- 1Department of Mechanical Engineering/Environmental Technology, OTH Amberg-Weiden, Amberg, Germany
- 2Department of Process Engineering, Nuremberg Tech, Nuremberg, Germany
- 3Chair of Food Biotechnology and Food Process Engineering, Technische Universität Berlin, Berlin, Germany
Extracellular polysaccharides, or exopolysaccharides are high–molecular weight sugar-based polymers expressed and secreted by many microorganisms. As host organisms, the functions of exopolysaccharides are diverse, ranging from physical protection via biofilm formation, adhesion, and water retention to biological functions that are not entirely understood such as viral attachment inhibition. Industrial applications of exopolysaccharides can be found in food texture modification; for example, utilizing the hydrocolloidal properties of exopolysaccharides for thickening and gelling purposes to improve food quality and texture. Over the last decade, biologically active exopolysaccharides produced by microalgae have received attention for their potential as antiviral, antibacterial and antioxidative compounds and in the applications. However, relatively low yield and productivity are the limiting factors for full-scale industrial application. In this study, the well-known prokaryotic phototrophic microorganism Arthrospira platensis and the comparatively unknown eukaryotic unicellular green alga Chlamydomonas asymmetrica were used to evaluate the influence of different process parameters on exopolysaccharides formation and productivity. In addition to the essential control variables (light and temperature), the influence of operational techniques (batch and turbidostat) were also investigated. Although the two studied algae are differently affected by above parameters. The light intensity was the most influential parameter observed in the study, leading to differences in exopolysaccharides concentrations by a factor of 10, with the highest measured concentration for A. platensis of cEPS = 0.138 g L−1 at 180 μmol m−2 s−1 and for C. asymmetrica of cEPS = 1.2 g L−1 at 1,429 μmol m−2 s−1. In continuous systems, the achieved exopolysaccharides concentrations were low compared to batch process, however, slightly higher productivities were reached. Regardless of all screened process parameters, C. asymmetrica is the better organism in terms of exopolysaccharides concentrations and productivity.
Introduction
Polymers based on sugars are well-known for their high diversity in chemical composition and secondary structure. The innumerable chemo-diversity based on glycosidic linkage of different sugars, as well as many modifications containing non-sugar constituents (sulfates, organic acid, methyl, amino acids, or amine), leads to a big variety of possible structures (Delattre et al., 2016). The polysaccharides (PS) have diverse structure and architecture based on their type and origin. PS have natural biological functions, starting from energy storage over structural support and cell adherence to protect the organism from various endangerments, e.g., heavy metal toxicity, desiccation, radiation, oxidation, viral infections, and predators (Li et al., 2001; Bazaka et al., 2011; Reichert et al., 2017; Fritzsche et al., 2021; Potnis et al., 2021). The protective properties are mainly found in the secreted PS, known as extracellular polysaccharides (EPS). This sub-category of PS can further be divided into cell-bound polysaccharides (BPS) and released polysaccharides (RPS) (Cruz et al., 2020). Besides EPS, PS are also present inside the cells, known as intracellular polysaccharides (IPS). Due to thickening, gelatinous properties and secondary functions, including emulsification, suspension stabilization, encapsulation, flocculation, film-forming and coating, PS are used in the food and cosmetic industries and also in several other processes where the rheological properties of water have to be altered for easier processing or higher commercial value (Sanford and Baird, 1983; Lewis et al., 2000; Paniagua-Michel et al., 2014; Kumar et al., 2018; Gouda et al., 2022). The biological functions are becoming increasingly interesting for commercial application, particularly in the field of nutraceuticals and therapeutics. Recent discoveries of the antioxidative, immunomodulatory, antitumor, antithrombotic, anticoagulant, anti-inflammatory, antimicrobial, and antiviral activities of PS are of particular interest (de Jesus et al., 2019). Whereas, PS from terrestrial plants, seaweed, fungi and non-phototrophic microorganisms are widely described in the literature, detailed information on chemical structure, rheologic properties, possible industrial relevance (i.e., information regarding process parameters and productivity) of PS obtained from microalgae and cyanobacteria are harder to find (Prybylski et al., 2020), which could be due to lower productivities of phototrophic microorganisms.
EPS from microalgae and cyanobacteria are mainly non-repeating, hetero-polysaccharides with glycosidic bonds to non-carbohydrate components. Pierre et al. (2019) attempted to summarize the available information about the structural deviation of EPS gained from microalgae and cyanobacteria. For phylogenetically closed eukaryotic microalgae, the group concluded some general information about the chemical composition of EPS; however, such statements are less clear for cyanobacteria. The intragenus heterogeneity of EPS composition is highly distinctive (Gaignard et al., 2019; Prybylski et al., 2020) and the chemical diversity is also reflected by the quantity of published biological activity of EPS, occasionally even hypothesizing structure-function relationships.
As the efficacy of these biopolymers is significant (Li et al., 2001; Bazaka et al., 2011; Reichert et al., 2017), the question arises why their commercial application, especially in the field of therapeutics, is not yet realized. One main reason lies in the economic perspective of the production process as the yield of EPS from microalgae and the cost for the upstream and downstream processing are not yet economically been found feasible and are currently the object of research projects, including Spiralg (BBI-H2020) or AnViPi (FNR Germany). The common aspect in these projects is the implantation of EPS production into a product cascade leading to a phase II biorefinery, which describes the concept of making use of side streams and byproducts to gain multiple end products from the biomass. Nevertheless, even if PS serve “only” as an added-value product, the question arises, which process parameters influence productivity or space-time yield to further increase the benefit of the process?
Regardless of whether microalgae and cyanobacteria grow by phototrophic, heterotrophic, or mixotrophic processes, the composition of the culture media has a significant influence on EPS production. Until now, the main focus of investigations was on the supply of the elements N, P, and S. It was found that starvation of these three nutritional components led to a higher expression of EPS into the supernatant, while the associated reduction of growth determines the overall productivity. Researchers reported optimal parameters for the supply of carbon with air being enriched with ~2% CO2 (Iqbal and Zafar, 1993; Cheng et al., 2015).
For phototrophic cultures, light supply is one of the critical process parameters concerning all products from microalgae or cyanobacteria. Generally, there is a positive effect of high light intensity on EPS (Delattre et al., 2016). When comparing different reports, one could conclude that EPS production is enhanced at low irradiations by increasing the light supply, leading to a light inhibition at high light intensities (Trabelsi et al., 2009; Delattre et al., 2016; Silva et al., 2020). The optimum light supply strongly depends on the production organism (Trabelsi et al., 2009; Silva et al., 2020) and the reactor system (Iqbal and Zafar, 1993).
The influence of temperature on productivity is not as easy to describe and is highly species- and strain-dependent. Notably, optimum temperature for EPS production does not always overlap with the optimum culture growth temperature. Botryococcus braunii is described as being highly dependent on a narrow temperature window in which, both fast growth and high EPS production, can be achieved (Iqbal and Zafar, 1993). For Anabaena sp., it was observed that a sudden increase in temperature from 30 to 35°C (optimum growth) to 40–45°C increased (4–5-fold) the release of EPS. Similar behavior is described for Dictyosphaerium chlorelloides, where an increase of 5°C decreases the growth by 30% but doubles the EPS production (Kumar et al., 2017). Other very relevant studies regarding the influence of light and temperature on EPS production for photoautotrophic growing microorganisms have been published. An overview of some outcomes is provided in Table 1.
