on the development of minimal processing for perishable food, where food safety is essential.
Lactic acid bacteria and its metabolites can be part of the preservation methods, either by adding the cells as protective culture, by adding the supernatant or by purifying their antimicrobial metabolites. Although LAB action has long be recognized in fermented foods, their use in preservation of fresh cut fruits and vegetables, need further research. The ecological role of LAB is still under investigation in nature, but they can be considered as a sustainable option for preservation of minimally processed food.
Author Contributions
JL-M and GN-M designed the outline and drafted the manuscript. NG-M, BR-C, and SP-V edited and helped on the final manuscript. All authors made an intellectual contribution to the work, and approved it for publication.
Funding
JL-M is a Research Fellow from the Consejo Nacional de Ciencia y Tecnología (Mexico) during his Ph.D. studies (Fellowship No. 618820).
Conflict of Interest Statement
The authors declare that the research was conducted in the absence of any commercial or financial relationships that could be construed as a potential conflict of interest.
References
Abedi, D., Feizizadeh, S., Akbari, V., and Jafarian-Dehkordi, A. (2013). In vitro anti-bacterial and anti- adherence effects of Lactobacillus delbrueckii subsp. bulgaricus on Escherichia coli. Res. Pharm. Sci. 8, 260–268.
Abriouel, H., Benomar, N., Cobo, A., Caballero, N., Fernández Fuentes, M. A., Pérez-Pulido, R., et al. (2012). Characterization of lactic acid bacteria from naturally fermented Manzanilla Alore-a green table olives. Food Microbiol. 32, 308–316. doi: 10.1016/j.fm.2012.07.006
Adam, E., Groenenboom, A., Kurm, V., Rajewska, M., Schimdt, R., Tyc, O., et al. (2016). Controlling the microbiome: microhabitat adjustments for successful biocontrol strategies soil and human gut. Front. Microbiol. 7:1079. doi: 10.3389/fmicb.2016.01079
Ahlberg, S., Joustsjoki, V., Laurikkala, S., Varmanen, P., and Korhonen, H. (2016). Aspergillus flavus growth inhibition by lactobacillus strains isolated from traditional fermented kenyan milk and maize products. Arch. Microbiol. 199, 457–464. doi: 10.1007/s00203-016-1316-3
Albadran, H. A., Chatzifragkou, A., Khutoryanskiy, V. V., and Charalampopoulos, D. (2015). Stability of probiotic Lactobacillus plantarum in dry microcapsules under accelerated storage conditions. Food Res. Int. 74, 208–216. doi: 10.1016/j.foodres.2015.05.016
Alikhani, M. (2014). Enhancing safety and shelf life of fresh-cut mango by application of edible coatings and microencapsulation technique. Food Sci. Nut. 2, 210–217. doi: 10.1002/fsn3.98
Amado, I., Vázquez, J., Guerra, N., and Pastrana, L. (2014). Thermal resistance of Salmonella enterica, Escherichia coli and Staphylococcus aureus isolated from vegetable feed ingredients. J. Sci. Food Agric. 94, 2274–2281. doi: 10.1002/jsfa.6554
Barba, F., Esteve, M., and Frigola, A. (2010). Ascorbic acid is the only bioactive that is better preserved by high hydrostatic pressure than by thermal treatment of a vegetable beverage. J. Agric. Food Chem. 58, 10070–10075. doi: 10.1021/jf1019483
Barbosa, C., Rui Alves, M., Rocha, S., and Oliveira, M. (2016). Modified atmosphere packaging of precooked vegetables: effect on physicochemical properties and sensory quality. Food Chem. 194, 391–398. doi: 10.1016/j.foodchem.2015.07.147
