- 1School of Fundamental Sciences, Massey University, Palmerston North, New Zealand
- 2MacDiarmid Institute for Advanced Material and Nanotechnology, Victoria University of Wellington, Wellington, New Zealand
Organogels are often made from solutions of proteins, polymers or fatty acids in oil. The macromolecular species crystallize, or assemble, into mesh structures that inhibit oil flow. This review focuses on using fumed silica nanoparticles as an alternative structuring agent. Fumed silica particles have a unique, branched morphology that means the particles aggregate into three-dimensional, interconnected networks. Two key aspects for formulating edible oleogels are addressed. Advances in our understanding of fumed silica particle aggregation in oil that point toward strategies for tuning the rheological properties of oleogels are examined. Secondly, the factors likely to affect the lipolysis of oils structured used fumed silica particles, and hence the bioavailability of ingredients loaded into the gels, are discussed. The next challenge for these promising materials is to target suitable applications for their use as fat replacement in foods.
Introduction
Gels made from edible oils are potential replacements for the fat in a range of food products (Martins et al., 2018; Puşcaş et al., 2020). The challenge in reducing the fat content in a food is finding an alternative that performs similar functions (Marangoni et al., 2020). Trans- and saturated fats influence food texture. Fats are structured by triglyceride molecules that crystallize into a space-filling network of colloidal crystals (Tang and Marangoni, 2006; Lupi et al., 2016). The presence of the network imparts an elastic structure, and hence a desirable mouthfeel (Sato and Ueno, 2014; Macias-Rodriguez and Marangoni, 2018). The structure of a food also affects its digestibility and the bioavailability of nutrients (Michalski et al., 2013). This can be due to lipid being trapped within the network, or to the network components interfering with digestion (McClements, 2018). Thus, the choice of gelling agent remains critical (Marangoni and Edmund, 2012; Rogers, 2018). This mini review will examine the strategy of using fumed silica to structure edible oils.
Fumed silica nanoparticles aggregate in vegetable oils and assemble into a three-dimensional network. The presence of the network immobilizes the oil into a solid-like material that holds its shape and does not leak. The focus of the mini review is on how the surface chemistry of fumed silica particles can be manipulated to optimize their effectiveness as structuring agents. The review is divided into three parts. Firstly, the colloid chemistry of fumed silica particles is summarized. Secondly, the relationship between the structure of the networks and the organogel rheology is described. Finally, the impact of the particles on the rate of lipid digestion and release of encapsulated ingredients is considered.
Colloid Chemistry of Fumed Silica
Fumed silica nanoparticles have a unique shape and surface chemistry that is central to their ability to form structural frameworks in solvents. The shape of the particles is due to them being synthesized by flame hydrolysis of SiCl4 at temperatures higher than 1,000°C (Hurd and Flower, 1988; Pratsinis, 1998). Chlorosilane reacts with hydrogen and oxygen to produce molten spheres of silica (called primary particles) with sizes between 5 and 30 nm. The primary particles collide together and fuse into chains of particles. The fused clusters are between 100 and 1,000 nm in length and have branched shapes, as shown in Figure 1a. Light scattering studies of the growth of fused clusters revealed that they have a fractal dimension of 1.5 ± 0.2 (Hurd and Flower, 1988; Pratsinis, 1998). Fumed silica powders are made of clusters entangled together into porous networks that extend up to 250 micrometers in size. The powders have specific surface areas that range between 50 and 400 m2 g−1.
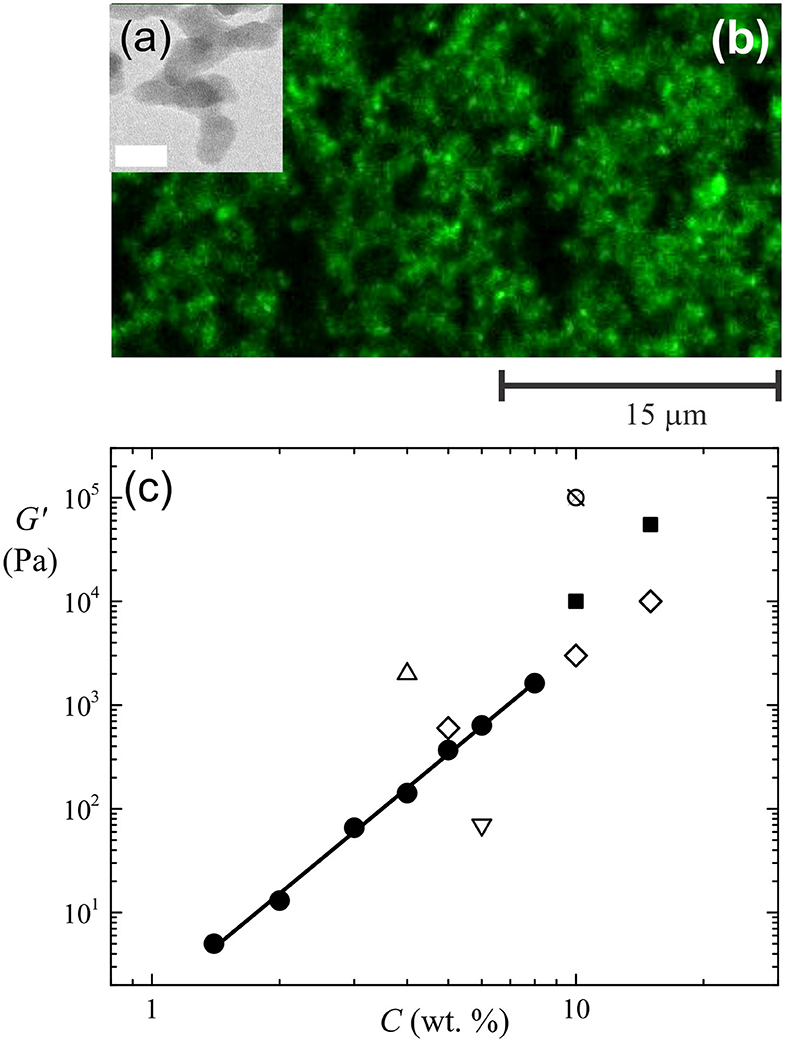
Figure 1. (a) Electron microscope image of primary particles fused together into a chain-like cluster of fumed silica. The white bar corresponds to 20 nm. (b) Confocal fluorescence image of the entangled aggregates in a gel of 5 wt. % fumed silica particles in olive oil. (c) Particle concentration (C) dependence of the elasticity (G') of gels of fumed silica in olive oil (•) (Whitby et al., 2018) and sunflower oil (■) (Patel et al., 2015b). The line is a power-law fit of the data for the olive oil gels with a scaling exponent, n = 3.5. For comparison, the elasticity of gels of glyceryl monostearate in a medium chain triglyceride oil (♢) (Cerqueira et al., 2017), ethyl cellulose in soybean oil (⊗) (Zetzl et al., 2014), berry wax in sunflower oil (∇) (Patel et al., 2015a) and carnauba wax in sunflower oil (Δ) (Patel et al., 2015a) is also shown.