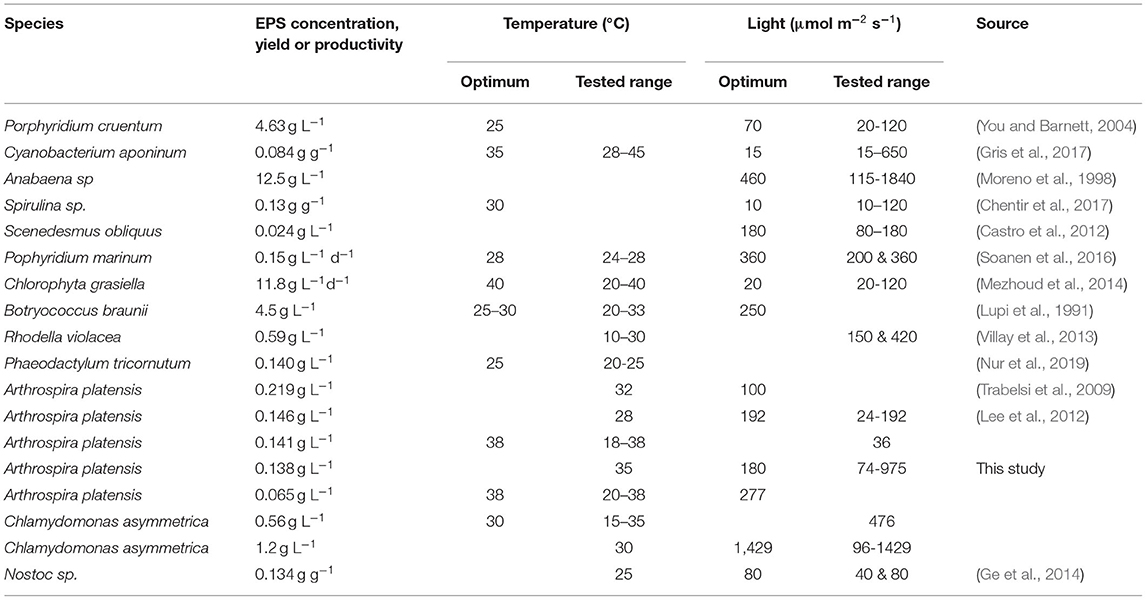
Table 1. Influences on light and temperature on EPS production in different photoautotrophic microorganisms.
In this study, the prokaryotic microalga Arthrospira platensis and the eukaryotic unicellular green alga Chlamydomonas asymmetrica were used to identify the influence of process parameters and operational techniques on the formation and productivity of EPS, hoping to find some intragenus trends by investigating to microorganism from different domains.
Arthrospira platensis is a well-known and studied cyanobacteria, limited information has been published about C. asymmetrica, a recent study by group of researchers shows its prospect as a lutein production organism, reporting lutein concentrations of up to 30 mg per gram dry biomass (Hahm et al., 2021).
Materials and Methods
Microalgae Strain and Cultivation
The cyanobacterium Arthrospira platensis (NIES-39) was obtained from the National Institute for Environmental Studies (NIES Collection, Tsukuba, JAPAN) and cultivated in SOT-media according to the formulation recommended by NIES (Ogawa and Terui, 1970). The green alga Chlamydomonas asymmetrica was self-isolated from freshwater habitats in South Korea. The cultivation medium of C. asymmetrica was the artificial fresh water medium (AF6), according to the formulation of Watanabe (Andersen et al., 2005).
The precultures of both strains were cultivated in shake flasks with a liquid height of approximately 3 cm (T = 25°C, PFD = 250 μmol m−2 s−1). For inoculation of the main experiments, biomass from the late exponential phase of the preculture was diluted to a starting optical density (OD) of 0.1. For the cultivation, cylindrical bubble column reactor systems (volume = ca. 800 mL, diameter = 5 cm) were used. The aeration was kept constant at approximately 1.0 vvm with sterile compressed air. For the experiments with C. asymmetrica, aeration occurred with air enriched with 3% CO2. Light was supplied continuously (24 h) from 4 directions (Mitras LB2, 60 daylight, GHL), and the intensity was measured at nine different points on the reactor surface using a light meter (LI-250A, LI-COR). The irradiation varied from 50 to 975 μmol m−2 s−1 for A. platensis and from 96 to 1,429 μmol m−2 s−1 for C. asymmetrica. The temperature ranged between 15 and 35°C for C. asymmetrica and 25–38°C for A. platensis. For temperature measurement, a combined pH/temperature electrode was placed in the reactor (Polilyte plus, Hamilton). The continuous reactors were controlled by an adjustable feed pump (SK-BQ50, Longer Precision Pump Co., Ltd, CHN) and a level controller. Samples were taken once the culture reached an equilibrium state for a set of parameters. In this study the results of EPS concentration, yield or space-time yields represent the average of three biological and a minimum three technical replicates and the error bars indicate the standard deviation with n > 9.
Biomass and EPS Quantification
For the tracking the culture development, the biomass was quantified in terms of OD at 750 nm [UV-1800 (Shimadzu), UV7 spectrometer (Mettler Toledo)], with the pure medium used as a blank value. Measurement at 750 nm avoids interference with light absorption of by cellular pigments (chlorophyll, carotenoids, and phycobilins). The OD values were calibrated to the dry biomass weight for each used photometer and species. The growth rates (μ [d−1]) were calculated by fitting the experimental data from the batch experiments to an empirical growth model (Jung et al., 2021). To ensure comparability of single cultivations with different process parameters, the model was fitted to all cultivations using a global light affinity constant KS and a temperature-dependent μmax. The light affinity constant (KS) for the two algae is different but assumed to be constant for different temperatures and light intensities. In the model used here, the light dependency of μ is supposed to follow a Monod kinetic so that the overall average growth rate (μmax) is global for different light intensities. The relative R2 combined with the standard percentage error (SPE) are used as an optimality criterion (Table 2).
The EPS quantification was conducted using a modified method of the total carbohydrate quantification developed by DuBois et al. (1951) and Nielsen (2003). Cell-free supernatant was used for the measurement so that the detected carbohydrates can be correlated to the RPS. Even though the total carbohydrate content in the media increases when the culture remains in the stationary phase, the result in this publication refer to the early stationary phase to avoid biases by leakage of intracellular polysaccharides (IPS).
Samples taken from the photobioreactors during cultivation were centrifuged (4,500 g, 10 min) and the cell-free supernatants were kept frozen (−20°C) for further analysis. For the Dubois assay, 160 μL of supernatant was added to 1.5 mL safety reaction tubes along with 160 μL of phenol solution (5 wt. %). Then, 800 μL of concentrated sulfuric acid (>95%) rapidly added to the mixture by pipetting. Reproducible mixing was ensured by sucking the reaction mixture into the pipette and ejecting it back into the reaction tube. The mixing was repeated three times. After incubation at room temperature (10 min., ca. 21°C), the reaction took place at 30°C for 20 min before recording the absorbance spectra between 190 and 1,100 nm using spectrophotometer (Mettler Toledo, UV7). From this spectrum the spectrum of deionized water was subtracted as a blank. Since the method requires a calibration in terms of a reference carbohydrate, aqueous glucose standards were analyzed along with the samples. The absorbance at 490 nm served as a reference for quantification. For the subsequent analysis, the raw spectra were smoothed with a triangular filter and then approximated with finite difference derivation.
