- 1Department of Grass and Forage Science/Organic Agriculture, Christian-Albrechts-University, Kiel, Germany
- 2Department of Agroecology, Aarhus University, Tjele, Denmark
- 3Grass Based Dairy Systems, Animal Production Systems Group, Wageningen University (WUR), Wageningen, Netherlands
Intensive confinement (IC) systems for dairying have become widespread during the last decades. However, potential advantages of alternative systems such as full-grazing (FG) or integrated dairy/cash-crop (IFG) systems with regards to better provision of ecosystem services are widely discussed. To investigate performance and environmental impacts, we compared four prevailing dairy systems using an on-farm research study. The farm types differed in their share of pasture access and quantity of resource inputs: (i) an IC with a high import of supplements and mineral fertilizers; (ii) a semi-confinement (SC) with daytime pasture access during summer and moderate import of supplementary feeds representing the base-line scenario; (iii) a FG based on grazed seeded grass-clover swards with no purchased N-fertilizers and low quantities of supplementary feeds; and (iv) an IFG comparable to FG but based on grass-clover leys integrated in a cash-crop rotation. Results revealed highest milk productivity (16 t energy-corrected-milk (ECM) ha−1) and farm-N-balance (230 kg N ha−1) in IC; however, the highest product carbon footprint (PCF; 1.2 CO2eq kg ECM−1) and highest N-footprint (13 g N kg ECM−1) were found in the baseline system SC. The FG and IFG revealed on average similar forage dry matter yields (10 – 11 t DM ha−1) at similar crude protein and net-energy-lactation ratios per kg DM-intake compared to the IC and SC. The PCF in FG were comparable to IC (0.9 vs. 1.1 kg CO2eq kg ECM−1) but at a lower N-footprint (9 vs. 12 g N kg ECM−1). However, despite low measured N-losses in the FG system, the farm-N-surplus was exceeded by 90 kg N ha−1. A further reduction was only possible in the IFG (50 kg N ha−1) by accounting for a potential N-carry-over from N-rich plant residues to the cash-crop unit, leading to the lowest PCF (0.6 kg CO2eq kg ECM−1) for the IFG, with still moderate milk yield levels (~10,500 kg ECM ha−1). According to this bottom-up approach based on field data, improved integrated grazing systems could provide an important opportunity to increase the ecosystem services from dairy farming, operating with land use efficiencies similar to IC.
Introduction
Ongoing intensification in agriculture has led in many developed countries worldwide to highly specialized dairy production systems, with declining numbers of farms, larger herd sizes, and increasing milk yields per hectare (Peyraud et al., 2014). In recent years, the spatial distribution of dairy farms has continued to shift into areas with lower land prices and unsuitable conditions for arable crop production, or regions that continue to specialize on animal husbandry due to the poor competition with other cash crop producers on the global market. For instance, in the European Union (EU) half of the livestock units are located on one-third of the agricultural area (Leterme et al., 2019). In the South Island Regions of New Zealand, where historically there were significant shares of oat and wheat production together with animal husbandry, the arable area for cash-crop production declined by 80%; at the same time the stocking rates increased by 150%. This was enabled by converting rain fed to irrigated grassland systems, which are now mainly used for dairying (Ledgard, 2013). As a result, the diversity of agricultural commodities produced on farms in these areas declined and undesired environmental impacts increased, including increases in nitrate concentrations in drinking water (Vogeler et al., 2014), and in ammonia (NH3) volatilization (Fowler et al., 2013), as well as loss of plant, insect and bird species diversity (Kleijn et al., 2009; Ledgard, 2013; Allan et al., 2014), and a decline in natural forest (Ledgard, 2013).
The concept of sustainable intensification has evolved as a response to the environmental challenges associated with agriculture (Davies et al., 2009) and has been a topic of interest in recent years (Garnett et al., 2013; Barnes et al., 2016; Reheul et al., 2017; Struik and Kuyper, 2017). Sustainable intensification involves simultaneously improving the productivity and environmental management of agricultural land (Buckwell, 2014). Closely linked to sustainable intensification is the concept of resource-use efficiency or eco-efficiency (Keating et al., 2010; Taube et al., 2014; Tittonell, 2014; Cook et al., 2015), in which the quantity of resource input, environmental loads, or ecosystem services provided is related to the unit of product (Wilkins, 2008). Ecosystem services can be manifold, however, according to the current common agricultural policy (CAP) of the European union (EU), greenhouse gas (GHG) mitigation and nutrient cycling are of foremost importance in order to tackle the most relevant environmental impact categories (i.e., climate change, eutrophication, biodiversity loss). Critical voices question the applicability of sustainable intensification in Europe by arguing that agricultural systems are already operating at a high production intensity and the concept would be an appropriate strategy for regions characterized by a large yield gap, as for instance in developing countries (Mueller et al., 2012). In regions with highly intensive crop and livestock production, however, sustainable intensification would appear less likely to provide benefits in terms of yield progress when considering the high risk of environmental threats (Garnett et al., 2013; van Grinsven et al., 2015). Some authors are therefore proposing the term “ecological intensification” instead of “sustainable intensification” for use in OECD countries (Godfray and Garnett, 2014). A study by Schiefer et al. (2016), classifying soil biochemical and physical properties as indicators of soil resilience, found only 40% of soils in the European Union (EU-25) to be suitable for sustainable intensification, while on the remaining land de-intensification or conversion from arable to grassland may be warranted. In this context, the term “sustainable extensification” was coined (Bluwstein et al., 2015; van Grinsven et al., 2015).
As one strategy toward ecological intensification, several authors have recommended a paradigm change from highly specialized production systems back to integrated crop livestock systems (ICLS) in order to increase diversity of land use and resource efficiency (Rockström et al., 2009; Godfray and Garnett, 2014). Different levels of ICLS production are currently discussed: (i) integration of crop and animal production by exchanging materials, (ii) complementary exchange of materials with each system taking the production requirements of the cooperatives into account, (iii) temporal and spatial integration on farm-level using fully or partly the same territory, and (iv) the extrapolation of (v) on the regional level (Moraine et al., 2014). A meta-analysis conducted by Peterson et al. (2020) covering data from Australia, North America and South America found that ICLS systems provide higher cash crop yields on fertile sandy loamy soils in comparison to non-integrated systems. This effect was, however, lower, if dual-purpose crops and other soil types were considered. According to Ryschawy et al. (2012) the economic benefit of ICLS depends on the production level and management, as a wide range of management options exists in comparison with more specialized systems. The majority of studies indicated positive environmental effects of ICLS systems (Ryschawy et al., 2012; Moraine et al., 2014; Peterson et al., 2020) due to improved C- and N-cycling among the systems (Lemaire et al., 2015) and consequently a lower demand for external resources. Thus, lower N and phosphate surpluses can be attained. Furthermore, most of the studies found a positive effect on soil organic carbon (SOC) with increased rates of C-sequestration and enhanced soil functioning properties. The latter has mainly been observed when grass or grass-clover was included within the crop rotation (Lemaire et al., 2015; Loges et al., 2018a) often referred to as the ley-phase. The increments of soil C stocks increase with the duration of the ley-phase (Lemaire et al., 2015). For leys, grass-clover swards (usually white clover) are most commonly recommended, as the clover as a forage legume provides additional N through biological nitrogen fixation (BNF). Greenhouse gas emissions, in particular those from the potent GHG nitrous oxide (N2O), as well as N-leaching losses, are low from grass-clover leys, even though high amounts of BNF (193–319 kg N ha−1 year−1) can be reached (Høgh-Jensen et al., 2004; Reinsch et al., 2020). This indicates there is an effective N-cycling in such systems (Schmeer et al., 2014; Reinsch et al., 2020). Beyond the mentioned ecosystems services from ley systems, there are additional questions on biodiversity that can be addressed with multispecies swards at low rates of mineral N are used. For instance, Ebeling et al. (2008) found a linear trend for pollinator visits with increasing numbers of flowering plant species. Moreover, the introduction of leys in arable-cropping systems reduces the pressure of undesired weeds (Connolly et al., 2018; MacLaren et al., 2019) and consequently the use of agrochemicals, which may thereby allow a further increases in the biodiversity of agricultural land (Holzschuh et al., 2007). However, even greater biodiversity increase can be expected by increasing crop diversity and from the increased landscape heterogeneity of integrated crop livestock systems (Sirami et al., 2019).
Research on alternative production systems for dairying in northwest Europe currently attracts a lot of attention from policy makers and from society in general, because of the environmental effects of mainstream intensive systems as well as the questionable economic performance of dairying since the abolition of the milk quota system. Moreover, producers are facing the challenge on how to re-direct their systems in order to reach the multidisciplinary aims (e.g., compliance with EU-Nitrate Directive, EU-Water Framework Directive, EU-NEC Directive) and to be in line with the EU climate target plan (Green deal) to become climate friendly by 2050 (EC, 2020). Nowadays, dairy husbandry in northwest Europe is the second largest sector in terms of output value from agriculture (Augère-Granier, 2018). The average stocking rate in the intensive dairy producing countries (Netherlands, Germany and Denmark) is >1.4 LU per ha of UAA (Eurostat, 2018), and average milk yields per cow are 9,247 litre per year (Eurostat, 2020). To maintain high milk yields per ha for the market, silage production and intensive supplementary feeding has increased in the last decades, at the cost of grazing and low-cost feeding (Taube et al., 2014). Additionally, high amounts of mineral fertilizers are purchased to maintain high forage yields and yield stability, despite high amounts of organic manures being available. Consequently, the total amounts of nutrient supplies from organic manures and purchased fertilizers on dairy farms often exceed the demand requirements for on-farm forage production, particularly from seasonal surpluses of manure. This necessitates a high share of exportation of manures to cash crop producers (Oenema et al., 2014). The N in animal manures also has a high potential for gaseous N-emissions, during both storage and application depending on time and technique used (Misselbrook et al., 2000). The farm-N surplus can exceed 200 kg N ha−1 year−1 particularly if there is substantial supplementary feeding (Akert et al., 2020). van Grinsven et al. (2013) calculated a range of social costs from the impact of reactive N on human health, ecosystems and climate of 10–30, 5–20, and 4–17 € per each kg N emitted in the EU-27 states. As one of the consequences, the European Nitrates Directive restricts the total amount of manure N to 170 kg ha−1 year−1 and ban slurry application from autumn and winter in order to reduce the N-pollution of groundwater bodies. This, in combination with the current spatial distribution of highly specialized systems, has led to logistic challenges, with long transport distances for manures, long manure storage durations, and high capacity manure storage facilities needed (Kuhn et al., 2018). This handling of manures increases the potential for NH3 volatilization, which decreases the N-use efficiency (NUE) of dairy farms to below 30% (Löw et al., 2020). Alternative solutions for dairy farms to use adapted forage crops, such as legumes, are limited as they provide additional N. This biologically fixed N has to be included in fertilizer planning and, as a consequence, more N has to be exported. In addition, farmers are often concerned that considerable yield losses could occur after the adoption of forage legumes, even though several studies have confirmed an effective N-fertilizer replacement value across a wide range of legume-based ley-mixtures (Suter et al., 2015). This, and the high inter-annual yield resilience under current and future climate change make such legume-based ley mixtures even more attractive (Lorenz et al., 2020).
Within the hierarchy of current developments in the dairy sector, there are discussions about whether a trend back to pasture-based production systems would contribute to reduced environmental impacts and more resilient farming systems in northwest Europe (Schils et al., 2019). The revenue of a dairy farm depends on the market price for milk, and the share of home-grown feed and use of external resources, like supplements. Several studies have revealed that the lowest costs per unit dry-matter intake (DM-intake) can be obtained through low-cost full-grazing strategies ensuring grazing almost year-round at a low share of supplementary feeding, as is common in Ireland, Australia and New Zealand. Such grazing strategies are best suited in regions with adequate rainfall and fertile soils, as found in parts of the Netherlands, northwest Germany and Denmark. The lowest feeding cost per liter of milk unit can be generated by a high home-grown forage use efficiency and low rates of supplementary feeding (Dillon et al., 2008). This can be achieved through a high frequency of short grazing intervals (i.e., rotational grazing), as this ensures that forage is offered with high energy and protein contents. Further improvements can be gained by including forage legumes in grass-clover swards, as this reduces costs further, and also can reduce GHG emissions (Li et al., 2011; Nyameasem et al., 2021). In comparison with confinement systems, these low-cost full-grazing strategies need adjustments in the choice of the animal breeds and calving interval. High yielding Holsteins often cannot realize a sufficient dry matter intake under grazing conditions and might be sensitive to variations in the herbage on offer and weather conditions (Heublein et al., 2017). Smaller cows, such as cross-breeds and Jerseys, are often judged as better grazers. Optimal grazing efficiency is often reached by synchronization of high (spring) pasture growth with the peak of lactation. To achieve this, seasonal spring calving with short calving intervals is the predominant calving pattern in pasture-based milk production.
However, such management results in lower productivity per animal, as well as per hectare of farmland as long as “imported land” is not included. This makes the overall environmental efficiency questionable, as it is often highlighted that high milk yields per cow result in a lower product carbon footprint (PCF). A negative relationship between the PCF and milk yield was found in a variety of studies (Christie et al., 2012; O'Brien et al., 2014; Zehetmeier et al., 2014). For example, Christie et al. (2012) explained 64% of the variation of the PCF by milk yield per cow, and Zehetmeier et al. (2014) explained 55% (Holstein Friesian cows) and 30% (Fleckvieh cows) of the milk PCF by the production system. Gerber et al. (2011) found that at energy corrected milk (ECM) yields below 2,000 kg per cow and year, an increase of milk yield provides a strong reduction of emissions per unit product, while above 6,000 kg the emissions stabilize. While the total energy requirement per cow increases with an increase in milk yield, the amount required for maintenance remains unchanged. This results in a decline of the proportion of total energy used for maintenance and the total energy requirement per kg of milk produced (Capper et al., 2009) in a ceteris paribus scenario (e.g., same number of lactations per cow). However, since it is very likely that higher milk performance per cow coincides with a reduced number of lactations, optimum levels of PCF do not increase linearly with milk performance. Considering more intensive systems with milk yields >5,000 kg energy corrected milk (ECM) per cow and year, Lorenz et al. (2019) found in a meta-analysis that low-cost full grazing systems show no disadvantages in the PCF in comparison with intensive confinement systems.
The suitability of ICLS systems for dairy production has, so far, been poorly evaluated on a regional level without taking high production intensity, such as for dairying in northwest Europe, into account. The insertion of leys into the crop rotation will also provide benefits for cash crop producers (e.g., nutrient provision, soil water retention, and control of pests) and also help ensure mutual outputs such as climate regulation and biodiversity, regardless of whether the ley is used for silage production, cut and carry, or grazing (Martin et al., 2020). However, where soil conditions allow grazing as a low resource-use practice the ley-phase should be sought to reach the maximum potential of ICLS.