The large surface area of fumed silica powders means that their surface chemistry is critical to how the powders function when dispersed in solvents (Iler and Iler, 1979; Legrand, 1998). The primary particles are composed of SiO4 tetrahedra. The atoms exposed on the particle surfaces are oxygen atoms that are part of siloxane groups (Si-O-Si), or silicon atoms that are part of silanol (Si-OH) groups (Vansant et al., 1995). The silanol groups are formed during particle synthesis, or by rehydroxylation of siloxane groups due to dissociative water adsorption. The surface reactivity of a powder depends on the number and distribution of silanol groups. Approximately every second silicon atom on a fumed silica surface bears a silanol group (Mathias and Wannemacher, 1988; Barthel, 1995). The groups are randomly distributed across the surface (Zaborski et al., 1989). Pristine fumed silica is hydrophilic and has a high surface energy due to their presence. Deactivation of the surface silanol groups by reaction with alkyl trichlorosilanes, or alkyl trialkoxysilanes, reduces the surface energy of the powder and the particle surfaces become hydrophobic (Gun'ko et al., 2000; Wang et al., 2000; Jiang et al., 2018).
The surface chemistry of fumed silica powders is modified by adsorption of lipid species when the powders are wetted by edible oils. The silanol groups on the particle surfaces are sites for molecular adsorption of species capable of forming hydrogen bonds, or of undergoing donor–acceptor interactions (Zhuravlev, 2000). The triglycerides in vegetable oils adsorb onto silica surfaces due to hydrogen bonding between the silanol groups and the ester carbonyl groups (Adhikari et al., 1994; Proctor et al., 1996). This reduces the proportion of silanol groups available to form hydrogen bonds.
Fumed silica tends to aggregate when dispersed in organic solvents (like edible oils). The interactions between colloidal particles are traditionally explained using DLVO theory. This approach approximates the total potential energy of interaction between two colloidal particles as the sum of their van der Waals interactions and the energy due to the overlap of their electrical double layers (Israelachvili, 2015). Double layer forces are weak in non-aqueous solvents (Christenson and Horn, 1985). Raghavan et al. (2000b) proposed that fumed silica particles aggregate due to van der Waals and hydrogen bonding interactions between silanol groups on adjacent hydrophilic fumed silica particles. The hydrogen bonding interactions enhance particle aggregation in solvents that interact only weakly with the particle surfaces (Raghavan et al., 2000b). In the case of silanised powders, they argued that a mismatch between the chemical nature of the solvent and the alkyl chains coating the silica surfaces leads to attractive interactions due to the negative free energy of mixing between the alkyl chains (Raghavan et al., 2000a). Thus, the interactions governing the aggregation of fumed silica particles are thought to be dispersion, solvation and hydrogen bonding forces.
There is evidence from measurements of the forces between silica surfaces in non-aqueous solvents to support these hypotheses. McNamee et al. (2004) found that electrostatic interactions between hydrophilic silica surfaces in dodecane were weak and long-ranged. No electrostatic repulsive forces were measurable between hydrophobic silica surfaces in dodecane (McNamee et al., 2004). Gee and Israelachvili (1990) measured attractive interactions between silica surfaces coated with hexadecyl hydrocarbon chains in tetradecane that could not be described by van der Waals forces alone. They proposed that the additional attractive interaction was due to entropic interactions between the hydrocarbon chains and the solvent molecules. Roke et al. (2005) probed the molecular structure of the interface between hydrophobic silica particles and hexadecane and observed that an ordered (frozen) interfacial layer formed as the temperature was lowered. They argued that this layer generates a short-range attraction between the particles. Although alkanes were used in these studies, it is likely that the findings can be generalized to dispersions of fumed silica in edible oils.
The attractive interactions between fumed silica particles cause them to assemble into three-dimensional networks in organic solvents. Electron microscopy imaging of frozen samples of fumed silica dispersions in sunflower oil by Patel et al. (2015b) confirmed the presence of fractal-like clusters of particles on the nanometre-scale. The kinetics of the aggregation between the clusters were followed indirectly using scattering techniques (Smith and Zukoski, 2006a,b; Nordström et al., 2012). Confocal microscopy imaging of fumed silica particle aggregates in olive oil showed that the clusters assemble into irregular, linear aggregate structures up to 20 μm in length (Whitby et al., 2018). The linear aggregates overlap and entangle together in a ramified manner on the microscopic scale, as shown in Figure 1b. Analysis of the spatial correlations between the particle aggregates revealed that the networks have a characteristic pore size. The sizes of the pore spaces depend on the fraction of silanol groups available to form hydrogen bonds between neighboring aggregates (Whitby et al., 2018). The hierarchy of fumed silica networks in edible oils resembles the different levels of structuring found in fat crystal networks (Tang and Marangoni, 2006).
