The Current and Future Role of Microbial Culture Collections in Food Security Worldwide
- 1Departamento de Ciencias Agronómicas y Veterinarias, Instituto Tecnológico de Sonora, Ciudad Obregón, Sonora, Mexico
- 2Campo Experimental Norman E. Borlaug, Instituto Nacional de Investigaciones Forestales, Agrícolas y Pecuarias, Ciudad Obregón, Mexico
- 3Instituto de Investigaciones Químico Biológicas, Universidad Michoacana de San Nicolás de Hidalgo, Morelia, Michoacán, Mexico
- 4Instituto de Microbiología y Zoología Agrícola, Instituto Nacional de Tecnología Agropecuaria (INTA-IMYZA), Castelar, Argentina
- 5School of Environmental Studies, Jadavpur University, Kolkata, India
Food security is the pillar of nutritional wellbeing for food availability, and is necessary to satisfy all physiological needs to thus maintain the general wellbeing of populations. However, global agricultural deficiencies occur due to rapid population growth, causing an increase in competition for resources; such as water, land, and energy, leading to the overexploitation of agro-ecosystems, and the inability to produce a suitable quantity of efficient food. Therefore, the development of sustainable agro-biotechnologies is vital to increase crop yield and quality, reducing the negative impacts caused by intensive non-sustainable agricultural practices. In this way, the genetic and metabolic diversity of soil and plant microbiota in agro-ecosystems are a current and promising alternative to ensure global food security. Microbial communities play an important role in the improvement of soil fertility and plant development by enhancing plant growth and health through several direct and/or indirect mechanisms. Thus, the bio-augmentation of beneficial microbes into agro-ecosystems not only generates an increase in food production but also mitigates the economic, social, and environmental issues of intensive non-sustainable agriculture. In this way, the isolation, characterization, and exploitation of preserved beneficial microbes in microbial culture collections (MCC) is crucial for the ex situ maintenance of native soil microbial ecology focused on driving sustainable food production. This review aims to provide a critical analysis of the current and future role of global MCC on sustainable food security, as providers of a large number of beneficial microbial strains with multiple metabolic and genetic traits.
Introduction
The rapid growth of the human population will increase the current food demand over the coming years, but intensive non-sustainable agricultural practices place food security, the economy, and the environment at a risk worldwide. Thus, in recent years, global society has faced new challenges related to the development of ecological, efficient, and sustainable alternatives to satisfy increasing food demand (Martínez-Castillo, 2016). For example, the abrupt changes in climatic conditions at a global scale have had immense negative impacts on agricultural production. The Intergovernmental Panel on Climate Change (IPCC) predicts a global average temperature increase of 1.4 to 5.8°C during the current century (2001–2100), decreasing the production of major cereal crops such as maize (20–45%), rice (20–30%), and wheat (5–50%) (Arora, 2019). In addition, climate change will modify the lifecycle stages and the development rates of phytopathogens and pests (Gupta et al., 2018), which will reduce annual agricultural production by up to 30% (Sharip et al., 2012).
On the other hand, soil degradation is the most significant environmental problem for food production, causing poverty and hunger in developing countries (Pereira et al., 2017). Approximately 52% of the land used for agriculture worldwide is moderately or severely affected by soil degradation (ELD, 2015), due to erosion, salinization, acidification, contamination, or compaction (Kopittke et al., 2019). Soil erosion is the largest contributor to land degradation worldwide, leading to the loss of 75 billion tones of fertile soil per year with an annual economic cost of about USD 400 billion (ELD, 2015; International Atomic Energy Agency (IAEA), 2015). Thus, farmers have relied on the use of high amounts of synthetic agro-inputs to fertilize crops and maintain phytosanitary control, which negatively impact human and environmental health (Villarreal-Delgado et al., 2018). In this sense, in the past 40 years, the use of these agro-inputs in agriculture has increased drastically, i.e., nitrogen (N) has increased by 7.4 times but the yield has only increased by 2.4 times in the same period, indicating that crops have reduced their ability to use N efficiently (Hirel et al., 2011), which increases the economic and environmental cost of food production (Sharip et al., 2012). Therefore, the development of innovative and sustainable agro-biotechnologies focused on solving environmental, economic, and social issues associated with current intensive non-sustainable agricultural practices is needed to contribute to food security (Cano et al., 2017).
Through time, microorganisms have been studied due to their fundamental importance in the maintenance of multiple functions and ecosystem services in the biosphere (Delgado-Baquerizo et al., 2016). Microorganisms are an essential part of human wellbeing, participating in medicine, agriculture, aquaculture, food industry, and biotechnology, among others (Weng et al., 2005; Díaz-Rodríguez et al., 2017). Since microbiota is found in every place on the planet, it plays vital roles in ecosystems, such as; (i) social and ecological sustainability, (ii) adaptation and mitigation of climate change, (iii) as a biotechnological resource for humanity, (iv) water cycling and nutrients, and (v) the increase of food production (Kalia and Gupta, 2005; Pajares et al., 2016).
In agro-ecosystems, microbial communities interact with crops through direct or indirect action mechanisms, regulating their growth and productivity by increasing tolerance to abiotic and biotic stress, plant nutrition, and antagonism against phytopathogenic agents (Santoyo et al., 2019). This particular group of microorganisms are commonly named plant growth-promoting microorganisms (PGPM). PGPM inhabit soil and plants, colonizing roots in 105-107 colony forming units (CFU) per gram of fresh root. These interactions have beneficial impacts on health and plant growth (Mishra et al., 2017; Valenzuela-Aragon et al., 2018).
Currently, some members of several microbial genera have been studied for their ability to produce phytohormones, and to solubilize phosphates, such as Micrococcus, Pseudomonas, Ralstonia, Enterobacter, Pantoea, Acinetobacter, Bacillus, Aeromonahy, Burkholderia, and Microbacterium (Lara and Negrete, 2015; Ali et al., 2017). Additionally, others are related to the tolerance of different types of biotic and abiotic stress, such as Pseudomonas, Bacillus, and Azospirillum for salinity stress; Burkholderia and Rhizobium for water stress; and Azotobacter and Bacillus for nutrient uptake efficiency (Rokhzadi and Toashih, 2011; Choudhary et al., 2016). Moreover, a group of microorganisms known as biological control agents (BCA) have shown the ability to inhibit the growth of phytopathogens and mitigate the incidence of plant diseases, i.e., Bacillus amyloliquefaciens and Microbacterium oleovorans have demonstrated the ability to control Fusarium verticillioides (Etcheverry et al., 2009); Trichoderma asperellum controls Fusarium wilt; Metschnikowia pulcherrima inhibits the development of Botrytis cinerea, Alternaria alternata, and Penicillium expansum (Köhl et al., 2019); bacteriophages control Erwinia amylovora and Ralstonia solanacearum; and agriphage controls Pseudomonas syringae and Xanthomonas campestris (Olanrewaju et al., 2017).
However, the agro-ecosystems are undergoing accelerated deterioration worldwide due to erosion, loss of organic carbon, nutrient depletion, soil sealing, climate change, and other threats, generating a loss of those promising PGPM genera (FAO, 2015a). Therefore, the conservation of this biological diversity is essential for its re-incorporation into agro-ecosystems. Thus, the role of microbial culture collections (MCC) is crucial in achieving this goal (Valenzuela-Ruiz et al., 2018). In this way, the emergence of biological concerns, world food insecurity, and the continuous discovery of new microbial species or subspecies (de los Santos Villalobos et al., 2019) creates the need not only to preserve these microorganisms but also to study them for the development of new agro-biotechnologies (de los Santos-Villalobos et al., 2018). Unfortunately, at present, almost all promising microorganisms are not fully exploited and correctly preserved, which impedes achieving a positive impact on the food security of the world in a sustainable way.
This review aims to highlight the importance of soil microbial resources for the development of sustainable agricultural practices, as well as to highlight the role of MCC as reservoirs and providers of PGPM. Finally, we critically analyze the current status and perspectives to increase the use, exchange, and exploitation of promising microbial strains preserved in these culture collections for sustainably contributing to global food security.
Global Agricultural Issues for Food Security
It is estimated that 38.5% of the surface of the planet is dedicated to agriculture, which has increased 1% per year, while food production has increased between 2 and 4% per year (FAO, 2017c). In addition, around 800 million people in the world, or 1 in every 9 humans, suffer from hunger; also, more than two million people suffer from nutrient deficiency, which is known as “hidden hunger” (FAO, 2017a). This is strong evidence of the global food security crisis, which is understood as the failure of nutritional wellbeing through the availability of food necessary to satisfy all physiological needs and maintain the health of the population (Wheeler and Von Braun, 2013). On the other hand, it is estimated that the global population will increase up to 10 billion people by 2050; therefore, agricultural production will have to increase between 50 and 100% to meet global food demands (FAO, 2017c).
The constant increase in the global human population has caused an increment in competition for resources such as water, land, and energy, leading to the overexploitation of agro-ecosystems. This scenario results in the inability to produce enough nutritious and equitable food, awakening the need to develop sustainable alternatives (Godfray et al., 2010; FAO, 2017b). For example, during the green revolution (1960–1980), crop production patterns changed considerably to meet the food demand of the growing world population between 1950 and 1998 (from 2.6 to 5.9 billion people, respectively) (Sunding and Zilberman, 2001). Thus, intensive non-sustainable agricultural practices such as the increased use of synthetic fertilizers and pesticides, large-scale irrigation, and new varieties of high-yield crops were implemented (Gliessman, 2002; Matson, 2012). However, food demand is still on the rise, and intensive non-sustainable agricultural practices have failed to continuously enhance crop yields and quality, generating serious in and ex situ environmental, economic, and social consequences (Matson, 2012; FAO, 2019).
Intensive non-sustainable agriculture exerts significant internal and external effects. There are two types of external effects: (1) outside the agro-ecosystem, where there is a depletion of groundwater and environmental contamination by the usage of synthetic agro-inputs; and (2) global effects, which result in greenhouse gas emissions, and animal, plant, and human diseases. In addition, as an internal effect (in the agro-ecosystem) soil degradation causes salinization, the reduction of organic matter content, and lowers plant nutrient use efficiency (Lal, 2015; Struik and Kuyper, 2017). For example, <40% of applied nitrogen fertilizers are used efficiently by plants, where the remaining 60% results in volatilization, accumulation in soils, leaching in rivers, lakes, and streams, among others (FAO, 2017d; Chandini et al., 2019). Moreover, according to Tsiafouli et al. (2015), intensive non-sustainable agriculture reduces the microbial diversity and population, which leads to processes of biological and chemical degradation in the soil. At present, soil degradation is increasing at a rate of 5–7 million hectares per year worldwide (International Atomic Energy Agency (IAEA), 2014), and is largely due to its exploitation to satisfy the rising food demand (Maura and Febles, 2018). This reduces the global food productivity by 12%, increasing food prices by 30% (ELD, 2015).
