- 1School of Basic Sciences, University of Santiago de Cali, Cali, Colombia
- 2International Center for Tropical Agriculture (CIAT), Palmira, Colombia
- 3Department of Nutrition, Faculty of Animal Science, Universidad Nacional Agraria La Molina, Lima, Peru
- 4Faculty of Natural and Exact Sciences, Universidad del Valle, Cali, Colombia
- 5Mohammed VI Polytechnic University (UM6P), AgroBioSciences (AgBS), Agricultural Innovations and Technology Transfer Centre (AITTC), Ben Guerir, Morocco
- 6Department of Animal Production, Faculty of Agricultural Sciences, Universidad Nacional de Colombia, Medellin, Colombia
- 7Institute of Biological, Environmental and Rural Sciences, Aberystwyth, United Kingdom
Forage grass nutritional quality directly affects animal feed intake, productivity, and enteric methane (CH4) emissions. This study evaluated the nutritional quality, in vitro enteric CH4 emission potential, and optimization of diets based on two widely grown tropical forage grasses either alone or mixed with legumes. The grasses Urochloa hybrid cv. Cayman (UHC) and U. brizantha cv. Toledo (UBT), which typically have low concentrations of crude protein (CP), were incubated in vitro either alone or mixed with the legumes Canavalia brasiliensis (CB) and Leucaena diversifolia (LD), which have higher CP concentrations. Substitution of 30% of the grass dry matter (DM) with CB or LD did not affect gas production or DM degradability. After 96 h of incubation, accumulated CH4 was 87.3 mg CH4 g−1 DM and 107.7 mg CH4 g−1 DM for the grasses alone (UHC and UBT, respectively), and 100.7 mg CH4 g−1 DM and 113.2 mg CH4 g−1 DM for combined diets (70% grass, 15% CB, and 15% LD). Diets that combined legumes (CB or LC) and grass (UHC or UBT) had higher CP contents, gross, and metabolizable energy (GE, ME, respectively) densities, as well as lower concentrations of neutral detergent fiber (NDF) and acid detergent lignin (ADL). The ME and nutritional variables such as NFD, tannins (T), and CP showed a positive correlation with in vitro net gas production, while ruminal digestibility was affected by CP, ADL, T, and GE. Optimal ratios of components for ruminant diets to reduce rumen net gas production and increase protein content were found with mixtures consisting of 60% grass (either UHC or UBT), 30% CB, and 10% LD. However, this ratio did not result in a decrease in CH4 production.
Introduction
Cattle and other ruminant livestock are a significant food source for the global human population and are good at converting fibrous species indigestible by humans into highly nutritious food (Wilkinson, 2011). This metabolic conversion is possible due to rumen-dwelling microorganisms that can break down low-quality fibrous plant material, with the formation of gases (methane [CH4] and CO2) that are expelled into the atmosphere, plus energy-rich compounds that are required to perform vital functions for both the population of rumen organisms and the host animal (Hyland et al., 2016; Cammack et al., 2018). However, this symbiosis between microorganisms and ruminants is negatively affected by the consumption of diets that are low in protein and high in insoluble fiber (Figueiras et al., 2010).
Therefore, in the search for suitable diets based on tropical forages that simultaneously meet the nutritional needs of livestock and decrease their impact on the environment, mixed production (i.e., agro-pastoral, silvopastoral, and agro-silvopastoral) systems are proposed as a viable option (Arango et al., 2020). In these systems, forage grasses and legumes are combined toward a process of sustainable intensification of livestock production, aiming at not only improving available feed for ruminants but also to restore degraded lands and increase system resilience to more frequent droughts and floods that are associated with climate change (Rao et al., 2015; Ku-Vera et al., 2020a). Furthermore, if properly managed, grass-legume tropical pastures can potentially accumulate large amounts of soil organic carbon; improve chemical, physical, and biological soil health characteristics; fix atmospheric nitrogen; inhibit soil nitrification; improve animal productivity and animal welfare; and reduce CH4 emissions per unit of livestock product (Peters et al., 2012; Rao et al., 2015; Aynekulu et al., 2020; Ku-Vera et al., 2020a; Vazquez et al., 2020).
Despite the multiple benefits of silvopastoral systems (SPS), the use of grass-legume associations is limited in tropical agricultural systems by several factors. These include reduced plant growth associated with interspecies competition and shading, the potentially low palatability of legumes, the reluctance of farmers to adopt new species due to a general lack of awareness of the benefits of these systems, and the limited availability of legume seeds (Karsten and Carlassare, 2002). However, the specific effects of each association depend on the plant species involved.
A widely studied species in the tropics is the shrub legume Leucaena sp., which when planted in SPS provides multiple benefits to grazing livestock, including the provision of high quality protein throughout the year without the need for nitrogen inputs from synthetic fertilizers (Shelton and Dalzell, 2007; Cook et al., 2020), increased forage biomass (Naranjo et al., 2012; Gaviria et al., 2015), improved voluntary forage intake (Cuartas Cardona et al., 2015; Gaviria-Uribe et al., 2015), increased animal productivity (Cuartas Cardona et al., 2014), and reduced CH4 emissions (Molina et al., 2015; Montoya-Flores et al., 2020). Canavalia sp. is a herbaceous legume that can grow in various Latin America locations by direct seeding, alone or in combination with tropical grasses, characterized by high concentrations of protein and high digestibility. However, the relationships between CH4 emissions (in vitro) and nutritional quality of the legumes Leucaena diversifolia (more information is available on Leucaena leucocephala) and Canavalia brasiliensis have been little studied despite their potentials when associated with tropical grasses such as Urochloa, which is an important forage grass genus that is widely used in Latin America, Australia and parts of Asia (Low, 2011).
This work aimed to evaluate the effect of mixing different ratios of relevant tropical grasses (Urochloa sp. cv. Cayman and Toledo) and legumes (Canavalia brasiliensis and Leucaena diversifolia) on diet nutritional quality, rumen degradability, and net in vitro total gas and CH4 production. In addition, using optimization analysis, we aimed to find out the ideal proportions of grass and legume(s) to not only reduce net gas production (as a possible indicator of CH4) at the rumen level but also to increase crude protein (CP) content in the diet.
Materials and Methods
Location
Forage samples were collected in the rainy season between April and May of 2016 from a silvopastoral experiment established at the International Center for Tropical Agriculture (CIAT), Palmira, Valle del Cauca, Colombia (3° 30′ 17′′ N and 76° 21′ 24′′ E) at an altitude of 965 meters above sea level. Soils are mollisols, with a pH of 7.2. During sample collection, average temperature was 25.4°C, average relative humidity was 65%, and total precipitation was 231 mm (5.5 mm day−1) and these conditions allowed good regrowth of forage for 56 days.
Forage Samples and Mixed Diets
The tropical forage species evaluated were the two grasses Urochloa hybrid (CIAT BR02/1752) cv. Cayman (UHC) and Urochloa brizantha (CIAT 26110) cv. Toledo (UBT), the herbaceous legume Canavalia brasiliensis (CIAT 17009) (CB), and the shrub legume Leucaena diversifolia (ILRI 15551) (LD). Forage materials were planted 2 years before the start of the experiment (2014). The forage crops did not receive any fertilizers, pesticides or irrigation. One kilogram of each of UHC, UBT, and CB were collected at the vegetative stage of development before the beginning of flowering (after 6 weeks of regrowth), by cutting at 10 cm above soil level. Young leaf and stem samples (2:1 ratio) of LD were also manually collected. Two gas production experiments were conducted at two different times: one with UHC, CB, and LD forages, and the other with UBT, CB, and LD. In each experiment the individual forages were evaluated alone (100% UHC or 100% UBT, 100% CB, and 100% LD) and in mixtures with different proportions of DM of grasses and legumes. We used the order (UHC or UBT) - CB - LD, on a DM basis, with the treatment proportions of 0-50-50; 50-50-0 and 50-0-50 which correspond to a mixture in equal proportions (50%) between two species, either a grass with one of the two legumes or with both legumes without UHB or UBT. The treatment denoted 70-30-0 corresponds to the incubated mixture of 70% grass DM plus 30% CB DM, while 70-15-15 refers to the DM proportions 70% UHC or UBT plus 15% CB and 15% LD. Finally, the treatment: 33.3-33.3-33.3, indicates a mixture in equal DM proportions of 33% of the forages (UHC or UBT): CB: LB. A total of nine different treatments were evaluated in each of the two experiments. The proportions of the forages incubated were determined in order to perform a simplex-centroid mixture design.