Rheology of Fumed Silica Oleogels
Network formation by fumed silica particles in organic solvents causes the liquid to thicken. Increasing the particle concentration in the oil transforms the viscous liquid into a gel, a self-supporting material (Kosinski and Caruthers, 1986; Khan and Zoeller, 1993; Chen et al., 2005; Sugino and Kawaguchi, 2017). The viscoelastic character of the gels resembles the rheology of fat crystal networks (Macias-Rodriguez and Marangoni, 2018). Using oleogels as replacements for solid fat in food requires being able to characterize their mechanical properties, and to alter these properties to match the desired texture of the food product.
The rheology of oil dispersions of fumed silica is quantified by measuring their response to oscillatory stress. Gelled dispersions of hydrophilic fumed silica in olive oil resist flowing and behave like viscoelastic solids, when small perturbations are applied (Whitby et al., 2018). This means that the connections between the overlapping silica aggregates are strong enough for the network to withstand the weight of the solvent trapped in the pores, and to store elastic strain. The solid-like behavior is characterized by the elastic storage modulus, G'. Larger perturbations cause a solid-liquid transition, due to energy being dissipated as the network of fractal clusters is broken apart (Whitby et al., 2018). The elastic structure of fumed silica organogels can be recovered once the applied stress is released (Patel et al., 2015b).
The rheological properties of fumed silica organogels are linked to the unique (fractal) shape and size of the aggregates formed by the particles. The elastic storage moduli of olive oil gels of hydrophilic fumed silica scale with the particle concentration (C) as G′ = aCn, where the scaling exponent n ~ 3.5, as shown in Figure 1c (Whitby et al., 2018). This is consistent with predictions made by physical models of the gels as space-filling networks of fractal clusters formed by diffusion limited aggregation between the clusters (Buscall et al., 1988). Similar behavior was observed for gels of fumed silica in mineral (Khan and Zoeller, 1993) and paraffin oils (Yziquel et al., 1999). The magnitude of the elasticity of the network structures in triglyceride solvents is, however, lower at a given particle concentration (Whitby et al., 2018). This is likely due to the impact of lipid adsorption on the hydrogen bonding capacity of the particle clusters and hence the strength of the links between the clusters in the network. Thus, the rheological properties of these systems are also linked to the interactions between the oil and the particles. Figure 1c shows that the elasticity of the gels is comparable in magnitude to that of oleogels formed using natural waxes, biopolymers and fatty acid crystals.
There is significant evidence that fumed silica gel rheology can be tuned by altering the interactions between the particles and hence the network morphology. Dispersions of methylated particles in olive oil tend to yield and flow even under small applied stresses (Whitby et al., 2018). Replacing some of the silanol groups on the particle surfaces with methyl groups reduces the hydrogen bonding capability of the particle aggregates. This was shown to lead to the formation of more tenuous networks with a larger average pore size (Whitby et al., 2018). Raghavan et al. (2000a) tuned the surface chemistry of fumed silica powders by reacting them with silanes of different chain length. They observed a correlation between the measured elastic moduli of dispersions of silanised fumed silica in polyethylene glycol and the calculated mismatch in solubility between the functional groups on the particle surfaces and the solvent. Patel et al. (2015b) found that the rheology of fumed silica dispersions in sunflower oil could be altered by thermal treatments. Wu and co-workers argued that heating fumed silica organogels affects the strength of hydrogen bonding interactions between the particles (Wu et al., 2012a,b).
Adding small amounts of a second solvent may also alter the interactions in fumed silica organogels. Providing the second solvent is not miscible in the edible oil, it may form liquid bridges between particles, and hence generate attractive capillary interactions between the particles (Koos and Willenbacher, 2011, 2012). This has not been investigated in edible oils gelled with fumed silica. But there is evidence in the literature that it is likely to enhance network formation. For example, the viscosity of suspensions of other food grade particles (cocoa and starch particles) in sunflower oil was manipulated by adding small volumes of water (Hoffmann et al., 2014).
Lipolysis of Mixtures of Fumed Silica and Edible Oils
Given the potential applications for organogels in foods, it is important to consider how the digestion of edible oils will be impacted by gelation with fumed silica. Digestion involves the hydrolysis of triglycerides by lipase enzymes (Friedman and Nylund, 1980; Wilde and Chu, 2011; Singh et al., 2015; Maldonado-Valderrama, 2019). The enzymes break the triglycerides down into fatty acids and glycerols that are adsorbed into the bloodstream. Lipases are surface active proteins that catalyze reactions at the interface between aqueous and oil phases (Carey et al., 1983; Schmid and Verger, 1998; Mu and Høy, 2004). This means that the edible oil must be emulsified for the enzyme to be effective. The reaction kinetics are affected by the size of the oil droplets formed during emulsification, and hence the interfacial area, as well as the composition of the interface (Armand et al., 1999; Reis et al., 2009; Malaki Nik et al., 2011; Guo et al., 2017; Raikos and Ranawana, 2017). A network of fumed silica particles within the edible oil will presumably hinder emulsification of the oil and slow the release of any active ingredients (like drugs) dissolved in the oil.
Lipid digestion from fumed silica organgels has yet to be investigated. But we can glean insights from studies of digestion of edible oils gelled with fatty acids (Iwanaga et al., 2010, 2012; Lupi et al., 2013, 2018) and ethylcellulose (O′Sullivan et al., 2017). For example, Iwanaga et al. (2010) investigated the rate of release of ibuprofen from soybean oil gelled using 12-hydroxystearic acid. They observed that the ibuprofen released more slowly into simulated intestinal fluids from gels than from solutions of the drug in soybean oil. Lupi et al. (2013) studied the release of ferulic acid from olive oil gelled with policosanol (a fatty acid) in gastro-intestinal simulating fluids. They observed two stages in the release process. Lupi et al. (2013) found they could manipulate the release rate in the first stage by altering the concentration of the policosanol, and hence the gel viscosity. They proposed that release was controlled by diffusion of the drug molecules through the gel during this stage. The second stage was characterized by a faster release rate (Lupi et al., 2013, 2018; Pereira Camelo et al., 2016). The rate exceeded predictions by models for the release of drugs from polymer matrices due to diffusion alone (Siepmann and Siepmann, 2008; Fredenberg et al., 2011). Lupi et al. (2013) proposed that disruption of the network of fatty acid crystals enhanced the rate of drug diffusion by increasing the lipid-water interfacial area. O′Sullivan et al. (2017) investigated the release of β-carotene from canola oil gelled with ethylcellulose. They found that the lipids in gels with weak mechanical properties were digested in simulated duodenal fluid at a similar rate to lipids in oil solutions of β-carotene. In contrast, the rate of lipid hydrolysis was slower from gels that were stiff enough to be cut up into pieces. O′Sullivan et al. (2017) argued that the canola oil was released more slowly from the stronger cellulose networks because they were harder to break apart. They observed variations in the rate of lipolysis in gels of similar strength and argued that the thickness of the walls in the cellulose networks must also affect their disruption.
