Application of Streptomyces Antimicrobial Compounds for the Control of Phytopathogens
- 1Food Science Research Department, Faculty of Chemical Sciences of the Autonomous University of Coahuila, Saltillo, Mexico
- 2Nanobioscience Group, Faculty of Chemical Sciences of the Autonomous University of Coahuila, Saltillo, Mexico
- 3Research and Technical Development Department, GreenCorp Biorganiks de Mexico S.A de C.V., Saltillo, Mexico
One of the relevant problems in today's agriculture is related to phytopathogenic microorganisms that cause between 30–40% of crop losses. Synthetic chemical pesticides and antibiotics have brought human and environmental health problems and microbial resistance to these treatments. So, the search for natural alternatives is necessary. The genus Streptomyces have broad biotechnological potential, being a promising candidate for the biocontrol of phytopathogenic microorganisms. The efficacy of some species of this genus in plant protection and their continued presence in the intensely competitive rhizosphere is due to its great potential to produce a wide variety of soluble bioactive secondary metabolites and volatile organic compounds. However, more attention is still needed to develop novel formulations that could increase the shelf life of streptomycetes, ensuring their efficacy as a microbial pesticide. In this sense, encapsulation offers an advantageous and environmentally friendly option. The present review aims to describe some phytopathogenic microorganisms with economic importance that require biological control. In addition, it focuses mainly on the Streptomyces genus as a great producer of secondary metabolites that act on other microorganisms and plants, exercising its role as biological control. The review also covers some strategies and products based on Streptomyces and the problems of its application in the field.
Introduction
Phytopathogens represent a global threat to the production of food and crops, causing devastating diseases that result in significant economic losses (Valdés et al., 2017). At least 30–40% of the crop losses are caused by phytopathogenic infections and represent $ 200 billion a year worldwide (Sawicka and Egbuna, 2019; FAO, 2020).
Phytopathogenic bacteria and fungi affect all plant food products, colonizing their surface or tissues. These microorganisms cause symptoms such as spots, blight, cankers, tissue rot, and hormonal imbalances that lead to excessive growth, growth retardation, atrophy, root branching, among others (Kannan et al., 2015a). Thus, these phytosanitary problems negatively affect the world's food supply.
Chemical pesticides and antibiotics have been used to fight microbial infections. However, the use of these compounds has brought different problems such as pathogen resistance to treatment, soil salinization, and environmental contamination (Petriccione et al., 2017; Rodríguez et al., 2020).
According to this background, it is necessary to look for new antimicrobials to control phytopathogenic bacteria and fungi as alternative methods of plant protection, respectful with the environment, and less dependent on chemicals.
Waksman and Henrici first characterized the genus Streptomyces in 1943. It is classified into the family of Streptomycetaceae based on its morphology and cell wall chemotype. Previous studies have reported that more than 74% of current antibiotics have been produced by the genus Streptomyces. Streptomyces species are abundant in the soil, and are important members of the plant growth-promoting rhizobacteria (PGPR). Some species have been used in agriculture as biocontrol agents (Wang et al., 2018). Streptomyces sp are Gram-positive filamentous soil bacteria producers of various secondary metabolites, including many valuable compounds such as antibiotics, volatile organic compounds, and enzymes. Also, it induces plant resistance to pathogens (Zhao et al., 2013; Faheem et al., 2015; Nah et al., 2021). Due to the extensive production of secondary metabolites, Streptomyces species have attracted much attention to the biological control of soil pathogens. The production and release of secondary metabolites depend on several factors, such as nutritional, biological, and environmental conditions. Furthermore, they can be induced or activated by other organisms when they invade the soil space, competing for available nutrients (Sun et al., 2016).
The genus Streptomyces has been widely studied due to its biotechnological potential as a promising candidate for use in the biocontrol of phytopathogenic microorganisms. However, more information is still needed to develop novel formulations that could increase the shelf life of Streptomycetes, thus ensuring their long-term viability, sporulation activity, and efficacy of their application as a microbial agrochemical. In this sense, encapsulation offers an advantageous and environmentally friendly option for developing new biological control products.
The present review aims to describe the phytopathogenic microorganisms requiring biological control, mechanisms of Streptomyces activity, and problems of their application under field conditions.
Crop Diseases
Crop diseases are a major threat because they drastically reduce agricultural production and quality. Therefore, early detection and classification of diseases are important challenges for agricultural production (Bhadur and Rani, 2020). The damage caused by agricultural pathogens is devastating. Losses in agriculture due to diseases worldwide amount to more than 220 billion dollars annually (FAO, 2020). Plant diseases can be caused by different bacterias, fungi, and other agents. Diseased plant symptoms can include leaf spots, leaf blights, root rot, fruit rot, fruit spots, wilting, dieback, and decline. The significant impact of plant diseases is reducing the food available for humans by ultimately decreasing crop yields. This can result in unsuitable food for humans or lead to hunger in some areas (Loey et al., 2020). Therefore, recognizing plant diseases is of utmost importance in recommending and choosing the treatment for diseased plants and preventing infections of uninfected plants. The plant leaf is the most common way of detecting plant diseases as it shows different symptoms for different diseases.
Main Phytopathogenic Bacteria
Phytobacteria are an important group of plant pathogens that reduce the yields of valuable crops. They spread easily and rapidly, causing severe bacterial infections challenging to control (Kering et al., 2019). Phytopathogenic bacteria cause disease in many plants, causing symptoms such as spots, cankers, rot, hormonal imbalances that lead to excessive plant growth or growth retardation, root branching, foliar epinasty, among others (Kannan et al., 2015a). These problems affect plants at a qualitative and quantitative level, negatively damaging the world food supply (Kannan et al., 2015b), generating devastating damage and significant economic losses worldwide each year to the food production chain. Among the phytopathogenic bacteria with the highest incidence in agriculture are Clavibacter michiganensis, Pseudomonas syringe, Pseudomonas corrugate, Xanthomonas campestris (Andrade-Bustamante et al., 2017), Erwinia amylovora, Ralstonia solanacearum, and Xylella fastidiosa (Martins et al., 2018). These pathogens cause specific diseases in plants using different infection mechanisms and pathogenicity mechanisms depending on the microorganism in question (Table 1). Some authors place these phytopathogens as those with the most significant economic impact worldwide.
Main Phytopathogenic Fungi
Phytopathogenic fungi pose serious problems worldwide in cultivating economically important plants, especially in the subtropical and tropical regions. Phytopathogenic fungi are responsible for diseases in many vegetable and fruit products, appearing during crop development or postharvest. In addition, the phytopathogenic fungus produces toxic metabolites that can be carcinogenic and constitute a risk to public health. In contrast, the growth of specific fungi results in nutritional and chemical changes, poor appearance, and food flavor development (Vashistha and Chaudhary, 2019). Among the phytopathogenic fungi with the highest incidence in agriculture and with the most significant economic impact are Fusarium, Botrytis cinerea, Alternaria alternata, and Colletotrichum spp. These pathogens cause specific diseases in plants using different infection mechanisms and pathogenicity mechanisms depending on the microorganism in question (Table 2).
Phytopathogenic Microorganism's Control
One of the traditional control methods is cultural control. Cultural control consists of standard agricultural practices to help prevent attacks by phytopathogens, make the environment less favorable for their development, destroy them, or reduce their damage (Valdés et al., 2017). The application of agricultural practices requires adequate knowledge about the physiology and phenology of cultivated plants and their agronomic characteristics, the modalities of suitable agricultural practices, and a good understanding of the biological behavior of local pests and their seasonal occurrence. Among the leading practices within cultural control, FAO (2021) recommends sowing healthy seeds, planting in soils without a history of the presence of these phytopathogens, using uncontaminated irrigation water, rotating crops with non-host crops such as garlic or onion, ensuring good soil drainage, control soil pests that can help spread and infection, and disinfect agricultural equipment with 2% sodium hypochlorite, agricultural iodine, or quaternary ammonia (Valdés et al., 2017). However, it is widespread that these practices are complemented with pesticides to increase their effectiveness.
The increasing use of synthetic chemicals to control phytopathogenic microorganisms has caused the development of pathogens' resistance to applied chemical products and the negative impact on the environment (Martins et al., 2018). The demand for chemical-free agrochemicals has increased due to the toxicity of the chemicals to humans. The control of diseases of microbial origin is based on the use of antibiotics and solutions whose main component is copper. The application of copper to the field has environmental impacts due to its toxicity in the soil and plants (Hippler et al., 2018). The use of cupric compounds as antimicrobial agents has provided the first evidence of cells resistant to this compound in Xanthomonas sp., Pseudomonas sp., and Erwinia sp (Martins et al., 2018).
Conventional chemicals do not always provide adequate infection control. Some of the phytopathogenic microorganisms colonize the intercellular spaces of the leaves and invade other parts of the plant (El Karkouri et al., 2010). In addition, frequent applications of synthetic agrochemicals increase the risk of microorganisms developing resistance. For example, B. cinerea is considered a high-risk pathogen in terms of resistance to fungicides (Bolívar-Anillo et al., 2020).
Chemical control by pesticides causes a lot of harm to humans and the environment. However, lack of access to effective pesticides and disease-resistant cultivars has motivated alternative strategies to control these diseases. For this reason, the development of complementary methods to chemical control, such as non-pathogenic microorganisms as biological control agents, new sources of natural antimicrobials that are more friendly to the environment and consumers, has been the focus of the scientific community.
If disease-resistant commercial cultivars are not available, the use of chemical control is widespread. The disadvantage of this method is the dangers to the environment and human health and its limited effectiveness. Crop rotation can prevent some diseases, but it is not always feasible; some pathogens can go undetected in asymptomatic hosts or survive for many years in the soil. This scenario justifies an urgent need for agents that effectively control phytopathogens.