Nutritional Quality
Samples were evaluated at the Forage Quality and Animal Nutrition Laboratory of CIAT. Samples were dried in a Memmert® UF 750 forced air oven at 60°C for 72 h and until constant weight was achieved. Samples were ground using a cutting mill (Retsch® SM 100, Haan, Germany) with a 1 mm sieve. The content of acid detergent fiber (ADF) and neutral detergent fiber (NDF) was determined using an Ankom 2000 fiber analyzer (Ankom Technology Corp., Macedon, NY, USA) following the method of Van Soest et al. (1991). The ash content was determined using the AOAC method (Association of Official Analytical Chemists, 1990); organic matter (OM) content was calculated as 1,000—ash concentration in g kg−1 DM. Gross energy (GE) density was determined using a Parr 6400 (Parr Instrument Company, Illinois, USA) isoperibol calorimeter in accordance with International Standardization Organization, 1998: ISO 9831:1998 specifications. Acid detergent lignin (ADL) content was determined using the method of ANKOM (2016). Total nitrogen content was determined using an autoanalyzer (Skalar Analytical B.V. Breda, Holland) after digestion with sulfuric acid and selenium (Krom, 1980; Searle, 1984). Crude protein (CP) content was estimated as 6.25 × total nitrogen content. Total phenol and tannin contents were determined using Folin-Ciocalteu's method (Makkar, 2003). The metabolizable energy (ME) was calculated according to Lindgren (1983) from the in vitro digestibility value obtained at 96 h.
In vitro Gas Production and Dry Matter Degradation
The methodology of Theodorou et al. (1994) was employed for in vitro gas production. Rumen fluid was drawn and mixed from two rumen-fistulated Brahman steers, grazing on a star grass (Cynodon plectostachyus)-dominated pasture with ad libitum access to mineralized salt. Briefly, ~1.0 g of dried/ground samples were placed in individual Wheaton bottles and inoculated with a rumen fluid/buffer solution mixture. After inoculation, all bottles were depressurized (at time 0) and placed in a water bath set at 39°C. Thereafter, pressure and volume measurements were taken at 3, 6, 9, 12, 24, 36, 48, 60, 72, and 96 h of incubation. After each reading, the bottles were gently shaken and placed back in the water bath. Pressure measurements were taken using an 8,40,065 wide-range pressure gauge (Sper Scientific, Arizona, USA) and a PS100 2-bar pressure transducer (Lutron Electronic Enterprise Co. Ltd., Taipei, Taiwan) connected to a three-way valve. The first output was connected to a 1′′ 22 G needle (25 mm × 0.7 mm), the second output to the transducer, the third to a 60 mL syringe, making it possible to record the gas volume removed at each time point required to reduce the bottle internal pressure to atmospheric pressure and to save gas samples for subsequent chromatographic analysis. Upon completion of the test, the contents of the bottles were filtered and dried in a forced-air oven at 105°C for 24 h to determine DM loss. Dry matter degradability (DMD, g kg−1) was calculated for each sample as the change in sample DM weight following incubation, divided by the starting sample DM weight, multiplied by 1,000.
Accumulated gas production (AGP) curves were fitted to the Gompertz model, as proposed by Lavrenčič et al. (1997), using the CurveExpert Professional® software, version 2.4.0 (Hyams, 2016). This model was used to evaluate the gas production points using the following equation:
Where a, b, and c are the equation parameters [a, maximum gas production; b, the difference between initial and final gas at time x; c, specific gas accumulation rate; and t, time (hours; h)], the accumulated gas production results were expressed on an organic matter (OM) basis. Other biologically significant values were calculated based on parameters a, b, and c. These included the time at inflection point (TIP, h), gas volume at inflection point (GIP, mL), maximum gas production rate (MGPR, mL h−1), and lag phase (LP or microbial settlement, h). These values were estimated using the following formulas:
where “e” is Euler's number, ca. 2.72.
Methane Measurements
Methane concentration was quantified in all gas samples collected at the Greenhouse Gas Laboratory of CIAT using a gas chromatograph (Shimadzu, Kyoto, Japan) equipped with a flame ionization detector. A three-meter long HayeSep N column was used and the mobile phase was high purity nitrogen at a flow rate of 35 mL min−1. The oven, injector, and detector temperatures were 250, 100, and 325°C, respectively.
Experimental Design and Data Analysis
The nutritional quality, DMD, and CH4 production data were analyzed using a randomized complete block design, where each treatment had three replicates at each time the readings were taken and three inoculums, the latter being the blocking factor. Mean comparisons were made using Tukey's test when a significant treatment effect (P < 0.05) was identified. To check for the normality of data distribution, the Shapiro-Wilk test was conducted on the original residuals using PROC GLM. To determine the correlations among the above variables, type II linear regressions were carried out using the bisector model linear functional relationship procedure of Genstat 18th Edition (VSN International Ltd., Hemel Hempstead, UK). All analyses were conducted using SAS® 9.4 Software (SAS Institute, 2012).
The completely randomized model was:
Where: Yij: observation of the j-th repetition of the i-th treatment; μi: mean value of the i-th treatment, eij: experimental error of unit ij
The linear regression model employed was:
Where: Yi: observation of the i-th variable response, corresponding with the i-th value xi of the x predictive variable (dependent variable); β0 and β1 are the regression parameters; xi is the independent variable; and e: experimental error of unit i.
Regression analysis of nutritional quality data (NDF, CP, ADL, GE, and ME) against AGP and DMD parameters was carried out to identify an optimal mixed-diet in which the nutritional quality could be improved (specifically CP) while at the same time reducing gas production. A simplex-centroid mix design was run, using the special cubic model as a response adjustment model using the StatPoint Technologies Inc., 2010: Statgraphics® software (Centurion XVI, version 16.1.18).
The complete simplified special cubic model was:
Where (y) is the crude protein (CP g kg−1) response variable or accumulated gas production (mL g−1 OM), x1, x1,2, x1,2,3 are the regression coefficients for individual ingredients and mix interactions; G, C, and L are the relative ratios of forage components (grass, CB, and LD).
Results
Nutritional Quality
The CP content of LD was 3.5 times greater than that of both grasses (Tables 1, 2 for UHC and UBT, respectively) and it was also greater than that of CB (P ≤ 0.05). The NDF contents ranged from 492 (CB) to 700 g kg−1 DM (100% UBT) (P < 0.0001) while the concentrations of ADF were less variable, ranging between 344 and 399 g kg−1 DM. For the treatments where different proportions of legumes and grasses were mixed, in both experiments it was observed that the CP content decreased as the proportion of grasses increased, however, the opposite occurred with the NDF content. The lignin content of CB was similar to that of UBT, whereas the lignin content of LD was similar to that of UHC. Legumes, especially LD, have higher GE contents than that reported for 100% grasses treatments or when grasses are replaced up to 30% by legumes (P = 0.001), however, this trend is reversed when ME is calculated, since LD treatments or the combination of legumes in equal proportions (50% LD + 50% CB) obtain the lowest values of ME. Much higher concentrations of phenols and tannins were measured in LD compared to both grasses and CB, and the concentrations of both of secondary metabolites were also higher in CB than in both grasses.
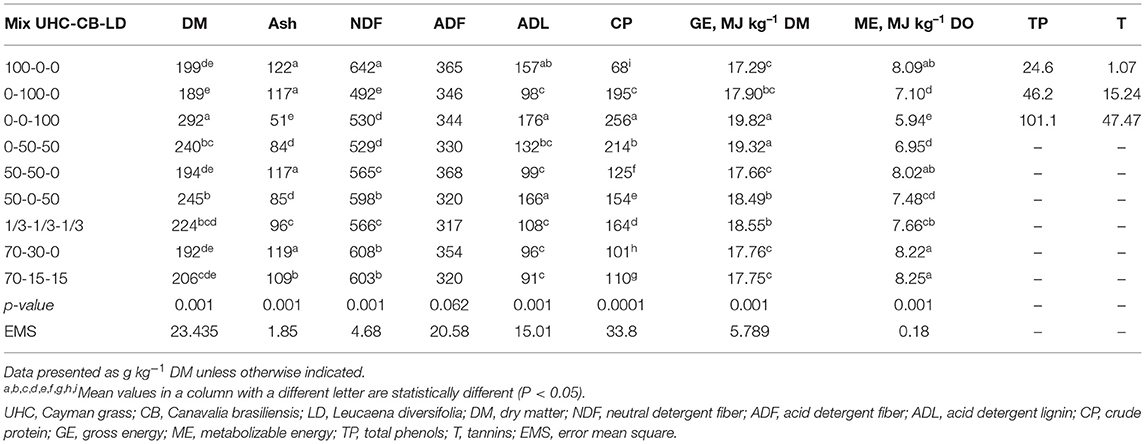
Table 1. Mean chemical composition of Urochloa hybrid grass cv. Cayman (UHC) and the two forage legumes, C. brasiliensis (CB) and L. diversifolia (LD), and their mixed proportions used in the study.