It is likely that similar behavior will be observed for edible oils gelled using fumed silica. The release of the encapsulated cargo during digestion will occur by a combination of two mechanisms, as illustrated in Figure 2A. One is diffusion through the thickened solvent. The rate of diffusion will depend on the concentration of fumed silica particles. The other mechanism involves disruption (sometimes called degradation, or erosion) of the silica particle network. This will increase the surface area of the lipid exposed to digestive fluids. The rate of lipid digestion will therefore depend on the influence of the fumed silica particles on the interactions between the lipase enzymes and the lipid-water interface. Although this has yet to be investigated for fumed silica gels, Prestidge and co-workers have studied the digestion of mixtures of edible oils and inorganic particles, including fumed silica (Joyce et al., 2016c, 2018). Their findings provide insights into how the porous shape of fused silica could be used to manipulate the size of the lipid-water interfacial area available for lipase adsorption.
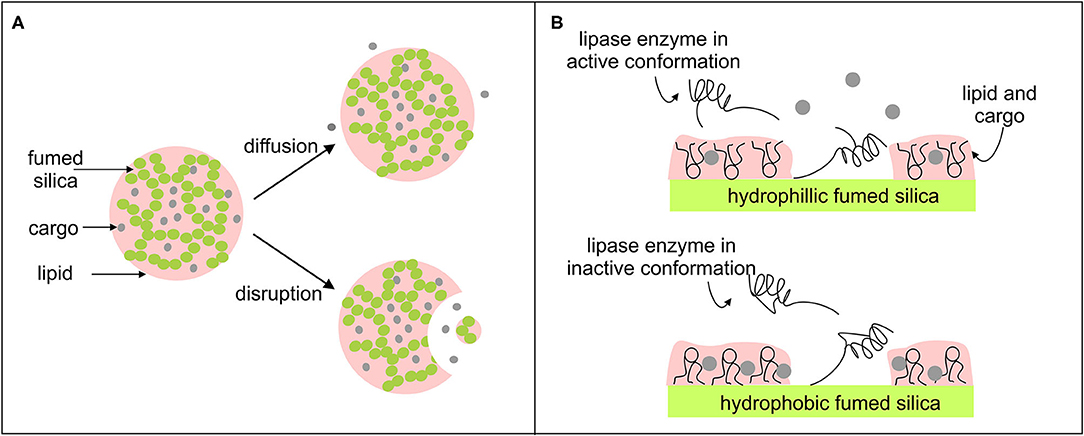
Figure 2. (A) Schematic of the release of encapsulated ingredients (cargo) from fumed silica organogels during digestion by diffusion through the thickened oil and disruption of the particle network. (B) Schematic of how the interplay between the surface chemistry of the fumed silica and the conformation of the enzyme and lipid molecules on the silica surface may affect cargo release.
Tan et al. (2010) found that dispersing hydrophilic fumed silica particles in droplets of a medium chain triglyceride oil (Miglyol 812) reduced the rate of lipolysis. They argued that the presence of the particles limited access of the lipase enzymes to the lipid interface. On the other hand, Tan et al. (2010) observed that lipid digestion from hybrid particles made from fumed silica and oil droplets was faster than digestion from the oil drops alone. Electron microscopy imaging of the hybrid materials showed that they were micrometers in size and made of a “sponge-like” network of the silica particles. The pores in the network were up to 200 nm in size, which was comparable to the average diameter of the oil droplets (~172 nm). The network remained intact for at least 1 h during lipolysis (Simovic et al., 2009). Confocal fluorescence microscopy imaging revealed that the oil was spread throughout the porous network (Tan et al., 2012). Joyce et al. (2014) demonstrated that the rate and extent of lipid digestion depended on the relative proportions of silica and oil. They found that that lipid digestion was enhanced from hybrids containing insufficient oil to coat the available pore space (Joyce et al., 2014). The rate of digestion decreased as the amount of oil increased. Lipid digestion from hybrid particles containing enough oil to exceed monolayer coverage of their pores was slower than digestion from oil droplets alone.
The surface chemistry of fumed silica also has a critical effect on the interactions between lipase and the lipid-water interface. The rate of lipid hydrolysis is faster from hybrids made of hydrophilic fumed silica, rather than hydrophobic fumed silica (Joyce et al., 2016b). Reis et al. (2006) found that lipases adsorbed on hydrophilic silica surfaces catalyzed hydrolysis reactions. Lipases bound to hydrophobic silica favored esterification of triglycerides instead. They argued that lipase enzymes adsorbed on hydrophilic silica adopt conformations that expose the active hydrophobic sites which catalyze lipid hydrolysis (Reis et al., 2006). Joyce et al. (2016a) used mass spectrometry to find evidence for differences in the orientation of lipase enzymes adsorbed on silica surfaces of different wettability. Evidence was also found for differences between the conformation of adsorbed lipids molecules on hydrophobic and hydrophilic silica (Joyce et al., 2015). Thus, the wettability of the silica surface may affect the orientation of lipid molecules and lipase enzymes adsorbed on the surface and hence the bioavailability of encapsulated ingredients, as illustrated in Figure 2B. The charge on the silica particles can also affect lipase action. Digestion products displace lipase enzymes from the lipid-water interface (Reis et al., 2008). Since hydrophilic surfaces are negatively charged, it is thought that electrostatic repulsions between the fatty acids and the silica network in the hybrids encourage removal of the digestion products, thus freeing up sites at the lipid-water interface for lipase adsorption (Joyce et al., 2014).