Barbosa, M., Todorov, S., Jurkiewicz, C., and Franco, B. (2015). Bacteriocin production by Lactobacillus curvatus MBSa2 entrapped in calcium alginate during ripening of salami for control of Listeria monocytogenes. Food Control 47, 147–153. doi: 10.1016/j.foodcont.2014.07.005
Barrett, D., and Lloyd, B. (2012). Advanced preservation methods and nutrient retention in fruits and vegetables. J. Sci. Food Agric. 92, 7–22. doi: 10.1002/jsfa.4718
Behsnilian, D., and Mayer-Miebach, E. (2017). Impact of blanching, freezing and frozen storage on the carotenoid profile of carrot slices (Daucus carota L. cv. Nutri Red). Food Control 73, 761–767. doi: 10.1016/j.foodcont.2016.09.045
Ben Ammar, J., Lanoisellé, J. L., Lebovka, N. I., Van Hecke, E., and Vorobiev, E. (2011). Impact of a pulsed electric field on damage of plant tissues: effects of cell size and tissue electrical conductivity. J. Food Sci. 76, E90–E97. doi: 10.1111/j.1750-3841.2010.01893.x
Bisen, A., Kumar Pandey, S., and Patel, N. (2010). Effect of skin coatings on prolonging shelf life of Kagzi lime fruits (Citrus aurantifolia S.). J. Food Sci. Technol. 49, 753–759. doi: 10.1007/s13197-010-0214-y
Borrero, J., Kelly, E., O'Connor, P., Kelleher, P., Scully, C., Cotter, P., et al. (2017). Purification, characterization and heterologous production of plantaricyclin 1A, a novel circular bacteriocin produced by Lactobacillus plantarum NI326. Appl. Environ. Microbiol. 84:e01801-17. doi: 10.1128/AEM.01801-17
Boyacioglu, O., Sharma, M., Sulakvelidze, A., and Goktepe, I. (2013). Biocontrol of Escherichia coli O157:H7 on fresh-cut leafy greens. Bacteriophage 3:e24620. doi: 10.4161/bact.24620
Caldera, L., and Franzetti, L. (2014). Effect of storage temperature on the microbial composition of ready-to-use vegetables. Curr. Microbiol. 68, 133–139. doi: 10.1007/s00284-013-0430-6
Cardoso-Ugarte, G. A., and Sosa-Morales, M. E. (2012). Propiedades del aceite esencial de albahaca (Ocimun basilicum L.) y sus aplicaciones en alimentos. Temas Selectos de Ingeniería de Alimentos 6, 54–65.
Castro-Rosas, J., Ferreira-Grosso, C., Gómez-Aldapa, C., Rangel-Vargas, E., Rodríguez-Marín, M., Guzmán-Ortiz, F., et al. (2017). Recent advances in microencapsulation of natural sources of antimicrobial compounds used in food - a review. Food Res. Int. 102, 575–587. doi: 10.1016/j.foodres.2017.09.054
Chaudhary, P., Jayaprakasha, G., Porat, R., and Patil, B. (2015). Influence of Modified atmosphere packaging on 'Star Ruby' grapefruit phytochemicals. J. Agric. Food Chem. 63, 1020–1028. doi: 10.1021/jf505278x
Chun, H., Kim, C., and Cho, Y. (2014). Microencapsulation of Lactobacillus plantarum using external ionic gelation method. Korean J. Food Sci. Anim. Resour. 34, 692–699. doi: 10.5851/kosfa.2014.34.5.692
Cizeikiene, D., Juodeikiene, G., Paskevicius, A., and Bartkiene, E. (2013). Antimicrobial activity of lactic acid bacteria against pathogenic and spoilage microorganisms isolated from food and their control in wheat bread. Food Control 31, 539–545. doi: 10.1016/j.foodcont.2012.12.004
Coda, R., Cassone, A., Rizzelo, C. G., Nionelli, L., Cardinali, G., and Gobbetti, M. (2011). Antifungal antivity of Wickerhamomyces anomalus and Lactobacillus plantarum during sourdough fermentation: identification of novel compounds and long-term effect during storage of wheat bread. Appl. Environ. Microbiol. 77, 3484–3492. doi: 10.1128/AEM.02669-10
Corbo, M., Speranza, B., Campaniello, D., Amato, D., and Sinigaglia, M. (2010). Fresh-cut fruits preservation: current status and emerging technologies. Curr. Res. Technol. Educ. Top. Appl. Microbiol. Microbial Biotechnol. 2, 1143–1154.
