- 1Graduate School of Agriculture, Hokkaido University, Sapporo, Japan
- 2Experiment Farm, Feld Science Center for Northern Biosphere, Hokkaido University, Sapporo, Japan
- 3Shizunai Livestock Farm, Field Science Center for Northern Biosphere, Hokkaido University, Shinhidaka, Japan
Reduction of chemical fertilizers and effective use of livestock excrement are required for the realization of sustainable agriculture and reduction of greenhouse gas (GHG) emissions. The purpose of this study was to estimate the reduction rate of GHG emissions represented by comparing global warming potential (GWP) using organic fertilizers instead of chemical fertilizers. The study was conducted in a managed grassland on Andosol in southern Hokkaido for 3 years from May 2017 to April 2020. There were five treatment plots: no fertilizer, chemical fertilizer, manure, slurry, and digestive fluid. Organic fertilizers were applied such that the amount of NPK did not exceed the recommended application rate, and the shortage was supplemented with chemical fertilizers. Fluxes in CO2 caused by heterotrophic respiration (RH), CH4, and N2O were measured using the closed chamber method. Net ecosystem carbon balance (NECB) was obtained as net primary production + organic fertilizer application—RH—harvest. The GWP was estimated by CO2 equivalent NECB and CH4 and N2O emissions in each treatment. Chemical fertilizer nitrogen application rates in the organic fertilizer treatments were reduced by 10% for manure, 19.7% for slurry and 29.7% for digestive fluid compared to chemical fertilizer only, but the grass yields were not significantly different among the fertilizer treatments. The 3-year NECB showed significantly smallest carbon loss in manure treatment, and smaller carbon loss in the organic fertilizer treatments than in the chemical fertilizer only. The reduction rate in the GWP with use of organic fertilizers relative to that of chemical fertilizer was 16.5% for slurry, 27.0% for digestive fluid, and 36.2% for manure. The NECB accounted for more than 90% of the GWP in all treatments. CH4 emissions were < 0.1% of the GWP. On the other hand, N2O emissions accounted for more than 5% of the GWP, and was larger in the order of slurry > chemical fertilizer only > digestive fluid > manure. As a conclusion, these organic fertilizers can be used without no reduction of crop yield instead of chemical fertilizer, however, manure is the best way to increase soil carbon and to decrease GWP, followed by digestive fluid.
Introduction
The anthropogenic impact on the climatic system has increased annually, and greenhouse gas (GHG) emissions in 2018 reached a record high of 55.3 Gt CO2 eq yr−1 (UNEP, 2019). Approximately 24% of the GHG emissions come from agriculture, forestry and other land use (AFOLU) (IPCC, 2014). Mitigation in the AFOLU sector is urgently needed.
Soil is the largest carbon storage pool, approximately twice the amount of carbon in the atmosphere and three times the amount in terrestrial biomass (Schlesinger and Jeffrey, 2000). However, agricultural soil looses soil carbon because of organic matter decomposition and erosion, and its recovery is required (Lal, 2020). Furthermore, agriculture is the largest source of CH4 and N2O (Blandford and Hassapoyannes, 2018). Therefore, improvement of carbon storage in farmland and reduction of CH4 and N2O emissions from farmland are important as climate change mitigation measures in agriculture.
Grasslands are a very important ecosystem for the production of herbivorous livestock (Soussana et al., 2007). Because grasslands are not tilled for several years to several decades, the organic matter content in the surface soil increases because of plant residues, livestock excreta, or organic matter derived from applied manure (Ciais et al., 2013). A 3-year study in valley inland and coastal grasslands in California showed that manure application increased soil carbon by 26 and 37%, respectively (Ryals et al., 2014). These show the soil carbon sequestration reducing the concentration of CO2 in the atmosphere (Paustian et al., 1997). Therefore, when organic matter application and no-tillage are adapted continuously, grasslands are expected to exhibit climate change mitigation effects as a carbon storage in agricultural soil.
Evaluation of carbon storage in agricultural land includes carbon output from the harvest system and carbon input into the system by the application of organic matter in addition to carbon cycling in the ecosystem through the atmosphere, plants, and soil (Shimizu et al., 2009). Studies on the net ecosystem carbon balance (NECB) and GHG balance caused by manure application in southern Hokkaido, Japan showed that although CO2 emissions increased because of manure application, there were no differences in CH4 and N2O emissions, and the carbon input from manure application reduced the global warming potential (GWP) (Mukumbuta et al., 2017a). However, N2O emissions in the manure and chemical fertilizer combinedly applied grasslands tended to be higher than the chemical fertilizer only applied grasslands (Shimizu et al., 2013). A study comparing the difference in CH4 and N2O emissions from soil with manure or slurry application in grasslands in northern Tochigi Prefecture, Japan, showed no significant difference between the two organic fertilizers (Mori and Hojito, 2015). Research on the environmental factors controlling CO2, N2O, and CH4 fluxes has shown that CO2 flux has a significant relationship with soil temperature (Shimizu et al., 2009), N2O flux increased from 60% water-filled pore space (WFPS) peaking at 80% WFPS (Katayanagi et al., 2008), and CH4 was normally absorbed by soil but the CH4 uptake decreased with nitrogen application (Hu et al., 2002), and CH4 emitted from poorly drained soil (Shimizu et al., 2013).
In recent years, the use of livestock manure for methane fermentation has increased from the perspective of treating livestock excrement (Holm-Nielsen et al., 2009). Biogas energy can be obtained by fermenting livestock manure. Utilizing the methane fermentation digestive fluid, which is the fermentation residue, as liquid fertilizer not only prevents the outflow of pollutants into rivers, but also provides a supply of nutrients to farmlands and resource-recycling for livestock farming. Despite being fermented, the methane fermentation digestive fluid can be used as a liquid fertilizer with the same components as the raw slurry material (Matsunaka et al., 2003) and has no foul odor as compared with the slurry (Immovilli et al., 2008).
Different organic fertilizers show different physicochemical properties (Harada et al., 1993; Mori and Hojito, 2015). In particular, methane fermentation digestive fluid tends to have a higher pH and a higher ammonium nitrogen concentration than slurries (Yuyama et al., 2007). Therefore, application of digestive fluid makes soil nutrient status and can reduces the application of chemical fertilizer. Furthermore, increase of soil pH decreased N2O emissions (Mukumbuta et al., 2018). However, digestive fluid had lower C/N ratio than raw slurry (Holly et al., 2017), which can increase N2O emission (Toma and Hatano, 2007). On the other hand, a study in Wisconsin showed there was no significant difference of N2O production between digestive fluid and raw slurry applications (Holly et al., 2017).
Effect of organic fertilizers on soil moisture is also an important factor. Digestive fluid has a high water content and increases soil moisture just after the application. Soil moisture is a significant factor influencing nitrogen mineralization, nitrification and denitrification, which strongly influence N2O production in soil (Linn and Doran, 1984). Also increase of soil moisture may increase CH4 in upland fields, and the CH4 emission remaining in the digestive fluid during the fermentation reaction can occur after application to upland fields (Nakamura et al., 2008).
Since organic fertilizers do not always contain NPK in the best balance for crop growth, it is necessary for farmers to properly manage nutrients for the application of organic fertilizer. For this, for example in Hokkaido, the local government suggests an upper limit of the application rate of organic fertilizer to prevent excessive nutrients being applied and recommends that insufficient nutrients induced by this is supplemented using chemical fertilizers (Hokkaido Government Agricultural Department, 2015). Therefore, NPK composition of organic fertilizers influence the application rate of organic fertilizer and reduction rate of chemical fertilizer, which influence NECB and GHG balance.
Therefore, in this study, influences of three organic fertilizers (manure, slurry and digestive fluid) treatments on NECB and GHG balance in grassland are compared with chemical fertilizer only and control (no fertilizer) treatments. The GHG emissions from grassland soil, crop growth and harvest and organic matter application with the five treatments for 3 years in a grassland southern Hokkaido, Japan were measured. The emission factor of N2O in managed upland soil which is used in the IPCC guideline for the National GHG Inventory Report (IPCC, 2006) was also calculated.
In this study, following results were expected: (1) The three types of organic fertilizers have the similar effect of fertilization and can reduce the amount of chemical fertilizer application rate; (2) N2O emissions are lower in organic fertilizer treatments than in chemical fertilizer only treatment due to the reduction in chemical fertilizer nitrogen application rate; (3) The contribution of CH4 emissions to total GHG emissions is small; (4) NECB becomes manure > slurry > digestive fluid treatments, which is significantly larger than that in chemical fertilizer only treatment.
Materials and Methods
Study Site
This study was conducted in a grassland cultivating reed canary grass in the Shizunai Experimental Livestock Farm, Field Science Center for the Northern Biosphere of Hokkaido University in Southern Hokkaido, Japan (Shizunai) (42°26′05.4″N, 142°28′52.1″ E) from May 2017 to April 2020. The study site has a humid continental climate, with cold winters and cool summers. The average temperature over the past 10 years (2007–2016) was 8.4°C, annual rainfall was 1,273 mm, deepest monthly snow was 1 to 22 cm, and snowfall of 10 cm or more was observed from December to March.