Future Outlook
Based on the evidence in the literature, using fumed silica to structure edible oils has advantages. The inorganic particles assemble into three dimensional, anisotropic networks which have similar structural and mechanical properties to networks of fat colloids. They could enhance the nutritional profiles of edible organogels by modulating the rate of lipid digestion and release of active ingredients encapsulated within the networks. The next step is to transform these structures into materials that mimic the texture and stability of fat and can be readily incorporated into food formulations.
Thus far, fumed silica oleogels have been incorporated into a limited number of composite materials for use in food products (Skelhon et al., 2012; Patel et al., 2015b; Chauhan et al., 2017a,b). For example, Skelhon et al. (2012) lowered the fat content of chocolate by replacing some of the cocoa butter with drops of fruit juice stabilized by a network of fumed silica in the remaining cocoa butter. They found that the chocolate emulsion formulations retained the crystalline fat structure needed for the chocolate to melt with the desired texture when eaten. Chauhan et al. (2017a,b) investigated the properties of composite networks of fumed silica and tripalmitin fat crystals used to gel soybean oil. They found that the composite networks retained the melting and rheological properties of full-fat networks. These results confirm the potential for creating healthier foods using hybrid mixtures of fumed silica organogels and solid fats.
Author Contributions
CW review the literature, drafted and edited the manuscript text, and prepared the figures for the manuscript.
Funding
This work was supported by the Massey University Research Fund and the MacDiarmid Institute for Advanced Materials and Nanotechnology.
Conflict of Interest
The author declares that the research was conducted in the absence of any commercial or financial relationships that could be construed as a potential conflict of interest.
References
Adhikari, C., Proctor, A., and Blyholder, G. D. (1994). Diffuse-reflectance fourier-transform infrared spectroscopy of vegetable oil triglyceride adsorption on silicic acid. J. Am. Oil Chem. Soc. 71, 589–594. doi: 10.1007/BF02540584
Armand, M., Pasquier, B., André, M., Borel, P., Senft, M., Peyrot, J., et al. (1999). Digestion and absorption of 2 fat emulsions with different droplet sizes in the human digestive tract. Am. J. Clin. Nutr. 70, 1096–1106. doi: 10.1093/ajcn/70.6.1096
Barthel, H. (1995). Surface interactions of dimethylsiloxy group-modified fumed silica. Colloids Surf. A 101, 217–226. doi: 10.1016/0927-7757(95)03179-H
Buscall, R., Mills, P. D. A., Goodwin, J. W., and Lawson, D. W. (1988). Scaling behaviour of the rheology of aggregate networks formed from colloidal particles. J. Chem. Soc. Fara. Trans. 84, 4249–4260. doi: 10.1039/f19888404249
Carey, M. C., Small, D. M., and Bliss, C. M. (1983). Lipid digestion and absorption. Ann. Rev. Physiol. 45, 651–677. doi: 10.1146/annurev.ph.45.030183.003251
Cerqueira, M. A., Fasolin, L. H., Picone, C. S. F., Pastrana, L. M., Cunha, R. L., and Vicente, A. A. (2017). Structural and mechanical properties of organogels: role of oil and gelator molecular structure. Food Res. Int. 96, 161–170. doi: 10.1016/j.foodres.2017.03.021
Chauhan, R. R., Dullens, R. P. A., Velikov, K. P., and Aarts, D. G. A. L. (2017a). The effect of colloidal aggregates on fat crystal networks. Food Funct. 8, 352–359. doi: 10.1039/C6FO01622G
Chauhan, R. R., Dullens, R. P. A., Velikov, K. P., and Aarts, D. G. A. L. (2017b). Exploring concentration, surface area and surface chemistry effects of colloidal aggregates on fat crystal networks. RSC Adv. 7, 28780–28787. doi: 10.1039/C7RA01803G
Chen, S., Øye, G., and Sjöblom, J. (2005). Rheological properties of silica particle suspensions in mineral oil. J. Dispersion Sci. Tech. 26, 791–798. doi: 10.1081/DIS-200063119
Fredenberg, S., Wahlgren, M., Reslow, M., and Axelsson, A. (2011). The mechanisms of drug release in poly(lactic-co-glycolic acid)-based drug delivery systems-a review. Int. J. Pharma. 415, 34–52. doi: 10.1016/j.ijpharm.2011.05.049
Friedman, H. I., and Nylund, B. (1980). Intestinal fat digestion, absorption, and transport. a review. Am. J. Clin. Nutr. 33, 1108–1139. doi: 10.1093/ajcn/33.5.1108
Gee, M. L., and Israelachvili, J. N. (1990). Interactions of surfactant monolayers across hydrocarbon liquids. J. Chem. Soc. Fara. Trans. 86, 4049–4058. doi: 10.1039/ft9908604049
Gun'ko, V. M., Vedamuthu, M. S., Henderson, G. L., and Blitz, J. P. (2000). Mechanism and kinetics of hexamethyldisilazane reaction with a fumed silica surface. J. Colloid Interface Sci. 228, 157–170. doi: 10.1006/jcis.2000.6934
Guo, Q., Ye, A., Bellissimo, N., Singh, H., and Rousseau, D. (2017). Modulating fat digestion through food structure design. Prog. Lipid Res. 68, 109–118. doi: 10.1016/j.plipres.2017.10.001
Hoffmann, S., Koos, E., and Willenbacher, N. (2014). Using capillary bridges to tune stability and flow behavior of food suspensions. Food Hydrocoll. 40, 44–52. doi: 10.1016/j.foodhyd.2014.01.027
Hurd, A. J., and Flower, W. L. (1988). In situ growth and structure of fractal silica aggregates in a flame. J. Colloid Interface Sci. 122, 178–192. doi: 10.1016/0021-9797(88)90301-3
Iler, R. K., and Iler, R. (1979). The chemistry of silica: solubility, polymerization, colloid and surface properties, and biochemistry. Angew. Chem. 92:328.