During the Dubois assay, ionic species of nitrous oxides, namely nitrate (NO) and especially nitrite (NO), also participate in reactions with phenol and form products with high absorbance over the full VIS-region of the spectrum. The assay was carried out for aqueous mixtures of glucose, fructose, sodium nitrate, and sodium nitrite to correct their influences. Afterward, the spectra of glucose and fructose, calculated from a calibration using pure aqueous standards, were subtracted from the processed spectrum of the sample to obtain the background caused by NO and NO. Using the spectral region with wavelengths above 600 nm, which is unaffected by the carbohydrate spectra, the obtained background at 490 nm was fitted to two characteristic points from the original spectra, by a combination of weighted multiple linear regression and an evolutionary algorithm for wavelength selection. With this method, the best fit (wR2 = 0.9929, wQ2 = 0.9900) was found to be:
The first summand of Equation 1 accounts for both NO and NO, whereas the second summand mainly considers NO, whose reaction products dominate the first order derivative of the spectra. For quantification purposes, Equation 1 is applied to correct the measured absorbance at 490 nm before calculating the total carbohydrate concentration from a calibration with aqueous glucose standards, which leads to Equation 2. The protocol of the Dubois assay remains unchanged.
The results of the EPS measurement is given in three different forms: the EPS concentration (cEPS) in g L−1; the specific product yield coefficient (YEPS/X) in g g−1, which calculates by the quotient of the EPS concentration and the measured dry biomass concentration (χ); and—as an indicator for productivity—the space-time yield of EPS (STYEPS) in g L−1 d−1 (Equation 3).
For batch processes delta (Δ) refers to the differences between inoculum and measurement. For continuous systems, the time difference is the hydraulic retention time, and cEPS refers to the difference between the concentrations at the inlet and outlet of the reactor. In this study the results of EPS concentration, yield or space-time yields represent the average of three biological and a minimum three technical replicates and the error bars indicate the standard deviation with n > 9.
Results
Growth Characterization
To avoid a false interpretation of the EPS concentration, the provided data of the batch experiments are derived from measurements in the early stationary phase, right after transmission from the exponential phase.
Figure 1 shows examples of A. platensis and C. asymmetrica growth to indicate the sampling point for EPS. The growth rates in this study refer to the highest achieved growth rates (μ) without light limitation, evaluated by fitting the experimental values to the model of Jung et al. (2021). The results and the quality of the fitting are summarized in Table 2.
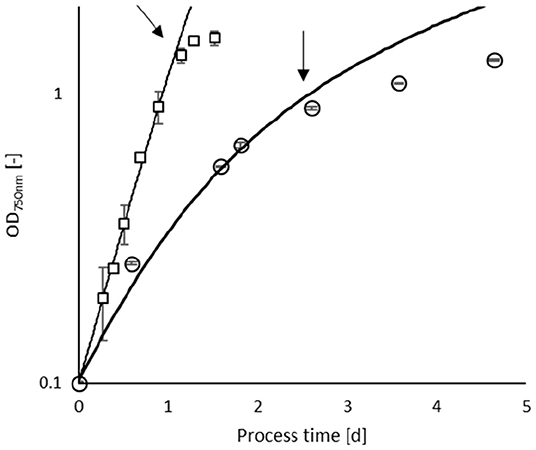
Figure 1. Culture development of Arthrospira platensis (open circles) cultivated at 35°C and 180 μmol m−2 s−1 and C. asymmetrica (open squares) at 30°C and 1,429 μmol m−2 s−1. The error bars indicate the standard deviation of three independent measurements. The solid lines indicate the simulation of the growth behavior. The arrows indicate the beginning of the stationary phase, where sampling was carried out.
Temperature Effects
The effect of temperature on the growth rate of A. platensis was determined between 20 and 38°C at a constant light intensity of 277 μmol m−2 s−1 (Figure 2A). The highest specific growth rate was observed at 38°C (μ= 1.98 d−1). With decreasing temperature, the specific growth rate steadily declines to 1.32 d−1 at 25°C, and then drops to 0.4 at 20°C. Due to the low growth at 20°C, the comparably high EPS concentrations of cEPS= 0.051 g L−1 (where YEPS/X = 0.053 g g−1) resulted in low EPS productivities of STYEPS = 0.003 g L−1 d−1.
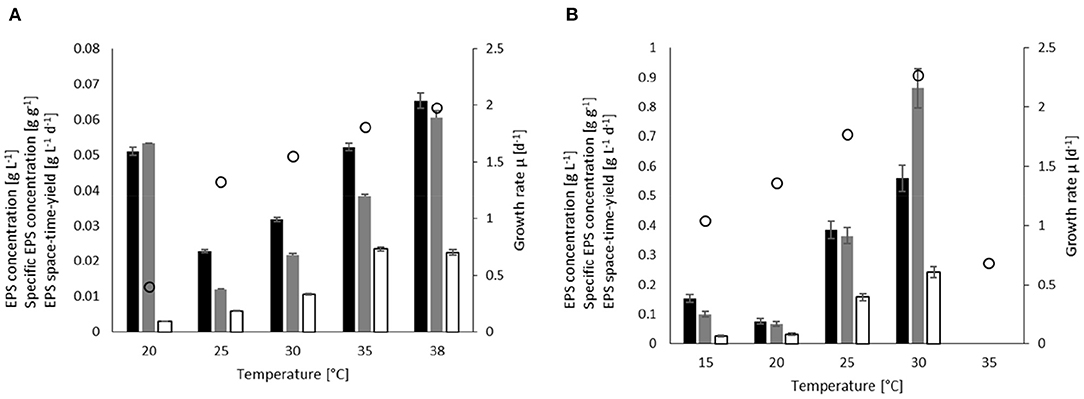
Figure 2. Effect of temperature on the EPS concentration (black), EPS yield (gray), space-time yield (white) and specific growth (white circles) in batch processes for (A) A. platensis cultivated at 277 μmol m−2 s−1, and (B) C. asymmetrica cultivated at 476 μmol m−2 s−1.
At temperature above 20°C, the EPS concentration and YEPS/X steadily increased from cEPS = 0.023 g L−1 (YEPS/X = 0.012 g g−1) at 25°C to cEPS = 0.065 g L−1 (YEPS/X = 0.066 g g−1) at 38°C. It should be noted that determined EPS yield/concentration at 20°C must be considered uncertain as the culture contained a lot of cell debris and small broken cells so that the quantified carbohydrates do not necessarily represent released EPS. The space-time yields also enhanced with increasing temperature and reached plateaus at approximately STYEPS = 0.02 g L−1 d−1 for the two highest temperatures.