On the other hand, climate change presents a huge challenge for agriculture. It is estimated that by 2050 the temperature of the planet will increase by an average of 3.5–4.7°C, causing warm winters and summers in many countries. This will have negative consequences for water supply, cooling, and food production (Leahy, 2019). However, agriculture also has a part in the contribution to global climate change through the production of synthetic agro-inputs, an activity that uses a lot of energy by burning fossil fuels and emitting CO2 into the atmosphere. Agriculture contributes 20% of the CO2eq emissions globally, while at the regional level, Africa and Latin America contributed the largest amount to the total CO2eq emissions due to agriculture, with a share of ~60% (FAO, 2020). In this way, climate change has different negative effects on ecological and physiological events in crops, such as changes in the soil microbial ecology, plant-microbiome interactions, plant growth rates, alterations in the distribution regimes, and proliferation of new phytopathogens, pests, and weeds (Mall et al., 2017). These problems will lead to increased crop production costs with lower yields (Nelson et al., 2009; Thomson et al., 2010). Thus, one of the main challenges for agriculture is the adaptation to anthropogenic and natural changes to increase crop yields by sustainable agro-biotechnologies (Foley, 2011), where one of the most promising alternatives is the use of the genetic and metabolic diversity of native microbiota in agro-ecosystems.
Microbial Diversity in Agro-Ecosystems
Soil is the thin layer that covers the Earth, made up of organic substances, living organisms, air, water, and mineral particles. Soil is a vital natural resource from which most of the global food is produced (Hartemink, 2016). Food production requires essential nutrients, metabolites, and water, among others, that are provided by soil to plants. Thus, soil fertility is directly related to the quantity and quality of produced food (FAO, 2015b). In addition, this matrix provides ecosystem services, such as water purification, degradation of pollutants, flood and climate regulation, food, fiber, and fuel supply, carbon retention, nutrient cycling, a source of pharmaceuticals, and genetic resources (Adhikari and Hartemink, 2016). Soil contains a large reservoir of microorganisms (1 × 109 microbial cells g−1 dry soil) and microbial diversity (1 × 105 microbial species g−1 dry soil) (Bodelier, 2011; Bhattarai et al., 2015). These microbial communities are responsible for carrying out between 80 and 90% of its biological processes, including biogeochemical cycles (indispensable for maintaining the equilibrium of agro-ecosystems), organic matter decomposition, soil formation, primary production, climate regulation, and disease control, among others (Nannipieri et al., 2017; Saccá et al., 2017).
Besides, microbial communities play an important role in improving soil fertility, where microbial genera such as Azotobacter, Azospirillum, Heliobacterium, Bradyrhizobium, Bacillus, Gluconacetobacter, Methylobacterium, Nitrosomonas, Nitrobacter, Klebsiella, and Pseudomonas are involved, for example, in the N cycle including N2 fixation, nitrification, denitrification, and ammonification (Pajares and Bohannan, 2016). On the other hand, microorganisms are involved in the land-atmosphere carbon (C) exchange, through the balance between respiration and photosynthesis by carbon fixers, such as autotrophic (chemoautotrophic and photoautotrophic) microbes (Gougoulias et al., 2014). These communities are also important in the phosphorus (P) cycle, due to their ability to solubilize phosphates, i.e., fungal (some species of Aspergillus and Penicillium) and bacterial (some species of Bacillus, Micrococcus, and Pseudomonas) genera are involved in phosphate solubilization by the production of organic acids and the excretion of protons during assimilation (Mullen, 2019). Thus, in recent years, more attention has been paid to the function of microorganisms in agro-ecosystems, since they play a fundamental role in plant health and food production, as well as the improvement of soil fertility (Johansson et al., 2004).
The Role of Plant Growth-Promoting Microorganisms (PGPM) in Food Production
The use of beneficial microbes, named plant growth-promoting microorganisms (PGPM), as microbial inoculants (biofertilizers) is a sustainable alternative to improve crop yields. PGPM (rhizobacteria, soil or endophytic bacteria, endo- or ectomycorrhizal fungi, cyanobacteria, and many others) can colonize soil and plants in significant quantities (105-107 CFU per gram of fresh root) and exert beneficial effects on plants through several mechanisms (Mishra et al., 2017). They can improve nutrient uptake, plant growth, and plant tolerance to abiotic and biotic stress, as well as biocontrol agents against plant pathogens and pests (Gangwar et al., 2017; Valenzuela-Ruiz et al., 2018).
Microbial inoculants are biodegradable bio-products that contain living or inactive cells of PGPM with the ability to colonize the rhizosphere or inner part of plants, and perform growth-promoting effects on plants (Umesha et al., 2018; Singh et al., 2019). These bio-products are an eco-friendly way to improve the growth of plants by reducing the damage caused by phytopathogens or pests and improving their resistance to abiotic stress (Chávez-Díaz et al., 2020). The use of PGPM in agriculture has gained increasing interest and is currently a sustainable alternative for global food production due to their positive impacts on plant growth (Parewa et al., 2018), mitigation of the pollution generated by agrochemicals, and the reduction of soil degradation (Dubey et al., 2017; Mishra et al., 2017).
In this sense, the earliest microorganisms used as inoculants were the “rhizobia,” diazotrophic bacteria able to colonize the rhizosphere and establish nodules in the roots of host plants (Ciancio et al., 2016; Berg et al., 2017). Currently, several microbial genera are used in the formulation of microbial inoculants due to their metabolic diversity, i.e., many Bacillus species induce growth promotion in plants, control phytopathogens, and are spore-forming bacteria-resistant to stressful conditions (Villarreal-Delgado et al., 2018; Ibarra-Villarreal et al., 2021). Some members of the genus Klebsiella and Rhizobium can fix nitrogen, solubilize organic and inorganic phosphates, produce 1-aminocyclopropane-1-carboxylate (ACC) deaminase, and produce phytohormones (Suliasih and Widawati, 2019). A large number of bacteria of the genus Azospirillum can produce phytohormones, polyamines, and fix atmospheric nitrogen (Cassán et al., 2014; Puente et al., 2018). Some strains of Enterobacter are biocontrol agents against phytopathogens, while some Arthrobacter strains can degrade a wide variety of compounds, including aromatic molecules, pesticides, and organochloride (Fernández-González et al., 2017). Besides, it has been reported that some Burkholderia species can bioremediate pollutants and are applied to biocontrol phytopathogens, can fix atmospheric nitrogen, and promote plant growth (Bolívar-Anillo et al., 2016). Some strains of Serratia induce plant growth by stimulating phytohormone production and phosphate solubilization, as well as inducing systemic resistance, enhancing salinity tolerance, and fixing atmospheric nitrogen (Devi et al., 2016; Singh and Jha, 2016; Moreno et al., 2018). However, these positive effects on crops caused by PGPM depend on the soil and climate conditions, crop genotype, plant phenological stage, root exudates, agricultural practices, the ability of PGPM to colonize the soil and plant tissues, and the application modes of beneficial strains, among others (Valenzuela-Aragon et al., 2018).
PGPM improve plant growth and health through direct, indirect, or dual mechanisms (Figure 1). They are considered to be direct mechanisms when bacteria begins to synthesize metabolites or when they increase the availability of nutrients to plants, the most common mechanisms being the production of phytohormones and ACC deaminase, nitrite production, sulfide oxidation, organic phosphate mineralization, and inorganic phosphorus solubilization. On the other hand, indirect mechanisms are related to the inhibition and elimination of phytopathogens through the competition for space and nutrients, the production of antibiotics, antimicrobial substances or lytic enzymes, interference in quorum sensing signaling, induced systemic resistance, and biofilm formation. Furthermore, there are mechanisms with dual activity (direct and indirect), where the most common is the production of siderophores, molecules that sequester iron making it available for the plants but less available to phytopathogens (Moreno et al., 2018; Parewa et al., 2018; Santoyo et al., 2019).
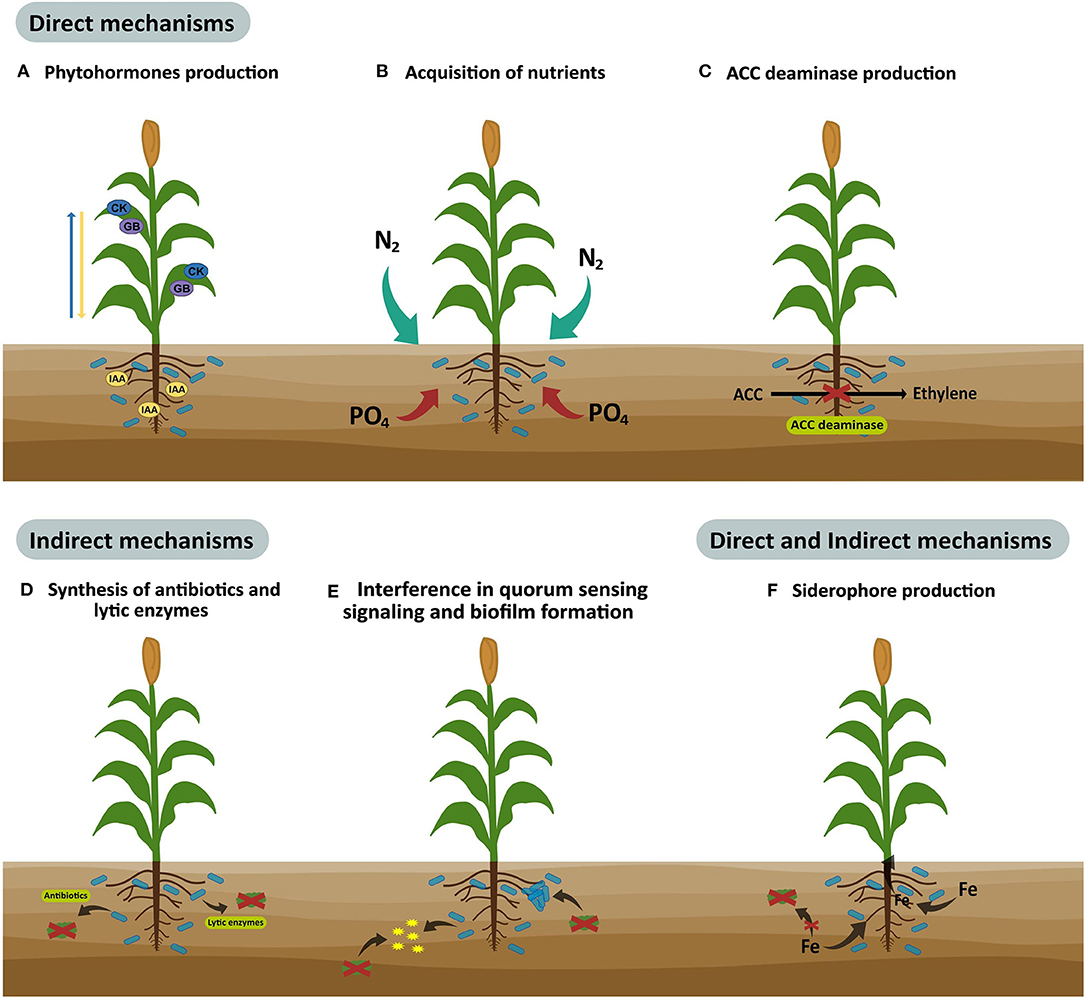
Figure 1. Direct, indirect, or dual mechanisms used by plant growth-promoting microorganisms to improve crop yield and quality.