Thus, as part of the debate on the development of more sustainable dairy systems within the frame of ecological intensification approaches that ensure appropriate production levels on the one hand, and additional ecosystem services on the other, four model scenarios along the hierarchy of current and possible systems in northwest Europe can be defined: intensive-confinement (IC), semi-confinement (SC) with a limited grazing period, full-grazing systems (FG) conducted on seeded permanent grassland, and an integrated full grazing system (IFG) predominately conducted on leys (Figure 1). In accordance with the meta-analysis from Lorenz et al. (2019) the thresholds between IC, SC, and FG systems are set by the forage intake from pasture. In the IC systems animals have no allowance for pasture, while in SC <50% of the feed intake is from pastures and a minimum 25% is from supplementary feeding, whereas in FG and IFG systems a minimum 50% of feed intake is from pastures with a maximum share of 25% from supplements. The main advantages of an IFG is the possibility of the inclusion of leys in a cash-crop arable system to accelerate C and N accumulation in the soil as a result of BNF from grass-clover leys and animal-N excreta; and in terms of forage provision the benefits of continuous progress in forage plant breeding, as expressed as high energy and protein values similar to concentrate feed, can be gained by frequent renovation of the seeded leys. Moreover, leys offer the opportunity to include alternative species such as forage herbs that may benefit the digestibility and forage intake of grazing animals (Loza et al., 2021) at low N2O emissions from pasture land (Nyameasem et al., 2021).
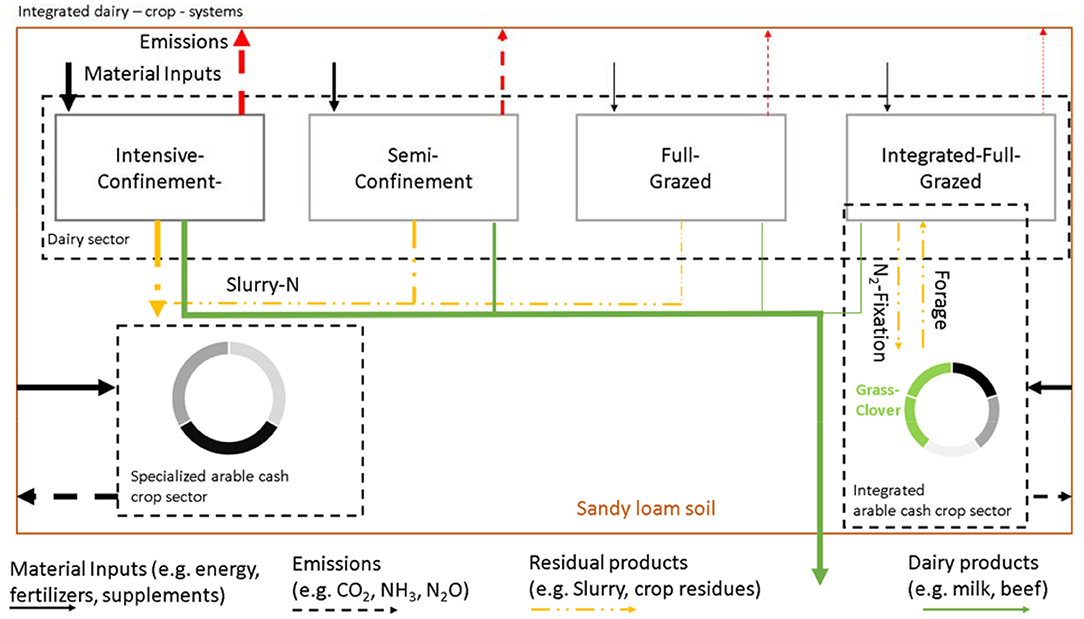
Figure 1. Assumption of input and emission flows within the prevailing dairy systems. The system boundaries indicate the scope of the conducted farm evaluation.
Lorenz et al. (2019), moreover, noticed that the efficiency of the proposed dairy systems depends on the farm management and that the evaluation of such systems needs accurate estimates of the forage efficiency. Accordingly, we decided to choose a set of representative farms together with the regional commercial dairy extension service, which fulfilled the above-mentioned thresholds of feed allowance, and to investigate them over 2 years using a bottom-up approach. Even though on-farm research approaches often lack the statistical accuracy provided in a randomized experimental design, they offer valuable insights into practical management approaches, which may be shown to be economical resilient over successive years. Moreover, to gain a representative picture on the effects on C- and N-cycles, systems have to be established over years before the long-term impacts can be investigated. This situation is difficult to duplicate in randomized plot experiments for dairy systems, as (i) dairy cattle have a large requirement for land, especially if fundamentally different production systems are compared, and (ii) long-term investigation would be necessary in order to take account of altered chemical soil properties that occur as a result of the management. Thus, all farms in our study had already been established for several years and were located on the same soil type. Productivity and environmental impacts such GHG-emissions and N-leaching from forage crop production were measured for two years and the PCF, NH3, and farm N-balance were calculated. The following hypotheses have been made:
• Increasing intensification in dairy causes higher emissions and high N-surpluses per ha and per kg of milk in comparison with semi-intensive systems that include grazing.
• Full grazing is more advantageous in terms of the mentioned ecosystem services, in particular low-cost systems without high use of external resources.
• The latter can be further improved by ICLS.
Materials and Methods
Research Area
The study was conducted in the eastern part of Schleswig-Holstein, northern Germany. The soil type in this region is predominantly sandy loam, and highly suitable for arable production with high yields of both cereals and forages (Loges et al., 2018a; Struck et al., 2019; Biernat et al., 2020b; Reinsch et al., 2020). Among the federal states of Germany, Schleswig-Holstein has the highest stocking density of dairy cattle (at 69 per km2; compared to average of 27 per km2) which may be attributed to its climate being well–suited to forage production. Moreover, farming practices are highly specialized toward either cash-crop production or dairying, with low interaction among these systems. This specialization results in high farm-N surpluses and high nitrate loads to the groundwater, particularly in areas where the highest numbers of dairy stock are located (Taube et al., 2015; Biernat et al., 2020). The region has a maritime climate with moderate long-term average temperatures of 8.9°C and annual rainfall of 737 mm. From the 1970s to 2019 the number of dairy farms in the region dropped from 8,000 to below 4,000 (Destatis, 2017). During the same period, the average milk yield per cow has increased from ~6.000 to ~9.000 kg ECM, with high variability in milk yields depending on system and specialization. Nowadays, most cows in the region are kept inside year-round, receiving together with grass, maize-silage (at 6 kg DM cow−1 day−1) and additional high inputs of supplementary concentrate feed (2.7 t DM cow−1 year−1) (LKSH, 2019). The average herd size is 150 cows and average annual milk yield is 8 920 kg ECM per cow. Access to full-pasture land is low as a proportion of the total number of dairy cows (<9%). Within this region and yield levels, we identified four prevailing farm types: (i) intensive-confinement (IC), (ii) semi-confinement (SC), (iii) full-grazed (FG), and (iv) integrated-full-grazed (IFG), with a gradient in the share of grazing and resource inputs (Table 1). The SC farm type represents the average production conditions in this region, and its production data are comparable to data from officially published farm surveys. The annual released farm survey data cover 430 representative farms across the country (LKSH, 2019). In SC the animals had, in addition to grass and maize silage, access to pasture during daytime from May-September on the land surrounding the cowshed. The IC system was chosen from the top 64 farms in this area according to the milk yield records (>10,000 kg ECM cow−1 year−1). The cows were confined year-round. Forage in IC was provided from grass and maize silage only. The FG and IFG systems were identified on the same soil type and these systems are not commonly practiced in this region. Thus, these farms acted as an alternative in comparison with the business-as-usual scenarios (IC and SC). The proposed grazing management conducted in FG and IFG followed the principles of rotational grazing adopted from systems as used in Ireland and New Zealand. For instance, the farm manager of FG was part of an international knowledge transfer network working on improved rotational grazing management. The IFG collaborated intensively with the Irish research center Teagasc (Loges et al., 2018b). Both the FG and IFG implemented rotational grazing on diverse grass-clover swards. Stocking rates for dairy cows on pastures in the FG and IFG were adjusted in accordance with non-destructive aboveground biomass measurements (AGB) obtained with a yield-plate-meter (Trott et al., 2002) to ensure an optimal forage allowance. The access to pasture ranged from 0 to 292 days year−1 among the systems. The N application from mineral fertilizer and slurry was on average 247 (IC) and 182 kg N ha−1 (SC). In FG and IFG the N-fertilization was provided by BNF and N-excreta from grazing animals. The farms IC, SC, and FG were exclusively specialized on dairy and forage production, whereas IFG was part of an ICLS. In detail, forage was offered as grazed grass-clover leys. These leys were plowed after two full grazing years (spring of the third year), and were followed by cash crops to benefit from the carry-over effect of N (Ncarry−over) from the grass-clover leys. As a result, the share of permanent grassland was lowest in IFG and highest in FG. New leys in IFG were established as an understorey in winter wheat prior to the first production year, with a seeding rate of 29 kg ha−1 (70% Lolium perenne, 20% Trifolium pratense, 10% Trifolium repens). In the FG system, arable grass was renovated every 5 years, of which 4 ha were plowed in late summer followed by a catch crop for winter grazing for heifers. The remaining arable grass was renovated in spring and reseeded at a rate of 30 kg ha−1 (80% Lolium perenne and 20% Trifolium repens). Permanent grassland management and silage production was conducted according to the local recommended practice comprising 3–5 silage cuts on grassland (Loges et al., 2018a) and maize seeding at the beginning of May and harvest at silage maturity at the beginning of October (Komainda et al., 2018). Where swards were grazed, the residual biomass after each of 8–10 grazing cycles per year was clipped when necessary and the clippings left as a mulch.
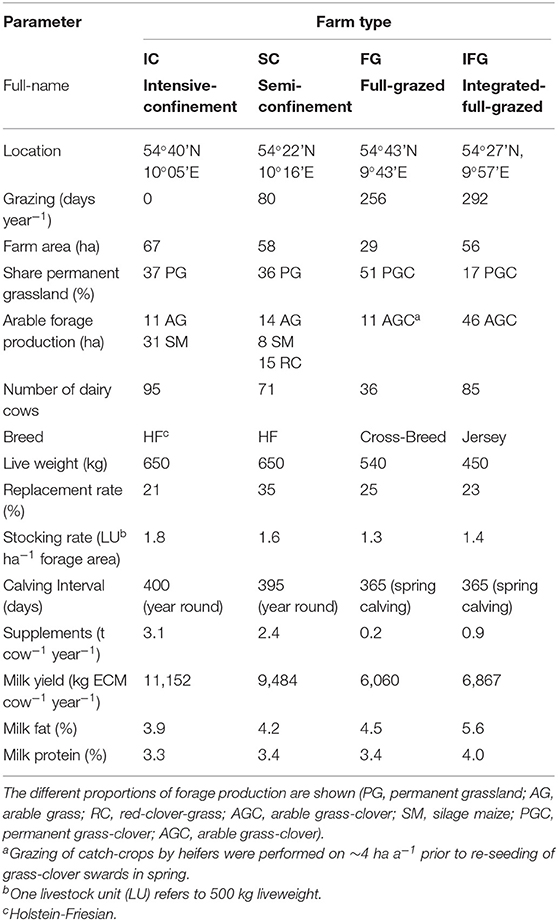
Table 1. Overview of the prevailing dairy production systems available in the case-study-area as average of two experimental years.
On-Farm Research Design
Each of the four dairy farms (representing systems IC, SC, FG, and IFG) was observed for 2 years during the period 2011–2019. The farms were selected on the basis of the above-mentioned production data representing for SC and IC the average and best 25% with regards to management and milk yield in the state. The grazing systems represent systems that are not common for the time being, but proposed promising alternatives, in comparison with confined systems. The IC and FG systems were observed during the years 2010 and 2011, and farm SC from 2014 to 2015. The IFG was observed from 2017 to 2019; however, because of heavy droughts during 2018 the data for this year were discarded as they were not comparable to the other study years. Weather data were recorded at weather stations from Germany's National Meteorological Service (DWD) close to the experimental sites. Average rainfall and daily temperature during the vegetation period (April–Sept.) and experimental years were 434 mm (SD 82) and 14°C (SD 0.5). According to simulation runs for a 4 cut silage system with the grass growth model FOPROQ (Torssell and Kornher, 1983; Herrmann et al., 2005), used by national recommendation services to estimate the optimal cutting date for grass silage in the state, the average grass silage yields within the climatic region of the analyzed farms showed only small differences for the simulated yields [~11 t DM ha−1 (SD 0.7)], indicating comparable climatic conditions during the different vegetation periods and experimental years. Soils on the investigated farms were classified as sandy loams, with the texture ranging from 56 to 76% sand, 16 to 29% silt, and 7 to 16% clay. For IC and SC the most common breed in the research area Holstein-Friesian was used. In the FG and IFG systems farm managers chose cows with a lower weight to ensure early and late grazing could take place with a minimum of sward damage from trampling.
Farm Management Data
Common farm management and herd data were collected throughout the study period. These data contained herd information (e.g., replacement rate, weeks of lactation, milk yield), farm management data (e.g., area of land, land-use, soil tillage intervals, and grassland management) as well as purchased resources by the farm manager (e.g., supplementary feeds, fertilizer, agro-chemicals).
Forage Productivity
The aboveground biomass (AGB) on grassland was measured by hand clipping with shears prior to the silage cut or grazing event. The sampling area was 0.25 m2 (with ten replicates per paddock) and the sward was cut leaving a stubble height of 50 mm. For all mown grassland there were 3–4 cuts, and for the pastures 3–11 rotations including silage cuts per experimental year according to the conducted grassland management of the different systems. The AGB for the pastures were further defined as AGBaccess. In addition, enclosures were installed on the pastures to measure the biomass growth during grazing events (AGBregrowth). After grazing the AGB residues (AGBresidues) were quantified by hand clipping as described above, but leaving 40 mm stubbles. The pasture-intake by grazing animals was calculated as:
For maize, ten plants in a maize row were harvested at silage maturity and cut at a stubble height of 20 cm. This procedure was replicated three times on each ha of maize. A sub-sample of ~100 g for grass and 1,000 g for maize, respectively, was taken to determine plant dry-matter (DM) content after drying in an oven at 58°C until constant weight. In addition, subsamples of the grass-clover swards were separated into the fractions grass and clover. Prior to forage quality analysis, dried subsamples were ground in a two-step procedure: first passing a 5-mm (Retsch, GmbH, Haan, Germany) and subsequently a 1-mm screen (FOSS, GmbH, Rellingen, Germany). Forage N content and net-energy-lactation (NEL) were estimated by Near-Infrared-Reflection-Spectroscopy with a NIRsystems 5000 scanning monochromator (FOSS, Silver Spring, Maryland, USA). The total crude protein (CP) was calculated by multiplying a factor of x 6.25 to the respective N-content.