The soil was derived from Tarumae (b) volcanic ash, and the mottled upper end of the layer appears within the 0–50 cm soil horizon, and consequently was classified as Wet Andosols (The Fifth Committee for Soil Classification and Nomenclature of the Japanese Society of Pedology, 2017). The soil properties of the 0–7 cm surface layer (Ap1) were pH (H2O) 5.64 ± 0.04, total carbon 36.7± 1.74 g kg−1, total nitrogen 2.7± 0.05 g kg−1, and C/N ratio 13.4. Before 2017, when this research began, the study site had been used as a grassland since 2009, and fertilization with chemical fertilizer and harvest were conducted twice a year. From 2009 to 2016, nitrogen, phosphorus, and potassium were applied as chemical fertilizer at an average of 86 kg T-N ha−1 yr−1, 71 kg P2O5 ha−1 yr−1, and 104 K2O ha−1 yr−1, respectively. Except for 2010, 10 Mg FM ha−1 yr−1 of manure was applied every year after September when the second grass was harvested, and liquid urine fertilizer was also applied in 2012.
Fertilization Treatments and Field Management
The study period consisted of 3 years from May 14, 2017 to April 26, 2020, including May 14, 2017 to April 26, 2018 (348 d), April 27, 2018 to April 26, 2019 (365 d), April 27, 2019, to April 26, 2020 (366 d). Fertilization was conducted twice a year with a base fertilizer (spring) and supplement fertilizer (summer). Harvest was performed twice a year for the first and second grasses. In this study, five treatments of fertilization were tested: no fertilizer (N), chemical fertilizer (F), manure (M), slurry (S), and digestive fluid (D). Fifteen subplots of 5 × 10 m were set up in five treatments × three replicates in a random block design. In each subplot, a 5 × 8 m vegetation survey area, a 5 × 2 m gas sampling area, and a 50 × 50 cm bare area, excluding roots, was set up in the gas sampling area. In the bare area, a root permeable sheet (BKS9812, TOYOBO CO. Ltd., OSAKA, Japan) was inserted at a depth of ~30 cm at the boundary with the planting area to prevent the entry of roots. Plants growing in the bare area during the survey period were regularly removed by hand.
Organic Fertilizer Used
Manure, slurry, and digestive fluid were used as organic fertilizers. Every year, the manure used was from Shizunai, and the digestive fluid was from Sapporo Experimental Farm, Field Science Center for the Northern Biosphere of Hokkaido University (Sapporo) (43°04′41.1″N, 141°20′03.6″E). The manure was made from a mixture of cow excreta, horse excreta, and bedding litter and turned over once every 10 days during winter. The slurry used was from Shizunai in 2017. However, the Shizunai slurry had a high water content and a low nitrogen content because it was mixed with rainwater. Due to this, in 2018 and 2019, Sapporo slurry was used. The slurry in Shizunai was from cow excreta, horse excreta, and rainwater and was stored in a slurry reservoir in the barn until use. The slurry in Sapporo was made from cattle, pig, and chicken excreta, and water was added as appropriate to increase fluidity. The digestive fluid used was from Sapporo, which was made from methane fermentation of the slurry in Sapporo. Each organic fertilizer was collected 1 month before application, and water content, pH, TN, -N, P, K, and TC were analyzed. The components of each organic fertilizer are shown in Table 1.
Design of Fertilization
Table 2 shows the application rates of organic and chemical fertilizers for each year. The nitrogen application rate depended on the legume rate (Hokkaido Government Agricultural Department, 2015). In 2017 and 2019, because the legume rate was 5–15%, nitrogen application rate was 100 kg ha−1. On the other hand, in 2018, the nitrogen application rate increased to 160 kg ha−1 because the legume rate decreased to < 5%. The organic fertilizer application rate was determined such that the organic fertilizer N, P, or K application rates did not exceed the recommended application rate of N, P, or K, and any shortage in N, P, or K was made up by chemical fertilizers. Chemical fertilizer was applied at the ratio of application at the base: supplement of 2:1, whereas organic fertilizer was applied only used as a base application. Both chemical and organic fertilizers were applied by top dressing.
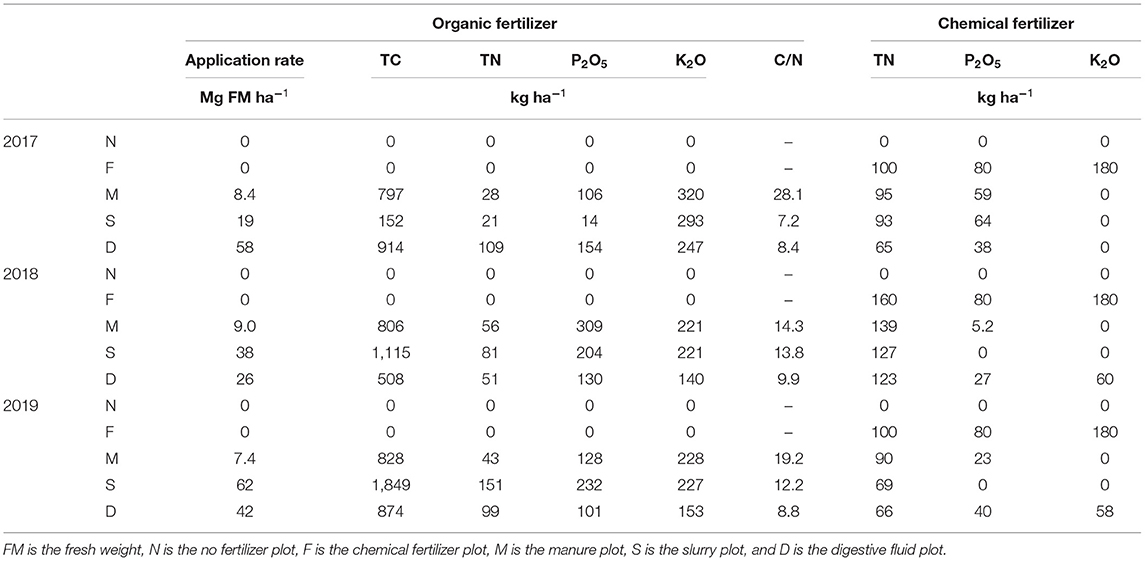
Table 2. Annual organic fertilizer application rate each year, chemical fertilizer application to chemical fertilizer plots, and chemical fertilizer supply to organic fertilizer plots.
Measurements
Environmental Factors
Daily air temperature and precipitation were obtained from the close Automated Meteorological Data Acquisition System (AMeDAS) station of the Japan Meteorological Agency, which are located about 14 km from the study site for air temperature and about 100 m from the study site for precipitation. Soil temperature at a 5 cm depth was measured at the same time as the gas flux measurements using a thermistor thermometer (CT-414WR, CUSTOM, Tokyo, Japan), and volumetric soil moisture content at 0–6 cm depth was measured using the frequency domain reflectometry (FDR) method (DIK-311A; Daiki, Saitama, Japan). The water-filled pore space (WFPS,%) was calculated as:
where θ is volumetric soil water content (m3 m−3) and p is soil porosity (m3 m−3); p was measured by a three-phase meter (DIK-1150; Daiki, Saitama, Japan).
Soil sampling was conducted at the same time as the gas flux measurement during from April to November when the soil was not frozen. The collected soil was sieved at 2 mm. Soil -N content was determined by water extraction with the ratio of soil: deionized water = 1:5, and the -N concentration in the water extraction was measured by ion chromatography (DIONEX ICS-1100; Thermo Fisher Scientific, MA, USA). Soil -N content was determined by KCl extraction with the ratio of soil:KCl (2 mol L−1) = 1:10, and the -N concentration in the KCl extraction was measured by the indophenol blue method.
Gas Fluxes
Soil CO2, CH4, and N2O fluxes were measured by the static closed chamber method (Toma and Hatano, 2007). In the gas sampling area of each treatment, chambers made of stainless steel were installed. Chambers with a diameter of 40 cm and a height of 30 cm were used to measure CH4 and N2O fluxes in the planting area, and those with a diameter of 20 cm and a height of 25 cm were used to measure the CO2 flux in the bare plot, which was assumed to correspond to microbial heterotrophic respiration (RH). The chambers were placed onto bases, which were permanently installed during the measurement period. The chamber bases were inserted into the soil to a depth of 5 cm for at least 12 h before the first gas sampling. During the snowfall period, chamber bases were set up directly onto the snow (Katayanagi and Hatano, 2012). Gas flux measurements were performed between 8:00 and 13:00 for seven consecutive days after the application of the fertilizer, once a week during the plant growing season and once a month during winter. Changes in the concentrations of CO2, CH4, and N2O in the headspace of the chambers with time was measured according to a previously reported procedure (Nakano et al., 2004; Toma and Hatano, 2007; Shimizu et al., 2013), that is, gas samples in the chamber headspace were taken at 0 and 6 min for CO2 and 0, 15, and 30 min for CH4 and N2O after closing chambers by using a 25 mL gas tight syringe. A gas sample of 250 mL was injected into a 500 mL Tedlar bag for CO2. For CH4 and N2O, each gas sample (20 mL) was placed into an evacuated glass vial (10 mL). The CO2 concentration was determined using an infrared CO2 analyzer (Model ZEP9GC11; Fuji Electric, Tokyo, Japan), and the CH4 and N2O concentrations were determined using gas chromatography equipped with a flame ionization detector (GC-8A; Shimadzu, Kyoto, Japan) and an electron capture detector (GC-14B; Shimadzu, Kyoto, Japan), respectively.