The experiments for determining the temperature influence on C. asymmetrica (Figure 2B) were performed at a light intensity of 476 μmol m−2 s−1. The green alga achieves slightly higher growth rates compared to A. platensis with an optimum temperature at ~30°C with μ = 2.27 d−1. Higher temperatures result in a substantial reduction of growth to μ = 0.7 d−1 at 35°C. While with decrease in the temperature, growth reduces steadily to a rate of 1.0 d−1 at 15°C.
The EPS concentration and yield at 15°C were higher than at 20°C, whereas the space-time yield at 20°C was 20% higher than at 15°C. The highest EPS concentration was measured at 30°C with cEPS = 0.56 g L−1, resulting in YEPS/X = 0.86 g g−1 and STYEPS = 0.24 g L−1d−1. It is worth mentioning that the temperature had the most substantial effect on EPS yield, resulting in a more than 10-fold increase compared to the lowest value obtained at 20°C (YEPS/X = 0.067 g g−1). Due to the high level of cell breakage in the experiments at 35°C, the carbohydrate quantification of the supernatant at this temperature is not displayed.
Light Effects
The experiments to investigate the influence of light on EPS production were conducted at 30°C for C. asymmetrica cultures and 35°C for A. platensis cultures. As the light supply in algae cultures is not a static parameter but is strongly influenced by the growing biomass (self-shading effects), different process modes were investigated. Batch processes, or repeated batches, are the most common production mode as the process control is straightforward. Furthermore, due to high biomass and product concentrations reached in batch processes, downstream processing is often more economical. Nevertheless, the drawback of this cultivation method is the changing biomass concentration and, hence, the impracticality of establishing a causal relation between light supply and product formation. Turbidostat process control overcomes these issues, as the culture is kept at a constant biomass concentration and, therefore, maintains a constant influence of self-shading. A constant biomass concentration of 1 g L−1 was chosen in our experiments.
Batch Cultivation
In the investigated range of light intensities, the influence of the light intensity on the growth rate of A. platensis (Figure 3A) can be described with the Monod kinetics, choosing the light supply as the limiting substrate. Using the kinetic model introduced by Jung et al. (2021), the biomass development of all cultures can be simulated using KS = 61.2 μmol m−2 s−1 and μmax = 2.39 d−1. The best match between model and experiments was achieved for the cultivation at 277 μmol m−2 s−1.
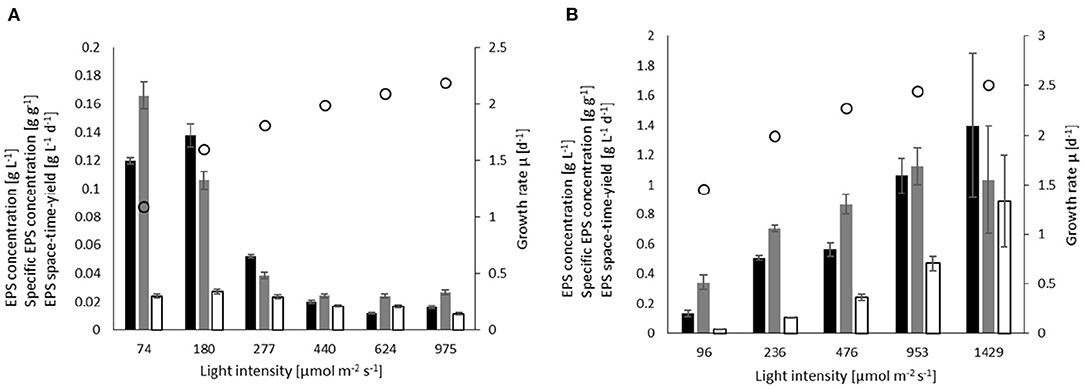
Figure 3. Influence of light on the EPS concentration (black), EPS yield (gray), space-time yield (white), and specific growth (white circles) in batch processes for (A) A. platensis cultivated at 35°C, and (B) C. asymmetrica cultivated at 30°C.
The highest EPS concentration at low light intensities was 0.138 g L−1 for cultivation at 180 μmol m−2 s−1, whereas in the cultivation at 74 μmol m−2 s−1 the highest EPS yield observed was 0.166 g g−1. The EPS concentration and yield at these low light intensities were 10-fold higher than in the experiments with irradiation of 440 μmol m−2 s−1 and above. The influence of light intensity on the space-time yield of EPS is not as strong, with the highest value of 0.024 g L−1 d−1 observed at 180 μmol m−2 s−1, and the lowest value of 0.012 g L−1 d−1 at 975 μmol m−2 s−1.
Chlamydomonas asymmetrica (Figure 3B) has a slightly higher light affinity (compared to A. platensis) with KS = 87 μmol m−2 s−1. The volume-averaged growth rate was estimated to be μmax = 2.64 d−1. The highest growth rate was 2.5 d−1 at 1,429 μmol m−2 s−1. At a light intensity of 96 μmol m−2 s−1, the culture still achieved a growth rate of 1.5 d−1. Light positively influences EPS production, leading to higher concentrations, yields, and space-time yields. The concentration increases approximately by a factor 10 from cEPS = 0.13 g L−1 at 96 μmol m−2 s−1 to cEPS = 1.4 g L−1 at the highest light intensity. As a consequence of the higher concentrations and growth rates, the space-time yield increases by a factor of 30, reaching STYEPS = 0.84 g L−1d−1 at 1,429 μmol m−2 s−1. The maximum EPS yield is obtained at 953 μmol m−2 s−1 where YEPS/X = 1.12 g g−1, which corresponds to a 3.2-fold increase compared to that at the lowest light intensity.
The separate investigations on the parameter influence of temperature and light on EPS production with C. asymmetrica lead to a combined optimum of 30°C and 1,429 μmol m−2 s−1. For A. platensis, the light intensity screening was not conducted at the suggested optimum temperature of 38°C; instead at 35°C. Additional cultivations using the combination of the indicated optimal parameters (e.g., 38°C and 180 μmol m−2 s−1) led to a concentration of cEPS = 0.116 g L−1, a yield of YEPS/X= 0.098 g g−1 and a space-time yield of STYEPS = 0.0259 g L−1d−1, which are just slightly lower than the values obtained at 35°C at the same light intensity.
Continuous Process
As steady-state conditions are not reached in batch cultures, conclusions about parameter influences may be strongly biased to the culture's state at the time of sampling. For example, the light availability in the culture decreases with increasing biomass concentration due to self-shading effects.
To overcome this, the influence of light supply was additionally investigated using a continuous, turbidostat process mode, with constant biomass concentration at 1 g L−1, which was ensured by regulating the dilution rate.
Unlike in the batch experiments, the turbidostat cultures of A. platensis showed no significant influence of light on the EPS concentration (average cEPS = 0.056 g L−1 ± 0.0036) and EPS yield (average YEPS/X = 0.059 g g−1 ± 0.0041) (Figure 4A). Compared to the batch experiments, this results in a decrease in EPS concentration and yield of more than 50%. For light intensities at ~450 μmol m−2 s−1, the EPS concentration and yield in the continuous process is about twice as high as in batch cultures. At this light intensity, the space-time yield in the turbidostat experiments reached the highest value of 0.080 g L−1 d−1, which is significantly higher than the space-time yield achieved in batch cultivations at comparable light intensities.
