- Department of Animal Science, University of Minnesota – Twin Cities, Saint Paul, MN, United States
Lactobacillus, the largest genus within the lactic acid bacteria group, has served diverse roles in improving the quality of foods for centuries. The heterogeneity within this genus has resulted in the industry's continued use of their well-known functions and exploration of novel applications. Moreover, the perceived health benefits in many applications have also made them fond favorites of consumers and researchers alike. Their familiarity lends to their utility in the growing “clean label” movement, of which consumers prefer fewer additions to the food label and opt for recognizable and naturally-derived substances. Our review primarily focuses on the historical use of lactobacilli for their antimicrobial functionality in improving preharvest safety, a critical step to validate their role as biocontrol agents and antibiotic alternatives in food animal production. We also explore their potential as candidates catering to the consumer-driven demand for more authentic, transparent, and socially responsible labeling of animal products.
The “Clean Label” Approach and Its Implication on Food Safety
Tremendous progress in processing methods over the past decades has extended the shelf life and enhanced the microbiological safety of foods. In conjunction with these innovations, interest in knowing how foods are produced has also increased among consumers in industrialized countries. The potential health implications of unfamiliar additives that are perceived as “less natural” have driven the popularity of the “clean label” movement in developed societies. Despite significance in market trends and its growing popularity, a consensus on what constitutes a clean label has yet to be established. However, several iterations and interpretations of the label were offered in both scientific literature and popular media. These proposed definitions range from the number of ingredients, their pronounceability, and their food preservation roles (Asioli et al., 2017). The variety of interpretations highlights the clean label subjectivity and its dependence on an individual's familiarity with food ingredients and production methods.
Simply eliminating certain ingredients to meet the clean label criteria comes at the risk of changes to food quality and safety that may do more harm than good. The absence of certain preservatives may reduce the shelf life of food, leading to an increased rate of waste due to spoilage, thus decreasing the sustainability of the production system (Delgado-Pando et al., 2021). Certain additives also play an integral role in controlling pathogens. Therefore, its removal may increase the risk of foodborne illness if no alternatives were applied to control pathogens appropriately (Papadochristopoulos et al., 2021). This situation is particularly challenging for food products with energy-dense compositions readily metabolized by microorganisms, such as those derived from animals.
Bacterial Pathogens—an Immediate Threat to Clean Label Initiatives in Food Animal Production
Animal agriculture provides a valuable source of nutritious products that play an essential role in global food security. As with the production of other foods, there are many control measures to ensure animal-derived products' safety. However, microbial pathogens, particularly bacteria, present one of the most significant concerns for consumer health and industry sustainability (Sofos, 2008). Food-producing animals may serve as reservoir hosts for many microorganisms, some of which have the potential to cause harm to humans. The presence of these bacteria in the animals does not always result in symptoms of infection, but they may cause illness in humans after ingestion at disease-causing amounts. Therefore, the microbiological safety of commodities derived from animals is a global concern as it directly impacts public health and animal food production.
During production, there are multiple stages at which these microbial hazards could be introduced to the animal or its products, including farms or processing plants. Animal products are among the most common food categories associated with outbreaks and outbreak-related human illnesses. Despite only accounting for 38% of outbreaks, foodborne outbreaks involving products from land animals accounted for 53% of illnesses and 27% of hospitalizations [CDC, 2019a. Although foodborne viral pathogens cause a greater number of illnesses, bacterial pathogens tend to cause more hospitalizations and deaths (Scallan et al., 2011). The pathogens responsible for outbreaks vary, with some pathogens more common than others. Among the many bacterial pathogens present in animals, Salmonella, Campylobacter, Escherichia coli, and Listeria are the predominant outbreak-related agents of concern in food animal production.
The transmission of pathogens from animals to humans is not limited to food consumption. However, only outbreaks where food consumption is the primary mode of transmission will be discussed in this review article. The major bacterial pathogens and the food-producing animals involved in their transmission are summarized in Table 1 based on data available on the National Outbreak Reporting System (NORS) (CDC, 2019b).
In 2017, 841 foodborne disease outbreaks in the United States resulted in 14,481 illnesses with 827 hospitalizations, 20 deaths, and 14 food recalls. Notably, animal-derived products were responsible for many outbreak-related illnesses, particularly poultry products. Turkeys came in first and chicken in third with 609 and 487 illnesses, respectively. Mollusks (41), fish (37), chicken (23), and beef (19) were also the most implicated food categories associated with a significant number of outbreaks (CDC, 2019b). Salmonella was the most common bacterium among the pathogens confirmed as the cause of these outbreaks. Although it came second to Norovirus in terms of illness and number of outbreaks, Salmonella caused a larger proportion of hospitalizations and deaths than all the other single confirmed pathogens.
Salmonella caused the majority of illnesses in 2017, causing 580, 421, and 299 illnesses in turkeys, fruits, and chickens, respectively (CDC, 2017). However, the report listed only Salmonella in fruits as responsible for a greater proportion of hospitalizations. This potentially indicates that infections resulting from the consumption of contaminated poultry are more common but less severe than those from fruits and vegetables. One factor that may affect the difference in their severity is the difference in preparation and consumption of these foods. Poultry products, especially poultry meats, are always consumed after cooking, whereas fruits and vegetables may be consumed raw. This suggests that the frequency of foodborne illness caused by animal products may potentially be reduced by proper preparation before consumption. Although the severity of the infection may be lesser, controlling the presence of the pathogen in the raw products may help lessen the likelihood of illness and lower the welfare and economic cost of foodborne diseases (Scharff, 2012).
Controlling Bacterial Pathogens
Producers and the food industry have strived to address these dynamic hazards. Over the years, they continued to develop more robust control measures to meet this challenge. The ubiquity of these pathogens and their persistence in environmental sources complicate the effort to keep them out of the system and make their complete eradication implausible (Hancock, 2001). However, limiting their presence within the products is a more achievable objective that would significantly reduce the magnitude of the risk associated with foodborne pathogens.
One way to prevent illnesses is by monitoring and removing the circulation of contaminated foods in the market. This is facilitated in part by the authority of the Food and Drug Administration (FDA) and the Food Safety and Inspection Service (FSIS) of the United States Department of Agriculture (USDA) to recall products that are suspected or confirmed to be contaminated with these pathogens. The presence of microbial pathogens like Salmonella in food products had accounted for almost 27% of all product recalls between 2004 and 2013 (Page, 2018). Although recalls may reduce the likelihood of illness, the initial contamination results in the waste of nutritious food products and may incur significant losses for the producers. The extent to which these incidences impact the market has yet to be understood (Van Heerde et al., 2007). However, it has become increasingly difficult to control with the globalization of the food supply chain, improvement in technology for pathogen detection, and changes in regulations and consumer demands (Lyles et al., 2008). Employing interventions during production would thus help prevent the incidence of foodborne illness, enhancing the sustainability of food production by reducing food waste (Gorton and Stasiewicz, 2017).
Historically, interventions targeted to enhance the microbial safety of animal products were conventionally applied during processing. Food safety control measures are currently explored at the pre-harvest stage to alleviate the burden at later stages of production. Implementing interventions at multiple points of the food supply chain creates a network that minimizes the risk of pathogens at the point of consumption. An additional factor to consider in developing effective control measures is the sustainability of the intervention and susceptibility to resistance. For example, a life cycle analysis performed on antimicrobial chemicals like peracetic acid (PAA) for processing beef carcasses noted that PAA had a higher environmental and human health impact than heat-based methods (Li et al., 2018). In recent years, the growing interest in the clean label among consumers and increasing restrictions on the use of antibiotics have led producers to explore interventions that are naturally derived and have greater familiarity with the general public, including probiotics.
Probiotics
Probiotic, a term translating to “for life,” was introduced at the turn of the twentieth century. Elie Metchnikoff proposed that ingestion of microbes could exert beneficial health-promoting effects for humans, particularly regarding the treatment of digestive diseases (Metchnikoff, 1908). In 2001, the Food and Agricultural Organization (FAO) of the United Nations and the World Health Organization (WHO) later defined probiotics as live microorganisms that may confer a health benefit to the host when consumed in adequate amounts (Preidis and Versalovic, 2009). The increased inclination toward preventative healthcare among the general public has contributed to the continued rise of the global market for probiotics.
Consumption of probiotics is not only restricted to humans, as they have also been adapted for use in animal agriculture. Producers supplement probiotics to animals for several reasons, including improving animal wellbeing and growth performance. Their utility as food safety interventions for reducing pathogens has been explored more recently. Several authors have compiled a list of characteristics that microorganisms should possess to be defined as a probiotic. The proposed criteria include (Dunne et al., 1999; Simmering and Blaut, 2001; Patterson and Burkholder, 2003):
1. Originate from the host
2. Display no pathogenic characteristics
3. Retain their viability and activity in delivery vehicles and other technological processes
4. Capable of withstanding gastric acid and bile
5. Adhere to intestinal epithelial tissue
6. Persist in the gastrointestinal tract, albeit for short periods
7. Produce antimicrobial substances
8. Modulate the immune system
9. Influence metabolic and microbial activities
In the context of animal agriculture, the term “direct-fed microbial” products (DFMs) are also used to refer to certain probiotic products (FDA, 1995). By contrast, the Bovine Alliance on Management and Nutrition argues that the two terms can be used interchangeably (Quigley, 2011). In their definition, probiotics are “live microbial feed supplement which beneficially affects the host by improving its intestinal microbial balance” (Heyman and Ménard, 2002).
Several microbes have also been utilized in food processes beyond their use in probiotics. Food biotechnologists are exploring the potential application of microbes to shorten the ingredients list, namely through in situ transformation of substrates within a food matrix into active molecules that contribute a specific function. Although many have been explored for their longstanding applications, such as in the production of fermented foods, its utility in non-fermented foods is also gaining interest (Perpetuini et al., 2021).
With the growing use of these live microorganisms, regulations have been put into place that may make it difficult for certain probiotics to obtain approval. Several countries have established lists of microorganisms allowed for use as probiotics. In 1995, the FDA issued Compliance Policy Guidelines (Sec. 689.100 Direct-Fed Microbial Products) outlining the specific requirements that a probiotic product must meet, including their labeling and claims made about the product (FDA, 1995). A key component to the guideline is the use of microorganisms listed under Section 36 of the official publication of the Association of American Feed Control Officials (AAFCO). If one or more microorganisms in the product are not on the list, it would be considered a food additive and must abide by the food additive regulation. Those containing only microorganisms listed under the AAFCO official publication without any therapeutic or function claims will be regulated as food and would not typically require FDA regulatory attention. Hence, products containing microorganisms not currently on the list would require additional investment to market. The list of microorganisms that have been reviewed and presented no safety concerns regarding use in DFM products by the FDA include many under the Lactobacillus species (AAFCO, 2018). Their familiarity with both regulatory agencies and the public may also lend to the potential utility of lactobacilli for the production of foods with clean labels.
Lactobacillus
Taxonomy
Lactobacilli are fastidious non-spore-forming Gram-positive rods. Bacteria within this genus are strictly fermentative and can tolerate or thrive under acidic conditions. They are also aerotolerant or anaerobic (Tannock, 2004). Their complex nutritional requirements impact their preferred habitats, as lactobacilli often populate nutrient-rich niches associated with plants and foods and in the bodies of humans and animals. The Lactobacillus genus within the Firmicutes phylum possesses a phylogenetic and metabolic diversity commonly observed among bacterial families. Most genera of lactic acid bacteria employ either glycolysis (homolactic metabolism) or utilize the phosphoketolase pathway (heterolactic metabolism) to metabolize glucose (Gänzle, 2015). By contrast, the Lactobacillus genus includes species that use one or the other, allowing for further differentiation among them. Homofermentative lactobacilli produce mainly lactic acid (>85%), whereas heterofermentative lactobacilli produce lactic acid, carbon dioxide, ethanol, and/or acetic acid in similar amounts (Tannock, 2004).
In addition to classification based on their metabolic repertoire, modern molecular tools have further categorized the genera into 24 phylogenetic groups, including members of the Pediococcus species. The diversity of phylogenetic groups within the genus has served as justification for their formal recognition as a separate genus, as illustrated with Pediococcus. Despite justification based on taxonomic considerations, the term “Lactobacillus” is deeply rooted within the food and health-related industries, in regulations, and among the public. Therefore, researchers have proposed using the term Lactobacillus sensu lato to include pediococci or the Lactobacillus Genus Complex when related genera such as Leuconostoc are included (Zheng et al., 2015).
The classification and nomenclature of this genus have changed considerably since it was first described in 1901 by Beijerinck. The interest in bacteria of this genus is partly exemplified by the number of species identified throughout the years. The number of species and subspecies in this genus had grown from 55 in 1986 to 103 in 2003 and 152 by 2005 (Bernardeau et al., 2006). As of 2015, more than 200 species and subspecies of Lactobacillus have been formally recognized (Sun et al., 2015). Whole-genome sequencing methods further demonstrated the heterogeneity of the Lactobacillus genus, leading to the proposed reclassification into 25 novel genera, which included the emended Lactobacillus genus (Zheng et al., 2020). The proposed genera names of the lactobacilli discussed in the review and select species reclassified under them are summarized in Table 2.
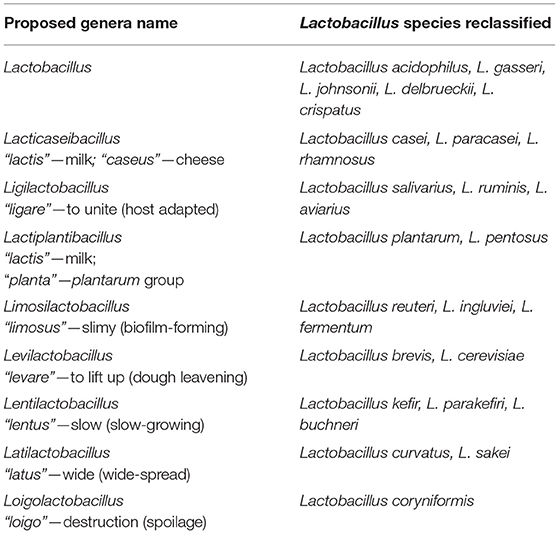
Table 2. Summary of selected genera proposed, and the Lactobacillus species reclassified under them.
Historical Perspectives and Applications
Organisms under the genus Lactobacillus have played a historical role in various applications, including food production and biotechnology. They were among the first organisms used in food processing and preservation by inhibiting other microorganisms that cause spoilage or illness (Bernardeau et al., 2006). Additionally, foods that require lactic acid fermentation often involve lactobacilli. These include applications as starter cultures in sourdough bread, fermented plants (kimchi, sauerkraut, and pickles), fermented meat (salami), and dairy products (yogurt, kefir, cheese). The use of lactobacilli in food fermentation predates even bacteriology, and their function in this application has since been studied extensively (Aryana and Olson, 2017).
