- 1Centro de Agricultura Ecológica y de Montaña, Área de Protección Vegetal, Centro de Investigaciones Científicas y Tecnológicas de Extremadura (CICYTEX), Plasencia, Spain
- 2Instituto de Investigaciones Agrarias Finca La Orden-Valdesequera, Área de Protección Vegetal, Centro de Investigaciones Científicas y Tecnológicas de Extremadura (CICYTEX), Badajoz, Spain
Phytophthora nicotianae is the pathogen that causes root and crown rot disease in open field paprika pepper crops of Extremadura (central-western Spain). A field experiment was established during spring, a period compatible with the pepper crop cycle in this region, to evaluate the effects of biofumigation with pellets of Brassica carinata defatted seed meal, at the rate recommended by the manufacturer (3 tons ha−1), on the survival and infectivity of P. nicotianae chlamydospores inoculum. Furthermore, three biofumigant rates (3, 6, and 20 tons ha−1) were assayed in laboratory experiments with natural soil inoculated with chlamydospores. In the field trial, the incorporation of pellets at 3 tons ha−1 did not produce remarkable changes in soil enzyme activity or soil properties. In both the field and laboratory tests, survival and infectivity in the biofumigated treatment at 3 tons ha−1 did not differ from those in the untreated control. On the contrary, the same rate added to autoclaved soil completely suppressed the inoculum, suggesting that the soil microbiota degrades B. carinata pellets before being effective against P. nicotianae. Increasing the recommended rate to 6 tons ha−1 decreased inoculum survival in the laboratory test and 20 tons ha−1 completely inhibited the inoculum, although the economic value of such a high rate application is a factor to be assessed. In phytotoxicity tests on radish and white mustard seeds with several concentrations (100, 50, 25, 10, 5, and 0%) of B. carinata pellets solution, no phytotoxic effect was observed with the two lowest concentrations, and no symptoms of phytotoxicity were found in the bioassays of pepper plants.
Introduction
Phytophthora nicotianae Breda de Haan (=P. parasitica Dastur) is a very damaging soil-borne plant pathogen (Erwin and Ribeiro, 1996) that causes significant losses in a large number of host plants (Panabières et al., 2016). In Extremadura region (central-western Spain), where paprika peppers are grown in open field, P. nicotianae survives mainly as chlamydospores and is the principal cause of root and crown rot in this crop (Rodríguez-Molina et al., 2010; Serrano-Pérez et al., 2017a). The banning of methyl bromide as a soil fumigant and restrictions on the use of other chemicals have made it necessary to develop sustainable alternatives for the management of this pathosystem.
In field trials in Extremadura, solarization in summer was effective in the inactivation of chlamydospores of P. nicotianae (Rodríguez-Molina et al., 2016), and several authors have described decreases in the density of this pathogen after soil solarization (Coelho et al., 1999; Lacasa et al., 2015). However, pepper for paprika is grown in open field in Extremadura during summer. Thus, solarization or biofumigation during the summertime combined with solarization (biosolarization) is not compatible with this crop cycle. Anaerobic soil disinfestation (ASD), i.e., incorporating high amounts of organic carbon under saturated soil conditions and sealing it with a plastic sheet, which leads to oxygen exhaustion by facultative anaerobes, has been shown to be an effective approach to control this pathogen (Serrano-Pérez et al., 2017b). Biofumigation is a term that refers to the suppression of soil pests and pathogens by volatile toxic compounds, mostly isothiocyanates (ITCs), which are released when the glucosinolates (GSLs) that Brassicaceae species contain in their tissues are hydrolyzed by the enzyme myrosinase (Kirkegaard et al., 1993; Angus et al., 1994). The products resulting from the hydrolysis, especially the ITCs, have shown extensive biocidal action, although they could have phytotoxic effects (Brown and Morra, 1997). The meaning of the term biofumigation is actually broader and it is associated with the release of biocidal compounds in the decomposition processes of plant tissues or any kind of organic matter (Bello et al., 2000; Gimsing and Kirkegaard, 2009). There is a considerable amount of literature on the success of this strategy, see descriptions in recent works (Brennan et al., 2020; Hanschen and Winkelmann, 2020; Santos et al., 2021). In a meta-analysis including information from 934 biofumigation tests with brassica residues, Morris et al. (2020) reported a decrease in disease incidence and about 30% increase in crop yields using this technique.
GSLs can be incorporated into the soil as fresh plant material (green manure), dried plant material, or seed meals (Matthiessen and Kirkegaard, 2006; Gimsing and Kirkegaard, 2009). The residual meal after oil extraction, the defatted seed meal (DSM), constitutes the major by-product (around 60% of the seed) of the extraction process (Santana-Méridas et al., 2012). Using DSM as a biofumigant is a sustainable way to upcycle this organic waste. Seed brassica meals have several advantages over green manures, such as their availability all year round and stable storage, with no change in their GLS profile, due to their low moisture content. In this study, commercial pellets (Biofence®, Triumph Italia SPA) produced from seed meal of Brassica carinata A. Braun selection ISCI 7 (Lazzeri et al., 2008; Furlan et al., 2010) were tested to control P. nicotianae. Seed meal is previously deffated to extract the oil, which increases its GSLs content. This formulation enables the application of doses that would be impossible to achieve with green amendments (Lazzeri et al., 2008). B. carinata is a well-studied species that provides a very suitable formulation for biofumigation (Porras and Dubey, 2011). Sinigrin is the principal glucosinolate in the B. carinata pellets and releases toxic allyl isothiocyanate (AITC) upon hydration (Galletti et al., 2008; Lazzeri et al., 2008).
In a previous paper, Serrano-Pérez et al. (2017a) showed that B. carinata pellets at the recommended commercial rate (3 tons ha−1) and the double of this (6 tons ha−1) decreased populations of P.nicotianae under the threshold of bioassay detection (<2 CFU g−1 of soil) and controlled the disease on pepper using autoclaved soil for the experiments. In some cases this product is not as effective when applied to natural soils (Guerrero et al., 2010; Núñez-Zofio et al., 2010; Núñez-Zofío et al., 2011). In a previous field experiment, the application of 3 tons ha−1 of B. carinata pellets in spring had no significant effect on the survival of P. nicotianae chlamydospores, regardless of the combination with Brassica cover crops (Rodríguez-Molina et al., 2016). In that study, it was suggested that the infectivity of the introduced inoculum was unexpectedly low, possibly by factors related to microbiological or chemical properties of the soil, decreases in density and weakening of inoculum, or even losses of pathogenicity of the P. nicotianae isolate used due to continuous culturing. Besides, the amount of water applied in that study after the pellets were incorporated may have been insufficient for optimizing the release of ITCs, according to Hanschen and Winkelmann (2020).
Several factors can influence the effectiveness of biofumigation with Brassica amendments in natural soils (Gimsing and Kirkegaard, 2009). In field conditions, GSLs can leach easily from the soil, or may be degraded and mineralized before forming ITCs (Gimsing et al., 2006, 2007; Laegdsmand et al., 2007). Also, ITCs are generally short-lived in natural soil (Gimsing and Kirkegaard, 2009). Sorption of ITCs by the organic matter in the soil or their volatilization, are other mechanisms that reduce the effect of Brassica amendments in soils (Kirkegaard et al., 1998; Gimsing and Kirkegaard, 2009).
Recent work proposes to apply this B. carinata pellets at higher rates than that recommended by the manufacturer (3 tons ha−1) (Garibaldi et al., 2010; Gilardi et al., 2016, 2020). Gilardi et al. (2016) reported a reduction in the severity of F. oxysporum f. sp. lactucae, with a significant increase in Pseudomonas soil population, after the addition of 2.5 g L−1 of B. carinata pellets to non-autoclaved blonde sphagnum peat mixed with soil.
The objectives of this work were (i) to evaluate the effect of B. carinata pellets at the recommended rate by the manufacturer on survival and infectivity of P. nicotianae in a field experiment and to know its effect on chemical and biological soil properties, (ii) to test increasing doses of pellets incorporated into an inoculated natural soil under controlled laboratory conditions, and (iii) to discard the possible phytotoxic effect of the pellets.
Materials and Methods
Inoculum Production
The isolate P-23 of P. nicotianae, isolated from a symptomatic pepper plant and previously evaluated for pathogenicity (Rodríguez-Molina et al., 2010), was used for the experiments.
Chlamydospores production followed the methodology described by Rodríguez-Molina et al. (2016), based on the culture of P. cinnamomi isolate in 150 ml of V8 juice broth in 20-cm Petri plates and incubation at 25°C in the dark for 10 days. Subsequently, the V8 juice broth was removed, replaced with distilled water and plates were incubated again in the dark at 18°C for 7 days. Then, the mycelium clumps were collected, washed with sterile distilled water, homogenized in 50 ml of sterile distilled water (homogenizer MICCRA D-1, ART-moderne Labortechnik, Germany) and sonicated (HD 2070, Sonoplus, Bandelin, Germany) on a 60-s cycle (active interval: 0.9 s, passive interval: 0.1 s). After centrifugation (2 min; 1,760 rpm), the resulting pellet was resuspended in distilled water. The concentration of chlamydospores was assessed with a Neubauer counting chamber and their viability was estimated by staining with Rose Bengal solution (Tsao, 1971).