The European Union and the United States are the primary consumers of chemical fungicides worldwide. However, since 2011 the use of these chemical agents has decreased, mainly in the US, perhaps due to environmental protection and consumer health regulations (Carbu et al., 2016). The main phytosanitary companies have been investing in the field of Biocontrol in response to legal restrictions and consumer demand for pesticide-free foods (Bolívar-Anillo et al., 2020). In 2011, the global biocontrol market had a $ 2.1 billion reported value and was influenced by the growing demand for organic products (Velivelli et al., 2014). In 2019, organic products had a more significant market share, representing around 65.0%. The use of metabolites produced by microorganisms or the microorganisms themselves to control plant diseases has received increased attention, with a few exceptions. They have no adverse effects on human or animal health and are friendly to the environment (Bolívar-Anillo et al., 2020). Biological methods to control plant pathogens have been studied for more than 70 years. Biological control agents can directly inhibit pathogens through physical contact or through specific mechanisms to combat the pathogen (hyperparasitism, predation, etc.).
Furthermore, they can act indirectly through general mechanisms that do not target a particular type of pathogen (stimulation of plant defenses, competition by substrates, etc.). Otherwise, they can act by antagonism of diverse pathways (antibiotics, lytic enzymes, etc.), which are compatible with each other and can act simultaneously or synergistically (Bardin et al., 2015). However, biological control agents' effectiveness depends on climate variation, ecological competition, the intrinsic traits of the biological control agents, the exercise of selection pressure, and the quality of the product as formulated.
Streptomyces as Biological Control Microorganisms
Generalities of the Streptomyces Genus
Germ tubes grow by spreading the tips of the hyphae and then branching off. Finally, a dense vegetative cell network forms the vegetative mycelium (Jones and Elliot, 2017).
The genus Streptomyces is the most prominent family Streptomycetaceae (order Actinomycetales), which comprises more than 700 species. They are Gram-positive, neutrophilic, facultative aerobic, mesophilic filamentous bacteria, with a growth temperature between 25 and 35°C, whose DNA has a G + C content higher than 70% (Botas Muñoz, 2013; Law et al., 2017). Microorganisms of the Streptomyces genus have a complex life cycle. Streptomyces species are spore-producing bacteria. Bacteria life cycle begins with the germination of spores. The germ tubes grow by extending the tips of the hyphae, then they branch. Eventually, a dense network of vegetative cells forms the vegetative mycelium (Jones and Elliot, 2017). Streptomyces' growth cycle has several stages: vegetative growth, aerial hyphae formation, and sporulation (Figure 1). These stages are subject to different genetic controls. The bld genes regulate the formation of aerial hyphae. The bld genes regulate the production of specific proteins of aerial hyphae and, in some cases, activate a specialized metabolism (Hengst et al., 2010). Subsequently, whi genes are activated that encode regulatory proteins, which promote cell division, chromosomal segregation, and spore maturation (Bush et al., 2013). The filamentous life cycle appears to be particularly efficient in the soil environment.
They undergo profound morphological and physiological changes that culminate in spores' formation and produce various secondary metabolites with numerous biotechnological applications. Streptomyces sp. is the primary natural source of bioactive secondary metabolites of great importance, such as antifungals, antivirals, antitumors, antihypertensives, immunosuppressants, and mainly antibiotics (McKenzie et al., 2010; de Lima Procópio et al., 2012). The genus Streptomyces alone accounts for approximately 75% of all bioactive compounds, including antibiotics (Usha Nandhini et al., 2018). The ability of this genus to produce antibiotics and other secondary metabolites has been an evolutionary advantage, facilitating its adaptation to different and variable stress situations. In addition, the diversity of their metabolism has given them the ability to colonize different habitats and use varied carbon and nitrogen sources. Although its optimal growth pH is between 6.5 and 8, other strains of this genus capable of growing both in media with pH 9 or higher have been isolated. Its presence in the soil is favored by its mycelial growth and its ability to form spores, constituting a dispersal system and a form of resistance that favors survival during long periods of water scarcity and nutrients (Botas Muñoz, 2013).
Streptomyces secondary metabolites (SMs) are synthesized by a group of enzymes encoded by their corresponding biosynthetic gene cluster (BGC). In general, they are subject to strict and complicated regulation at the transcriptional level. Streptomyces SM biosynthesis is regulated through multiple regulatory pathways induced by both nutritional and environmental stimuli (Sun et al., 2017; Nah et al., 2021). However, several global regulatory systems present in most Streptomyces species can control morphological differentiation and SM production. The SM biosynthetic gene sets are also subject to pathway-specific regulation by linked regulatory genes (van der Heul et al., 2018). These pathway-specific regulatory genes are transcriptionally regulated in most Streptomyces species (Xia et al., 2020). Among the global regulatory proteins, the WhiB-like (Wbl) family of proteins is only present in Actinobacteria. Genome sequencing revealed the prevalence of Wbl throughout the species. Fourteen Wbl proteins were identified in S. coelicolor, with 11 encoded on the chromosome and three encoded on the sizeable linear plasmid, SCP1. The WblAsco is a WhiB4 ortholog, one of three members of the Wbl-family that regulates both SM differentiation and biosynthesis. In S. coeilcolor, a WblAsco mutant exhibits some aerial hyphae that do not sporulate and appear thinner than the wild type (Fowler-Goldsworthy et al., 2011).
Most Streptomyces are efficient rhizosphere colonizers. They can also be endophytes that colonize the inner tissues of host plants (Sousa and Olivares, 2016). These characteristics may be due to multiplication rate, antibiotics, controlled gene expression quorum detection, cellulases, chitinase, lipase, and β-1,3-glucanase production, siderophore, phytohormones, or amino acids synthesis. The attraction of Streptomyces exudate to the rhizosphere is achieved through the chemotactic movement of these microbes (Olanrewaju and Babalola, 2019).
Streptomyces Production of Metabolites for Biological Control
Several studies have reported the effects of beneficial microorganisms and secondary plant metabolites as a bio-alternative to control phytopathogenic bacteria and fungi. These bioactive compounds tend to affect several pathogenic species, are biodegradable and friendly to the environment (Valdés et al., 2017). The use of microbial metabolites or the microorganisms themselves to control plant diseases has received greater attention because they do not negatively affect human or animal health, are respectful of the environment and do not affect other beneficial organisms (Syed Ab Rahman et al., 2018). Although biological methods to control plant pathogens have been studied for more than 70 years, biocontrol products represent only 3.5% of the global pesticide market, which is still dominated by synthetic pesticides (Carbu et al., 2016).
Streptomyces' antagonistic activities against pathogens can be achieved through three main mechanisms: competition for nutrients and space, antibiosis, and parasitism (Boukaew and Prasertsan, 2014). Streptomyces spp can colonize the surfaces of plant roots, survive in various soil types, and produce spores that allow them to remain longer and in different extreme conditions (Law et al., 2017). Streptomyces strains have a broad spectrum of antibiotics, volatile organic compounds (VOC), which act against pathogens, disrupting bacterial cell-cell communication (quorum sensing), and a variety of enzymes that degrade the cell wall of fungi (Dias et al., 2017; Wonglom et al., 2019; Song et al., 2020) (Table 3).
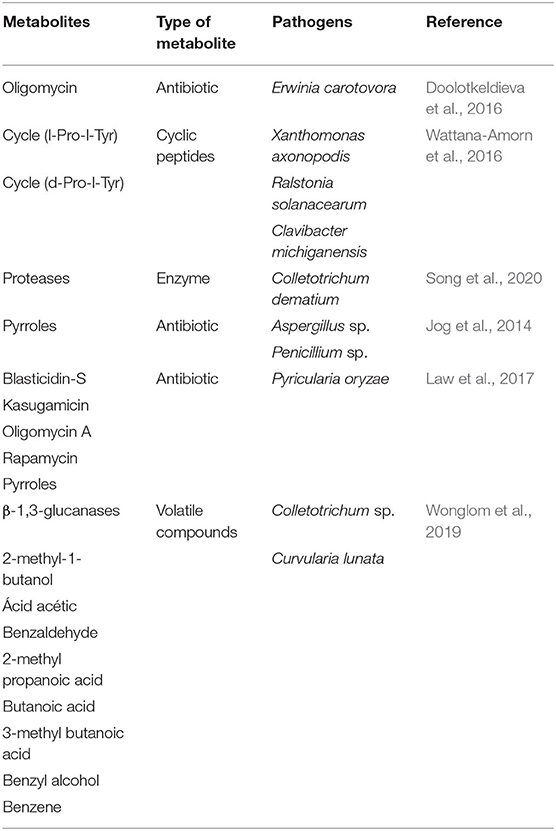
Table 3. Compounds produced by Streptomyces spp. with antimicrobial activity against phytopathogens.
Streptomyces as a Producer of Lytic Enzymes
Streptomycetes synthesize several lytic enzymes during their metabolic processes. They contribute substantially to carbon recycling by degrading different biopolymers that come from dead plants and animal material. These organic compounds, which include xylan, chitin, and cellulose, are degraded by exoenzymes. The products are channeled into the cell by specific carbohydrate importers that generally recognize mono- and disaccharides. Bentley et al. (2002), after sequencing all the genomes of the model organism Streptomyces coelicolor A3, reported a considerable number of 172 genes encoding secreted proteins such as hydrolases, chitinases, cellulases, lipases, nucleases, and proteases, as well as the appearance of 81 ATP-binding cassettes (ABC) permeases that are possibly used for the uptake of sugars, oligopeptides, nucleosides, and for drug export. Exoenzymes and ABC systems are 5- to 10-times higher than those of other bacteria, underscoring the general metabolic capacity of streptomycetes (Vurukonda et al., 2018).