Furthermore, novel applications of these organisms have also been explored for their potential applications for clean label strategies. Lactic acid bacteria, including lactobacilli, can produce a variety of exopolysaccharides that possess various bioactivity. For example, in situ production of exopolysaccharides from sucrose by certain cereal-associated lactobacilli have the potential to replace the use of additives such as hydrocolloids in bread (Tieking et al., 2003). Lactic acid bacteria have also served as an essential component of starter cultures in products such as fermented meats. They contribute many functions, from reducing meat pH to the development of flavor. More recently, their utility in reducing nitrate to nitrites has been explored for meat production. Several lactobacilli have exhibited nitrate-reducing capabilities, including Lactobacillus plantarum, L. farciminis, and L. coryniformis from spinach extract (Kim et al., 2017).
Lactobacilli can also inhabit vertebrate hosts, such as humans and food-producing animals, and have a particular affinity for organs that store food like the forestomach or crop (Duar et al., 2017). Although less is known about the lactobacilli found in live hosts than those involved in food fermentation, many have explored and studied their functionality as probiotics (Tannock, 2004).
The historical applications of bacteria within this genus have led to its acceptance as “Generally Recognized as Safe” (GRAS) by the FDA and a Qualified Presumption of Safety by the European Food Safety Authority (EFSA) (Sun et al., 2015). Their utility, approval by national and international regulations, and widespread recognition among consumers have contributed to its dominance within the probiotic market. Many commercially available probiotics for humans and animals today include lactobacilli. Because of these factors, it is no surprise that studies investigating the lactobacillus species have continued to this day, including exploration of their utility against foodborne illness-causing pathogens.
Food Safety Applications in Animal Production
The genus Lactobacillus is exceptional not only in diversity from a taxonomic perspective but also in its applications. Many factors contribute to their role for various purposes, such as fermentation, preservation, and contribution to food texture. This section focuses on the traits with which lactobacilli can be utilized to improve the food safety of animal-derived products. In this regard, factors that do not directly impact the microbial safety of animal production are beyond the scope of this review. Extensive reviews of the potential applications of lactobacilli and their metabolites for other applications related to clean label food production are available (Papadochristopoulos et al., 2021; Perpetuini et al., 2021; Vargas and Simsek, 2021).
The mechanisms behind lactobacilli's antimicrobial activity against bacterial pathogens appear to be multifactorial, and each Lactobacillus strain may have unique properties. Several significant mechanisms of action that have been studied against bacterial pathogens include competitive exclusion, enhancement of epithelial barrier function, modulation of the immune system, and production of antimicrobial metabolites (Figure 1).
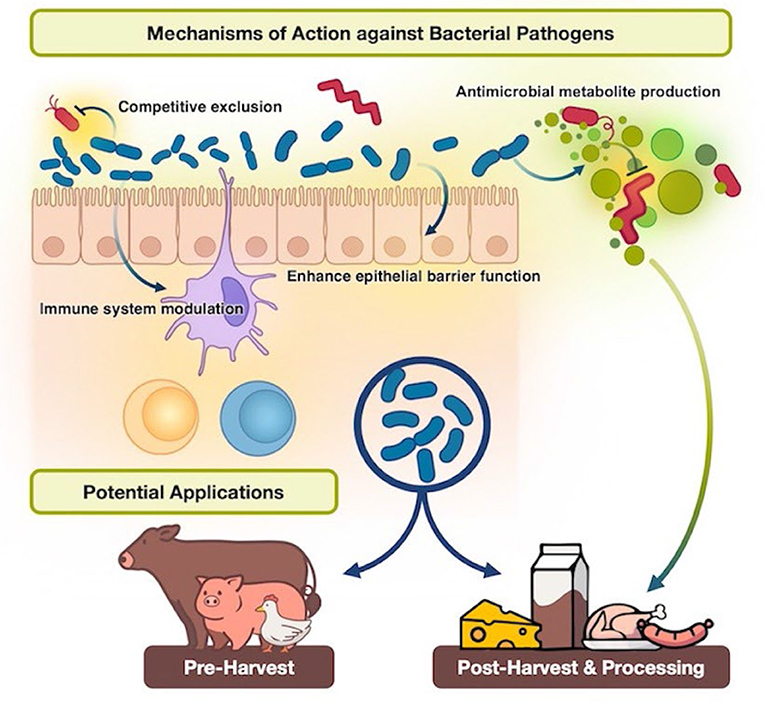
Figure 1. The mechanisms behind the antimicrobial activity of lactobacilli against bacterial pathogens.
Mechanisms of Action Against Bacterial Pathogens
Competitive Exclusion
Animals are typically exposed to microorganisms from their parents and environments at birth, which may confer protection against pathogenic organisms. Many attribute the concept behind competitive exclusion to the work of Nurmi and Rantala, who administered the intestinal microflora from healthy adult broilers to newly hatched chicks as an intervention against S. enterica serovar Infantis (Nurmi and Rantala, 1973). This protection involves competition for attachment sites and nutrients between the bacterial populations in the gastrointestinal tract. Therefore, inoculation of young animals with beneficial microbes would allow those organisms to establish in the intestinal tract before exposure to other microorganisms, thus preventing potential colonization with pathogenic microorganisms. Studies on the competitive exclusion of pathogens by lactobacilli dates back to 1985, where Reid and associates explored the use of autochthonous Lactobacillus casei against uropathogens in rats (Reid et al., 1985). The idea has since been explored to more extraordinary lengths, and many other lactobacilli strains were found effective in preventing colonization and invasion of pathogenic bacteria in vitro. Lactobacillus rhamnosus DR20 and Lactobacillus acidophilus HN017 were effective against E. coli O15:H7 colonization and invasion in vitro (Gopal et al., 2001). Lactobacillus crispatus ZJ001 inhibited the adhesion of E. coli O157:H7 and S. enterica serovar Typhimurium, and the surface layer proteins contributed to this inhibition (Chen et al., 2007).
Enhance Epithelial Barrier Function
The gastrointestinal tract represents one of the largest interfaces where the external environment interacts with the body. In addition to its role in nutrient digestion, it also serves as a critical physical barrier separating commensal and pathogenic microbes from the underlying cells. Hence, the integrity of the epithelial layer is important to protect the host against pathogens. Disruption to the epithelial barrier may lead to loss of immune tolerance to the microflora. It could also cause inflammatory responses that may facilitate pathogen colonization and infection. In this regard, several probiotic organisms have demonstrated the ability to enhance the epithelial barrier function through several approaches. For example, Lactobacillus casei prevented cytokine-induced epithelial barrier dysfunction in human intestinal epithelial cells (Eun et al., 2011). Similarly, Lactobacillus acidophilus ATCC 4356 enhanced intestinal epithelial barrier function and prevented invasion of entero-invasive E. coli in vitro (Resta-Lenert, 2003). Lactobacillus casei strain DN114 001 was also found to reduce paracellular permeability due to entero-pathogenic E. coli (Ingrassia et al., 2005; Parassol et al., 2005). Live Lactobacillus rhamnosus strain GG was found to prevent morphological changes to the epithelial cell barrier due to entero-hemorrhagic E. coli (Parassol et al., 2005). Additionally, Lactobacillus plantarum 299v was found to protect against E. coli-induced increase in intestinal permeability of rats (Mangell et al., 2002). Colonization of Lactobacillus reuteri ATCC 55730 was found to influence local immune cell populations and contribute to maintaining the health of healthy recipients' gastrointestinal tract (Valeur et al., 2004). Similarly, Lactobacillus frumenti supplemented by oral gavage to early-weaned piglets improved the intestinal epithelial barrier function (Hu et al., 2018).
Modulation of Immune System
Lactobacilli may exert beneficial effects in reducing pathogenic organisms within the host through immunomodulation. This may include up-regulation of anti-inflammatory factors, suppression of pro-inflammatory factors, as well as overall enhancement of immunity (Haller, 2000; Lin et al., 2008). Several studies have also explored the immunomodulation properties of lactobacilli, including work by Koenen and associates demonstrating that contact with lactobacilli resulted in a proliferation of spleen lymphocytes and induction of humoral response to model antigens in vitro (Koenen et al., 2004). Mice fed Lactobacillus rhamnosus strain HN001 displayed a lower mortality rate following S. enterica serovar Typhimurium challenge and a reduced rate of translocation of S. enterica serovar Typhimurium to visceral organs like the spleen and liver. The lactobacilli-fed mice also exhibited less severe pathophysiological impact following the challenge than the control group; the effect was attributed to the stimulation of the immune system as determined by higher titers of anti-Salmonella antibodies in the serum and intestinal tract (Gill et al., 2001). A study involving IL-10-deficient mice found a decrease in colonic Lactobacillus levels and increases in other organisms, which were associated with the development of colitis. However, repopulation with Lactobacillus reuteri alleviated the defect in bacterial colonization and thus prevented the development of colitis (Madsen et al., 1999).
The way by which lactobacilli exert their effects on the intestinal tract is not restricted to their ability to colonize. Lactobacilli which do not colonize their hosts, indirectly exert their effect either through their transient presence or by modulating the existing microbial community. Metabolites secreted by Lactobacillus acidophilus ATCC 53103 were found to protect the intestinal barrier and preserve tight junction permeability in Caco-2 cells (Guo et al., 2017). Another study explored the lysate of Lactobacillus casei DN-114 and found that it could improve gut barrier function and modulate mucosal immune response even in the absence of live bacteria (Zakostelska et al., 2011). Furthermore, Lactobacillus reuteri ATCC PTA 6475 converted L-histidine into histamine, which suppressed the production of pro-inflammatory cytokines (Thomas et al., 2012).
Antimicrobial Metabolite Production
In addition to lactobacilli's competitive exclusion and mucosal immunity stimulation, they were also found to produce antimicrobial substances that contributed to their mechanism of action against pathogens. Competition between microorganisms within a habitat as diversely populated as the gastrointestinal tract has driven the development of antimicrobial metabolite production to aid in an organism's survival. These characteristics may also be utilized to help control the presence of pathogenic microorganisms in animal production. Acetic acid, lactic acid, hydrogen peroxide, and antimicrobial compounds are among the metabolites produced by lactobacilli that have demonstrated inhibitory activity against pathogens.
Hydrogen Peroxide
Hydrogen peroxide (H2O2) is a common antibacterial the FDA has affirmed as generally recognized as safe for specific use in food production (CFR 21 § 184.1) (FDA, 2021). Production of H2O2 by bacteria is also a documented mechanism to inhibit the growth of other organisms. That is why H2O2-producing lactobacilli have been sought by scientists for their potential antimicrobial properties (Berthier, 1993). Due to the lack of heme, lactobacilli do not utilize the cytochrome system for terminal oxidation. The cytochrome system produces water from the reduction of oxygen. Instead, they use flavoproteins that generally convert oxygen to H2O2. Furthermore, in the absence of heme protein catalase, the generated H2O2 accumulates to amounts that can exert antagonistic activity against other microorganisms (Eschenbach et al., 1989). Furthermore, lactobacilli were also found more resistant to H2O2 compared to pathogens. In the absence of catalase, it was hypothesized that the greater resistance was due to their development of a mechanism to utilize H2O2 using riboflavin (Seeley and Del Rio-Estrada, 1951; Wheater et al., 1952).
Production of H2O2 is strain-specific and is also affected by environmental factors. The factors affecting the formation of H2O2 by lactobacilli include aeration, carbohydrate source, storage conditions, and the Lactobacillus strain itself. Aerated cultures were found to have between two to three times greater concentrations of H2O2 compared to unaerated cultures of Lactobacillus delbrueckii subspecies bulgaricus (PMID: 10618234). Dahiya and Speck (1968) observed the most significant accumulation of H2O2 with Lactobacillus lactis in neutral pH at 5°C when dextrose was the carbohydrate source. Starvation of Lactobacillus delbrueckii subspecies lactis I also impacted H2O2 production. The results also indicated that lactate oxidase was involved in the production of H2O2 from D-lactate (Villegas and Gilliland, 2006). Static incubation of Lactobacillus acidophilus strains yielded smaller amounts of H2O2 than those incubated with continuous shaking (Collins and Aramaki, 1980). These studies also indicated that different strains of the same Lactobacillus species produce varying amounts of these metabolites. Brashears et al. (1998) discovered that H2O2 production was the primary mechanism by which Lactobacillus lactis exhibited antagonistic activity against E. coli O157:H7 when co-cultured in Tryptic Soy broth at 7°C.
Organic Acids
Lactobacilli are part of a collective group commonly referred to as lactic acid bacteria due to their formation of lactic acid from carbohydrate metabolism. Although bacteria within the Lactobacillus species are known for lactic acid production, they may also produce other acids among the metabolites generated. Production of these acidic metabolites that lower the pH of the environment is part of the mechanism by which some lactobacilli inhibit the growth and survival of other bacteria, including pathogens. Acidification is a well-characterized method for food preservation used for animal products such as fermented sausages (Ammor and Mayo, 2007).
As the name implies, lactic acid is the primary acid associated with lactobacilli, and it is an abundant, naturally occurring organic acid. In addition to its natural presence in foods as a product of in situ microbial fermentation, lactic acid is also a metabolic intermediate for most living organisms, including humans (Datta and Henry, 2006). Lactic acid is also currently applied as a rinsing agent for the processing of beef, pork, and chicken carcasses. The antimicrobial property of lactic acid is generally attributed to the acidification of the cytoplasm that affects the transmembrane pH gradient, decreasing the amount of available energy for cells to grow. Acidification of the medium was found to be the mechanism by which Lactobacillus casei strain GG inhibited S. enterica serovar Typhimurium as the inhibition was found to be pH-dependent (Hudault et al., 1997; Lehto and Salminen, 1997). Similarly, pH adjustment of Lactobacillus culture supernatants reduced the magnitude by which they inhibited Campylobacter jejuni. Among the four strains tested, Lactobacillus crispatus JCM 5810 was the most effective against Campylobacter jejuni in vitro. It was also found to produce more acid than Lactobacillus acidophilus and Lactobacillus gallinarum (Neal-McKinney et al., 2012).
However, different strains of lactobacilli produce different metabolites at varying concentrations making it challenging to distinguish the role of individual compounds. It is reported that multiple Lactobacillus strains exerted antimicrobial effects on S. enterica serovar Typhimurium that is strain-specific and could be attributed to other substances, not lactic acid (Fayol-Messaoudi et al., 2005). Although the extent to which lactic acid contributes to the antibacterial activity of lactobacilli varies between strains, its presence may contribute to a synergistic action with other compounds that the bacteria produce. For example, lactic acid impacts the integrity of E. coli O157:H7 and S. enterica serovar Typhimurium outer membrane, which sensitizes the pathogens to detergents and lysozyme (Alakomi et al., 2005). Against Salmonella, it was observed that lactic acid caused the release of substantial portions of lipopolysaccharide on the outer membrane. Furthermore, the impact of lactic acid was stronger on the pathogen than hydrochloric acid at the same pH (Midolo et al., 1995). Therefore, lactic acid may increase the potency of other antimicrobial substances. Particularly those unable to effectively penetrate the outer membrane of Gram-negative bacteria due to their size or lipophilic nature.