Iwanaga, K., Kawai, M., Miyazaki, M., and Kakemi, M. (2012). Application of organogels as oral controlled release formulations of hydrophilic drugs. Int. J. Pharma. 436, 869–872. doi: 10.1016/j.ijpharm.2012.06.041
Iwanaga, K., Sumizawa, T., Miyazaki, M., and Kakemi, M. (2010). Characterization of organogel as a novel oral controlled release formulation for lipophilic compounds. Int. J. Pharma. 388, 123–128. doi: 10.1016/j.ijpharm.2009.12.045
Jiang, J., Cao, J., Wang, W., and Xue, J. (2018). How silanization influences aggregation and moisture sorption behaviours of silanized silica: analysis of porosity and multilayer moisture adsorption. Roy. Soc. Open Sci. 5:180206. doi: 10.1098/rsos.180206
Joyce, P., Gustafsson, H., and Prestidge, C. A. (2018). Engineering intelligent particle-lipid composites that control lipase-mediated digestion. Adv. Colloid Interface Sci. 260, 1–23. doi: 10.1016/j.cis.2018.08.001
Joyce, P., Kempson, I., and Prestidge, C. A. (2015). QCM-D and ToF-SIMS investigation to deconvolute the relationship between lipid adsorption and orientation on lipase activity. Langmuir 31, 10198–10207. doi: 10.1021/acs.langmuir.5b02476
Joyce, P., Kempson, I., and Prestidge, C. A. (2016a). Orientating lipase molecules through surface chemical control for enhanced activity: a QCM-D and ToF-SIMS investigation. Colloids Surf. B 142, 173–181. doi: 10.1016/j.colsurfb.2016.02.059
Joyce, P., Tan, A., Whitby, C. P., and Prestidge, C. A. (2014). The role of porous nanostructure in controlling lipase-mediated digestion of lipid loaded into silica particles. Langmuir 30, 2779–2788. doi: 10.1021/la500094b
Joyce, P., Whitby, C. P., and Prestidge, C. A. (2016b). Interfacial processes that modulate the kinetics of lipase-mediated catalysis using porous silica host particles. RSC Adv. 6, 43802–43813. doi: 10.1039/C6RA08934H
Joyce, P., Whitby, C. P., and Prestidge, C. A. (2016c). Nanostructuring biomaterials with specific activities towards digestive enzymes for controlled gastrointestinal absorption of lipophilic bioactive molecules. Adv. Colloid Interface Sci. 237, 52–75. doi: 10.1016/j.cis.2016.10.003
Khan, S. A., and Zoeller, N. J. (1993). Dynamic rheological behavior of flocculated fumed silica suspensions. J. Rheol. 37, 1225–1235. doi: 10.1122/1.550378
Koos, E., and Willenbacher, N. (2011). Capillary forces in suspension rheology. Science 331, 897–900. doi: 10.1126/science.1199243
Koos, E., and Willenbacher, N. (2012). Particle configurations and gelation in capillary suspensions. Soft Matter 8, 3988–3994. doi: 10.1039/c2sm07347a
Kosinski, L. E., and Caruthers, J. M. (1986). The effect of particle concentration on the rheology of polydimethylsiloxane filled with fumed silica. J. App. Polym. Sci. 32, 3393–3406. doi: 10.1002/app.1986.070320203
Lupi, F., Mancina, V., Baldino, N., Parisi, O., Scrivano, L., and Gabriele, D. (2018). Effect of the monostearate/monopalmitate ratio on the oral release of active agents from monoacylglycerol organogels. Food Funct. 9, 3278–3290. doi: 10.1039/C8FO00594J
Lupi, F. R., Gabriele, D., Baldino, N., Mijovic, P., Parisi, O. I., and Puoci, F. (2013). Olive oil/policosanol organogels for nutraceutical and drug delivery purposes. Food Funct. 4, 1512–1520. doi: 10.1039/c3fo60259a
Lupi, G. V., Baldino, N., de Cindio, B., Fischer, P., and Gabriele, D. (2016). The effects of intermolecular interactions on the physical properties of organogels in edible oils. J. Colloid Interface Sci. 483, 154–164. doi: 10.1016/j.jcis.2016.08.009
Macias-Rodriguez, B. A., and Marangoni, A. A. (2018). Linear and nonlinear rheological behavior of fat crystal networks. Cri. Rev. Food Sci. Nutr. 58, 2398–2415. doi: 10.1080/10408398.2017.1325835
Malaki Nik, A., Wright, A. J., and Corredig, M. (2011). Impact of interfacial composition on emulsion digestion and rate of lipid hydrolysis using different in vitro digestion models. Colloids Surf. B 83, 321–330. doi: 10.1016/j.colsurfb.2010.12.001
Maldonado-Valderrama, J. (2019). Probing in vitro digestion at oil-water interfaces. Curr. Opin. Colloid Interf. Sci. 39, 51–60. doi: 10.1016/j.cocis.2019.01.004
Marangoni, A. G., and Edmund, D. C. (2012). Organogels: an alternative edible oil-structuring method. J. Am. Oil Chem. Soc. 89, 749–780. doi: 10.1007/s11746-012-2049-3
Marangoni, A. G., van Duynhoven, J. P. M., Acevedo, N. C., Nicholson, R. A., and Patel, A. R. (2020). Advances in our understanding of the structure and functionality of edible fats and fat mimetics. Soft Matter 16, 289–306. doi: 10.1039/C9SM01704F
Martins, A. J., Vicente, A. A., Cunha, R. L., and Cerqueira, M. A. (2018). Edible oleogels: an opportunity for fat replacement in foods. Food Funct. 9, 758–773. doi: 10.1039/C7FO01641G
Mathias, J., and Wannemacher, G. (1988). Basic characteristics and applications of aerosil: 30. The chemistry and physics of the aerosil surface. J. Colloid Interface Sci. 125, 61–68. doi: 10.1016/0021-9797(88)90054-9