Corbo, M. R., Campaniello, D., Speranza, B., Bevilacqua, A., and Sinigaglia, M. (2015). Non-conventional tools to preserve and prolong the quality of minimally-processed fruits and vegetables. Coatings 5, 931–961. doi: 10.3390/coatings5040931
Crittenden, R., Weerakkody, R., Sanguansri, L., and Augustin, M. (2006). Synbiotic microcapsules that enhance microbial viability during non-refrigerated storage and gastrointestinal transit. Appl. Environ. Microbiol. 72, 2280–2282. doi: 10.1128/AEM.72.3.2280-2282.2006
de Vos, P., Faas, M. M., Spasojevic, M., and Sikkema, J. (2010). Encapsulation for preservation of functionality and targeted delivery of bioactive food components. Int. Dairy J. 20, 292–302. doi: 10.1016/j.idairyj.2009.11.008
EÇA, K., Sartori, T., and Menegalli, F. (2014). Films and edible coatings containing antioxidants- a review. Campinas 17, 98–112. doi: 10.1590/bjft.2014.017
Elayaraja, S., Annamalai, N., Mayavu, P., and Balasubramanian, T. (2014). Production, purification and characterization of bacteriocin from Lactobacillus murinus AU06 and its broad antibacterial spectrum. Asian Pac. J. Trop. Biomed. 4(Suppl. 1), S305–S311. doi: 10.12980/APJTB.4.2014C537
El-Mabrok, A. S. W., Hassan, Z., Mokhtar, A. M., Hussain, K. M. A., and Abdul Kahar, F. K. S. B. (2012). Screening of lactic acid bacteria as biocontrol against Colletotrichum capsici on Chilli Bangi. Res. J. Appl. Sci. 7, 466–473. doi: 10.3923/rjasci.2012.466.473
Elsser-Gravesen, D., and Elsser-Gravesen, A. (2014). Biopreservatives. Adv. Biochem. Eng. Biotechnol. 143, 29–49. doi: 10.1007/10_2013_234
Fhoula, I., Najjari, A., Turki, Y., Jaballah, S., Boudabous, A., and Ouzari, H. (2013). Diversity and antimicrobial properties of lactic acid bacteria isolated from rhizosphere of olive trees and desert truffles of tunisia. Biomed Res. Int. 2013:405708. doi: 10.1155/2013/405708
Gasperini, L., Mano, J. F., and Reis, R. L. (2014). Natural polymers for the microencapsulation of cells. J. R. Soc. Interface 11:20140817. doi: 10.1098/rsif.2014.0817
Gayán, E., García-Gonzalo, D., Álvarez, I., and Condón, S. (2014). Resistance of Staphylococcus aureus to UV-C light and combined UV-heat treatments at mild temperatures. Int. J. Food Microbiol. 172, 30–39. doi: 10.1016/j.ijfoodmicro.2013.12.003
Gayán, E., Serrano, M. J., Pagán, R., Álvarez, I., and Condón, S. (2015). Environmental and biological factors influencing the UV-C resistance of Listeria monocytogenes. Food Microbiol. 46, 246–253. doi: 10.1016/j.fm.2014.08.011
Ge, C., Bohrerova, Z., and Lee, J. (2013). Inactivation of internalized Salmonella Typhimurium in lettuce and green onion using ultraviolet C irradiation and chemical sanitizers. J. Appl. Microbiol. 114, 1415–1424. doi: 10.1111/jam.12154
Ghanbari, M., Jami, M., Kneifel, W., and Domig, K. (2013). Antimicrobial activity and partial characterization of bacteriocins produced by lactobacilli isolated from Sturgeon fish. Food Control 32, 379–385. doi: 10.1016/j.foodcont.2012.12.024
Ghazvini, R., Kouhsari, E., Zibafar, E., Hashemi, S. J., Amini, A., and Niknejad, F. (2016). Antifungal activity and aflatoxin degradation of Bifidobacterium bifidum and Lactobacillus fermentum against toxigenic Aspergillus parasiticus. Open Microbiol. J. 10, 197–201. doi: 10.2174/1874285801610010197
Grosu-Tudor, S., Pelinescu, D., Stancu, M., and Zamfir, M. (2014). Characterization of some bacteriocins produced by lactic acid bacteria isolated from fermented foods. World J. Microbiol. Biotechnol. 30, 2459–2469. doi: 10.1007/s11274-014-1671-7
Hempel, A., O'Sullivan, M. G., Papkovsky, D. B., and Kerry, J. P. (2013). Nondestructive and continuous monitoring of oxygen levels in modified atmosphere packaged ready-to-eat mixed salad products using optical oxygen sensors, and its effects on sensory and microbiological counts during storage. J. Food Sci. 78, S1057–S1062. doi: 10.1111/1750-3841.12164
Hernández Saldaña, O. F., De la Fuente Salcido, N. M., Valencia Posadas, M., and Barboza Corona, J. E. (2016). Aislamiento y caracterización de bacterias productoras de péptidos antimicrobianos obtenidas a partir de leche de cabra. Invest. Desarrol. Ciencia y Tecnología Alimentos. 1, 429–435.