Antimicrobial Substances
The catalog of lactobacilli-produced antimicrobial substances effective against pathogens continues to grow as more have been studied. These include a heterogeneous group of ribosome-synthesized bactericidal peptides collectively referred to as bacteriocins. The discovery and successful commercialization of nisin is part of the catalysts that led to the resurgence in investigations of these proteinaceous antimicrobial compounds (Nes et al., 1996). They are typically small, heat-stable, and may have a broad or narrow spectrum of activity against other bacteria. The producer is often immune to their bacteriocin, though it typically targets closely related organisms and occupies similar ecological niches as the producing organism (Cotter et al., 2005). Bacteriocins produced by lactobacilli can be found in one of four major classes of antimicrobial proteins.
Class I consists of small gene-encoded peptides that contain modified amino acids lanthionine or 3-methyl-lanthionine. Hence they are often referred to as lantibiotics (Jung, 1991). These residues are responsible for the unique structural characteristics of lantibiotics. Additionally, lantibiotics often undergo extensive post-translational modification, such as the substitution of D-alanine for L-serine that further add unique residues to their structure (Xie and van der Donk, 2004). This class also includes the widely utilized food preservative nisin produced by Lactococcus lactis. Though this class is not prominent among Lactobacillus species, it is produced by several strains, including Lactobacillus sake L45 (Mørtvedt et al., 1991).
The Class II bacteriocins are more common and mainly grouped based on the absence of lanthionine in their structure. They are typically small, heat-stable, hydrophobic peptides that undergo minimal post-translational modification. They also possess a heterogeneity that allows further classification into sub-groups. However, these categorizations have changed over time with the accumulation of knowledge regarding bacteriocins and their functionality (Nes et al., 1996). Historically, there are four subclasses in which bacteriocins in Class II may be sorted, including subclasses A through D. Subclass A are pediocin-like single peptides with a characteristic amino acid sequence motif near its N-terminus (Eijsink et al., 1998). They have demonstrated a narrow spectrum of activity and high specificity against Listeria monocytogenes (Montville and Chen, 1998). Subclass B is known as the two-peptide bacteriocin because it typically requires the interaction of two peptides for its total activity (Garneau et al., 2002). Subclass C, formerly classified as Class V bacteriocins, are grouped based on their cyclic structure (Maqueda et al., 2004). Several lactobacilli, including Lactobacillus gasseri LA39 and Lactobacillus reuteri LA6, have demonstrated the production of cyclic bacteriocins (Kawai et al., 2004). The remaining Class II bacteriocins that fit neither subclasses mentioned above are then categorized in subclass D. This miscellaneous subclass includes non-pediocin, single linear peptides that have in some cases been further grouped based on their leader sequences (Diep and Nes, 2002). More recently, the fifth subclass of Class II bacteriocins was proposed, including peptides rich in serine residues at its carboxy-terminal region and with non-ribosomal siderophore-type post-translational modification (Cotter et al., 2013).
Most bacteriocins in Class II act on the bacterial membrane, resulting in membrane permeability in the target bacteria. The class IIa bacteriocin produced by Lactobacillus salivarius NRRL B-30514 isolated from broiler ceca demonstrated in vitro inhibition of Campylobacter jejuni and reduced colonization in chickens (Stern et al., 2006). Similarly, the broad-spectrum class II bacteriocin, Abp118, produced by Lactobacillus salivarius UCC118, was found to be the primary mechanism by which the probiotic exerted its antimicrobial effect against Listeria monocytogenes infection in mice (Corr et al., 2007). They also observed protection against S. enterica serovar Typhimurium UK1, a pathogen that is naturally resistant to the bacteriocin. This suggests that Lactobacillus salivarius UCC118 may also confer protection against pathogens through a mode independent of bacteriocin production and possibly either through competitive exclusion or immunomodulatory mechanisms. Although Abp118 had only demonstrated activity against closely related bacteria in vitro, supplementation with Lactobacillus salivarius UCC118 had affected the Gram-negative microorganisms in the murine and swine microbiota (Riboulet-Bisson et al., 2012).
Class III bacteriocins typically have higher molecular weights (>30 kDa) and are more sensitive to heat than other classes. Cotter et al. proposed that these proteins be called “bacteriolysins” instead of bacteriocins. They possess a distinct mechanism of action through catalyzing cell-wall hydrolysis leading to lysis of sensitive cells (Cotter et al., 2005). However, this definition would exclude that large molecular weight, non-lytic, and heat-labile bacteriocins such as Helveticin J produced by Lactobacillus helveticus 481 (Joerger and Klaenhammer, 1990).
Klaenhammer's class IV bacteriocins are associated with non-proteinaceous, lipid, or carbohydrate moieties that contribute to their activity (Klaenhammer, 1993). Similar to Class III bacteriocins, Cotter et al. (2005) proposed that this classification be excluded due to the lack of convincing evidence of those within this class. However, Stepper and associates countered this point based on their demonstration of glycopeptide bacteriocin Glycocin F produced by Lactobacillus plantarum KW30 (Stepper et al., 2011). The first Lactobacillus bacteriocin to be purified and characterized was the macromolecular lipo-carbohydrate protein produced by Lactobacillus fermenti 466 (De Klerk, 1967; De Klerk and Smit, 1967). In contrast, restructuring the classes altogether has been proposed, dividing Class III as lysins and non-lytic proteins and creating a new Class IV in which the cyclic bacteriocins of Class IIc would be categorized (Heng et al., 2007).
Pre-Harvest Applications
Several major foodborne pathogens like Salmonella, Campylobacter jejuni, and E. coli O157:H7 share common epidemiological characteristics, including initiation into the food cycle through ingestion of contaminated feed or water by a food-producing animal, leading to the pathogens' colonization within their gastrointestinal tract. Once colonized, the transmission of these pathogens through fecal shedding or gastrointestinal contents may contaminate the environment. This further exacerbates the challenge by potentially propagating the initial pathogen population, increasing the risk of foodborne illness. Furthermore, the presence of these pathogens in animal production systems may also result in contamination of other food products where byproducts of animal agriculture are utilized. For example, cattle were named the potential source of the E. coli O157:H7 implicated in the 2018 multistate outbreak involving romaine lettuce (FDA, 2018). Therefore, developing interventions that target pathogens in live animals is critical to enhancing food safety.
Evidence supporting the antimicrobial properties by which lactobacilli inhibit foodborne bacterial pathogens has continued to grow since their mechanisms of action continue to be elucidated. Likewise, the inventory of products that have incorporated lactobacilli for use in animal agriculture has grown over the past few decades. Many products that utilize live microorganisms can be grouped into two categories based on their intended functions. The first and more predominant category includes products that broadly target the improvement or maintenance of animal health without making specific claims regarding their effect on particular pathogens of concern to food safety. These products are often fed to livestock and poultry to improve their performance. Although improvements to animal health theoretically would make them less susceptible to colonization with pathogens, many of the foodborne pathogens of concern to humans are carried asymptomatically in the intestinal tract of livestock and poultry. By contrast, the other category of products establishes claims based on direct and potentially quantifiable impact on the presence of these pathogens within the animals (Joerger and Ganguly, 2017). The efficacy of lactobacilli-based products against foodborne-illness-causing pathogens has been tested in several food-producing animals at the pre-harvest production stage. The studies suggest that not only are the effects of Lactobacillus-containing products different based on the strain of lactobacilli used but that one strain may also vary in its efficacy against different pathogenic bacteria. Utilizing lactobacilli-based strategies at the pre-harvest stage of production would help fortify the interventions applied during processing by limiting the initial pathogen load. Alleviating this burden would allow post-harvest interventions to meet the clean label requirements without increasing food safety risks.
Poultry
The earliest investigation into the use of microbiota interventions for food safety can be dated back to the 1950s, when Milner and Schaffer observed resistance to Salmonella infection in chicks provided mature microbiota (Milner and Shaffer, 1952). However, the commercialization of microbial preparations was not available until the 1970s (Nurmi and Rantala, 1973). Since then, a growing body of research has investigated the use of a variety of lactobacilli against different pathogens. Lactobacillus acidophilus given to gnotobiotic chicks effectively reduced fecal shedding and mortality due to E. coli (Watkins et al., 1982). A single oral dose of Lactobacillus johnsonii FI9785 in specific pathogen-free chicks reduced Clostridium perfringens colonization and delayed invasion of organs by E. coli but were not effective against S. enterica serovar Enteritidis (La Ragione et al., 2004). By contrast, Lactobacillus salivarius CTC2197 given in conjunction with S. enterica serovar Enteritidis by oral gavage into the proventriculus of day-old chicks resulted in complete clearance of the pathogen after 21 days (Pascual et al., 1999). The use of Lactobacillus salivarius and other organisms in competitive exclusion products demonstrated efficacy in reducing Salmonella carriage in challenged chicks by up to 2.5 log CFU/cecal content (Zhang et al., 2007). A cocktail of 11 Lactobacillus isolates, including 3 Lactobacillus fermentum, 2 Lactobacillus casei, 2 Lactobacillus cellobiosus, and 1 Lactobacillus helveticus, reduced S. enterica serovar Enteritidis populations in the ceca of broiler chicks (Wolfend et al., 2007). The same probiotic mix administered 1 h after the challenge with either S. enterica serovar Enteritidis, S. enterica serovar Typhimurium, or S. enterica serovar Heidelberg was also found to reduce their recovery from the day of hatch broiler chicks and turkey poults (Higgins et al., 2007; Menconi et al., 2011). A systematic review of competitive exclusion products found that products containing lactobacilli reduced the prevalence of Salmonella over time and were effective in lowering cecal Salmonella concentration in broiler chickens (Kerr et al., 2013). More recently, a study investigating the use of kefir-originated Lactobacillus kefiranofaciens DN1 also observed a reduction in S. enterica serovar Enteritidis colonization in chickens (Bae et al., 2020). A study investigating four homofermentative Lactobacillus strains found that Lactobacillus crispatus yielded the greatest reduction in the number of chicks colonized with Campylobacter jejuni and lowered the microbial load (Neal-McKinney et al., 2012). The use of avian-specific probiotics containing Lactobacillus acidophilus resulted in a reduction in Campylobacter jejuni shedding and colonization in market age broilers (Morishita et al., 1997). However, the efficacy of lactobacilli against Campylobacter jejuni is not limited to the use of live cultures. Supplementing feed with purified bacteriocin produced by broiler chicken-derived Lactobacillus salivarius also reduced Campylobacter jejuni colonization in chickens (Stern et al., 2006).
Swine
Lactobacillus represents one of the significant bacterial genus reported in the porcine gastrointestinal tract (Pedersen and Tannock, 1989; Valeriano et al., 2017). The efficacy of lactobacilli-based treatments has also been explored in swine models, though most studies measured their impact based on performance metrics. Several more general studies have demonstrated that supplementation of Lactobacillus plantarum 4.1 and Lactobacillus reuteri 3S7 had decreased the Enterobacteriaceae population in pigs (De Angelis et al., 2007). Oral supplementation of Lactobacillus fermentum I5007 in piglets reduced Clostridium populations in their gastrointestinal tracts (Liu et al., 2014). Weaned piglets fed Lactobacillus rhamnosus GG was observed to reduce inflammation due to S. enterica serovar Infantis infection (Yang et al., 2017). Feed fermented by Lactobacillus zeae exhibited a protective effect against Salmonella infection in pigs (Yin et al., 2014). Supplementation of weaned piglets with cultures that include Lactobacillus murinus, Lactobacillus salivarius, and Lactobacillus pentosus was found to reduce fecal S. enterica serovar Typhimurium (Casey et al., 2007). A Lactobacillus-based probiotic consisting of Lactobacillus reuteri was found to reduce S. enterica serovar Typhimurium in the feces of challenged piglets but had negligible impact on E. coli numbers (Sonia et al., 2014). In contrast, Lactobacillus sobrius DSM 16698 was found to reduce enterotoxigenic E. coli F4 in ileal lumen samples of weaned piglets (Konstantinov et al., 2008). A cocktail of Lactobacillus gasseri, Lactobacillus reuteri, Lactobacillus acidophilus, and Lactobacillus fermentum was found to enhance resistance against E. coli in weaned piglets (Huang et al., 2004).
Ruminants
Escherichia coli O157:H7 is a pathogen of significant concern in beef production following the outbreak it caused in the early 1990s (Bell, 1994). Hence, the use of lactobacilli-containing products to improve the safety of cattle-derived products often explores their utility against this pathogen. Among the lactobacilli of commercial use, Lactobacillus acidophilus is a prominent strain that has been extensively studied, including its effect on pathogen carriage in livestock. A Lactobacillus-based DFM product consisting of a combination of Lactobacillus acidophilus NP51 and Lactobacillus crispatus was found to reduce the prevalence of E. coli O157:H7 in cattle (Brashears et al., 2003). Daily supplementation of 9 Log CFU Lactobacillus acidophilus NP51 per steer was also found to reduce E. coli O157 prevalence in fecal and hide samples (Elam et al., 2003; Russell and Axtell, 2005). Similarly, Lema et al. (2001) found a reduction in fecal shedding of E. coli O157:H7 was observed in sheep fed a dried fermentation product consisting of a mixture of Lactobacillus acidophilus, Lactobacillus casei, Lactobacillus fermentum, Lactobacillus plantarum, and Streptococcus faecium (Lema et al., 2001). Lactobacillus-containing products may also vary in their efficacy against different pathogenic bacteria. For example, it may reduce fecal shedding of E. coli O157:H7 but have limited effects on Salmonella (Tabe et al., 2008). However, these effects are not always consistent as a reduction in Salmonella shedding has been reported (Stephens et al., 2007). Using autochthonous Lactobacillus casei and Lactobacillus salivarius of bovine origin was also observed to reduce the severity of S. enterica serovar Dublin infection in calves (Frizzo et al., 2012).