Studies with S. lividans reveal that there are two commonly used pathways for secreting extracellular proteins. The Sec pathway is the primary system used to release extracellular proteins through the cell membrane. A secondary system, also found in S. lividans, is the Tat pathway. The proteins secreted by Tat have the property of appearing wholly folded in the culture supernatant (Berks et al., 2000). Different types of proteins have been detected with the predicted signal peptides Sec or Tat and the antibiotic undecylprodigiosin and others proteins lacking signal peptides in vesicles within droplets found in Streptomyces. This has harmful effects on fungi and is a new way of providing proteins with potentially different applications (Gullón and Mellado, 2018).
Volatile Organic Compounds (VOCs)
The biological roles of VOCs are only now beginning to be analyzed, and initial studies show that they can have wide-ranging effects on both their producing organisms and their neighbors. VOCs can alter the antibiotic resistance profiles of bacteria, act as antifungal or antibiotic compounds, promote group behaviors such as motility and biofilm formation, and induce widespread changes in the gene expression of nearby microbes (Jones et al., 2018). VOCs can act as signaling molecules that influence gene expression, metabolism, and group behavior of responding microorganisms (Jones and Elliot, 2017). Trichoderma species use VOCs to coordinate the conidia of separate colonies (Nemčovič et al., 2008). Bacillus species use VOCs for biofilms production in response to volatile acetic acid (Chen et al., 2015). Streptomyces VOC production has been found to allow environmental conditions such as pH to be modulated. These compounds can act as signaling molecules, affecting gene expression and response group behavior within microbial communities. Most studies have explored the ecological roles of VOCs in communication and competition between unrelated species.
The antimicrobials production by Streptomyces strains can be significantly increased depending on different nutritional and cultivation conditions. A deficit of nutrients can influence the development of hyphae and, therefore, produce these antimicrobial compounds. These environmental signals are interpreted by cells as stress, so these compounds are considered stress metabolites due to their role in adaptability. The production of these metabolites involves some genes encoding phosphorylated guanosine nucleotide phosphate (ppGpp) (an important protein for metabolite synthesis) and regulatory proteins (Sivapragasam et al., 2017). Antibiotic production is triggered by alarmone ppGpp due to nutritional stress. In addition, some species-specific enzymes, such as lysosomal acid lipase and Streptomyces antibiotic regulatory proteins, are important in regulating metabolic pathways. The type of signals received and sent by Streptomyces determines the metabolites that will be produced. For example, the attack of pathogens activates a signal, the deficit of nutrients sends a signal, etc. The α-butyrolactones are among the main signaling molecules in PSPG cell-cell communication (Olanrewaju and Babalola, 2019).
Therefore, the constitution of the culture medium and the metabolic capacity of the producer organism is of critical importance to produce secondary metabolites (Gao et al., 2009; Thakur et al., 2009). The basal medium can play an essential role in secondary metabolites production through fermentation. The main parameters that can influence are the nutrients (nitrogen, source of phosphorus, and carbon), growth rate, enzymatic inactivation, and some variable conditions such as oxygen supply, temperature, light, and pH. Furthermore, the production of valuable metabolites by actinomycetes differs qualitatively and quantitatively according to the used strains (Oskay, 2011). Effect of temperature, pH, agitation, concentrations of soybean meal, and glycerol on clavulanic acid production by Streptomyces DAUFPE 3060 was investigated by Viana et al. (2010). These authors reported that the highest antibiotic concentration (494 mg/L) was obtained after 48 h at 150 rpm, 32°C, pH 6.0, 5.0 g/L of glycerol, and 20 g/L of soybeans. On the other hand, Oskay (2011) reported the best conditions to produce antimicrobials from Streptomyces sp. KGG32 were at pH 7.5, 30°C, 120 h in a medium with 2% sucrose.
Activation of Plant Resistance Mechanisms
As we mentioned earlier, Streptomyces is considered PGPR because these bacteria are among the most effective and eco-friendly methods for managing plant disease (Compant et al., 2005). In addition, PGPR as a biocontrol agent has several benefits over chemical practices because PGPR is non-toxic, and naturally occurring microorganisms having endurable applications (Loey et al., 2020).
Streptomyces induce plant resistance to pathogens (Faheem et al., 2015). This last mechanism generally depends on the plant's jasmonic acid and ethylene (Pieterse et al., 2014). It results in a faster and stronger activation of defense responses when attacked by microorganisms, insects, or subjected to abiotic stress. This response is frequently associated with the development of local and systemic immunity in plants (Lucas et al., 2014). Plants contain systematic and structural antibiotic barriers that include antimicrobial chemicals such as phenolics, terpenoids, i.e. phytoalexins, and nitrogen-containing compounds, as well as small peptides; all of these can be secreted into infected areas (Kessler, 2011). Its mechanisms of action influence the regulation of plant resistance to a broad spectrum of phytopathogens (Kannan et al., 2015a). Plants also produce secondary metabolites and enzymes synthesized by a defense system called Pathogen Associated Molecular Patterns (PAMPs). Chitinases, peroxidases, and polyphenol oxidases are plant defense enzymes during pathogenesis and resistance against infections. Secondary metabolites comprise three chemically distinct groups (terpenes, phenolics, nitrogen, and sulfur-containing compounds) that defend plants against pathogenic microorganisms and abiotic stress (Kannan et al., 2015a).
Streptomyces Producer of Siderophores
Siderophores are chelating compounds with a high affinity for iron. They are related to mechanisms that can be used in biological control to suppress plant diseases. Siderophores produced by microorganisms in the rhizosphere can increase the availability and iron absorption. In general, siderophores are produced under iron-limiting conditions to sequester less available iron from the environment (Hider and Kong, 2010). Therefore, depriving a pathogen of iron ultimately leads to its inhibition. In addition, siderophores also enhance plant growth, promote rhizobacteria, and exclude other microorganisms from the ecological niche provided by the rhizosphere (Haas and Défago, 2005). Iron is an important mineral for plant growth and is available in the soil as insoluble Fe3+ form. Plants like oats are capable of assimilating iron through these microbial siderophores. Several root-colonizing actinobacteria have been reported to produce siderophores (Palaniyandi et al., 2013). Dimkpa et al. (2008) reported that hydroxamate siderophore produced by Streptomyces acidiscabies E13 promoted Vigna unguiculata under nickel stress. Hydroxamate siderophores secreted by Streptomyces tendae F4 significantly promoted the growth and improved cadmium (Cd) uptake by sunflower plants. They implicated this function in the phytoremediation of Cd-contaminated soil (Dimkpa et al., 2008). Streptomyces strain C, a siderophore producer, improved iron acquisition and wheat growth promotion under salinity conditions (Sadeghi et al., 2017). Streptomyces sp. MA37 synthesizes two siderophores belonging to the hydroxamate class, Legonoxamine A and B (Maglangit et al., 2019).
Application of Antimicrobial Compounds Produced by Streptomyces sp. in Agriculture
Formulations with a broad spectrum of antimicrobial action, promoting plant growth, longer shelf life, and disease suppression under field conditions would be highly attractive for technological exploitation and commercialization. Many reports suggest that commercial biocontrol agents induce plant growth and stress resistance, are easy to administer, and increase plant yield. However, for any agro-pharmaceutical industry, the biggest challenge to the success of a commercial product is its “formulation.” The formulations must include the new microorganism(s) in suitable concentrations as an active ingredient and a set of other inert ingredients to obtain a commercial product suitable for use in the field. In addition, commercial formulations must comply with all laws on agrochemicals and microbial inoculants and the requirements of producers, such as repeated positive results, easy handling, and reasonable prices. Concerning these considerations, microbial inoculants have a significant problem specific to microorganisms: loss of viability during storage complicates the need for longer shelf life and stability under typical grower storage conditions (Bhardwaj et al., 2014). Therefore, a storage period is required between the production of the formulation and the time of its use. Improving the shelf life of the formulation while maintaining its biological characteristics is an essential aspect of the formulation technique (Saberi-Riseh et al., 2021). In addition, the storage period and transport conditions must be taken into account when designing an inoculant and its packaging. A suitable formulation for biocontrol agents is the most complex and expensive part of chemical pesticides. Therefore, developing the application of biological control methods is necessary to create the importance of environmental health.
As prosperous and promising sources of agroactive compounds and biological control tools, actinomycetes have increased interest in various agricultural sectors. In the last 30 years, about 60% of the new herbicides and insecticides reported have been produced using Streptomyces because three-quarters of all this genus can produce some class of antibiotics. In comparison, by increasing our knowledge of the mechanisms activated by actinomycetes to enhance nutrient uptake by plants, stimulate and increase plant production of phytohormones, extensive research is being carried out around the world for the development of efficient formulations containing actinomycetes as their active ingredients. Although biological control with PGPR is an acceptable green approach, very few actinomycete-based products are currently marketed (Vurukonda et al., 2018).
As promising and prosperous sources of agroactive compounds and biocontrol control tools, actinomycetes have gained increasing interest in various agricultural sectors. In the last 30 years, about 60% of the new reported herbicides and insecticides have been produced using Streptomyces because three-quarters of all this genus can produce some class of antibiotics. In comparison, by increasing knowledge of the mechanisms activated by actinomycetes to enhance nutrient uptake by plants, stimulate and increase plant production of phytohormones, and suppress plant diseases, extensive research is being conducted throughout the world for the development of efficient formulations containing actinomycetes as their active ingredients. Although biocontrol with plant growth-promoting rhizobacteria is an acceptable green approach, very few actinomycete-based products are currently marketed (Vurukonda et al., 2018).