The average daily DM-intake of feed by cows was estimated according to the equation from Gruber et al. (2004), which takes into account the milk yield, the share of different forage types, the MJ NEL kg DM−1 and CP-content of feeds as variables. The average milk urea nitrogen (MUN) was calculated as a function of the average CP in forage (Spek et al., 2013) and DM-intake, whereas the daily N-excretion (Nex) was estimated by daily DM-intake, its CP-content and live-weight following the approach by Nennich et al. (2005).
N2O-Emissions and N-Leaching
Fluxes of N2O on each field and dairy system were measured with the static closed chamber method. The minimum sampling frequency was once a week in all crops, taken between 10 a.m. and 12 p.m. In a pre-treatment, four collars for maize and grassland cut for silage and ten collars for pastures (d = 60 cm, h = 15 cm), made from polyvinyl chloride (PVC), were installed into the soil to a depth of 10 cm. The chambers were placed at uniform distances between the replicates in order to capture a representative area across each forage crop (avg. investigated field size 0.75 ha). The collars were removed during maize and grass-cut harvesting and tillage operations but remained in the grazed fields. During the flux measurements collars were closed gas tight with white PVC chambers (d = 60 cm, h = 35 cm). Gas samples were taken at 0, 20, 40, and 60 min after closure through a gas-tight septum on the top of the chamber using a 30 ml syringe. Samples were directly transferred into 12 ml pre-evacuated septum-capped vials (Labco, High Wycombe, UK). In the first 2 weeks after fertilizer application and grazing events, measurements were conducted more frequently at irregular intervals but at a minimum of two times per week. Gas samples were analyzed for N2O and CH4 through a gas chromatograph (SCION 456-GC, Bruker, Leiderdorp, Netherlands). The change of gas concentration in the chamber headspace during the measurement period was calculated by linear regression.
To determine N leaching to the groundwater over the winter period, soil water samples were taken using ceramic suction cups (Mullit, pore size 1 μm, length 54 mm, diameter 20 mm., ecoTech. Bonn, Germany). Sixteen ceramic suction cups were installed during the two experimental years and per crop at a depth of 75 cm and at an angle of 60° to minimize preferential flow and sampled from October to March. Prior to the first sampling date the suction cups were installed 6-months before the first sample was taken to ensure adequate time for soil settlement. To collect free drainage water a vacuum of 0.4 bars was applied to all suction cups. Soil water samples were obtained weekly until April. During sampling, four of the respective sixteen suction cup samples were mixed leading to four samples in total each week from every field. Leachate samples were stored at −20°C until analysis. Concentrations of total-N were determined photometrically using a dual channel continuous flow analyzer (SKALAR Analytical Instrument, Breda, the Netherlands). The amount of percolating water was calculated by a climatic water balance model, using weather and soil data gathered from the experimental site, actual evapotranspiration (Mohrlok, 2009), and specific crop coefficients (Löpmeier, 1994; Häckel, 1999) to correct evaporation.
Product Carbon Footprint (PCF)
The PCF for milk production of the four contrasting dairy farms was calculated using measured data for N2O as direct emissions and N-leaching as an indirect source for N2O-emissions from land-use. Additional indirect N2O emissions from NH3 volatilization in the cowshed were calculated according to Burgos et al. (2010). The emission factor (EF) for NH3 volatilization from grazing animals were based on a review analysis of Sommer et al. (2019). Other gaseous N-emissions during manure application followed the methodology of the IPCC guidelines (IPCC, 2006; Mogensen et al., 2014). Methane emissions from ruminal digestion was calculated according to Schils et al. (2007).
In order to account for the on-farm soil carbon changes (SOC) of the tested production systems a simple approach developed by Petersen et al. (2013) was used. In this approach the different crops and management systems are compared to a reference system to estimate potential soil carbon changes. For the reference system a continuous long-term experiment with winter-rye cultivation, without any manure and fertilizer amendments, was chosen (for details see Merbach et al., 2000). Carbon inputs from roots and exudates were calculated as a ratio of AGB according to Taghizadeh-Toosi et al. (2014). For the various crops, different land-use factors (grassland:1, arable grass: 0.93, cropland: 0.8) and soil tillage factors (grassland:1.1, arable grass: 1.1, cropland: 1) were utilized, according to IPCC (2006). Emissions from external resources were adapted from the ecoinvent (vers. 3.3) data basis (econinvent, 2016). Estimates for the required off-farm cropland were based on the purchased supplements by the farm manager. Land requirement for soybean meal were calculated according to 10-year-average yield values (2007–2017) of soybean in Argentina (FAOSTAT, 2017), assuming an oil content of 20% and losses during the milling process of 2%. Supplementary feed is made from co-products from the production of plant-based oils, starch and sugar. Imported feeds with relevant by-products, such as oils and sugar were considered on the basis of the mass allocation for soy, canola, sunflowers and sugar beet (Dalgaard et al., 2008).
For the slurry exported from dairy farms for cash crop production on other land, it was assumed that every exported kg N would replace the equivalent of one kg mineral fertilizer. To follow the concept of consequential LCA production, costs for ammonium nitrate were used (ecoinvent 3.3) as the GHG savings for exported nitrogen in organic manures. Transport distances to cash crop producers and related GHG emissions were assumed to be negligible and ignored in this calculation. In the IFG, grass-clover was used as the forage base and the residual N in stubble and roots were considered as an export. The N from both above- and belowground crop residues was considered, with an equivalent of 0.51. This factor is based on observations from Huss-Danell et al. (2007) from a N-partitioning experiment on red-clover grassland. To calculate the global warming potential (GWP) per ha, the respective value for each trace gas over a life-span of 100 years was used (CO2 = 1, N2O = 265, CH4 = 28) and expressed in CO2-equivalents (CO2eq). The efficiency of the systems with regards to climate change was calculated relatively, on the basis of the functional unit energy corrected milk (ECM), according to Sjaunja (1990).
Ammonia Emissions and Farm-N-Balance
Ammonia emissions per kg energy-corrected-milk (ECM) were calculated in accordance with the emission factors described above [section Product Carbon Footprint (PCF)]. The on-farm N-balance was calculated by the sum of nitrogen inputs and deduction of nitrogen outputs at farm-gate. For nitrogen inputs all purchased mineral fertilizers, straw, seeds and their respective N-contents, as well as biological nitrogen fixation (BNF) were considered. The BNF was calculated using the fraction of AGB derived from clover yield together with the modeling approach of Høgh-Jensen et al. (2004) and the N-cycling on pastures derived from Nex. The N deposition from rainfall was used in the N-balance calculation, with a long-term annual average N deposition (1989–2005) in this area of 12.5 kg N ha−1 year−1.
Nitrogen outputs consisted of milk and meat. The nitrogen export of milk was estimated by dividing the protein yield by 6.38 (ISO, 2014). For dairy cows the live-weight with a carcass weight of 46% was used. The protein content of carcass was assumed as 19% and converted to nitrogen using the factor 6.25. The annual meat export was calculated on the basis of the replacement rate. This also included the export of calves from the farm. After deduction of slurry demand on farm and gaseous-N losses during storage the surplus of slurry-N was taken as export-N. Fertilization management on the farm was considered to be in accordance with the farm management records.
Results
Forage Productivity
Measured average forage yields on farm were comparable at 10–11 t DM ha−1 year−1. The average CP contents differed, with 16, 15, 21, and 21% of DM in the IC, SC, FG, and IFG. However, when considering forage imports CP in the feed ratio increased to 20 and 16% CP for IC and SC, whereas CP slightly decreased in FG and IFG, due to the lower CP contents in the supplementary feed. Average net-energy lactation in forage was 7 MJ NEL kg DM−1 in all systems. NEL was mainly provided by silage maize in IC and SC and highly digestible grazed grass-clover in FG and IFG. Feed imports provided additional energy in total, in particular in SC, IC, and IFG, but without changing the average energy content of 7 MJ NEL kg DM-intake−1. Calculated daily DM-intake showed a range of 17–24 kg day−1 for dairy cows. Lowest values were found in IFG and FG and highest in IC (Table 2).
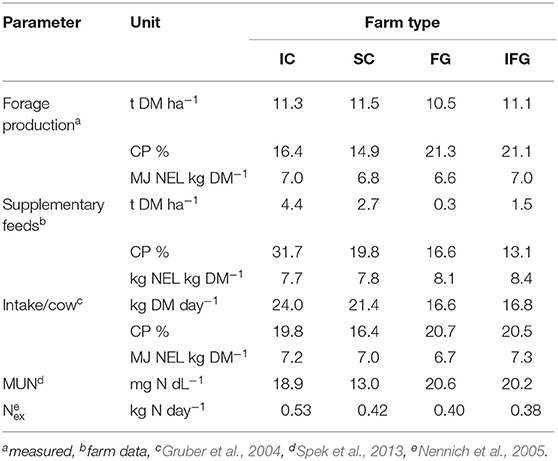
Table 2. Measured average DM-yields, energy- (MJ NEL kg DM−1) and crude-protein (CP) contents from forage production and DM-intake (forage + supplements) from the two experimental years, and the predicted milk urea (MUN) contents and annual N-excretion (Nex) per cow among the different systems (IC, intensive-confinement; SC, semi-confinement; FG, full-grazed; IFG, integrated-full-grazed).
N2O-Emissions and N-Leaching
Measured annual N2O emissions were in the ranges of 0.7–4.7 in IC, 1.1–11.9 in SC, 0.9–2.6 in FG, and 0.1–0.7 kg N2O-N ha−1 year−1 in IFG (Figure 2). Highest mean annual N2O emissions were measured in the SC system, which comprises N-inputs from mineral and organic fertilizers as well as N-excretion from grazed pastures. The N2O-emissions from the grazed grass-clover swards that received no additional N fertilization were generally low, showing maximum annual emissions of 1.6 kg N2O-N ha−1 year−1 in FG. The IFG system showed similar emissions on permanent grassland, but had lower emissions on grazed arable grass-clover leys in comparison with FG. Higher emissions in the grazed arable grass systems in FG were mainly a result of the grazed catch crops during winter prior to grassland sowing in spring (data not shown). Annual N2O emissions from silage maize were elevated in comparison with grassland in the IC and SC systems, with average emissions of 4.3 kg N2O-N ha−1 year−1. In comparison, grassland used for silage revealed 1.7 kg N2O-N ha−1 year−1. The total N-fertilization from mineral fertilizer and cattle slurry applied was on average 191 kg N ha−1 year−1 for silage maize and 284 kg N ha−1 year−1 for the permanent grassland utilized by mowing in IC and SC (Figure 2). Thus, emission factors for N-applied, calculated from the measured N2O emissions, were higher for silage maize (2.3% of each kg N-applied) and slightly lower for mown grassland (0.6%) compared to the current EF of the IPCC guidelines. For grazing systems, the IPCC takes an EF of 2% of excreted-N into account. Annual N-deposits excreted by cows during grazing were calculated as 131 in FG and 141 kg N ha−1 year−1 in the IFG. The calculated EF values were 1.2 and 0.6% in FG and IFG and thus higher for FG; however, they are in accordance with the IFG as currently shown in the IPCC refinement report for other N-inputs in wet climates.
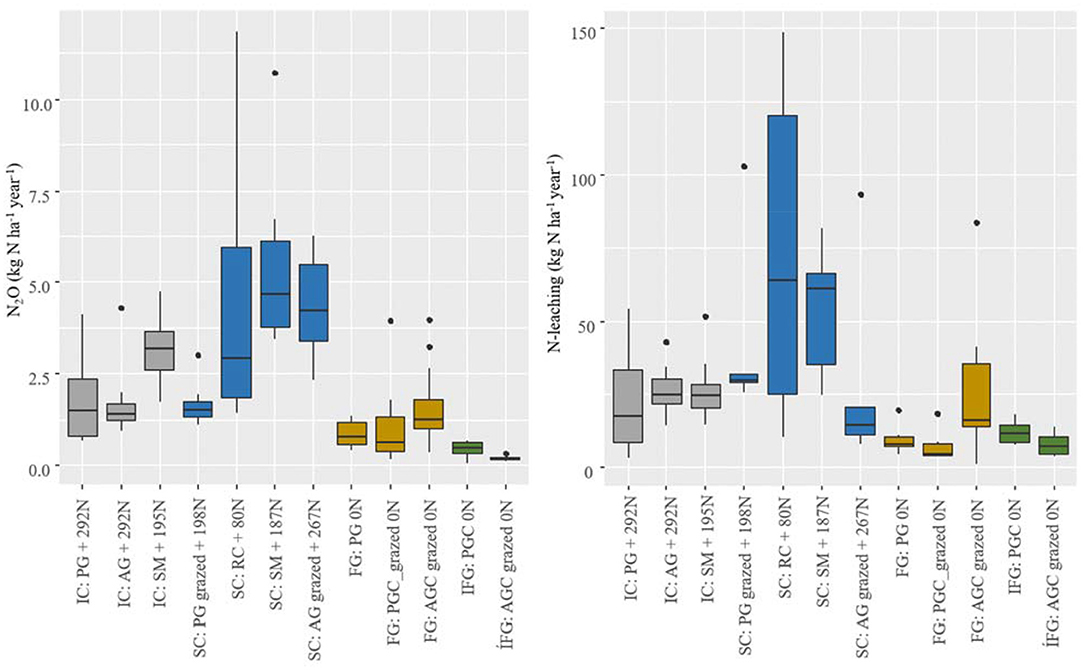
Figure 2. Box-plots present the measured accumulated N2O emissions and N-leaching to ground or drainage water losses across the different farm types (IC, intensive-confinement; SC, semi-confinement; FG, full-grazing; IFG, integrated full-grazing) and forage crops (PG, permanent grassland; AG, arable grass; RC, red-clover-grass; AGC, arable grass-clover; SM, silage maize; PGC, permanent grass-clover; AGC, arable grass-clover). The amount of N applied (kg N ha−1) by mineral fertilizer and slurry is given in the figure legend. N-losses of two experimental years are shown. The box represents the 25th and 75th percentile. The black line in the box is the median. The whiskers represent the minimum and maximum values. Dots are outliers.
Nitrogen leaching was on average over the two experimental years 50, 25, 15, and 10 kg N ha−1 year−1 for SC, IC, FG and IFG. High N-leaching losses were observed in particular for N-fertilized arable red-clover grass swards (73 kg N) and silage maize (37 kg N) in SC. For N-fertilized permanent grassland and arable grass in the SC and IC system, leaching losses of 31 kg N ha−1 were measured. Non-N-fertilized and mown permanent grass-clover revealed 16 kg N ha−1; the grazed permanent grass-clover showed leaching losses of 24 kg N ha−1, and grazed arable grass-clover swards revealed slightly lower losses of 21 kg N ha−1. However, N losses in the latter were dependent on sward age with increasing increments of N-losses for each additional year of grazing management (data not shown).