Heydari, R., Bavandi, S., and Javadian, S. (2015). Effect of sodium alginate coating enriched with horsemint (Mentha longifolia) essential oil on the quality of bighead carp fillets during storage at 4°C. Food Sci. Nutr. 3, 188–194. doi: 10.1002/fsn3.202
Hu, M., Zhao, H., Zhang, C., Yu, J., and Lu, Z. (2013). (2013). Purification and characterization of plantaricin 163, a novel bacteriocin produced by Lactobacillus plantarum 163 isolated from traditional Chinese fermented vegetables. J. Agric. Food Chem. 61, 11676–11682. doi: 10.1021/jf403370y
Ilango, S., Pandey, R., and Antony, U. (2016). Functional characterization and microencapsulation of probiotic bacteria from koozh. J. Food Sci. Technol. 53, 977–989. doi: 10.1007/s13197-015-2169-5
Islam, M., Cheol-Heui, Y., Yun-Jaie, C., and Chong-Su, C. (2010). Microencapsulation of live probiotic bacteria. J. Microbiol. Biotechnol. 20, 1367–1377. doi: 10.4014/jmb.1003.03020
Jeddi, M., Yunesian, M., Gorji, M., Noori, N., Pourmand, M., and Khaniki, G. (2014). Microbial evaluation of fresh, minimally-processed vegetables and bagged sprouts from chain supermarkets. J. Health Popul. Nutr. 32, 391–399.
Jones, L., Worobo, R., and Smart, C. (2014). UV light inactivation of human and plant pathogens in unfiltered surface irrigation water. Appl. Environ. Microbiol. 80, 849–854. doi: 10.1128/AEM.02964-13
Jung, Y., Jang, H., and Matthews, K. (2014). Effect of the food production chain from farm practices to vegetable processing on outbreak incidence. Microbial Biotechnol. 7, 517–527. doi: 10.1111/1751-7915.12178
Khalil, M., El-Sheek, M., El-Adawi, H., El-Deeb, N., and Hussein, M. (2015). Efficacy of microencapsulated lactic acid bacteria in Helicobacter pylori eradication therapy. J. Res. Med. Sci. 20, 950–957. doi: 10.4103/1735-1995.172782
Kim, S. Y., Kim, B. M., Kim, J. B., Shanmugavelan, P., Kim, H. W., Kim, S. Y., et al. (2014). Effect of steaming, blanching and high temperature/high pressure processing on the amino acid contents of commonly consumed Korean vegetables and pulses. Prev. Nutr. Food Sci. 19, 220–226. doi: 10.3746/pnf.2014.19.3.220
Kwak, M. K., Liu, R., Kim, M. K., Moon, D., Kim, A. H., Song, S. H., et al. (2014). Cyclic dipeptides from lactic acid bacteria inhibit the proliferation of pathogenic fungi. J. Microbiol. 52, 64–70. doi: 10.1007/s12275-014-3520-7
Lal Basediya, A., Samuel, D., and Beera, V. (2013). Evaporative cooling system for storage of fruits and vegetables-a review. J. Food Sci. Technol. 50, 429–442. doi: 10.1007/s13197-011-0311-6
Leelavatcharamas, V., Arbsuwan, N., Apiraksakorn, J., Laopaiboon, P., and Kishida, M. (2011). Thermotolerant bacteriocin producing lactic acid bacteria isolated from Thai local fermented foods and their bacteriocin productivity. Biocontrol Sci. 16, 33–40. doi: 10.4265/bio.16.33
Li, D., Ni, K., Pang, H., Wang, Y., Cai, Y., and Jin, Q. (2015). Identification and antimicrobial activity detection of Lactic Acid Bacteria isolated from corn stover silage. Asian Australas. J. Anim. Sci. 28, 620–631. doi: 10.5713/ajas.14.0439