Post-Harvest or Processing Applications
Monitoring foodborne illness-causing pathogens is especially critical at the post-harvest stages of production following the products' departure from the farm and moving closer to the consumers. The products are either prepared for further processing, packaged, or sent to retail stores or restaurants at this phase. Animal agriculture and the products it generates make up a major industry contributing to the United States' economy, and multiple Federal agencies closely oversee the production processes. For example, the USDA's FSIS inspects production facilities and routinely performs evaluations to ensure compliance with the performance standards designated for specific pathogens of interest. For this reason, many facilities had employed post-harvest interventions before pre-harvest interventions were explored. Furthermore, investigations into effective interventions have also been incentivized by the implementation of the Pathogen Reduction: Hazard Analysis and Critical Control Point PR:HACCP Systems rule in 1996 (9 CFR Part 304) (FSIS, 1996). The ruling emphasized the importance of controlling foodborne illness-causing pathogens at all stages in the farm-to-table continuum because introduction and propagation can happen at any point (Hogue et al., 1998). Interventions designated for this purpose include physical approaches such as using High-Pressure Processing (HPP) or chemicals such as organic acids. The use of lactic acid bacteria and their metabolites have been explored for use against spoilage, as exemplified by the commercial success of nisin (Delves-Broughton et al., 1996). However, investigations on their applications against foodborne pathogens are scarce in comparison.
Lactobacilli had historical applications in food preservation by fermentation, which has been a part of human cultural history all over the globe. It is the root of food biotechnology and was used to elongate the shelf life and enhance the safety of many foodstuffs, including animal-derived products such as milk and meat. However, several strains of lactobacilli may be involved in spoilage and the development of undesired sensory properties in animal-derived food products. For instance, the presence of Lactobacillus plantarum was found to accelerate the spoilage of vacuum-packaged beef (Egan and Shay, 1982). Similarly, Lactobacillus alimentarius was also found to contribute to spoilage of marinated herring by gas production (Lyhs et al., 2001). However, different lactobacilli play different roles for the same category of products. For example, some lactobacilli are important in cheese ripening, whereas others may cause defects (Khalid and Marth, 1990). Exploration of lactobacilli isolated from Danbo cheese found that Lactobacillus paracasei strains were beneficial for cheese flavor, whereas Lactobacillus plantarum introduced off-flavors (Antonsson, 2003). Lactobacillus plantarum was also considered a contaminant in the production of Cheddar cheese due to their production of diacetyl (Keenan and Lindsay, 1968). Therefore, careful consideration of the specific desired properties in a food product is necessary when deciding on which lactobacilli or the myriad of metabolites they produce to select.
The earliest description of the preservation of foods through the antagonistic growth of microorganisms was discussed several decades ago (Hurst, 1972). Lactic acid bacteria and their bacteriocins in products like milk and vacuum packaged meats were discussed. This was later termed “biological preservation” or “biopreservation” to distinguish the use of naturally occurring metabolites from synthetic, chemical preservatives (Stiles, 1996). There are multiple routes by which an organism can be utilized for biopreservation. The first is the injection of live protective bacterial cultures into the food product for the purpose of competitive exclusion, potentially by in situ production of inhibitory metabolites. Alternatively, they could forgo the bacterial cultures and add the antagonistic substances alone, either purified or within the spent culture medium in which the bacteria were cultivated. Several lactobacilli have been explored in this regard.
Live Lactobacillus Culture
Lactobacilli have been associated with a variety of animal-derived food products. Lactobacillus delbrueckii subspecies bulgaricus and Lactobacillus helveticus are the prominent strains in Swiss-type cheese. Lactobacillus bulgaricus is similarly crucial in yogurt production, whereas Lactobacillus acidophilus is known in acidophilus milk. Lactobacillus sake and Lactobacillus curvatus can grow under refrigerated conditions and thus are often isolated from raw and fermented meat. In such cases, the growth of the lactobacilli and its metabolites during fermentation may help preserve and protect the products. Several investigations into the use of live lactobacilli cultures for biopreservation have observed their antimicrobial potential against several foodborne pathogens in foods. For example, the addition of Lactobacillus sakei 2a in cheese spread was found to inhibit Listeria monocytogenes at 4°C, and an even more significant reduction to below detection limits was observed at 15°C (Martinez et al., 2015). The addition of Lactobacillus lactis cells to raw chicken breast meat was found to reduce the survival of E. coli O157:H7 during storage at 5°C (Brashears et al., 1998). Similarly, the use of Lactobacillus fermentum ACA-DC179 was shown to be effective against S. enterica serovar Enteritidis in raw chicken meat (Maragkoudakis et al., 2009). Another example involving lactocin-producing Lactobacillus curvatus CRL705, and Lactobacillus sake was found effective against Listeria in vacuum-packaged beef and cured raw pork, respectively (Schillinger et al., 1991; Castellano et al., 2010). Lactobacillus sakei TH1 was also effective as a protective culture in cooked ham and servelat sausage (Norwegian non-fermented cooked sausage) (Bredholt et al., 2001). Furthermore, studies have also demonstrated that the antimicrobial activity of certain bacteriocin-producing lactobacilli differs between the product in which it was applied and that combination of different strains can enhance their potential. For example, bacteriocin-producing Lactobacillus sakei CWBI-B1365 and Lactobacillus curvatus CWBI-B28 effectively inhibited Listeria monocytogenes in raw beef, and could only impede the pathogen in poultry meat when both strains were combined (Dortu et al., 2008). Furthermore, the availability of the substrates within the product that the lactobacilli could utilize also influences their activity against the pathogens. For example, the meat-derived Lactobacillus bavaricus MN was also found to inhibit Listeria monocytogenes during refrigerated storage in vacuum packaged beef that was minimally heat-treated. Greater inhibition was observed with the addition of glucose available through the gravy and higher inoculum of the lactobacilli (Winkowski et al., 1993).
Although the introduction of these cultures may confer protection against potentially pathogenic microorganisms, the presence of live bacterial cultures, especially at greater concentrations, also risks potential adventitious growth that may contribute to the exacerbation of spoilage. One way lactobacilli may contribute to organoleptic changes is by accumulating metabolic end products such as hydrogen sulfide, resulting in an undesirable sulfur odor (Egan et al., 1989). However, this was associated with the predominance of only certain strains of Lactobacillus sake. It was noted that the production of hydrogen sulfide is also related to the growth of other organisms such as Shewanella putrefaciens and psychrotrophic Enterobacteriaceae in vacuumed packaged meat with a pH greater than six. Part of the mechanism by which lactobacilli may inhibit the growth of these organisms in these anaerobic conditions is through lowering the pH by the production of acids. Previous investigations have found that in situ production of lactic acid had been possible with Lactobacillus plantarum in pork and beef (Guerrero et al., 1995). Nonetheless, exploration of the use of the metabolites and inhibitory substances has been increasing to maintain uniformity.
Postbiotics—Antimicrobial Metabolites
The presence of live lactobacilli strains may confer many health benefits in addition to their potential inhibition of pathogenic microorganisms. However, changes in environmental conditions can result in unanticipated growth within the product. This may inadvertently result in physicochemical and microbiological changes, affecting the sensory attributes of the inoculated product. This risk of adventitious growth of the lactobacilli may be mitigated by eliminating the live cultures and using only their metabolites within these products. Additionally, the use of metabolites may be an avenue whereby spent culture media from growing the lactobacilli for use as probiotics may be valorized.
As previously outlined, several antimicrobial metabolites from lactobacilli have been explored as interventions against pathogenic organisms. They do not fit into the traditional definition of probiotics due to the absence of the live lactobacilli and are instead referred to as “postbiotics.” Postbiotics include the bioactive compounds generated within a matrix after fermentation by lactobacilli or other probiotics. Their historical use has allowed for a generally agreed-upon definition outlining what constitutes a “prebiotic” and “probiotic.” By contrast, no consensus exists for postbiotics due to their relative novelty compared to the two latter “-biotics” (Guerrero et al., 1995). Martín and Langella have defined it as “non-viable bacterial products or metabolic products from microorganisms that have biologic activity in the host” (Martín and Langella, 2019). Therefore, the individual components or the compounds within the matrix will be discussed separately in this section.
Lactic acid is one of the most prominent metabolites that is widely adopted in part for this purpose. However, it is not always the primary product of lactobacilli fermentation, depending on the strain and substrate conversion. For instance, lactobacilli like Lactobacillus pentosus produce primarily acetic acid in hydrolysates of vine shoots (Bustos et al., 2008). By contrast, homofermentative strains such as Lactobacillus plantarum are known to metabolize pentose or hexose sugars primarily to lactic acid (Okano et al., 2009). Additionally, metabolic engineering may also be employed to produce enantiomerically pure L-Lactic acid, as demonstrated with modified Lactobacillus helveticus CNRZ32 (Kylä-Nikkilä et al., 2000). Organic acids like lactic acid have shown potential in reducing foodborne pathogens in various food products (Castillo et al., 1998, 2001; Carpenter et al., 2011; Mikołajczyk, 2015). However, the extent to which lactobacilli produce the acids used for these purposes is unclear. However, the fermentation process is still the leading method in lactic acid production, with several drawbacks, such as the challenges associated with isolation of the chemical after fermentation. These factors have driven the development of chemical synthesis methods that have also been incentivized by the widespread use of lactic acid for applications outside of food products such as in chemical, pharmaceutical, and cosmetic industries. Significant progress has been observed after several decades of intense investigation into the chemo-catalyzed conversion of the traditional feedstock into lactic acid without fermentation (Shuklov et al., 2016).
Another prominent metabolite that is of interest in food production is bacteriocins. Despite the increasing number of bacteriocins gaining the GRAS status by the FDA, their adoption for commercial applications has been limited. The Lactococcus lactis-produced bacteriocin, nisin, remains the most widely used in food products (Soltani et al., 2021). However, studies have also explored the utility of bacteriocins produced by lactobacilli. Plantaricin BM-1, produced by Lactobacillus plantarum BM-1, from traditionally fermented Chinese meat products, was found effective in controlling the growth of Listeria monocytogenes in cooked ham (Zhou et al., 2015). Similarly, pentocin 31-1 from Lactobacillus pentosus 31-1 was found effective in suppressing the growth of Listeria during chilled storage of pork meat (Zhang et al., 2010). Katla et al. (2001, 2002) reported that Sakacin P produced by Lactobacillus sakei was effective against Listeria monocytogenes in chicken cold cuts and vacuum packaged salmon. Mills et al. (2011) reported that the use of Lactobacillus plantarum LMG P-26357 culture supernatant, which contained plantaricin 423, demonstrated anti-listerial activity against Listeria innocua that is more effective than the nisin producer alone (Mills et al., 2011). Furthermore, a combination of the bacteriocin produced by Lactobacillus reuteri INIA PRO 137, reuterin, with nisin resulted in a reduction of Listeria monocytogenes and Staphylococcus aureus in milk (Arqués et al., 2011). Lactobacillus plantarum ALC 01 secreting the bacteriocin pediocin AcH was also found effective against cheese-borne outbreak causing Listeria monocytogenes (Loessner et al., 2003). Combining nisin with curvaticin 13, produced by Lactobacillus curvatus SB13, also exhibited a more significant inhibitory effect against Listeria monocytogenes than individual bacteriocins (Bouttefroy and Millière, 2000). Incorporating cell-free supernatants from Lactobacillus curvatus P99 into films demonstrated the ability to control Listeria monocytogenes in sliced cheese (Marques et al., 2017). Ghalfi et al. (2006) explored several approaches to utilizing bacteriocin from Lactobacillus curvatus CWBI-B28 against Listeria monocytogenes in cold-smoked salmon. They found that all tested methods were effective against Listeria, though the use of immobilized lactobacilli suspension was the most effective in obtaining complete inactivation of the pathogen (Ghalfi et al., 2006).
Isolation and purification of individual bacteriocins present additional challenges to commercializing these antibacterial peptides. Thus, the antimicrobial efficacy of these substances is often evaluated within the culture supernatant of the organism. Arakawa et al. (2009) assessed the effectiveness of bacteriocins produced by Lactobacillus gasseri LA39 and LA158 (gassericins) by adding the neutralized de Man, Rogosa and Sharpe (MRS) culture supernatants. They reported that this method is a viable option for evaluating antibacterial properties as no other matrix component was found to have antimicrobial properties (Arakawa et al., 2009). In addition to the challenges associated with purification, the large-scale production of bacteriocins is still limited. Like organic acids, the chemical synthesis of certain bacteriocins is possible and may employ one of many approaches and methodologies. However, in contrast to lactic acid production, this process is in its infancy and has several setbacks it must overcome before commercialization. Particularly regarding low yield and optimization for large-scale production has not been demonstrated (Hemu et al., 2016). However, peptide synthesis continues to gain attention, particularly toward methods that could improve their antimicrobial potency and stability. Therefore, with the need for bioactive bacteriocins in the food and pharmaceutical industries, it is likely that progress in chemical synthesis approaches will continue in addition to recombinant technologies. These are not the only challenges with using bacteriocins as interventions in food systems, as there are additional factors to consider that may affect their efficacy. For example, certain bacteriocins are limited in their spectrum of activity, and their hydrophobic nature may limit their distribution within the food matrix to the fat phase.
These pathogens may also develop resistance to purified bacteriocins as with other established antimicrobials. This is particularly challenging to mitigate for antimicrobials with single-hit mechanisms like the Lactobacillus plantarum-produced bacteriocin pediocin AcH (Loessner et al., 2003). As not all pathogens are initially susceptible to bacteriocins, it is uncertain whether the resistance observed developed among previously susceptible pathogenic strains. However, the spontaneous emergence of mutants resistant to high concentrations of bacteriocins has been reported in bacteriocin-sensitive pathogens populations (Gravesen et al., 2002). Therefore, approaches to overcome these drawbacks have been explored. One method to increase their antibacterial activity while reducing the likelihood of resistance includes coupling lactobacilli-based treatments with other interventions.
Combination of Lactobacilli-Based Treatments With Other Interventions
One of the compelling challenges in employing any intervention targeted against foodborne pathogens is minimizing the impact on the final food product. This is an ongoing challenge for current commercialized interventions such as High-Pressure Processing (HPP). HPP is a non-thermal, commercialized processing method that has been found effective in reducing foodborne pathogens. Several investigations have noted that Gram-negative bacteria like E. coli and Salmonella were more susceptible to high pressure than Gram-positive organisms like Listeria monocytogenes, potentially due to differences in their peptidoglycan layer (Kelemen and Sharpe, 1979; Vachon et al., 2002; Wuytack et al., 2002). This method is favored particularly for clean label prospects as it employs only physical methods for pathogen control that would not lengthen the ingredients list. Although HPP may contribute less to sensory attributes alterations than chemical or thermal interventions, changes still occur and are not negligible. HPP use at higher pressure may induce irreversible modifications to the texture and color of meat that would affect consumer acceptability. However, these higher pressures are often necessary to ensure microbial inactivation and prevent their recovery during storage (Garriga et al., 2004). Other interventions against foodborne pathogens, including those that utilize lactobacilli and their metabolites, face a similar challenge to balance the intensity to which their application yields a considerable reduction of pathogens and that the product remains acceptable for the market.