Product Carbon Footprint (PCF)
The largest contribution to the total global warming potential (GWP) per ha in all systems was from enteric CH4 emissions, as a result of ruminal digestion. In addition, with energy expenditure for milking and animal housing, the share was 45 and 44% in the IC and SC system (Table 3). Due to lower milk yields and lower share of other emission sources, the enteric fermentation had a larger contribution in the grazing systems, FG and IFG, and accounted, on average, for 66% in both systems. Taking the feed imports as well as the inputs of fertilizers and seeds into account, forage production showed the second largest share of emissions with 26, 28, 14, and 14% of GWP in the IC, SC, FG, and IFG systems. The third largest contributor in the IC and SC was manure management at the farm facilities. With increasing share of grazing (0% IC, 22% SC, 70% FG, and 80 IFG% expressed as a percentage of days on which they were grazed per year) the importance of manure management on the total GWP declined from 23 (IC) to 9% (IFG). Greenhouse gas emitted from the young stock showed a range of 6–14% of total GWP, with lowest shares in IC and IFG due to lowest replacement rates (see Table 1). Soil carbon sequestration reduced the GWP in all systems, as the high share of grass in the forage crop rotation, together with slurry inputs, led to positive soil carbon balances. Highest sequestration rates were observed in the IFG system due to the high acreage of 2-year grazed grass-clover leys (Table 3). Calculated annual sequestration potential was 0.24, 0.36, 0.47, and 0.56 t C ha−1 across the systems IC, SC, FG, and IFG. With the exception of SC all farms are eligible to receive credits for the substitution of mineral fertilizers by slurry exports. However, there were large differences among the systems with highest exports in IC (Table 3). The accounting of credits for BNF as a by-product was only applicable in the IFG, as leys were part of ICLS. On the other farms N circulated only in the forage crop system leading to a potential excess of N (see section Farm-N-Surplus and Losses of Reactive Nitrogen). The PCF for each kg ECM produced was in the range of 0.9–1.3 kg CO2eq with lowest values in IFG and highest in SC. Taking soil carbon sequestration and consequential impacts due to greenhouse gas (GHG) crediting into account, the PCF was reduced in all systems but was still lowest in IFG (Table 3).
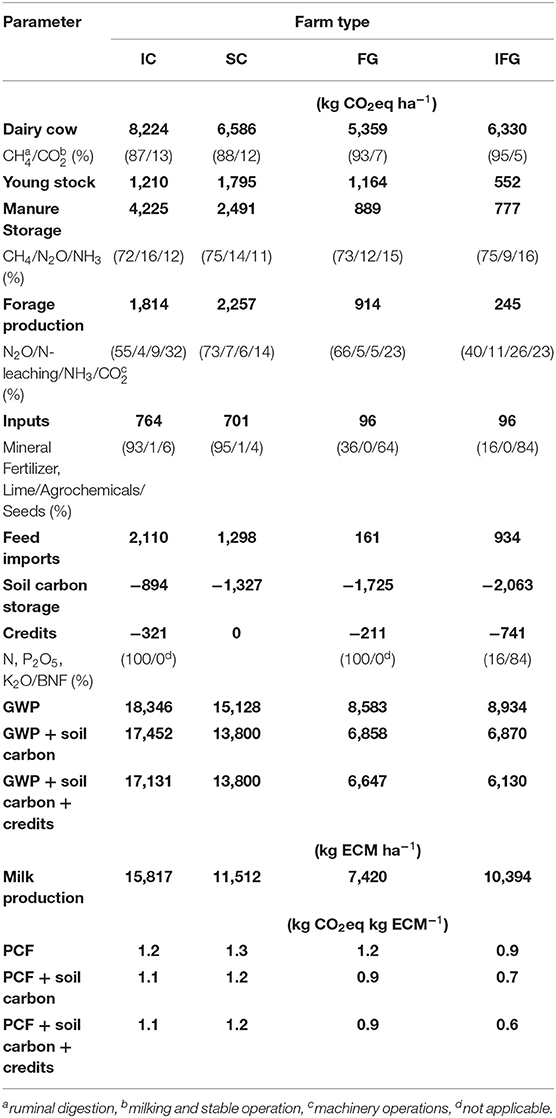
Table 3. Global warming potential (GWP) per ha in the different dairy systems and system units expressed in kg CO2-equivalents (CO2eq) per ha.
Farm-N-Surplus and Losses of Reactive Nitrogen
The farm N-balance declined from 229 to 50 kg N ha−1 year−1 in the order of IC, SC, FG and IFG. In the IC and SC systems, highest imports at farm gate were provided by mineral fertilizer and feed imports, whereas N-imports by supplements exceeded 200 kg N ha−1 year−1 in the IC due to a high share of soybean meal. In the FG and IFG, biological nitrogen fixation (BNF) had, with 130 and 121 kg N ha−1 year−1, the largest contribution to N-inputs, leading to comparable total average N-inputs per ha of 121 kg N ha−1 year−1. Milk provided the largest N-export from the farm in IC (69%), SC (91%), and FG (60%) systems. However, these figures were different for the IFG, showing a share of 40%. Exports from BNF in the form of plant residual matter was only applicable in the IFG system, and this influenced the farm-N-balance positively (Table 4).
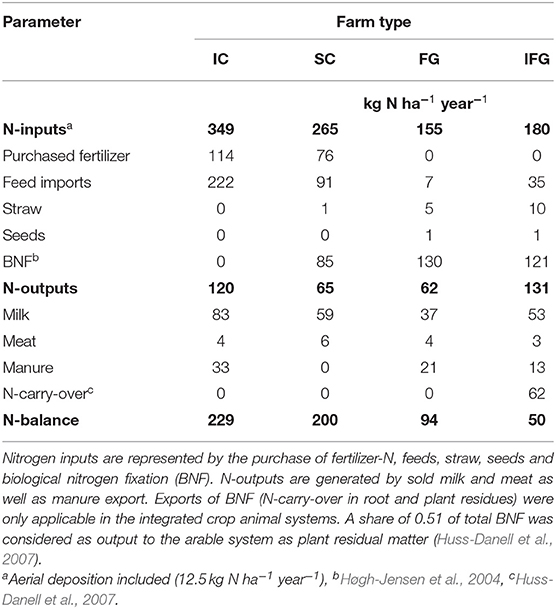
Table 4. N-balance of the prevailing farm types (IC, intensive-confinement; SC, semi-confinement; FG, full-grazing; IFG, integrated full-grazing).
Total predicted and measured losses of reactive N from the farm were generally in accordance with the calculated farm-N-balance, showing slightly lower values (Table 5). NH3 emissions from manure storage and N-application were the largest contributors with a share of 66–85% to the total N-losses. The total NH3-emissions amounted to 160 kg N ha−1 year−1 in IC, whereas in SC, FG, and IFG these losses were reduced by 37, 72, and 70%. The range of N-leaching on total losses was 13–31%, with highest losses in IC and SC. Measured N2O-emission were with 2–3% of minor relevance in total. The N-footprint per kg milk produced was highest for SC and lowest for IFG (Table 5).
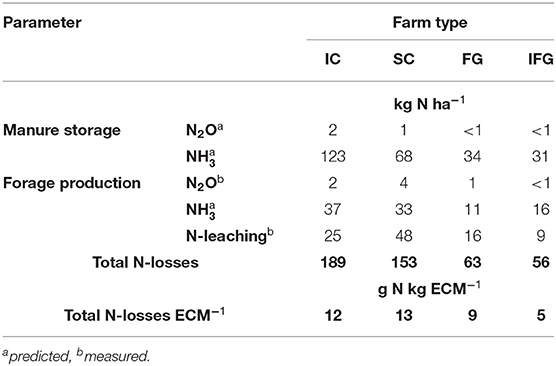
Table 5. Measured and predicted losses expressed in kg N ha−1 of reactive nitrogen from the different systems (IC, intensive-confinement; SC, semi-confinement; FG, full-grazing; IFG, integrated full-grazing) as well as the N-footprint per kg energy corrected milk (g N kg ECM−1).
Considering the calculated N-balance (Table 4) and leaving the N-carry-over in IFG aside, 17, 23, 32, and 50% of N-losses of the farm N balance were not accounted for in the IC, SC, FG, and IFG, respectively. However, when accounting for soil C sequestration, with the site-specific C/N ratio, N accumulation in the soil amounted to 24, 36, 47, and 57 kg N ha−1. This reduced the percentage of N not accounted for to +7, +5, −17, and −1%.
The relative differences of environmental impacts compared to IC indicated a 18 and 43% lower PCF; 59 and 78% lower farm-N-balance, and 72 and 71% lower NH3-emissions per ha in FG and IFG. SC showed a 11% higher PCF and 13 and 38% lower N-balance and NH3 volatilization.
Milk yield per ha on the farm was 15,817, 11,512, 7,420, and 10,485 kg ECM ha−1, resulting in a land requirement of 0.6, 0.9, 1.3, and 1.0 m2 kg ECM−1 in IC, SC, FG, and IFG. However, taking imported land from purchased supplementary feeds into account, this relative perspective changed to 1.2, 1.2, 1.4, and 1.3 m2 kg ECM−1. With increasing milk yields per ha the farm N-surplus increased but did not show a clear linear trend among the systems. In contrast, the GHG emissions per ha correlated clearly positively with the N surplus on farm (Figure 3A). However, lowest N-surplus does not necessarily provide the lowest PCF (Figure 3B).
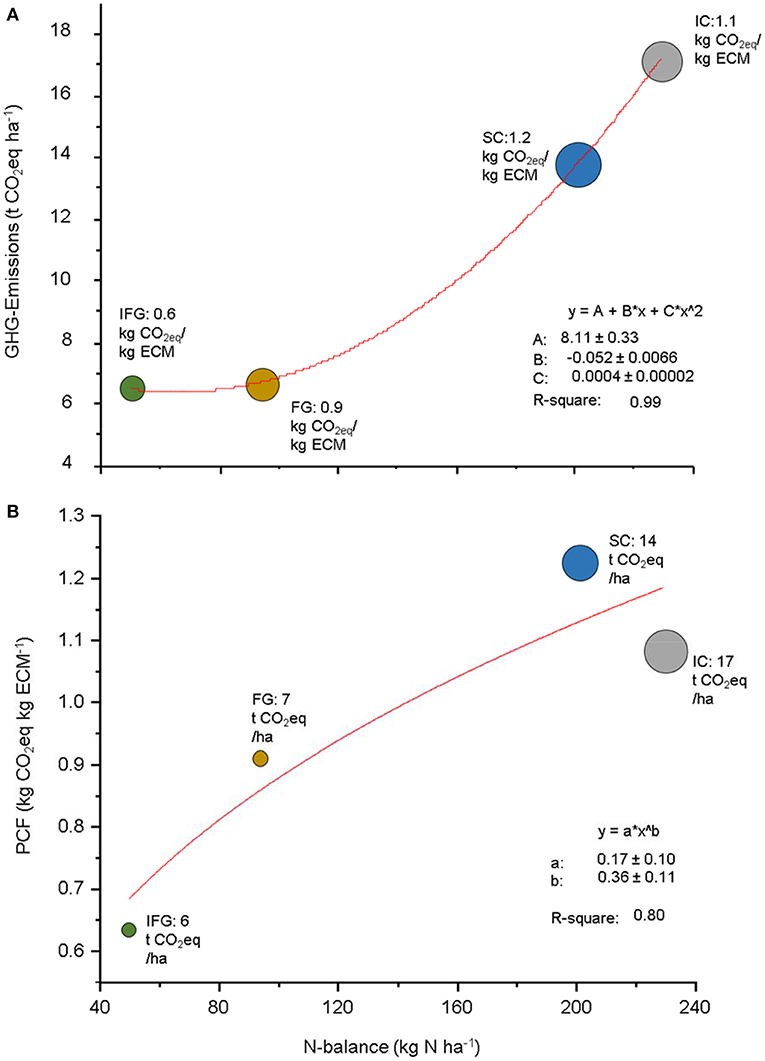
Figure 3. Relationship between the farm-N-balance, GHG-emissions and product carbon footprint (PCF), among the different systems (IC, intensive-confinement; SC, semi-confinement; FG, full-grazing; IFG, integrated full-grazing). Results of the regression analysis are shown. Legends on the bubbles show either the PCF (A) or the GHG-emissions per ha (B).
Discussion
Forage Productivity
On sandy loamy soil, high productivity of forage crops can be achieved, particularly in areas with temperate maritime climate and evenly distributed rainfall (Loges et al., 2018a; Struck et al., 2019). The most important driver is the nutrient availability, which is mainly constrained by N (Biernat et al., 2020b). In high-input dairy systems, N is provided by slurry and purchased mineral fertilizers, whereas in low-cost systems the use of external N resources can be avoided by using forage legumes and their ability for BNF (Reinsch et al., 2020). The N available on farm, and consequently the total N in slurry, is further increased by imported N-rich feeds. Consequently, the forage DM yield in the different observed dairy systems did not differ significantly, as there was sufficient N available either through artificial N-fertilizer imports (IC and SC) or BNF (FG and IFG) on grassland. Nevens and Reheul (2003) reported from a 31-year ley-arable trial, on the same soil type as used in our study, that grazed leys provide similar amounts of forage to those of old permanent grassland swards, even at lower rates of fertilizer application. Low yield responses of pastures to N-fertilization were also reported elsewhere and can be explained by the N-return from grazing animals (Viljoen et al., 2020). This effect is more likely in grass-clover swards, where forages with high N contents are offered, leading to a higher N status in the soil during the grazing period. Moreover, the high yields from the observed leys, without any disadvantage to permanent grassland, can be explained by the chosen forage legume-based seed mixture, which can lead to benefits in herbage growth and subsequent yield (Nyfeler et al., 2011; Lüscher et al., 2014). Environmental factors are the largest explanatory variables that affect yield differences between years. Changes in the climate in northwest Europe are anticipated in the coming decades, and in response to these changes grassland productivity may increase by 11% by 2050 (Höglind et al., 2013). However, severe droughts will also become more likely, reducing the yield in those years considerably below the long-term average. In the IFG system, the leys were established as an understorey prior to the first production year. The rooting system of the established red-clover mixture allowed a higher yield resilience to droughts, in comparison with that of pure grass or grass-white clover swards (Lorenz et al., 2020). This is particularly important in this research area, where periods with lower rainfall often occur between May and June, increasing the inter-annual yield volatility.