PGPM can exert more than one growth promotion mechanism and may be used in different stages of the plant growth cycle (Gupta et al., 2015; Parewa et al., 2018). For example, strains of Pseudomonas fluorescens are the most used bacteria for preventing plant diseases through antibiotics production, which also stimulate plant growth by amino acids, specific growth promoters, and the synthesis of hormones. Additionally, strains of Bacillus subtilis promote plant growth by phytohormones and cause resistance against Fusarium oxysporum in tomatoes, and Rhizoctonia solani and Phytophthora nicotianae in tobacco plants (Villarreal-Delgado et al., 2018). Also, Trichoderma species produce phytohormones and solubilize phosphates, generating growth-promoting effects, and the control of pathogenic fungi in soil (Peláez-Álvarez et al., 2016; Leal-Almanza et al., 2018). Arbuscular mycorrhizal fungi (AMF) are the most prevalent PGPM found in agricultural soils (Nadeem et al., 2014; Nath and Meena, 2018). These are root obligate symbiotrophs that provide essential nutrients, pathogen protection, and stress tolerance to the host plant (Begum et al., 2019). AMF, symbiotic, and free-living PGPM have beneficial effects that increase plant health, growth, and yields, under optimal or stressful conditions (Nadeem et al., 2014). Thus, synergistic interactions between AMF and other PGPM can enhance the nutrient uptake and growth-promoting effects, in some cases to a greater extent compared to individual microbial strains. For example, these microbial interactions improve the phosphorus bioavailability and absorption by plants (Nanjundappa et al., 2019), a mechanism used by these beneficial microbial consortia for solubilizing phosphates is organic acid production (acetic, lactic, gluconic, formic, and oxalic acids, etc.), which solubilize phosphate rocks by acidification processes. Moreover, co-inoculated PGPM can produce chelating substances effective for calcium, aluminum, and iron causing the liberation of inorganic phosphate (Beltrán, 2014; Prabhu et al., 2019). On the other hand, the production of inorganic acids (sulfuric acid, nitric acid, and carbonic acid) by beneficial microbial consortia is involved in phosphate solubilization, but in the absence of acid production, the phosphate solubilization occurs by proton extrusion with nitrogen. The organic phosphate-solubilization by the co-inoculation of beneficial microbes is carried out by enzyme activity, once phosphatase and C-P lyase hydrolyze ester bonds of phosphonates (Prabhu et al., 2019).
Several different studies have suggested that microbial inoculants based on compatible microbial consortia may confer an advantage over formulations based on single strains (Egamberdieva et al., 2013). This is due to the ability for combining different functional metabolites and capabilities to improve soil quality and crop yield (Thilagar et al., 2016). For example, the co-inoculation in black bean (Phaseolus vulgaris) with Trichoderma atroviride and Bacillus subtilis showed a significant growth promotion in plant-dry biomass (43% over the control) compared to un-inoculated plants; while the individual inoculation of T. atroviride or B. subtilis increased only 2% or 34% in this trait, respectively (Yobo et al., 2011). Similarly, the co-inoculation of Trichoderma asperellum and arbuscular mycorrhizal fungi (Gigaspora margarita and Acaulospora tuberculata) showed a significant increase in plant height (>20 cm), as well as root and shoot fresh weights (>4.7 and >6.9 g/plant, respectively) compared to the un-inoculated treatment (Tchameni et al., 2011). The co-inoculation of rhizobia-Azospirillum in legumes showed a greater production of flavonoids in plants, which triggered the expression of the Rhizobium nod gene (Marks et al., 2013; Puente et al., 2018). Puente et al. (2019) confirmed that soybean seeds inoculated (at sowing) with Bradyrhizobium japonicum E109 and later foliar-sprayed with A. brasilense Az39 showed higher fresh and dry shoot biomass, more nodules, higher leghemoglobin levels, and increased nitrogen and protein content in grains, in comparison with the B. japonicum E109-alone treated seeds.
At present, several successful applications of microbial inoculants have been reported, with an increase of up to 10–30% in crop yields, and reductions of the amount of applied chemical fertilizers by up to 50% (Alori and Babalola, 2018; Parewa et al., 2018). In this way, the use of microbial inoculants is growing. The global market for biofertilizers is projected to reach 2.6 billion USD at a CAGR of 14.42% by the end of the year 2023 (Market Research, 2018). Thus, the extensive use of microbial inoculants is an alternative to improve soil fertility, crop quality, and yields, and contribute to mitigating the effects of climate change, as well as to increase the development of regional economies by the establishment of sustainable agribusiness (de los Santos-Villalobos et al., 2018). In this way, the preservation of isolated PGPM and their re-incorporation into agro-ecosystems is vital to maintain their ecological functions (Wood et al., 2015), for which their safeguarding in certified MCC makes them available for bioprospecting (de los Santos-Villalobos et al., 2018; Toader et al., 2019). Therefore, these MCC should focus on the study of metabolic, molecular, and functional traits of promising PGPM for developing sustainable agro-biotechnological alternatives (Smith, 2003; de los Santos-Villalobos et al., 2018).
Considerations and Challenges in the Use of Microbial Inoculants
PGPM can be used as biofertilizers and/or biopesticides, depending on their genetic and metabolic diversity. However, several microbial strains can exhibit different results in the same plant species and vice versa. In this way, there are several successful experiments at the laboratory and/or greenhouse level; however, those promising results sometimes are highly variable in the field, due to the complexity of the agro-ecosystem (Timmusk et al., 2017). Thus, it is important to select a specific microbial inoculant according to agroecosystem conditions, crop genotype, agricultural practices, climatic conditions, and expected benefits (Martínez-Viveros et al., 2010). The microbial inoculant efficiency depends on the establishment of its PGPM in the host plant and the rhizosphere, which is based on the inoculated PGPM-plant-microbiota competition mediated through the plant exudates (Jin et al., 2019). Besides, the climatic condition is another crucial factor involved in microbial inoculant effectiveness in the field. According to global estimates, abiotic factors lead to crop yield losses of 50%, temperature variations are the main contributor (27%); however, salinity (10%), drought (9%), and other forms of stress (4%) also affect crop yields and the success of microbial inoculants (de los Santos-Villalobos et al., 2018; Kaur et al., 2018; Ibarra-Villarreal et al., 2021). Still, little is known about the factors that control the competition of microbial species in the field; therefore, it is critical to have a higher number of cells to compete with native microorganisms and produce an increase in productivity (Compant et al., 2019). The estimated PGPM concentration required to cause positive effects in plants cannot be established as a general standard because it varies from one species to another; however, some microbial inoculants contain from 107 to 109 CFU/g of bioproduct, another influential factor is the lack of common international standards (Zayed, 2016).
On the other hand, the success of microbial inoculants depends on their application modes (plant tissues, rhizosphere, seeds, and/or bulk soil). These modes should be defined according to the type of crop, soil properties, climatic conditions, and agricultural practices in the field (Patil and Solanki, 2016; Kaushik and Djiwanti, 2019). Thus, it is important to highlight that the use of microbial inoculants in the field under different biotic and abiotic factors is a promising agro-biotechnological alternative to contribute to food safety sustainably.
Role of MCC in the Preservation of the Beneficial Microbial Diversity
The progress of microbiology brought the need to establish collections of microbial cultures to study and ex situ preserve the biodiversity in ecosystems and the distribution of promising microbial strains to produce goods and services. Subsequently, advances in molecular biology stimulated the development of intensive programs for bioprospecting all these microorganisms (Altier, 2013).
MCC, also known as biological resource centers (BRC), are primary suppliers of culturable microorganisms, replicable parts of these (i.e., DNA, genomes, and plasmids), and viable but not yet culturable microorganisms in biological or environmental matrices (Kurtzman and Labeda, 2009; Smith et al., 2014). According to Smith et al. (2014), the general concept of an MCC or BRC includes “the provision of services and repositories of the living cells, genomes of organisms, and information relating to heredity and the functions of biological systems.” The importance of MCC has been recognized by the Organization for Economic Cooperation and Development (OECD), which is a promoter of policies to improve economic wellbeing and social welfare at an international level (Smith et al., 2014). Therefore, these MCC have two main functions: (i) the conservation of agro-ecosystems through the isolation and preservation of the microbial diversity, providing a biological safeguard service; and (ii) the study and easy access of microorganisms, reference strains, and microbial resources to the public to generate biotechnological strategies (Mahilum, 2009).
The first MCC that provided a public service was established in Prague in 1890 by Professor Frantisek Král. Some of the strains that were deposited for the first time in this culture collection are still available (Smith et al., 2014). Later, other culture collections were created, such as the Mycothèque de l'Universitée Catholique de Louvain (MUCL) established in 1894 in Belgium, the Collection of the Centraalbureau voor Schimmelcultures (CBS) in 1906 in Holland, both specialized in fungi. Then, the American Type Culture Collection (ATCC) was established in the United States in 1925, which preserves different types of microorganisms (Sharma et al., 2017). Until 1920, the main role of MCC was initially for their value in carrying out both taxonomic and epidemiological studies; now culture collections have adopted new technologies to characterize and add value to the services they offer. In addition, with the advancement of biochemical and physiological studies, the conservation of microorganisms has been improving (Malik and Claus, 1987; Sharma and Shouche, 2014).