Lu, H., Zhu, J., Li, J., and Chen, J. (2015a). Effectiveness of active packaging on control of Escherichia coli O157:H7 and total aerobic bacteria on iceberg lettuce. J. Food Sci. 80, M1325–M1329. doi: 10.1111/1750-3841.12878
Lu, L., Zhao, L., Zhang, C., Kong, X., Hua, Y., and Chen, Y. (2015b). Comparative effects of Ohmic induction cooker and electric stove heating on soymilk trypsin inhibitor inactivation. J. Food Sci. 80, C495–C503. doi: 10.1111/1750-3841.12773
Menconi, A., Kallapura, G., Latorre, J., Morgan, J., Pumford, N., Gargis, B., et al. (2014). Identification and characterization of lactic acid bacteria in a commercial probiotic culture. Biosci. Microbiota Food Health 33, 25–30. doi: 10.12938/bmfh.33.25
Monika, Savitri, Kumar, V., Kumari, A., Angmo, K., and Bhalla, T. C. (2017). Isolation and characterization of lactic acid bacteria from traditional pickles of Himachal Pradesh, India. J. Food Sci. Technol. 54, 1945–1952. doi: 10.1007/s13197-017-2629-1
Muhialdin, B. J., Hassan, Z., Abu Bakar, F., and Saari, N. (2016). Identification of antifungal peptides produced by Lactobacillus plantarum IS10 grown in the MRS broth. Food Control 59:27–30. doi: 10.1016/j.foodcont.2015.05.022
Neri, L., Hernando, I., Pérez-Munuera, I., Sacchetti, G., and Pittia, P. (2014). Mechanical properties and microstructure of frozen carrots during storage as affected by blanching in water and sugar solutions. Food Chem. 144, 65–73. doi: 10.1016/j.foodchem.2013.07.123
Nguyen, N., Dong, N., Nguyen, H., and Lee, P. (2015). Lactic acid bacteria: promising supplements for enhancing the biological activities of Kombucha. Springerplus 4:91. doi: 10.1186/s40064-015-0872-3
Olvera-García, Y., Serrano-Maldonado, C. E., and Quirasco, M. (2015). Detección de proteínas con actividad antibacteriana producidas por bacterias ácido lácticas. Biotecnología 19, 25–43.
Otero-Pazos, P., Gonzalez-Vallejo, V., Rodríguez-Bernaldo de Quiro, A., Moreno-Bondi, M., Sendo, R., Angulo, I., et al. (2014). Active food packaging based on molecularly imprinted polymers: study of the release kinetics of ferulic acid. J. Agric. Food Chem. 62, 11215–11221. doi: 10.1021/jf5035042
Özer, B., Avni, H., Atamer, M., and Hayaloglu, A. (2009). Improving the viability of Bifidobacterium bifidum BB-12 and Lactobacillus acidophilus LA-5 in white brined cheese by microencapsulation. Int. Dairy J. 19, 22–29. doi: 10.1016/j.idairyj.2008.07.001
Parnell, J., Berka, R., Young, H., Sturino, J., Kang, Y., Barnhart, D., et al. (2016). From the lab to the farm: an industrial perspective of plant beneficial microorganisms. Front. Plant Sci. 7:1110. doi: 10.3389/fpls.2016.01110
Parra, R. (2010a). Microencapsulación de alimentos. Rev. Fac. Nal. Agr. Medellín 63, 5669–5684. doi: 10.15446/rfnam
Parra, R. (2010b). Bacterias Ácido Lácticas: Papel Funcional En Los Alimentos. Facultad de Ciencias Agropecuarias, Universidad Pedagógica y Tecnológica de Colombia.