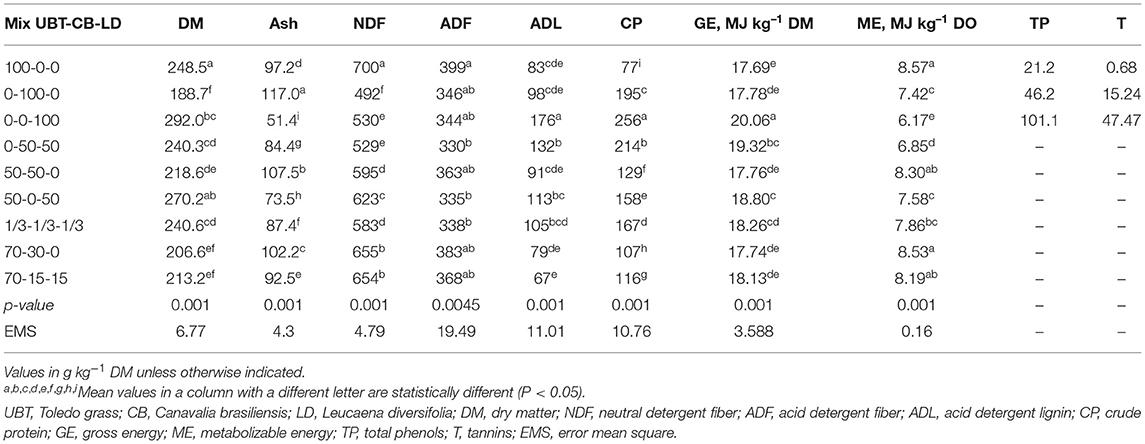
Table 2. Mean chemical composition of Urochloa brizantha cv. Toledo (UBT) and the two forage legumes, C. brasiliensis (CB) and L. diversifolia (LD), and their mixed proportions used in the study.
Gas Production and Dry Matter Degradation
The total volume of gas produced during the fermentation process ranged from 150 to 255 mL g−1 OM (Tables 3, 4 for UHC and UBT, respectively). The diet combinations from both systems had a very fast fermentation rate, as evidenced by the low TIP and LP values. The lowest total accumulated gas production values at 96 h occurred with the LD-only treatment in both systems, a value that was almost 0.6 of that from the diet comprising UHC, LD, and CB in a ratio of 70:15:15 and UBT-only diet, respectively (Figures 1, 2).
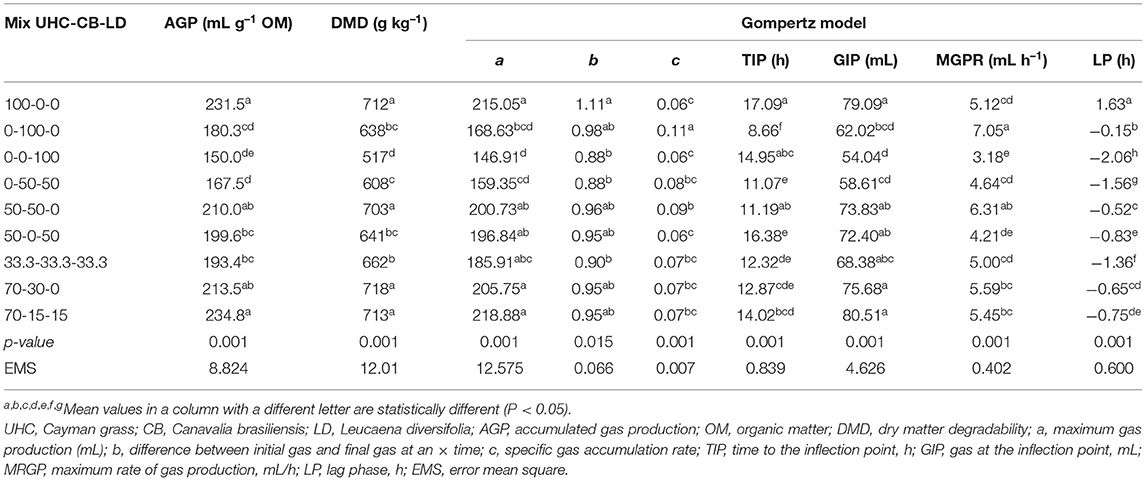
Table 3. Accumulated gas production (AGP; mL g−1 OM), dry matter degradability (DMD), and profiles of the adjustment made using the Gompertz model for UHC, CB, LD, and their mixes.
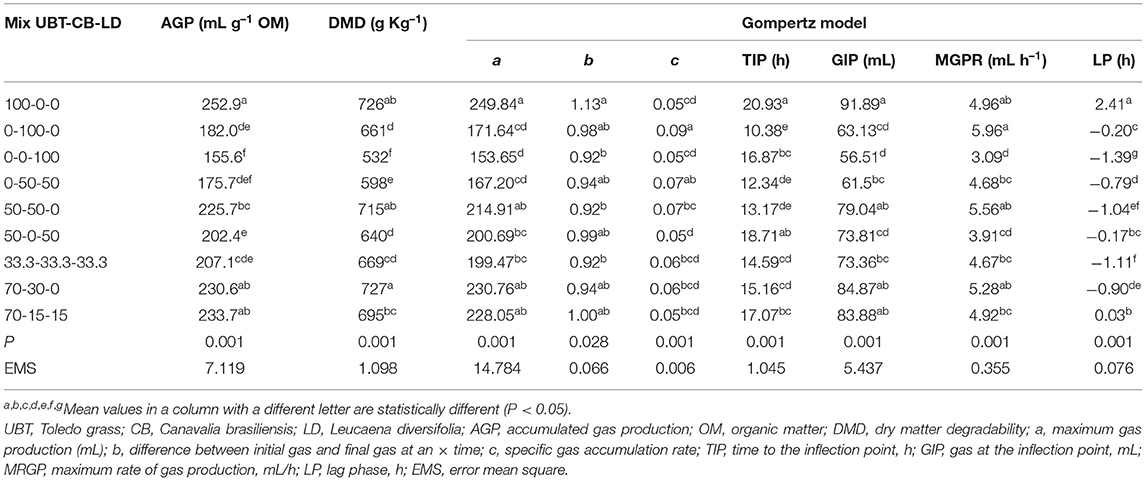
Table 4. Accumulated gas production (AGP; mL g−1 OM), dry matter degradability (DMD), and profiles of the adjustment made using the Gompertz model for UBT, CB, LD, and their mixes.
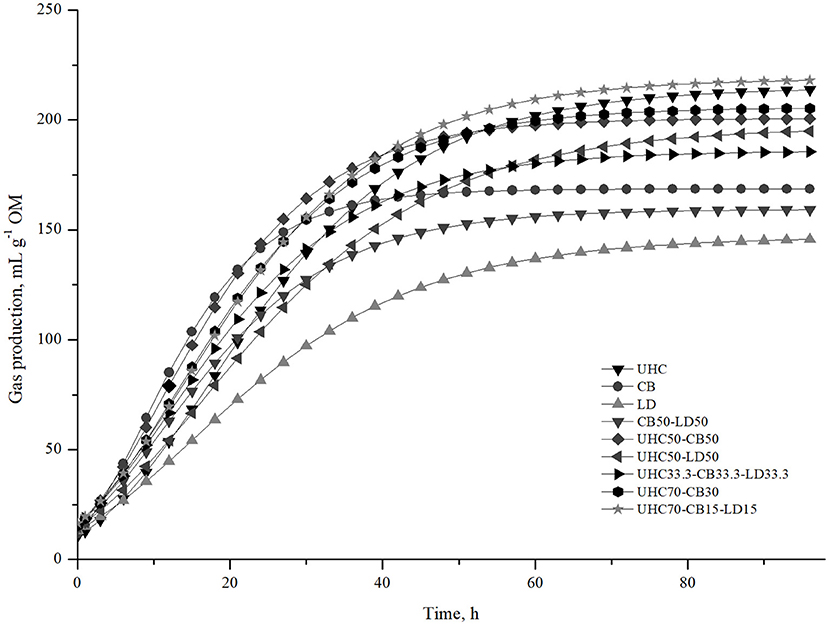
Figure 1. Modeled mean accumulated gas production (mL g−1 OM) for UHC, CB, LD, and 6 dietary mixtures. UHC, Cayman 100%; CB, Canavalia 100%; LD, Leucaena 100%; CB50LD50, Canavalia 50% + Leucaena 50%; UHC50CB50, Cayman 50% + Canavalia 50%; UHC50LD50, Cayman 50% + Leucaena 50%; UHC33.3CB33.3LD33.3, Cayman 33.3% + Canavalia 33.3% + Leucaena 33.3%; UHC70CB30, Cayman 70% + Canavalia 30%; UHC70CB15LD15, Cayman 70% + Canavalia 15% + Leucaena 15%.