For field and laboratory experiments, inoculum bags were prepared using field disinfected soil (autoclaved twice 1 h at 120°C) which subsequently was infested with chlamydospores of P. nicotianae, according to the procedure described by Rodríguez-Molina et al. (2016). The soil was placed inside the Agryl cloth bags which were closed with string. Bags with 5 g of soil and 500 chlamydospores g−1 were prepared for the laboratory trials, and bags with 100 g of soil and 50 chlamydospores g−1 for the field trial.
Field Experiment
The trial was performed in a field with sandy loam soil (pH = 6.5; organic matter = 0.55%) at the Agricultural Research Institute Finca La Orden-Valdesequera (Extremadura, central-western Spain). The experimental design was a randomized complete block design with 4 replicates. The experimental plot size was 1.5 ×1.5 m, and each plot was artificially infested with 4 inoculum bags, with 100 g of soil and 50 chlamydospores per gram of dry soil each, which were buried at 20-cm depth, as used by Serrano-Pérez et al. (2017b).
The trial was conducted using commercial pellets of defatted B. carinata seed meal (BioFence®, organic N 6%, P 2.2%, K 2%, organic C 52%; Triumph Italia SPA). The concentration of sinigrin, the predominant glucosinolate, was 84.31 micromol g−1 dry weight (Rodríguez-Molina et al., 2021) and the pellets also contained active myrosinase (Galletti et al., 2008). The pellets were added to the soil at 3 tons ha−1, according to the manufacturer's recommendations, and incorporated 20-cm depth using a motorized tiller. The plots were covered with transparent polyethylene film (0.05 mm thick). Two control treatments were included: control non-amended (CP) and control non-amended and without plastic cover (C). The soil was irrigated with 50 mm of water at the beginning of the experiment and again after 14 days with the same dose to maintain it near saturation [volumetric water content (VWC) at 0.21 m3 m−3] in the first 24-cm depth. The treatment was carried out for 4 weeks. Plastic covers were removed after that time and inoculum bags were dug up for determining inoculum survival and infectivity. A composite soil sample was collected at 0 to 25-cm depth from each plot to analyze soil physicochemical properties and microbial activity.
Soil Temperature, Moisture, and pH During the Field Experiment
As was performed in Serrano-Pérez et al. (2017b), soil temperature was continuously monitored at 20-cm depth, using soil probes and an automatic data logging system (HOBO Weather Logger, Pocasset, MA, USA) as well as moisture (volumetric water content, VWC) using 10HS sensors (ECH2O, Decagon Devices Inc., Pullman, WA, USA), during the 4 weeks. Soil samples were collected before the beginning of the treatments and every 7 days during the trial to determine the evolution of soil pH. A pH electrode (HI12963, Hanna Instruments Inc., Woonsocket, RI, USA), in a 1:1 v/v slurry of soil and deionized water, was used for pH determination.
Weed Biomass and Native Fusarium spp. Population Density
To evaluate the possible herbicidal effect of the biofumigation treatment, all vegetation grown during the treatments within the quadrat of each experimental plot was cut, weighed and dried in the oven (102°C) and the dry weight m−2 was calculated. Total Fusarium density (CFU g−1) in each plot was estimated on two occasions: before and after application of the treatments. Soil samples were air-dried, crushed and sieved (0.2 mm mesh size), and Fusarium density was evaluated by the soil-plate method (Warcup, 1950) using Komada's medium modified (Tello et al., 1991). Four replicates were prepared per soil sample.
Soil Physicochemical Properties
Soil samples were randomly collected from each field plot at two times: (i) before treatments application (0 weeks) and (ii) at the end of the experiment (4 weeks). Before analysis, soils were air-dried and ground to pass through a 2-mm sieve. Organic matter (OM) was determined by dichromate oxidation (Walkley and Black, 1934). The electrical conductivity (EC) was measured in water at a soil:extractant ratio of 1:5. Ammonium () content was determined after extraction with 2 M KCl (Mulvaney, 1996). Available phosphorus (P) was measured using the molybdate reactive method (Murphy and Riley, 1962) after bicarbonate extraction (Olsen et al., 1954).
Soil Enzyme Activity
Enzyme activities were determined on the same soil samples collected for physicochemical characterization. The methodology used for the determination of each of the enzymes analyzed can be found in Serrano-Pérez et al. (2017b). Briefly, the β-glucosidase activity was determined as the amount of p–nitrophenol (PNP) formed from p–nitrophenyl-β-D-Glucoside (PGN) (Eivazi and Tabatabai, 1988). Acid phosphatase activity was determined at pH 6.3, using 16mM p-nitrophenyl phosphate (EC 206.353.9) as substrate (Tabatabai and Bremner, 1969). For both enzyme activities, the concentration of PNP was determined photometrically at 400 nm. Dehydrogenase activity was determined by measuring the amount of triphenylformazan (TPF) released after incubating the soil with 2,3,5-triphenyl-tetrazolium chloride. TPF was extracted with methanol (Trevors et al., 1982) and determined by reading at 490 nm. Urease activity was assayed by the method modified by Nannipieri et al. (1980). The was measured colorimetrically at 667 nm (Mulvaney, 1996).
Laboratory Experiments
The soil used in the laboratory experiment was taken from the field experiment plot at the Agricultural Research Institute Finca La Orden-Valdesequera. Before use, the soil was sieved (2 mm sieve).
The experimental set up to evaluate the effectiveness of different rates of pellets is practically the same as the one proposed by Serrano-Pérez et al. (2017b), in which closed controlled-temperature system was established to emulate the physical, chemical and microbial changes that take place in the field during the treatment. In this system, chlamydospores were in contact with all compounds released into the soil solution during the biofumigation process or only exposed to the volatile compounds generated during the treatments. The controlled system consisted of 1-liter airtight glass containers (14-cm height and 10-cm diam) filled with 600 g of soil mixed with the pellets and inoculated with a suspension of chlamydospores. The inoculum concentration was 50 chlamydospores g−1 dry soil. In addition, small bags of soil (5 g) inoculated with 100 chlamydospores g-1 were prepared and hung in the headspace of the container, avoiding contact with the soil placed at the bottom of the container. Ninety ml of tap water was added to saturate the bottom soil and the containers were hermetically sealed for 4 weeks. They were placed in a programmable incubator with a complete randomized design with 4 replicates per treatment. The temperature regime in the incubator was: 17.5°C for 5 h/day; 22.5°C for 5 h/day; 27.5°C for 4 h/day; 32.5°C for 2 h/day; 27.5°C for 3 h/day; 22.5°C for 5 h/day. These temperatures were selected as they had been recorded during a spring solarization field trial in Extremadura (Rodríguez-Molina et al., 2016).
Three different pellets rates were assayed: 3 tons ha−1 (BF3 = biofumigant commercial rate), 6 tons ha−1 (BF6), and 20 tons ha−1 (BF20). For the conversion between weight and surface area units, the bulk density of the soil (1.163 g cm−3) and a product incorporation depth of 20-cm were considered. Control non-amended and inoculated (CPhy+) and non-amended and non-inoculated (CPhy–) were included in the experiment. Besides, to verify the importance of soil microbiota in the process, a treatment with autoclaved soil (1 h at 120°C twice in 2 consecutive days) mixed with the pellets at the commercial field rate (3 tons ha−1) was included (BF3-AS).
When finished the incubation period, the containers were opened, and the soil from the inoculum bags was analyzed to estimate the number of chlamydospores of P. nicotianae surviving after the volatile exposure as described by Serrano-Pérez et al. (2017b) as follows: “the 5 g of soil from the bags were added to 45 ml of 0.25% water-agar (1:10, v:v) and stirred for 2 min; five 1-ml aliquots from this slurry were spread evenly over each of five Petri plates containing 12 ml of NARPH medium (Romero et al., 2007) giving the detection threshold of 2 colony-forming units (CFU) g−1 of soil. After 48 h of incubation in the dark at 25°C, the soil overlay was removed by gently washing the agar surface with tap water. Macroscopically visible colonies of P. nicotianae were counted and reported as CFU g−1 dry soil.”
In the treated soil at the bottom of the containers, inoculum survival and infectivity were analyzed.
Survival and Infectivity of Inoculum
For the assessment of inoculum survival, 2 g soil samples (10 samples per container in laboratory experiments and 3 samples per inoculum bag in the field experiment) were analyzed for the presence of P. nicotianae. The 2 g soil samples were placed in 9-cm Petri plates and flooded with distilled water. Immature carnation petals floating on the water were used as baits for the detection of P. nicotianae (Tello et al., 1991). Results of survival are expressed as the percentage of soil samples in which P. nicotianae was detected.