Thus, specific requirements have been established to maintain and ensure the quality and diffusion of all services provided by MCC (Smith, 1996; González and Jiménez, 2013), which can be divided into four categories (Figure 2). The first category is microbial culture characterization and authentication (A), which has the purpose of strain identification, as well as their preservation. This should ensure contamination-free cultures, the survival of at least 70% of cells, and stable maintenance of the preserved microorganisms (Escobar et al., 2016). The second category is the establishment of protocols (B), which focuses on the standardization of the protocols for the culture collections operation, from the preservation of different types of microorganisms to the maintenance of specialized equipment. Besides, it is necessary to have trained staff in cutting-edge technologies for the preservation, growth, and identification of microbes. The third category is diffusion (C), which is focused on all aspects related to achieving a greater scope and accessibility of the generated information (documentation and catalogs) to the general public. The last category is policies and standards (D), which seeks compliance with national and international laws, regulations, and policies about the safety, shipping, exchange of microorganisms, and other matters (González and Jiménez, 2013; Sharma and Shouche, 2014).
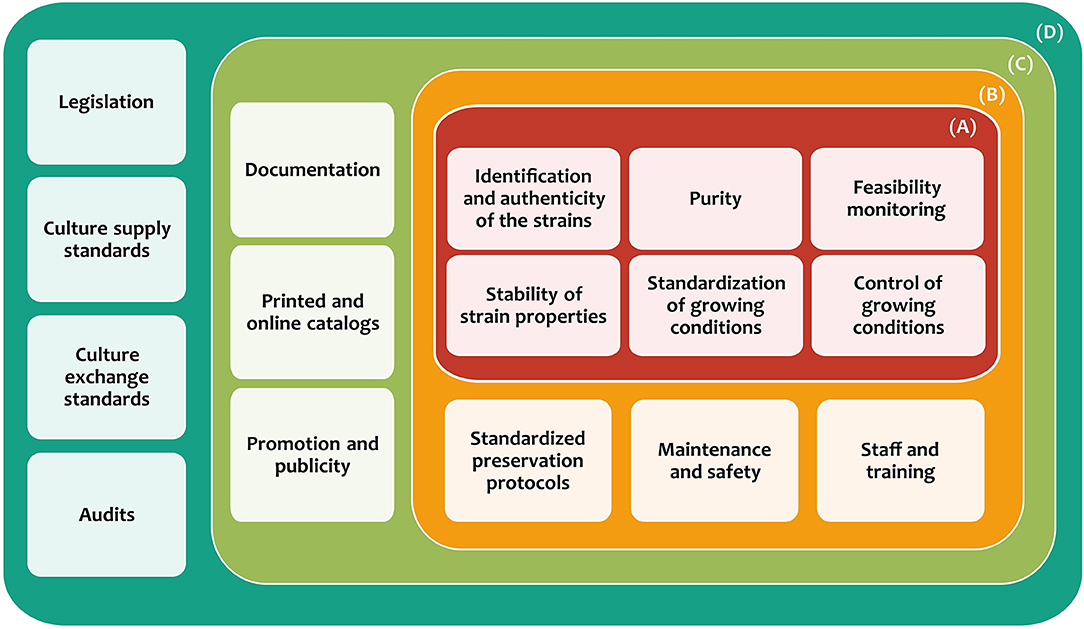
Figure 2. Categories and requirements for the establishment and maintenance of microbial culture collections. (A) Microbial culture characterization and authentication, (B) establishment of protocols, (C) diffusion, and (D) policies and standards.
At present, the World Federation for Culture Collections (WFCC) is the main organization that coordinates the activities of MCC worldwide (Sharma et al., 2017). It aims to promote and support the establishment and monitoring of MCC, by providing an information network between affiliated collections and users (Federación mundial de Colecciones de Cultivo, 2010). The WFCC supports the World Data Center of Microorganisms (WDCM) for the compilation of culture collection data, management, services provided, and most recent research with an online international database (Sharma et al., 2017). Currently, the WDCM lists 802 collections in 78 regions, of which 295 are located in Asia, 257 in Europe, 202 in America, 42 in Oceania, and 18 in Africa. The country with the largest number of MCC is Brazil (86), followed by Thailand (66) and China (48) (WDCM, 2020a). These MCC provide different services, such as: consulting, strain distribution, strain identification, patent deposits, storage service, and training (Sharma and Shouche, 2014; WDCM, 2020a). In this way, around the world, culture collections play a fundamental role in the preservation of microbial diversity, and the accessibility of axenic and stable promising strains for agriculture, genetics, industrial and medical microbiology, marine biology, and food safety, among others (Table 1) (Sharma et al., 2017).
The conservation of biodiversity and genetic resources provide essential support for emerging eco-efficient biotechnologies, in both the developed and developing world (OECD, 2009). The most economically powerful countries with the greatest scientific-technological development have created highly-specialized institutions to harbor large culture collections (approximately 98% of known microorganisms are preserved) such as the United States of America, China, Belgium, Japan, and India (WDCM, 2020a). Besides, many countries or individual institutions obtain official support for these culture collections; however, the establishment of MCC requires a constant source of funding that not all of them can obtain. Also, the records that include MCC must be digitized for use in international schemes (CGRFA, 2007). For example, in the region of Latin America and the Caribbean, there are valuable specialized MCC created and maintained by universities and research institutes; however, there is not a systematized information source about their progress, limitations, efforts, and initiatives.
Globally, several MCC are recognized for their role in the preservation of microorganisms with international or regional importance, which has a high impact on biotechnology at all levels, including food security (Table 1). For example, WDCM (at an international level) promotes the development of culture collections of microorganisms supporting the existing ones by providing advice and assistance (González and Jiménez, 2013). Similarly, ATCC safeguards and distributes standard reference microorganisms since they have a wide variety of high-quality biological materials (microorganisms, cell lines, and bioproducts) intended for scientific research (ATCC, 2020). One more example is the Colección de Microorganismos Edáficos y Endófitos Nativos (COLMENA), which is focused on the preservation, characterization, classification, and re-incorporation of native microorganisms to agro-ecosystems in Mexico, to reduce the loss of microbial diversity associated with food production (de los Santos-Villalobos et al., 2018). At present, a total of 3,272,734 microorganisms have been registered in the WDCM, of which 1,429,816 are bacteria (43.7%); 872,094 are fungi (26.6%); 39,491 are viruses (1.2%); and 33,020 are cell lines (1.0%) (WDCM, 2020a). Also, the WDCM has elaborated a catalog of reference strains for easy access, which are recommended for their use in quality control, regulated by different ISO standards. For this, the WDCM assigns a unique identification number to every reference strain, while the culture collection acronyms followed by a number are used as an identifier (at the strain level) for its global identification and tracking (Wu et al., 2016). This catalog contains a total of 196 reference strains of 131 species of bacteria (Table 2), fungi, and yeast (Table 3) (WDCM, 2019). Currently, according to scientific literature, many microbial species preserved in the WDCM could be grouped as PGPM, due to their large diversity of metabolic capabilities with wide agro-biotechnological applications. However, the number of AMF preserved in MCC is lower; probably due to limitations in the specific conversation methodologies since these microorganisms depend on their host plant to complete their life cycle, which makes it difficult to grow in pure synthetic media (Lalaymia et al., 2013). Thus, only two MCC (registered in WFCC) are specialized in these types of microorganisms holding 1,302 AMF strains in total (WDCM, 2020b). Therefore, it is necessary to improve short and long-term preservation methodologies for these microorganisms, which (like others PGPM) play a fundamental role in the growth regulation and nutrients acquisition by plants in ecosystems (Lalaymia et al., 2013; Begum et al., 2019).
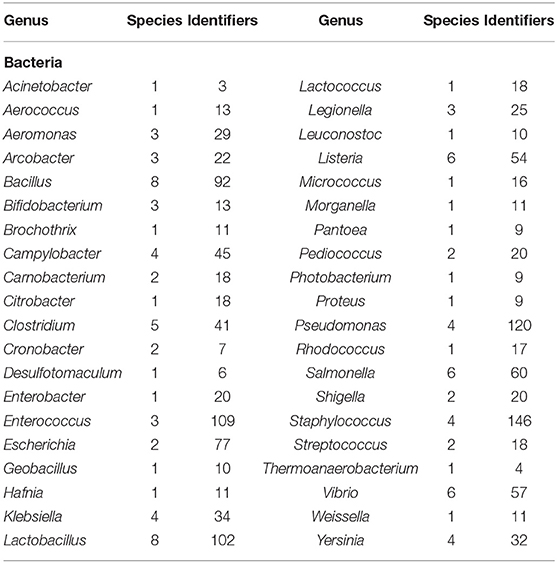
Table 2. The number of bacterial species in the WDCM reference strain catalog that have identifier numbers for the reference strains (WDCM, 2019).
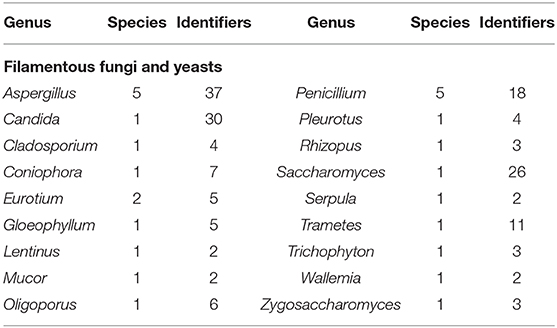
Table 3. The number of filamentous fungi and yeasts species in the WDCM reference strain catalog that have identifier numbers for the reference strains (WDCM, 2019).
On the other hand, the offered services by most of the MCC around the world are limited to the preservation, deposit, and transfer of microorganisms, and do not thoroughly study the strain capabilities; usually, this is left only to interested researchers or professionals. For this reason, more MCC focused, not only on microbial preservation, but also dedicated to the research, teaching, and characterization of promising strains for biotechnological applications are needed (de los Santos-Villalobos et al., 2018). Furthermore, only a few culture collections have a public catalog containing information about preserved microorganisms grouped by function, biosafety, taxonomy, and biotechnological applications, among others. This is a strong limitation for the extensive use of PGPM as microbial inoculants since it complicates the selection of promising strains. For this reason, MCC must also make the biological data of microorganisms more accessible to the community, including (i) taxonomy, (ii) source of isolation, including the biological and environmental context in which strains were found, (iii) evolution, (iv) metabolic-functional traits, and v) ecological relationship. All these data should be accessible to the general public through an online catalog (databases) with open access to make it easier to transfer microbial strains (Caktu and Turkoglu, 2011; Cruz-Leyva et al., 2015). However, at present, only 53% of MCC have a digital catalog of preserved microorganisms (WDCM, 2020a), the digitization and update of information for each preserved microorganism is one of the major limitations of culture collections. Thus, the expected positive impacts of preserved PGPM in MCC to global food security will be reached when, at least, three stages are linked: (i) the isolation of microbial strains in agro-ecosystems, (ii) the preservation of these strains in an MCC, and (iii) bioprospecting these promising strains to transfer and develop sustainable agro-biotechnological alternatives (Figure 3). Finally, rules and procedures have been established for the transfer and exploitation of genetic resources preserved in MCC, such as (i) the Budapest Treaty that mentions the International Recognition of the Deposit of Microorganisms for the Patent Procedure, established in 1980 (Organización Mundial de la Propiedad Intelectual (OMPI), 2017); and (ii) the Nagoya Protocol (implemented in October 2014), which aims for the fair and equitable sharing of the benefits derived from the use of genetic resources (Sharma et al., 2017). The Budapest Treaty regulates the process of patent deposits in an international framework; thus, a patent deposit made with one International Depositary Authority (IDA) is sufficient and recognized by all member states of Budapest. Nonmember countries may also accept deposits according to the Budapest Treaty as per the norms of the country (Bussas et al., 2017). On the other hand, the Nagoya Protocol is an agreement that complements the Convention on Biological Diversity. It provides a transparent and legal framework to effectively implement the beneficial sharing between providers and users of genetic resources, by contributing to the establishment of more predictable conditions to access the genetic resources, conservation, and sustainable uses of biodiversity (Convention on Biological Diversity, 2015).