Some products based on Streptomyces spp have been developed and tested successfully to control some plant diseases. For example, Streptomyces griseoviridis strain K61 (MycostopR) has been used for Ceratocystis radicicola control, which causes black burns on date palms, and for the management of Fusarium oxysporum f. lycopersici and Verticillium dahliae in tomatoes. In addition, other products such as ActinovateR, Micro108R, and Action IronR have been made from S. lydicus WYEC108, and YAN TEN S. saraceticus has been made from S. saraceticus KH400 (Law et al., 2017; Bubici, 2018). In addition, RhizovitR from S. rimosus is used to control fungi such as Pythium spp., Phytophthora spp., Rhizoctonia solani, and Alternaria brassicola and Botrytis sp (Marten et al., 2000).
Mycoshield, Cuprimic 100 and 500, Mycoject are products based on S. rimosus to control fire spots caused by Erwinia amylovora and diseases caused by Pseudomonas and Xanthomonas sp. Agrimycin, Paushak, Cuprimicin 17, AAstrepto 17, AS-50, and Dustret are products based on S. griseus for the control of bacterial rot and canker caused by Xanthomonas oryzae, Xanthomonas citri, and Pseudomonas tabaci in stone fruits, citrus, olive trees, vegetables, potatoes, tobacco, cotton, and ornamental plants (Vurukonda et al., 2018). Four Streptomyces strains (S. lydicus WYEC108, S. violaceusniger YCED9, S. griseoviridis K61, and S. saraceticus KH400) are included in six distinct commercial preparations for the Biocontrol of fungal and bacterial soil diseases (Palaniyandi et al., 2013). These strains are usually very versatile in controlling phytopathogens. They can prevent due to their antifungal metabolites and the broad impact of their chitinases and glucanases. Three purified metabolites synthesized by Streptomyces spp (polyoxin D, streptomycin, and kasugamycin) are currently distributed as sprayed foliar fungicides and bactericides. Phosphinothricin, a glutamine synthetase inhibitor produced by Streptomyces spp, has been used since the 1990s as a herbicide, especially in the selection process for transgenic plants (Schwartz et al., 2004).
Main Problems of Formulations Based on Streptomyces
Biological control agents probably do not show the same results in vitro and in vivo, being one of the crucial challenges in the industrial development of this type of bioproduct. The effectiveness of biocontrol agents is affected by soil organic matter, pH, nutrient levels, and moisture levels. In addition, variations in environmental conditions can vary the results of biological control agents that work well in vitro; an experimentally excellent biological control agent may fail in greenhouse or field experiments. Therefore, environmental variables must be considered when selecting an efficient biological control agent for a given location. Ideally, the experimentally tested microorganisms should be isolated from the same agricultural area (Suprapta, 2012).
Streptomyces spp has a great potential to become an essential component of modern agriculture as biofertilizers and biocontrol agents, with the ability to dominate agricultural markets for the decades to come. Actinomycetes, particularly Streptomyces, are among the most promising microorganisms for improving overall soil health and increasing agricultural productivity. However, the development and efficient control are also hampered because Streptomycetes' production of antimicrobial molecules is strictly dependent on the substrate where they grow (Bibb, 2013). Furthermore, research results of the microorganism application for efficient control are generally not produced in commercial fields or greenhouses. Abiotic factors include soil type and characteristics such as nutrient levels, water content, pH and trace metals, climate, and agricultural practices. Besides, biotic factors that include crop species, plant genetics, root exudation profiles, plant age at the time of application, and competing microorganisms from the plant and soil microbiome (Newitt et al., 2019) are not considered when performing in vitro evaluations and influence the efficiency of Streptomyces for biological control.
Abiotic factors, such as soil type and characteristics such as nutrient levels, water content, pH and trace metals, climate, and agricultural practices, affect the effectiveness of the applied treatment. Additionally, biotic factors, including crop species, plant genetics, root exudation profiles, plant age at the time of application, and competing microorganisms of the plant and soil microbiome (Newitt et al., 2019), are those that are not considered during in vitro evaluations of the efficacy of Streptomyces for biological control.
The environmental risks associated with the inoculation of streptomycetes in agricultural settings are related to the lack of available data on their impact on natural microbial communities (Rafii et al., 1988). Furthermore, the release of microorganisms to the environment may pose a risk related to the possible horizontal transfer of complete or partial gene clusters; this could be particularly risky in the case of antibiotic resistance. However, this risk could be reduced during the search and study of potential biocontrollers, which must be precisely characterized for missing genes or clusters of known antibiotic-resistant genes before registration (Egan et al., 2001).
It is crucial to ensure that the Streptomyces strains are not phytotoxic when released into crops. However, if they are phytotoxic, they could be used to develop bioherbicides. Taxtomine A is a cellulose synthase inhibitor produced by Streptomyces used for this purpose (Bignell et al., 2014; Francis et al., 2015). The CebR cellobiose sensor is a transcriptional repressor of the taxtomin A gene group. The CebR repressor strictly regulates taxtomin A biosynthesis. In parallel, strains that do not synthesize taxtomin A are used as biological control agents to compete asymptomatically with virulent wild-type strains (Rey and Dumas, 2017). Their specificity and environmental toxicity are questionable, but identifying such active compounds has likely been neglected because they are amenable to screening and not attractive for pharmaceutical research. The risks of horizontal gene transfer and the development of antibiotic resistance in unselected bacterial species are crucial ethical issues still debated in the scientific community (Kurosawa et al., 2008; Schlatter and Kinkel, 2015). The stability of the metabolite formulation is also much less challenging than that of living microorganisms. Therefore, the use of Streptomyces metabolites instead of strains can accelerate the substitution of chemically synthesized pesticides.
Use of Encapsulated Formulations for Their Application in the Biological Control of Phytopathogens
Encapsulation is an advanced technology that can overcome the shortcomings of other formulations, extend the life of microorganisms, and increase their efficiency by controlling the release of microorganisms (John et al., 2011). Encapsulation is the technique applied for food constituents, enzymes, or other substances placed in a small capsule. These capsules release their content under certain conditions. The capsule wall can be composed of several polymers, decomposed by scratching, the internal pressure of the capsule, dissolved or hydrolyzed, and released by the microorganisms. For encapsulation related to controlled release, viable cells, materials, and used formulation conditions must be soft, non-toxic, and exhibit the characteristic of controllable destruction (Pour et al., 2019). Different processes, which including spray drying, spray cooling, gelation, solvent evaporation, coacervation, and extrusion are commonly used to produce encapsulated products (Li et al., 2019). There are several types of capsules, depending on the material. For example, in agriculture, polymeric capsules have been used. They are nanoparticles formed by a polymer matrix (natural or synthetic) surrounding chemical or biological molecules to protect them from extreme environments. These capsules type has been applied to improve plant growth and protect plants against pathogens (Chronopoulou et al., 2019).
A different kind of encapsulation, such as liposomes, has been used to provide antimicrobial treatments. Using these materials in agriculture as vehicles for formulations provides an advantage, a slow or delayed-release, which can help absorb the active agent in the plant's vascular system and be safer for the environment (Mikhaylov et al., 2011). Liposomes containing hydrolase enzymes that break down the cell wall of some fungi have been applied. For example, in tomato plants, the encapsulation of chitinase and laminarinase in soy lecithin liposomes maintained an enzymatic activity that allowed the control of F. oxysporum and more significant plant growth (Ilyina et al., 2013). In addition, liposomes are safer, more stable, and smaller than other encapsulation systems, thus achieving a much higher dispersion level (Yoon et al., 2014).
The encapsulation of microbial cells in polymers is a branch of biotechnology. The viability over time of the encapsulated cells depends on the concentration and type of coating used, the size of the capsule, which microorganism is being used and the initial number of cells. Encapsulation can protect the microorganism from biotic and abiotic stress (Saberi-Rise and Moradi-Pour, 2020). The encapsulated cells can slowly release the microorganisms into the soil with the controlled system and have more prolonged effectiveness.
Bacillus licheniformis encapsulated in alginate-chitosan nanoparticles beads supplemented with rice starch has been evaluated for its plant growth enhancement and disease control. The results show plants treated with encapsulated Bacillus licheniformis have enhanced disease suppression of Sclerotium rolfsii (Panichikkal et al., 2021). Saberi-Riseh and Moradi-Pour (2021) prepared formulation is a mixture of gellan and chitosan with Streptomyces fulvissimus Uts22 by spray drying. The authors reported that the increase in the bacterial release was gradual, and the highest amount of bacteria was released on the 50th day. Greenhouse experiments showed that plants treated with S. fulvissimus Uts22 microcapsules had the highest efficiency with 90% disease control against Gaeumannomyces graminis var. tritici. In addition, S. palmae CMU-AB204T spore encapsulating in alginate beads against Ganoderma boninense, revealed the lowest severity of foliar symptoms at 4.5% and reduced the percentage of disease severity by 75.8% (Kanaporn et al., 2020). Pour et al. (2019) report that Pseudomonas fluorescens strains nanocomposite beads showed a longer shelf life than non-coating bacteria and better control Fusarium solani disease's efficacy under greenhouse gas conditions.
In recent years, interest in nanoencapsulation has increased because this technology has allowed the protection and controlled release of bioactive compounds, more excellent resistance to temperature and pH variations, economic viability, less product contamination, and better stability (Geor Malar et al., 2020). Nanoencapsulation is a method of formulating and loading a substance in nanosized carriers that can hold, carry, and deliver the molecule to the targeted site. Nanotechnology applications are already being explored in biomedicine, diagnosis, and antibacterial treatments; however, their implementation in crop protection is relatively recent. The nanoencapsulation systems provide a high surface area compared to large particles. Therefore they have better functional properties such as increased solubility, high adsorption, controlled-release, and improved bioavailability (Bahrami et al., 2020). In addition, being surrounded by wall materials, as a result of the encapsulation process, protects antimicrobial agents against environmental stresses and controls their release over time. For example, Imran et al. (2016) showed that a controlled release and high retention of antimicrobial peptide nisin in coating materials could be obtained by encapsulating nisin into nanoliposome.