The soil remaining in the containers (580 g per container) and in the bags (94 g per bag) after sampling for survival assessment was used for chlamydospores infectivity bioassays on pepper plants. The soil was put into 250-cm3 plastic pots (5 pots per container, with 116 g soil each, and 1 pot per inoculum bag). The soil in each pot was mixed with a previously disinfected (autoclaved for 1 h at 120°C) substrate of peat and vermiculite (1:3, v:v). The ratio soil:substrate (v:v) was 1:2 in pots from container and 1:2.5 in pots from bags. One pepper seedling (Capsicum annuum L. cv. Jaranda) at the 2 to 4-true-leaf stage was transplanted into each pot and the pots were placed in a growth chamber with a 16 h light at 28°C/8 h dark at 24°C cycle. Disease symptoms were assessed weekly for 2 months, when the bioassay was concluded. To confirm plant death by P. nicotianae, as the plants died, fragments of the crown and tap water-washed roots were plated on potato dextrose agar and NARPH medium. At the end of the bioassay, roots of all plants were inspected for the presence of disease symptoms and analyzed. Infectivity results are expressed as the percentage of diseased plants. As the blocks were kept separate, there were 4 replicates per treatment.
Phytotoxicity Test of B. carinata Pellets
Radish (Raphanus sativus L.) and white mustard (Sinapis alba L.) seeds were used in these experiments as sensitive species to phytotoxic metabolites. The germination tests were conducted according to methods described previously (Zucconi et al., 1981; Carballo et al., 2009) with slight modifications. A pellet solution was prepared using sterile distilled water at 1:5 ratio (w:v), stirred vigorously for 30 min, and then centrifuged 1 min at 2,500 rpm. Dilutions from the solution were prepared using sterile distilled water to give the following concentrations: 100, 50, 25, 10, 5, and 0%. A sterile filter paper was placed inside each 55-mm diameter Petri plate, and 10 disinfected seeds were placed on the filter paper. One milliliter of each dilution was used to moisten the paper, and the plates were closed. Ten replicates per dilution and species were prepared. Seeds were incubated at 25°C in darkness, and germination and root length were recorded after 3 days. If the primary root was higher than 1 mm, seed germination was considered positive. The germination index (GI) was calculated for each dilution and species with the mean from 10 replicates according to the following formula: GI = G/G(control) × L/L(control), where G and G(control) are the mean percentage of germination with B. carinata pellets and with the control, respectively, and L and L(control) are the mean root lengths with B. carinata pellets and with the control, respectively.
Data Analysis
Data of survival and infectivity of inoculum were analyzed by a Generalized Linear Model (GLM) with a binomial error distribution and logit link (binary dependent variable: detection/non-detection of P. nicotianae for Survival, and disease/non-disease for Infectivity). The treatment was included as fixed independent factor with three levels in the field experiment and five levels in the laboratory experiment and post-hoc comparisons of means were performed by Tukey's tests. The program R Core Team (2020) was used for these analyses.
Data of P. nicotianae chlamydospores survival in laboratory experiments, expressed as CFU g−1 dry soil, were subjected to one-way ANOVA on log-transformed data [log(x+1)] followed by Tukey's multiple range test. Field pH was analyzed with two-way ANOVA for repeated measures (rmANOVA), including statistical significance for the effects of treatment (between-subjects factor) and sampling time (within-subjects factor), as well as the interactions between them. The degrees of freedom were corrected using Greenhouse-Geisser estimates of sphericity. Data on soil enzyme activity and soil properties were also subjected to two-way rmANOVA, studying the effects of treatments (between-subjects factor) and sampling time (within-subjects factor) and their interactions. Post-rmANOVA means comparisons were carried out with Bonferroni's correction. Data of Fusarium spp. population density was analyzed using Kruskal-Wallis non-parametric test. Phytotoxicity data were subjected to two-way ANOVA with dose and species as factors followed by Tukey's multiple range tests. Effects and differences were considered significant at P < 0.05. All analyses were performed with the software package SPSS version 20.0 (SPSS Inc., Chicago, Illinois, USA).
Results
Soil Temperature, Moisture, and pH During the Field Experiment
Soil temperatures at 20-cm in plastic-covered treatments (CP and BF3) fluctuated between 15 and 35°C, and a similar pattern in soil temperature was observed in these treatments. In covered plots, the greatest number of cumulative hours registered (~200 h) were between 20 and 25°C and only around 150 h above 25°C (Figure 1). Soil temperatures in the non-covered treatment fluctuated between 12 and 27.5°C, with the highest number of accumulated hours (217 h) around 17.5°C and only 15 h above 25°C.
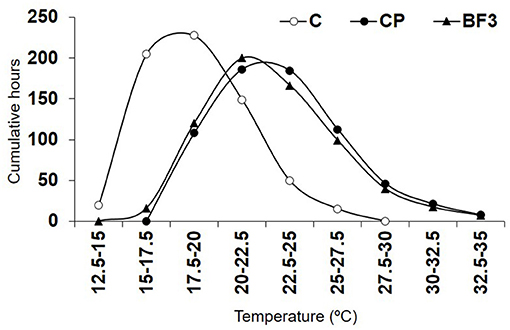
Figure 1. Mean soil temperature at 20-cm depth during the biofumigation field experiment, expressed as cumulative hours at different temperature ranks. Control non-amended without plastic cover (C); control non-amended (CP); Brassica carinata pellets 3 tons ha−1 (BF3). Values represent means of data collected from two sensors.
Soil moisture in covered plots was maintained around field capacity (20 to 22 m3 m−3 of VWC) throughout the experiment, while in control without plastic cover soil moisture decreased, inconstantly due to occasional rains, to values of 12 m3 m−3 of VWC.
Soil pH did not significantly change in time, either in the control treatments (C and CP) or in BF3 treatment (Figure 2). Soil pH was kept between 6.5 and 7.2, and no differences were found in pH values among treatments throughout the experiment.
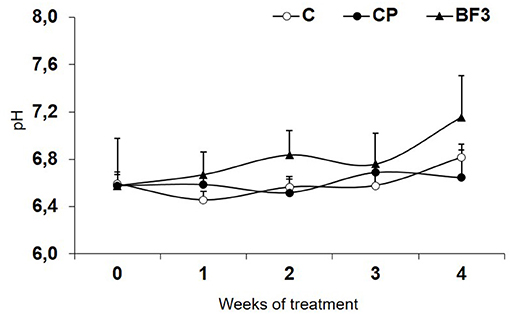
Figure 2. Evolution of soil pH during the biofumigation field experiment. Control non-amended without plastic cover (C); control non-amended (CP); Brassica carinata pellets 3 tons ha−1 (BF3). Values are means ± SD (n = 4).
Effect of Commercial Rate of B. carinata Pellets on Survival and Infectivity of Chlamydospores on Pepper in the Field Experiment
There was no noticeable effect of the biofumigation with B. carinata pellets on the survival of P. nicotianae in soil from recovered field inoculum bags. The estimated survival was very high for all treatments, and no statistical differences were found in any case (Chi2 = 1.363; P = 0.506). The percentages of positive baits detected were 88 ± 14% (mean ± sd; n = 4) in CP and 94 ± 13% in BF3, achieving 100 ± 0% of positive baits when the plastic cover was not used (C). The use of a plant disease bioassay confirmed the pathogenicity of the surviving population of P. nicotianae. There was no significant effect of treatment on infectivity (Chi2 = 8.6 e−6; P = 1.00), with trends very similar to that observed related to survival. Both covered treatments (CP and BF3) showed the same infectivity results (88 ± 14% of diseased pepper plants), while all plants showed root and crown rot disease in control without plastic cover (C).
Effects of Commercial Rate of B. carinata Pellets on Weed Biomass and Native Fusarium spp. Population Density in the Field Experiment
Weed biomass was not affected by treatment [F(2) = 1.869, P = 0.210]. Biofumigated plots (BF3) had the highest level of weeds (31.5 ± 39 g of dry weight m−1; n = 4), with Cyperus rotundus and Setellaria media being the predominant species, although these results were inconsistent due to differences between blocks and did not show significant differences compared with CP (23.8 ± 5.5 g of dry weight m−1). Weed populations were lower in the non-covered control (C) (1.43 ± 1.9 g of dry weight m−1), probably due to less favorable temperature and moisture conditions for seed germination in these plots.
Four Fusarium species were isolated from the soil before and after the treatments: F. oxysporum, F. solani, F. roseum and F. moniliforme. The total Fusarium spp. population density was over 3,982 ± 1,795 CFU g−1 of soil (n = 48) in all plots at the end of the experiment. The Kruskal-Wallis test conducted did not show differences among treatments [H(2) = 1.69, P = 0.428].
Effects of Commercial Rate of B. carinata Pellets on Soil Physicochemical Properties and Soil Enzyme Activity in the Field Experiment
Physicochemical soil properties before and after field experiment are presented in Table 1. Before pellet incorporation (0 weeks), no significant differences were found between plots for any of the soil properties studied, indicating the homogeneity of the plots before the setting up of the trial. At the end of the field experiment, there were significant increases in EC. No significant increase was observed in OM in BF3 treatment. The increases in were significant in all cases, but no differences were found between treatments at the end of the experiment. The increases in available P were significant only in BF3, although there were no differences among treatments at the end of the experiment.