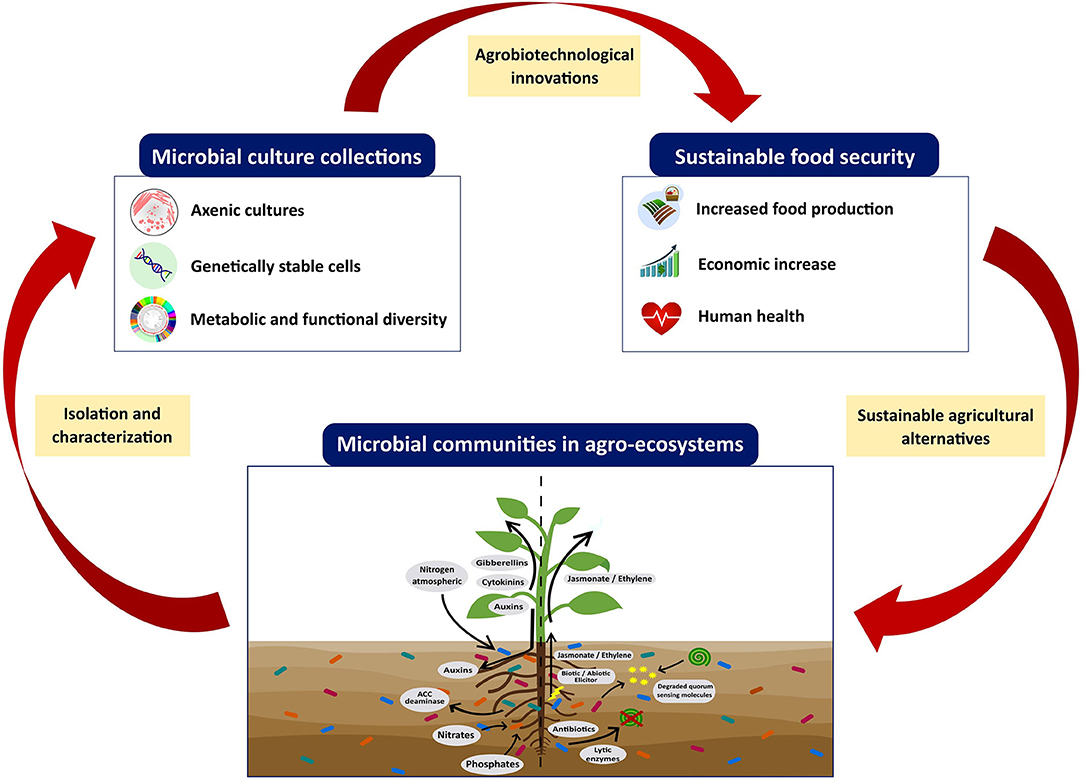
Figure 3. The role of microbial culture collections on global food security providing plant growth-promoting microorganisms for the innovation of sustainable agro-biotechnologies.
Conclusions
The concern for sustainable food production that meets the needs of the global human population has become a crucial issue in the agricultural sector. Thus, the development of new sustainable agro-biotechnological alternatives to increase crop yields and quality, and restore soil fertility, is vital to satisfy the current and future food demand worldwide. In this way, several PGPM represent a feasible and eco-friendly strategy to combat challenges of food security and climate change, due to their metabolic and genetic diversity. Regarding the agro-biotechnological potentials of PGPM, microbial communities in agro-ecosystems have become more relevant in recent years for the development of new microbial inoculants. These bioproducts can increase nutrition and stress tolerance in crops. Thus, the use of microbial inoculants can contribute to reducing the economic, environmental, and health costs of food production, but there are many considerations and challenges to address in order for them to be effective in the field. Besides, since there is no single universal solution for all these issues, the integration of microbial inoculants with other technologies (sustainable soil management, new genetic varieties of crops, and efficient use of agro-inputs, among others) are necessary to enhance the expected positive effects.
In this sense, the success of the extensive use of microbial inoculants depend on the isolation, identification, and characterization of promising PGPM, which should be preserved in MCC for (i) the ex situ conservation of these biological components of agro-ecosystems, (ii) the study of these microbial resources, and (iii) the transfer of these biological cultures to develop new agro-biotechnologies. Thus, it is essential to solving bottlenecks in the functioning of MCC, mainly the digitization, easy access, and dissemination of biological information for each preserved microbial strain (including metabolic and genetic diversity). This will lead to the extensive use of PGPM for the development of new strategies to increase sustainable food production. Thus, the role of global MCC is vital to provide biological resources that directly support soil health, sustainable food generation, and the wellbeing of the human population.
Author Contributions
Conceptualization, supervision, and validation were developed by SS-V. All authors contributed to manuscript revision, and have read and approved the submitted version. All authors wrote, visualized, and edited the manuscript.
Funding
The authors acknowledge the support of the Instituto Tecnológico de Sonora (ITSON) Project PROFAPI 2020_0013 Bacillus sp. TSO9: afiliación taxonómica a nivel del genoma e identificación de genes asociados a la promoción del crecimiento en el trigo. In addition, the master's scholarship granted by the National Council of Science and Technology (CONACYT) to Alondra María Díaz Rodríguez (CVU 908966).
Conflict of Interest
The authors declare that the research was conducted in the absence of any commercial or financial relationships that could be construed as a potential conflict of interest.
Acknowledgments
The authors thank Valeria Valenzuela Ruiz and Paulina Yunive Armenta Valdez for their support in English corrections and the format of this manuscript, respectively.
References
Adhikari, K., and Hartemink, A. E. (2016). Linking soils to ecosystem services-A global review. Geoderma 262, 101–111. doi: 10.1016/j.geoderma.2015.08.009
Ali, S., Charles, T. C., and Glick, B. R. (2017). “Endophytic phytohormones and their role in plant growth promotion,” in Functional Importance of the Plant Microbiome, ed S. Doty (Cham: Springer), 89–105.
Alori, E. T., and Babalola, O. O. (2018). Microbial inoculants for improving crop quality and human health in Africa. Front. Microbiol. 9:2213. doi: 10.3389/fmicb.2018.02213
Arora, N. K. (2019). Impact of climate change on agriculture production and its sustainable solutions. Environ. Sustain. 2, 95–96. doi: 10.1007/s42398-019-00078-w
ATCC (2020). About ATCC and Collection Holdings. Available online at: https://www.atcc.org/About (accessed September 27, 2020).
Begum, N., Qin, C., Ahanger, M. A., Raza, S., Khan, M. I., Ahmed, N., et al. (2019). Role of arbuscular mycorrhizal fungi in plant growth regulation: implications in abiotic stress tolerance. Front. Plant Sci. 10:1068. doi: 10.3389/fpls.2019.01068
Beltrán, M. (2014). Phosphate solubilization as a microbial strategy for promoting plant growth. Cienc. Tecnol. Agropecuaria 15, 101–113.
Berg, G., Köberl, M., Rybakova, D., Müller, H., Grosch, R., and Smalla, K. (2017). Plant microbial diversity is suggested as the key to future biocontrol and health trends. FEMS Microbiol. Ecol. 93:fix050. doi: 10.1093/femsec/fix050
Bhattarai, A., Bhattarai, B., and Pandey, S. (2015). Variation of soil microbial population in different soil horizons. J. Microbiol. Exp. 2, 75–78. doi: 10.15406/jmen.2015.02.00044
Bodelier, P. (2011). Toward understanding, managing, and protecting microbial ecosystems. Front. Microbiol. 2:80. doi: 10.3389/fmicb.2011.00080
Bolívar-Anillo, H. J., Contreras-Zentella, M. L., and Teherán-Sierra, L. G. (2016). Burkholderia tropica una bacteria con gran potencial para su uso en la agricultura. TIP 19, 102–108. doi: 10.1016/j.recqb.2016.06.003
Bussas, V., Sharma, A., and Shouche, Y. (2017). “IP and the Budapest Treatydepositing biological material for patent purposes,” in Microbial Resources, ed I. Kurtböke (London: Academic Press), 275–292.
Caktu, K., and Turkoglu, E. A. (2011). Microbial culture collections: the essential resources for life. Gazi Univ. J. Sci. 24, 175–180.
Cano, A., Vélez, D., and Morgado, C. A. (2017). The role of biotechnology in agricultural production and food supply. Cien. Inv. Agr. 44, 1–11. doi: 10.7764/rcia.v44i1.1567
Cassán, F. D., Vanderleyden, J., and Spaepen, S. (2014). Physiological and agronomical aspects of phytohormone production by model plant growth-promoting rhizobacteria (PGPR) belonging to the genus Azospirillum. J. Plant. Growth Regul. 33, 440–459. doi: 10.1007/s00344-013-9362-4
CGRFA (2007). Recursos Genéticos Microbianos en el Simposio de Recursos Genéticos Para América Latina y el Caribe Contribución de Uruguay. Available onlie at: http://www.fao.org/3/a-k0103e.pdf (accessed November 21, 2020).
Chandini, Kumar, R., Kumar, R., and Prakash, O. (2019). “Chapter-5: the impact of chemical fertilizers on our environment and ecosystem,” in Research Trends in Environmental Sciences, 2nd Edn., ed P. Sharma (New Delhi: AkiNik Publications), 69–86.