The gas flux from the soil was calculated using the following linear regression equation (Toma et al., 2011):
where F is the gas flux (mg C m−2 h−1 for CO2 and CH4, mg N m−2 h−1 for N2O); ρ is the density of each gas under standard conditions (CO2 = 1.997 × 106 mg m−3, CH4 = 0.717 × 106 mg m−3, N2O = 1.978 × 106 mg m−3), V is the volume of the chamber (m3), A is the surface area of the chamber (m2), Δc/Δt is the rate of change in gas concentration in the head space of the chamber during the sampling time (10−6 m3 m−3 h−1); T is the air temperature inside the chamber (°C); and α is the ratio of molar mass of carbon to the molecular weight of CO2 and CH4, or of nitrogen to N2O. For N2O and CH4, theΔc/Δt of R more than 0.95 was used for the flux calculation. The flux of CO2 was calculated at two points of 0 and 6 min based on the theoretical consideration that the increase in the CO2 concentration in the chamber loses linearity after about 8 min (Nakano et al., 2004). However, the relationship between the multiple-times sampling and the two-points sampling is linear of 1: 1 (Mukumbuta et al., 2017b).
Cumulative gas emissions were calculated by linear interpolation between sampling events and numerical integration of the underlying area using the trapezoid rule as follows (Jin et al., 2010):
where Ri is the mean gas flux (mg m−2 h−1) of the two successive sampling dates, Di is the number of days in the sampling interval and n is the number of sampling times.
Plant Production and Harvest
Aboveground biomass, belowground biomass were measured four times a year including two times of harvest, mid-April (beginning of crop growing season), late June (the first crop harvest), early September (the second crop harvest), and early November (end of crop growing season), that is, total 13 times from April 2017 to April 2020. Grass samples were taken from the vegetation survey areas of each treatment plot. All the aboveground biomass, including green and dead biomass were collected from the 0.5 × 0.5 m quadrate in April and November. Aboveground biomass at the time of harvest was obtained as the sum of harvest and residue. The harvest was measured by clipping at 5 cm above the ground in the 1 × 1 m quadrate. The residue was measured by collecting the stubbles and dead biomass in a 0.5 × 0.5 m quadrate. Regarding the belowground biomass, the root samples were collected from the 0.5 × 0.5 m area × 0.3 m deep by collecting soil and passing through an 8 mm sieve in the field. Roots were washed in a 2 mm sieve in the laboratory. All the samples were oven-dried at 70°C for 72 h and weighed. Each dried sample was analyzed for total carbon content.
Net primary production (NPP) was estimated as the increments of aboveground and belowground biomass (Mu et al., 2006), that is, annual aboveground NPP (ANPP) and belowground NPP (BNPP) were estimated as follows:
where H and R are the harvest and the residue at crop harvest, respectively (1 and 2 in the parentheses mean the first and second crop harvest, respectively); ABb, Abe, and ABb' are the aboveground biomass at the beginning and the end of crop growing season and the beginning of crop growing season in next year, respectively.
Equation 4 can be shortened as follows:
As belowground biomass is not harvested, BNPP can be obtained as follows:
where BBb and BBb' are the belowground biomass at the beginning of crop growing season and in the next year, respectively.
Calculations
N2O Emission Factor
The N2O emission factor indicates the cumulative N2O emissions per unit applied nitrogen. According to the calculation method proposed by Shimizu et al. (2013), the N2O emission factors derived from chemical fertilizers and organic fertilizers were calculated as follows:
where EFCF is the N2O emission factor for chemical fertilizer (%), EFOF is the N2O emission factor for organic fertilizer (%); ECF, ENF, and EOF are the N2O emissions in the chemical fertilizer plot, no fertilizer plot, and organic fertilizer plot, respectively (kg N ha−1); NCF in CF plot and NCF in OF plot were the chemical fertilizer N application rates in the chemical fertilizer plot and organic fertilizer plot (kg N ha−1), respectively, and NOF in the OF plot was the organic fertilizer N application rate (kg N ha−1).
Net Ecosystem Carbon Balance
Net ecosystem carbon balance (NECB) was obtained as net biome production (Schulze et al., 2000). The NECB in agricultural land was obtained by adding carbon input from the application of organic fertilizer (Cinput) and carbon export via harvest (Coutput) for net ecosystem production (NEP). The NEP is estimated as the difference between net primary production (NPP) by photosynthesis of plants and heterotrophic respiration (RH) by decomposition of soil organic matter. Concerning carbon emission with CH4 flux, in uplands, CH4 flux is known to be very small compared to CO2 (Toma et al., 2011), therefore it was not included in the NECB calculation. Therefore, NECB (Mg C ha−1 yr−1) is calculated as follows:
GWP and GHG Balance
The NECB and cumulative emissions of CH4 and N2O were converted to GWPCO2, GWPCH4, and GWPN2O, respectively, using the CO2 conversion coefficient [CO2:1, CH4:28, N2O: 265 (IPCC, 2014)]. The GHG balance (Mg CO2 eq ha−1 yr−1) was obtained as GWP, which are the sum of GWPCO2, GWPCH4, and GWPN2O as follows:
Statistical Analysis
The Shapiro-Wilk test was performed on each GHG flux, environmental factors, cumulative GHG emissions, carbon balance, and GHG balance to confirm normality. If normality was not found, logarithmic conversion was performed and the test was performed again to confirm normality. Differences in GHG emissions among years and among treatments were tested using a two-way analysis of variance (ANOVA). Differences in 3-year total GHG emissions, net ecosystem carbon balance and GHG balance among treatments were tested using a one-way ANOVA. If a significant difference (p < 0.05) occurred in the test, multiple comparisons were performed using the Tukey HSD method. In order to explain the relationship between the C/N and N2O emission factors of organic fertilizers, the normality of each was confirmed by the Shapiro-Wilk test, and a simple regression analysis was performed. The analysis was performed using R (R Development Core Team, 2018; version 3.5.1).
Results
Environmental Factors
Air temperature was highest in August and lowest in February (Figure 1A). The average annual temperatures during the study period in each year were 8.0, 8.4, and 8.9°C in 2017, 2018, and 2019, respectively, which were lower, similar, and higher than the average values for the last 10 years (8.4°C), respectively. Annual precipitation during the study period of each year was 1,227, 1,254, and 1,227 mm in 2017, 2018, and 2019, respectively, and the average annual precipitation for the past 10 years was 1,273 mm (Figure 1A).
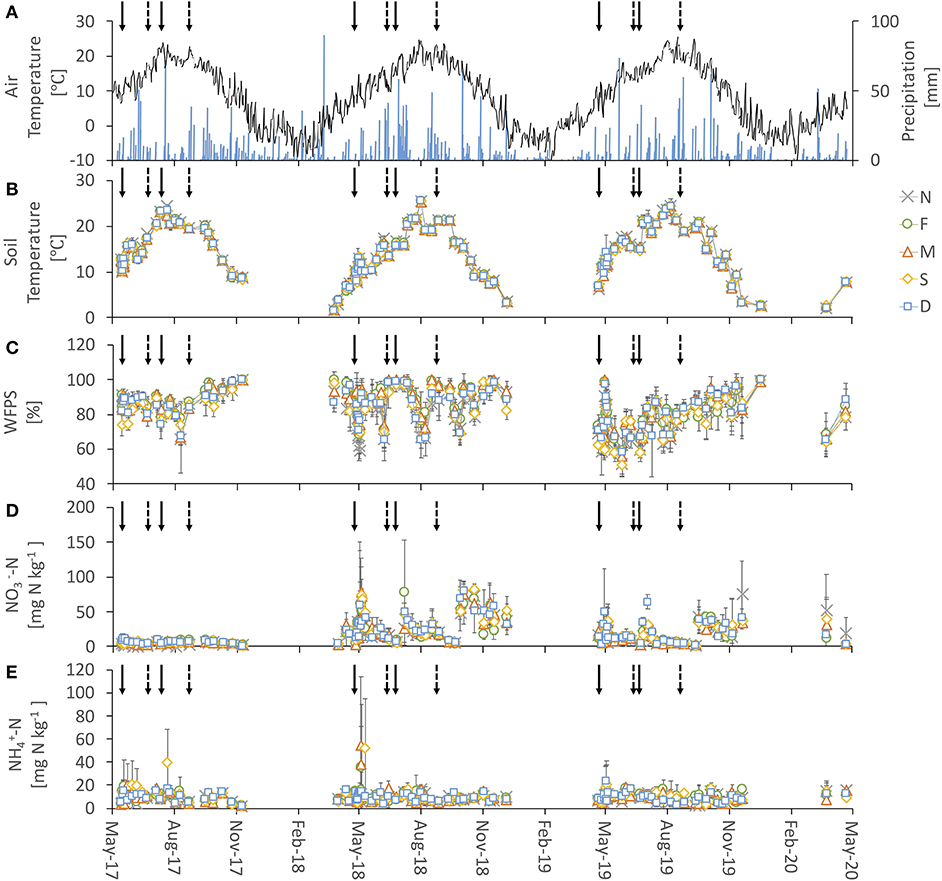
Figure 1. Change in air temperature and precipitation (A), soil temperature at a depth of 5 cm (B), water filled pore space (WFPS) at the 0–6 cm soil depth (C), soil -N content (D), soil -N content (E). Error bars represent standard deviations, solid and broken arrows represent fertilization and harvest, respectively. n = 3.