In contrast to the very similar DM yield of the various systems, the observed forage systems differed in their ratio of CP and energy. The forage production in the IC and SC systems were dominated by grass and maize silage providing a ratio of 20–22 (g CP MJ NEL−1), whereas FG and IFG showed an outstanding ratio of 31. However, with addition of the supplementary feeds, the ratio increased to 28 in IC but remained constant in SC as a result of a disadvantageous feeding strategy. Thus, the low-cost grazing systems (FG and IFG) produced a high share of CP on their farm itself, mainly as white and red-clover having a CP content, which is slightly lower but with a high potential for supplementary feed substitution at moderate milk yield losses (Schulz et al., 2018). However, improvements in feeding with balanced CP/NEL ratios across the grazing season become even more important in FG and IFG, as the forage quality is determined by the cultivation strategy of grass-clover leys and the typical annual growth patterns. Seeded grass-clover leys can show a high biomass accumulation by the forage legume components, which are determined by a higher proportion of grass in the total yield during spring, and a lower proportion of grass and more legume in late summer and autumn (Lorenz et al., 2020). Typically, this can lead to a surplus of ruminal-N and elevated milk urea contents in the second half of the year, therefore requiring use of energy-rich supplements necessary to maintain an optimal ruminal-N-balance and to reduce the risk of high urea nitrogen contents (Selbie et al., 2015), and also making NH3- volatilization (Burgos et al., 2010) and N-leaching more likely (Cichota et al., 2012). In the IFG system, this was balanced by a moderate import of starch-rich components like cereals, which increases the milk yield per cow and ha compared to FG. In the FG system the swards were renovated every 5 years but were not part of an integrated crop system. Thus, the control of the desired sward composition was more challenging as renovated permanent white-clover grassland swards can show a high share of legumes 2–5 years after renovation, thereby producing a surplus of BNF and CP (Reinsch et al., 2020). This was present by highest CP-contents in offered forage and highest annual average milk urea nitrogen (MUN) in FG (Table 2) as result of a lack of supplementation of energy. Thus, with regard to the feeding strategy rotational grazed grass-clover swards can generate high yields, which can compete with intensively fertilized forage systems in terms of energy- and protein yields. However, the absence of moderate supplementation may cause undesired feed-backs on animal health and milk yields, and therefore a balanced CP to energy ration should be sought.
N2O-Emissions and N-Leaching
The measured N2O-emissions on the different farms showed typical patterns throughout the year, with high emission peaks during winter and after N-application (data not shown). Thus, in the N-fertilized arable and permanent grassland sites the calculated EF was close to the recommended value of the IPCC guidelines (IPCC, 2006), due to the dominance of annual emissions from peaks shortly after application. Higher emissions from silage maize in comparison with grass have already been reported elsewhere; this is due to high application rates of slurry during spring, which is incorporated into the soil prior to maize sowing (Struck et al., 2020). Under these conditions, with high amounts of N and easily decomposable organic matter, high N2O-fluxes from soil heterotrophic denitrifies are likely. These can further be accelerated by additional N from soil organic matter mineralization induced by soil tillage activities (Struck et al., 2019, 2020). In the grazed systems, the annual emissions were significantly lower than the former IPPC default factor advised (IPCC, 2006); however, they are in accordance with the refined factors currently released for wet areas (IPCC, 2019). Such lower emission factors have also been reported from other grazing experiments in South Africa (Smit et al., 2020) and New Zealand (van der Weerden et al., 2020) as well as on the same experimental site over a long gradient of plant diversity and environments (Nyameasem et al., 2021). In addition to N-excreted by grazing animals, BNF was a major N-input in the IF and IFG systems. Even without the use of mineral N-fertilizers positive N-balances were achieved, particularly if the proportion of forage legumes within the ley is high (Reinsch et al., 2020). However, symbiotically fixed N is mostly captured in plant tissues, such as leaves and roots, but it becomes available erratically, depending on soil moisture, temperature, and soil and residue management. At the same time grasslands can show a large quantity of C and N sequestration in the soil, thereby avoiding the majority of N-losses (Loges et al., 2018a; Reinsch et al., 2018a). Moreover, the plant uptake of decomposed N is efficient in low-input systems characterized by low N2O-emissions (Schmeer et al., 2014) and N-leaching (Reinsch et al., 2018b). Nevertheless, if BNF is not accounted for in N-fertilizer planning, N-leaching losses over the drainage period are likely to be increased, due to mineralization of the plant residues, in particular in temperate grassland areas where air temperatures often remain above 5°C during winter. This might explain the high measured N-leaching losses under red clover-grass swards in the SC system in both experimental years, where in addition to the predicted BNF of 305 kg N ha−1 there was also a N-fertilizer rate of 80 kg N ha−1. However, in the grass-clover swards of FG and IFG only minor leaching losses were measured, which is in line with several other studies elsewhere on comparable soil types (Reinsch et al., 2018b; Biernat et al., 2020b). Under grazing situations N is frequently returned in the excreta. The higher availability of N in grazed swards changes the sward composition in comparison with mown red clover-grass toward one with a higher share of grasses with the consequence of a higher root length density, measured on the same site (Chen et al., 2016). This accelerates the N-uptake by the sward, which may have improved the N-efficiency of the grazed grass-clover system in FG and IFG in comparison with SC. Moreover, the SC system showed the highest N-footprint of all farm types leading to an acceleration of N in the system, which increased the level of total measured N-losses in all forage crops in comparison with IC, FG, and IFG. In this context, the farm management is of critical importance, as N-losses on the farm are exacerbated by disadvantageous feeding strategies, manure management and management of forage production.
Product Carbon Footprint (PCF)
Several studies have proposed that GHG emissions per product unit are negatively correlated with increasing milk yield per cow (Lesschen et al., 2011). Other studies mentioned that this has to be further differentiated by the production system used (Rotz et al., 2010; Zehetmeier et al., 2014; Lorenz et al., 2019). In confinement systems, large amounts of resources, usually imported, lead at some point to a compensation of reduced GHG-emissions per product unit on farm. In comparison, low-cost systems generate their milk yield exclusively from the on-farm produced forage, which in turn leads to lower milk yields per ha on-farm but also to a lower use of resources, thus contributing negatively to the PCF. Lorenz et al. (2019) found that a milk yield of 6,000 kg ECM per cow−1 and year−1 provided by low-cost grazing is not disadvantageous in comparison to that of a confinement system, which uses a high share of imported supplementary feeds in order to achieve milk yields of 10,000 kg ECM per cow−1 and year−1. This can be achieved by lower GHG emissions at the same level of feed-efficiency (kg of ECM produced per kg of DMI) (Drews et al., 2020). This was also demonstrated in our study, where IC and FG showed a similar PCF despite the large differences in milk yields per cow and the good herd performance in IC with a low replacement rate. Lorenz et al. (2019) further found that an increase in milk yield in grazing systems would show a higher GHG mitigation per kg ECM in comparison with additional milk yield increases in confinement systems, as the latter are already operating on a high production level. In our study the difference in PCF between FG and IFG accounted for 200 g CO2eq per kg ECM milk, which is in line with the values reported by Lorenz et al. (2019), who predicted a GHG mitigation of 120 g CO2eq due to an increase of animal performance by ~1t ECM cow−1 year−1 for low-cost grazing systems. With regards to the supplementary feed intake, the FGI showed a higher share compared to FG with 18 vs. 3%. Thus, it can be assumed that the FGI system, within its defined thresholds, is almost producing on its lowest level the potential PCF. In contrast, the mitigation potential by milk yield increases in IC was estimated to be 60 g CO2eq kg ECM−1, which provided already 35% of the daily DM-intake by supplementary feeds. The SC system showed the highest PCF in our study as a result of the poor feeding strategy and high N-losses. Using the production parameters on farm as an indicator, the SC system represents a business-as-usual scenario, with a milk yield and replacement rate comparable to a larger farm survey in this area (Drews et al., 2020). In comparison, the IC, FG and IFG represent more specialized systems of either intensive confinement or intensive grazing. These farms showed lower replacement rates and higher forage quality, indicating that specialized systems have higher management skills ensuring a higher resource efficiency and hence a lower PCF.
Further GHG mitigations were achieved on all farms when soil C-sequestration was also considered. Permanent grassland and leys offer the opportunity to sequester carbon, which on sandy loam soils has been reported to be as high as ~4.8 t CO2eq ha−1 year−1 (Loges et al., 2018a). However, frequent soil tillage due to grassland renovation or land-use change to other forage crops can substantially reduce the potential C-sequestration to a lower level (Loges et al., 2018a; Reinsch et al., 2018a). Thus, after 2 years of ley farming most of the sequestered carbon can be lost during the following arable phase, if non-appropriate soil tillage practices are used. This somehow increases the uncertainty in evaluation ICLS (Reinsch et al., 2021) and potentially overestimates the sequestration rate in IFG as a high share of leys were present. However, the same uncertainty is given for permanent grassland in IC and SC as old swards with a high external N-input are likely to represent a C source rather than a considerable sink (Poyda et al., 2021). Additional C-sequestration was achieved by the return of animal excreta, regardless of whether slurry was applied or the deposition of dung from grazing animals were considered, the recycling of C and nutrients increases biomass yields and thus higher allocation of C plant residues. Loges et al. (2018a) found that young permanent grass-clover swards, when managed under cutting and fertilized with a moderate application rate of cattle slurry, will increase the C-sequestration by about 500 kg C ha−1 in comparison with management without slurry-N (Loges et al., 2018a). This rate is in line with a maximum predicted sequestration rate of 560 kg C ha−1 in IFG. Even though for permanent systems the duration of highly positive sequestration rates is limited, even after 20 years no equilibrium is reached on sandy loamy soils (Reinsch et al., 2018, Reinsch et al., 2021). The soil C-sequestration on external farms due to the exported slurry was ignored in this study, thereby likely underestimating the GHG savings from manure exports, which was only accounted for by crediting the substitution of mineral fertilizer using the nutrient contents in the slurry as a reference. Further crediting was applied for the IFG as plant mineralizable-N can be transferred to the integrated cropping system by using the same piece of land. The efficiency of plant removals is dependent on the soil tillage management and the post-cropping systems, whereas removal of leys in spring guarantees a higher N-use efficiency (Biernat et al., 2020b). However, plowing of grass-clover leys can cause distinct N2O-peaks as N can become enriched in the upper soil layer at warmer temperatures that coincide with low rainfall during spring (Reinsch et al., 2018b). Consequently, such credits have to be taken with care, as the potential substitution of mineral fertilizers, which we accounted for in the subsequent cropping system, relies on minimal N-losses. Costa et al. (2020) reviewed 3,180 articles on the effect of integrating legumes within crop rotations and found considerable knowledge gaps in taking the legume-N carry-over effect into account in life-cycle-assessment (LCA) studies. They recommend that full crop rotations should be evaluated rather than to focus on only one crop. This applies also to ICLS and further research is needed to apply evidence-based results of cash crop producers (Biernat et al., 2020a,b) to our evaluated dairy systems. However, we argue that this credit gives the maximum threshold of a best-practice approach achieving a PCF+soil carbon+credits of 0.6 kg CO2eq kg ECM−1, indicating a mitigation potential of 600 g CO2eq kg ECM−1 along the gradient of SC>IC>FG>IFG on sandy loamy soils. It has to be noted that the PCF calculation did not include emissions from produced infrastructure (e.g., machinery, cowshed). In grazing systems there is lower requirement for capital goods, of which the FG and IFG systems would further benefit in terms of their resource use. However, these investments need only to be considered for a specific duration of PCF calculation and therefore it depends on the age of the investments and their lifespan. Therefore, estimates on capital goods are difficult and are not influenced by the management strategy in the short term, and for these reasons are often not considered in LCA studies (Yan et al., 2011).
Farm-N-Balance and Losses of Reactive Nitrogen
The region where the study was conducted currently accounts for around 1 Mio. ha of agricultural land, which is dominated by specialized intensive arable cropping and dairy systems. Taube et al. (2015) estimated that in districts in this region, where dairy units are typically located, the average farm-N-balance exceeds 150 kg N ha−1. Several programmes were released to counteract such high N-surpluses. The main foci during the last years have included fertilizer planning recommendations, legal adjustments in the fertilizer regulation, and efforts to strengthen the N-exports from animal husbandries to the regions with intensive cash-crop production. However, latest reports show that these attempts are not successful in reducing the N-surplus in the dairy sector significantly (Henning and Taube, 2020). The main reason for this is that animal manures are prone to high N-losses by NH3-volatilization. Thus, the majority of N-losses in the IC and SC systems occurred due to the manure management. Mitigation can be achieved on the farm by manure storage covers and low emission spreading techniques (Maris et al., 2021) or slurry acidification (Seidel et al., 2017). However, mitigation of NH3- would increase the N-content in applied slurry, which in turn decreases the possible application amount of manure on the farm itself and thus requires export, which is often limited by large transport distances. Another option is to reduce the amount of mineral fertilizer-N. This can be sought by the mentioned efficient use of animal manures (which in the EU is restricted to 170 kg N ha−1) and the use of legumes. Even though BNF is an efficient way to capture N in crops, it represents an N-input in the system, which has to be considered in the fertilizer planning. Otherwise, the farm-N-balance cannot be improved significantly. The Baltic Marine Environment Protection Commission (HELCOM) further suggests that the share of grazing should be increased to reduce NH3 pollutants to marine bodies. Due to these explained causalities, the farm-N-balance and total N-losses decreased with the share of grazing and reduced fertilizer inputs. Accordingly, the nitrogen-use efficiency (NUE) for IC, SC, FG and IFG is 34, 24, 40, and 72%. These figures are in the range found by other authors (Löw et al., 2020), although Löw et al. (2020) also found slightly increasing NUE for confined systems in comparison with grazing. Their finding mainly relies on their particular circumstances, as low-cost grazing systems using forage legumes as the N source were not taken into account, making mineral N-imports of ~178 kg N ha−1 necessary. In contrast, in our investigated system FG and IFG showed self-sufficiency of N as a result of BNF. Despite the absence of mineral fertilizer use and high share of grazing the FG systems still revealed a farm N-balance of 94 kg N ha−1. However, due to the high share of pastures a high share of the farm surplus is related to N stock changes in organic matter, rather than to losses of reactive N to the environment, which were quantified as <65 kg N ha−1 year−1 in FG. With regards to the calculated NH3- losses on pastures the current IPCC factor of 0.2 for N-excretion and slurry was applied. The majority of NH4-N, which after its deposition is prone to NH3-voltalization to the air, is present in the excreted urine. The DM content of urine (~1% DM) is low in comparison to slurry (~8% DM) ensuring a fast infiltration into the soil, where further NH3-losses are avoided. However, using the milk urea content as a proxy, highest values were found for the FG system, making a potential overestimate of NH3 questionable. Further reductions could be achieved by an optimized feeding strategy (see section Forage Productivity) or urease inhibitors. Nevertheless, limits in further reduction are reached as N-residuals enriched by BNF accumulate, if high shares of legumes are sought. Further mitigation can be achieved in the ICLS, where the carry-over effect of legume-N can be used by subsequent crops as illustrated in the IFG.