Chávez-Díaz, I. F., Molina, L. X. Z., Cárdenas, C. I. C., Anaya, E. R., Ramírez, S. R., and de los Santos-Villalobos, S. (2020). Consideraciones sobre el uso de biofertilizantes como alternativa agro-biotecnológica sostenible para la seguridad alimentaria en México. Rev. Mex. Cienc. Agríc. 11, 1423–1436. doi: 10.29312/remexca.v11i6.2492
Choudhary, D. K., Kasotia, A., Jain, S., Vaishnav, A., Kumari, S., Sharma, K. P., et al. (2016). Bacterial-mediated tolerance and resistance to plants under abiotic and biotic stresses. J. Plant Growth Regul. 35, 276–300. doi: 10.1007/s00344-015-9521-x
Ciancio, A., Pieterse, C. M. J., and Mercado-Blanco, J. (2016). Editorial: harnessing useful rhizosphere microorganisms for pathogen and pest biocontrol. Front. Microbiol. 7:1620. doi: 10.3389/fmicb.2016.01620
Compant, S., Samad, A., Faist, H., and Sessitsch, A. (2019). A review on the plant microbiome: Ecology, functions, and emerging trends in microbial application. J. Adv. Res. 19, 29–37. doi: 10.1016/j.jare.2019.03.004
Convention on Biological Diversity (2015). About the Nagoya Protocol. UNO Environment Programme. Available online at: https://www.cbd.int/abs/about/ (accessed August 17, 2020).
Cruz-Leyva, M. C., Zamudio-Maya, M., Corona-Cruz, A. I., González-de la Cruz, J. U., and Rojas-Herrera, R. A. (2015). Importancia y estudios de las comunidades microbianas en los recursos y productos pesqueros. Ecosist. Recur. Agropecuarios 2, 99–115.
de los Santos Villalobos, S., Robles Montoya, R. I., Parra Cota, F. I., Larsen, J., Lozano, P., and Tiedje, J. M. (2019). Bacillus cabrialesii sp. nov., an endophytic plant growth promoting bacterium isolated from wheat (Triticum turgidum subsp. durum) in the Yaqui Valley, Mexico. Int. J. Syst. Evol. Microbiol. 69, 3939–3945. doi: 10.1099/ijsem.0.003711
de los Santos-Villalobos, S., Parra-Cota, F. I., Herrera-Sepúlveda, A., Valenzuela-Aragón, B., and Estrada-Mora, J. (2018). Colmena: colección de microorganismos edáficos y endófitos nativos, para contribuir a la seguridad alimentaria nacional. Rev. Mex. Cienc. Agríc. 9, 191–202. doi: 10.29312/remexca.v9i1.858
Delgado-Baquerizo, M., Maestre, F. T., Reich, P. B., Jeffries, T. C., Gaitan, J. J., Encinar, D., et al. (2016). Microbial diversity drives multifunctionality in terrestrial ecosystems. Nat. Commun. 7:10541. doi: 10.1038/ncomms10541
Devi, K. A., Pandey, P., and Sharma, G. D. (2016). Plant growth-promoting endophyte serratia marcescens AL2-16 enhances the growth of Achyranthes aspera L., a medicinal plant. Hayati J. Biosci. 23, 173–180. doi: 10.1016/j.hjb.2016.12.006
Díaz-Rodríguez, A., Ibarra-Villareal, A., de los Santos-Villalobos, S., Parra-Cota, F. I., Herrera-Sepúlveda, A., and Soto-Padilla, M. Y. (2017). Potencial biotecnológico y ambiental de los microorganismos. La Soc. Acad. 1, 52–63.
Dubey, R., Tripathi, V., Edrisi, S., Bakshi, M., Dubey, P., Singh, A., et al. (2017). “Role of plant growth-promoting microorganisms in sustainable agriculture and environmental remediation,” in Advances in PGPR Research, eds H. B. Singh, B. K. Sarma, C. Keswani (Wallingford: CABI), 75–124.
Egamberdieva, D., Berg, G., Lindström, K., and Räsänen, L. A. (2013). Alleviation of salt stress of symbiotic Galega officinalis L. (goat's rue) by co-inoculation of Rhizobium with root-colonizing Pseudomonas. Plant Soil. 369, 453–465. doi: 10.1007/s11104-013-1586-3
ELD (2015). Report for Policy and Decision Makers: Reaping Economic and Environmental Benefits from Sustainable Land Management. Economics of Land Degradation (ELD) Initiative, Bonn, Germany. Available online at: https://www.eld-initiative.org/ (accessed September 4, 2020).
Escobar, L. P. B., Ríos, D. M. F., Gómez, M. O., and Franco, M. (2016). Colección de cepas microbianas usadas en formación y proyectos de investigación aplicada en el centro para la formación cafetera SENA Regional Caldas. Rev. Nova. 2, 8–15. doi: 10.23850/25004476.615
Etcheverry, M. G., Scandolara, A., Nesci, A., Ribeiro, M. S., Pereira, P., and Battilani, P. (2009). Biological interactions to select biocontrol agents against toxigenic strains of Aspergillus flavus and Fusarium verticillioides from maize. Mycopathologia 167, 287–295. doi: 10.1007/s11046-008-9177-1
FAO (2015a). Los Suelos Están en Peligro, Pero la Degradación Puede Revertirse. Available online at: http://www.fao.org/news/story/es/item/357165/icode/ (accessed August 17, 2020).
FAO (2015b). Healthy Soils are the Basis for Healthy Food Production. Available online at: http://www.fao.org/soils-2015/news/news-detail/es/c/277721/#:$\sim$:text=Los%20suelos%20sanos%20producen%20cultivos,las%20personas%20y%20a%20los%20animales.&text$=$Los%20suelos%20proporcionan%20los%20nutrientes,necesitan%20para%20crecer%20y%20florecer (accessed August 17, 2020).
FAO (2017a). Sustainable Development Goal 2. End hunger, achieve food security and improved nutrition and promote sustainable agriculture. Available online at: http://www.fao.org/sustainable-development-goals/goals/goal-2/en/ (accessed August 17, 2020).
FAO (2017b). The Future of Food and Agriculture - Trends and Challenges. Available online at: http://www.fao.org/3/a-i6583e.pdf (accessed August 17, 2020).
FAO (2017c). Towards Zero Hunger and Sustainability. The FAO Multipartner Programme Support Mechanism. Available online at: http://www.fao.org/documents/card/es/c/fa6a801c-5bd4-4522-a2ff-bfbef1e56529/ (Accessed August 17, 2020).
FAO (2017d). Water Pollution from Agriculture: A Global Review. Available online at: http://www.fao.org/3/a-i7754e.pdf (accessed August 17, 2020).
FAO (2019). FAOSTAT. Crops. Food and Agriculture Organization of the United Nations. Available online at: https://www.fao.org/faostat/en/#data/QC/visualize (Accessed August 17, 2020).
FAO (2020). The Contribution of Agriculture to Greenhouse Gas Emissions. Environment Statistics. Available online at: http://www.fao.org/economic/ess/environment/data/emission-shares/en/ (accessed August 17, 2020).
Federación mundial de Colecciones de Cultivo (2010). Recomendaciones Para el Establecimiento Y Funcionamiento de Colecciones de Cultivos de Microorganismos. Available online at: http://www.wfcc.info/pdf/Guia_WFCC_espa_ol.pdf (accessed September 4, 2020).
Fernández-González, A. J., Martínez-Hidalgo, P., and Cobo-Díaz, J. F. (2017). The rhizosphere microbiome of burned holm-oak: potential role of the genus Arthrobacter in the recovery of burned soils. Sci. Rep. 7:6008. doi: 10.1038/s41598-017-06112-3
Foley, J. A. (2011). Can we feed the world and sustain the planet? Sci. Am. 305, 60–65. doi: 10.1038/scientificamerican1111-60
Gangwar, M., Saini, P., Nikhanj, P., and Kaur, S. (2017). “Plant growth-promoting microbes (PGPM) as potential microbial bio-agents for eco-friendly agriculture,” in Advances in Soil Microbiology: Recent Trends and Future Prospects, Vol. 4, Microorganisms for Sustainability, eds T. Adhya, B. Mishra, K. Annapurna, D. Verma, U. Kumar (Singapore: Springer), 37–55.
Gliessman, S. R. (2002). Agroecología: Procesos Ecológicos en Agricultura Sostenible. Turrialba: CATIE.
Godfray, H. C., Beddington, J. R., Crute, I. R., Haddad, L., Lawrence, D., Muir, J. F., et al. (2010). Food security: the challenge of feeding 9 billion people. Science 327, 812–818. doi: 10.1126/science.1185383
González, D., and Jiménez, J. (2013). Microbial collections: importance, establishment and regulation. Hechos Microbiol. 4, 23–33.
Gougoulias, C., Clark, J. M., and Shaw, L. J. (2014). The role of soil microbes in the global carbon cycle: tracking the below-ground microbial processing of plant-derived carbon for manipulating carbon dynamics in agricultural systems. J. Sci. Food Agric. 94, 2362–2371. doi: 10.1002/jsfa.6577
Gupta, G., Parihar, S., Kumar, N., Kumar, S., and Singh, V. (2015). Plant growth promoting rhizobacteria (PGPR): current and future prospects for development of sustainable agriculture. J. Microbial Biochem. Technol. 2, 96–102. doi: 10.4172/1948-5948.1000188
Gupta, S., Sharma, D., and Gupta, M. (2018). “Climate change impact on plant diseases: opinion, trends and mitigation strategies,” in Microbes for Climate Resilient Agriculture, eds P. L. Kashyap, A. K. Srivastava, S. P. Tiwari, and S. Kumar (Hoboken: John Wiley & Sons, Inc.), 41–56.
Hartemink, A. E. (2016). The definition of soil since the early 1800s. Adv. Agron. 137, 73–126. doi: 10.1016/bs.agron.2015.12.001
Hirel, B., Tétu, T., Lea, P. J., and Dubois, F. (2011). Improving nitrogen use efficiency in crops for sustainable agriculture. Sustainability 3, 1452–1485. doi: 10.3390/su3091452
Ibarra-Villarreal, A. L., Gándara-Ledezma, A., Godoy-Flores, A. D., Herrera-Sepúlveda, A., Díaz-Rodríguez, A. M., Parra-Cota, F. I., et al. (2021). Salt-tolerant Bacillus species as a promising strategy to mitigate the salinity stress in wheat (Triticum turgidum subsp. durum). J. Arid Environ. 186:104399. doi: 10.1016/j.jaridenv.2020.104399
International Atomic Energy Agency (IAEA) (2014). Guidelines for Using Fallout Radionuclides to Assess Erosion and Effectiveness of Soil Conservation Strategies. Vienna: IAEA.