Soil temperature tended to be similar to air temperature during the no-freeze period and ranged from 1.6 to 25.6°C, which was significantly lower in 2018 than in 2017 and 2019, although there was no significant difference among the treatments (Figure 1B).
WFPS ranged from 38 to 100%, tended to increase after heavy rainfall, and to decline when there was high temperature and no rainfall (Figure 1C). The WFPS was significantly lower in the no-fertilizer plot and the slurry plot in 2019, and there was no significant difference among the other treatment plots.
Soil -N content showed almost no peak in 2017, but several peaks after fertilization in 2018 and 2019 (Figure 1D). The highest mean -N was 82.6 mg kg−1 in the slurry plot. Conversely, the lowest average -N was 75.3 mg kg−1 in the non-fertilized plot.
Soil -N content showed high peak in the slurry plot (39.7 mg kg−1) after topdressing in 2017 (Figure 1E). In 2018, peaks were observed in the chemical fertilizer plot (36.1 mg kg−1), manure plot (54.8 mg kg−1), and slurry plot (52.3 mg kg−1) immediately after the first fertilizer application. In 2019, no significant peak was observed in any treatment plot. The highest mean -N content was in the slurry plot at 10.8 mg kg−1. Conversely, the lowest mean of -N content was 9.0 mg kg−1 in the non-fertilized plot.
GHG Fluxes
The CO2 (RH) flux ranged from −49 (3 March, 2020) to 262 mg C m−2 h−1 (22 August, 2017) and increased with increasing temperature (Figure 2A). Additionally, a decrease in CO2 (RH) flux was observed when WFPS was 100%.
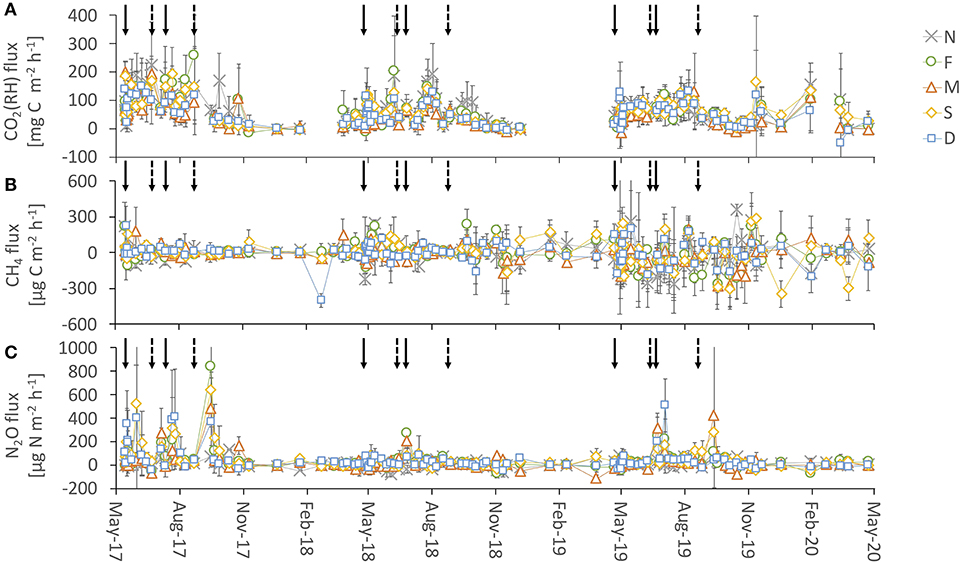
Figure 2. Change in CO2(RH) flux (A), CH4 flux (B), and N2O flux (C). Error bars represent standard deviations, solid and broken arrows represent fertilization and harvest, respectively. n = 3.
The CH4 flux ranged from −401 to 357 μg C m−2 h−1 and fluctuated highly (Figure 2B). The peaks of CH4 flux were observed after the application of organic fertilizers in 2017 and 2018, especially in the slurry plot in the winter of 2018. In 2019, there was a large daily fluctuation with high CH4 uptake by the soil.
The N2O flux ranged from −115 to 839 μg N m−2 h−1 just after the application of organic fertilizer (Figure 2C). A peak of N2O flux occurred in the digestive fluid plot in 2017 (347.82 μg N m−2 h−1). On the other hand, in 2018 and 2019, no peak in N2O flux was observed just after the application of organic fertilizer. The peak of N2O flux was smaller throughout the year in 2018 than in 2017 and 2019.
There was no significant correlation between N2O flux and soil -N and -N contents (Figures 3A,B). However, the emission peaks of N2O flux larger than 191 μg N m−2 h−1 of top 5% were clearly observed and tended to increase when the soil -N content was 2–12 mg N kg−1 (except for one at the time of just after supplement fertilizer application for second crop in digestive fluid treatment in 2019). Concerning soil -N content, almost all N2O fluxes including the high peaks were found in 5–18 mg N kg−1.
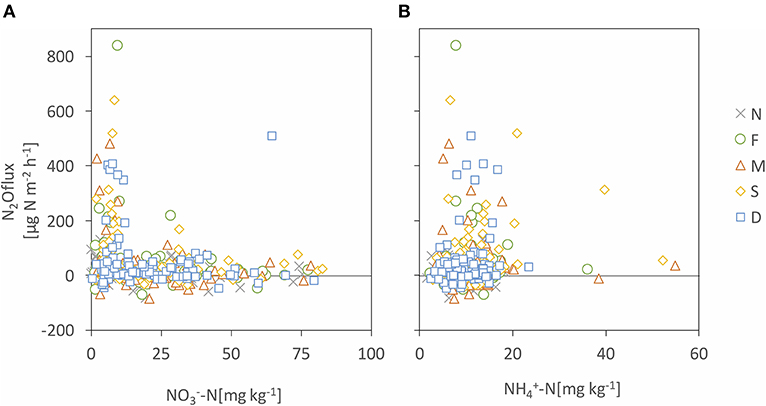
Figure 3. Relationship between N2O flux and soil -N content (A) and -N content (B). N is the no fertilizer plot, F is the chemical fertilizer plot, M is the manure plot, S is the slurry plot, and D is the digestive fluid plot.
There was no difference in the relationship between gas flux and environmental factors caused by fertilization treatment (Figure 4). The CO2 flux increased with increasing soil temperature and decreasing WFPS (Figures 4A,B). There was no significant correlation between CH4 flux and soil temperature and WFPS (Figures 4C,D). The emission peak of N2O flux larger than 191 μg N m−2 h−1 of top 5% was observed when the soil temperature was 12–23°C and the WFPS was 80–100%. However, at just after the harvest of first crop, all treatments showed high peaks in the range of 60–70% WFPS (Figures 4E,F).
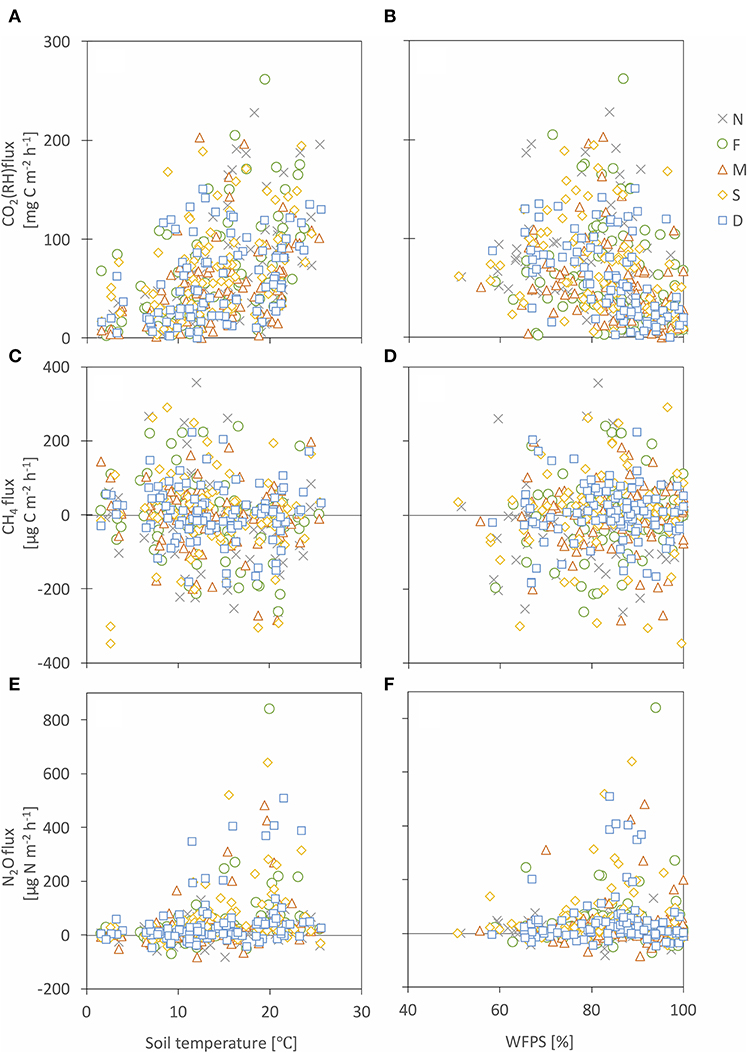
Figure 4. Relationship between CO2(RH) flux and soil temperature (A) and WFPS (B), CH4 flux and soil temperature (C), and WFPS (D), and N2O flux and soil temperature (E) and WFPS (F). N is the no fertilizer plot, F is the chemical fertilizer plot, M is the manure plot, S is the slurry plot, and D is the digestive fluid plot.