The development of agrochemicals nanoformulations for applying fertilizer and pesticides is one aspect of the agriculture sector receiving increasing interest (Kim et al., 2018). Nanoencapsulated pesticide formulation could help to reduce the phytotoxicity of the active ingredients. Thus enhanced solubility and stability need to be demonstrated by protecting pesticides in nanocapsules. However, challenges associated with the development of encapsulated pesticides ensure excellent reproducibility, selectivity, specificityand its controlled release (Nuruzzaman et al., 2016). On the other hand, nanocomposite beads for biological control agents can be resolved several basic plant needs. That way, the living factor trapped in these nanocomposite beads, with controlled release, has better longevity and chance of survival, and better efficiency. And also, the use of nanoparticles can stimulate plant resistance and increase plant growth (Pour et al., 2019).
Some research had addressed the use of nanoencapsulated natural products for disease management. Sattary et al. (2020) report that was encapsulating essential oils (EOs) in silica nanoparticles (SNPs) successfully increased their stability in the environment and EOs-controlled release, reduced fungicidal dose against Gaeumannomyces graminis var. tritici. It thus enhanced their efficacy compared to pure EOs in both in-vitro and in vivo conditions. Covering EOs-SNPs in alginate resulted in better disease control than non-alginate EOs-SNPs. Weria et al. (2019) encapsulated Thymus daenensis L., and Anethum graveolens L. essential oils in copper nanoparticles and evaluated their antifungal activity against the phytopathogen Colletotrichum nymphaeae. Encapsulation of these EOs with copper reduced mycelium growth even by 90% after 9 days of treatment.
The use of nanoparticles in plant disease management is a novel area that may prove very effective in the future. The use of nanomaterials and biocompatible compounds in nanoencapsulation is an important step to increase the efficiency of these agents under stressful environmental conditions for the microorganism.
Therefore, encapsulation systems are an attractive and advantageous alternative for releasing a product for the biological control of phytopathogens. However, encapsulated Streptomyces products, be it cell culture, spores, or fermented extracts, are not yet commercially available to control phytopathogenic bacteria.
Conclusions
The search for new natural and environmentally friendly antimicrobials to control phytopathogenic diseases is necessary. The Streptomyces genus has been widely studied and used for the biological control of phytopathogenic microorganisms. Streptomyces spp has excellent potential as a component of modern agricultural practices as biocontrol agents and biofertilizers, with the ability to dominate agricultural markets for decades to come. Their biotechnological potential makes them a promising candidate for use in agriculture. However, some unsolved problems need to be considered to reproduce the results of the controlled laboratory environment in large-scale field trials and commercialization. Still, more attention is required to develop novel formulations that can increase the shelf life of streptomycetes, thus ensuring their long-term viability, sporulation activity, and efficacy as microbial-based agrochemicals. Furthermore, the potential of streptomycetes to control postharvest bacterial and fungal diseases of fruits and vegetables with encapsulated Streptomyces products is unexplored.
Author Contributions
SP-M: contributions to the writing of the work and contributions to the conception and design of the work. CA, OA-P, RR-H, MC-G, RA, JA, and MG: contributions to the writing of the work and document content review. AI: contributions to the writing of the work, contributions to the conception and design of the work, document content review, and provide approval for publication of the content. All authors contributed to the article and approved the submitted version.
Funding
This research was funded by the Mexican Council of Science and Technology (CONACYT) with the financial support to carry out the project under the Cátedras-CONACYT program (grant 729).
Conflict of Interest
The authors declare that the research was conducted in the absence of any commercial or financial relationships that could be construed as a potential conflict of interest.
Publisher's Note
All claims expressed in this article are solely those of the authors and do not necessarily represent those of their affiliated organizations, or those of the publisher, the editors and the reviewers. Any product that may be evaluated in this article, or claim that may be made by its manufacturer, is not guaranteed or endorsed by the publisher.
References
AbuQamar, S., Moustafa, K., and Tran, L. S. P. (2017). Mechanisms and strategies of plant defense against Botrytis cinerea. Crit. Rev. Biotechnol. 37, 262–274. doi: 10.1080/07388551.2016.1271767
Almeida, R. P. P., Coletta-Filho, H. D., and Lopes, J. R. S. (2014). “Xylella fastidiosa,” in Manual of Security Sensitive Microbes and Toxins, ed. D. Liu (Boca Raton, FL: CRC Press). doi: 10.1201/b16752-83
Andrade-Bustamante, G., Manelik García-López, A., Cervantes-Díaz, L., Aíl-Catzim, C. E., Borboa-Flores, J., and Rueda-Puente, E. O. (2017). Estudio del potencial biocontrolador de las plantas autóctonas de la zona árida del noroeste de México: control de fitopatógenos. Rev. Facul. Ciencias Agrar. 49, 127–142.
Aoki, T., O'Donnell, K., and Geiser, D. M. (2014). Systematics of key phytopathogenic Fusarium species: current status and future challenges. J. General Plant Pathol. 80, 189–201. doi: 10.1007/s10327-014-0509-3
Bahrami, A., Delshadi, R., Assadpour, E., Jafari, S. M., and Williams, L. (2020). Antimicrobial-loaded nanocarriers for food packaging applications. Adv. Colloid Interface Sci. 278:102140. doi: 10.1016/j.cis.2020.102140
Bardin, M., Ajouz, S., Comby, M., Lopez-Ferber, M., Graillot, B., Siegwart, M.C., et al. (2015). Is the efficacy of biological control against plant diseases likely to be more durable than that of chemical pesticides? Front. Plant Sci. 6:566. doi: 10.3389/fpls.2015.00566
Bentley, S. D., Chater, K. F., Cerdeno-Tarraga, A. M., Challis, G. L., Thomson, N. R., James, K. D., et al. (2002). Complete genome sequence of the model actinomycete Streptomyces coelicolor A3(2). Nature 417, 141–147. doi: 10.1038/417141a
Berks, B. C., Sargent, F., and Palmer, T. (2000). The Tat protein export pathway. Mol. Microbiol. 35, 260–274. doi: 10.1046/j.1365-2958.2000.01719.x
Bhadur, G., and Rani, R. (2020). Agricultural crops disease identification and classification through leaf images using machine learning and deep learning technique: a review. SSRN Electron. J. 2020, 1–9. doi: 10.2139/ssrn.3564973
Bhardwaj, D., Ansari, M. W., Sahoo, R. K., and Tuteja, N. (2014). Biofertilizers function as key player in sustainable agriculture by improving soil fertility plant tolerance and crop productivity. Microb. Cell Fact. 13:66. doi: 10.1186/1475-2859-13-66
Bibb, M. J. (2013). Understanding and manipulating antibiotic production in actinomycetes. Biochem. Soc. Trans. 41, 1355–1364. doi: 10.1042/BST20130214
Bignell, D. R., Francis, I. M., Fyans, J. K., and Loria, R. (2014). Thaxtomin A production and virulence are controlled by several bld gene global regulators in Streptomyces scabies. Mol. Plant Microbe Interact. 27, 875–885. doi: 10.1094/MPMI-02-14-0037-R
Bolívar-Anillo, H. J., Garrido, C., and Collado, I. G. (2020). Endophytic microorganisms for Biocontrol of the phytopathogenic fungus Botrytis cinerea. Phytochem. Rev. 19, 721–740. doi: 10.1007/s11101-019-09603-5
Botas Muñoz, A. M. (2013). Regulación del metabolismo en Streptomyces : Control por ArgR. Doctoral dissertation, Universidad de León
Boukaew, S., and Prasertsan, P. (2014). Suppression of rice sheath blight disease using a heat stable culture filtrate from Streptomyces philanthi RM-1-138. Crop Protect. 61, 1–10. doi: 10.1016/j.cropro.2014.02.012
Bubici, G. (2018). Streptomyces spp. as biocontrol agents against Fusarium species. CAB Rev. Perspect. Agric. Vet. Sci. Nutr. Nat. Resour. 13, 1–15. doi: 10.1079/PAVSNNR201813050
Bush, M. J., Bibb, M. J., Chandra, G., Findlay, K. C., and Buttner, M. J. (2013). Genes required for aerial growth, cell division, and chromosome segregation are targets of whi a before sporulation in Streptomyces venezuelae. MBio 4, e00684–13. doi: 10.1128/mBio.00684-13
CABI (2017). Xanthomonas campestris pv. campestris (black rot). Available online at: https://www.cabi.org/isc/datasheet/56919 (accessed April 1, 2021).