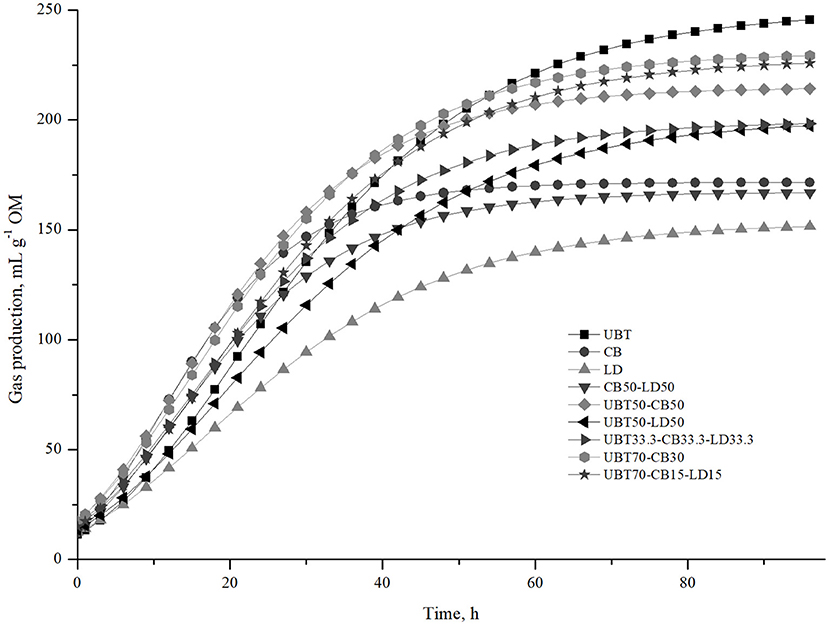
Figure 2. Accumulated gas production (AGP; mL g−1 OM) for UBT, CB, LD, mixed diets. UBT, Toledo 100%; CB, Canavalia 100%; LD, Leucaena 100%; CB50LD50, Canavalia 50% + Leucaena 50%; UBT50CB50, Toledo 50% + Canavalia 50%; UBT50LD50, Toledo 50% + Leucaena 50%; UBT33.3CB33.3LD33.3, Toledo 33.3% + Canavalia 33.3% + Leucaena 33.3%; UBT70CB30, Toledo 70% + Canavalia 30%; UBT70CB15LD15, Toledo 70% + Canavalia 15% + Leucaena 15%.
The highest (inverse) correlation was observed between the content of CP and the AGP values (R2 = 0.919; Table 5). In contrast, ME content and gas production were positively related, i.e., the higher the ME content, the higher the gas production (R2 = 0.907). Other strong inverse relationships were observed between the concentration of ADL and DMD g kg−1, GE density and DMD g kg−1, and between T and AGP, and T and DMD.
The correlation analysis results provided the basis for carrying out the optimization objective of selecting the best forage combination for increasing the CP concentration of a dietary mix while decreasing AGP. In the case of the UHC-based treatments, the percentage variance accounted for by these two parameters was 87.9% for CP and 84.3% for AGP. In the UBT-based treatments, the percentage variance accounted for CP and AGP was 87.8 and 87.9%, respectively. Table 6 shows the restrictions used for obtaining a suitable inclusion of grasses and legumes, as well as the ratio of the best mix found (optimized) and the CP and AGP obtained with the specific mix.
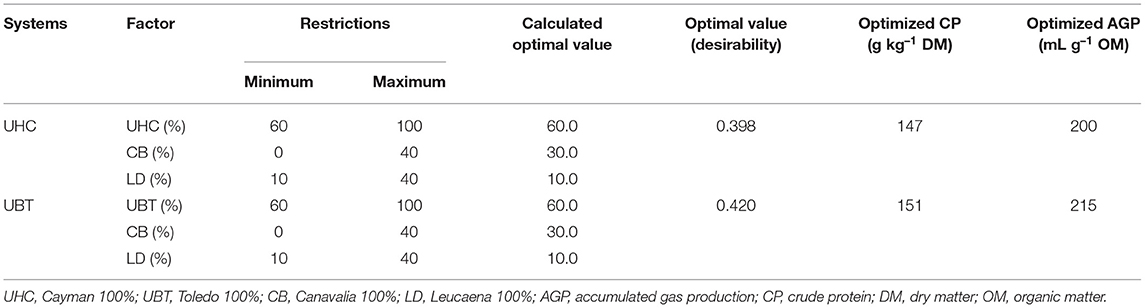
Table 6. Optimization of the crude protein (CP; maximize) and accumulated gas production (AGP; minimize) response variables in the UHC and UBT forage systems.
Methane Production
When incubated alone, CH4 production from CB started declining rapidly after 60 h in measurements of both grasses (Tables 7, 8 for UHC and UBT, respectively). The same CH4 accumulation trend was observed with the other diets for 96 h. It is worth noting that the largest production of CH4 in the UHC diets came from the 70% UHC: 15% CB: 15% LD diet. The incorporation of legumes into the UBT system contributed to decreased CH4 production compared to the 100% of UBT diet.
Discussion
Feeds intended for livestock are typically evaluated individually to determine their nutritional values and not integrated with a diet (Tang et al., 2008). Evaluations of individual forages does not allow us to determine interactions with other dietary components in the digestion process (Moss et al., 1992). Although the values of some nutritional parameters of diet components are additive (e.g., CP concentrations), there are possible interactions and synergies between different feeds in a diet and their nutritional values (e.g., energy yield and CP concentrations) that could not be evaluated independently (Tang et al., 2008). This situation can be explained at the rumen level, because depending on the type of diet, some synergy or antagonism may develop due to co-existence of nutrients and their interactions with different microorganisms (i.e., bacteria, protozoa, fungi, and methanogenic archaea) in the rumen (Cammack et al., 2018).
In this investigation, great variability in nutritional composition was found among the different forage diets. For example, the legumes contained twice as much CP as the two grasses evaluated, and the grasses had higher concentrations of NDF than the legumes. Similarly, concentrations of phenolic compounds were lower in the grasses than the legumes. These findings concur with data reported in the literature for these tropical species (Lee, 2018; Cook et al., 2020; Gaviria-Uribe et al., 2020), where CP values for grasses can range between 40 and 140 g kg−1 DM, and for both legumes studied here, shrub and herbaceous, ranged between 190 and 250 g CP kg−1 DM. However, the CP content obtained in the present study was slightly lower than that reported by Peters et al. (2002) for U. brizantha cv. Toledo who stated that under optimal conditions CP content ranges between 90 and 120 g kg−1 DM. Likewise, the NDF content was within the range of 600 and 800 g kg−1 DM reported for U. brizantha sp. (Cook et al., 2020; Gaviria-Uribe et al., 2020). However, forage quality has been shown to be closely related to pasture age (Vendramini et al., 2014; Gaviria-Uribe et al., 2020) and the time of the year (Demarchi et al., 2016; Abdalla et al., 2019). The ADL content of Urochloa grasses was 86 and 157 g/kg DM, both values were between the ranges reported by Wassie et al. (2018), according to these authors ADL content can vary between 91.2 and 186.9 g/kg depending on ecotype, regrowth age (60, 90 and 120 d) and altitude of the sowing site (1,230, 1,774, and 2,650 masl). It is noteworthy that little information is available on the ADL content of Urochloa hybrid cv. Cayman. The ME values found for the legume L. diversifolia are slightly lower (8.6 MJ ME kg−1 DM) than the results reported by Geleti et al. (2013), while the ME for grasses are above those obtained by Nguku (2015) for 9 grasses of the genus Urochloa, whose values ranged between 6.6 and 5.9 MJ ME kg−1 DM. However, this variable, as well as the rest of the nutritional components of the diet, can vary according to the age of the species and time of year (Givens et al., 1993, Nguku, 2015). In the present investigation, there were differences in ME content between legumes and grasses, contrary to what was reported by Evitayani et al. (2004), who found average values of 7.6 ± 0.14 and 7.3 ± 0.12 MJ ME kg−1 DM for grasses and legumes, respectively. Likewise, the highest ME concentrations were for the treatments: 100-0-0, 70-30-0 or 70-15-15, this may favor the synthesis of microbial proteins at the rumen level (Krizsan et al., 2020).