In this regard, the combination of multiple interventions has been explored to enhance their efficacy against foodborne pathogens. Hurdle technology involves combining more than one approach to ensuring the control of pathogens in food products. The earliest descriptions of the concept suggest that it was conceived in the early 1990s by Leistner, who discussed the importance of several factors that contribute to preserving most foods (Leistner, 1992). The more prominent preservative factors in food production included temperature, water activity, acidity, preservatives, and competitive microorganisms. Leistner dubbed each factor as hurdles selected and intentionally applied based on their targeted applications (Leistner, 2000). Combination of multiple hurdles allowed for careful adjustments with each intervention. The conditions are optimized to ensure microbial safety without any deleterious effects on the sensory properties of the product.
Several studies have explored the effect of different combinations of interventions, including those paired with lactobacilli-based treatments. For example, combinations of bacteriocins from either different strains of lactobacilli or other lactic acid bacteria were found to enhance pathogen reduction and mitigate resistance (Bouttefroy and Millière, 2000; Arqués et al., 2011). This was also exemplified by combining a Lactobacillus-based probiotic culture with a commercial organic acid mix, which yielded a more consistent reduction of S. enterica serovar Enteritidis in the ceca and crop of broiler chicks than the organic acid mix alone (Wolfend et al., 2007). Reduction in cecal S. enterica serovar Heidelberg has also been observed in turkey poults provided a combination of Lactobacillus salivarius and Lactobacillus ingluviei with trans-cinnamaldehyde, the primary component of cinnamon bark essential oil (Dewi et al., 2021). Lactobacillus plantarum, in combination with essential oils, reduced the presence of undesired microorganisms in mayonnaise, thereby enhancing food safety while maintaining desirable organoleptic traits (Teneva et al., 2021). Additionally, HPP in combination with extracts from Lactobacillus casei OSY-LB6A exhibited synergy against a strain of Listeria monocytogenes resistant to pressure (Chung and Yousef, 2010). Permeabilization of the outer membrane of Gram-negative pathogens like E. coli by high pressure may sensitize the organism to other treatments, including bacteriocins (Hauben et al., 1996).
Additional Considerations and Concluding Remarks
Consumer-driven changes have led the industry to pursue more authentic, transparent, simple, and socially responsible production of foods from animals. However, meeting these demands is complex as animal-derived products often go through many stages, from their origins in hatcheries and breeding units to primary production, transport, harvest, processing, and post-processing steps. Furthermore, food animal production systems are burdened by a myriad of emerging pathogenic organisms that require timely refinement of control measures that potentially need the inclusion of novel compounds to preserve the quality and safety yet may not be familiar to the average consumer. Thus, producers must consider the public perception of the intervention they employ in addition to its safety and efficacy.
Lactobacillus has been historically used at various stages in animal food production systems, and technological advances have allowed researchers to characterize these organisms in greater depth, including investigating their genomic and metabolic activities for process improvements along the chain. Despite the advances made over the last decade, further exploration into the efficacy of lactobacilli-based interventions is necessary to recognize the full potential of this diverse bacterial genus. Ongoing investigations have also begun characterizing their synergism with other approaches that are either established or emerging and fitting to the demands of the clean label movement. Furthermore, the “Long-standing Presumption of Safety” status conferred to lactobacilli will minimize regulatory obstacles to their advancement.
Although the potential utility of lactobacilli in enhancing the microbial safety of animal-derived foods is the main subject of interest, the consumers' perception of the ingredients and manufacturing processes are also critical factors to consider for the development of interventions. Thus, elements such as regional preferences, consumer profiles, and how the products will be marketed are essential considerations in this context. Consumer research conducted by Ingredion found that products such as yeast extract and ascorbic acid were found to have varying levels of acceptability across different countries in Europe. By contrast, terms such as “natural flavors” and “natural colors” received unanimous acceptance (Ingredion, 2014). In this regard, consumers may be more receptive to lactobacilli-based ingredients if their association with familiar and healthy foods is addressed. The rise in popularity of fermented foods is associated with increasing evidence of its health-promoting properties and has brought even regional fermented foods and beverages to a global market.
Regional differences also exist regarding food safety concerns, which may not be as prominent for consumers in advanced economies with greater confidence in their respective food safety systems (Sutherland et al., 2020). However, special consideration may be observed with animal-derived products such as meat and poultry, considering their increased demand in emerging nations. Greater willingness to pay for environmentally certified lamb products was observed among consumers in India compared to their counterparts in China and the United Kingdom (Tait et al., 2016). Similarly, consumers were found to accept direct-fed microbials to enhance the food safety of beef against E. coli. They were willing to pay a premium to reduce the food safety risk (Britwum and Yiannaka, 2019). The challenge is to find a way to effectively communicate the use of preharvest interventions on the food label such that consumers are aware of its utility.
With growing enthusiasm for beneficial microbes among the general public, Lactobacillus-based clean label approaches can be envisioned and applied at different levels of production and processing, taking the financial implications of adoption also into consideration. Although a comprehensive approach targeting all segments from animal care on farms to the product sold in retail stores would be ideal, investigating the impact of their use in pre-slaughter stages on the clean label prospects of the final product without compromising the quality and safety, could also be assessed. The future is bright for Lactobacillus-based approaches as the industry moves forward in this direction using well-characterized strains or identifying novel strains catering to the clean label needs.
Author Contributions
AK conceptualized the idea. GD drafted the review and worked jointly with AK to expand the idea, design the outline, and make modifications and revisions to form the final manuscript. Both authors contributed to the article and approved the submitted version.
Funding
Funds for publication of the manuscript comes from the Minnesota Agricultural Experimentation Station Project MIN-16-120.
Conflict of Interest
The authors declare that the research was conducted in the absence of any commercial or financial relationships that could be construed as a potential conflict of interest.
Publisher's Note
All claims expressed in this article are solely those of the authors and do not necessarily represent those of their affiliated organizations, or those of the publisher, the editors and the reviewers. Any product that may be evaluated in this article, or claim that may be made by its manufacturer, is not guaranteed or endorsed by the publisher.
Acknowledgments
The authors would like to thank the United States Department of Agriculture NIFA grant #2018-68003-27464 and the Minnesota Turkey Research and Promotion Council Grants #2018-02 and 2019-02 that investigated Lactobacillus-based approaches in pre-slaughter turkey production as thought-provoking platforms foreseeing their potential clean–label use in animal production systems.
References
AAFCO (2018). 2018 AAFCO Annual Meeting Committee Reports. Available online at: https://www.aafco.org/Portals/0/SiteContent/Meetings/Annual/2018/2018_Annual_Committee_Report_Book.pdf
Alakomi, H., Skyttä, E., Saarela, M., and Helander, I. M. (2005). Lactic acid permeabilizes gram-negative bacteria by disrupting the outer membrane lactic acid permeabilizes gram-negative bacteria by disrupting the outer membrane. Appl. Environ. Microbiol. 66, 2000–2005. doi: 10.1128/AEM.66.5.2001-2005.2000.Updated
Ammor, M. S., and Mayo, B. (2007). Selection criteria for lactic acid bacteria to be used as functional starter cultures in dry sausage production: an update. Meat Sci. 76, 138–146. doi: 10.1016/j.meatsci.2006.10.022
Antonsson, M. (2003). Lactobacillus strains isolated from Danbo cheese as adjunct cultures in a cheese model system. Int. J. Food Microbiol. 85, 159–169. doi: 10.1016/S0168-1605(02)00536-6
Arakawa, K., Kawai, Y., Iioka, H., Tanioka, M., Nishimura, J., Kitazawa, H., et al. (2009). Effects of gassericins A and T, bacteriocins produced by Lactobacillus gasseri, with glycine on custard cream preservation. J. Dairy Sci. 92, 2365–2372. doi: 10.3168/jds.2008-1240
Arqués, J. L., Rodríguez, E., Nuñez, M., and Medina, M. (2011). Combined effect of reuterin and lactic acid bacteria bacteriocins on the inactivation of foodborne pathogens in milk. Food Control. 22, 457–461. doi: 10.1016/j.foodcont.2010.09.027
Aryana, K. J., and Olson, D. W. (2017). A 100-year review: Yogurt and other cultured dairy products. J. Dairy Sci. 100, 9987–10013. doi: 10.3168/jds.2017-12981
Asioli, D., Aschemann-Witzel, J., Caputo, V., Vecchio, R., Annunziata, A., Næs, T., et al. (2017). Making sense of the “clean label” trends: a review of consumer food choice behavior and discussion of industry implications. Food Res. Int. 99, 58–71. doi: 10.1016/j.foodres.2017.07.022
Bae, D., Kim, D.-H., Chon, J.-W., Song, K.-Y., and Seo, K.-H. (2020). Synergistic effects of the early administration of Lactobacillus kefiranofaciens DN1 and Kluyveromyces marxianus KU140723-05 on the inhibition of Salmonella Enteritidis colonization in young chickens. Poult. Sci. 99, 5999–6006. doi: 10.1016/j.psj.2020.07.032
Bell, B. P. (1994). A multistate outbreak of Escherichia coli O157:H7—associated bloody diarrhea and hemolytic uremic syndrome from hamburgers. JAMA 272, 1349. doi: 10.1001/jama.1994.03520170059036
Bernardeau, M., Guguen, M., and Vernoux, J. P. (2006). Beneficial lactobacilli in food and feed: long-term use, biodiversity, and proposals for specific and realistic safety assessments. FEMS Microbiol. Rev. 30, 487–513. doi: 10.1111/j.1574-6976.2006.00020.x
Berthier, F. (1993). On the screening of hydrogen peroxide-generating lactic acid bacteria. Lett. Appl. Microbiol. 16, 150–153. doi: 10.1111/j.1472-765X.1993.tb01381.x
Bouttefroy, A., and Millière, J.-B. (2000). Nisin–curvaticin 13 combinations for avoiding the regrowth of bacteriocin resistant cells of Listeria monocytogenes ATCC 15313. Int. J. Food Microbiol. 62, 65–75. doi: 10.1016/S0168-1605(00)00372-X
Brashears, M. M., Galyean, M. L., Loneragan, G. H., Mann, J. E., and Killinger-Mann, K. (2003). Prevalence of Escherichia coli O157:H7 and performance by beef feedlot cattle given lactobacillus direct-fed microbials. J. Food Prot. 66, 748–754. doi: 10.4315/0362-028X-66.5.748
Brashears, M. M., Reilly, S. S., and Gilliland, S. E. (1998). Antagonistic action of cells of Lactobacillus lactis toward Escherichia coli O157:H7 on refrigerated raw chicken meat. J. Food Prot. 61, 166–170. doi: 10.4315/0362-028X-61.2.166
Bredholt, S., Nesbakken, T., and Holck, A. (2001). Industrial application of an antilisterial strain of Lactobacillus sakei as a protective culture and its effect on the sensory acceptability of cooked, sliced, vacuum-packaged meats. Int. J. Food Microbiol. 66, 191–196. doi: 10.1016/S0168-1605(00)00519-5
Britwum, K., and Yiannaka, A. (2019). Consumer willingness to pay for food safety interventions: the role of message framing and issue involvement. Food Policy 86, 101726. doi: 10.1016/j.foodpol.2019.05.009
Bustos, G., Moldes, A. B., Cruz, J. M., and Domínguez, J. M. (2008). Influence of the metabolism pathway on lactic acid production from hemicellulosic trimming vine shoots hydrolyzates using Lactobacillus pentosus. Biotechnol. Prog. 21, 793–798. doi: 10.1021/bp049603v
Carpenter, C. E., Smith, J. V., and Broadbent, J. R. (2011). Efficacy of washing meat surfaces with 2% levulinic, acetic, or lactic acid for pathogen decontamination and residual growth inhibition. Meat Sci. 88, 256–260. doi: 10.1016/j.meatsci.2010.12.032
Casey, P. G., Gardiner, G. E., Casey, G., Bradshaw, B., Lawlor, P. G., Lynch, P. B., et al. (2007). A five-strain probiotic combination reduces pathogen shedding and alleviates disease signs in pigs challenged with Salmonella enterica serovar Typhimurium. Appl. Environ. Microbiol. 73, 1858–1863. doi: 10.1128/AEM.01840-06
Castellano, P., González, C., Carduza, F., and Vignolo, G. (2010). Protective action of Lactobacillus curvatus CRL705 on vacuum-packaged raw beef. Effect on sensory and structural characteristics. Meat Sci. 85, 394–401. doi: 10.1016/j.meatsci.2010.02.007
Castillo, A., Lucia, L. M., Goodson, K. J., Savell, J. W., and Acuff, G. R. (1998). Comparison of water wash, trimming, and combined hot water and lactic acid treatments for reducing bacteria of fecal origin on beef carcasses. J. Food Prot. 61, 823–828. doi: 10.4315/0362-028X-61.7.823
Castillo, A., Lucia, L. M., Roberson, D. B., Stevenson, T. H., Mercado, I., and Acuff, G. R. (2001). Lactic acid sprays reduce bacterial pathogens on cold beef carcass surfaces and in subsequently produced ground beef. J. Food Prot. 64, 58–62. doi: 10.4315/0362-028X-64.1.58
CDC (2017). NORS Dashboard. Natl. Outbreak Report. Syst. Available online at: https://www.cdc.gov/nors/index.html (accessed May 23, 2018).
CDC (2019a). Surveillance for Foodborne Disease Outbreaks United States, 2017: Annual Report. Atlanta, GA. Available online at: https://www.cdc.gov/fdoss/pdf/2017_FoodBorneOutbreaks_508.pdf
CDC (2019b). National Outbreak Reporting System (NORS). Available online at: https://www.cdc.gov/nors/index.html (accessed March 5, 2020).