Carbu, M., Gonzalez-Rodriguez, V., and Garrido, C. (2016). “New biocontrol strategies for strawberry fungal pathogens,” in Strawberry: Growth, Development and Diseases, eds A. Husaini, D. Neri (Boston: CABI)
Chatterjee, S., Almeida, R. P. P., and Lindow, S. (2008). Living in two worlds: the plant and insect lifestyles of Xylella fastidiosa. Annu. Rev. Phytopathol. 46, 243–271. doi: 10.1146/annurev.phyto.45.062806.094342
Chen, Y., Gozz, K., Yan, F., and Chai, Y. (2015). Acetic acid acts as a volatile signal to stimulate bacterial biofilm formation. MBio 6, e00392–15. doi: 10.1128/mBio.00392-15
Chronopoulou, L., Donati, L., Bramosanti, M., Rosciani, R., Palocci, C., Pasqua, G., et al. (2019). Microfluidic synthesis of methyl jasmonate-loaded PLGA nanocarriers as a new strategy to improve natural defenses in Vitis vinifera. Sci Rep. 9:18322. doi: 10.1038/s41598-019-54852-1
Coburn, B., Sekirov, I., and Finlay, B. B. (2007). Type III secretion systems and disease. Clin. Microbiol. Rev. 20, 535–549. doi: 10.1128/CMR.00013-07
Compant, S., Duffy, B., Nowak, J., Cle, C., and Barka, E. A. (2005). Use of plant growth-promoting bacteria for biocontrol of plant diseases: Principles, mechanisms of action and future prospects. Appl. Environ. Microbiol. 71, 4951–4959. doi: 10.1128/AEM.71.9.4951-4959.2005
Cook, R. J. (2010). “Fusarium root, crown and foot roots and associated seedling diseases,” in Compendium of Wheat Diseases and Pests, 3rd edition, eds W. W. Bockus, R. L. Bowden, R. M. Hunger, W. L. Morrill, T. D. Murray, and R. W. Smiley (St. Paul, MN: APS Press), 37–39
da Silva, L. L., Moreno, H. L. A., Correia, H. L. N., Santana, M. F., and de Queiroz, M. V. (2020). Colletotrichum: species complexes, lifestyle, and peculiarities of some sources of genetic variability. Appl. Microbiol. Biotechnol. 104, 1891–1904. doi: 10.1007/s00253-020-10363-y
de Lima Procópio, R. E., da Silva, I. R., Martins, M. K., de Azevedo, J. L., and de Araújo, J. M. (2012). Antibiotics produced by Streptomyces. Braz. J. Infect. Dis. 16, 466–471. doi: 10.1016/j.bjid.2012.08.014
Dean, R., van Kan, J., and Pretorius, Z. A. (2012). The top 10 fungal pathogens in molecular plant pathology. Mol. Plant Pathol. 13, 1–17. doi: 10.1111/j.1364-3703.2012.2011.00783.x
Dias, M. P., Bastos, M. S., Xavier, V. B., Cassel, E., Astarita, L. V., and Santarém, E. R. (2017). Plant growth and resistance promoted by Streptomyces spp. in tomato. Plant Physiol. Biochem. 118, 479–493. doi: 10.1016/j.plaphy.2017.07.017
Dimkpa, C. O., Svatoš, A., Dabrowska, P., Schmidt, A., Boland, W., and Kothe, E. (2008). Involvement of siderophores in the reduction of metal-induced inhibition of auxin synthesis in Streptomyces spp. Chemosphere. 74, 19–25. doi: 10.1016/j.chemosphere.2008.09.079
Doolotkeldieva, T., Bobusheva, S., and Suleymankisi, A. (2016). Biological control of Erwinia carotovora ssp. by Streptomyces species. Adv. Microbiol. 06, 104–114. doi: 10.4236/aim.2016.62011
Egan, S., Wiener, P., Kallifidas, D., and Wellington, E. M. (2001). Phylogeny of Streptomyces species and evidence for horizontal transfer of entire and partial antibiotic gene clusters. Antoine Leeuwenhoek 79, 127–133. doi: 10.1023/A:1010296220929
El Karkouri, A., El Hassani, F. Z., El Mzibri, M., Benlemlih, M., and El Hassouni, M. (2010). Isolation and identification of an actinomycete strain with a biocontrol effect on the phytopathogenic Erwinia chrysanthemi 3937VIII responsible for soft rot disease. Ann. Microbiol. 60, 263–268. doi: 10.1007/s13213-010-0036-1
Elsharkawy, M., Derbalah, A., Hamza, A., and El-Shaer, A. (2020). Zinc oxide nanostructures as a control strategy of bacterial speck of tomato caused by Pseudomonas syringae in Egypt. Environ. Sci. Pollut. Res. 27, 19049–19057. doi: 10.1007/s11356-018-3806-0
Faheem, M., Raza, W., Zhong, W., Nan, Z., Shen, Q., and Xu, Y. (2015). Evaluation of the biocontrol potential of Streptomyces goshikiensis YCXU against Fusarium oxysporum f. sp. niveum. Biol. Control 81, 101–110. doi: 10.1016/j.biocontrol.2014.11.012
FAO (2020). Crop Prospects and Food Situation—Quaterly Global Report. Crop Prospects and Food. Available online at: http://www.fao.org (accessed March 26, 2021).
FAO (2021). Integrated Pest and Pesticide Management. Available online at: http://www.fao.org/pest-and-pesticide-management/ipm/principles-and-practices/es/ (accessed March 26, 2021).
Fowler-Goldsworthy, K., Gust, B., Mouz, S., Chandra, G., Findlay, K. C., and Chater, K. F. (2011). The actinobacteria-specific gene wblA controls major developmental transitions in Streptomyces coelicolor A3(2). Microbiology 157, 1312–1328. doi: 10.1099/mic.0.047555-0
Francis, I. M., Jourdan, S., Fanara, S., Loria, R., and Rigali, S. (2015). The cellobiose sensor CebR is the gatekeeper of Streptomyces scabies pathogenicity. MBio 6, e02018–14. doi: 10.1128/mBio.02018-14
Gao, H., Liu, M., Liu, J., Dai, H., Zhou, X., Liu, X., et al. (2009). Medium optimization for the production of avermectin B1a by Streptomyces avermitilis 14-12A using response surface methodology. Bioresour. Technol. 100, 4012–4016. doi: 10.1016/j.biortech.2009.03.013
Geor Malar, C., Seenuvasan, M., Kumar, K. S., Kumar, A., and Parthiban, R. (2020). Review on surface modification of nanocarriers to overcome diffusion limitations: an enzyme immobilization aspect. Biochem. Eng. J. 158:107574. doi: 10.1016/j.bej.2020.107574
González, I., Yailén, A., and Peteira, B. (2012). Aspectos generales de la interacción Fusarium oxysporum f. sp. lycopersici-tomate. Rev. Protec. Veg. 27, 1–7.
Gullón, S., and Mellado, R. P. (2018). The cellular mechanisms that ensure an efficient secretion in Streptomyces. Antibiotics. 7:33. doi: 10.3390/antibiotics7020033
Haas, D., and Défago, G. (2005). Biological control of soilborne pathogens by fluorescent pseudomonads. Nat. Rev. Microbiol. 3, 307–319. doi: 10.1038/nrmicro1129
Hengst, C. D., den Tran, N. T., Bibb, M. J., Chandra, G., Leskiw, B. K., and Buttner, M. J. (2010). Genes essential for morphological development and antibiotic production in Streptomyces coelicolor are targets of BldD during vegetative growth. Mol. Microbiol. 78, 361–379. doi: 10.1111/j.1365-2958.2010.07338.x
Hider, R. C., and Kong, X. (2010). Chemistry and biology of siderophores. Nat. Prod. Rep., 27: 637–657. doi: 10.1039/b906679a
Hippler, F. W. R., Boaretto, R. M., Dovis, V. L., Quaggio, J. A., Azevedo, R. A., and Mattos-Jr, D. (2018). Oxidative stress induced by Cu nutritional disorders in Citrus depends on nitrogen and calcium availability. Sci. Rep. 8:1641. doi: 10.1038/s41598-018-19735-x
Ilyina, A., Leon-Joublanc, E., Balvantin-Garcia, C., Montañez-Saenz, J. C., Rodríguez-Garza, M. M., Segura Ceniceros, E. P., et al. (2013). Free and encapsulated chitinase and laminarinase as biological agents against Fusarium oxysporum. African J. Microbiol. Res. 7, 4501–4511. doi: 10.5897/AJMR12.2056
Imran, M., Revol-Junelles, A. M., Francius, G. G., and Desobry, S. P. (2016). Diffusion of fluorescently labeled bacteriocin from edible nanomaterials and embedded nano-bioactive coatings. ACS Appl. Mater. Interfaces 8, 21618–21631. doi: 10.1021/acsami.6b04621
Jog, R., Pandya, M., Nareshkumar, G., and Rajkumar, S. (2014). Mechanism of phosphate solubilization and antifungal activity of Streptomyces spp. isolated from wheat roots and rhizosphere and their application in improving plant growth. Microbiology 160, 778–788. doi: 10.1099/mic.0.074146-0
John, R. P., Tyagi, R., Brar, S., Surampalli, R., and Prévost, D. (2011). Bio-encapsulation of microbial cells for targeted agricultural delivery. Crit. Rev. Biotechnol. 31, 211–226. doi: 10.3109/07388551.2010.513327
Jones, S., Pham, C., McKillip, J., Zambri, M., Carlson, E., and Elliot, M. (2018). Streptomyces volatile compounds influence exploration and microbial community dynamics by altering iron availability. BioRxiv 396606. doi: 10.1101/396606
Jones, S. E., and Elliot, M. A. (2017). Streptomyces Exploration: Competition, Volatile Communication and New Bacterial Behaviours. New York, NY: Elsevier. doi: 10.1016/j.tim.2017.02.001
Joshi, R. (2018). A Review on Colletotrichum spp. Virulence mechanism against host plant defensive factors. Journal of Medicinal Plants Studies, 6, 64–67. doi: 10.22271/plants.2018.v6.i6b.02