For the in vitro analysis, the highest gas production and degradability rates were obtained for samples of both grasses that were individually incubated and when 30% legumes were added to these grasses. Despite this, there was a clear pattern and as the level of inclusion of legumes increased, gas production and degradability decreased. Blümmel et al. (1997) suggested that a feed consisting of a mix of different kinds of ingredients can result in asynchrony in releasing nutrients, thus changing both the biomass of microorganisms produced and gas produced by them. In addition, one factor that can affect the fermentation and gas production of feeds is the configuration of their cell wall polysaccharides (Molina-Botero et al., 2020, Valencia-Salazar et al., 2021). Therefore, the digestibility values depend upon their composition of structural carbohydrates, including the concentration of lignin (Barahona and Sánchez, 2005) and the protein included in the diet or treatment evaluated. This postulate agrees with the high correlation values obtained in this study between nutritional compounds such as CP or ADL and variables such as DMD or AGP. Similar results were reported by Lee (2018) where 136 forage plant species or hybrid cultivars grown in 30 countries were evaluated, finding that parameters such as ADF, NDF, ADL content had a correlation >0.7 with DMD or OMD. Although Lee (2018) affirmed that there is a positive correlation (0.62, respectively) between CP and DMD, in the current study there was an inverse correlation between both parameters, perhaps due to the concomitant increase of the content of anti-nutritional compounds associated with the inclusion of CB and/or LD, which could potentially mask the full expression of a diet rich in CP and GE, as was also reported by Jayanegara et al. (2011).
It is clear that to increase our understanding of the nutritive value of forage mixtures composed of tropical forages, the action of the various secondary metabolites (i.e., tannins, saponins) that are present in some legumes must be taken into consideration (Tiemann et al., 2008a,b; Lascano and Cárdenas, 2010). The effect of secondary metabolites depends on their concentration or proportion to the substrate with which they interact. For example, tannins can be found both in the cell wall and inside the cytoplasmic vacuoles of some legumes, primarily in the form of condensed tannins (McAllister et al., 1994; Patra et al., 2017) and their effect depends on their concentration or ratio with the substrate with which they interact. High concentrations of tannins, such as the ones found in diets containing legumes (CB and LD) can delay the digestion of forages by reducing the activity of fibrolytic enzymes (Archimède et al., 2016; Henke et al., 2017; Ku-Vera et al., 2020b). This phenomenon is related to the microbial degradation of structural polysaccharides, and the rate and extent of forage degradation (Archimède et al., 2016; Henke et al., 2017). Likewise, a negative effect has been shown on protein degradation when tannins encapsulate it at low rumen pH (Hess et al., 2003; Archimède et al., 2016). The described tannin effect could explain our results obtained in this study, as in the treatments with an inclusion between 50 and/or 100% of some of these two containing-tannin- legumes (15.2 and 47.5 g kg−1 DM for Canavalia and Leucaena, respectively) and total phenols (46.2 and 101.1 g kg−1 DM) a reduction in digestibility variables and therefore in gas production was observed. These results are in contrast to the 100% grass treatments where the values of tannins and total phenols did not exceed 1.07 g T kg−1 DM and 24.2 g TP kg−1 DM. This observation is consistent with the study of Seresinhe et al. (2012), where a strong inverse relationship was found between tannin concentration and gas production. Tolera et al. (1998) reported condensed tannins content ranging from 7.1 to 13.5% in LD. This concentration of tannins could have bacteriostatic effects on some populations, leading to lower digestibility of the fermented material (Tavendale et al., 2005).
Evaluation of the AGP and CP content in a mix of the three dietary components (grass, CB, and LD) yielded an optimal diet ratio of 60% grass (UHC or UBT), 30% CB, and 10% LD. It should be clarified that although a reduction in gas production was pursued as a measure to reduce CH4 production and emission, it was never intended to be zero. This expectation is because gas production is of great importance to maintain ideal conditions inside the rumen. For example, in the case of cattle it is important that the formation and utilization of metabolic hydrogen is synchronized (Calsamiglia et al., 2005) in the metabolic pathway that is responsible for glucose oxidation (glycolysis). This is required to regenerate the reducing power of cofactors such as NAD+ and FAD+, while increasing the synthesis the synthesis of adenosine triphosphate, promoting the growth of other microbial species (e.g., fibrolytic) and helps to regulate the osmotic pressure inside the rumen (Yokoyama and Johnson, 1993; Calsamiglia et al., 2005). Regarding the proportions established in the evaluated diets, this is consistent with the observations of Rojas et al. (2005), who suggested that the percentage of legumes should range from 30 to 40% in mixtures of grasses and legumes to improve the quality of the diet and have an optimal protein:energy balance at the rumen level. Moreover, these proportions coincide with those found in experiments with ruminants fed with tropical legumes that are rich in tannins and whose results affirm that DM intake was reduced when the amount of CT exceeds 50 g kg−1 DM (Patra and Saxena, 2011). Likewise, cattle systems where the diet is composed of 100% low quality grasses, have low productive indexes due to the low CP concentration, required by ruminal microorganisms for the breakdown of carbohydrates, in addition to a reduction in DM intake due to the high content of structural carbohydrates (Krizsan et al., 2010).
Enteric CH4 emission rates are associated with the physicochemical characteristics of the diet (e.g., CP and NDF contents), which have a direct impact on diet intake (Gaviria-Uribe et al., 2020) and eating frequency (Grant et al., 2015). Several studies have evaluated the effect of adding a legume to a grass on CH4 production both in vitro (Tope et al., 2013; Molina-Botero et al., 2020) and in vivo (Molina-Botero et al., 2019a,b; Gaviria-Uribe et al., 2020; Montoya-Flores et al., 2020). Nevertheless, the conclusions drawn from these studies are unclear, as in some cases the addition of a legume increased in vitro CH4 production (Carulla et al., 2005; Molina-Botero et al., 2020), but in others, it had the opposite effect (Lee et al., 2004). In our case, net CH4 production per kg of DM did not differ between treatments containing legumes (up to 30% inclusion) and grasses alone, but less gas was produced when 100% legumes were incubated. A similar trend was observed for CH4 production per unit DMD, being most noticeable for the treatment of 100% CB. When comparing both legumes, we observed that CB was characterized by containing less NDF and ADL than LD, contributing to improved digestibility and therefore higher gas production. This finding coincides with the conclusion reached by Hess et al. (2003), who stated that the difference in in vitro CH4 production among various kinds of forages could be accounted for by the differences between the ratios of digestible carbohydrates and cellulose. Likewise, Patra and Saxena (2010) proposed that the presence of secondary metabolites can affect methanogenesis. However, this was not observed in the present study, because the inclusion of up to 30% of legumes did not reduce in vitro CH4 production. In addition, a greater reduction would be expected with the LD treatment alone, since it contained a greater amount of total phenols and tannins compared to CB alone. These results can be explained by indirect effects of other secondary compounds present in these species, such as mimosine, alkaloids, saponins, steroids, among others that were not evaluated (Hu et al., 2005; Oseni et al., 2011). With our results, it should not be ignored that the in vitro technique, despite being an artificial system, is a viable option to initially simulate possible dietary combinations of forages (Danielsson et al., 2017) that can then be validated using ruminants. This is why we highlight the importance of including legumes in cattle diets as a strategy to reduce CH4 emissions.
Although it was not the primary aim of this study, the use of herbaceous and shrub legumes was shown to have potential positive environmental benefits besides improving nutritive values of diets for ruminants. Vazquez et al. (2020) showed how combining the three types of forages tested here clearly improved chemical, physical and biological soil health characteristics. In addition, the use of shrubs and trees in silvopastoral systems have shown the capacity to sequester greater amounts of carbon at a system level (Aynekulu et al., 2020).
Conclusions
Diets that combined legumes (CB or LC) with grass (UHC or UBT) had higher protein contents and gross and metabolizable energy densities, as well as decreased concentrations of NDF and lignin. Metabolizable energy and nutritional compounds such as NDF, T, and CP had a high correlation with net gas production, while ruminal digestibility was affected by CP, ADL, GE, T, and other unidentified compounds provided by CB and/or LD.
Optimal ratios of dietary components in both systems were found with mixtures consisting of 60% grass (either UHC or UBT), 30% CB, and 10% LD. The system containing UHC yielded the best combination in terms of an increase in CP and a decrease in AGP. However, this ratio did not result in a decrease in methane production. Therefore, further characterization of the content and activity of other secondary metabolites, perhaps present in both legumes, is required to better explain the behavior response resulting from grass-legume interactions.
Data Availability Statement
The raw data supporting the conclusions of this article will be made available by the authors, without undue reservation.
Ethics Statement
The animal study was reviewed and approved by Colombian law No. 84/1989 and the Ethics Committee of the International Center for Tropical Agriculture.
Author Contributions
SQ-A, IM-B, JR-N, and RB-R: conceptualization. SQ-A and IM-B: methodology. SQ-A, IM-B, and JM: formal analysis. SQ-A: writing—original draft preparation. SQ-A, IM-B, IR, RB-R, NC, JA, and JM: writing—review and editing. RB-R and JA: supervision. JA: project administration. NC, JM, and JA: funding acquisition. All authors contributed to the article and approved the submitted version.