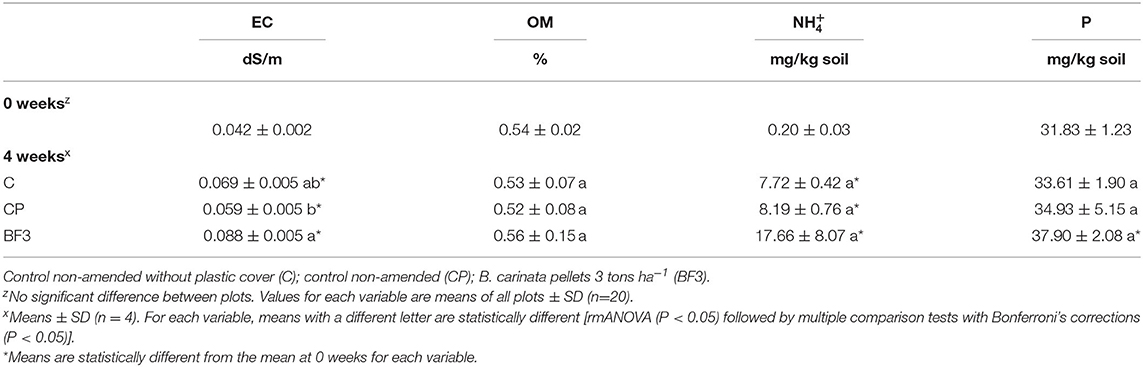
Table 1. Soil properties before Brassica carinata pellets incorporation (0 weeks) and at the end of the treatments in the field experiment (4 weeks).
Soil enzymatic activities before and after field experiment are presented in Table 2. Just as with the physicochemical properties, there were no differences among plots at the beginning of the experiments. The treatment with pellets (BF3) did not enhance the soil enzymatic activities, and the results showed no significant differences compared with the controls (C and CP) for any enzyme studied.
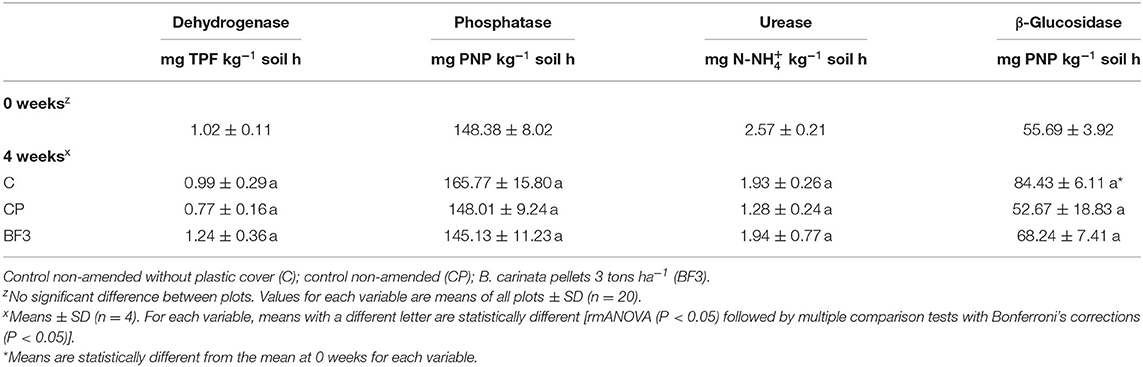
Table 2. Soil enzyme activities before Brassica carinata pellets incorporation (0 weeks) and at the end of the treatments in the field experiment (4 weeks).
Effects of Volatiles Released From B. carinata Pellets on Chlamydospores in the Laboratory Experiments
Volatile compounds released into the headspace of containers from the biofumigant rate BF3 did not suppress the chlamydospore germination of P. nicotianae (Table 3). Similarly, doubling the recommended commercial rate (BF6) was also ineffective on chlamydospore inactivation. However, when the pellets rate was increasing (BF20) or the rate BF3 was added into autoclaved soil (BF3-AS) no germination of chlamydospores was observed in any plate.
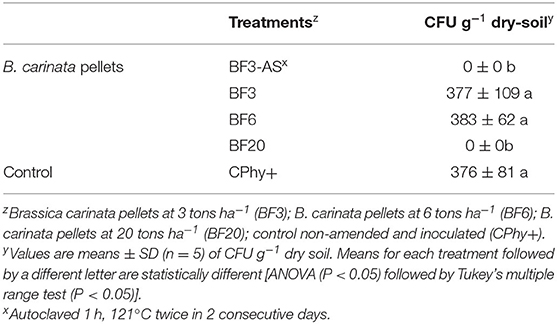
Table 3. Number of chlamydospores of Phytophthora nicotianae surviving in the inoculum bags after the exposure to the volatile compounds generated during the biofumigation process in the laboratory experiments.
Effects of B. carinata Pellets on Survival and Infectivity of Chlamydospores on Pepper in the Laboratory Experiments
All rates of pellets assayed decreased the infectivity of the pathogen with respect to the control without pellets and the effect of treatment was significant on infectivity (Chi2 = 10.17; P = 0.037). No significant differences were detected between BF3 and BF6 biofumigant rates and the control treatment (Figure 3). This lack of significance may be due to low infectivity reported in the control treatment (25% of diseased plants). Marginally significant differences (P = 0.056) were detected between the BF20 and BF3-AS treatments and the control treatment. The effect of the B. carinata pellets was more noticeable on the survival of P. nicotianae inoculum than on infectivity on pepper (Chi2 = 66.23; P < 0.001). The doubled commercial rate (BF6) reduced the inoculum survival significantly, but no differences were found between the commercial rate BF3 and the control without pellets. On the contrary, when the rate BF3 was added into the autoclaved soil (BF3-AS) or the rate was increased (BF20), the infectivity on pepper and the inoculum survival were drastically reduced (Figure 3).
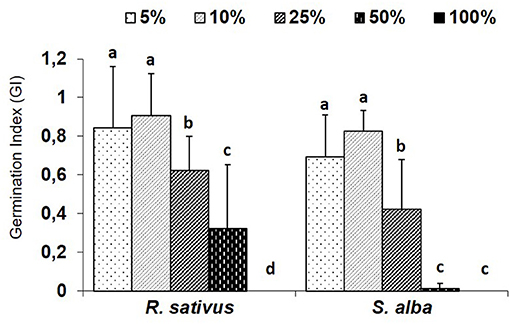
Figure 3. Survival and infectivity of Phytophthora nicotianae after laboratory experiments. Brassica carinata pellets at 3 tons ha−1 (BF3); B. carinata pellets at 6 tons ha−1 (BF6); control non-amended and inoculated (CPhy+). Treatment followed by AS was prepared with autoclaved soil. Survival was determined by detecting P. nicotianae with carnation petals as baits and was expressed as % of positive baits (n = 10). Infectivity was determined by bioassays with pepper plants and expressed as % of diseased plants (n = 5). Values are means ± SD (n = 4). For survival, different letters in bars indicate significant differences [Chi2 = 66.23; P < 0.001, followed by Tukey's multiple range test (P < 0.05)]. For infectivity, different letters in bars indicate marginally significant differences (Chi2 = 10.17; P = 0.037), followed by Tukey's multiple range test (P < 0.056).
Phytotoxicity Test of B. carinata Pellets
The germination indices (GI) of radish and mustard with increasing B. carinata pellet dilutions found in the plate experiment are shown in Figure 4. The ANOVA indicated that the dilution had a significant effect [F(4) = 69.46; P < 0.001], while the interaction between the two factors (dilution × species) was not significant [F(4) = 1.697; P = 0.158). The GI was gradually decreasing for rising concentrations higher than 10%, and no germination was observed either with 100% concentration in radish seeds or with 50 and 100% concentrations in mustard. Mustard seeds were significantly more sensitive [F(1) = 13.358; P < 0.001] than radish seeds, with lower GI for all dilutions assayed.
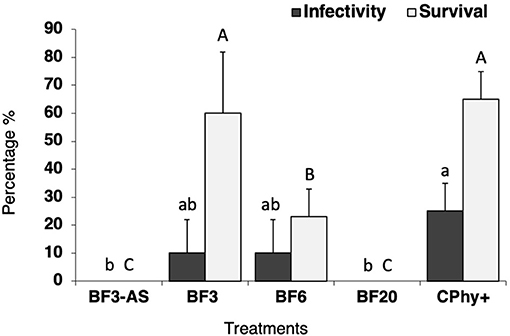
Figure 4. Phytotoxic effect of dilution ratios of Brassica carinata pellets extract on the germination index of radish (Raphanus sativus) and mustard (Sinapis alba). Values are means ± SD (n = 10). For each species, bars with a different letter are significantly different [ANOVA (P < 0.05) followed by Tukey's multiple range test (P < 0.05)].
Discussion
The ability of B. carinata commercial pellets (Biofence ®) against P. nicotianae has been previously demonstrated to inhibit the mycelial growth and the germination of chlamydospores and reduce the survival and infectivity of the inoculum on pepper plants in laboratory conditions (Serrano-Pérez et al., 2017a). However, it is noteworthy that the experiment was carried out with autoclaved soil, without any soil microorganism interference in biofumigation effectiveness.
The present work was carried out to elucidate the effect of B. carinata pellets at the recommended rate by the manufacturer (3 tons ha−1) on the survival and infectivity of P. nicotianae in a field experiment. Previous works that applied pellets at similar rates, combined or not with plastic cover and fresh Brassica amendments during the spring, did not show the expected results to replace chemical treatments for the control of P. nicotianae in Extremadura (Lacasa et al., 2015; Rodríguez-Molina et al., 2016).