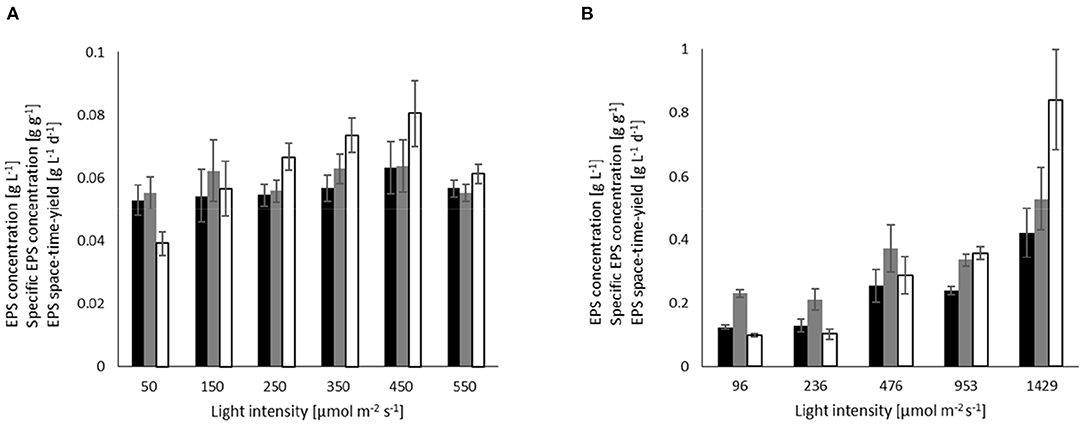
Figure 4. Influence of light on the EPS concentration (black), EPS yield (gray), and space-time yield (white) in turbidostat processes at a biomass concentration of 1 g L−1 for (A) A. platensis cultivated at 35°C, and (B) C. asymmetrica cultivated at 30°C.
Chlamydomonas asymmetrica similarly response to light intensities in the continuous processes (Figure 4B) as observed in the batch cultivations. Accordingly, EPS concentration, yield, and space-time yield increase with stronger irradiation. At 1,429 μmol m−2 s−1, the EPS concentration (cEPS = 0.056 g L−1) is only one-third, and the yield (YEPS/X = 0.52 g g−1) is half of the respective values in the batch culture. However, the space-time yield in both cultivation systems is comparable to each other and takes values of 0.84 g L−1 (conti) and 0.88 g L−1 (batch), respectively.
Discussion
The effects of light and temperature on EPS production were evaluated in two different phototrophic organisms, the cyanobacterium A. platensis, and the green alga C. asymmetrica. Both process parameters influenced the amount of produced EPS and differ for both organisms. To estimate the comparability of different parameter settings regarding EPS production, we fitted the results of the growth behavior with the growth model of Jung et al. (2021). The significant fit of the model to the experimental data, using the same kinetic parameters (, μmax) for all temperature and light intensity variations, indicates a low bias to other influencing factors. When evaluating all experiments by comparing the experimental biomass development of the cultures with the growth model, a significance level of P < 0.005 is estimated for the experiments with A. platensis and C. asymmetrica, by using the Chi-square test (χ2 = 1.2, n = 63) The residuals between the model and the experimental data are independent and well-distributed. Regarding the growth behavior of A. platensis, in this study the evaluated growth parameter KS (61,2 μmol m−2 s−1) is similar to those published by Levert and Xia (2001) and Cornet and Dussap (2009) with around 80 μmol m−2 s−1. Using a KS value of 80 μmol m−2 s−1 for fitting our data (μmax remain unchanged) leads to insignificant changes of the quality of the fit. For example, it was observed that an increase of the quality of the fit by 5 percent points (average relative error) for the culture 975 μmol m−2 s−1 and 35°C. Fitting the model to the experimental data for culture growth at 25°C and 277 μmol m−2 s−1 lead to a 3 percent point decrease of the average relative error. The maximum growth rate at 35°C is estimated by the model to μmax = 2.12 d−1, which is in agreement with the values given from Huang and Chen, who reported global growth rate of μmax = 2.0 d−1 (Huang and Chen, 1986), and the growth rate published by Lee et al. with μmax = 2.06 d−1 (Lee et al., 1987). The standard textbook for A. platensis cultivations from Vonshak states the optimum growth temperature window between 35 and 38°C (Vonshak, 1997), which is congruent to the data of current study. In the current study, the growth reduced by 42% comparing the highest and the lowest growth rate, the same reduction in growth by temperature is reported by De Oliveira et al. (1999), investigating a temperature range (20–35°C).
In the case of C. asymmetrica, such a comparison with literature data is strongly biased, as the only published growth parameters regarding this green alga originates from the authors of this study. However, regarding an evaluated culture growth of μ≈2.5 d−1 at optimum conditions, a light-dependent growth being describable by Monod kinetics with an optimal growth temperature of 30°C is unexceptional and lies within an expectancy range (Lee et al., 2015). Thus, the goodness-of-fit of the model and the comparability of the current findings and growth data to published data leads to the conclusion that the growth behaviors and culture developments observed in these experiments are plausible.
Regarding the EPS production of both investigated microalgae, it should be stated that C. asymmetrica achieves 10-fold higher EPS concentrations than A. platensis (up to cEPS = 1.4 g L−1), yields (up to YEPS/X = 1.12 g g−1) and space-time yields (up to STYEPS = 0.84 g L−1 d−1). These high values for EPS production are similar to those described for Phorphyridium sp. with concentrations cEPS> 1 g L−1 (Singh et al., 2000) but are even surpassed by the EPS production of Botryococcus braunii, which is reported with cEPS = 4.5 g L−1 (Lupi et al., 1991). Comparing EPS data with literature can be error-prone, as the culture conditions or age at harvesting are often not clearly stated. The measurable carbohydrates in the supernatant increase with cell breakage, especially noticeable with prolonged retention in the stationary phase. Margo et al. reported for A. platensis a 2-fold rise in EPS concentration compared to early stationary phase (cEPS = 0.055 g L−1 and death phase (cEPS = 0.108 g L−1) (Magro et al., 2018). The data from the early phase, as well as the EPS concentrations published by Strieth et al. (Strieth et al., 2020), are a similar range as the concentrations in current experiments (0.012–0.138 g L−1) reported here.