Funding
This work was implemented as part of the CGIAR Research Program (CRP) on Climate Change, Agriculture and Food Security (CCAFS), and the Livestock CRP which are carried out with support from CGIAR Fund Donors and through bilateral funding agreements. For details, please visit https://ccafs.cgiar.org/donors. We also acknowledge the financial assistance of BBSRC grants: UK—CIAT Joint Centre on Forage Grasses for Africa (BBS/OS/NW/000009); RCUK-CIAT Newton Fund—Toward climate-smart forage-based diets for Colombian livestock (BB/R021856/1); Advancing sustainable forage-based livestock production systems in Colombia (CoForLife) (BB/S01893X/1) and GROW Colombia from the UK Research and Innovation (UKRI) Global Challenges Research Fund (GCRF) (BB/P028098/1).
Conflict of Interest
The authors declare that the research was conducted in the absence of any commercial or financial relationships that could be construed as a potential conflict of interest.
References
Abdalla, A., Abdalla Filho, A., Natel, A., Louvandini, H., Piccolo, M., Nechet, K. L., et al. (2019). Nutritive value and enteric methane production of Brachiaria spp. under elevated [CO2]. Int. J. Plant Product. 14, 119–126. doi: 10.1007/s42106-019-00072-6
ANKOM (2016). Acid Detergent Lignin Method (in Beakers). Retrieved from: https://www.ankom.com/sites/default/files/document-files/Method_8_Lignin_in_beakers.pdf (accessed January 15, 2021).
Arango, J., Ruden, A., Martinez-Baron, D., Loboguerrero, A. M., Berndt, A., Chacón, M., et al. (2020). Ambition meets reality: achieving GHG emission reduction targets in the livestock sector of Latin America. Front. Sustain. Food Syst. 4:65. doi: 10.3389/fsufs.2020.00065
Archimède, H., Rira, M., Barde, D. J., Labirin, F., Marie-Magdeleine, C., Calif, B., et al. (2016). Potential of tannin-rich plants, Leucaena leucocephala, Glyricidia sepium and Manihot esculenta, to reduce enteric methane emissions in sheep. J. Anim. Physiol. Anim. Nutr. 100, 1149–1158. doi: 10.1111/jpn.12423
Association of Official Analytical Chemists (1990). Ash of Animal Feed. 942.05 Official Methods of Analysis, 15th Edn. Rockville, MD: A.O.A.C.
Aynekulu, E., Suber, M., van Noordwijk, M., Arango, J.;, Roshetko, J. M., and Rosenstock, T. S. (2020). Carbon storage potential of silvopastoral systems of Colombia. Land 9:309. doi: 10.3390/land9090309
Barahona, R., and Sánchez, M. (2005). Limitaciones físicas y químicas de la digestibilidad de pastos tropicales y estrategias para aumentarla. Ciencia y Tecnología Agropecuaria 6, 69–62. doi: 10.21930/rcta.vol6_num1_art:39
Blümmel, M., Makkar, H. P. S., and Becker, K. (1997). In vitro gas production: a technique revisited. J. Anim. Physiol. Anim. Nutr. 77, 24–34. doi: 10.1111/j.1439-0396.1997.tb00734.x
Calsamiglia, S., Castillejos, L., and Busquet, M. (2005). “Estrategias nutricionales para modificar la fermentación ruminal en vacuno lechero,” in XXI Curso de Especialización, eds P. G. Rebollar, C. de Blass, and G. G. Mateos (Barcelona: Sitio Argentino de Producción Animal), 161–185.
Cammack, K. M., Austin, K. J., Lamberson, W. R., Conant, G. C., and Cunningham, H. C. (2018). RUMINANT NUTRITION SYMPOSIUM: tiny but mighty: the role of the rumen microbes in livestock production. Anim. Sci. J. 96, 752–770 doi: 10.1093/jas/skx053
Carulla, J. E., Kreuzer, M., Machmuller, A., and Hess, H. D. (2005). Supplementation of Acacia mearnsii tannins decreases methanogenesis and urinary nitrogen in forage-fed sheep. Aust. J. Agric. Res. 56, 961–970. doi: 10.1071/AR05022
Cook, B. G., Pengelly, B. C., Schultze-Kraft, R., Taylor, M., Burkart, S., Cardoso Arango, J. A., et al. (2020). Tropical Forages: An Interactive Selection Tool, 2nd and Revised Edn. Cali; Nairobi: International Center for Tropical Agriculture (CIAT); Colombia and International Livestock Research Institute (ILRI). Available online at: www.tropicalforages.info (accessed January 15, 2021).
Cuartas Cardona, C. A., Naranjo Ramírez, J. F., Tarazona Morales, A. M., Correa Londoño, G. A., and Barahona Rosales, R. (2015). Dry matter and nutrient intake and diet composition in Leucaena leucocephala-based intensive silvopastoral systems. Trop. Subtrop. Agroecosyst. 18, 303–311.
Cuartas Cardona, C. A., Naranjo Ramírez, J. F., Tarazona Morales, A. M., Murgueitio Restrepo, E., Chará Orozco, J. D., Ku Vera, J., et al. (2014). Contribution of intensive silvopastoral systems to animal performance and to adaptation and mitigation of climate change. Revista Colombiana de Ciencias Pecuarias 27, 76–94.
Danielsson, R., Ramin, M., Bertilsson, J., Lund, P., and Huhtanen, P. (2017). Evaluation of a gas in vitro system for predicting methane production in vivo. J. Dairy Sci. 100, 8881–8894. doi: 10.3168/jds.2017-12675
Demarchi, J., Manella, M., Primavesi, O., Frighetto, R., Romero, L., Berndt, A., et al. (2016). Effect of seasons on enteric methane emissions from cattle grazing Urochloa brizantha. J. Agric. Sci. 8, 106–115. doi: 10.5539/jas.v8n4p106
Evitayani, E., Warly, L., Fariani, A., Ichinohe, T., and Fujihara, T. (2004). Study on nutritive value of tropical forages in North Sumatra, Indonesia. Asian Austr. J. Anim. Sci. 17, 1518–1152. doi: 10.5713/ajas.2004.1518
Figueiras, J. F., Detmann, E., Paulino, M. F., Valente, T. N. P., de Campos Valadares Filho, S., and Lazzarin, I. (2010). Intake and digestibility in cattle under grazing supplemented with nitrogenous compounds during dry season. R. Bras. Zootec. 39, 1303–1312. doi: 10.1590/S1516-35982010000600020
Gaviria, X., Rivera, J. E., and Barahona, R. (2015). Calidad nutricional y fraccionamiento de carbohidratos y proteína en los componentes forrajeros de un sistema silvopastoril intensivo. Pastos y Forrajes 38, 194–201.
Gaviria-Uribe, X., Bolivar, D., Rosenstock, T., Molina-Botero, I. C., Chirinda, N., Barahona, R., et al. (2020). Nutritional quality, voluntary intake and enteric methane emissions of diets based on novel Cayman grass and its associations with two Leucaena shrub legumes. Vet. Sci. Front. 7:579189. doi: 10.3389/fvets.2020.579189
Gaviria-Uribe, X., Naranjo-Ramírez, J. F., Bolívar-Vergara, D. M., and Barahona-Rosales, R. (2015). Consumo y digestibilidad en novillos cebuínos en un sistema silvopastoril intensivo. Arch. De Zootec. 64, 21–27. doi: 10.21071/az.v64i245.370
Geleti, D., Hailemariam, M., Mengistu, A., and Tolera, A. (2013). Nutritive value of selected browse and herbaceous forage legumes adapted to medium altitude subhumid areas of western Oromia, Ethiopia. Glob. Vet. 11, 809–816. doi: 10.5829/idosi.gv.2013.11.6.8216
Givens, D. I., Moss, A. R., and Adamson, A. H. (1993). Influence of growth stage and season on the energy value of fresh herbage. 1. Changes in metabolizable energy content. Grass For. Sci. 48, 166–174. doi: 10.1111/j.1365-2494.1993.tb01849.x
Grant, R. J., Dann, H. M., and Woolpert, M. E. (2015). Time required for adaptation of behavior, feed intake, and dietary digestibility in cattle. J. Dairy Sci. 98(Suppl. 2):312.