Chen, X., Xu, J., Shuai, J., Chen, J., Zhang, Z., and Fang, W. (2007). The S-layer proteins of Lactobacillus crispatus strain ZJ001 is responsible for competitive exclusion against Escherichia coli O157:H7 and Salmonella Typhimurium. Int. J. Food Microbiol. 115, 307–312. doi: 10.1016/j.ijfoodmicro.2006.11.007
Chung, H.-J., and Yousef, A. E. (2010). Synergistic effect of high pressure processing and Lactobacillus casei antimicrobial activity against pressure resistant Listeria monocytogenes. N. Biotechnol. 27, 403–408. doi: 10.1016/j.nbt.2010.04.007
Collins, E. B., and Aramaki, K. (1980). Production of hydrogen peroxide by Lactobacillus acidophilus. J. Dairy Sci. 63, 353–357. doi: 10.3168/jds.S0022-0302(80)82938-9
Corr, S. C., Li, Y., Riedel, C. U., O'Toole, P. W., Hill, C., and Gahan, C. G. M. (2007). Bacteriocin production as a mechanism for the antiinfective activity of Lactobacillus salivarius UCC118. Proc. Natl. Acad. Sci. U.S.A. 104, 7617–7621. doi: 10.1073/pnas.0700440104
Cotter, P. D., Hill, C., and Ross, R. P. (2005). Bacteriocins: developing innate immunity for food. Nat. Rev. Microbiol. 3, 777–788. doi: 10.1038/nrmicro1273
Cotter, P. D., Ross, R. P., and Hill, C. (2013). Bacteriocins — a viable alternative to antibiotics? Nat. Rev. Microbiol. 11, 95–105. doi: 10.1038/nrmicro2937
Dahiya, R. S., and Speck, M. L. (1968). Hydrogen peroxide formation by lactobacilli and its effect on Staphylococcus aureus. J. Dairy Sci. 51, 1568–1572. doi: 10.3168/jds.S0022-0302(68)87232-7
Datta, R., and Henry, M. (2006). Lactic acid: recent advances in products, processes and technologies — a review. J. Chem. Technol. Biotechnol. 81, 1119–1129. doi: 10.1002/jctb.1486
De Angelis, M., Siragusa, S., Caputo, L., Ragni, A., Burzigotti, R., and Gobbetti, M. (2007). Survival and persistence of Lactobacillus plantarum 4.1 and Lactobacillus reuteri 3S7 in the gastrointestinal tract of pigs. Vet. Microbiol. 123, 133–144. doi: 10.1016/j.vetmic.2007.02.022
De Klerk, H. C. (1967). Bacteriocinogeny in Lactobacillus fermenti. Nature 214, 609–609. doi: 10.1038/214609a0
De Klerk, H. C., and Smit, J. A. (1967). Properties of a Lactobacillus fermenti bacteriocin. J. Gen. Microbiol. 48, 309–316. doi: 10.1099/00221287-48-2-309
Delgado-Pando, G., Ekonomou, S. I., Stratakos, A. C., and Pintado, T. (2021). Clean label alternatives in meat products. Foods 10, 1615. doi: 10.3390/foods10071615
Delves-Broughton, J., Blackburn, P., Evans, R. J., and Hugenholtz, J. (1996). Applications of the bacteriocin, nisin. Antonie Van Leeuwenhoek 69, 193–202. doi: 10.1007/BF00399424
Dewi, G., Manjankattil, S., Peichel, C., Johnson, T. J., Noll, S., Cardona, C., et al. (2021). “Effect of turkey-derived Lactobacillus probiotics and trans-cinnamaldehyde against multidrug-resistant Salmonella Heidelberg in turkey poults,” in Proceedings of IAFP 2021 (Phoenix, AZ). Available online at: https://iafp.confex.com/iafp/2021/onlineprogram.cgi/Paper/26620
Diep, D., and Nes, I. (2002). Ribosomally synthesized antibacterial peptides in gram positive bacteria. Curr. Drug Targets 3, 107–122. doi: 10.2174/1389450024605409
Dortu, C., Huch, M., Holzapfel, W. H., Franz, C. M. A. P., and Thonart, P. (2008). Anti-listerial activity of bacteriocin-producing Lactobacillus curvatus CWBI-B28 and Lactobacillus sakei CWBI-B1365 on raw beef and poultry meat. Lett. Appl. Microbiol. 47, 581–586. doi: 10.1111/j.1472-765X.2008.02468.x
Duar, R. M., Lin, X. B., Zheng, J., Martino, M. E., Grenier, T., Pérez-Muñoz, M. E., et al. (2017). Lifestyles in transition: evolution and natural history of the genus Lactobacillus. FEMS Microbiol. Rev. 41, S27–S48. doi: 10.1093/femsre/fux030
Dunne, C., Murphy, L., Flynn, S., O'Mahony, L., O'Halloran, S., Feeney, M., et al. (1999). “Probiotics: from myth to reality. Demonstration of functionality in animal models of disease and in human clinical trials,” in Lactic Acid Bacteria: Genetics, Metabolism and Applications (Dordrecht: Springer Netherlands), 279–292.
Egan, A. F., and Shay, B. J. (1982). Significance of lactobacilli and film permeability in the spoilage of vacuum-packaged beef. J. Food Sci. 47, 1119–1122. doi: 10.1111/j.1365-2621.1982.tb07630.x
Egan, A. F., Shay, B. J., and Rogers, P. J. (1989). Factors affecting the production of hydrogen sulphide by Lactobacillus sake L13 growing on vacuum-packaged beef. J. Appl. Bacteriol. 67, 255–262. doi: 10.1111/j.1365-2672.1989.tb02493.x
Eijsink, V. G. H., Skeie, M., Middelhoven, P. H., Brurberg, M. B., and Nes, I. F. (1998). Comparative studies of class IIa bacteriocins of lactic acid bacteria. Appl. Environ. Microbiol. 64, 3275–3281. doi: 10.1128/AEM.64.9.3275-3281.1998
Elam, N. A., Gleghorn, J. F., Rivera, J. D., Galyean, M. L., Defoor, P. J., Brashears, M. M., et al. (2003). Effects of live cultures of Lactobacillus acidophilus (strains NP45 and NP51) and Propionibacterium freudenreichii on performance, carcass, and intestinal characteristics, and Escherichia coli strain O157 shedding of finishing beef steers. J. Anim. Sci. 81, 2686–2698. doi: 10.2527/2003.81112686x
Eschenbach, D. A., Davick, P. R., Williams, B. L., Klebanoff, S. J., Young-Smith, K., Critchlow, C. M., et al. (1989). Prevalence of hydrogen peroxide-producing Lactobacillus species in normal women and women with bacterial vaginosis. J. Clin. Microbiol. 27, 251–256. doi: 10.1128/jcm.27.2.251-256.1989
Eun, C., soo Kim, Y., seok Han, D., soo Choi, J., hyun Lee, A., reum Park, Y., and kyung (2011). Lactobacillus casei prevents impaired barrier function in intestinal epithelial cells. APMIS 119, 49–56. doi: 10.1111/j.1600-0463.2010.02691.x
Fayol-Messaoudi, D., Berger, C. N., Coconnier-Polter, M.-H., Liévin-Le Moal, V., and Servin, A. L. (2005). pH-, lactic acid-, and non-lactic acid-dependent activities of probiotic lactobacilli against Salmonella enterica serovar Typhimurium. Appl. Environ. Microbiol. 71, 6008–6013. doi: 10.1128/AEM.71.10.6008-6013.2005
FDA (1995). CPG Sec. 689.100 Direct-Fed Microbial Products. Available online at: https://www.fda.gov/regulatory-information/search-fda-guidance-documents/cpg-sec-689100-direct-fed-microbial-products
FDA (2018). Environmental Assessment of Factors Potentially Contributing to the Contamination of Romaine Lettuce Implicated in a Multi-State Outbreak of E. coli O157:H7. Outbreaks Foodborne Illn. Available online at: https://www.fda.gov/food/outbreaks-foodborne-illness/environmental-assessment-factors-potentially-contributing-contamination-romaine-lettuce-implicated (accessed July 5, 2020).
FDA (2021). Section 184.1 - Substances Added Directly to Human Food Affirmed as Generally Recognized as Safe (GRAS). Available online at: https://www.ecfr.gov/cgi-bin/text-idx?SID=6dc7a326a8995a0dcda570a110183975&mc=true&node=pt21.3.184&rgn=div5#se21.3.184_11
Frizzo, L. S., Zbrun, M. V., Soto, L. P., Bertozzi, E., Sequeira, G. J., Marti, L. E., et al. (2012). Pathogen translocation and histopathological lesions in an experimental model of Salmonella Dublin infection in calves receiving lactic acid bacteria and lactose supplements. J. Vet. Sci. 13, 261. doi: 10.4142/jvs.2012.13.3.261
FSIS (1996). Pathogen Reduction; Hazard Analysisand Critical Control Point (HACCP)Systems. Available online at: https://www.govinfo.gov/content/pkg/FR-1996-07-25/pdf/96-17837.pdf
Gänzle, M. G. (2015). Lactic metabolism revisited: metabolism of lactic acid bacteria in food fermentations and food spoilage. Curr. Opin. Food Sci. 2, 106–117. doi: 10.1016/j.cofs.2015.03.001
Garneau, S., Martin, N. I., and Vederas, J. C. (2002). Two-peptide bacteriocins produced by lactic acid bacteria. Biochimie 84, 577–592. doi: 10.1016/S0300-9084(02)01414-1
Garriga, M., Grèbol, N., Aymerich, M. T., Monfort, J. M., and Hugas, M. (2004). Microbial inactivation after high-pressure processing at 600 MPa in commercial meat products over its shelf life. Innov. Food Sci. Emerg. Technol. 5, 451–457. doi: 10.1016/j.ifset.2004.07.001
Ghalfi, H., Allaoui, A., Destain, J., Benkerroum, N., and Thonart, P. (2006). Bacteriocin activity by Lactobacillus curvatus cwbi-b28 to inactivate Listeria monocytogenes in cold-smoked salmon during 4°c storage. J. Food Prot. 69, 1066–1071. doi: 10.4315/0362-028X-69.5.1066
Gill, H. S., Shu, Q., Lin, H., Rutherfurd, K. J., and Cross, M. L. (2001). Protection against translocating Salmonella Typhimurium infection in mice by feeding the immuno-enhancing probiotic Lactobacillus rhamnosus strain HN001. Med. Microbiol. Immunol. 190, 97–104. doi: 10.1007/s004300100095
Gopal, P. K., Prasad, J., Smart, J., and Gill, H. S. (2001). In vitro adherence properties of Lactobacillus rhamnosus DR20 and Bifidobacterium lactis DR10 strains and their antagonistic activity against an enterotoxigenic Escherichia coli. Int. J. Food Microbiol. 67, 207–216. doi: 10.1016/S0168-1605(01)00440-8
Gorton, A., and Stasiewicz, M. J. (2017). Twenty-two years of U.S. meat and poultry product recalls: implications for food safety and food waste. J. Food Prot. 80, 674–684. doi: 10.4315/0362-028X.JFP-16-388
Gravesen, A., Ramnath, M., Rechinger, K. B., Andersen, N., Jänsch, L., Héchard, Y., et al. (2002). High-level resistance to class IIa bacteriocins is associated with one general mechanism in Listeria monocytogenes. Microbiology 148, 2361–2369. doi: 10.1099/00221287-148-8-2361
Guerrero, I., Mendiolea, R., Ponce, E., and Prado, A. (1995). Inoculation of lactic acid bacteria on meat surfaces as a means of decontamination in semitropical conditions. Meat Sci. 40, 397–411. doi: 10.1016/0309-1740(94)00024-2
Guo, S., Gillingham, T., Guo, Y., Meng, D., Zhu, W., Walker, W. A., et al. (2017). Secretions of Bifidobacterium infantis and Lactobacillus acidophilus protect intestinal epithelial barrier function. J. Pediatr. Gastroenterol. Nutr. 64, 404–412. doi: 10.1097/MPG.0000000000001310
Haller, D. (2000). Non-pathogenic bacteria elicit a differential cytokine response by intestinal epithelial cell/leucocyte co-cultures. Gut 47, 79–87. doi: 10.1136/gut.47.1.79
Hancock, D. (2001). The control of VTEC in the animal reservoir. Int. J. Food Microbiol. 66, 71–78. doi: 10.1016/S0168-1605(00)00487-6
Hauben, K. J. A., Wuytack, E. Y., Soontjens, C. C. F., and Michiels, C. W. (1996). High-pressure transient sensitization of Escherichia coli to lysozyme and nisin by disruption of outer-membrane permeability. J. Food Prot. 59, 350–355. doi: 10.4315/0362-028X-59.4.350
Hemu, X., Qiu, Y., Nguyen, G. K. T., and Tam, J. P. (2016). Total synthesis of circular bacteriocins by butelase 1. J. Am. Chem. Soc. 138, 6968–6971. doi: 10.1021/jacs.6b04310
Heng, N. C. K., Wescombe, P. A., Burton, J. P., Jack, R. W., and Tagg, J. R. (2007). “The diversity of bacteriocins in gram-positive bacteria,” in Bacteriocins, eds M. A. Riley and M. A. Chavan (Berlin: Springer Berlin Heidelberg), 45–92.