GHG Emissions
The result of two-way ANOVA shows that CO2(RH) emissions exhibited significant difference among years but no significant difference among the treatments (Table 3). It was maximum in the chemical fertilizer treatment in 2017, in no fertilizer treatment in 2018, and in the slurry treatment in 2019.
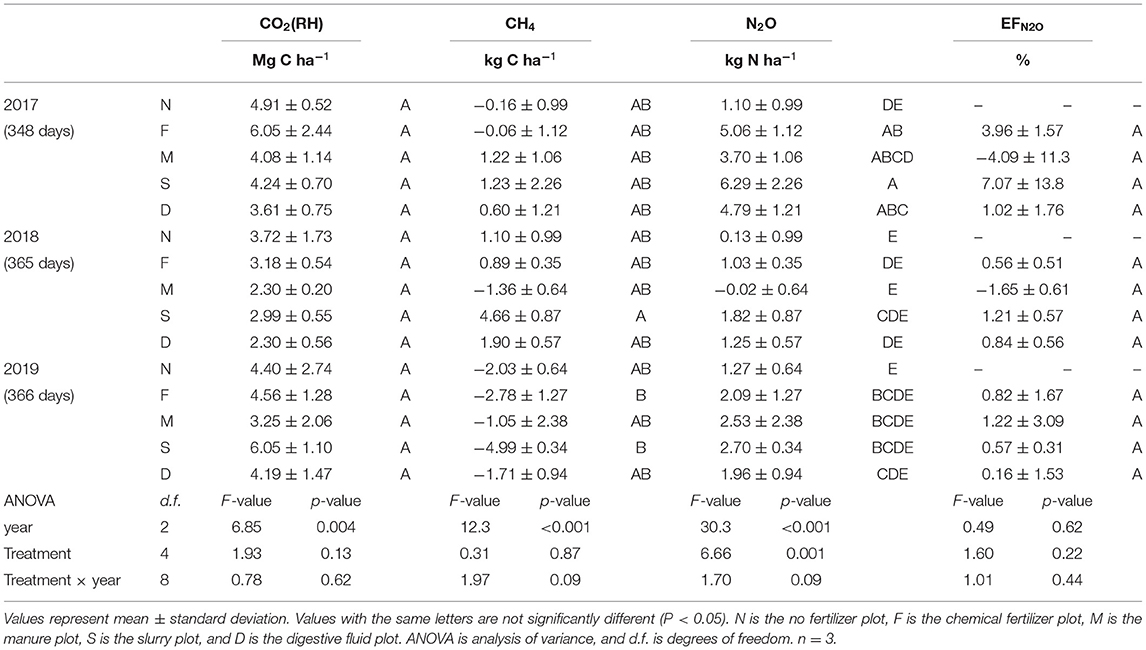
Table 3. Cumulative CO2(RH), CH4, and N2O emissions and the N2O emission factor (EFN2O) for each year.
The result of ANOVA showed that CH4 emissions exhibited no significant differences among years and treatments (Table 3). However, CH4 uptake was observed with the no fertilizer or chemical fertilizer treatments in 2017, manure treatment in 2018, and all treatments in 2019 with lower precipitation.
The result of ANOVA showed that N2O emissions exhibited significant differences among years and treatments (Table 3). N2O emission was significantly higher in fertilizer treatments than in no fertilizer treatment, but there was no significant difference among the fertilizer treatments. However, the slurry treatment tended to be the highest N2O emission for all years.
The result of ANOVA showed no significant difference in the 3-year total CO2(RH) among the treatments (Table 4). However, it tended to be the highest in the chemical fertilizer treatment (13.79 Mg C ha−1), and among the organic fertilizer treatments, slurry > digestive fluid > manure.
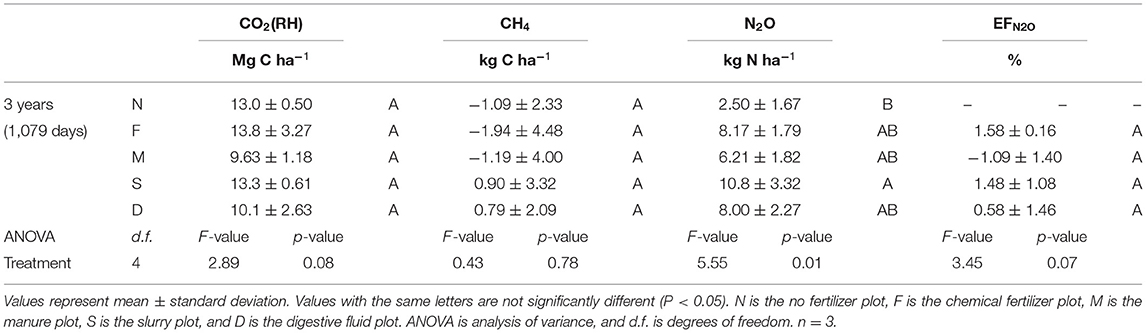
Table 4. Cumulative CO2(RH), CH4, and N2O emissions and the N2O emission factor (EFN2O) for 3-year total.
There was no significant difference in the 3-year total CH4 emission among the treatments (Table 4). However, 3-year total CH4 emission tended to be highest in the slurry treatment (0.90 kg C ha−1) and lowest in the manure treatment (−1.19 kg C ha−1) among the organic fertilizer.
There was significant difference in 3-year total N2O emission among the treatments (Table 4). Three-year total N2O emission was highest in the slurry treatments (10.8 kg N ha−1) and lowest in the manure treatment (6.21 kg N ha−1), although there was no significant difference among the fertilizer treatments.
N2O Emission Factor
There was not a substantial variability on the N2O emission factor among years and treatments (Table 3). The 3-year average of the N2O emission factor was in the order chemical fertilizer > slurry > digestive fluid > manure (Table 4). However, among the organic fertilizer treatments, there was a significant negative relationship between the C/N ratio and N2O emission factor (Figure 5).
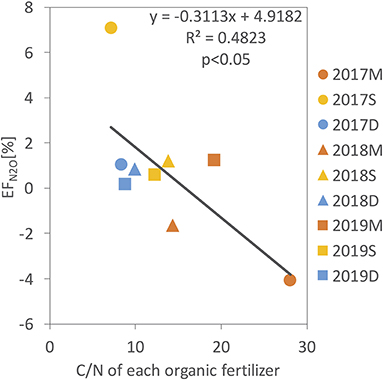
Figure 5. Relationship between N2O emission factor and C/N ratio of organic fertilizers. M is the manure plot, S is the slurry plot, and D is the digestive fluid plot. Solid line reveals the result of simple regression analysis.
Grass Yield
The 3-year cumulative grass yield was not significantly different among fertilizer treatments and was significantly higher than that of the no fertilizer treatment (Figure 6). This was achieved despite of the reduction of chemical fertilizer for nitrogen by 10.0–29.4%, phosphorus by 56.3–73.3%, and potassium by 78.2–100% in 3 years as the concentrations of phosphorus and potassium in organic fertilizers were high (Table 2). Thus, the fertilizer application design for each fertilizer area was appropriate.
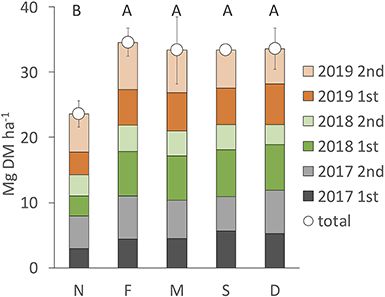
Figure 6. Cumulative dry matter yield of grass. N is the no fertilizer plot, F is the chemical fertilizer plot, M is the manure plot, S is the slurry plot, and D is the digestive fluid plot. Error bars represent standard deviations.