Pessione, E. (2012). Lactic acid bacteria contribution to gut microbiota complexity: lights and shadows. Front. Cell. Infect. Microbiol. 2:86. doi: 10.3389/fcimb.2012.00086
Raeisi, M., Tabaraei, T., Hashemi, M., and Behnampour, N. (2016). Effect of sodium alginate coating incorporated with nisin, Cinnamomum zeylanicum, and rosemary essential oils on microbial quality of chicken meat and fate of Listeria monocytogenes during refrigeration. Int. J. Food Microbiol. 238, 139–145. doi: 10.1016/j.ijfoodmicro.2016.08.042
Raeisi, M., Tajik, H., Aliakbarlu, J., and Valipour, S. (2014). Effect of carboximetil cellulose edible coating containing Zataria multiflora essential oil and grape seed extract on chemical attributes of rainbow trout meat. Vet. Res. Forum 5, 89–93.
Reina, L., Breidt, F., Fleming, H., and Kathariou, S. (2005). Isolation and selection of lactic acid bacteria as biocontrol agents for nonacidified, refrigerated pickles. J. Food Sci. 70, 7–11. doi: 10.1111/j.1365-2621.2005.tb09050.x
Renna, M., Gonnella, M., De candia, S., and Serio, F. (2017). Efficacy of combined sous vide-microwave cooking for food pathogen inactivation in ready-to-eat chicory stems. J. Food Sci. 82, 1664–1671. doi: 10.1111/1750-3841.13719
Reverte-Ors, J., Pedre-o-Molina, J., Fernández, P., Lozano-Guerrero, A., Periago, P., and Díaz-Morcillo, A. (2017). A novel technique for sterilization using a power self-regulated single-mode microwave cavity. Sensors (Basel) 17, 1309. doi: 10.3390/s17061309
Ruíz-Martínez, M. A., Martín-Villena, M. J., Morales-Hernández, M. E., and Gallardo-Lara, V. (2009). Técnicas de microencapsulación: una propuesta para microencapsular. Ars. Pharm. 50, 43–50.
Russo, P., de Chiara, M., Vernile, A., Amodio, M., Arena, M., Capozzi, V., et al. (2014). Fresh-cut Pinneapple as a new carrier of probiotic lactic acid bacteria. Biomed Res. Int. 2014:309183. doi: 10.1155/2014/309183
Sabir, A., Sabir, F., and Kara, Z. (2010). Effects of modified atmosphere packing and honey dip treatments on quality maintenance of minimally processed grape cv. Razaki (V. vinifera L.) during cold storage. J. Food Sci. Technol. 48, 312–318. doi: 10.1007/s13197-011-0237-z
Sánchez-Ortega, I., García-Almendárez, B., Santos-López, E., Amaro-Reyes, A., Barboza-Corona, J., and Regalado, C. (2014). Antimmicrobial edible films and coatings for meat and meat products preservation. Sci. World J. 2014:248935. doi: 10.1155/2014/248935
Settanni, L., and Corsetti, A. (2008). Application of bacteriocins in vegetable food biopreservation. Int. J. Food Microbiol. 121, 123–138. doi: 10.1016/j.ijfoodmicro.2007.09.001
Shafiur-Rahman, M. (2007). Handbook of Food Preservation. Boca Ratón, FL: RC Press; Taylor and Francis Group LLC.
Sharma, S. S., Singh, R., and Rana, S. (2011). Bioactive peptides: a review. Int. J. Bioautomation 15, 223–250.