Henke, A., Dickhoefer, U., Westreicher-Kristen, E., Knappstein, K., Molkentin, J., Hasler, M., et al. (2017). Effect of dietary Quebracho tannin extract on feed intake, digestibility, excretion of urinary purine derivatives and milk production in dairy cows. Arch. Anim. Nutr. 71, 37–53. doi: 10.1080/1745039X.2016.1250541
Hess, H. D., Monsalve, L. M., Lascano, C. E., Carulla, J. E., Diaz, T. E., and Kreuzer, M. (2003). Supplementation of a tropical grass diet with forage legumes and Sapindus saponaria fruits: effects on in vitro ruminal nitrogen turnover and methanogenesis. Crop Pasture Sci. 54, 703–713. doi: 10.1071/AR02241
Hu, W. L., Liu, J. X., Ye, J. A., Wu, Y. M., and Guo, Y. Q. (2005). Effect of tea saponin on rumen fermentation in vitro. Anim. Feed Sci. Technol. 120, 333–339. doi: 10.1016/j.anifeedsci.2005.02.029
Hyams, D. G. (2016). CurveExpert Professional (Release 2.4.0) [Computer Software]. Chattanooga, TN: Hyams Development. Available online at: http://www.curveexpert.net (accessed January 15, 2021).
Hyland, J. J., Styles, D., Jones, D. J., and Williams, A. P. (2016). Improving livestock production efficiencies presents a major opportunity to reduce sectoral greenhouse gas emissions. Agric. Syst. 147, 123–131 doi: 10.1016/j.agsy.2016.06.006
International Standardization Organization (1998). ISO 9831:1998: Animal Feeding Stuffs Animal Products, and Faeces or Urine - Determination of Gross Calorific Value - Bomb Calorimeter Method. Switzerland: ISO.
Jayanegara, A., Wina, E., Soliva, C. R., Marquardt, S., Kreuzer, M., and Leiber, F. (2011). Dependence of forage quality and methanogenic potential of tropical plants on their phenolic fractions as determined by principal component analysis. Anim. Feed Sci. Technol. 163, 231–243. doi: 10.1016/j.anifeedsci.2010.11.009
Karsten, H. D., and Carlassare, M. (2002). Describing the botanical composition of a mixed species northeastern US pasture rotationally grazed by cattle. Crop Sci. 42, 882–889. doi: 10.2135/cropsci2002.0882
Krizsan, S. J., Ahvenjärvi, S., and Huhtanen, P. (2010). A meta-analysis of passage rate estimated by rumen evacuation with cattle and evaluation of passage rate prediction models. J. Dairy Sci. 93, 5890–5901. doi: 10.3168/jds.2010-3457
Krizsan, S. J., Pang, D., Fatehi, F., Rinne, M., and Huhtanen, P. (2020). Metabolisable energy of grass and red clover silages fed to sheep at maintenance level. Animal 14, 753–762 doi: 10.1017/S1751731119002556
Krom, M. D. (1980). Spectrophotometric determination of ammonia: a study of a modified Berthelot reaction using salicylate and dichloroisocyanurate. Analyst 105, 305–316. doi: 10.1039/an9800500305
Ku-Vera, J. C., Castelán-Ortega, O. A., Galindo-Maldonado, F. A., Arango, J., Chirinda, N., Jiménez-Ocampo, R., et al. (2020a). Review: strategies for enteric methane mitigation in cattle fed tropical forages. Animal 4, s453–s463. doi: 10.1017/S1751731120001780
Ku-Vera, J. C., Jiménez-Ocampo, R., Valencia-Salazar, S., Flores-Santiago, E. J., Montoya-Flores, M. D., Molia-Botero, I. C., et al. (2020b). Role of secondary plant metabolites on enteric methane mitigation in ruminants. Front. Vet. Sci. 7:584. doi: 10.3389/fvets.2020.00584
Lascano, C. E., and Cárdenas, E. (2010). Alternatives for methane emission mitigation in livestock systems. R. Bras. Zootec. 39, 175–182. doi: 10.1590/S1516-35982010001300020
Lavrenčič, A., Stefanon, B., and Susmel, P. (1997). An evaluation of the Gompertz model in degradability studies of forage chemical components. Anim. Sci. J. 64, 423–431. doi: 10.1017/S1357729800016027
Lee, J. M., Woodward, S. L., Waghorn, G. C., and Clark, D. A. (2004). Methane emissions by dairy cows fed increasing proportions of white clover (Trifolium repens) in pasture. Proc. N. Zeal. Grass. Assoc. 66, 151–155. doi: 10.33584/jnzg.2004.66.2552
Lee, M. A. (2018). A global comparison of the nutritive values of forage plants grown in contrasting environments. J. Plant Res. 131, 641–654. doi: 10.1007/s10265-018-1024-y
Lindgren, E. (1983). Recalibration of the VOS Method for Determination of Energy Content in Forages (Nykalibrering av VOS-metoden för bestämning av energivärde hos vallfoder). Working Paper. Uppsala: Swedish University of Agricultural Sciences (In Swedish).
Low, S. G. (2011). Signal grass (Urochloa decumbens) toxicity in grazing ruminants. Agriculture 5, 971–990. doi: 10.3390/agriculture5040971
Makkar, H. P. (2003). “Measurement of total phenolics and tannins using Folin-Ciocalteu method,” in Quantification of Tannins in Tree and Shrub Foliage: A Laboratory Manual, ed H. Makkar (Dordrecht: Kluwer Academic Publisher), 49–50. doi: 10.1007/978-94-017-0273-7_3
McAllister, T. A., Bae, H. D., Jones, G. A., and Cheng, K. J. (1994). Microbial attachment and feed digestion in the rumen. Anim. Sci. J. 72, 3004–3018. doi: 10.2527/1994.72113004x
Molina, I. C., Donney‘s, G., Montoya, S., Rivera, J. E., Villegas, G., Chará, J., et al. (2015). La inclusión de Leucaena leucocephala reduce la producción de metano de terneras Lucerna alimentadas a base de Cynodon plectostachyus y Megathyrsus maximus. Livestock Research for Rural Development, 27. Available online at: http://www.lrrd.org/lrrd27/5/moli27096.html
Molina-Botero, I. C., Arroyave-Jaramillo, J., Valencia-Salazar, S., Barahona-Rosales, R., Aguilar-Pérez, C. F., Ayala Burgos, A., et al. (2019b). Effects of tannins and saponins contained in foliage of Gliricidia sepium and pods of Enterolobium cyclocarpum on fermentation, methane emissions and rumen microbial population in crossbred heifers. Anim. Feed Sci. Technol. 251:1–11. doi: 10.1016/j.anifeedsci.2019.01.011
Molina-Botero, I. C., Mazabel, J., Arceo-Castillo, J., Urrea-Benitez, J. L., Olivera-Castillo, L., Barahona-Rosales, R., et al. (2020). Effect of the addition of Enterolobium cyclocarpum pods and Gliricidia sepium forage on dry matter degradation, volatile fatty acid con-centration, and in vitro methane production. Trop. Anim. Health Prod. 52, 2787–2798. doi: 10.1007/s11250-020-02324-4
Molina-Botero, I. C., Montoya, D., Zavala, L., Barahona, R., Arango, J., and Ku, J. (2019a). Effects of long-term diet supplementation with Gliricidia sepium foliage mixed with Enterolobium cyclocarpum pods on enteric methane, apparent digestibility, and rumen microbial population in crossbred heifers. J. Anim. Sci. 97, 1619–1633. doi: 10.1093/jas/skz067
Montoya-Flores, M. D., Molina-Botero, I. C., Arango, J., Romano-Muñoz, J. L., Solorio-Sánchez, F. J., Aguilar-Pérez, C. F., et al. (2020). Effect of dried leaves of Leucaena leucocephala on rumen fermentation, rumen microbial population, and enteric methane production in crossbred heifers. Animals 10:300. doi: 10.3390/ani10020300
Moss, A. R., Givens, D. I., and Phipps, R. H. (1992). Digestibility and energy value of combinations of forage mixtures. Anim. Feed Sci. Technol. 39, 151–172. doi: 10.1016/0377-8401(92)90038-8
Naranjo, J. F., Cuartas, C. A., Murgueitio, E., Chará, J., and Barahona, R. (2012). Balance de gases de efecto invernadero en sistemas silvopastoriles intensivos con Leucaena leucocephala en Colombia. LRRD 24:150.
Nguku, S. A. (2015). An evaluation of brachiaria grass cultivars productivity in semi arid Kenya (Doctoral dissertation). Available online at: http://repository.seku.ac.ke/handle/123456789/1380 (accessed January 15, 2021).
Oseni, O. A., Ibeto, A. U., and Aruna, M. O. (2011). Effects of dehusking on the composition of phytochemicals nutrients, antinutrients, minerals and in-vitro multi enzyme digestibility of the seed of Brazilian Jack beans (Canavalia braziliensis). Int. Res. J. Biotechnol. 2, 192–197.