Heyman, M., and Ménard, S. (2002). Probiotic microorganisms: how they affect intestinal pathophysiology. Cell. Mol. Life Sci. 59, 1151–1165. doi: 10.1007/s00018-002-8494-7
Higgins, J. P., Higgins, S. E., Vicente, J. L., Wolfenden, A. D., Tellez, G., and Hargis, B. M. (2007). Temporal effects of lactic acid bacteria probiotic culture on Salmonella in neonatal broilers. Poult. Sci. 86, 1662–1666. doi: 10.1093/ps/86.8.1662
Hogue, A. T., White, P. L., and Heminover, J. A. (1998). Pathogen reduction and hazard analysis and critical control point (HACCP) systems for meat and poultry. Vet. Clin. North Am. Food Anim. Pract. 14, 151–164. doi: 10.1016/S0749-0720(15)30286-3
Hu, J., Chen, L., Zheng, W., Shi, M., Liu, L., Xie, C., et al. (2018). Lactobacillus frumenti facilitates intestinal epithelial barrier function maintenance in early-weaned piglets. Front. Microbiol. 9, 897. doi: 10.3389/fmicb.2018.00897
Huang, C., Qiao, S., Li, D., Piao, X., and Ren, J. (2004). Effects of lactobacilli on the performance, diarrhea incidence, vfa concentration and gastrointestinal microbial flora of weaning pigs. Asian Austral. J. Anim. Sci. 17, 401–409. doi: 10.5713/ajas.2004.401
Hudault, S., Liévin, V., Bernet-Camard, M. F., and Servin, A. L. (1997). Antagonistic activity exerted in vitro and in vivo by Lactobacillus casei (strain GG) against Salmonella Typhimurium C5 infection. Appl. Environ. Microbiol. 63, 513–518. doi: 10.1128/AEM.63.2.513-518.1997
Hurst, A. (1972). Interactions of food starter cultures and food-borne pathogens: the antagonism between Streptococcus lactis and sporeforming microbes. J. Milk Food Technol. 35, 418–423. doi: 10.4315/0022-2747-35.7.418
Ingrassia, I., Leplingard, A., and Darfeuille-Michaud, A. (2005). Lactobacillus casei DN-114 001 inhibits the ability of adherent-invasive Escherichia coli isolated from crohn's disease patients to adhere to and to invade intestinal epithelial cells. Appl. Environ. Microbiol. 71, 2880–2887. doi: 10.1128/AEM.71.6.2880-2887.2005
Ingredion (2014). The Clean Label Guide to Europe. Available online at: https://www.ingredion.es/content/dam/ingredion/pdf-downloads/emea/87-TheCleanLabelGuidetoEuropefromIngredion.pdf
Joerger, M. C., and Klaenhammer, T. R. (1990). Cloning, expression, and nucleotide sequence of the Lactobacillus helveticus 481 gene encoding the bacteriocin helveticin J. J. Bacteriol. 172, 6339–6347. doi: 10.1128/JB.172.11.6339-6347.1990
Joerger, R. D., and Ganguly, A. (2017). Current status of the preharvest application of pro- and prebiotics to farm animals to enhance the microbial safety of animal products. Microbiol. Spectrum 5, PFS-0012-2016. doi: 10.1128/microbiolspec.PFS-0012-2016
Jung, G. (1991). Lantibiotics—ribosomally synthesized biologically active polypeptides containing sulfide bridges and α,β-didehydroamino acids. Angew. Chemie Int. Ed. English 30, 1051–1068. doi: 10.1002/anie.199110513
Katla, T., Møretrø, T., Aasen, I. M., Holck, A., Axelsson, L., and Naterstad, K. (2001). Inhibition of Listeria monocytogenes in cold smoked salmon by addition of sakacin P and/or live Lactobacillus sakei cultures. Food Microbiol. 18, 431–439. doi: 10.1006/fmic.2001.0420
Katla, T., Moretro, T., Sveen, I., Aasen, I. M., Axelsson, L., Rorvik, L. M., et al. (2002). Inhibition of Listeria monocytogenes in chicken cold cuts by addition of sakacin P and sakacin P-producing Lactobacillus sakei. J. Appl. Microbiol. 93, 191–196. doi: 10.1046/j.1365-2672.2002.01675.x
Kawai, Y., Ishii, Y., Arakawa, K., Uemura, K., Saitoh, B., Nishimura, J., et al. (2004). Structural and functional differences in two cyclic bacteriocins with the same sequences produced by lactobacilli. Appl. Environ. Microbiol. 70, 2906–2911. doi: 10.1128/AEM.70.5.2906-2911.2004
Keenan, T. W., and Lindsay, R. C. (1968). Diacetyl production and utilization by Lactobacillus species. J. Dairy Sci. 51, 188–191. doi: 10.3168/jds.S0022-0302(68)86948-6
Kelemen, M. V., and Sharpe, J. E. (1979). Controlled cell disruption: a comparison of the forces required to disrupt different micro-organisms. J. Cell Sci. 35, 431–441. doi: 10.1242/jcs.35.1.431
Kerr, A. K., Farrar, A. M., Waddell, L. A., Wilkins, W., Wilhelm, B. J., Bucher, O., et al. (2013). A systematic review-meta-analysis and meta-regression on the effect of selected competitive exclusion products on Salmonella spp. prevalence and concentration in broiler chickens. Prev. Vet. Med. 111, 112–125. doi: 10.1016/j.prevetmed.2013.04.005
Khalid, N. M., and Marth, E. H. (1990). Lactobacilli — their enzymes and role in ripening and spoilage of cheese: a review. J. Dairy Sci. 73, 2669–2684. doi: 10.3168/jds.S0022-0302(90)78952-7
Kim, T.-K., Seo, D.-H., Sung, J.-M., Ku, S.-K., Jeon, K.-H., Kim, Y.-B., et al. (2017). Study of optimization of natural nitrite source production from spinach. Korean J. Food Sci. Technol. 49, 459–461. doi: 10.9721/KJFST.2017.49.4.459
Klaenhammer, T. R. (1993). Genetics of bacteriocins produced by lactic acid bacteria. FEMS Microbiol. Rev. 12, 39–85. doi: 10.1111/j.1574-6976.1993.tb00012.x
Koenen, M. E., Kramer, J., van der Hulst, R., Heres, L., Jeurissen, S. H. M., and Boersma, W. J. A. (2004). Immunomodulation by probiotic lactobacilli in layer- and meat-type chickens. Br. Poult. Sci. 45, 355–366. doi: 10.1080/00071660410001730851
Konstantinov, S. R., Smidt, H., Akkermans, A. D. L., Casini, L., Trevisi, P., Mazzoni, M., et al. (2008). Feeding of Lactobacillus sobrius reduces Escherichia coli F4 levels in the gut and promotes growth of infected piglets. FEMS Microbiol. Ecol. 66, 599–607. doi: 10.1111/j.1574-6941.2008.00517.x
Kylä-Nikkilä, K., Hujanen, M., Leisola, M., and Palva, A. (2000). Metabolic engineering of Lactobacillus helveticus CNRZ32 for production of purel-(+)-lactic acid. Appl. Environ. Microbiol. 66, 3835–3841. doi: 10.1128/AEM.66.9.3835-3841.2000
La Ragione, R. M., Narbad, A., Gasson, M. J., and Woodward, M. J. (2004). In vivo characterization of Lactobacillus johnsonii FI9785 for use as a defined competitive exclusion agent against bacterial pathogens in poultry. Lett. Appl. Microbiol. 38, 197–205. doi: 10.1111/j.1472-765X.2004.01474.x
Lehto, E. M., and Salminen, S. J. (1997). Inhibition of Salmonella Typhimurium adhesion to Caco-2 cell cultures by Lactobacillus strain GG spent culture supernate: only a pH effect? FEMS Immunol. Med. Microbiol. 18, 125–132. doi: 10.1111/j.1574-695X.1997.tb01037.x
Leistner, L. (1992). Food preservation by combined methods. Food Res. Int. 25, 151–158. doi: 10.1016/0963-9969(92)90158-2
Leistner, L. (2000). Basic aspects of food preservation by hurdle technology. Int. J. Food Microbiol. 55, 181–186. doi: 10.1016/S0168-1605(00)00161-6
Lema, M., Williams, L., and Rao, D. (2001). Reduction of fecal shedding of enterohemorrhagic Escherichia coli O157:H7 in lambs by feeding microbial feed supplement. Small Rumin. Res. 39, 31–39. doi: 10.1016/S0921-4488(00)00168-1
Li, S., Kinser, C., Ziara, R. M. M., Dvorak, B., and Subbiah, J. (2018). Environmental and economic implications of food safety interventions: Life cycle and operating cost assessment of antimicrobial systems in U.S. beef packing industry. J. Clean. Prod. 198, 541–550. doi: 10.1016/j.jclepro.2018.07.020
Lin, Y. P., Thibodeaux, C. H., Peña, J. A., Ferry, G. D., and Versalovic, J. (2008). Probiotic Lactobacillus reuteri suppress proinflammatory cytokines via c-Jun. Inflamm. Bowel Dis. 14, 1068–1083. doi: 10.1002/ibd.20448
Liu, H., Zhang, J., Zhang, S., Yang, F., Thacker, P. A., Zhang, G., et al. (2014). Oral administration of Lactobacillus fermentum I5007 favors intestinal development and alters the intestinal microbiota in formula-fed piglets. J. Agric. Food Chem. 62, 860–866. doi: 10.1021/jf403288r
Loessner, M., Guenther, S., Steffan, S., and Scherer, S. (2003). A pediocin-producing Lactobacillus plantarum strain inhibits Listeria monocytogenes in a multispecies cheese surface microbial ripening consortium. Appl. Environ. Microbiol. 69, 1854–1857. doi: 10.1128/AEM.69.3.1854-1857.2003
Lyhs, U., Korkeala, H., Vandamme, P., and Björkroth, J. (2001). Lactobacillus alimentarius: a specific spoilage organism in marinated herring. Int. J. Food Microbiol. 64, 355–360. doi: 10.1016/S0168-1605(00)00486-4
Lyles, M. A., Flynn, B. B., and Frohlich, M. T. (2008). All supply chains don't flow through: understanding supply chain issues in product recalls. Manag. Organ. Rev. 4, 167–182. doi: 10.1111/j.1740-8784.2008.00106.x
Madsen, K. L., Doyle, J. S., Jewell, L. D., Tavernini, M. M., and Fedorak, R. N. (1999). Lactobacillus species prevents colitis in interleukin 10 gene–deficient mice. Gastroenterology 116, 1107–1114. doi: 10.1016/S0016-5085(99)70013-2
Mangell, P., Nejdfors, P., Wang, M., Ahrné, S., Weström, B., Thorlacius, H., et al. (2002). Lactobacillus plantarum 299v inhibits Escherichia coli-induced intestinal permeability. Dig. Dis. Sci. 47, 511–516. doi: 10.1023/a:1017947531536
Maqueda, M., Galvez, A., Bueno, M., Sanchez-Barrena, M., Gonzalez, C., Albert, A., et al. (2004). Peptide AS-48: prototype of a new class of cyclic bacteriocins. Curr. Protein Pept. Sci. 5, 399–416. doi: 10.2174/1389203043379567
Maragkoudakis, P. A., Mountzouris, K. C., Psyrras, D., Cremonese, S., Fischer, J., Cantor, M. D., et al. (2009). Functional properties of novel protective lactic acid bacteria and application in raw chicken meat against Listeria monocytogenes and Salmonella Enteritidis. Int. J. Food Microbiol. 130, 219–226. doi: 10.1016/j.ijfoodmicro.2009.01.027
Marques, J., de, L., Funck, G. D., Dannenberg, G., da, S., Cruxen, C. E., dos, S., Halal, S. L.M., et al. (2017). Bacteriocin-like substances of Lactobacillus curvatus P99: characterization and application in biodegradable films for control of Listeria monocytogenes in cheese. Food Microbiol. 63, 159–163. doi: 10.1016/j.fm.2016.11.008
Martín, R., and Langella, P. (2019). Emerging health concepts in the probiotics field: streamlining the definitions. Front. Microbiol. 10, 1047. doi: 10.3389/fmicb.2019.01047
Martinez, R. C. R., Staliano, C. D., Vieira, A. D. S., Villarreal, M. L. M., Todorov, S. D., Saad, S. M. I., et al. (2015). Bacteriocin production and inhibition of Listeria monocytogenes by Lactobacillus sakei subsp. sakei 2a in a potentially synbiotic cheese spread. Food Microbiol. 48, 143–152. doi: 10.1016/j.fm.2014.12.010
Menconi, A., Wolfenden, A. D., Shivaramaiah, S., Terraes, J. C., Urbano, T., Kuttel, J., et al. (2011). Effect of lactic acid bacteria probiotic culture for the treatment of Salmonella enterica serovar Heidelberg in neonatal broiler chickens and turkey poults. Poult. Sci. 90, 561–565. doi: 10.3382/ps.2010-01220
Metchnikoff, E. (1908). The Prolongation of Life. ed P. C. Mitchell. New York, NY; London: G.P. Putnam's Sons.
Midolo, P. D., Lambert, J. R., Hull, R., Luo, F., and Grayson, M. L. (1995). In vitro inhibition of Helicobacter pylori NCTC 11637 by organic acids and lactic acid bacteria. J. Appl. Bacteriol. 79, 475–479. doi: 10.1111/j.1365-2672.1995.tb03164.x
Mikołajczyk, A. (2015). Evaluation of the effects of a mixture of organic acids and duration of storage on the survival of Salmonella on turkey carcasses. J. Food Prot. 78, 585–589. doi: 10.4315/0362-028X.JFP-14-135
Mills, S., Serrano, L. M., Griffin, C., O'Connor, P. M., Schaad, G., Bruining, C., et al. (2011). Inhibitory activity of Lactobacillus plantarum LMG P-26358 against Listeria innocua when used as an adjunct starter in the manufacture of cheese. Microb. Cell Fact. 10, S7. doi: 10.1186/1475-2859-10-S1-S7
Milner, K. C., and Shaffer, M. F. (1952). Bacteriologic stupies of experimental salmonella infections in chicks. J. Infect. Dis. 90, 81–96. doi: 10.1093/infdis/90.1.81
Montville, T. J., and Chen, Y. (1998). Mechanistic action of pediocin and nisin: recent progress and unresolved questions. Appl. Microbiol. Biotechnol. 50, 511–519. doi: 10.1007/s002530051328
Morishita, T. Y., Aye, P. P., Harr, B. S., Cobb, C. W., and Clifford, J. R. (1997). Evaluation of an avian-specific probiotic to reduce the colonization and shedding of Campylobacter jejuni in broilers. Avian Dis. 41, 850. doi: 10.2307/1592338
Mørtvedt, C. I., Nissen-Meyer, J., Sletten, K., and Nes, I. F. (1991). Purification and amino acid sequence of lactocin S, a bacteriocin produced by Lactobacillus sake L45. Appl. Environ. Microbiol. 57, 1829–1834. doi: 10.1128/aem.57.6.1829-1834.1991
Neal-McKinney, J. M., Lu, X., Duong, T., Larson, C. L., Call, D. R., Shah, D. H., et al. (2012). Production of organic acids by probiotic lactobacilli can be used to reduce pathogen load in poultry. PLoS ONE 7, e43928. doi: 10.1371/journal.pone.0043928
Nes, I. F., Diep, D. B., Håvarstein, L. S., Brurberg, M. B., Eijsink, V., and Holo, H. (1996). Biosynthesis of bacteriocins in lactic acid bacteria. Antonie Van Leeuwenhoek 70, 113–128. doi: 10.1007/BF00395929
Nurmi, E., and Rantala, M. (1973). New aspects of Salmonella infection in broiler production. Nature 241, 210–211. doi: 10.1038/241210a0
Okano, K., Yoshida, S., Tanaka, T., Ogino, C., Fukuda, H., and Kondo, A. (2009). Homo -lactic acid fermentation from arabinose by redirection of the phosphoketolase pathway to the pentose phosphate pathway in lactate dehydrogenase gene-deficient Lactobacillus plantarum. Appl. Environ. Microbiol. 75, 5175–5178. doi: 10.1128/AEM.00573-09