Pros and Cons of the Examined Systems
In our comparison of four potential dairy production systems based on farm management data, measurement results and empirical methods, the IFG systems as examples for and ICLS (level iii) approach performed best with regards to the environmental impacts per ha and per product unit but also on a very high-level regarding land use efficiency. At the same time adequate milk yields and forage yields were generated, which were comparable to SC systems. This was mainly provided by the low resource use of external inputs and high forage yields from grazed grass-clover leys. The additional credit of N-carry-over to a potential cash-crop system increased these benefits further and might have positive effects on the cash-crop system as well, if the N status in the soil as a result of the advantageous pre-crop (2 years of leys) are taken into account in the fertilizer planning of the cash crop producers. In comparison, farms that are focused exclusively on dairy, such as IC, SC, and FG, are not capable of reducing their N-surplus below 90 kg N ha−1 because of the continued N-accumulation in the soil and volatile N-losses. Comparing the environmental impacts from specialist pure confinement or full-grazing, the IC system showed on the one hand a higher N-surplus and N-footprint per ECM milk compared to the low-cost FG, but only slight differences in the PCF. This implies a higher negative impact on a regional scale (e.g., on groundwater and surface water quality) in the IC but the differences considered on a global scale (i.e., potential influences on climate change) are negligible. However, several uncertainties have to be considered as the environmental impacts from external resources, e.g., imported supplementary feeds, can show wide differences depending on its origin (e.g., deforestation issues), which cannot be controlled at the regional level or by the producers themselves, indicating a higher uncertainty of results for IC and SC compared to FG. Nevertheless, according to the results obtained here and in the context of sandy loam soils, further improvements at regional and global levels can only achieved if N and C-cycles are coupled better by integrating dairy farming into an ICLS (Soussana and Lemaire, 2014).
Highlighting the disadvantages of an ICLS in combination with low-cost grazing, it has to be considered that the milk yield per ha was considerably lower compared with that of the IC. This can lead to income losses, though this will depend on the milk price and feed costs. At present, specialist IC systems in combination with large herd sizes (economy of scale) may have a higher potential to compete financially with the world milk market. In comparison, average dairy farms such as SC currently having a negative turn-over (after deducing of feed costs) (LKSH, 2019). However, low cost-grazing systems provide forage at low costs resulting in high revenue per kg milk solid (White et al., 2002). Continued technical innovation increasing animal performance from grazed grass, increasing herd genetic potential and developing labor efficient lower fixed-cost systems are essential in this context (Dillon et al., 2008). Moreover, dairy production in the EU also relies on subsidies. With regards to the current CAP, environmental indicators (e.g., GHG emissions, farm N-surplus, agro-biodiversity, and animal welfare) are becoming of increasing importance in order to achieve the additional public payments (EU, 2021). This may, in turn, increase the farm revenue considerably, if environmental services linked to dairy production are fulfilled. Beyond these policy developments, there is also a continuous increase in consumer acceptance of sustainably produced dairy products, and increased readiness by consumers to pay a higher price for labeled produce that reflects this (Kühl et al., 2017). However, it also has to be highlighted that specialized confinement systems are less dependent on soil physical and soil chemical properties, as the utilization of grass and maize by cutting is generally easier than managing intensive rotational grazing. In addition, high grazing yields can be only maintained if soils and swards are not easily damaged by overgrazing or trampling, even when high stocking rates are necessary to fulfill the economic requirements for productivity per ha. Moreover, water availability during summer plays a crucial role as yield losses due to drought would make undesired feed purchases necessary, and thereby reduce the efficiency of the proposed grazing systems. Irrigation as an alternative solution would require investment and processing cost as well as questionable resource efficiency in areas where access to groundwater supplies is limited (Emadodin and Reinsch, 2018). Moreover, irrigation in grazing systems can increase undesired GHG emissions from pasture land considerably (Smit et al., 2020). Therefore, specialized IC systems will continue to play an important role in the future on the export markets, and structural change is expected to continue to disadvantage the SC system. There are additional constraints, if ICLS systems for dairy are sought at high use intensities. The claimed high milk yields at balanced CP/NEL ratios can make supplementary feeding necessary. On the one hand they could be produced locally; for instance, in the IFG system cereals for energy supplementation were provided in the cash-crop unit. On the other hand, however, increasing land consumption would accelerate local competition for food vs. feed. In comparison, most confinement systems in northwest Europe import a high share canola meal, which is a by-product of the oil industry (Broderick et al., 2015). van Hal et al. (2019) mentioned that the use of by-products, which are unsuitable for direct human consumption, increases the overall efficiency of a system even in the LCA perspective. Thus, the evaluated IFG system in our study needs further improvements as more then 80% of the supplementary feeds were provided from locally grown cereals. More maize grown for silage instead of cereals as an additional crop rotation segment might be an appropriate option regarding land use efficiency; however, on the basis of this study integrated dairy systems in the cash-crop sector may provide several positive opportunities (Figure 4) and should be implemented into the local policy.
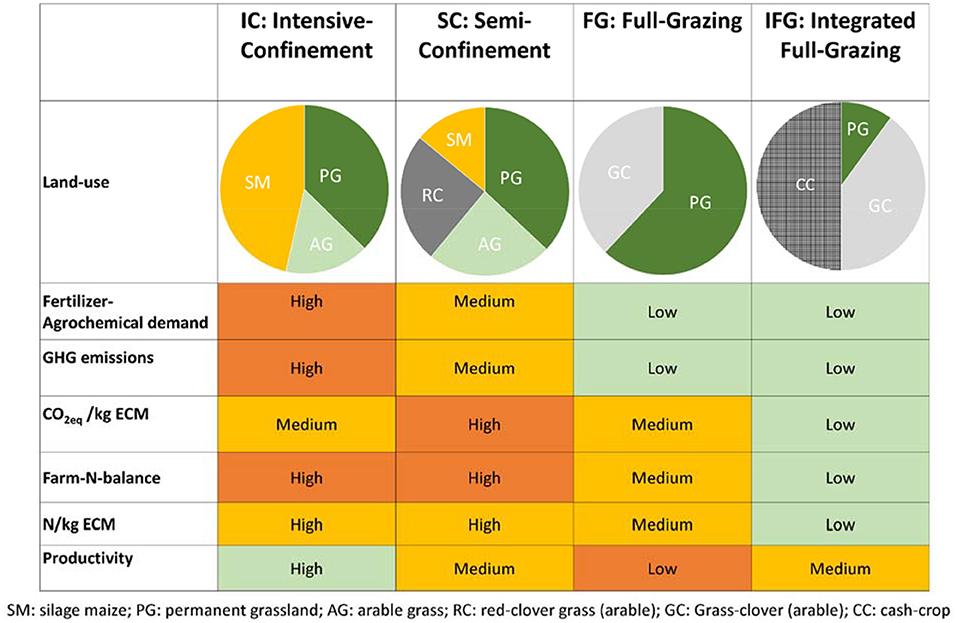
Figure 4. Overview of different ecosystem services and productivity provided by the different land-use types and resource efforts in the investigated prevailing dairy systems.
Conclusion
Considering the environmental goals of the EU (e.g., “Green Deal”) for the agricultural sector, there is a need to identify best strategies for agricultural land use linked to dairy systems. An important question concerns the current dominant high input/high out models, and whether they are appropriate for the land-use challenges of minimizing carbon and nitrogen footprints and for maintaining appropriate levels of biodiversity. The on-farm research study on four farms representing different strategies for land use and milk production on sandy loam soils in northwest Germany has confirmed that ongoing intensification in the dairy sector is not in line with the need for reductions in GHG and nitrogen emissions per kg ECM produced on the regional level and thus not in line with ecological intensification. Full-grazing systems in areas with adequate rainfall and appropriate soil conditions offer the opportunity to improve the overall efficiency but only when low input systems are sought. However, the farm-N-balance as well as impacts on climate change can be improved further if integrated systems are favored. This requires exchanging by-products and soil fertility in the context of a local spatial distribution and by making all arable systems more resilient. This study provides a first step toward further analysis and extrapolation regarding the economic resilience of the proposed system in the future.
Data Availability Statement
The original contributions presented in the study are included in the article/supplementary material, further inquiries can be directed to the corresponding author/s.
Author Contributions
FT, RL, and TR: conceptualization. TR and CL: writing original draft preparation. CK and TR: data analysis. IV, FT, CSM, and RL: writing—review and editing. CK: visualization. FT: supervision. FT and RL: funding acquisition. All authors contributed to the article and approved the submitted version.
Funding
This project was partly financed by the European Union Interreg IVA programme, Resource Efficiency and Management Optimization in Dairy Farming (47-2.2-09) and from SusAn, an ERA-Net co-funded under European Union's Horizon 2020 research and innovation program, Grant Agreement no. 696231.
Conflict of Interest
The authors declare that the research was conducted in the absence of any commercial or financial relationships that could be construed as a potential conflict of interest.
Acknowledgments
We thank Mr. Lehmann, Mr. Köpke, and Mr. Riecken and their families for provision of their farm lands for experimental purposes. Further we thank Mirja Kämper, Rita Kopp, Keanu Heuck, and Thomas Ehmsen for their support in intensive management of the field plots as well as Philipp Schönbach and Sabine Mues for their assistance in the data compilation and analysis. Moreover, we thank Heike Lorenz, Arne Poyda, and Alan Hopkins for valuable assistance during the manuscript preparation.
References
Akert, F. S., Dorn, K., Frey, H., Hofstetter, P., Berard, J., Kreuzer, M., et al. (2020). Farm-gate nutrient balances of grassland-based milk production systems with full- or part-time grazing and fresh herbage indoor feeding at variable concentrate levels. Nutr. Cycl. Agroecosyst. 117, 383–400. doi: 10.1007/s10705-020-10072-y
Allan, E., Bossdorf, O., Dormann, C. F., Prati, D., Gossner, M. M., Tscharntke, T., et al. (2014). Interannual variation in land-use intensity enhances grassland multidiversity. Proc. Natl. Acad. Sci. U.S.A. 111, 308–313. doi: 10.1073/pnas.1312213111
Augère-Granier, M. (2018). “The EU dairy sector. Main features, challenges and prospects,” in European Parliament Briefings. Brussels: European Commission. Available online at: http://www.europarl.europa.eu/RegData/etudes/BRIE/2018/630345/EPRS_BRI(2018)630345_EN.pdf
Barnes, A. P., Lucas, A., and Maio, G. (2016). Quantifying ambivalence towards sustainable intensification: an exploration of the UK public's values. Food Sec. 8, 609–619. doi: 10.1007/s12571-016-0565-y
Biernat, L., Taube, F., Loges, R., Klu,ß, C., and Reinsch, T. (2020a). Nitrous oxide emissions and methane uptake from organic and conventionally managed arable crop rotations on farms in Northwest Germany. Sustainability 12:3240. doi: 10.3390/su12083240
Biernat, L., Taube, F., Vogeler, I., Reinsch, T., Klu,ß, C., and Loges, R. (2020b). Is organic agriculture in line with the EU-Nitrate directive? On-farm nitrate leaching from organic and conventional arable crop rotations. Agric. Ecosyst. Environ. 298:106964. doi: 10.1016/j.agee.2020.106964
Bluwstein, J., Braun, M., and Henriksen, C. B. (2015). Sustainable extensification as an alternative model for reducing GHG emissions from agriculture. the case of an extensively managed organic farm in Denmark. Agroecol. Sust. Food. 39, 551–579. doi: 10.1080/21683565.2015.1013240
Broderick, G. A., Faciola, A. P., and Armentano, L. E. (2015). Replacing dietary soybean meal with canola meal improves production and efficiency of lactating dairy cows. J. Dairy Sci. 98, 5672–5687. doi: 10.3168/jds.2015-9563
Buckwell, A. (2014). The Sustainable Intensification of European Agriculture: A Review Sponsored by the RISE Foundation. Brussels: Rural Investment Support for Europe.
Burgos, S. A., Embertson, N. M., Zhao, Y., Mitloehner, F. M., DePeters, E. J., and Fadel, J. G. (2010). Prediction of ammonia emission from dairy cattle manure based on milk urea nitrogen: relation of milk urea nitrogen to ammonia emissions. J. Dairy Sci. 93, 2377–2386. doi: 10.3168/jds.2009-2415
Capper, J. L., Cady, R. A., and Bauman, D. E. (2009). The environmental impact of dairy production: 1944 compared with 2007. J. Anim. Sci. 87, 2160–2167. doi: 10.2527/jas.2009-1781
Chen, S. M., Lin, S., Loges, R., Reinsch, T., Hasler, M., and Taube, F. (2016). Independence of seasonal patterns of root functional traits and rooting strategy of a grass-clover sward from sward age and slurry application. Grass Forage Sci. 71, 607–621. doi: 10.1111/gfs.12222
Christie, K. M., Gourley, C. J. P., Rawnsley, R. P., Eckard, R. J., and Awty, I. M. (2012). Whole-farm systems analysis of Australian dairy farm greenhouse gas emissions. Anim. Prod. Sci. 52:998. doi: 10.1071/AN12061
Cichota, R., Snow, V. O., Vogeler, I., Wheeler, D. M., and Shepherd, M. A. (2012). Describing N leaching from urine patches deposited at different times of the year with a transfer function. Soil Res. 50:694. doi: 10.1071/SR12208
Connolly, J., Sebastià, M.-T., Kirwan, L., Finn, J. A., Llurba, R., Suter, M., et al. (2018). Weed suppression greatly increased by plant diversity in intensively managed grasslands: a continental-scale experiment. J. Appl. Ecol. 55, 852–862. doi: 10.1111/1365-2664.12991
Cook, S., Silici, L., Adolph, B., and Darrah, S. (2015). Sustainable Intensification Revisited. London: IIED Issue Paper. IIED.
Costa, M. P., Chadwick, D., Saget, S., Rees, R. M., Williams, M., and Styles, D. (2020). Representing crop rotations in life cycle assessment: a review of legume LCA studies. Int. J. Life Cycle Assess. 25, 1942–1956. doi: 10.1007/s11367-020-01812-x
Dalgaard, R., Schmidt, J., Halberg, N., Christensen, P., Thrane, M., and Pengue, W. A. (2008). LCA of soybean meal. Int. J. Life Cycle Assess. 13, 240–254. doi: 10.1065/lca2007.06.342
Davies, B., Baulcombe, D., Crute, I., Dunwell, J., Gale, M., Jones, J., et al. (2009). Reaping the Benefits: Science and the Sustainable Intensification of Global Agriculture (London: Royal Society).