Sealing the soil following biofumigant incorporation retains fumigants and creates an anaerobic environment if enough water is applied. The treatments under these conditions have been found effective against soil-borne pathogens through different mechanisms, including the production of organic acids via anaerobic decomposition of the added organic matter, production of volatiles (Momma et al., 2006; Mazzola and Hewavitharana, 2014), and biocontrol activity of fungal and bacterial communities that grow during the process (Momma et al., 2010; Mowlick et al., 2012, 2013; Butler et al., 2014; Rosskopf et al., 2015). In the present study, to ensure that an anaerobic soil environment was achieved in the biofumigation process, the soil was abundantly watered, according to Serrano-Pérez et al. (2017b). The results of the field experiment of the present study are consistent with previous works (Lacasa et al., 2015; Rodríguez-Molina et al., 2016), although initially it was considered that the lack of pathogenicity of the P. nicotianae isolate used, or the insufficient water applied after pellet incorporation into the soil (Hanschen and Winkelmann, 2020) could have been a contributing factor in the results obtained by Rodríguez-Molina et al. (2016). Furthermore, the P. nicotianae isolate (P-23) infectivity used in the current work was higher than the infectivity of the isolate used previously (P-13) in Rodríguez-Molina et al. (2016), evidencing the inefficacy of B. carinata pellets at that rate (3 tons ha−1) to inactivate the chlamydospores in natural soils.
When similar treatments were combined with solarization in summer, both survival and infectivity of chlamydospores of P. nicotianae and oospores of P. capsici were significantly reduced, but the high soil temperature registered was the main factor to inactivate the inoculum (Guerrero et al., 2010; Rodríguez-Molina et al., 2016). In a strawberry field trial, the combination of solarization with 2 tons ha−1 of B. carinata pellets significantly increased the percentage of plant survival with respect to the untreated control (Domínguez et al., 2014).
The present work aimed to identify changes in chemical and biological soil properties with the incorporation of the pellets into natural soils. The incorporation of organic matter can enhance microbial biomass and enzyme activities due to increased organic C content in the soil (Haynes, 1999). In our field experiment, dehydrogenase levels in the soil before treatments were like those reported in other studies (Leirós et al., 2000; Trasar-Cepeda et al., 2000; Quilchano and Marañón, 2002), and the incorporation of pellets at 3 tons ha−1 did not lead to a significant increase in enzyme activity of the soil. However, dehydrogenase activity increased slightly in biofumigated soil. Similar results have been reported in soils treated with the same pellets rate at the end of the summer season (Núñez-Zofío et al., 2011). The incorporation of pellets into the soil did not produce remarkable changes in the soil properties. Soil pH was minimally affected, suggesting that the buffer capacity of the soil maintained stable pH values. Higher concentrations of were measured in biofumigated plots, but the results did not differ significantly.
Although some authors have reported the allelopathic effect of Brassicas on weeds (Boydston and Hang, 1995; Eberlein et al., 1998; Krishnan et al., 1998; Mattner et al., 2008), this effect was not observed in our field experiment. The biofumigation with 3 tons ha−1 of pellets had not either significant effect on total Fusarium spp. density in soil. However, repeated applications of biosolarization using manure amendments have shown similar or even more significant reductions on Fusarium population than the effect of methyl bromide (Martínez et al., 2011).
We intended to understand the lack of success of the commercial pellets in field conditions through the standardized laboratory experiments that were set up to emulate the biofumigation process in field with different pellets rates. The toxicity of the B. carinata pellets added to the soil against chlamydospores of P. nicotianae was verified in this study, although it depended on the rate and on the presence of soil microbiota. The commercial rate (BF3; 3 tons ha−1) reduced populations of P. nicotianae below the limits of detection of our assay (<2 CFU g−1 of soil) and controlled disease on pepper, but only when the pellets were added to autoclaved soil. The effects observed in the present study against P. nicotianae agreed with previous results when vermiculite-inoculum was used under controlled conditions (Serrano-Pérez et al., 2015). However, the control efficacy was drastically reduced when the same rate of pellets was incorporated into non-autoclaved soil. Some authors reported that the biological activity of pure 2-propenil isothiocyanate (=AITC), as well as Brassica tissues, was reduced when it was incorporated into soils (Matthiessen and Shackleton, 2005). A laboratory study showed an efficacy of only 27% on Verticillium dahliae microsclerotia using 1.6 g kg−1 of B. carinata pellets (equivalent to BF3 dose) in naturally infested soil (Wei et al., 2016). On the contrary, a study demonstrated that incorporating this commercial product into pasteurized compost at the same rate as the BF3 treatment significantly reduced the carpogenic germination of Sclerotinia sclerotiorum sclerotia comparing with the untreated control (Warmington and Clarkson, 2016).
Some authors associate the failure of biofumigation with B. carinata pellets (3 tons ha−1) to the high organic carbon content of the soil (5 to 10% of humus) (Neubauer et al., 2014). However, this factor does not seem to be the leading cause of the inefficacy of the lower pellets rates in our experiments, given the low organic carbon content in the soil.
In this study, it was proved that the biofumigation with commercial B. carinata pellets at 3 ton ha−1 was ineffective in reducing infectivity and survival of chlamydospores when applied with the spring soil temperatures of western Spain. The results suggest that microbial degradation of the ITCs is the main factor involved in the unsatisfactory efficacy of the B. carinata pellets in our experiments. Numerous authors have reported the degradation of ITCs by soil microorganisms (Borek et al., 1995; Rumberger and Marschner, 2003; Warton et al., 2003; Price et al., 2005; Gimsing and Kirkegaard, 2009; Hanschen et al., 2015). Three times more AITC was found in autoclaved soil compared with non-autoclaved soil (Price et al., 2005), and phenylethyl-isothiocyanate added to the soil was degraded within 96 h by soil microorganism (Rumberger and Marschner, 2003). Pellets are rich in organic carbon and nitrogen and are chemically and physically readily available for soil microbial degradation (Hu et al., 2011).
The volatile compounds released by the lower doses (BF3 and BF6) in non-autoclaved soil did not inhibit the germination of the chlamydospores in the bags hung in the containers' headspace. For soil disinfestation via biofumigation, high isothiocyanate levels are needed (Hanschen and Winkelmann, 2020). Isothiocyanate levels in soils after biofumigation can range widely from 1 to 100 nmol isothiocyanate g−1 soil (Gimsing and Kirkegaard, 2009). Mazzola and Brown (2010) and Wang and Mazzola (2019) reported that the Brassicaceae seed meals optimized for biofumigation have a glucosinolate range from 170 μmol up to 303 μmol g−1 seed meal. According to Rodríguez-Molina et al. (2021), B. carinata pellets (Biofence ®) used in the present study has a concentration of 84.31 μmol g−1, which is below this range.
There was a decrease in the survival of the chlamydospores treated with the BF6 rate suggesting that other factors than GSL hydrolysis may be involved in suppressing P. nicotianae (Mazzola et al., 2001; Weerakoon et al., 2012; Hanschen et al., 2015). Besides ITCs, numerous other sulfur-containing products have been identified as secondary products of glucosinolates in Brassica tissues (Gamliel and Stapleton, 1993; Wang et al., 2009), which may have influenced the effectiveness of the rate BF6 of pellets in the laboratory experiments. The B. carinata pellets added to non-autoclaved soil could provide food for the resident communities involved in natural disease suppressiveness by regulating soil bacterial community structure (Mazzola et al., 2001). Furthermore, according to Rosskopf et al. (2015), the effects due to volatile fatty acids generated by anaerobiosis should not be excluded when adding a high dose of labile carbon.
The review of previous works using B. carinata pellets, the promising results of the double commercial rate to decrease survival of P. nicotianae, and the drastic reduction of propagules of P. nicotianae when the maximum rate of 20 tons ha−1 was added in our experiments suggest that an increase in the amount of amendment incorporated into natural soils would be necessary to suppress soil-borne pathogens. Gilardi et al. (2014, 2015) did not obtain reasonable control of P. nicotianae on tomato or P. capsici on zucchini by adding 2.5 g L−1 of B. carinata pellets. Previously, some authors had shown that these pellets at 2 g L−1 or 4 g L−1 doses were ineffective in controlling Fusarium wilt of lettuce and basil (Garibaldi et al., 2010). Moreover, unsatisfactory results on mortality of Verticillium dahliae microsclerotia using 4 tons ha−1 of B. juncea seed meal have been found (Neubauer et al., 2015).
The laboratory experiments revealed the drastic reduction of propagules of P. nicotianae when the maximum rate (20 tons ha−1) was added. The values are barely distinguishable from Gilardi et al. (2020), who used 15 tons ha−1 to control Rhizoctonia solani on lettuce. This rate was included in our experiments according to the suggestion of Butler et al. (2014) for ASD when soil temperatures were low (15–25°C) (Serrano-Pérez et al., 2017b). However, the tested application rate was more than 6 times greater than the suggested for the Biofence® product for biofumigation treatment. This rate is so high that it is likely to be neither sustainable nor applicable under field conditions. The economic value of such a high-rate application should be evaluated (Gilardi et al., 2020), as well as the associated environmental risks.