Although the effect of temperature on EPS production is known to be inconsistent between different organisms (Delattre et al., 2016), in current experiments, increasing temperature had a positive influence on EPS production by A. platensis and C. asymmetrica. In a study about exopolysaccharides from cyanobacteria, Nicolaus et al. (1999) described for A. platensis that in the absence of NaCl the EPS production slightly decreased with increasing temperatures, which is contradictory to the current findings and those of Trabelsi et al. (2009) and Lee et al. (2012). This may lead to the conclusion that the absence of NaCl was the primary influencing factor. Trabelsi et al. and Lee et al. investigated the influence of light and temperature on EPS production. Both publications show increasing EPS release at higher cultivation temperatures. The highest concentration reported by Trabelsi et al. is cEPS = 0.297 g L−1 at 35 °C and 180 μmol m−2 s−1, whereas Lee et. al reached cEPS = 0.141 g L−1 at 38°C and 36 μmol m−2 s−1 (Trabelsi et al., 2009; Lee et al., 2012). Both findings are comparable to the current study of EPS concentrations of cEPS = 0.138 g L−1 at 35°C and 180 μmol m−2 s−1. The differences in the total amount of EPS may originate in the different harvesting times. Our experimental setup was designed to harvest at the early stationary phase, not to be biased by the release of IPS due to cell lysis. In contrast, the previous report used data form the late stationary phase. The comparison between growth and EPS concentration published by Trabelsi et al. perfectly shows the accelerated increase of EPS concentration after reaching the stationary phase, where biomass starts declining (Trabelsi et al., 2009). Studies on the temperature dependency of the cyanobacterium Cyanobacterium aponinum showed that the highest EPS productivity was at 35°C (STYEPS~9 mg L−1 d−1), whereas the highest growth rate was at 40°C. Although, the productivity of A. platensis is about twice as high (STYEPS = 24 mg L−1 d−1) at similar culture conditions (76 μmol m−2 s−1, 35°C), the observed trend of reaching the highest EPS productivity at slightly lower temperatures than the optimum growth is similar (Gris et al., 2017).
In the well-researched review by Delattre et al. a general rule-of-thumb was formulated: higher light intensities lead to higher EPS release (Delattre et al., 2016). Results of current study regarding the influence of light on EPS production by C. asymmetrica are supporting this statement. In both experimental approaches, the batch and the turbidostat processes with increase of illumination in the range of 96–1,429 μmol m−2 s−1 enhanced EPS production in terms of concentration, yield, and space-time yield. However, the results obtained for A. platensis showed a different relationship with respect to light than previously described, Trabelsi et al. were able to show a significant increase of EPS-production with stronger illumination in the range between 50 and 180 μmol m−2 s−1. A similar range was investigated by Lee et al. (2012) by observing a maximum EPS release at 180 μmol m−2 s−1 with cEPS = 0.146 g L−1. This is in accordance with the findings of Dejsungkranont et al., who proposed an optimum light intensity of 203 μmol m−2 s−1 for EPS production (Dejsungkranont et al., 2017). Those results support our findings that the optimum light intensity is around 100–200 μmol m−2 s−1 with maximum EPS concentrations of cEPS = 0.138 g L−1 The significant decrease in EPS production by increasing irradiation in the range of 180–975 μmol m−2 s−1 correlates with the findings of Silva et al., who reported a reduction in EPS yield from YEPS/X = 0.12 g g−1 at 200 μmol m−2 s−1 to YEPS/X = 0.054 g g−1 at 1,000 μmol m−2 s−1 (Silva et al., 2020). In different experiments of current study, the specific EPS concentration dropped from YEPS/X = 0.106 g g−1 at 180 μmol m−2 s−1 to YEPS/X = 0.026 g g−1 at 975 μmol m−2 s−1. It should be mentioned here that the highest specific EPS concentration was reached at 74 μmol m−2 s−1 with YEPS/X = 0.166 g g−1.
Interestingly, the experiments in the continuous processes showed a lower light influence on EPS concentration and yield than in the results of the batch experiments, with a slight positive effect on the space-time yield, which can be explained by the higher dilution rates. Comparing the two process modes, the results of current study indicate that higher EPS concentrations can be achieved compared to continuous systems. The cultivation of A. platensis at higher light intensities (above 180 μmol m−2 s−1), is an exception, since the continuous system performed better under these conditions. Nevertheless, this strongly resembles the Gaden type III category of processes, traditionally operated in batch mode (Gaden, 2000).
Conclusions
For A. platensis, the light intensity with the highest EPS release was found to be 180 μmol m−2 s−1. Stronger irradiations resulted in a substantial decrease in EPS production. The experiments regarding the temperature influences, conducted at a light intensity of 277 μmol m−2 s−1, suggested an optimum for EPS production at 38°C. However, the best combination of process conditions was found to be 180 μmol m−2 s−1 and 35°C with cEPS = 0.138 g L−1. The optimum parameter combination for C. asymmetrica, according to our results, is 30°C and 1,429 μmol m−2 s−1 where an EPS concentration of was reached. Combining the high EPS concentrations with the fast growth, C. asymmetrica is the better production organism, reaching 30 times the space-time yield of A. platensis with STYEPS = 0.86 g L−1 d−1. Although the turbidostat process with C. asymmetrica led to the highest space-time yield in our experiments, continuous systems are generally not suitable for high production rates—especially at low biomass concentrations.
Data Availability Statement
The raw data supporting the conclusions of this article will be made available by the authors, without undue reservation.
Author Contributions
S-HJ, NZ, FB, CM, and CL planned and designed the research and interpreted the results. S-HJ, NZ, and FB performed the experiments and proceeding of the raw data. S-HJ and CL wrote the manuscript. S-HJ, NZ, FB, UT, CR, CM, and CL revised the manuscript. All authors read and approved the final manuscript.
Funding
This work was funded by the German Federal Ministry for Education and Research (BMBF 03SF0457) and the German Federal Ministry of Food and Agriculture (FNR 22017518 and 2219NR287).
Conflict of Interest
The authors declare that the research was conducted in the absence of any commercial or financial relationships that could be construed as a potential conflict of interest.
Publisher's Note
All claims expressed in this article are solely those of the authors and do not necessarily represent those of their affiliated organizations, or those of the publisher, the editors and the reviewers. Any product that may be evaluated in this article, or claim that may be made by its manufacturer, is not guaranteed or endorsed by the publisher.
References
Andersen, R. A., Berges, J. A., Harrison, P. J., and Watanabe, M. M. (2005). Recipes for freshwater and seawater media. Algal Cult. Tech. 429–538. doi: 10.1016/B978-012088426-1/50027-5
Bazaka, K., Crawford, R. J., Nazarenko, E. L., and Ivanova, E. P. (2011). Bacterial extracellular polysaccharides. Adv. Exp. Med. Biol. 715, 213–226. doi: 10.1007/978-94-007-0940-9_13
Castro, N., Jaramillo, A., Prieto, R., and Marino, G. (2012). Analysis of the effect of the interactions among three processing variables for the production of exopolysaccharides in the microalgae Scenedesmus obliquus. Vitae 19, 60–69.