Net Ecosystem Carbon Balance
NECB in all treatments was negative, that is, the ecosystem lost carbon (Table 5). NECB was significantly lower in no fertilizer and chemical fertilizer treatments than the manure treatment. Although there was no significant difference among the fertilizer treatments, NECB tended to be larger in organic fertilizer treatments than in the chemical fertilizer treatment. ANPP was significantly lower in the no fertilizer treatment than the fertilizer treatment, and there was no significant difference among the chemical and organic fertilizer treatments. On the other hand, BNPP was negative, although there was no significant difference. Although ANPP + BNPP was positive, NEP was negative because of a larger RH than ANPP+BNPP. Therefore, a larger NECB in the organic fertilizer plot was caused by the contribution of Cinput with organic fertilizer application, that is, carbon input by organic fertilizer enhances soil carbon sequestration. NECB in organic fertilizer plots tended to be in the order manure > digested fluid > slurry.
GHG Balance
The contribution of CH4 emission to the GWP for 3 years was very small, which was < 0.1%. On the other hand, the contribution of N2O emissions to the GWP was larger than 5% (Table 6). The 3-year GWP was significantly smaller in the manure plot (43.1 ± 2.8 Mg CO2 eq ha−1 yr−1) than that in the no fertilizer (65.4 ± 3.7 Mg CO2 eq ha−1 yr−1) and chemical fertilizer plots (67.4 ± 13.6 Mg CO2 eq ha−1 yr−1), but there was no significant difference among the organic fertilizer treatments. The cumulative values for organic fertilizer treatments were slurry > digestive fluid > manure, and the GWP was 16.5, 27.0, and 36.2% smaller than those in the chemical fertilizer treatment, respectively.
Discussion
Effect of Different Fertilizers on the Net Ecosystem Carbon Balance
In this study, the no-fertilizer treatment exhibited a significantly smaller NECB than did the fertilizer treatments. All organic fertilizers from manure, slurry, and digestive fluid tended to have a larger NECB, although there was no significant difference compared to that of the chemical fertilizer treatment. This indicates that organic fertilizers have larger carbon storage than do chemical fertilizers. Previous studies conducted on grasslands also showed higher NECB in manure treatment than in chemical fertilizer treatment (Matsuura et al., 2014; Shimizu et al., 2015; Mukumbuta et al., 2017a). In this study, NECB was negative for 3 years and all treatment plots became carbon sources. On the other hand, in previous studies by Matsuura et al. (2014) and Shimizu et al. (2015), NECB was positive in the manure treatment. The manure application rate in the previous study was 2.1–7.7 Mg C ha−1 year−1, whereas that in this study was 0.15–1.9 Mg C ha−1 year−1. This depended on the raw material of the manure. The manure used in the previous study was a bark manure with a lower C/N ratio and lower potassium content.
Therefore, to increase soil organic carbon using organic fertilizer, the quality of organic fertilizer, especially the ratio of carbon to nutrients, should be taken into consideration.
Effect of Different Fertilizer on the CH4 and N2O Emissions
In this study, the slurry treatment showed the highest CH4 emission in 3-year total, although CH4 emissions were not significantly different (Table 4). However, CH4 emission showed a large variation among the years. Additionally, a previous study showed that direct CH4 emission from organic fertilizer often occurred just after the application of organic fertilizer to the soil (Mori and Hojito, 2015). It was also expected that the anaerobic conditions produced by the liquid fertilizer in the slurry and digestive fluid would promote CH4 emission immediately after fertilization (da Silva Cardoso et al., 2020). However, in this study, no peak of CH4 flux was observed immediately after fertilization. This was probably because the temperature was relatively low immediately after fertilization and no microbial degradation occurred (Ryals and Silver, 2013).
The relationship between N2O flux and environmental factors was not significantly different among fertilizer treatments. That is, N2O flux peaks were observed when the soil -N content was 2 to 12 mg N kg−1 (except for one plot of just after supplement fertilizer application for second crop in digestive fluid treatment in 2019) (Figure 3A), and the WFPS was 80–100% (Figure 4F). These suggest that the N2O emission occurred through denitrification in all fertilizer treatments (Takakai et al., 2006). However, at just after the first crop harvest in 2019, the large N2O peaks in the lower WFPS than 80%. This was probably because the stronger effect of the disturbance by harvest on N2O emission than the effect of WFPS, which was shown by Li et al. (2015).
The application of organic fertilizers increases nitrogen mineralization in the soil and, from a physical point of view, increased the water retention of the soil, which increase N2O emissions (Ryals and Silver, 2013). In particular, the application of slurry tends to promote denitrification to increase N2O production (Rochette et al., 2004). On the other hand, it has also been reported that there was no significant difference in annual N2O emission in a grassland in Hokkaido between chemical fertilizer treatment (0.6 kg N ha−1 yr−1) and digestive fluid treatment (0.7 kg ha−1 yr−1) (Sawamoto et al., 2010). In this study, N2O emissions from organic fertilizer treatments were higher than that of no fertilizer treatment (Tables 3, 4). The slurry treatment exhibited the highest N2O emission, and the manure treatment had the lowest N2O emissions among the fertilizer treatments. However, there was no significant difference among the organic fertilizer treatments and between organic and chemical fertilizer treatments. Similarly, in a previous study conducted in the same Andosols, no significant difference was found between the manure treatment and the chemical fertilizer treatment, although it tended to be higher in the manure treatment (Shimizu et al., 2010; Mukumbuta et al., 2017a). These results suggest that the high soil organic matter content of the Andosols reduce the effect of organic matter application.
Importance of Fertilization Design on Crop Yield
The fact that there was no significant difference in the grass yield between the chemical fertilizer treatment and the organic fertilizer treatments (Figure 6) was that the fertilizer application design was correctly performed using a combination of chemical and organic fertilizers without significantly increasing nitrogen loss of the organic fertilizer compared to that of the chemical fertilizer (Sawamoto et al., 2010; Mori and Hojito, 2015). It has been reported that field surplus nitrogen, which is calculated as the difference between nitrogen input by fertilizer application and nitrogen output by plant uptake is a good indicator of N2O emissions (Shimizu et al., 2010). Field surplus nitrogen did not correlate with plant nitrogen uptake but correlated with N2O emission and -N leaching (Nagatake et al., 2018).
Comparison of the N2O Emission Factors of Organic Fertilizer
The N2O emission factors in this study were 0.6–4.0%, −4.1–1.2%, 0.6–7.1%, and 0.2–1.0% for chemical fertilizer, manure, slurry, and digestive fluid, respectively. There was no significant difference among years or fertilizer treatments (Table 3), although chemical fertilizer treatment tended to have higher emission factor than organic fertilizer treatments. However, on average, there was a significant negative correlation with the C/N ratio of organic fertilizers (Figure 5). This result was consistent with the results on farmland where N2O emissions were measured using organic matter containing manure and plant residues (Akiyama and Tsuruta, 2003; Huang et al., 2004; Toma and Hatano, 2007; He et al., 2019). The C/N ratio of the microbes in soil is 5–10; that is, the synthesis of the cell requires nitrogen in an amount of 1/5–1/10 that of the carbon, and some of the nitrogen mineralized through organic matter decomposition will be taken up by the microbes. The lower the C/N ratio of organic matter applied to the soil, the greater the amount of mineralized nitrogen released into the soil because there is more mineralized nitrogen than the microbes can uptake. On the contrary, the higher the C/N ratio of organic matter into the soil, the lower the release of mineral nitrogen into the soil because more mineralized nitrogen is taken up by the cells (Ruser et al., 2001). Soil mineral nitrogen (-N and -N) and easily decomposable organic carbon are substrates for nitrification and denitrification that cause soil N2O emissions. In this study, soil -N and -N content tended to be larger in slurry with a low C/N ratio than in manure with a high C/N ratio (Figures 1E,F). Therefore, it is considered that the slurry releases more mineralized nitrogen into the soil, which enhances nitrification and denitrification, and promotes N2O emission.
In this study, N2O emission factor is obtained by subtracting N2O emissions from chemical fertilizers and soil-derived emissions (Equations 7 and 8). Negative N2O emission factor of organic fertilizer shown in Table 3 suggests that the application of organic fertilizer denitrifies N2O derived from chemical fertilizer. Several reports show the lower N2O emission factor of organic matter than that of chemical fertilizer only (Toma and Hatano, 2007; Toma et al., 2007; Jin et al., 2010; Mori and Hojito, 2012; Shimizu et al., 2013; De Rosa et al., 2018).
Comparison of Global Warming Potential Among Fertilizers
The cumulative GWP for 1,079 days tended to be smaller for the organic fertilizer treatments than in the no fertilizer and chemical fertilizer treatments, especially the manure treatment, which had a significantly smaller GWP (Table 6). As suggested by Mukumbuta and Hatano (2020), estimates of the NECB showed that organic fertilizers tended to have a higher soil carbon sequestration effect than chemical fertilizers (Table 5), which is thought to reduce the GWP of organic fertilizers. Comparing organic fertilizer treatments, the cumulative GWP was the largest in the slurry, which showed the highest N2O emission factor because of the lowest C/N ratio (Figure 5). Although manure application requires relatively higher chemical fertilizer nitrogen application rate among the organic fertilizer treatments (Table 2), manure application increased soil carbon sequestration and reduced N2O emissions, resulted in the highest reduction of GWP. Digestive fluid application reduced chemical fertilizer nitrogen application most, and reduced N2O emission next of manure.