GI is a complete indicator to describe the potential phytotoxicity (Zucconi et al., 1981; Emino and Warman, 2004; Tiquia, 2010). A GI lower than 0.5 indicates strong phytotoxicity (Zucconi et al., 1981). According to this criterion, the pellets showed high phytotoxicity when were applied at high concentrations. No phytotoxicity symptoms have been observed in the pepper plants used in the bioassays. Therefore, the phytotoxicity of Biofence® at the tested doses is considered unlikely. Given that this finding is based on a limited number of plants, the results from such observation should therefore be treated with considerable caution. In Wang and Mazzola (2019) research, Brassica juncea and S. alba seed meal showed phytotoxicity and tree mortality when it was applied at 6.6 tons ha−1.
The soil microbiota degrades the rate of B. carinata pellets recommended by the manufacturer (3 tons ha−1) before being effective against P. nicotianae in the field and laboratory conditions studied. Increasing the recommended rate to 6 tons ha−1 decreases inoculum survival in the laboratory test and has no phytotoxic effects. Although pellets at 20 tons ha−1 completely inhibited the inoculum, the cost of such a high rate of the product could be the limiting factor for its application. Phytotoxic effects of Biofence® at the tested doses are considered unlikely.
Data Availability Statement
The raw data supporting the conclusions of this article will be made available on request from the authors.
Author Contributions
The study conception and design were performed by RM-MC and PS-P. The first draft of the manuscript was written by PS-P. All authors contributed to material preparation, data collection, analysis, commented on previous versions of the manuscript, read, and approved the final manuscript.
Funding
This research was supported by INIA project RTA2011-00005-C03-02, Research Group AGA001 (GR18196) of Junta de Extremadura and European Regional Development Funds. PS-P was the recipient of a FPI fellowship from Instituto Nacional de Investigación y Tecnología Agraria y Alimentaria (INIA, Spain) (BOE 2 mayo 2012).
Conflict of Interest
The authors declare that the research was conducted in the absence of any commercial or financial relationships that could be construed as a potential conflict of interest.
Acknowledgments
The authors are grateful to two referees, whose suggestions greatly improved the manuscript. We are also grateful to C. Pérez-Izquierdo for his help with the GLM analysis and to C. Palo Osorio, E.J. Palo Núñez, and M. Rodríguez Esteban for their technical assistance.
References
Angus, J. F., Gardner, P. A., Kirkegaard, J. A., and Desmarchelier, J. M. (1994). Biofumigation: isothiocyanates released from brassica roots inhibit growth of the take-all fungus. Plant Soil 162, 107–112. doi: 10.1007/BF01416095
Bello, A., López-Perez, J. A., Sanz, R., Escuer, M., and Herrero, J. (2000). “Biofumigation and organic amendments,” in Regional Workshop on Methyl Bromide Alternatives for North Africa and Southern European Countries, United Nations Environment Programme (UNEP) (Paris), 113–141.
Borek, V., Elberson, L. R., McCaffrey, J. P., and Morra, M. J. (1995). Toxicity of aliphatic and aromatic isothiocyanates to eggs of the black vine weevil (Coleoptera, Curculionidae). J. Econ. Entomol. 88, 1192–1196. doi: 10.1093/jee/88.5.1192
Boydston, R., and Hang, A. (1995). Rapeseed (Brassica napus) green manure crop suppresses weeds in potato (Solanum tuberosum). Weed Sci. Soc. Am. 9, 669–675. doi: 10.1017/S0890037X00024039
Brennan, R. J. B., Glaze-Corcoran, S., Robert, W., and Hasmemi, M. (2020). Biofumigation: an alternative strategy for the control of plant parasitic nematodes. J. Integr. Agric. 19, 1680–1690. doi: 10.1016/S2095-3119(19)62817-0
Brown, P. D., and Morra, M. J. (1997). Control of soil-borne plant pests using glucosinolate-containing plants. Adv. Agron. 61, 167–231. doi: 10.1016/S0065-2113(08)60664-1
Butler, D. M., Kokalis-Burelle, N., Albano, J. P., McCollum, T. G., Muramoto, J., Shennan, C., et al. (2014). Anaerobic soil disinfestation (ASD) combined with soil solarization as a methyl bromide alternative: vegetable crop performance and soil nutrient dynamics. Plant Soil 378, 365–381. doi: 10.1007/s11104-014-2030-z
Carballo, T., Gil, M. V., Calvo, L. F., and Morán, A. (2009). The influence of aeration system, temperature and compost origin on the phytotoxicity of compost tea. Compost Sci. Util. 17, 127–139. doi: 10.1080/1065657X.2009.10702411
Coelho, L., Chellemi, D. O., and Mitchell, D. J. (1999). Efficacy of solarization and cabbage amendment for the control of Phytophthora spp. in north Florida. Plant Dis. 83, 293–299. doi: 10.1094/PDIS.1999.83.3.293
Domínguez, P., Miranda, L., Soria, C., de los Santos, B., Chamorro, M., Romero, F., et al. (2014). Soil biosolarization for sustainable strawberry production. Agron. Sustain. Dev. 34, 821–829. doi: 10.1007/s13593-014-0211-z
Eberlein, C. V., Morra Matthew, J., Guttieri Mary, J., Brown Paul, D., and Brown, J. (1998). Glucosinolate production by five field-grown Brassica napus cultivars used as green manures. Weed Technol. 12, 712–718. doi: 10.1017/S0890037X00044596
Eivazi, F., and Tabatabai, M. A. (1988). Glucosidases and galactosidases in soils. Soil Biol. Biochem. 20, 601–606. doi: 10.1016/0038-0717(88)90141-1
Emino, E. R., and Warman, P. R. (2004). Biological assay for compost quality. Compost Sci. Util. 12, 342–348. doi: 10.1080/1065657X.2004.10702203
Erwin, D. C., and Ribeiro, O. K. (1996). Phytophthora Diseases Worldwide. American Phytopathological Society (APS Press).
Furlan, L., Bonetto, C., Finotto, A., Lazzeri, L., Malaguti, L., Patalano, G., et al. (2010). The efficacy of biofumigant meals and plants to control wireworm populations. Ind. Crop. Prod. 31, 245–254. doi: 10.1016/j.indcrop.2009.10.012
Galletti, S., Sala, E., Leoni, O., Burzi, P. L., and Cerato, C. (2008). Trichoderma spp. tolerance to Brassica carinata seed meal for a combined use in biofumigation. Biol. Control 45, 319–327. doi: 10.1016/j.biocontrol.2008.01.014
Gamliel, A., and Stapleton, J. J. (1993). Effect of chicken compost or ammonium phosphate and solarization on pathogen control, rhizosphere microorganisms, and lettuce growth. Plant Dis. 77:886. doi: 10.1094/PD-77-0886
Garibaldi, A., Gilardi, G., Clematis, F., Gullino, M. L., Lazzeri, L., and Malaguti, L. (2010). Effect of green Brassica manure and Brassica defatted seed meals in combination with grafting and soil solarization against Verticillium wilt of eggplant and Fusarium wilt of lettuce and basil. Acta Horticult. 883, 295–302. doi: 10.17660/ActaHortic.2010.883.36
Gilardi, G., Demarchi, S., Gullino, M. L., and Garibaldi, A. (2014). Managing Phytophthora crown and root rot on tomato by pre-plant treatments with biocontrol agents, resistance inducers, organic and mineral fertilizers under nursery conditions. Phytopathol. Mediterr. 53, 205–215. doi: 10.14601/Phytopathol_Mediterr-12361
Gilardi, G., Demarchi, S., Gullino, M. L., and Garibaldi, A. (2015). Nursery treatments with non-conventional products against crown and root rot, caused by Phytophthora capsici, on zucchini. Phytoparasitica 43, 501–508. doi: 10.1007/s12600-015-0461-6
Gilardi, G., Pugliese, M., Gullino, M. L., and Garibaldi, A. (2016). Effect of different organic amendments on lettuce Fusarium wilt and on selected soilborne microorganisms. Plant Pathol. 65, 704–712. doi: 10.1111/ppa.12460
Gilardi, G., Pugliese, M., Gullino, M. L., and Garibaldi, A. (2020). Evaluation of different carbon sources for anaerobic soil disinfestation against Rhizoctonia solani on lettuce in controlled production systems. Phytopathol. Mediterr. 59, 77–96. doi: 10.14601/Phyto-10911
Gimsing, A. L., and Kirkegaard, J. A. (2009). Glucosinolates and biofumigation: fate of glucosinolates and their hydrolysis products in soil. Phytochem. Rev. 8, 299–310. doi: 10.1007/s11101-008-9105-5
Gimsing, A. L., Poulsen, J. L., Pedersen, H. L., and Hansen, H. C. B. (2007). Formation and degradation kinetics of the biofumigant benzyl isothiocyanate in soil. Environ. Sci. Technol. 41, 4271–4276. doi: 10.1021/es061987t
Gimsing, A. L., Sørensen, J. C., Tovgaard, L., Jørgensen, A. M. F., and Hansen, H. C. B. (2006). Degradation kinetics of glucosinolates in soil. Environ. Toxicol. Chem. 25, 2038–2044. doi: 10.1897/05-610R.1
Guerrero, M. M., Ros, C., Lacasa, C. M., Martínez, V., Lacasa, A., Fernández, P., et al. (2010). Effect of biosolarization using pellets of Brassica carinata on soil-borne pathogens in protected pepper crops. Acta Horticult. 60, 337–344. doi: 10.17660/ActaHortic.2010.883.42
Hanschen, F. S., and Winkelmann, T. (2020). Biofumigation for fighting replant disease-a review. Agronomy 10:425. doi: 10.3390/agronomy10030425
Hanschen, F. S., Yim, B., Winkelmann, T., Smalla, K., and Schreiner, M. (2015). Degradation of biofumigant isothiocyanates and allyl glucosinolate in soil and their effects on the microbial community composition. PLoS ONE 10:e0132931. doi: 10.1371/journal.pone.0132931
Haynes, R. J. (1999). Labile organic matter fractions and aggregate stability under short-term, grass-based leys. Soil Biol. Biochem. 31, 1821–1830. doi: 10.1016/S0038-0717(99)00102-9
Hu, P., Wang, A. S., Engledow, A. S., Hollister, E. B., Rothlisberger, K. L., Matocha, J. E., et al. (2011). Inhibition of the germination and growth of Phymatotrichopsis omnivora (cotton root rot) by oilseed meals and isothiocyanates. Appl. Soil Ecol. 49, 68–75. doi: 10.1016/j.apsoil.2011.06.014
Kirkegaard, J. A., Angus, J. F., Gardner, P. A., and Cresswell, H. P. (1993). “Benefits of brassica break crops in the Southeast wheatbelt,” in Proceedings of the 17th Australian Agronomy Conference 2015 (Adelaide, SA), 282–285.