McClements, D. J. (2018). The biophysics of digestion: lipids. Curr. Opin. Food Sci. 21, 1–6. doi: 10.1016/j.cofs.2018.03.009
McNamee, C. E., Tsujii, Y., and Matsumoto, M. (2004). Interaction forces between two silica surfaces in an apolar solvent containing an anionic surfactant. Langmuir 20, 1791–1798. doi: 10.1021/la035730+
Michalski, M. C., Genot, C., Gayet, C., Lopez, C., Fine, F., Joffre, F., et al. (2013). Multiscale structures of lipids in foods as parameters affecting fatty acid bioavailability and lipid metabolism. Prog. Lipid Res. 52, 354–373. doi: 10.1016/j.plipres.2013.04.004
Mu, H., and Høy, C.-E. (2004). The digestion of dietary triacylglycerols. Prog. Lipid Res. 43, 105–133. doi: 10.1016/S0163-7827(03)00050-X
Nordström, J., Aguilera, L., and Matic, A. (2012). Effect of lithium salt on the stability of dispersions of fumed silica in the ionic liquid BMImBF4. Langmuir 28, 4080–4085. doi: 10.1021/la204555g
O′Sullivan, C. M., Davidovich-Pinhas, M., Wright, A. J., Barbut, S., and Marangoni, A. G. (2017). Ethylcellulose oleogels for lipophilic bioactive delivery – effect of oleogelation on in vitro bioaccessibility and stability of beta-carotene. Food Funct. 8, 1438–1451. doi: 10.1039/C6FO01805J
Patel, A. R., Babaahmadi, M., Lesaffer, A., and Dewettinck, K. (2015a). Rheological profiling of organogels prepared at critical gelling concentrations of natural waxes in a triacylglycerol solvent. J. Agric. Food Chem. 63, 4862–4869. doi: 10.1021/acs.jafc.5b01548
Patel, A. R., Mankoc, B., Bin Sintang, M. D., Lesaffer, A., and Dewettinck, K. (2015b). Fumed silica-based organogels and ‘aqueous-organic' bigels. RSC Adv. 5, 9703–9708. doi: 10.1039/C4RA15437A
Pereira Camelo, S. R., Franceschi, S., Perez, E., Girod Fullana, S., and Ré, M. I. (2016). Factors influencing the erosion rate and the drug release kinetics from organogels designed as matrices for oral controlled release of a hydrophobic drug. Drug Dev. Indus. Pharm. 42, 985–997. doi: 10.3109/03639045.2015.1103746
Pratsinis, S. E. (1998). Flame aerosol synthesis of ceramic powders. Prog. Energy Combust. Sci. 24, 197–219. doi: 10.1016/S0360-1285(97)00028-2
Proctor, A., Adhikari, C., and Blyholder, G. D. (1996). Lipid adsorption on commercial silica hydrogels from hexane and changes in triglyceride complexes with time. J. Am. Oil Chem. Soc. 73, 693–698. doi: 10.1007/BF02517942
Puşcaş, A., Mureşan, V., Socaciu, C., and Muste, S. (2020). Oleogels in food: a review of current and potential applications. Foods 9:70. doi: 10.3390/foods9010070
Raghavan, S. R., Hou, J., Baker, G. L., and Khan, S. A. (2000a). Colloidal interactions between particles with tethered nonpolar chains dispersed in polar media: direct correlation between dynamic rheology and interaction parameters. Langmuir 16, 1066–1077. doi: 10.1021/la9815953
Raghavan, S. R., Walls, H. J., and Khan, S. A. (2000b). Rheology of silica dispersions in organic liquids: new evidence for solvation forces dictated by hydrogen bonding. Langmuir 16, 7920–7930. doi: 10.1021/la991548q
Raikos, V., and Ranawana, V. (2017). Designing emulsion droplets of foods and beverages to enhance delivery of lipophilic bioactive components - a review of recent advances. Int. J. Food Sci. Tech. 52, 68–80. doi: 10.1111/ijfs.13272
Reis, P., Holmberg, K., Debeche, T., Folmer, B., Fauconnot, L., and Watzke, H. (2006). Lipase-catalyzed reactions at different surfaces. Langmuir 22, 8169–8177. doi: 10.1021/la060913s
Reis, P., Holmberg, K., Miller, R., Krägel, J., Grigoriev, D. O., Leser, M. E., et al. (2008). Competition between lipases and monoglycerides at interfaces. Langmuir 24, 7400–7407. doi: 10.1021/la800531y
Reis, P., Holmberg, K., Watzke, H., Leser, M. E., and Miller, R. (2009). Lipases at interfaces: A review. Adv. Colloid Interface Sci. 147–148, 237–250. doi: 10.1016/j.cis.2008.06.001
Rogers, M. A. (2018). Hansen solubility parameters as a tool in the quest for new edible oleogels. J. Am. Oil Chem. Soc. 95, 393–405. doi: 10.1002/aocs.12050
Roke, S., Buitenhuis, J., Miltenburg, J. C. v, Bonn, M., Blaaderen, A., et al. (2005). Interface–solvent effects during colloidal phase transitions. J. Phys. Condens. Matter 17, S3469–S3479. doi: 10.1088/0953-8984/17/45/036
Sato, K., and Ueno, S. (2014). “Physical properties of fats in food,” in Fats in Food Technology (Chichester: John Wiley & Sons), 1–38. doi: 10.1002/9781118788745.ch1
Schmid, R. D., and Verger, R. (1998). Lipases: interfacial enzymes with attractive applications. Angew. Chem. Int. Ed. 37, 1608–1633. doi: 10.1002/(SICI)1521-3773(19980703)37:12<1608::AID-ANIE1608>3.0.CO;2-V
Siepmann, J., and Siepmann, F. (2008). Mathematical modeling of drug delivery. Int. J. Pharma. 364, 328–343. doi: 10.1016/j.ijpharm.2008.09.004