Conclusion
The effects of application of manure, slurry, and digestive fluid on GHG emissions from a grassland on Andosol in a cold temperate climate were evaluated under fertilization management in accordance with regional recommendations. In the plots where organic fertilizer was applied, the amount of chemical fertilizer input could be reduced while maintaining yield. The relationships between CO2, CH4, and N2O emissions and the soil environmental factors (soil -N and -N contents, temperature, and WFPS) were not influenced by the type of fertilizer. The N2O emission factor was highest in the slurry treatment and lowest in the manure treatment, showing a negative correlation with the C/N ratio of organic fertilizers. Additionally, the application of these organic fertilizers has been shown to improve ecosystem carbon balance and reduce the GHG balance. When the GWP for each fertilizer was evaluated based on the results of this 3-year study, it was suggested that manure is the best way to increase soil carbon and to decrease GHG emissions, followed by digestive fluid.
Data Availability Statement
The original contributions presented in the study are included in the article, further inquiries can be directed to the corresponding author.
Author Contributions
RH planned this study, compiled all the data, and edited the manuscript. RK, CS, and KY measured greenhouse gas emissions and environmental factors and wrote the results and discussion. AN designed the experimental field, measurement procedures, and sampling schedule. YY and JD measured greenhouse gases and soil environmental factors. NY and KT produced slurries and methane fermentation digestive fluid at the Sapporo Farm. MK produced manure used at the Shizunai livestock farm and managed the field work. All authors contributed to the article and approved the submitted version.
Funding
This study was supported by Ministry of Agriculture, Forestry and Fisheries of Japan, via the Commissioned project study, named Development of the climate change mitigation technology in livestock sector.
Conflict of Interest
The authors declare that the research was conducted in the absence of any commercial or financial relationships that could be construed as a potential conflict of interest.
References
Akiyama, H., and Tsuruta, H. (2003). Effect of organic matter application on N2O, NO, and NO2 fluxes from an andisol field. Global Biogeochem. Cycles 17, 1–16. doi: 10.1029/2002gb002016
Blandford, D., and Hassapoyannes, K. (2018). The Role of Agriculture in Global GHG Mitigation. OECD Food, Agriculture and Fisheries Papers, No. 112. Paris: OECD Publishing.
Ciais, P., Sabine, C., Bala, G., Bopp, L., Brovkin, V., Canadell, J., et al. (2013). “The physical science basis,” in Contribution of Working Group I to the Fifth Assessment Report of the Intergovernmental Panel on Climate Change (IPCC), 465–570. doi: 10.1017/CBO9781107415324.015
da Silva Cardoso, A., Junqueira, J. B., Reis, R. A., and Ruggieri, A. C. (2020). How do greenhouse gas emissions vary with biofertilizer type and soil temperature and moisture in a tropical grassland? Pedosphere 30, 607–617. doi: 10.1016/S1002-0160(20)60025-X
De Rosa, D., Rowlings, D. W., Biala, J., Scheer, C., Basso, B., and Grace, P. R. (2018). N2O and CO2emissions following repeated application of organic and mineral N fertiliser from a vegetable crop rotation. Sci. Total Environ. 637–638, 813–824. doi: 10.1016/j.scitotenv.2018.05.046
Harada, Y., Haga, K., Osada, T., and Koshino, M. (1993). Quality of compost produced from animal wastes. JARQ 26, 238–246.
He, T., Yuan, J., Luo, J., Wang, W., Fan, J., Liu, D., et al. (2019). Organic fertilizers have divergent effects on soil N2O emissions. Biol. Fertil. Soils 55, 685–699. doi: 10.1007/s00374-019-01385-4
Hokkaido Government Agricultural Department (2015). Chapter V Grasses and Forage crops. In Hokkaido fertilizer recommendations 2015. Hokkaido.
Holly, M. A., Larson, R. A., Powell, J. M., Ruark, M. D., and Aguirre-Villegas, H. (2017). Greenhouse gas and ammonia emissions from digested and separated dairy manure during storage and after land application. Agricult. Ecosyst. Environ. 239, 410–419. doi: 10.1016/j.agee.2017.02.007
Holm-Nielsen, J. B., Al Seadi, T., and Oleskowicz-Popiel, P. (2009). The future of anaerobic digestion and biogas utilization. Bioresour. Technol. 100, 5478–5484. doi: 10.1016/j.biortech.2008.12.046
Hu, R., Hatano, R., Kusa, K., and Sawamoto, T. (2002). Effect of nitrogen fertilization on methane flux in a structured clay soil cultivated with onion in central hokkaido, japan. Soil Sci. Plant Nutr. 48, 797–804. doi: 10.1080/00380768.2002.10408705
Huang, Y., Zou, J., Zheng, X., Wang, Y., and Xu, X. (2004). Nitrous oxide emissions as influenced by amendment of plant residues with different C:N ratios. Soil Biol. Biochem. 36, 973–981. doi: 10.1016/j.soilbio.2004.02.009
Immovilli, A., Fabbri, C., and Valli, L. (2008). Odour and ammonia emissions from cattle slurry treated with anaerobic digestion. Chem. Eng. Trans. 15, 247–254.
IPCC (2006). “2006 IPCC guidelines for national greenhouse gas inventories,” in Prepared by the National Greenhouse Gas Inventories Programme. eds H. S. Eggleston, L. Buendia, K. Miwa, T. Ngara, and K. Tanabe K (Hayama: IGES).
IPCC (2014). “Climate change 2014: synthesis report,” in Contribution of Working Groups I, II and III to the Fifth Assessment Report of the Intergovernmental Panel on Climate Change. eds Core Writing Team, R. K. Pachauri, and L. A. Meyer (Geneva: IPCC).
Jin, T., Shimizu, M., Marutani, S., Desyatkin, A. R., Iizuka, N., Hata, H., et al. (2010). Effect of chemical fertilizer and manure application on N2O emission from reed canary grassland in Hokkaido, Japan. Soil Sci. Plant Nutr. 56, 53–65. doi: 10.1111/j.1747-0765.2010.00447.x
Katayanagi, N., and Hatano, R. (2012). N2O emissions during the freezing and thawing periods from six fields in a livestock farm, southern Hokkaido, Japan. Soil Sci. Plant Nutr. 58, 261–271. doi: 10.1080/00380768.2012.670810
Katayanagi, N., Sawamoto, T., Hayakawa, A., and Hatano, R. (2008). Nitrous oxide and nitric oxide fluxes from cornfield, grassland, pasture and forest in a watershed in Southern Hokkaido, Japan. Soil Sci. Plant Nutr. 54, 662–680. doi: 10.1111/j.1747-0765.2008.00284.x
Lal, R. (2020). Managing soils for negative feedback to climate change and positive impact on food and nutritional security. Soil Sci. Plant Nutr. 66, 1–9. doi: 10.1080/00380768.2020.1718548
Li, M., Shimizu, M., and Hatano, R. (2015). Evaluation of N2O and CO2 hot moments in managed grassland and cornfield, southern Hokkaido, Japan. Catena 133, 1–13. doi: 10.1016/j.catena.2015.04.014
Linn, D. M., and Doran, J. W. (1984). Effect of water filled pore space on carbon dioxide and nitrous oxide production in tilled and non-tilled soils. Soil Sci. Soc. Am. J. 48, 1267–1272. doi: 10.2136/sssaj1984.03615995004800060013x
Matsunaka, T., Kumai, M., and Senntoku, A. (2003). Evaluation of anaerobically digested cattle slurry as a nitrogen source for orchard grass. Japan. J. Soil Sci. Plant Nutr. 74, 31–38. doi: 10.20710/dojo.74.1_31
Matsuura, S., Miyata, A., Mano, M., Hojito, M., Mori, A., Kano, S., et al. (2014). Seasonal carbon dynamics and the effects of manure application on carbon budget of a managed grassland in a temperate, humid region in Japan. Grassland Sci. 60, 76–91. doi: 10.1111/grs.12042
Mori, A., and Hojito, M. (2012). Effect of combined application of manure and fertilizer on N2O fluxes from a grassland soil in Nasu, Japan. Agricult. Ecosyst. Environ. 160, 40–50. doi: 10.1016/j.agee.2011.07.018
Mori, A., and Hojito, M. (2015). Effect of dairy manure type and supplemental synthetic fertilizer on methane and nitrous oxide emissions from a grassland in Nasu, Japan. Soil Sci. Plant Nutr. 61, 347–358. doi: 10.1080/00380768.2014.981676