Kanaporn, S., Wasu, P., Mihoko, M., Kazuyuki, D., Kazuro, S., and Saisamorn, L. (2020). Streptomyces palmae CMU-AB204T, an antifungal producing-actinomycete, as a potential biocontrol agent to protect palm oil producing trees from basal stem rot disease fungus, Ganoderma boninens. Biol. Control 148:104307. doi: 10.1016/j.biocontrol.2020.104307
Kannan, V., Bastas, K., and Antony, R. (2015a). Plant pathogenic bacteria: an overview. Sustain. App. Controll. Plant Path. Bacteria 1, 1–16. doi: 10.1201/b18892-2
Kannan, V., Bastas, K., and Devi, R. (2015b). Scientific and economic impact of plant pathogenic bacteria. Sustain. App. Controll. Plant Path. Bacteria 4, 369–392. doi: 10.1201/b18892
Kering, K. K., Kibii, B. J., and Wei, H. (2019). Biocontrol of phytobacteria with bacteriophage cocktails. Pest Manag. Sci. 75, 1775–1781. doi: 10.1002/ps.5324
Kessler, A. (2011). Plant defense: Warding off attack by pathogens, herbivores, and parasitic plants, by Dale R. Walters. Q. Rev. Biol. 86, 356–357. doi: 10.1086/662497
Kharadi, R. R., and Sundin, G. W. (2020). Dissecting the process of xylem colonization through biofilm formation in Erwinia amylovora. J. Plant Pathol. 2020, 1–10. doi: 10.1007/s42161-020-00635-x
Kim, D. Y., Kadam, A., Shinde, S., Saratale, R. G., Patra, J., and Ghodake, G. (2018). Recent developments in nanotechnology transforming the agricultural sector: a transition replete with opportunities. J. Sci. Food Agric. 98, 849–864. doi: 10.1002/jsfa.8749
Kurosawa, K., Ghiviriga, I., Sambandan, T. G., Lessard, P. A., Barbara, J. E., Rha, C., et al. (2008). Rhodostreptomycins, antibiotics biosynthesized following horizontal gene transfer from Streptomyces padanus to Rhodococcus fascians. J. Am. Chem. Soc. 130, 1126–1127. doi: 10.1021/ja077821p
Law, J. W. F., Ser, H. L., Khan, T. M., Chuah, L. H., Pusparajah, P., Chan, K. G., et al. (2017). The potential of Streptomyces as biocontrol agents against the rice blast fungus, Magnaporthe oryzae (Pyricularia oryzae). Front. Microbiol. 8:3. doi: 10.3389/fmicb.2017.00003
Li, T., Teng, D., Mao, R., Hao, Y., Wang, X., and Wang, J. (2019). Recent progress in preparation and agricultural application of microcapsules. J. Biomed. Mater. Res. Part A. 107, 2371–2385. doi: 10.1002/jbm.a.36739
Loey, M., ElSawy, A., and Afify, M. (2020). Deep learning in plant diseases detection for agricultural crops: a survey. Int. J. Serv. Sci. Manag. Eng. Technol. 11, 41–58. doi: 10.4018/IJSSMET.2020040103
Lucas, J. A., García-Cristobal, J., Bonilla, A., Ramos, B., and Gutierrez-Mañero, J. (2014). Beneficial 759 rhizobacteria from rice rhizosphere confers high protection against biotic and abiotic stress inducing 760 systemic resistance in rice seedlings. Plant Physiol. Bioch. 82, 44–53. doi: 10.1016/j.plaphy.2014.05.007
Maglangit, F., Him Tong, M., Jaspars, M., Kyeremeh, K., and Deng, H. (2019). Legonoxamines A-B, two new hydroxamate siderophores from the soil bacterium, Streptomyces sp. MA37. Tetrahedron Lett. 60, 75–79. doi: 10.1016/j.tetlet.2018.11.063
Mansfield, J., Genin, S., Magori, S., Citovsky, V., Sriariyanum, M., Ronald, P., et al. (2012). Top 10 plant pathogenic bacteria in molecular plant pathology. Mol. Plant Pathol., 13, 614–629. doi: 10.1111/j.1364-3703.2012.00804.x
Marin-Felix, Y., Hernández-Restrepo, M., Iturrieta-González, I., García, D., Gené, J., Groenewald, J. Z., et al. (2019). Genera of phytopathogenic fungi: GOPHY 3. Stud. Mycol. 94, 1–124. doi: 10.1016/j.simyco.2019.05.001
Marten, P., Bruckner, S., Minkwitz, A., Luth, P., and Berg, G. (2000). RhizovitR: Impact and formulation of a new baterial product. In: Koch E, Leinonen P (eds), Formulation of Microbial Inoculants, COST Action 830 / Microbial inoculants for agriculture and environment. Berlin: Arno Brynda, 78–82
Martins, P. M. M., Merfa, M. V., Takita, M. A., and De Souza, A. A. (2018). Persistence in phytopathogenic bacteria: Do we know enough? Front. Microbiol. 9:1099. doi: 10.3389/fmicb.2018.01099
McKenzie, N. L., Thaker, M., Koteva, K., Hughes, D. W., Wright, G. D., and Nodwell, J. R. (2010). Induction of antimicrobial activities in heterologous streptomycetes using alleles of the Streptomyces coelicolor gene absA1. J. Antibiot. 63, 177–182. doi: 10.1038/ja.2010.13
Mikhaylov, G., Mikac, U., Magaeva, A. A., Itin, V. I., Naiden, E. P., Psakhye, I., et al. (2011). Ferriliposomes as an MRI-visible drug-delivery system for targeting tumours and their microenvironment. Nat. Nanotechnol. 6, 592–604. doi: 10.1038/nnano.2011.112
Nah, H.-J., Park, J., Choi, S., and Kim, E.-S. (2021). WblA, a global regulator of antibiotic biosynthesis in Streptomyces. J. Ind. Microbiol. Biotechnol. 48:kuab007. doi: 10.1093/jimb/kuab007
Nemčovič, M., Jakubíková, L., Víden, I., and Farkaš, V. (2008). Induction of conidiation by endogenous volatile compounds in Trichoderma spp. FEMS Microbiol. Lett. 284, 231–236. doi: 10.1111/j.1574-6968.2008.01202.x
Newitt, J. T., Prudence, S. M. M., Hutchings, M. I., and Worsley, S. F. (2019). Biocontrol of cereal crop diseases using streptomycetes. Pathogens 8:78. doi: 10.3390/pathogens8020078
Nuruzzaman, M., Rahman, M. M., Liu, Y., and Naidu, R. (2016). Nanoencapsulation, nano-guard for pesticides: a new window for safe application. J. Agric. Food Chem. 64, 1447–1483. doi: 10.1021/acs.jafc.5b05214
Olanrewaju, O. S., and Babalola, O. O. (2019). Streptomyces: implications and interactions in plant growth promotion. Appl. Microbiol. Biotechnol. 103, 1179–1188. doi: 10.1007/s00253-018-09577-y
Oskay, M. (2011). Effects of some environmental conditions on biomass and antimicrobial metabolite production by Streptomyces sp., KGG32. Int. J. Agric. Biol. 13, 317–324
Palaniyandi, S. A., Yang, S. H., Zhang, L., and Suh, J. W. (2013). Effects of actinobacteria on plant disease suppression and growth promotion. Appl. Microbiol. Biotechnol. 97, 9621–9636. doi: 10.1007/s00253-013-5206-1
Panichikkal, J., Puthiyattil, N., and Raveendran, A. (2021). Application of encapsulated bacillus licheniformis supplemented with chitosan nanoparticles and rice starch for the control of Sclerotium rolfsii in Capsicum annuum (L.) seedlings. Curr. Microbiol. 78, 911–919. doi: 10.1007/s00284-021-02361-8
Petriccione, M., Zampella, L., Mastrobuoni, F., and Scortichini, M. (2017). Occurrence of copper-resistant Pseudomonas syringae pv. syringae strains isolated from rain and kiwifruit orchards also infected by P. s. pv. actinidiae. Eur. J. Plant Pathol. 149, 953–968. doi: 10.1007/s10658-017-1246-1
Pieterse, C. M. J., Zamioudis, C., Berendsen, R. L., Weller, D. M., Van Wess, S. C. M., and Bakker, P. A. H. M. M. (2014). Induced systemic resistance by beneficial microbes. Annu. Rev. Phytopathol. 52, 347–375. doi: 10.1146/annurev-phyto-082712-102340
Pour, M. M., Saberi-Riseh, R., Mohammadinejad, R., and Hosseini, A. (2019). Investigating the formulation of alginate- gelatin encapsulated Pseudomonas fluorescens (VUPF5 and T17-4 strains) for controlling Fusarium solani on potato. Int. J. Biol. Macromol. 133, 603–613. doi: 10.1016/j.ijbiomac.2019.04.071
Rafii, F., Crawford, D. L., Bleakley, B. H., and Wang, Z. (1988). Assessing the risks of releasing recombinant Streptomyces in soil. Microbiol. Sci. 12, 358–362
Rey, T., and Dumas, B. (2017). Plenty is no plague: Streptomyces symbiosis with crops. Trends Plant Sci. 22, 30–37. doi: 10.1016/j.tplants.2016.10.008
Rodríguez, M., Torres, M., Blanco, L., Béjar, V., Sampedro, I., and Llamas, I. (2020). Plant growth-promoting activity and quorum quenching-mediated biocontrol of bacterial phytopathogens by Pseudomonas segetis strain P6. Sci. Rep. 10, 1–12. doi: 10.1038/s41598-020-61084-1
Saberi-Rise, R., and Moradi-Pour, M. (2020). The effect of Bacillus Subtilis Vru1 encapsulated in alginate -bentonite coating enriched with titanium nanoparticles against rhizoctonia solani on bean. Int. J. Biol. Macromol. 152, 1089–1097. doi: 10.1016/j.ijbiomac.2019.10.197
Saberi-Riseh, R., Moradi-Pour, M., Mohammadinejad, R., and Thakur, V. K. (2021). Biopolymers for biological control of plant pathogens: advances in microencapsulation of beneficial microorganisms. Polymers 13:1938. doi: 10.3390/polym13121938
Saberi-Riseh, R., and Moradi-Pour, M. A. (2021). Novel encapsulation of Streptomyces fulvissimus Uts22 by spray drying and its biocontrol efficiency against Gaeumannomyces graminis the causal agent of take-all disease in wheat. Pest. Manag. Sci. doi: 10.1002/ps.6469. [Epub ahead of print].