Destatis (2017). Statistische Berichte / C / I / C / II [SH]: Bodennutzung und Ernte in Schleswig Holstein. Available online at: https://www.statistischebibliothek.de/mir/receive/SHSerie_mods_00000006 (accessed May 8, 2021).
Dillon, P., Hennessy, T., Shalloo, L., Thorne, F., and Horan, B. (2008). Future outlook for the Irish dairy industry: a study of international competitiveness, influence of international trade reform and requirement for change. Int. J. Dairy Technol. 61, 16–29. doi: 10.1111/j.1471-0307.2008.00374.x
Drews, J., Czycholl, I., and Krieter, J. (2020). A life cycle assessment study of dairy farms in northern Germany: the influence of performance parameters on environmental efficiency. J. Environ. Manage. 273:111127. doi: 10.1016/j.jenvman.2020.111127
Ebeling, A., Klein, A.-M., Schumacher, J., Weisser, W. W., and Tscharntke, T. (2008). How does plant richness affect pollinator richness and temporal stability of flower visits? Oikos 117, 1808–1815. doi: 10.1111/j.1600-0706.2008.16819.x
EC (2020). Stepping Up Europe's 2030 Climate Ambition. Brussels: European Commission. Available online at: https://eur-lex.europa.eu/legal-content/EN/TXT/?uri=CELEX:52020DC0562 (accessed May 8, 2021).
Emadodin, I., and Reinsch, T. (2018). Assessing the impact of land use change on aridification in semiarid land. Land Degrad. Dev. 29, 3423–3431. doi: 10.1002/ldr.3108
EU (2021). Commission Publishes List of Potential Eco-Schemes. Agriculture and Rural Development European Comission.
Eurostat (2018). Livestock Density by NUTS 2 Regions, EU-28, 2016 (livestock units per hectare utilised agricultural area) (Luxemburg).
Eurostat (2020). Dairy Cows, Milk Production and the Apparent Milk Yield, 2018 (dairy cow numbers; raw cows' milk tonnes). Statistics Explained - Milk and Milk Product Statistics (Luxemburg).
FAOSTAT (2017). Crop Statistics. Food and Agriculture Organization of the United Nations (FAO). Available online at: http://www.fao.org/faostat/en/#data/QC (accessed May 8, 2021).
Fowler, D., Coyle, M., Skiba, U., Sutton, M. A., Cape, J. N., Reis, S., et al. (2013). The global nitrogen cycle in the twenty-first century. Phil. Trans. R. Soc. B 368:20130164. doi: 10.1098/rstb.2013.0164
Garnett, T., Appleby, M. C., Balmford, A., Bateman, I. J., Benton, T. G., Bloomer, P., et al. (2013). Sustainable intensification in agriculture: premises and policies. Science 341, 33–34. doi: 10.1126/science.1234485
Gerber, P., Vellinga, T., Opio, C., and Steinfeld, H. (2011). Productivity gains and greenhouse gas emissions intensity in dairy systems. Livestock Sci. 139, 100–108. doi: 10.1016/j.livsci.2011.03.012
Godfray, H. C. J., and Garnett, T. (2014). Food security and sustainable intensification. Phil. Trans. R. Soc. B 369:20120273. doi: 10.1098/rstb.2012.0273
Gruber, L., Schwarz, F. J., Erdin, D., Fischer, B., Steingass, H., Meyer, U., et al. (2004). Vorhersage der Futteraufnahme von Milchkühen - Datenbasis von 10 Forschungs- und Universitätsinstituten Deutschlands, Österreichs und der Schweiz. VDLUFA-Kongress. Rostock: VDLUFA, 484–504. Available online at: https://www.vdlufa.de/download/Kongressband_2004.pdf
Henning, C. H. C. A., and Taube, F. (2020). “Zweiter Nährstoffbericht des Landes Schleswig-Holstein im Auftrag des Ministeriums für Energiewende, Landwirtschaft, Umwelt, Natur und Digitalisierung des Landes Schleswig-Holstein (MELUND)”. Available online at: https://www.schleswig-holstein.de/DE/Fachinhalte/G/grundwasser/Downloads/naehrstoffbericht_2020.pdf (accessed May 8, 2021).
Herrmann, A., Kelm, M., Kornher, A., and Taube, F. (2005). Performance of grassland under different cutting regimes as affected by sward composition, nitrogen input, soil conditions and weather—a simulation study. Europ. J. Agron. 22, 141–158. doi: 10.1016/j.eja.2004.02.002
Heublein, C., Dohme-Meier, F., Südekum, K. H., Bruckmaier, R. M., Thanner, S., and Schori, F. (2017). Impact of cow strain and concentrate supplementation on grazing behaviour, milk yield and metabolic state of dairy cows in an organic pasture-based feeding system. Animal 11, 1163–1173. doi: 10.1017/S1751731116002639
Høgh-Jensen, H., Loges, R., Jørgensen, F. V., Vinther, F. P., and Jensen, E. S. (2004). An empirical model for quantification of symbiotic nitrogen fixation in grass-clover mixtures. Agric. Syst. 82, 181–194. doi: 10.1016/j.agsy.2003.12.003
Höglind, M., Thorsen, S. M., and Semenov, M. A. (2013). Assessing uncertainties in impact of climate change on grass production in Northern Europe using ensembles of global climate models. Agric. For. Meteorol. 170, 103–113. doi: 10.1016/j.agrformet.2012.02.010
Holzschuh, A., Steffan-Dewenter, I., Kleijn, D., and Tscharntke, T. (2007). Diversity of flower-visiting bees in cereal fields: effects of farming system, landscape composition and regional context. J. Appl. Ecol. 44, 41–49. doi: 10.1111/j.1365-2664.2006.01259.x
Huss-Danell, K., Chaia, E., and Carlsson, G. (2007). N2 fixation and nitrogen allocation to above and below ground plant parts in red clover-grasslands. Plant Soil. 299, 215–226. doi: 10.1007/s11104-007-9376-4
IPCC (2006). 2006 IPCC Guidelines for National Greenhouse Gas Inventories, Prepared by the National Greenhouse Gas Inventories Programme, eds H. S. Eggleston, L. Buendia, K. Miwa, T. Ngara and K. Tanabe (IGES). Available online at: https://www.ipcc-nggip.iges.or.jp/public/2006gl/ (accessed May 8, 2021).
IPCC (2019). 2019 Refinement to the 2006 IPCC Guidelines for National Greenhouse Gas Inventories, eds E. Calvo Buendia, K. Tanabe, A. Kranjc, J. Baasansuren, M. Fukuda, S. Ngarize, et al. IPCC. Available online at: https://www.ipcc-nggip.iges.or.jp/public/2019rf/ (accessed May 8, 2021).
ISO (2014). Milk and Milk Products - Determination of Nitrogen Content - Part 1: Kjeldahl Principle and Crude Protein Calculation (ISO 8968-1:2014). Geneva: Beuth publishing DIN.
Keating, B. A., Carberry, P. S., Bindraban, P. S., Asseng, S., Meinke, H., and Dixon, J. (2010). Eco-efficient agriculture: concepts, challenges, and opportunities. Crop Sci. 50, 109–119. doi: 10.2135/cropsci2009.10.0594
Kleijn, D., Kohler, F., Báldi, A., Batáry, P., Concepción, E. D., Clough, Y., et al. (2009). On the relationship between farmland biodiversity and land-use intensity in Europe. Proc. R. Soc. B 276, 903–909. doi: 10.1098/rspb.2008.1509
Komainda, M., Taube, F., Kluß, C., and Herrmann, A. (2018). Effects of catch crops on silage maize (Zea mays L.): yield, nitrogen uptake efficiency and losses. Nutr. Cycl. Agroecosyst. 110, 51–69. doi: 10.1007/s10705-017-9839-9
Kühl, S., Gassler, B., and Spiller, A. (2017). Labeling strategies to overcome the problem of niche markets for sustainable milk products: the example of pasture-raised milk. J. Dairy Sci. 100, 5082–5096. doi: 10.3168/jds.2016-11997
Kuhn, T., Kokemohr, L., and Holm-Müller, K. (2018). A life cycle assessment of liquid pig manure transport in line with EU regulations: a case study from Germany. J. Environ. Manage. 217, 456–467. doi: 10.1016/j.jenvman.2018.03.082
Ledgard, G. (2013). Land Use Change in the Southland Region. Environment Southland Technical Report. Invercargill: Environment Southland.
Lemaire, G., Gastal, F., Franzluebbers, A., and Chabbi, A. (2015). Grassland–cropping rotations: an avenue for agricultural diversification to reconcile high production with environmental quality. Environ. Manage. 56, 1065–1077. doi: 10.1007/s00267-015-0561-6
Lesschen, J. P., van den Berg, M., Westhoek, H. J., Witzke, H. P., and Oenema, O. (2011). Greenhouse gas emission profiles of European livestock sectors. Anim. Feed Sci. Technol. 166–167, 16–28. doi: 10.1016/j.anifeedsci.2011.04.058
Leterme, P., Nesme, T., Regan, J., and Korevaar, H. (2019). “Environmental benefits of farm- and district-scale crop-livestock integration,” in Agroecosystem Diversity (Elsevier), 335–349. doi: 10.1016/B978-0-12-811050-8.00021-2
Li, D., Lanigan, G., and Humphreys, J. (2011). Measured and simulated nitrous oxide emissions from ryegrass- and ryegrass/white clover-based grasslands in a moist temperate climate. PLoS ONE 6:e26176. doi: 10.1371/journal.pone.0026176
LKSH (2019). Ergebnisse der Vollkostenrechung der Rinderspezialberatung in Schleswig-Holstein. Landwirtschaftskammer Schleswig-Holstein (LKSH). Available online at: https://www.lksh.de/fileadmin/PDFs/Landwirtschaft/Tier/Rinder_Report_2018.pdf (accessed May 8, 2021).
Loges, R., Bunne, I., Reinsch, T., Malisch, C., Kluß, C., Herrmann, A., et al. (2018a). Forage production in rotational systems generates similar yields compared to maize monocultures but improves soil carbon stocks. Eur. J. Agron. 97, 11–19. doi: 10.1016/j.eja.2018.04.010
Loges, R., Mues, S., Kluß, C., Reinsch, T., Lorenz, H., Humphreys, J., et al. (2018b). Eco-efficient milk production in northern Germany inspired by the Irish rotational grazing system. Sustainable meat and milk production from grasslands. Grassland Sci. Eur. 23, 577–579.
Löpmeier, F. J. (1994). Berechnung der bodenfeuchte und verdunstung mittels agrarme-teorologischer modelle. Zeitschrift für Bewässerungswirtschaft 29, 157–167.
Lorenz, H., Reinsch, T., Hess, S., and Taube, F. (2019). Is low-input dairy farming more climate friendly? A meta-analysis of the carbon footprints of different production systems. J. Clean. Prod. 211, 161–170. doi: 10.1016/j.jclepro.2018.11.113
Lorenz, H., Reinsch, T., Kluß, C., Taube, F., and Loges, R. (2020). Does the admixture of forage herbs affect the yield performance, yield stability and forage quality of a grass clover ley? Sustainability 12:5842. doi: 10.3390/su12145842
Löw, P., Karatay, Y. N., and Osterburg, B. (2020). Nitrogen use efficiency on dairy farms with different grazing systems in northwestern Germany. Environ. Res. Comm. 2:105002. doi: 10.1088/2515-7620/abc098
Loza, C., Reinsch, T., Loges, R., Taube, F. G., J, Kluß, C., et al. (2021). Methane emission and milk production from jersey cows grazing perennial ryegrass-white clover and multispecies forage mixtures. Agriculture 11:175. doi: 10.3390/agriculture11020175
Lüscher, A., Mueller-Harvey, I., Soussana, J. F., Rees, R. M., and Peyraud, J. L. (2014). Potential of legume-based grassland-livestock systems in Europe: a review. Grass Forage Sci. 69, 206–228. doi: 10.1111/gfs.12124
MacLaren, C., Storkey, J., Strauss, J., Swanepoel, P., and Dehnen-Schmutz, K. (2019). Livestock in diverse cropping systems improve weed management and sustain yields whilst reducing inputs. J. Appl. Ecol. 56, 144–156. doi: 10.1111/1365-2664.13239
Maris, S. C., Abalos, D., Capra, F., Moscatelli, G., Scaglia, F., Cely Reyes, G. E., et al. (2021). Strong potential of slurry application timing and method to reduce N losses in a permanent grassland. Agric. Ecosyst. Environ. 311:107329. doi: 10.1016/j.agee.2021.107329
Martin, G., Durand, J.-L., Duru, M., Gastal, F., Julier, B., Litrico, I., et al. (2020). Role of ley pastures in tomorrow's cropping systems. A review. Agron. Sustain. Dev. 40:17. doi: 10.1007/s13593-020-00620-9
Merbach, W., Garz, J., Schliephake, W., Stumpe, H., and Schmidt, L. (2000). The long-term fertilization experiments in Halle (Saale), Germany - Introduction and survey. J. Plant Nutr. Soil Sci. 163, 629–638. doi: 10.1002/1522-2624(200012)163:6<629::AID-JPLN629>3.0.CO;2-P
Misselbrook, T. H., Van Der Weerden, T. J., Pain, B. F., Jarvis, S. C., Chambers, B. J., Smith, K. A., et al. (2000). Ammonia emission factors for UK agriculture. Atmos. Environ. 34, 871–880. doi: 10.1016/S1352-2310(99)00350-7
Mogensen, L., Kristensen, T., Nguyen, T. L. T., Knudsen, M. T., and Hermansen, J. E. (2014). Method for calculating carbon footprint of cattle feeds – including contribution from soil carbon changes and use of cattle manure. J. Clean. Prod. 73, 40–51. doi: 10.1016/j.jclepro.2014.02.023
Mohrlok, U. (2009). Bilanzmodelle in der Grundwasserhydraulik. Habilitation thesis, Karlsruhe University, Germany.