Papadochristopoulos, A., Kerry, J. P., Fegan, N., Burgess, C. M., and Duffy, G. (2021). Natural anti-microbials for enhanced microbial safety and shelf-life of processed packaged meat. Foods 10, 1598. doi: 10.3390/foods10071598
Parassol, N., Freitas, M., Thoreux, K., Dalmasso, G., Bourdet-Sicard, R., and Rampal, P. (2005). Lactobacillus casei DN-114 001 inhibits the increase in paracellular permeability of enteropathogenic Escherichia coli-infected T84 cells. Res. Microbiol. 156, 256–262. doi: 10.1016/j.resmic.2004.09.013
Pascual, M., Hugas, M., Badiola, J. I., Monfort, J. M., and Garriga, M. (1999). Lactobacillus salivarius CTC2197 prevents Salmonella Enteritidis colonization in chickens. Appl. Environ. Microbiol. 65, 4981–4986. doi: 10.1128/AEM.65.11.4981-4986.1999
Patterson, J., and Burkholder, K. (2003). Application of prebiotics and probiotics in poultry production. Poult. Sci. 82, 627–631. doi: 10.1093/ps/82.4.627
Pedersen, K., and Tannock, G. W. (1989). Colonization of the porcine gastrointestinal tract by lactobacilli. Appl. Environ. Microbiol. 55, 279–283. doi: 10.1128/aem.55.2.279-283.1989
Perpetuini, G., Chuenchomrat, P., Pereyron, V., Haure, M., Lorn, D., Quan, L.-H., et al. (2021). Microorganisms, the ultimate tool for clean label foods? Inventions 6, 31. doi: 10.3390/inventions6020031
Preidis, G. A., and Versalovic, J. (2009). Targeting the human microbiome with antibiotics, probiotics, and prebiotics: gastroenterology enters the metagenomics era. Gastroenterology 136, 2015–2031. doi: 10.1053/j.gastro.2009.01.072
Quigley, J. (2011). Direct-Fed Microbials (Probiotics) in Calf Diets. Available online at: https://www.aphis.usda.gov/animal_health/nahms/dairy/downloads/bamn/BAMN11_Probiotics.pdf
Reid, G., Chan, R. C., Bruce, A. W., and Costerton, J. W. (1985). Prevention of urinary tract infection in rats with an indigenous Lactobacillus casei strain. Infect. Immun. 49, 320–324. doi: 10.1128/iai.49.2.320-324.1985
Resta-Lenert, S. (2003). Live probiotics protect intestinal epithelial cells from the effects of infection with enteroinvasive Escherichia coli (EIEC). Gut 52, 988–997. doi: 10.1136/gut.52.7.988
Riboulet-Bisson, E., Sturme, M. H. J., Jeffery, I. B., O'Donnell, M. M., Neville, B. A., Forde, B. M., et al. (2012). Effect of Lactobacillus salivarius bacteriocin Abp118 on the mouse and pig intestinal microbiota. PLoS ONE 7, e31113. doi: 10.1371/journal.pone.0031113
Russell, S. M., and Axtell, S. P. (2005). Monochloramine versus sodium hypochlorite as antimicrobial agents for reducing populations of bacteria on broiler chicken carcasses. J. Food Prot. 68, 758–763. doi: 10.4315/0362-028X-68.4.758
Scallan, E., Hoekstra, R. M., Angulo, F. J., Tauxe, R. V., Widdowson, M. A., Roy, S. L., et al. (2011). Foodborne illness acquired in the United States-major pathogens. Emerging Infect. Dis. 17, 7–15. doi: 10.3201/eid1701.P11101
Scharff, R. L. (2012). Economic burden from health losses due to foodborne illness in the United States. J. Food Prot. 75, 123–131. doi: 10.4315/0362-028X.JFP-11-058
Schillinger, U., Kaya, M., and Lücke, F.-K. (1991). The behavior of Listeria monocytogenes in meat and its control by a bacteriocin-producing strain of Lactobacillus sake. J. Appl. Bacteriol. 70, 473–478. doi: 10.1111/j.1365-2672.1991.tb02743.x
Seeley, H. W., and Del Rio-Estrada, C. (1951). The role of riboflavin in the formation and disposal of hydrogen peroxide by Streptococcus faecalis. J. Bacteriol. 62, 649–656. doi: 10.1128/jb.62.5.649-656.1951
Shuklov, I. A., Dubrovina, N. V., Kühlein, K., and Börner, A. (2016). Chemo-catalyzed pathways to lactic acid and lactates. Adv. Synth. Catal. 358, 3910–3931. doi: 10.1002/adsc.201600768
Simmering, R., and Blaut, M. (2001). Pro- and prebiotics - the tasty guardian angels? Appl. Microbiol. Biotechnol. 55, 19–28. doi: 10.1007/s002530000512
Sofos, J. N. (2008). Challenges to meat safety in the 21st century. Meat Sci. 78, 3–13. doi: 10.1016/j.meatsci.2007.07.027
Soltani, S., Hammami, R., Cotter, P. D., Rebuffat, S., Ben Said, L., Gaudreau, H., et al. (2021). Bacteriocins as a new generation of antimicrobials: toxicity aspects and regulations. FEMS Microbiol. Rev. 45, fuaa039. doi: 10.1093/femsre/fuaa039
Sonia, T. A., Ji, H., Hong-Seok, M., and Chul-Ju, Y. (2014). Evaluation of Lactobacillus and Bacillus-based probiotics as alternatives to antibiotics in enteric microbial challenged weaned piglets. African J. Microbiol. Res. 8, 96–104. doi: 10.5897/AJMR2013.6355
Stephens, T. P., Loneragan, G. H., Karunasena, E., and Brashears, M. M. (2007). Reduction of Escherichia coli O157 and Salmonella in feces and on hides of feedlot cattle using various doses of a direct-fed microbial. J. Food Prot. 70, 2386–2391. doi: 10.4315/0362-028X-70.10.2386
Stepper, J., Shastri, S., Loo, T. S., Preston, J. C., Novak, P., Man, P., et al. (2011). Cysteine S -glycosylation, a new post-translational modification found in glycopeptide bacteriocins. FEBS Lett. 585, 645–650. doi: 10.1016/j.febslet.2011.01.023
Stern, N. J., Svetoch, E. A., Eruslanov, B. V., Perelygin, V. V., Mitsevich, E. V., Mitsevich, I. P., et al. (2006). Isolation of a Lactobacillus salivarius strain and purification of its bacteriocin, which is inhibitory to Campylobacter jejuni in the chicken gastrointestinal system. Antimicrob. Agents Chemother. 50, 3111–3116. doi: 10.1128/AAC.00259-06
Stiles, M. E. (1996). Biopreservation by lactic acid bacteria. Antonie Van Leeuwenhoek 70, 331–345. doi: 10.1007/BF00395940
Sun, Z., Harris, H. M. B., McCann, A., Guo, C., Argimón, S., Zhang, W., et al. (2015). Expanding the biotechnology potential of lactobacilli through comparative genomics of 213 strains and associated genera. Nat. Commun. 6, 8322. doi: 10.1038/ncomms9322
Sutherland, C., Sim, C., Gleim, S., and Smyth, S. J. (2020). Consumer insights on Canada's food safety and food risk assessment system. J. Agric. Food Res. 2, 100038. doi: 10.1016/j.jafr.2020.100038
Tabe, E. S., Oloya, J., Doetkott, D. K., Bauer, M. L., Gibbs, P. S., and Khaitsa, M. L. (2008). Comparative effect of direct-fed microbials on fecal shedding of Escherichia coli O157:H7 and Salmonella in naturally infected feedlot cattle. J. Food Prot. 71, 539–544. doi: 10.4315/0362-028X-71.3.539
Tait, P., Saunders, C., Guenther, M., and Rutherford, P. (2016). Emerging versus developed economy consumer willingness to pay for environmentally sustainable food production: a choice experiment approach comparing Indian, Chinese and United Kingdom lamb consumers. J. Clean. Prod. 124, 65–72. doi: 10.1016/j.jclepro.2016.02.088
Tannock, G. W. (2004). A special fondness for lactobacilli. Appl. Environ. Microbiol. 70, 3189–3194. doi: 10.1128/AEM.70.6.3189-3194.2004
Teneva, D., Denkova, Z., Denkova-Kostova, R., Goranov, B., Kostov, G., Slavchev, A., et al. (2021). Biological preservation of mayonnaise with Lactobacillus plantarum LBRZ12, dill, and basil essential oils. Food Chem. 344, 128707. doi: 10.1016/j.foodchem.2020.128707
Thomas, C. M., Hong, T., van Pijkeren, J. P., Hemarajata, P., Trinh, D. V., Hu, W., et al. (2012). Histamine derived from probiotic Lactobacillus reuteri suppresses TNF via modulation of pka and erk signaling. PLoS ONE 7, e31951. doi: 10.1371/journal.pone.0031951
Tieking, M., Korakli, M., Ehrmann, M. A., Gänzle, M. G., and Vogel, R. F. (2003). In Situ production of exopolysaccharides during sourdough fermentation by cereal and intestinal isolates of lactic acid bacteria. Appl. Environ. Microbiol. 69, 945–952. doi: 10.1128/AEM.69.2.945-952.2003
Vachon, J. F., Kheadr, E. E., Giasson, J., Paquin, P., and Fliss, I. (2002). Inactivation of foodborne pathogens in milk using dynamic high pressure. J. Food Prot. 65, 345–352. doi: 10.4315/0362-028X-65.2.345
Valeriano, V. D. V., Balolong, M. P., and Kang, D.-K. (2017). Probiotic roles of Lactobacillus sp. in swine: insights from gut microbiota. J. Appl. Microbiol. 122, 554–567. doi: 10.1111/jam.13364
Valeur, N., Engel, P., Carbajal, N., Connolly, E., and Ladefoged, K. (2004). Colonization and immunomodulation by Lactobacillus reuteri ATCC 55730 in the human gastrointestinal tract. Appl. Environ. Microbiol. 70, 1176–1181. doi: 10.1128/AEM.70.2.1176-1181.2004
Van Heerde, H., Helsen, K., and Dekimpe, M. G. (2007). The impact of a product-harm crisis on marketing effectiveness. Mark. Sci. 26, 230–245. doi: 10.1287/mksc.1060.0227
Vargas, M. C. A., and Simsek, S. (2021). Clean label in bread. Foods 10, 2054. doi: 10.3390/foods10092054
Villegas, E., and Gilliland, S. E. (2006). Hydrogen peroxide production by Lactobacillus delbrueckii subsp. Lactis I at 5°C. J. Food Sci. 63, 1070–1074. doi: 10.1111/j.1365-2621.1998.tb15857.x
Watkins, B. A., Miller, B. F., and Neil, D. H. (1982). In vivo inhibitory effects of Lactobacillus acidophilus against pathogenic Escherichia coli in gnotobiotic chicks. Poult. Sci. 61, 1298–1308. doi: 10.3382/ps.0611298
Wheater, D. M., Hirsch, A., and Mattick, A. T. R. (1952). Possible identity of ‘Lactobacillin’ with hydrogen peroxide produced by lactobacilli. Nature 170, 623–624. doi: 10.1038/170623a0
Winkowski, K., Crandall, A. D., and Montville, T. J. (1993). Inhibition of Listeria monocytogenes by Lactobacillus bavaricus MN in beef systems at refrigeration temperatures. Appl. Environ. Microbiol. 59, 2552–2557. doi: 10.1128/AEM.59.8.2552-2557.1993
Wolfend, A. D., Vicente, J. L., Higgins, J. P., Andreatti Filho, R. L., Higgins, S. E., Hargis, B. M., et al. (2007). Effect of organic acids and probiotics on Salmonella Enteritidis infection in broiler chickens. Int. J. Poult. Sci. 6, 403–405. doi: 10.3923/ijps.2007.403.405
Wuytack, E. Y., Diels, A. M., and Michiels, C. W. (2002). Bacterial inactivation by high-pressure homogenisation and high hydrostatic pressure. Int. J. Food Microbiol. 77, 205–212. doi: 10.1016/S0168-1605(02)00054-5
Xie, L., and van der Donk, W. A. (2004). Post-translational modifications during lantibiotic biosynthesis. Curr. Opin. Chem. Biol. 8, 498–507. doi: 10.1016/j.cbpa.2004.08.005
Yang, G.-Y., Yu, J., Su, J.-H., Jiao, L.-G., Liu, X., and Zhu, Y.-H. (2017). Oral administration of Lactobacillus rhamnosus GG ameliorates Salmonella infantis-induced inflammation in a pig model via activation of the IL-22BP/IL-22/STAT3 pathway. Front. Cell. Infect. Microbiol. 7, 323. doi: 10.3389/fcimb.2017.00323
Yin, F., Farzan, A., Wang, Q., Yu, H., Yin, Y., Hou, Y., et al. (2014). Reduction of Salmonella enterica serovar Typhimurium DT104 infection in experimentally challenged weaned pigs fed a Lactobacillus-fermented feed. Foodborne Pathog. Dis. 11, 628–634. doi: 10.1089/fpd.2013.1676
Zakostelska, Z., Kverka, M., Klimesova, K., Rossmann, P., Mrazek, J., Kopecny, J., et al. (2011). Lysate of probiotic Lactobacillus casei DN-114 001 ameliorates colitis by strengthening the gut barrier function and changing the gut microenvironment. PLoS ONE 6, e27961. doi: 10.1371/journal.pone.0027961
Zhang, G., Ma, L., and Doyle, M. P. (2007). Salmonellae reduction in poultry by competitive exclusion bacteria Lactobacillus salivarius and Streptococcus cristatus. J. Food Prot. 70, 874–878. doi: 10.4315/0362-028X-70.4.874
Zhang, J., Liu, G., Li, P., and Qu, Y. (2010). Pentocin 31-1, a novel meat-borne bacteriocin and its application as biopreservative in chill-stored tray-packaged pork meat. Food Control 21, 198–202. doi: 10.1016/j.foodcont.2009.05.010
Zheng, J., Ruan, L., Sun, M., and Gänzle, M. (2015). A genomic view of lactobacilli and pediococci demonstrates that phylogeny matches ecology and physiology. Appl. Environ. Microbiol. 81, 7233–7243. doi: 10.1128/AEM.02116-15
Zheng, J., Wittouck, S., Salvetti, E., Franz, C. M. A. P., Harris, H. M. B., Mattarelli, P., et al. (2020). A taxonomic note on the genus Lactobacillus: Description of 23 novel genera, emended description of the genus Lactobacillus Beijerinck 1901, and union of Lactobacillaceae and Leuconostocaceae. Int. J. Syst. Evol. Microbiol. 70, 2782–2858. doi: 10.1099/ijsem.0.004107
Zhou, H., Xie, Y., Liu, H., Jin, J., Duan, H., and Zhang, H. (2015). Effects of two application methods of plantaricin BM-1 on control of Listeria monocytogenes and background spoilage bacteria in sliced vacuum-packaged cooked ham stored at 4°C. J. Food Prot. 78, 1835–1841. doi: 10.4315/0362-028X.JFP-14-594
Keywords: Lactobacillus, clean label antimicrobials, foodborne disease, food animal safety, preharvest and postharvest factors
Citation: Dewi G and Kollanoor Johny A (2022) Lactobacillus in Food Animal Production—A Forerunner for Clean Label Prospects in Animal-Derived Products. Front. Sustain. Food Syst. 6:831195. doi: 10.3389/fsufs.2022.831195
Received: 08 December 2021; Accepted: 08 March 2022;
Published: 29 April 2022.
Edited by:
Hsin-Bai Yin, Beltsville Agricultural Research Center, Agricultural Research Service (USDA), United StatesReviewed by:
Guadalupe Virginia Nevárez-Moorillón, Autonomous University of Chihuahua, MexicoSwarnalee Dutta, Jeonbuk National University, South Korea
Copyright © 2022 Dewi and Kollanoor Johny. This is an open-access article distributed under the terms of the Creative Commons Attribution License (CC BY). The use, distribution or reproduction in other forums is permitted, provided the original author(s) and the copyright owner(s) are credited and that the original publication in this journal is cited, in accordance with accepted academic practice. No use, distribution or reproduction is permitted which does not comply with these terms.
*Correspondence: Anup Kollanoor Johny, YW51cGpvaG5AdW1uLmVkdQ==