Simovic, S., Heard, P., Hui, H., Song, Y., Peddie, F., Davey, A. K., et al. (2009). Dry hybrid lipid–silica microcapsules engineered from submicron lipid droplets and nanoparticles as a novel delivery system for poorly soluble drugs. Mol. Pharm. 6, 861–872. doi: 10.1021/mp900063t
Singh, H., Ye, A., and Ferrua, M. J. (2015). Aspects of food structures in the digestive tract. Curr. Opin. Food Sci. 3, 85–93. doi: 10.1016/j.cofs.2015.06.007
Skelhon, T. S., Grossiord, N., Morgan, A. R., and Bon, S. A. F. (2012). Quiescent water-in-oil Pickering emulsions as a route toward healthier fruit juice infused chocolate confectionary. J. Mat. Chem. 22, 19289–19295. doi: 10.1039/c2jm34233b
Smith, W. E., and Zukoski, C. F. (2006a). Aggregation and gelation kinetics of fumed silica-ethanol suspensions. J. Colloid Interface Sci. 304, 359–369. doi: 10.1016/j.jcis.2006.09.016
Smith, W. E., and Zukoski, C. F. (2006b). Role of solvation forces in the gelation of fumed silica-alcohol suspensions. J. Colloid Interface Sci. 304, 348–358. doi: 10.1016/j.jcis.2006.08.021
Sugino, Y., and Kawaguchi, M. (2017). Fumed and precipitated hydrophilic silica suspension gels in mineral oil: stability and rheological properties. Gels 3:32. doi: 10.3390/gels3030032
Tan, A., Martin, A., Nguyen, T.-H., Boyd, B. J., and Prestidge, C. A. (2012). Hybrid nanomaterials that mimic the food effect: controlling enzymatic digestion for enhanced oral drug absorption. Angew. Chem. Int. Ed. 51, 5475–5479. doi: 10.1002/anie.201200409
Tan, A., Simovic, S., Davey, A. K., Rades, T., Boyd, B. J., and Prestidge, C. A. (2010). Silica nanoparticles to control the lipase-mediated digestion of lipid-based oral delivery systems. Mol. Pharm. 7, 522–532. doi: 10.1021/mp9002442
Tang, D., and Marangoni, A. G. (2006). Quantitative study on the microstructure of colloidal fat crystal networks and fractal dimensions. Adv. Colloid Interface Sci. 128-130, 257–265. doi: 10.1016/j.cis.2006.11.019
Vansant, E. F., van Der Voort, P., and Vrancken, K. C. (1995). Characterization and Chemical Modification of the Silica Surface. Amsterdam: Elsevier.
Wang, R., Guo, J., Baran, G., and Wunder, S. L. (2000). Characterization of the State of order of octadecylsilane chains on fumed silica. Langmuir 16, 568–576. doi: 10.1021/la9908081
Whitby, C. P., Krebsz, M., and Booty, S. J. (2018). Understanding the role of hydrogen bonding in the aggregation of fumed silica particles in triglyceride solvents. J. Colloid Interface Sci. 527, 1–9. doi: 10.1016/j.jcis.2018.05.029
Wilde, P. J., and Chu, B. S. (2011). Interfacial & colloidal aspects of lipid digestion. Adv. Colloid Interface Sci. 165, 14–22. doi: 10.1016/j.cis.2011.02.004
Wu, X.-J., Wang, Y., Wang, M., Yang, W., Xie, B.-H., and Yang, M.-B. (2012a). Structure of fumed silica gels in dodecane: enhanced network by oscillatory shear. Colloid Poly. Sci. 290, 151–161. doi: 10.1007/s00396-011-2535-4
Wu, X.-J., Wang, Y., Yang, W., Xie, B.-H., Yang, M.-B., and Dan, W. (2012b). A rheological study on temperature dependent microstructural changes of fumed silica gels in dodecane. Soft Matter 8, 10457–10463. doi: 10.1039/c2sm25668a
Yziquel, F., Carreau, P. J., and Tanguy, P. A. (1999). Non-linear viscoelastic behavior of fumed silica suspensions. Rheol. Acta 38, 14–25. doi: 10.1007/s003970050152
Zaborski, M., Vidal, A., Ligner, G., Balard, H., Papirer, E., and Burneau, A. (1989). Comparative study of the surface hydroxyl groups of fumed and precipitated silicas. I. grafting and chemical characterization. Langmuir 5, 447–451. doi: 10.1021/la00086a028
Zetzl, A. K., Gravelle, A. J., Kurylowicz, M., Dutcher, J., Barbut, S., and Marangoni, A. G. (2014). Microstructure of ethylcellulose oleogels and its relationship to mechanical properties. Food Struc. 2, 27–40. doi: 10.1016/j.foostr.2014.07.002
Keywords: fumed silica aggregation, fumed silica gel rheology, oleogel, silica-lipid hybrid, fused silica
Citation: Whitby CP (2020) Structuring Edible Oils With Fumed Silica Particles. Front. Sustain. Food Syst. 4:585160. doi: 10.3389/fsufs.2020.585160
Received: 19 July 2020; Accepted: 28 September 2020;
Published: 22 October 2020.
Edited by:
Luiz Henrique Fasolin, Campinas State University, BrazilReviewed by:
Zhao-Yan Sun, Chinese Academy of Sciences, ChinaVesna Radovanović, Environmental Consulting Agency, Serbia
Copyright © 2020 Whitby. This is an open-access article distributed under the terms of the Creative Commons Attribution License (CC BY). The use, distribution or reproduction in other forums is permitted, provided the original author(s) and the copyright owner(s) are credited and that the original publication in this journal is cited, in accordance with accepted academic practice. No use, distribution or reproduction is permitted which does not comply with these terms.
*Correspondence: Catherine P. Whitby, Yy5wLndoaXRieUBtYXNzZXkuYWMubno=