International Atomic Energy Agency (IAEA) (2015). “Un terreno estable: técnicas nucleares para frenar la erosión del suelo en Vietnam,” in Átomos Para la Paz y el Desarrollo. Edición Especial del Boletín del OIEA Sobre los Usos Pacíficos de la Tecnología Nuclear, ed M. Gaspar (México: Organismo Internacional de Energía Atómica), 14–15.
Jin, Y., Zhu, H., Luo, S., Yang, W., Zhang, L., Li, S., et al. (2019). Role of maize root exudates in promotion of colonization of Bacillus velezensis strain S3-1 in rhizosphere soil and root tissue. Curr. Microbiol. 76, 855–862. doi: 10.1007/s00284-019-01699-4
Johansson, J. F., Paul, L. R., and Finlay, R. D. (2004). Microbial interactions in the mycorrhizosphere and their significance for sustainable agriculture. FEMS Microbiol. Ecol. 48, 1–13. doi: 10.1016/j.femsec.2003.11.012
Kalia, A., and Gupta, R. P. (2005). Conservation and utilization of microbial diversity. NBA Sci. Bull. 1, 1–40.
Kaur, J., Pandove, G., and Gangwar, M. (2018). Mitigating the impact of climate change by use of microbial inoculants. J. Pharm. Innov. 7, 279–288.
Kaushik, S., and Djiwanti, S. R. (2019). “Nanofertilizers: smart delivery of plant nutrients,” in Nanotechnology for Agriculture: Crop Production and Protection, eds D. G. Panpatte and Y. K. Jhala (Singapore: Springer), 59–72. doi: 10.1007/978-981-32-9374-8_3
Köhl, J., Kolnaar, R., and Ravensberg, W. J. (2019). Mode of action of microbial biological control agents against plant diseases: relevance beyond efficacy. Front. Plant Sci. 19:845. doi: 10.3389/fpls.2019.00845
Kopittke, P. M., Menzies, N. W., Wang, P., McKenna, B. A., and Lombi, E. (2019). Soil and the intensification of agriculture for global food security. Environ. Int. 132:105078. doi: 10.1016/j.envint.2019.105078
Kurtzman, C. P., and Labeda, D. P. (2009). “Type culture collections and their databases,” in Encyclopedia of Microbiology, ed M. Schaechter (Oxford: Elsevier), 306–312.
Lal, R. (2015). Restoring soil quality to mitigate soil degradation. Sustainability 7, 5875–5895. doi: 10.3390/su7055875
Lalaymia, I., Cranenbrouck, S., and Declerck, S. (2013). Maintenance and preservation of ectomycorrhizal and arbuscular mycorrhizal fungi. Mycorrhiza 24, 323–337. doi: 10.1007/s00572-013-0541-8
Lara, C., and Negrete, J. L. (2015). Efecto de un bioinoculante a partir de consorcios microbianos nativos fosfato solubilizadores, en el desarrollo de pastos Angleton (Dichantium aristatum). Rev. Colomb. Biotecnol. 17, 122–130. doi: 10.15446/rev.colomb.biote.v17n1.50741
Leahy, S. (2019). By 2050, Many U.S. Cities Will Have Weather Like They've Never Seen. National Geographic. Available online at: https://www.nationalgeographic.com/environment/2019/07/major-us-cities-will-face-unprecedente-climates-2050/ (accessed August 17, 2020).
Leal-Almanza, J., Gutiérrez-Coronado, M. A., Castro-Espinoza, L., Lares-Villa, F., Cortés-Jiménez, J. M., and de los Santos-Villalobos, S. (2018). Vegetable growth promoter microorganisms with agricultural plaster on potatoes (Solanum tuberosum L.) under shadow housing. Agrociencia 52, 1149–1159.
Mahilum, L. (2009). “The importance of microbial culture collection and gene banks in biotechnology,” in Biotechnology, eds H. W. Doelle, J. S. Rokem, and M. Berovic (Paris: Encyclopedia of Life Support Systems), 227–238.
Malik, K. A., and Claus, D. (1987). Bacterial culture collections: their importance to biotechnology and microbiology. Biotechnol. Genet. Eng. 5, 137–198. doi: 10.1080/02648725.1987.10647837
Mall, R. K., Gupta, A., and Sonkar, G. (2017). “Effect of climate change on agricultural crops: current developments,” in Biotechnology and Bioengineering Crop Modification, Nutrition, and Food Production, eds S. Dubey, A. Pandey, and R. Sangwa (Amsterdam: Elsevier), 23–46.
Marks, B. B., Megías, M., Nogueira, M. A., and Hungria, M. (2013). Biotechnological potential of rhizobial metabolites to enhance the performance of Bradyrhizobium spp. and Azospirillum brasilense inoculants with soybean and maize. AMB Express 3:21. doi: 10.1186/2191-0855-3-21
Martínez-Castillo, R. (2016). Sustainable agricultural production systems. Tecn. Marcha 29, 70–85. doi: 10.18845/tm.v29i5.2518
Martínez-Viveros, O., Jorquera, M. A., Crowley, D. E., Gajardo, G., and Mora, M. L. (2010). Mechanisms and practical considerations involved in plant growth promotion by rhizobacteria. J. Soil Sci. Plant Nutr. 10, 293–319. doi: 10.4067/S0718-95162010000100006
Matson, P. A. (2012). Seeds of Sustainability Lessons from the Birthplace of the Green Revolution. Washington, DC: Island Press.
Maura, A. V., and Febles, J. M. (2018). Una aproximación a los costos ambientales en los suelos ferralíticos rojos para el logro de la sostenibilidad. COFIN 12, 192–208.
Mishra, J., Singh, R., and Arora, N. K. (2017). “Plant growth-promoting microbes: diverse roles in agriculture and environmental sustainability,” in Probiotics and Plant Health, eds V. Kumar, M. Kumar, S. Sharma, and R. Prasad (Singapore: Springer), 71–111.
Moreno, A., García, V., Reyes, J. L., Vásquez, J., and Cano, P. (2018). Rizobacterias promotoras del crecimiento vegetal: una alternativa de biofertilización para la agricultura sustentable. Rev. Colomb. Biotecnol. 20, 68–83. doi: 10.15446/rev.colomb.biote.v20n1.73707
Mullen, M. D. (2019). “Phosphorus in soils-biological interactions,” in Reference Module in Earth Systems and Environmental Sciences, ed S. Elias (Amsterdam: Elsevier), 1–8.
Nadeem, S. M., Ahmad, M., Zahir, Z. A., Javaid, A., and Ashraf, M. (2014). The role of mycorrhizae and plant growth promoting rhizobacteria (PGPR) in improving crop productivity under stressful environments. Biotechnol. Adv. 32, 429–448. doi: 10.1016/j.biotechadv.2013.12.005
Nanjundappa, A., Bagyaraj, D. J., Saxena, A. K., Kumar, M., and Chakdar, H. (2019). Interaction between arbuscular mycorrhizal fungi and Bacillus spp. in soil enhancing growth of crop plants. Fungal Biol. Biotechnol. 6:23. doi: 10.1186/s40694-019-0086-5
Nannipieri, P., Ascher, J., Ceccherini, M. T., Landi, L., Pietramellara, G., and Renella, G. (2017). Microbial diversity and soil functions. Eur. J. Soil Sci. 68, 12–26. doi: 10.1111/ejss.4_12398
Nath, D., and Meena, V. S. (2018). “Mycorrhizae: a potential microorganism and its implication in agriculture,” in Role of Rhizospheric Microbes in Soil, ed V. Meena (Singapore: Springer), 251–276.
Nelson, G. C., Rosegrant, M. W., Koo, J., Robertson, R., Sulser, T., Zhu, T., et al. (2009). Climate Change: Impact on Agriculture and Costs of Adaptation. Washington, DC: International Food Policy Research Institute Press (IFPRI).
Olanrewaju, O. S., Glick, B. R., and Babalola, O. O. (2017). Mechanisms of action of plant growth promoting bacteria. World J. Microbiol. Biot. 33:197. doi: 10.1007/s11274-017-2364-9
Organización Mundial de la Propiedad Intelectual (OMPI) (2017). Tratado de Budapest Sobre el Reconocimiento Internacional del Depósito de Microorganismos a Los Fines del Procedimiento en Materia de Patentes. Available online at: https://www.wipo.int/treaties/es/text.jsp?file_id=283785 (accessed August 17, 2020).
Pajares, S., and Bohannan, B. J. M. (2016). Ecology of nitrogen fixing, nitrifying, and denitrifying microorganisms in tropical forest soils. Front. Microbiol. 7:1045. doi: 10.3389/fmicb.2016.01045
Pajares, S., Bohannan, B. J. M., and Souza, V. (2016). Editorial: the role of microbial communities in tropical ecosystems. Front. Microbiol. 7:1805. doi: 10.3389/fmicb.2016.01805
Parewa, H. P., Meena, V. S., Jain, L. K., and Choudhary, A. (2018). “Sustainable crop production and soil health management through plant growth-promoting rhizobacteria,” in Role of Rhizospheric Microbes in Soil, ed V. Meena (Singapore: Springer), 299–329. doi: 10.1007/978-981-10-8402-7_12
Patil, H. J., and Solanki, M. K. (2016). “Microbial inoculant: modern era of fertilizers and pesticides,” in Microbial Inoculants in Sustainable Agricultural Productivity, eds D. Singh, H. Singh, and R. Prabha (New Delhi: Springer), 319–343.
Peláez-Álvarez, A., de los Santos-Villalobos, S., Yépez, E., Parra-Cota, F. I., and Reyes-Rodríguez, R. (2016). Synergistic effect of Trichoderma asperelleum T8A and captan 50®against Colletotrichum gloeosporioides (Penz.). Rev. Mex. Cienc. Agríc. 7, 1401–1412. doi: 10.29312/remexca.v7i6.202
Pereira, P., Brevik, E. C., Oliva, M., Estebaranz, F., Depellegrin, D., Novara, A., et al. (2017). “Goal Oriented Soil Mapping,” in Soil Mapping and Process Modeling for Sustainable Land Use Management, eds P. Pereira, E. Brevik, M. Muñoz, and B. Miller (Elsevier), 61–83.
Prabhu, N., Borkar, S., and Garg, S. (2019). “Chapter 11: phosphate solubilization by microorganisms: mechanisms, applications and advances,” in Advances in Biological Science Research, eds S. N. Meena and M. M. Naik (London: Elsevier), 161–176.