Kirkegaard, J. A., Sarwar, M., and Matthiessen, J. N. (1998). Assessing the biofumigation potential of crucifers. Acta Horticult. 459, 105–111. doi: 10.17660/ActaHortic.1998.459.10
Krishnan, G., Holshouser, D. L., and Nissen, S. J. (1998). Weed control in soybean (Glycine max) with green manure crops. Weed Technol. 12, 97–102. doi: 10.1017/S0890037X00042639
Lacasa, C. M., Martínez, V., Hernández, A., Ros, C., Lacasa, A., del Mar Guerrero, M., et al. (2015). Survival reduction of Phytophthora capsici oospores and P. nicotianae chlamydospores with Brassica green manures combined with solarization. Sci. Hortic. 197, 607–618. doi: 10.1016/j.scienta.2015.10.024
Laegdsmand, M., Gimsing, A. L., Strobel, B. W., Sørensen, J. C., Jacobsen, O. H., and Hansen, H. C. B. (2007). Leaching of isothiocyanates through intact soil following simulated biofumigation. Plant Soil 291, 81–92. doi: 10.1007/s11104-006-9176-2
Lazzeri, L., Leoni, O., De Nicola, G., Cinti, S., Malaguti, L., Curto, G., et al. (2008). Use of Seed Flour as Soil Pesticide. European Patent EP1530421B1. Munich: EPO
Leirós, M. C., Trasar-Cepeda, C., Seoane, S., and Gil-Sotres, F. (2000). Biochemical properties of acid soils under climax vegetation (Atlantic oakwood) in an area of the European temperate–humid zone (Galicia, NW Spain): general parameters. Soil Biol. Biochem. 32, 733–745. doi: 10.1016/S0038-0717(99)00195-9
Martínez, M. A. C., Martínez, M. A. C., Bielza, P., Tello, J., Lacasa, A., Martínez, M. C. A., et al. (2011). Effect of biofumigation with manure amendments and repeated biosolarization on Fusarium densities in pepper crops. J. Ind. Microbiol. Biotechnol. 38, 3–11. doi: 10.1007/s10295-010-0826-2
Matthiessen, J. N., and Kirkegaard, J. A. (2006). Biofumigation and enhanced biodegradation: opportunity and challenge in soilborne pest and disease management. Crit. Rev. Plant Sci. 25, 235–265. doi: 10.1080/07352680600611543
Matthiessen, J. N., and Shackleton, M. A. (2005). Biofumigation: environmental impacts on the biological activity of diverse pure and plant-derived isothiocyanates. Pest Manag. Sci. 61, 1043–1051. doi: 10.1002/ps.1086
Mattner, S. W., Porter, I. J., Gounder, R. K., Shanks, A. L., Wren, D. J., and Allen, D. (2008). Factors that impact on the ability of biofumigants to suppress fungal pathogens and weeds of strawberry. Crop Prot. 27, 1165–1173. doi: 10.1016/j.cropro.2008.02.002
Mazzola, M., and Brown, J. (2010). Efficacy of brassicaceous seed meal formulations for the control of apple replant disease in conventional and organic production systems. Plant Dis. 94, 835–842. doi: 10.1094/PDIS-94-7-0835
Mazzola, M., Granatstein, D. M., Elfving, D. C., and Mullinix, K. (2001). Suppression of specific apple root pathogens by Brassica napus seed meal amendment regardless of glucosinolate content. Phytopathology 91, 673–679. doi: 10.1094/PHYTO.2001.91.7.673
Mazzola, M., and Hewavitharana, S. (2014). “Carbon source-dependent volatile production and ASD efficacy for suppression of apple root pathogens and parasites. Acta Hortic. 1044, 209–214. doi: 10.17660/ActaHortic.2014.1044.24
Momma, N., Momma, M., and Kobara, Y. (2010). Biological soil disinfestation using ethanol: effect on Fusarium oxysporum f. sp. lycopersici and soil microorganisms. J. Gen. Plant Pathol. 76, 336–344. doi: 10.1007/s10327-010-0252-3
Momma, N., Yamamoto, K., Simandi, P., and Shishido, M. (2006). Role of organic acids in the mechanisms of biological soil disinfestation (BSD). J. Gen. Plant Pathol. 72, 247–252. doi: 10.1007/s10327-006-0274-z
Morris, E. K., Fletcher, R., and Veresoglou, S. D. (2020). Effective methods of biofumigation: a meta-analysis. Plant Soil 446, 379–392. doi: 10.1007/s11104-019-04352-y
Mowlick, S., Hirota, K., Takehara, T., Kaku, N., Ueki, K., and Ueki, A. (2012). Development of anaerobic bacterial community consisted of diverse clostridial species during biological soil disinfestation amended with plant biomass. J. Soil Sci. Plant Nutr. 58, 273–287. doi: 10.1080/00380768.2012.682045
Mowlick, S., Yasukawa, H., Inoue, T., Takehara, T., Kaku, N., Ueki, K., et al. (2013). Suppression of spinach wilt disease by biological soil disinfestation incorporated with Brassica juncea plants in association with changes in soil bacterial communities. Crop Prot. 54, 185–193. doi: 10.1016/j.cropro.2013.08.012
Mulvaney, R. L. (1996). Methods of Soil Analysis. Part 3. Chemical Methods. Madison, WI: Soil Science Society of America, Inc. 1123–1184.
Murphy, J., and Riley, J. (1962). A modified single solution method for the determination of phosphate in natural waters. Anal. Chim. Acta 27, 31–36. doi: 10.1016/S0003-2670(00)88444-5
Nannipieri, P., Ceccanti, B., Cervelli, S., and Matarese, E. (1980). Extraction of phosphatase, urease, proteases, organic carbon, and nitrogen from soil. Soil Sci. Soc. Am. J. 44, 1011–1016. doi: 10.2136/sssaj1980.03615995004400050028x
Neubauer, C., Heitmann, B., and Müller, C. (2014). Biofumigation potential of Brassicaceae cultivars to Verticillium dahliae. Eur. J. Plant Pathol. 140, 341–352. doi: 10.1007/s10658-014-0467-9
Neubauer, C., Hüntemann, K., Heitmann, B., and Müller, C. (2015). Suppression of Verticillium dahliae by glucosinolate-containing seed meal amendments. Eur. J. Plant Pathol. 142, 239–249. doi: 10.1007/s10658-015-0607-x
Núñez-Zofio, M., Garbisu, C., and Larregla, S. (2010). Application of organic amendments followed by plastic mulching for the control of Phytophthora root rot of pepper in Northern Spain. Acta Horticult. 883, 353–360. doi: 10.17660/ActaHortic.2010.883.44
Núñez-Zofío, M., Larregla, S., and Garbisu, C. (2011). Application of organic amendments followed by soil plastic mulching reduces the incidence of Phytophthora capsici in pepper crops under temperate climate. Crop Prot. 30, 1563–1572. doi: 10.1016/j.cropro.2011.08.020
Olsen, S. R., Cole, C. V., Watanabe, F. S., and Dean, L. A. (1954). Estimation of available phosphorus in soils by extraction with sodium bicarbonate. Circular USDA 939:19.
Panabières, F., Ali, G. S., Allagui, M. B., Dalio, R. J., Gudmestad, N. C., Kuhn, M. L., et al. (2016). Phytophthora nicotianae diseases worldwide: new knowledge of a long-recognised pathogen. Phytopathol. Mediterr. 55:20. doi: 10.14601/Phytopathol_Mediterr-16423
Porras, M., and Dubey, N. K. (2011). “Current status of natural products in pest management with special reference to Brassica carinata as a biofumigant,” in Natural Products in Plant Pest Management, ed N. K. Dubey (Wallingford: CABI, Northworthy), 205–217.