Mu, Z., Kimura, S. D., and Hatano, R. (2006). Estimation of global warming potential from upland cropping systems in central Hokkaido, Japan. Soil Sci. Plant Nutr. 52, 371–377. doi: 10.1111/j.1747-0765.2006.00046.x
Mukumbuta, I., and Hatano, R. (2020). Do tillage and conversion of grassland to cropland always deplete soil organic carbon? Soil Sci. Plant Nutr. 66, 76–83. doi: 10.1080/00380768.2019.1676135
Mukumbuta, I., Shimizu, M., and Hatano, R. (2017a). Mitigating global warming potential and greenhouse gas intensities by applying composted manure in cornfield: a 3-year field study in an Andosol soil. Agriculture 7, 1–20. doi: 10.3390/agriculture7020013
Mukumbuta, I., Shimizu, M., Jin, T., Nagatake, A., Hata, H., Kondo, S., et al. (2017b). Nitrous and nitric oxide emissions from a cornfield and managed grassland: 11 years of continuous measurement with manure and fertilizer applications, and land-use change. Soil Sci. Plant Nutr. 63, 185–199. doi: 10.1080/00380768.2017.1291265
Mukumbuta, I., Uchida, Y., and Hatano, R. (2018). Evaluating the effect of liming on N2O fluxes from denitrification in an Andosol using the acetylene inhibition and N-15 isotope tracer methods. Biol. Fertil. Soils 54, 71–81. doi: 10.1007/s00374-017-1239-4
Nagatake, A., Mukumbuta, I., Yasuda, K., Shimizu, M., Kawai, M., and Hatano, R. (2018). Temporal dynamics of nitrous oxide emission and nitrate leaching in renovated grassland with repeated application of manure and/or chemical fertilizer. Atmosphere 9:485. doi: 10.3390/atmos9120485
Nakamura, M., Yuyama, Y., Fujikawa, T., Yamaoka, M., and Shimizu, N. (2008). GHG emission mitigation effect by methane fermentation system with digested liquid to utilize as fertilizer. J. Japan. Soc. Irrigat. Drainage Rural Eng. 76, 981–985. doi: 10.11408/jjsidre2007.76.11_981
Nakano, T., Sawamoto, T., Morishita, T., Inoue, G., and Hatano, R. (2004). A comparison of regression methods for estimating soil-atmosphere diffusion gas fluxes by a closed-chamber technique. Soil Biol. Biochem. 36, 107–113. doi: 10.1016/j.soilbio.2003.07.005
Paustian, K., Andrén, O., Janzen, H. H., Lal, R., Smith, P., Tian, G., et al. (1997). Agricultural soils as a sink to mitigate CO2 emissions. Soil Use Manag.13, 230–244. doi: 10.1111/j.1475-2743.1997.tb00594.x
Rochette, P., Angers, D. A., Chantigny, M. H., Bertrand, N., and Côté, D. (2004). Carbon dioxide and nitrous oxide emissions following fall and spring applications of pig slurry to an agricultural soil. Soil Sci. Soc. Am. J. 68, 1410–1420. doi: 10.2136/sssaj2004.1410
Ruser, R., Flessa, H., Schilling, R., Beese, F., and Munch, J. C. (2001). Effect of crop-specific field management and N fertilization on N2O emissions from a fine-loamy soil. Nutr. Cycling Agroecosyst. 59, 177–191. doi: 10.1023/A:1017512205888
Ryals, R., Kaiser, M., Torn, M. S., Berhe, A. A., and Silver, W. L. (2014). Impacts of organic matter amendments on carbon and nitrogen dynamics in grassland soils. Soil Biol. Biochem. 68, 52–61. doi: 10.1016/j.soilbio.2013.09.011
Ryals, R., and Silver, W. L. (2013). Effects of organic matter amendments on net primary productivity and greenhouse gas emissions in annual grasslands. Ecol. Appl. 23, 46–59. doi: 10.1890/12-0620.1
Sawamoto, T., Yoshida, R., Abe, K., and Matsunaka, T. (2010). No significant difference in N2O emission, fertilizer-induced N2O emission factor and CH4 absorption between anaerobically digested cattle slurry and chemical fertilizer applied timothy (Phleum pratense L.) sward in central Hokkaido, Japan. Soil Sci. Plant Nutr. 56, 492–502. doi: 10.1111/j.1747-0765.2010.00465.x
Schlesinger, W. H., and Jeffrey, A. A. (2000). Soil respiration and the global carbon cycle. Entomol. Exp. Appl. 103, 239–248. doi: 10.1023/A
Schulze, E. D., Wirth, C., and Heimann, M. (2000). Managing forests after Kyoto. Science 289, 2058–2059. doi: 10.1126/science.289.5487.2058
Shimizu, M., Hatano, R., Arita, T., Kouda, Y., Mori, A., Matsuura, S., et al. (2013). The effect of fertilizer and manure application on CH4 and N2O emissions from managed grasslands in Japan. Soil Sci. Plant Nutr. 59, 69–86. doi: 10.1080/00380768.2012.733926
Shimizu, M., Limin, A., Desyatkin, A. R., Jin, T., Mano, M., Ono, K., et al. (2015). Effect of manure application on seasonal carbon fluxes in a temperate managed grassland in Southern Hokkaido, Japan. Catena 133, 474–485. doi: 10.1016/j.catena.2015.05.011
Shimizu, M., Marutani, S., Desyatkin, A. R., Jin, T., Hata, H., and Hatano, R. (2009). The effect of manure application on carbon dynamics and budgets in a managed grassland of Southern Hokkaido, Japan. Agricult. Ecosyst. Environ. 130, 31–40. doi: 10.1016/j.agee.2008.11.013
Shimizu, M., Marutani, S., Desyatkin, A. R., Jin, T., Nakano, K., Hata, H., et al. (2010). Nitrous oxide emissions and nitrogen cycling in managed grassland in Southern Hokkaido, Japan. Soil Sci. Plant Nutr. 56, 676–688. doi: 10.1111/j.1747-0765.2010.00496.x
Soussana, J. F., Allard, V., Pilegaard, K., Ambus, P., Amman, C., Campbell, C., et al. (2007). Full accounting of the greenhouse gas (CO2, N2O, CH4) budget of nine European grassland sites. Agricult. Ecosyst. Environ. 121, 121–134. doi: 10.1016/j.agee.2006.12.022
Takakai, F., Morishita, T., Hashidoko, Y., Darung, U., Kuramochi, K., Dohong, S., et al. (2006). Effects of agricultural land-use change and forest fire on N2O emission from tropical peatlands, Central Kalimantan, Indonesia. Soil Sci. Plant Nutr. 52, 662–674. doi: 10.1111/j.1747-0765.2006.00084.x
The Fifth Committee for Soil Classification Nomenclature of the Japanese Society of Pedology (2017). Soil Classification System of Japan. Japanese Society of Pedology. Available online at: http://pedology.jp/img/Soil%20Classification%20System%20of%20Japan.pdf (accessed December 25, 2020).
Toma, Y., Fernandez, F. G., Sato, S., Izumi, M., Hatano, R., Yamadam, T., et al. (2011). Carbon budget and methane and nitrous oxide emissions over the growing season in a Miscanthus sinensis grassland in Tomakomai, Hokkaido, Japan. Glob. Change Biol. Bioenergy 3, 116–134. doi: 10.1111/j.1757-1707.2010.01070.x
Toma, Y., and Hatano, R. (2007). Effect of crop residue C:N ratio on N2O emissions from gray lowland soil in Mikasa, Hokkaido, Japan: Original article. Soil Sci. Plant Nutr. 53, 198–205. doi: 10.1111/j.1747-0765.2007.00125.x
Toma, Y., Kimura, S. D., Hirose, Y., Kusa, K., and Hatano, R. (2007). Variation in the emission factor of N2O derived from chemical nitrogen fertilizer and organic matter: a case study of onion fields in Mikasa, Hokkaido, Japan. Soil Sci. Plant Nutr. 53, 692–703. doi: 10.1111/j.1747-0765.2007.00184.x
Keywords: CH4, global warming potential, manure, methane fermentation digestive fluid, slurry, N2O, soil carbon sequestration
Citation: Kitamura R, Sugiyama C, Yasuda K, Nagatake A, Yuan Y, Du J, Yamaki N, Taira K, Kawai M and Hatano R (2021) Effects of Three Types of Organic Fertilizers on Greenhouse Gas Emissions in a Grassland on Andosol in Southern Hokkaido, Japan. Front. Sustain. Food Syst. 5:649613. doi: 10.3389/fsufs.2021.649613
Received: 05 January 2021; Accepted: 12 March 2021;
Published: 08 April 2021.
Edited by:
Nikolaos Katsoulas, University of Thessaly, GreeceReviewed by:
Marta Andrea Alfaro, Institute of Agricultural Research, ChileLutz Merbold, Agroscope, Switzerland
Copyright © 2021 Kitamura, Sugiyama, Yasuda, Nagatake, Yuan, Du, Yamaki, Taira, Kawai and Hatano. This is an open-access article distributed under the terms of the Creative Commons Attribution License (CC BY). The use, distribution or reproduction in other forums is permitted, provided the original author(s) and the copyright owner(s) are credited and that the original publication in this journal is cited, in accordance with accepted academic practice. No use, distribution or reproduction is permitted which does not comply with these terms.
*Correspondence: Ryusuke Hatano, aGF0YW5vQGNoZW0uYWdyLmhva3VkYWkuYWMuanA=