Sadeghi, A., Koobaz, P., Azimi, H., Karimi, E., and Akbari, A. R. (2017). Plant growth promotion and suppression of Phytophthora drechsleri damping-off in cucumber by cellulase-producing Streptomyces. BioControl 62, 805–819. doi: 10.1007/s10526-017-9838-4
Sattary, M., Amini, J., and Hallaj, R. (2020). Antifungal activity of the lemongrass and clove oil encapsulated in mesoporous silica nanoparticles against wheat's take-all disease. Pestic. Biochem. Phys. 170:104696. doi: 10.1016/j.pestbp.2020.104696
Sawicka, B., and Egbuna, C. (2019). “Pests of agricultural crops and control measures,” in Natural Remedies for Pest, Disease and Weed Control, 1–16. doi: 10.1016/B978-0-12-819304-4.00001-4
Schlatter, D. C., and Kinkel, L. L. (2015). Do tradeoffs structure antibiotic inhibition, resistance, and resource use among soilborne Streptomyces. BMC Evol. Biol. 15:186doi: 10.1186/s12862-015-0470-6
Schwartz, D., Berger, S., Heinzelmann, E., Muschko, K., Welzel, K., and Wohlleben, W. (2004). Biosynthetic gene cluster of the herbicide phosphinothricin tripeptide from Streptomyces viridochromo genes Tü494. Appl. Environ. Microbiol. 70, 7093–7102. doi: 10.1128/AEM.70.12.7093-7102.2004
Singh, V., Shrivastava, A., and Jadon, S. (2015). Alternaria diseases of vegetable crops and its management control to reduce the low production. Int. J. Agric. Sci. 7, 834–840.
Sivapragasam, S., Deochand, D. K., Meariman, J. K., and Grove, A. (2017). The stringent response induced by phosphate limitation promotes purine salvage in Agrobacterium fabrum. Biochem 56, 5831–5843. doi: 10.1021/acs.biochem.7b00844
Song, L., Jiang, N., Wei, S., Lan, Z., and Pan, L. (2020). Isolation, screening, and identification of actinomycetes with antifungal and enzyme activity assays against Colletotrichum dematium of Sarcandra glabra. Mycobiology 48, 37–43. doi: 10.1080/12298093.2020.1716604
Sousa, J. A. de. J., and Olivares, F. L. (2016). Plant growth promotion by streptomycetes: Ecophysiology, mechanisms and applications. Chem. Biol. Technol. Agric. 3:24. doi: 10.1186/s40538-016-0073-5
Sun, D., Liu, C., Zhu, J., and Liu, W. (2017). Connecting metabolic path-ways: sigma factors in Streptomyces spp. Front. Microbiol. 8:2546. doi: 10.3389/fmicb.2017.02546
Sun, J., Pei, Y., Li, E., Li, W., Hyde, K. D., Yin, W. B., et al. (2016). A new species of Trichoderma hypoxy-lon harbours abundant secondary metabolites. Sci. Rep. 6:37369. doi: 10.1038/srep37369
Suprapta, D. N. (2012). Potential of microbial antagonists as biocontrol agents against plant fungal pathogens. J. ISSAAS 18, 1–8.
Syed Ab Rahman, S., Singh, E., Pietersen, C., and Schenck, P. (2018). Emerging microbial biocontrol strategies for plant pathogens. Plant Sci. 267, 102–111. doi: 10.1016/j.plantsci.2017.11.012
Thakur, D., Bora, T. C., Bordoloi, G. N., and Mazumdar, S. (2009). Influence of nutrition and culturing conditions for optimum growth and antimicrobial metabolite production by Streptomyces sp. 201. J. Mycol. Med. 19, 161–167. doi: 10.1016/j.mycmed.2009.04.001
Usha Nandhini, S., Sudha, S., Anusha Jeslin, V., and Manisha, S. (2018). Isolation, identification and extraction of antimicrobial compounds produced by Streptomyces sps from terrestrial soil. Biocatal. Agric. Biotechnol. 15, 317–321. doi: 10.1016/j.bcab.2018.06.024
Valdés, R., Castillo, F., and Cabello, J. (2017). Review of antibacterial activity of plant extracts and growth-promoting microorganism (Gpm) against phytopathogenic. Eur. J. 4, 11–36.
van der Heul, H. U., Bilyk, B. L., McDowall, K. J., Seipke, R. F., and van Wezel, G. P. (2018). Regulation of antibiotic production in Actinobacteria: new perspectives from the post-genomic era. Nat. Prod. Rep., 35, 575–604. doi: 10.1039/C8NP00012C
Vashistha, A., and Chaudhary, A. (2019). Effects of biocontrol agents and heavy metals in controlling soilborne phytopathogens. Int. J. Herb. Med. 7, 41–45.
Velivelli, S. L., De Vos, P., Kromann, P., Declerck, S., and Prestwich, B. D. (2014). Biological control agents: from field to market, problems, and challenges. Trends Biotechnol. 32, 493–496. doi: 10.1016/j.tibtech.2014.07.002
Viana, D. A., Carneiro-Cunha, M. N., Araújo, J. M., Barros-Neto, B., Lima-Filho, J. L., Converti, A., et al. (2010). Screening of variables influencing the clavulanic acid production by Streptomyces DAUFPE 3060 Strain. Appl. Biochem. Biotechnol. 160, 1797–1807. doi: 10.1007/s12010-009-8671-3
Vurukonda, S. S. K. P., Giovanardi, D., and Stefani, E. (2018). Plant growth promoting and biocontrol activity of Streptomyces spp. as endophytes. Int. J. Mol. Sci. 19:952. doi: 10.3390/ijms19040952
Wang, C., Wang, Y., Ma, J., Hou, Q., Liu, K., Ding, Y., et al. (2018). Screening and whole-genome sequencing of two Streptomyces species from the rhizosphere soil of peony reveal their characteristics as plant growth-promoting rhizobacteria. Biomed Res. Int. 2018:2419686. doi: 10.1155/2018/2419686
Wattana-Amorn, P., Charoenwongsa, W., Williams, C., Crump, M. P., and Apichaisataienchote, B. (2016). Antibacterial activity of cyclo(L-Pro-L-Tyr) and cyclo(D-Pro-L-Tyr) from Streptomyces sp. strain 22-4 against phytopathogenic bacteria. Nat. Prod. Res. 30, 1980–1983. doi: 10.1080/14786419.2015.1095747
Weria, W., Saadi, S., Jahanshir, A., Somaieh, H., Shima, Y., and Filippo, M. (2019). Enhancement of the antifungal activity of thyme and dill essential oils against Colletotrichum nymphaeae by nano-encapsulation with copper NPs. Ind. Crops Prod. 132, 213–225. doi: 10.1016/j.indcrop.2019.02.031
Wonglom, P., Suwannarach, N., Lumyong, S., Ito, S., Matsui, K., and Sunpapao, A. (2019). Streptomyces angustmyceticus NR8-2 as a potential microorganism for the biological control of leaf spots of Brassica rapa subsp. pekinensis caused by Colletotrichum sp. and Curvularia lunata. Biol. Control 138:104046. doi: 10.1016/j.biocontrol.2019.104046
Xia, H., Li, X., Li, Z., Zhan, X., Mao, X., and Li, Y. (2020). The Application of regulatory cascades in Streptomyces: yield enhancement and metabolite mining. Front. Microbiol. 11:406. doi: 10.3389/fmicb.2020.00406
Xin, X. F., Kvitko, B., and He, S. Y. (2018). Pseudomonas syringae: what it takes to be a pathogen. Nat. Rev. Microbiol. 16, 316–328. doi: 10.1038/nrmicro.2018.17
Yoon, Y., Kwon, Y. S., Cho, H. S., Heo, S. H., Park, K. S., Park, S. G., et al. (2014). Ultrasound-mediated gene and drug delivery using a microbubble-liposome particle system. Theranostics 4, 1133–1144. doi: 10.7150/thno.9945
Keywords: antimicrobials, biological control, Streptomyces, phytopathogen, antibiotic
Citation: Pacios-Michelena S, Aguilar González CN, Alvarez-Perez OB, Rodriguez-Herrera R, Chávez-González M, Arredondo Valdés R, Ascacio Valdés JA, Govea Salas M and Ilyina A (2021) Application of Streptomyces Antimicrobial Compounds for the Control of Phytopathogens. Front. Sustain. Food Syst. 5:696518. doi: 10.3389/fsufs.2021.696518
Received: 16 April 2021; Accepted: 06 August 2021;
Published: 09 September 2021.
Edited by:
Karl Matthews, Rutgers, The State University of New Jersey, United StatesReviewed by:
Yanhong Liu, United States Department of Agriculture (USDA), United StatesAbhinav Upadhyay, University of Connecticut, United States
Copyright © 2021 Pacios-Michelena, Aguilar González, Alvarez-Perez, Rodriguez-Herrera, Chávez-González, Arredondo Valdés, Ascacio Valdés, Govea Salas and Ilyina. This is an open-access article distributed under the terms of the Creative Commons Attribution License (CC BY). The use, distribution or reproduction in other forums is permitted, provided the original author(s) and the copyright owner(s) are credited and that the original publication in this journal is cited, in accordance with accepted academic practice. No use, distribution or reproduction is permitted which does not comply with these terms.
*Correspondence: Anna Ilyina, annailina@uadec.edu.mx