Moraine, M., Duru, M., Nicholas, P., Leterme, P., and Therond, O. (2014). Farming system design for innovative crop-livestock integration in Europe. Animal 8, 1204–1217. doi: 10.1017/S1751731114001189
Mueller, N. D., Gerber, J. S., Johnston, M., Ray, D. K., Ramankutty, N., and Foley, J. A. (2012). Closing yield gaps through nutrient and water management. Nature 490, 254–257. doi: 10.1038/nature11420
Nennich, T. D., Harrison, J. H., VanWieringen, L. M., Meyer, D., Heinrichs, A. J., Weiss, W. P., et al. (2005). prediction of manure and nutrient excretion from dairy cattle. J. Dairy Sci. 88, 3721–3733. doi: 10.3168/jds.S0022-0302(05)73058-7
Nevens, F., and Reheul, D. (2003). Permanent grassland and 3-year leys alternating with 3 years of arable land: 31 years of comparison. Europ. J. Agron. 19, 77–90. doi: 10.1016/S1161-0301(02)00021-7
Nyameasem, J. K., Malisch, C. S., Loges, R., Taube, F., Kluß, C., Vogeler, I., et al. (2021). Nitrous oxide emission from grazing is low across a gradient of plant functional diversity and soil conditions. Atmosphere 12, 223–248. doi: 10.3390/atmos12020223
Nyfeler, D., Huguenin-Elie, O., Suter, M., Frossard, E., and Lüscher, A. (2011). Grass–legume mixtures can yield more nitrogen than legume pure stands due to mutual stimulation of nitrogen uptake from symbiotic and non-symbiotic sources. Agric. Ecosyst. Environ. 140, 155–163. doi: 10.1016/j.agee.2010.11.022
O'Brien, D., Brennan, P., Humphreys, J., Ruane, E., and Shalloo, L. (2014). An appraisal of carbon footprint of milk from commercial grass-based dairy farms in Ireland according to a certified life cycle assessment methodology. Int. J. Life Cycle Assess. 19, 1469–1481. doi: 10.1007/s11367-014-0755-9
Oenema, O., de Klein, C., and Alfaro, M. (2014). Intensification of grassland and forage use: driving forces and constraints. Crop Pasture Sci. 65:524. doi: 10.1071/CP14001
Petersen, B. M., Knudsen, M. T., Hermansen, J. E., and Halberg, N. (2013). An approach to include soil carbon changes in life cycle assessments. J. Clean. Prod. 52, 217–224. doi: 10.1016/j.jclepro.2013.03.007
Peterson, C. A., Deiss, L., and Gaudin, A. C. M. (2020). Commercial integrated crop-livestock systems achieve comparable crop yields to specialized production systems: a meta-analysis. PLoS ONE 15:e0231840. doi: 10.1371/journal.pone.0231840
Peyraud, J.-L., Taboada, M., and Delaby, L. (2014). Integrated crop and livestock systems in Western Europe and South America: a review. Eur. J. Agron. 57, 31–42. doi: 10.1016/j.eja.2014.02.005
Poyda, A., Reinsch, T., Struck, I. J., Skinner, R. H., Kluß, C., and Taube, F. (2021). Low assimilate partitioning to root biomass is associated with carbon losses at an intensively managed temperate grassland. Plant Soil 460, 31–50. doi: 10.1007/s11104-020-04771-2
Reheul, D., Cougnon, M., Kayser, M., Pannecoucque, J., Swanckaert, J., De Cauwer, B., et al. (2017). Sustainable intensification in the production of grass and forage crops in the Low Countries of north-west Europe. Grass Forage Sci. 72, 369–381. doi: 10.1111/gfs.12285
Reinsch, T., Loges, R., Klu,ß, C., and Taube, F. (2018a). Renovation and conversion of permanent grass-clover swards to pasture or crops: effects on annual N2O emissions in the year after ploughing. Soil Till Res. 175, 119–129. doi: 10.1016/j.still.2017.08.009
Reinsch, T., Loges, R., Kluß, C., and Taube, F. (2018b). Effect of grassland ploughing and reseeding on CO2 emissions and soil carbon stocks. Agric. Ecosyst. Environ. 265, 374–383. doi: 10.1016/j.agee.2018.06.020
Reinsch, T., Malisch, C., Loges, R., and Taube, F. (2020). Nitrous oxide emissions from grass–clover swards as influenced by sward age and biological nitrogen fixation. Grass Forage Sci. 75, 372–384. doi: 10.1111/gfs.12496
Reinsch, T., Struck, I. J. A., Loges, R., Kluß, C., and Taube, F. (2021). Soil carbon dynamics of no-till silage maize in ley systems. Soil Till Res. 209:104957. doi: 10.1016/j.still.2021.104957
Rockström, J., Steffen, W., Noone, K., Persson, Å., Chapin, F. S., Lambin, E., et al. (2009). Planetary boundaries exploring the safe operating space for humanity. Ecol. Soc. 14, 1–32. doi: 10.5751/ES-03180-140232
Rotz, C. A., Montes, F., and Chianese, D. S. (2010). The carbon footprint of dairy production systems through partial life cycle assessment. J. Dairy Sci. 93, 1266–1282. doi: 10.3168/jds.2009-2162
Ryschawy, J., Choisis, N., Choisis, J. P., Joannon, A., and Gibon, A. (2012). Mixed crop-livestock systems: an economic and environmental-friendly way of farming? Animal 6, 1722–1730. doi: 10.1017/S1751731112000675
Schiefer, J., Lair, G. J., and Blum, W. E. H. (2016). Potential and limits of land and soil for sustainable intensification of European agriculture. Agric. Ecosyst. Environ. 230, 283–293. doi: 10.1016/j.agee.2016.06.021
Schils, R., Philipsen, B., Hoekstra, N., Holshof, G., Zom, R., Hoving, I., et al. (2019). amazing grazing: a public and private partnership to stimulate grazing practices in intensive dairy systems. Sustainability 11:5868. doi: 10.3390/su11205868
Schils, R. L. M., Olesen, J. E., del Prado, A., and Soussana, J. F. (2007). A review of farm level modelling approaches for mitigating greenhouse gas emissions from ruminant livestock systems. Livestock Sci. 112, 240–251. doi: 10.1016/j.livsci.2007.09.005
Schmeer, M., Loges, R., Dittert, K., Senbayram, M., Horn, R., and Taube, F. (2014). Legume-based forage production systems reduce nitrous oxide emissions. Soil Till Res. 143, 17–25. doi: 10.1016/j.still.2014.05.001
Schulz, F., Westreicher-Kristen, E., Knappstein, K., Molkentin, J., and Susenbeth, A. (2018). Replacing maize silage plus soybean meal with red clover silage plus wheat in diets for lactating dairy cows. J. Dairy Sci. 101, 1216–1226 doi: 10.3168/jds.2017-13605
Seidel, A., Pacholski, A., Nyord, T., Vestergaard, A., Pahlmann, I., Herrmann, A., et al. (2017). Effects of acidification and injection of pasture applied cattle slurry on ammonia losses, N 2 O emissions and crop N uptake. Agric. Ecosyst. Environ. 247, 23–32. doi: 10.1016/j.agee.2017.05.030
Selbie, D. R., Buckthought, L. E., and Shepherd, M. A. (2015). The challenge of the urine patch for managing nitrogen in grazed pasture systems. Adv. Agron. 129, 229–292. doi: 10.1016/bs.agron.2014.09.004
Sirami, C., Gross, N., Baillod, A. B., Bertrand, C., Carrié, R., Hass, A., et al. (2019). Increasing crop heterogeneity enhances multitrophic diversity across agricultural regions. Proc. Natl. Acad. Sci. U.S.A. 116, 16442–16447. doi: 10.1073/pnas.1906419116
Sjaunja, L. O. (1990). A Nordic Proposal for an Energy-Corrected Milk (ECM) Formula. 27th Session International Committee for Recording and Productivity of Milk Animals. Paris.
Smit, H. P. J., Reinsch, T., Swanepoel, P. A., Kluß, C., and Taube, F. (2020). Grazing under irrigation affects N2O-emissions substantially in South Africa. Atmosphere 11:925. doi: 10.3390/atmos11090925
Sommer, S. G., Webb, J., and Hutchings, N. D. (2019). New emission factors for calculation of ammonia volatilization from European livestock manure management systems. Front. Sustain. Food Syst. 3:101. doi: 10.3389/fsufs.2019.00101
Soussana, J.-F., and Lemaire, G. (2014). Coupling carbon and nitrogen cycles for environmentally sustainable intensification of grasslands and crop-livestock systems. Agric. Ecosyst. Environ. 190, 9–17 doi: 10.1016/j.agee.2013.10.012
Spek, J. W., Dijkstra, J., Van Duinkerken, G., and Bannink, A. (2013). A review of factors influencing milk urea concentration and its relationship with urinary urea excretion in lactating dairy cattle. J. Agric. Sci. 151, 407–423. doi: 10.1017/S0021859612000561
Struck, I. J., Reinsch, T., Herrmann, A., Kluß, C., Loges, R., and Taube, F. (2019). Yield potential and nitrogen dynamics of no-till silage maize (Zea mays L.) under maritime climate conditions. Eur. J. Agron. 107, 30–42. doi: 10.1016/j.eja.2019.04.009
Struck, I. J. A., Taube, F., Hoffmann, M., Kluß, C., Herrmann, A., Loges, R., et al. (2020). Full greenhouse gas balance of silage maize cultivation following grassland: Are no-tillage practices favourable under highly productive soil conditions? Soil Till Res. 200:104615. doi: 10.1016/j.still.2020.104615
Struik, P. C., and Kuyper, T. W. (2017). Sustainable intensification in agriculture: the richer shade of green. a review. Agron. Sustain. Dev. 37:39. doi: 10.1007/s13593-017-0445-7
Suter, M., Connolly, J., Finn, J. A., Loges, R., Kirwan, L., Sebastià, M.-T., et al. (2015). Nitrogen yield advantage from grass-legume mixtures is robust over a wide range of legume proportions and environmental conditions. Glob. Change Biol. 21, 2424–2438. doi: 10.1111/gcb.12880
Taghizadeh-Toosi, A., Christensen, B. T., Hutchings, N. J., Vejlin, J., Kätterer, T., Glendining, M., et al. (2014). C-TOOL: a simple model for simulating whole-profile carbon storage in temperate agricultural soils. Ecol. Model. 292, 11–25. doi: 10.1016/j.ecolmodel.2014.08.016
Taube, F., Gierus, M., Hermann, A., Loges, R., and Schönbach, P. (2014). Grassland and globalization - challenges for north-west European grass and forage research. Grass Forage Sci. 69, 2–16. doi: 10.1111/gfs.12043
Taube, F., Henning, C., Albrecht, E., Reinsch, T., and Kluß, C. (2015). Nährstoffbericht des Landes Schleswig-Holstein 2016. Im Auftrag des Ministeriums für Energiewende, Landwirtschaft, Umwelt und ländliche Räume des Landes S-H. Available online at: https://www.schleswig-holstein.de/DE/Fachinhalte/G/grundwasser/Downloads/naehrstoffbericht.pdf (accessed May 8, 2021).
Tittonell, P. (2014). Ecological intensification of agriculture—sustainable by nature. Curr. Opin. Env. Sus. 8, 53–61. doi: 10.1016/j.cosust.2014.08.006
Torssell, B. W. R., and Kornher, A. (1983). Validation of a yield prediction model for temporary grasslands [Sweden]. Swed. J. Agri. Res. 13, 125–135.
Trott, H., Ingwersen, B., Wachendorf, M., and Taube, F. (2002). Estimation of dry matter yield on permanent grassland by means of height assessment. Pflanzenbauwissenschaften 6, 78–83.
van der Weerden, T. J., Noble, A. N., Luo, J., de Klein, C. A. M., Saggar, S., Giltrap, D., et al. (2020). Meta-analysis of New Zealand's nitrous oxide emission factors for ruminant excreta supports disaggregation based on excreta form, livestock type and slope class. Sci. Total Environ. 732:139235. doi: 10.1016/j.scitotenv.2020.139235
van Grinsven, H. J. M., Erisman, J. W., de Vries, W., and Westhoek, H. (2015). Potential of extensification of European agriculture for a more sustainable food system, focusing on nitrogen. Environ. Res. Lett. 10:025002. doi: 10.1088/1748-9326/10/2/025002
van Grinsven, H. J. M., Holland, M., Jacobsen, B. H., Klimont, Z., Sutton, M. A., and Jaap Willems, W. (2013). Costs and Benefits of Nitrogen for Europe and Implications for Mitigation. Environ. Sci. Technol. 47, 3571–3579. doi: 10.1021/es303804g
van Hal, O., Weijenberg, A. A. A., de Boer, I. J. M., and van Zanten, H. H. E. (2019). Accounting for feed-food competition in environmental impact assessment: towards a resource efficient food-system. J. Clean. Prod. 240:118241. doi: 10.1016/j.jclepro.2019.118241
Viljoen, C., van der Colf, J., and Swanepoel, P. A. (2020). Benefits are limited with high nitrogen fertiliser rates in kikuyu-ryegrass pasture systems. Land 9:173. doi: 10.3390/land9060173
Vogeler, I., Vibart, R., Mackay, A., Dennis, S., Burggraaf, V., and Beautrais, J. (2014). Modelling pastoral farm systems — scaling from farm to region. Sci. Total Environ. 482–483, 305–317. doi: 10.1016/j.scitotenv.2014.02.134
White, S. L., Benson, G. A., Washburn, S. P., and Green, J. T. (2002). Milk production and economic measures in confinement or pasture systems using seasonally calved holstein and jersey cows. J. Dairy Sci. 85, 95–104. doi: 10.3168/jds.S0022-0302(02)74057-5
Wilkins, R. J. (2008). Eco-efficient approaches to land management: a case for increased integration of crop and animal production systems. Phil. Trans. Biol. Sci. B 363, 517–525. doi: 10.1098/rstb.2007.2167
Yan, M.-J., Humphreys, J., and Holden, N. M. (2011). An evaluation of life cycle assessment of European milk production. J. Environ. Manage. 92, 372–379. doi: 10.1016/j.jenvman.2010.10.025
Keywords: forage-productivity, rotational-grazing, PCF, soil-carbon-storage, farm-N-balance, ley farming, dairy cows
Citation: Reinsch T, Loza C, Malisch CS, Vogeler I, Kluß C, Loges R and Taube F (2021) Toward Specialized or Integrated Systems in Northwest Europe: On-Farm Eco-Efficiency of Dairy Farming in Germany. Front. Sustain. Food Syst. 5:614348. doi: 10.3389/fsufs.2021.614348
Received: 05 October 2020; Accepted: 26 April 2021;
Published: 26 May 2021.
Edited by:
Fred Provenza, Utah State University, United StatesReviewed by:
Philippe V. Baret, Catholic University of Louvain, BelgiumMichael O'Donovan, Moorepark Animal and Grassland Research Centre, Teagasc, Ireland
Copyright © 2021 Reinsch, Loza, Malisch, Vogeler, Kluß, Loges and Taube. This is an open-access article distributed under the terms of the Creative Commons Attribution License (CC BY). The use, distribution or reproduction in other forums is permitted, provided the original author(s) and the copyright owner(s) are credited and that the original publication in this journal is cited, in accordance with accepted academic practice. No use, distribution or reproduction is permitted which does not comply with these terms.
*Correspondence: Thorsten Reinsch, dHJlaW5zY2hAZ2ZvLnVuaS1raWVsLmRl