Patra, A. K., Park, T., Kim, M., and Yu, Z. (2017). Rumen methanogens and mitigation of methane emissions by anti-methanogenic compounds and substances. J. Anim. Sci. Biotechnol. 8:13. doi: 10.1186/s40104-017-0145-9
Patra, A. K., and Saxena, J. (2010). A new perspective on the use of plant secondary metabolites to inhibit methanogenesis in the rumen. Phytochemistry 71, 1198–1222. doi: 10.1016/j.phytochem.2010.05.010
Patra, A. K., and Saxena, J. (2011). Exploitation of dietary tannins to improve rumen metabolism and ruminant nutrition. J. Sci. Food Agric. 91, 24–37. doi: 10.1002/jsfa.4152
Peters, M., Franco, L., Schmidt, A., and Hincapié, B. (2002). Especies Forrajeras Multipropósito: Opciones para productores de Centroamérica. Cali: Centro Internacional de Agricultura Tropical (CIAT); Bundesministerium für Wirtschaftliche Zusammenarbeit und Entwicklung (BMZ); Deutsche Gesellschaft für Technische Zusammenarbeit (GTZ), 113.
Peters, M., Rao, I. M., Fisher, M. J., Subbarao, G., Martens, S., Herrero, G., et al. (2012). “Tropical forage-based systems to mitigate greenhouse gas emissions,” in Eco-Efficiency: From Vision to Reality, ed C. H. Hershey, (Cali: Centro Internacional de Agricultura Tropical (CIAT)), 20.
Rao, I., Peters, M., Castro, A., Schultze-Kraft, R., White, D., Fisher, M., et al. (2015). LivestockPlus - the sustainable intensification of forage-based agricultural systems to improve livelihoods and ecosystem services in the tropics. Trop. Grass. For. Trop. 3:59. doi: 10.17138/TGFT(3)59-82
Rojas, S., Olivares, J., Jimenez, R., and Hernández, E. (2005). Manejo de praderas asociadas de gramíneas y leguminosas para pastoreo en el trópico. Revista Electronica de Veterinaria 6, 103–122.
SAS Institute (2012). User's Guide: Statistics (Version 9.4) [Computer Software]. Cary, NC: SAS Institute Inc.
Searle, P. L. (1984). The Berthelot or indophenol reaction and its use in the analytical chemistry of nitrogen. A review. Analyst 109, 549–568. doi: 10.1039/an9840900549
Seresinhe, T., Madushika, S. A. C., Seresinhe, Y., Lal, P. K., and Orskov, E. R. (2012). Effects of tropical high tannin non legume and low tannin legume browse mixtures on fermentation parameters and methanogenesis using gas production technique. Asian Austral. J. Anim. 25, 1404–1410. doi: 10.5713/ajas.2012.12219
Shelton, H. M., and Dalzell, S. A. (2007). Production, economic and environmental benefits of leucaena pastures. Trop. Grass. For. Trop. 41, 174–190.
StatPoint Technologies Inc. (2010). STATGRAPHICS® Centurion XVI (Version 16.1.18, 32 Bits) [Computer Software]. The Plains, VA: Available online at: http://www.statgraphics.net/ (accessed January 15, 2021).
Tang, S. X., Tayo, G. O., Tan, Z. L., Sun, Z. H., Wang, M., Ren, G. P., et al. (2008). Use of in vitro gas production technique to investigate interactions between rice straw, wheat straw, maize stover and alfalfa or clover. Asian Austral. J. Anim. 21, 1278–1285. doi: 10.5713/ajas.2008.70447
Tavendale, M. H., Meagher, L. P., Pacheco, D., Walker, N., Attwood, G. T., and Sivakumaran, S. (2005). Methane production from in vitro rumen incubations with Lotus pedunculatus and Medicago sativa, and effects of extractable condensed tannin fractions on methanogenesis. Anim. Feed Sci. Technol. 123, 403–419. doi: 10.1016/j.anifeedsci.2005.04.037
Theodorou, M. K., Williams, B. A., Dhanoa, M. S., McAllan, A. B., and France, J. (1994). A simple gas production method using a pressure transducer to determine the fermentation kinetics of ruminant feeds. Anim. Feed Sci. Technol. 48, 185–197. doi: 10.1016/0377-8401(94)90171-6
Tiemann, T. T., Lascano, C. E., Kreuzer, M., and Hess, H. D. (2008a). The ruminal degradability of fibre explains part of the low nutritional value and reduced methanogenesis in highly tanniniferous tropical legumes. J. Sci. Food Agric. 88, 1794–1803. doi: 10.1002/jsfa.3282
Tiemann, T. T., Lascano, C. E., Wettstein, H.-R., Mayer, A. C., Kreuzer, M., and Hess, H. D. (2008b). Effect of the tropical tannin-rich shrub legumes Calliandra calothyrsus and Flemingia macrophylla on methane emission and nitrogen and energy balance in growing lambs. Animal 2, 790–799. doi: 10.1017/S1751731108001791
Tolera, A., Seyoum, M., and Sundstol, F. (1998). “Nutritive evaluation of Leucaena leucocephala, L. diversifolia and L. pallida in Awassa, Southern Ethiopia,” in Proceedings of a Workshop held in Hanoi, Vietnam 9-14 February 1998: Leucaena - Adaptation, Quality and Farming Systems eds H. M. Shelton, R. C. Gutteridge, B. F. Mullen, and R. A. Bray (ACIAR Proceedings), 261–264.
Tope, A. F., Oluwafemi, O. J., Oladunni, O. F., and Aderemi, A. D. (2013). Feeding values of seven browse tree foliages mixed in varying proportions with Panicum maximum for feeding ruminants. Am. J. Sci. 9, 64–71.
Valencia-Salazar, S. S., Jiménez-Ferrer, G., Arango, J., Molina-Botero, I., Chirinda, N., Piñeiro-Vázquez, A., et al. (2021). Enteric methane mitigation and fermentation kinetics of forage species from Southern Mexico: in vitro screening. Agrofor. Syst. 95, 293–305. doi: 10.1007/s10457-020-00585-4
Van Soest, P., Robertson, J., and Lewis, B. (1991). Methods for dietary fiber, neutral detergent fiber and non-starch polysaccharides in relation to animal nutrition. J. Dairy Sci. 74, 3583–3597. doi: 10.3168/jds.S0022-0302(91)78551-2
Vazquez, E., Teutscherova, N., Lojka, B., Arango, J., and Pulleman, M. (2020). Pasture diversification affects soil macrofauna and soil biophysical properties in tropical (silvo)pastoral systems. Agric. Ecosyst. Environ. 302:107083. doi: 10.1016/j.agee.2020.107083
Vendramini, J. M., Sollenberger, L. E., Soares, A. B., da Silva, W. L., Sanchez, J. M., Valente, A. L., et al. (2014). Harvest frequency affects herbage accumulation and nutritive value of brachiaria grass hybrids in Florida. Trop. Grass. For. Trop. 2, 197–206. doi: 10.17138/TGFT(2)197-206
Wassie, W. A., Tsegay, B. A., Wolde, A. T., and Limeneh, B. A. (2018). Evaluation of morphological characteristics, yield and nutritive value of brachiaria grass ecotypes in northwestern Ethiopia. Agric. Food Secur. 7, 1–10. doi: 10.1186/s40066-018-0239-4
Keywords: Canavalia brasiliensis, in-vitro fermentation, Leucaena sp., nutritional quality, Urochloa brizantha cv. Toledo, Urochloa hybrid cv. Cayman
Citation: Quintero-Anzueta S, Molina-Botero IC, Ramirez-Navas JS, Rao I, Chirinda N, Barahona-Rosales R, Moorby J and Arango J (2021) Nutritional Evaluation of Tropical Forage Grass Alone and Grass-Legume Diets to Reduce in vitro Methane Production. Front. Sustain. Food Syst. 5:663003. doi: 10.3389/fsufs.2021.663003
Received: 02 February 2021; Accepted: 10 May 2021;
Published: 14 June 2021.
Edited by:
Bruno José Rodrigues Alves, Brazilian Agricultural Research Corporation (EMBRAPA), BrazilReviewed by:
Luis Alonso Villalobos Villalobos, University of Costa Rica, Costa RicaSiriwan Martens, Saxon State Office for Environment, Agriculture and Geology, Germany
Daniel Casagrande, Universidade Federal de Lavras, Brazil
Copyright © 2021 Quintero-Anzueta, Molina-Botero, Ramirez-Navas, Rao, Chirinda, Barahona-Rosales, Moorby and Arango. This is an open-access article distributed under the terms of the Creative Commons Attribution License (CC BY). The use, distribution or reproduction in other forums is permitted, provided the original author(s) and the copyright owner(s) are credited and that the original publication in this journal is cited, in accordance with accepted academic practice. No use, distribution or reproduction is permitted which does not comply with these terms.
*Correspondence: Jacobo Arango, ai5hcmFuZ29AY2dpYXIub3Jn
†These authors have contributed equally to this work