Price, A. J., Charron, C. S., Saxton, A. M., and Sams, C. E. (2005). Allyl isothiocyanate and carbon dioxide produced during degradation of Brassica juncea tissue in different soil conditions. HortSci. 40, 1734–1739. doi: 10.21273/HORTSCI.40.6.1734
Quilchano, C., and Marañón, T. (2002). Dehydrogenase activity in Mediterranean forest soils. Biol. Fertil. Soils 35, 102–107. doi: 10.1007/s00374-002-0446-8
R Core Team (2020). R: A language and environment for statistical computing. R Foundation for Statistical Computing. Viena. Available online at: http://www.r-project.org/index.html
Rodríguez-Molina, M. C., Fernández-Rebollo, P., Serrano-Pérez, P., De Santiago, A., Hidalgo-Fernández, M. T., and Campos-Navarro, F. J. (2021). Biofumigation with Brassica seed-based products combined with calcium carbonate to control Phytophthora cinnamomi root rot in cork and holm oaks. Eur. J. Plant Pathol. 159, 471–483 doi: 10.1007/s10658-020-02175-7
Rodríguez-Molina, M. C., Morales-Rodríguez, M. C., Osorio, C. P., Núñez, E. P., Alonso, E. V., Maya, M. S. D., et al. (2010). Short communication. Phytophthora nicotianae, the causal agent of root and crown rot (Tristeza disease) of red pepper in La Vera region (Cáceres, Spain). Spanish J. Agric. Res. 8, 770–774. doi: 10.5424/sjar/2010083-1277
Rodríguez-Molina, M. C., Serrano-Pérez, P., and Palo, C. (2016). Effect of biofumigation with Brassica pellets combined with Brassicaceae cover crops and plastic cover on the survival and infectivity of inoculum of Phytophthora nicotianae Breda de Haan. Pest Manag. Sci. 72, 1295–1301. doi: 10.1002/ps.4144
Romero, M. A., Sánchez, J. E., Jiménez, J. J., Belbahri, L., Trapero, A., Lefort, F., et al. (2007). New Pythium taxa causing root rot on mediterranean Quercus species in south-west Spain and Portugal. J. Phytopathol. 155, 289–295. doi: 10.1111/j.1439-0434.2007.01230.x
Rosskopf, E. N., Serrano-Pérez, P., Hong, J., Shrestha, U., del Carmen Rodríguez-Molina, M., Martin, K., et al. (2015). “Anaerobic soil disinfestation and soilborne pest management,” in Organic Amendments and Soil Suppressiveness in Plant Disease Management, eds M. K. Meghvansi and A. Varma (Cham: Springer), 277–305.
Rumberger, A., and Marschner, P. (2003). 2-Phenylethylisothiocyanate concentration and microbial community composition in the rhizosphere of canola. Soil Biol. Biochem. 35, 445–452. doi: 10.1016/S0038-0717(02)00296-1
Santana-Méridas, O., González-Coloma, A., and Sánchez-Vioque, R. (2012). Agricultural residues as a source of bioactive natural products. Phytochem. Rev. 11, 447–466. doi: 10.1007/s11101-012-9266-0
Santos, C. A. D., Abboud, A. C. D. S., and Carmo, M. G. F. D. (2021). Biofumigation with species of the Brassicaceae family: a review. Ciência Rural 51. doi: 10.1590/0103-8478cr2020040
Serrano-Pérez, P., Palo, C., and Rodríguez-Molina, M. (2017a). Efficacy of Brassica carinata pellets to inhibit mycelial growth and chlamydospores germination of Phytophthora nicotianae at different temperature regimes. Sci. Hortic. 216, 126–133. doi: 10.1016/j.scienta.2017.01.002
Serrano-Pérez, P., Rodríguez-Molina, M., Palo-Osorio, C., and Palo-Núñez, E. (2015). “Control of Phytophthora nicotianae using Brassica pellet and chicken poultry pellet incorporated into the soil under controlled conditions,” in International Plant Protection Congress (Berlin), 121.
Serrano-Pérez, P., Rosskopf, E., De Santiago, A., and Rodríguez-Molina, M. (2017b). Anaerobic soil disinfestation reduces survival and infectivity of Phytophthora nicotianae chlamydospores in pepper. Sci. Hortic. 215, 38–48. doi: 10.1016/j.scienta.2016.12.003
Tabatabai, M. A., and Bremner, J. M. (1969). Use of p-nitrophenyl phosphate for assay of soil phosphatase activity. Soil Biol. Biochem. 1, 301–307. doi: 10.1016/0038-0717(69)90012-1
Tello, J., Varés, F., and Lacasa, A. (1991). “Análisis de muestras,” in Manual de Laboratorio. Diagnóstico de Hongos, Bacterias y Nematodos Fitopatógenos, ed MAPA (Madrid: MAPA, Dirección General de Sanidad de la Producción Agraria), 39–48.
Tiquia, S. M. (2010). Reduction of compost phytotoxicity during the process of decomposition. Chemosphere 79, 506–512. doi: 10.1016/j.chemosphere.2010.02.040
Trasar-Cepeda, C., Leirós, M. C., and Gil-Sotres, F. (2000). Biochemical properties of acid soils under climax vegetation (Atlantic oakwood) in an area of the European temperate–humid zone (Galicia, NW Spain): general parameters. Soil Biol. Biochem. 32, 733–745. doi: 10.1016/S0038-0717(99)00196-0
Trevors, J. T., Mayfield, C. I., and Inniss, W. E. (1982). Measurement of electron transport system (ETS) activity in soil. Microb. Ecol. 8, 163–168. doi: 10.1007/BF02010449
Tsao, P. H. (1971). Chlamydospore formation in sporangium-free liquid cultures of Phytophthora parasitica. Phytopathology 61, 1412–1413 doi: 10.1094/Phyto-61-1412
Walkley, A., and Black, I. A. (1934). An examination of the Degtjareff method for determining soil organic matter, and a proposed modification of the chromic acid titration method. Soil Sci. 37, 29–38. doi: 10.1097/00010694-193401000-00003
Wang, D., Rosen, C., Kinkel, L., Cao, A., Tharayil, N., and Gerik, J. (2009). Production of methyl sulfide and dimethyl disulfide from soil-incorporated plant materials and implications for controlling soilborne pathogens. Plant Soil 324, 185–197. doi: 10.1007/s11104-009-9943-y
Wang, L., and Mazzola, M. (2019). Field evaluation of reduced rate Brassicaceae seed meal amendment and rootstock genotype on the microbiome and control of apple replant disease. Phytopathology 109, 1378–1391. doi: 10.1094/PHYTO-02-19-0045-R
Warcup, J. H. (1950). The soil-plate method for isolation of fungi from soil. Nature 165, 150–151. doi: 10.1038/166117b0
Warmington, R., and Clarkson, J. P. (2016). Volatiles from biofumigant plants have a direct effect on carpogenic germination of sclerotia and mycelial growth of Sclerotinia sclerotiorum. Plant Soil 401, 213–229. doi: 10.1007/s11104-015-2742-8
Warton, B., Matthiessen, J. N., and Shackleton, M. A. (2003). Cross-enhancement: enhanced biodegradation of isothiocyanates in soils previously treated with metham sodium. Soil Biol. Biochem. 35, 1123–1127. doi: 10.1016/S0038-0717(03)00164-0
Weerakoon, D. M. N., Reardon, C. L., Paulitz, T. C., Izzo, A. D., and Mazzola, M. (2012). Long-term suppression of Pythium abappressorium induced by Brassica juncea seed meal amendment is biologically mediated. Soil Biol. Biochem. 51, 44–52. doi: 10.1016/j.soilbio.2012.03.027
Wei, F., Passey, T., and Xu, X. (2016). Effects of individual and combined use of bio-fumigation-derived products on the viability of Verticillium dahliae microsclerotia in soil. Crop Prot. 79, 170–176. doi: 10.1016/j.cropro.2015.09.008
Keywords: biodisinfestation, Biofence, Brassica carinata, soil disinfestation, Phytophthora, phytotoxicity
Citation: Serrano-Pérez P, De Santiago A and Rodríguez-Molina MC (2021) Biofumigation With Pellets of Defatted Seed Meal of Brassica carinata: Factors Affecting Performance Against Phytophthora nicotianae in Pepper Crops. Front. Sustain. Food Syst. 5:664531. doi: 10.3389/fsufs.2021.664531
Received: 05 February 2021; Accepted: 18 May 2021;
Published: 10 June 2021.
Edited by:
María Del Mar Guerrero, Murcian Institute for Agrarian and Food Research and Development (IMIDA), SpainReviewed by:
Juan Fernando Hirzel, Instituto de Investigaciones Agropecuarias, ChileSorkunde Mendarte, Neiker Tecnalia, Spain
Copyright © 2021 Serrano-Pérez, De Santiago and Rodríguez-Molina. This is an open-access article distributed under the terms of the Creative Commons Attribution License (CC BY). The use, distribution or reproduction in other forums is permitted, provided the original author(s) and the copyright owner(s) are credited and that the original publication in this journal is cited, in accordance with accepted academic practice. No use, distribution or reproduction is permitted which does not comply with these terms.
*Correspondence: María del Carmen Rodríguez-Molina, Y2FybWVuLnJvZHJpZ3VlekBqdW50YWV4LmVz