Cheng, Y.-S., Labavitch, J. M., and VanderGheynst, J. S. (2015). Elevated CO 2 concentration impacts cell wall polysaccharide composition of green microalgae of the genus Chlorella. Lett. Appl. Microbiol. 60, 1–7. doi: 10.1111/lam.12320
Chentir, I., Hamdi, M., Doumandji, A., HadjSadok, A., Ouada, H. B., Nasri, M., et al. (2017). Enhancement of extracellular polymeric substances (EPS) production in Spirulina (Arthrospira sp.) by two-step cultivation process and partial characterization of their polysaccharidic moiety. Int. J. Biol. Macromol. 105, 1412–1420. doi: 10.1016/j.ijbiomac.2017.07.009
Cornet, J.-F., and Dussap, C.-G. (2009). A Simple and reliable formula for assessment of maximum volumetric productivities in photobioreactors. Biotechnol. Prog. 25, 424–435. doi: 10.1002/btpr.138
Cruz, D., Vasconcelos, V., Pierre, G., Michaud, P., and Delattre, C. (2020). Exopolysaccharides from cyanobacteria: strategies for bioprocess development. Appl. Sci. 10:3763. doi: 10.3390/app10113763
de Jesus, C. S., de Jesus Assis, D., Rodriguez, M. B., Menezes Filho, J. A., Costa, J. A. V., de Souza Ferreira, E., et al. (2019). Pilot-scale isolation and characterization of extracellular polymeric substances (EPS) from cell-free medium of Spirulina sp. LEB-18 cultures under outdoor conditions. Int. J. Biol. Macromol. 124, 1106–1114. doi: 10.1016/j.ijbiomac.2018.12.016
De Oliveira, M. A. C. L., Monteiro, M. P. C., Robbs, P. G., and Leite, S. G. F. (1999). Growth and chemical composition of Spirulina maxima and Spirulina platensis biomass at different temperatures. Aquac. Int. 7, 261–275. doi: 10.1023/A:1009233230706
Dejsungkranont, M., Yusuf, C., and Sarote, S. (2017). Simultaneous production of C-phycocyanin and extracellular polymeric substances by photoautotrophic cultures of Arthrospira platensis. J. Chem. Technol. Biotechnol. 29, 2709–2718. doi: 10.1002/jctb.5293
Delattre, C., Pierre, G., Laroche, C., and Michaud, P. (2016). Production, extraction and characterization of microalgal and cyanobacterial exopolysaccharides. Biotechnol. Adv. 34, 1159–1179. doi: 10.1016/j.biotechadv.2016.08.001
DuBois, M., Gilles, K., Hamilton, J. K., Rebers, P. A., and Smith, F. (1951). A colorimetric method for the determination of sugars. Nature 168:167. doi: 10.1038/168167a0
Fritzsche, S., Blenk, P., Christian, J., Castiglione, K., and Becker, A. M. (2021). Inhibitory properties of crude microalgal extracts on the in vitro replication of cyprinid herpesvirus 3. Sci. Rep. 11:23134. doi: 10.1038/s41598-021-02542-2
Gaden, E. L.. (2000). Fermentation process kinetics. Biotechnol. Bioeng. 67, 629–635. doi: 10.1002/(SICI)1097-0290(20000320)67:6<629::AID-BIT2>3.0.CO;2-P
Gaignard, C., Laroche, C., Pierre, G., Dubessay, P., Delattre, C., Gardarin, C., et al. (2019). Screening of marine microalgae: investigation of new exopolysaccharide producers. Algal Res. 44:101711. doi: 10.1016/j.algal.2019.101711
Ge, H., Xia, L., and Zhou, X. (2014). Effects of light intensity on components and topographical structures of extracellular polysaccharides from the cyanobacteria Nostoc sp. J. Microbiol. 52, 179–183. doi: 10.1007/s12275-014-2720-5
Gouda, M., Tadda, M. A., Zhao, Y., Farmanullah, F., Chu, B., Li, X., et al. (2022). Microalgae bioactive carbohydrates as a novel sustainable and eco-friendly source of prebiotics: emerging health functionality and recent technologies for extraction and detection. Front. Nutr. 9:806692. doi: 10.3389/fnut.2022.806692
Gris, B., Sforza, E., Morosinotto, T., and Bertucco, A. (2017). Influence of light and temperature on growth and high-value molecules productivity from Cyanobacterium aponinum. J. Appl. Phycol. 29, 1781–1790. doi: 10.1007/s10811-017-1133-3
Hahm, J., Bauer, A., Jung, S.-H., Zell, N., Boßle, F., Buchholz, R., et al. (2021). Process parameter screening for the microalga Chlamydomonas asymmetrica in batch and turbidostat cultivations. Chemie Ing. Tech. 93, 1565–1572. doi: 10.1002/cite.202100046
Huang, S.-Y., and Chen, C.-P. (1986). Growth kinetics and cultivation of Spirulina platensis. J. Chin. Inst. Eng. 9, 355–363. doi: 10.1080/02533839.1986.9676900
Iqbal, M., and Zafar, S. I. (1993). Effects of photon flux density, CO2, aeration rate, and inoculum density on growth and extracellular polysaccharide production by Porphyridium cruentum. Folia Microbiol. 38, 509–514. doi: 10.1007/BF02814405
Jung, S., McHardy, C., Rauh, C., Luzi, G., Delgado, A., Buchholz, R., et al. (2021). A new approach for calculating microalgae culture growth based on an inhibitory effect of the surrounding biomass. Bioprocess Biosyst. Eng. 44, 1671–1684. doi: 10.1007/s00449-021-02550-6
Kumar, D., Kaštánek, P., and Adhikary, S. P. (2018). Exopolysaccharides from cyanobacteria and microalgae and their commercial application. Curr. Sci. 115, 234–241. doi: 10.18520/cs/v115/i2/234-241
Kumar, D., Kvíderová, J., Kaštánek, P., and Lukavský, J. (2017). The green alga Dictyosphaerium chlorelloides biomass and polysaccharides production determined using cultivation in crossed gradients of temperature and light. Eng. Life Sci. 17, 1030–1038. doi: 10.1002/elsc.201700014
Lee, E., Jalalizadeh, M., and Zhang, Q. (2015). Growth kinetic models for microalgae cultivation: a review. ALGAL 12, 497–512. doi: 10.1016/j.algal.2015.10.004
Lee, H., Erickson, L., and Yang, S. (1987). Kinetics and bioenergetics of light-limited photoautotrophic growth of Spirulina platensis. Biotechnol. Bioeng. 29, 832–843. doi: 10.1002/bit.260290705
Lee, M., Chen, Y., and Peng, T. (2012). Two-stage culture method for optimized polysaccharide production in Spirulina platensis. J. Sci. Food. Agric. 2012, 1562–1569. doi: 10.1002/jsfa.4743
Levert, J. M., and Xia, J. (2001). Modeling the growth curve for Spirulina (Arthrospira) maxima, a versatile microalga for producing uniformly labelled compounds with stable isotopes. J. Appl. Phycol. 13, 359–367. doi: 10.1023/A:1017924422164
Lewis, T., Nichols, P. D., and McMeekin, T. A. (2000). Evaluation of extraction methods for recovery of fatty acids from lipid-producing microheterotrophs. J. Microbiol. Methods 43, 107–116. doi: 10.1016/S0167-7012(00)00217-7
Li, P., Harding, S. E., and Liu, Z. (2001). Cyanobacterial exopolysaccharides: their nature and potential biotechnological applications. Biotechnol. Genet. Eng. Rev. 18, 375–404. doi: 10.1080/02648725.2001.10648020