Puente, M. L., Gualpa, J. L., Lopez, G. A., Molina, R. M., Carletti, S. M., and Cassán, F. D. (2018). The benefits of foliar inoculation with Azospirillum brasilense in soybean are explained by an auxin signaling model. Symbiosis 76, 41–49. doi: 10.1007/s13199-017-0536-x
Puente, M. L., Zawoznik, M., de Sabando, M. L., Perez, G., Gualpa, J. L., Carletti, S. M., et al. (2019). Improvement of soybean grain nutritional quality under foliar inoculation with Azospirillum brasilense strain Az39. Symbiosis 77, 41–47. doi: 10.1007/s13199-018-0568-x
Rokhzadi, A., and Toashih, V. (2011). Nutrient uptake and yield of chickpea (Cicer arietinum L.) inoculated with plant growth promoting rhizobacteria. Aust. J. Crop Sci. 5, 44–48.
Saccá, M. L., Barra Caracciolo, A., Di Lenola, M., and Grenni, P. (2017). “Ecosystem services provided by soil microorganisms,” in Soil Biological Communities and Ecosystem Resilience, eds M. Lukac, P. Grenni, and M. Gamboni (Cham: Springer), 9–24.
Santoyo, G., Sánchez-Yáñez, M., and de los Santos-Villalobos, S. (2019). “Methods for detecting biocontrol and plant growth-promoting traits in rhizobacteria,” in Biology Research, eds D. Reinhardt and A. K. Sharma (Singapore: Springer), 133–149.
Sharip, Z., Schooler, S. S., Hipsey, M. R., and Hobbs, R. J. (2012). Eutrophication, agriculture and water level control shift aquatic plant communities from floating-leaved to submerged macrophytes in Lake Chini, Malaysia. Biol. Invasions 14, 1029–1044. doi: 10.1007/s10530-011-0137-1
Sharma, A., and Shouche, Y. (2014). Microbial culture collection (MCC) and international depositary authority (IDA) at national centre for cell science, Pune. Indian J. Microbiol. 54, 129–133. doi: 10.1007/s12088-014-0447-y
Sharma, S. K., Saini, S., Verma, A., Sharma, P. K., Lal, R., Roy, M., et al. (2017). National agriculturally important microbial culture collection in the global context of microbial culture collection centres. Proc. Natl. Acad. Sci. India Sect. B. Biol. Sci. 89, 405–418. doi: 10.1007/s40011-017-0882-8
Singh, M., Singh, D., Gupta, A., Pandey, K. D., Singh, P. K., and Kumar, A. (2019). “Plant growth promoting rhizobacteria,” in PGPR Amelioration in Sustainable Agriculture, eds A. Kishore, A. Kumar, and P. Kumar (Amsterdam: Woodhead Publishing), 41–66.
Singh, R. P., and Jha, P. N. (2016). The multifarious PGPR Serratia marcescens CDP-13 augments induced systemic resistance and enhanced salinity tolerance of wheat (Triticum aestivum L.). PLoS One 11:e0155026. doi: 10.1371/journal.pone.0155026
Smith, D. (1996). “Quality systems for management of microbial collections,” in Culture Collections to Improve the Quality of Life, eds R. A. Samson, J. A. Stalpers, D. van der Mei, and A. H. Stouthamer (Netherlands: Centraalbureau voor Schimmelcultures), 137–143.
Smith, D. (2003). Culture collections over the world. Int. Microbiol. 6, 95–100. doi: 10.1007/s10123-003-0114-3
Smith, D., McCluskey, K., and Stackebrandt, E. (2014). Investment into the future of microbial resources: culture collection funding models and BRC business plans for biological resource centres. SpringerPlus 3:81. doi: 10.1186/2193-1801-3-81
Struik, P. C., and Kuyper, T. W. (2017). Sustainable intensification in agriculture: the richer shade of green. A review. Agron. Sustain. Dev. 37:39. doi: 10.1007/s13593-017-0445-7
Suliasih, S., and Widawati, S. (2019). The application of Klebsiella sp. and Rhizobium radiobacter as biofertilizer and Palm Oil Mills Effluent (POME) as organic fertilizer on growth of Paraserianthes falcataria. IOP Conf. Ser. Earth Environ. Sci. 308:012057. doi: 10.1088/1755-1315/308/1/012057
Sunding, D., and Zilberman, D. (2001). “Chapter 4: The agricultural innovation process: Research and technology adoption in a changing agricultural sector,” in Handbook of Agricultural Economics, eds B. L. Gardner and G. C. Rausser (Elsevier), 207–261.
Tchameni, S. N., Ngonkeu, M. E. L., Begoude, B. A. D., Nana, L. W., Fokom, R., Owona, A. D., et al. (2011). Effect of Trichoderma asperellum and arbuscular mycorrhizal fungi on cacao growth and resistance against black pod disease. Crop Prot. 30, 1321–1327. doi: 10.1016/j.cropro.2011.05.003
Thilagar, G., Bagyaraj, D. J., and Rao, M. S. (2016). Selected microbial consortia developed for chilly reduces application of chemical fertilizers by 50% under field conditions. Sci. Hortic. 198, 27–35. doi: 10.1016/j.scienta.2015.11.021
Thomson, L. J., Macfadyen, S., and Hoffmann, A. (2010). Predicting the effects of climate change on natural enemies of agricultural pests. Biol. Control 52, 296–306. doi: 10.1016/j.biocontrol.2009.01.022
Timmusk, S., Behers, L., Muthoni, J., Muraya, A., and Aronsson, A.-C. (2017). Perspectives and challenges of microbial application for crop improvement. Front. Plant Sci. 8:49. doi: 10.3389/fpls.2017.00049
Toader, G., Chiurciu, C., Burnichi, F., Petre, C., Chi?onu, P., Maierean, N., et al. (2019). Research on the action of fertilizers based on bacterial cultures on some agricultural crops in Romania. Lucrări Ştiinţifice Manag. Agricol. 21, 71–76.
Tsiafouli, M. A., Thébault, E., Sgardelis, S. P., De Ruiter, P. C., Van Der Putten, W. H., Birkhofer, K., et al. (2015). Intensive agriculture reduces soil biodiversity across Europe. Glob. Change Biol. 21, 973–985. doi: 10.1111/gcb.12752
Umesha, S. K., Singh, P., and Singh, R. (2018). “Microbial biotechnology and sustainable agriculture,” in Biotechnology for Sustainable Agriculture, eds R. L. Singh and S. Mondal (Amsterdam: Woodhead Publishing), 185–205.
Valenzuela-Aragon, B., Parra-Cota, F. I., Santoyo, G., Arellano-Wattenbarger, G. L., and de los Santos-Villalobos, S. (2018). Plant-assisted selection: a promising alternative for in vivo identification of wheat (Triticum turgidum L. subsp. Durum) growth promoting bacteria. Plant soil 435, 367–384. doi: 10.1007/s11104-018-03901-1
Valenzuela-Ruiz, V., Ayala-Zepeda, M., Arellano-Wattenbarger, G. L., Parra-Cota, F. I., García-Pereyra, G., Aviña-Martínez, G. N., et al. (2018). Las colecciones microbianas y su potencial contribución a la seguridad alimentaria actual y futura. Rev. Latinoam. Recur. Nat. 14, 18–25.
Villarreal-Delgado, M. F., Villa-Rodríguez, E. D., Cira-Chávez, L. A., Estrada-Alvarado, M. I., Parra-Cota, F. I., and de los Santos-Villalobos, S. (2018). The genus Bacillus as a biological control agent and its implications in agricultural biosecurity. Mex. J. Phytopathol. 36, 95–130. doi: 10.18781/R.MEX.FIT.1706-5
WDCM (2019). Reference Strain Catalogue Pertaining to Organisms for Performance Testing Culture Media. 29th version. Available online at: http://refs.wdcm.org/pdf/WDCM%20Catalogue%20Version_V29.pdf (accessed August 17, 2020).
WDCM (2020a). Statistics. Available online at: http://www.wfcc.info/ccinfo/statistics/ (accessed November 21, 2020).
WDCM (2020b). Culture Collection Information Worldwide. Available online at: http://www.wfcc.info/ccinfo/collection (accessed August 17, 2020).
Weng, Z., Esther, O., and Molina, Á. (2005). Conservación de microorganismos:'Qué debemos conocer? Rev. Cubana Hig. Epidemiol. 43, 1–5.
Wheeler, T., and Von Braun, J. (2013). Climate change impacts on global food security. Science 341, 508–513. doi: 10.1126/science.1239402
Wood, S. A., Almaraz, M., Bradford, M. A., McGuire, K. L., Naeem, S., Neill, C., et al. (2015). Farm management, not soil microbial diversity, controls nutrient loss from smallholder tropical agriculture. Front. Microbiol. 6:90. doi: 10.3389/fmicb.2015.00090
Wu, L., Sun, Q., Desmeth, P., Sugawara, H., Xu, Z., McCluskey, K., et al. (2016). World data centre for microorganisms: an information infrastructure to explore and utilize preserved microbial strains worldwide. Nucleic Acids Res. 45, 611–618. doi: 10.1093/nar/gkw903
Yobo, K. S., Laing, M. D., and Hunter, C. H. (2011). Effects of single and combined inoculations of selected Trichoderma and Bacillus isolates on growth of dry bean and biological control of Rhizoctonia solani damping-off. Afr. J. Biotechnol. 10, 8746–8756. doi: 10.5897/AJB10.2213
Keywords: agriculture, biological control agents (BCAs), microbial inoculants, sustainability, climate chage, plant growth-promoting microorganisms (PGPM)
Citation: Díaz-Rodríguez AM, Salcedo Gastelum LA, Félix Pablos CM, Parra-Cota FI, Santoyo G, Puente ML, Bhattacharya D, Mukherjee J and de los Santos-Villalobos S (2021) The Current and Future Role of Microbial Culture Collections in Food Security Worldwide. Front. Sustain. Food Syst. 4:614739. doi: 10.3389/fsufs.2020.614739
Received: 07 October 2020; Accepted: 09 December 2020;
Published: 14 January 2021.
Edited by:
Everlon Cid Rigobelo, Universidade Estadual Paulista, BrazilReviewed by:
Eleonora Egidi, Western Sydney University, AustraliaSenga Robertson-Albertyn, University of Dundee, United Kingdom
Copyright © 2021 Díaz-Rodríguez, Salcedo Gastelum, Félix Pablos, Parra-Cota, Santoyo, Puente, Bhattacharya, Mukherjee and de los Santos-Villalobos. This is an open-access article distributed under the terms of the Creative Commons Attribution License (CC BY). The use, distribution or reproduction in other forums is permitted, provided the original author(s) and the copyright owner(s) are credited and that the original publication in this journal is cited, in accordance with accepted academic practice. No use, distribution or reproduction is permitted which does not comply with these terms.
*Correspondence: Sergio de los Santos-Villalobos, sergio.delossantos@itson.edu.mx
†These authors have contributed equally to this work