Siroli, L., Patrignani, F., Serrazanetti, D., Tabanelli, G., Mantanari, C., Gardini, F., et al. (2015). Lactic acid bacteria and natural antimicrobials to improve the safety and shelf-life of minimally processed sliced apples and lamb's lettuce. Food Microbiol. 47, 74–84. doi: 10.1016/j.fm.2014.11.008
Snyder, A., and Worobo, R. (2013). Chemical and genetics characterization of bacteriocins: antimicrobial peptides for food safety. J. Sci. Food Agric. 94, 28–44. doi: 10.1002/jsfa.6293
Solanki, H., Pawar, D., Shah, D., Prajapati, V., Jani, G., Mulla, A., et al. (2013). Development of microencapsulation delivery system for long term preservation of probiotics as biotherapeutics agent. Biomed Res. Int. 2013:620719. doi: 10.1155/2013/620719
Swain, M., Anandharaj, M., Ray, R., and Rani, R. (2014). Fermented fruits and vegetables of Asia: a potential source of probiotics. Biotechnol. Res. Int. 2014:250424. doi: 10.1155/2014/250424
Trabelsi, I., Ayadi, D., Bejar, W., Bejar, S., Chouayekh, H., and Ben Salah, R. (2013). Effects of Lactobacillus plantarum immobilization in alginate coated with chitosan and gelatin on antibacterial activity. Int. J. Biol. Macromol. 64, 84–89. doi: 10.1016/j.ijbiomac.2013.11.031
Trias, R., Ba-eras, L. L., Montesinos, E., and Badosa, E. (2008b). Lactic acid bacteria from fresh fruit and vegetables as biocontrol agents of phytopathogenic bacteria and fungi. Int. Microbiol. 11, 231–236. doi: 10.2436/20.1501.01.66
Trias, R., Bañeras, L., and Montesinos, E. (2008a). Bioprotection of Golden delicious apples and iceberg lettuce against foodborne bacterial pathogens by lactic acid bacteria. Int. J. Food Microbiol. 123, 50–60. doi: 10.1016/j.ijfoodmicro.2007.11.065
Wada, T., Noda, M., Kashiwabara, F., Jeon, H., Shirakawa, A., Yabu, H., et al. (2009). Characterization of four plasmids harbored in a Lactobacillus brevis strain encoding a novel bacteriocin, brevicin 925A and construction of a shuttle vector for lactic acid bacteria and Escherichia coli. Microbiology 155, 1726–1737. doi: 10.1099/mic.0.022871-0
Wong, C. B., Khoo, B. Y., Sasidharan, S., Piyawattanametha, W., Kim, S. H., Khemthongcharoen, N., et al. (2015). Inhibition of Staphylococcus aureus by crude and fractionated extract from lactic acid bacteria. Benef. Microbes 6, 129–140. doi: 10.3920/BM2014.0021
Keywords: food safety, Lactic acid bacteria, biopreservation, antimicrobial peptides, bacteriocin
Citation: Linares-Morales JR, Gutiérrez-Méndez N, Rivera-Chavira BE, Pérez-Vega SB and Nevárez-Moorillón GV (2018) Biocontrol Processes in Fruits and Fresh Produce, the Use of Lactic Acid Bacteria as a Sustainable Option. Front. Sustain. Food Syst. 2:50. doi: 10.3389/fsufs.2018.00050
Received: 22 December 2017; Accepted: 25 July 2018;
Published: 16 August 2018.
Edited by:
Lourdes Maria Correa Cabral, Empresa Brasileira de Pesquisa Agropecuária (EMBRAPA), BrazilReviewed by:
Pablo Fuciños, Laboratório Ibérico Internacional de Nanotecnologia (INL), PortugalSabu Abdulhameed, Kannur University, India
Guillermo Alfredo Picó, Institute of Biotechnological and Chemical Processes Rosario (IPROBYQ), Argentina
Copyright © 2018 Linares-Morales, Gutiérrez-Méndez, Rivera-Chavira, Pérez-Vega and Nevárez-Moorillón. This is an open-access article distributed under the terms of the Creative Commons Attribution License (CC BY). The use, distribution or reproduction in other forums is permitted, provided the original author(s) and the copyright owner(s) are credited and that the original publication in this journal is cited, in accordance with accepted academic practice. No use, distribution or reproduction is permitted which does not comply with these terms.
*Correspondence: Guadalupe V. Nevárez-Moorillón, vnevare@uach.mx