Lupi, F. M., Fernandes, H. M. L., Sá-Correia, I., and Novais, J. M. (1991). Temperature profiles of cellular growth and exopolysaccharide synthesis by Botryococus braunii Kütz. UC 58. J. Appl. Phycol. 3, 35–42. doi: 10.1007/BF00003917
Magro, F. G., Margarites, A. C., Reinehr, C. O., Gonçalves, G. C., Rodigheri, G., Costa, J. A. V., et al. (2018). Spirulina platensis biomass composition is influenced by the light availability and harvest phase in raceway ponds. Environ. Technol. 39, 1868–1877. doi: 10.1080/09593330.2017.1340352
Mezhoud, N., Zili, F., Bouzidi, N., Helaoui, F., Ammar, J., and Ouada, H. B. (2014). The effects of temperature and light intensity on growth, reproduction and EPS synthesis of a thermophilic strain related to the genus Graesiella. Bioprocess Biosyst. Eng. 37, 2271–2280. doi: 10.1007/s00449-014-1204-7
Moreno, J., Vargas, M. A., Olivares, H., Rivas, J., and Guerrero, M. G. (1998). Exopolysaccharide production by the cyanobacterium Anabaena sp. ATCC 33047 in batch and continuous culture. J. Biotechnol. 60, 175–182. doi: 10.1016/S0168-1656(98)00003-0
Nicolaus, B., Panico, A., Lama, L., Romano, I., Manca, M. C., Giulio, A., et al. (1999). Chemical composition and production of exopolysaccharides from representative members of heterocystous and non-heterocystous cyanobacteria. Phytochemistry 52, 639–647. doi: 10.1016/S0031-9422(99)00202-2
Nielsen, S. S, . (ed.). (2003). “Phenol-sulfuric acid method for total carbohydrates,” in Food Analysis Laboratory Manual. Food Science Texts Series (Boston, MA: Springer). doi: 10.1007/978-1-4419-1463-7_6
Nur, M. M. A., Swaminathan, M. K., Boelen, P., and Buma, A. G. J. (2019). Sulfated exopolysaccharide production and nutrient removal by the marine diatom Phaeodactylum tricornutum growing on palm oil mill effluent. J. Appl. Phycol. 31, 2335–2348. doi: 10.1007/s10811-019-01780-2
Ogawa, T., and Terui, G. (1970). Studies on the growth of Spirulina platensis. (I) On the pure culture of Spirulina platensis. J. Ferment. Technol. 48, 361–367.
Paniagua-Michel, J., de, J., Olmos-Soto, J., and Morales-Guerrero, E. R. (2014). Algal and microbial exopolysaccharides: new insights as biosurfactants and bioemulsifiers. Adv Food Nutr Res. 73, 221–257. doi: 10.1016/B978-0-12-800268-1.00011-1
Pierre, G., Delattre, C., Dubessay, P., Jubeau, S., Vialleix, C., Cadoret, J. P., et al. (2019). What is in store for EPS microalgae in the next decade? Molecules 24:4296. doi: 10.3390/molecules24234296
Potnis, A. A., Raghavan, P. S., and Rajaram, H. (2021). Overview on cyanobacterial exopolysaccharides and biofilms : role in bioremediation. Rev. Environ. Sci. Bio Tech. 20, 781–794. doi: 10.1007/s11157-021-09586-w
Prybylski, N., Toucheteau, C., El Alaoui, H., Bridiau, N., Maugard, T., Abdelkafi, S., et al. (2020). “Bioactive polysaccharides from microalgae,” in Handbook of Microalgae-Based Processes and Products (Elsevier), 533–571. doi: 10.1016/B978-0-12-818536-0.00020-8
Reichert, M., Bergmann, S. M., Hwang, J., Buchholz, R., and Lindenberger, C. (2017). Antiviral activity of exopolysaccharides from Arthrospira platensis against koi herpesvirus. J. Fish Dis. 40, 1441–1450. doi: 10.1111/jfd.12618
Sanford, P. A., and Baird, J. (1983). “Industrial utilization of polysaccharides,” in The Polysaccharides (London: Academic Press). doi: 10.1016/B978-0-12-065602-8.50012-1
Silva, M. B. F., Azero, E. G., Teixeira, C. M. L. L., and Andrade, C. T. (2020). Influence of culture conditions on the production of extracellular polymeric substances (EPS) by Arthrospira platensis. Bioresour. Bioprocess. 7:47. doi: 10.1186/s40643-020-00337-3
Singh, S., Arad, S., and Richmond, A. (2000). Extracellular polysaccharide production in outdoor mass cultures of Porphyridium sp. in flat plate glass reactors. J. Appl. Phycol. 12, 269–275. doi: 10.1023/A:1008177002226
Soanen, N., Silva, E., Da Gardarin, C., Michaud, P., and Laroche, C. (2016). Improvement of exopolysaccharide production by Porphyridium marinum. Bioresour. Technol. 213, 231–238. doi: 10.1016/j.biortech.2016.02.075
Strieth, D., Stiefelmaier, J., Wrabl, B., Schwing, J., Schmeckebier, A., Nonno, S., et al. (2020). A new strategy for a combined isolation of EPS and pigments from cyanobacteria. J. Appl. Phycol. 32, 1729–1740. doi: 10.1007/s10811-020-02063-x
Trabelsi, L., Ben Ouada, H., Bacha, H., and Ghoul, M. (2009). Combined effect of temperature and light intensity on growth and extracellular polymeric substance production by the cyanobacterium Arthrospira platensis. J. Appl. Phycol. 21, 405–412. doi: 10.1007/s10811-008-9383-8
Villay, A., Laroche, C., Roriz, D., El Alaoui, H., Delbac, F., and Michaud, P. (2013). Optimisation of culture parameters for exopolysaccharides production by the microalga Rhodella violacea. Bioresour. Technol. 146, 732–735. doi: 10.1016/j.biortech.2013.07.030
Vonshak, A.. (1997). Spirulina: Growth, Physiology and Biochemistry. ed. A. Vonshak. London: Taylor and Francis Ltd,.
Keywords: A. platensis, C. asymmetrica, exopolysaccharides, light, temperature, batch, turbidostat
Citation: Jung S-H, Zell N, Boßle F, Teipel U, Rauh C, McHardy C and Lindenberger C (2022) Influence of Process Operation on the Production of Exopolysaccharides in Arthrospira platensis and Chlamydomonas asymmetrica. Front. Sustain. Food Syst. 6:883069. doi: 10.3389/fsufs.2022.883069
Received: 24 February 2022; Accepted: 22 April 2022;
Published: 19 May 2022.
Edited by:
Lutz Grossmann, University of Massachusetts Amherst, United StatesReviewed by:
Hema Rajaram, Bhabha Atomic Research Centre (BARC), IndiaPriscilla Bezerra, Université Clermont Auvergne, France
Copyright © 2022 Jung, Zell, Boßle, Teipel, Rauh, McHardy and Lindenberger. This is an open-access article distributed under the terms of the Creative Commons Attribution License (CC BY). The use, distribution or reproduction in other forums is permitted, provided the original author(s) and the copyright owner(s) are credited and that the original publication in this journal is cited, in accordance with accepted academic practice. No use, distribution or reproduction is permitted which does not comply with these terms.
*Correspondence: Sun-Hwa Jung, Uy5KdW5nQG90aC1hdy5kZQ==