- 1Environmental Science Division, Argonne National Laboratory, Lemont, IL, United States
- 2Department of Entomology, University of Illinois at Urbana-Champaign, Urbana, IL, United States
- 3Energy Resources Center, University of Illinois at Chicago, Chicago, IL, United States
- 4Strategic Energy Analysis Center, National Renewable Energy Laboratory, Golden, CO, United States
Achieving decarbonization goals to address global climate change and increasing energy needs requires significant continued investments in solar energy. The expansion of utility-scale solar development across the globe has increased the pressure on land resources for energy generation and other land uses (e.g., agriculture, biodiversity conservation). To address this growing issue, greater emphasis has been placed on solar development strategies that maximize the benefits of solar energy generation and multiple ecosystem services, such as the development of agrivoltaics systems that co-locate solar energy production and various forms of conservation and agricultural land uses. The purpose of this paper is to systematically synthesize the potential ecosystem services of agrivoltaics and summarize how these development strategies could address several United Nations Sustainable Development Goals (SDGs). Our review will focus on four broad potential ecosystem services of agrivoltaics: (1) energy and economic benefits; (2) agricultural provisioning services of food production and animal husbandry; (3) biodiversity conservation; and (4) regulating ecosystem services such as carbon sequestration and water and soil conservation. In particular, we will highlight the state of the science, challenges, and knowledge gaps that represent opportunities for further study to better understand how solar energy deployment can facilitate sustainable development.
Introduction
Achieving decarbonization goals to mitigate climate change and meet our increasing global energy needs will require significant continued investments in solar energy. Solar energy development is currently the fastest growing electrical generation utility sector in the world (REN21, 2021). In the United States, solar energy electricity generation has seen >20x growth in the past decade (Energy Information Administration (EIA), 2021a) and is projected to be the dominant renewable source of electricity by 2040 [Energy Information Administration (EIA), 2021b].
Utility-scale solar energy developments [ground-mounted photovoltaic (PV) facilities >1MW] are expected to comprise the greatest portion of future solar electricity generation in the U.S. [U.S. Department of Energy (DOE), 2021a] and considerable amounts of land will be needed to meet these projections. Globally, meeting net zero goals could require ~135 million acres (55 million ha) of land for solar energy production by 2050, assuming a global increase in development to about 18 TW installed capacity [International Energy Agency (IEA), 2021] and solar energy land use of 7.5 ac/MW (3.0 ha/MW) (Heath et al., 2022). Some estimates are even higher – 22 TW installed capacity by 2050, or about 165 million acres (67 million ha) (Fischer et al., 2022). The expansion of utility-scale solar development globally has increased the pressure on land resources for energy generation and other land uses (e.g., agriculture). Therefore, sustained development of solar energy will depend on finding renewable energy solutions that synergize the co-benefits of energy production, ecosystem services, and other land uses. To address this growing issue, greater emphasis has been placed on solar development land sharing strategies that maximize the outputs of solar energy generation and multiple ecosystem services.
One such land sharing strategy, broadly termed “agrivoltaics,” has emerged as a promising approach to improve land productivity and maximize synergies among energy, food, and environmental security (Dupraz et al., 2011; Hernandez et al., 2019; Al Mamun et al., 2022). Here, we define agrivoltaic (AV) systems as the co-location of ground-mounted solar energy development and one or more of the following agricultural activities: crop cultivation (“AV-cropping”), animal husbandry (“AV-animal,” e.g., livestock grazing, apiaries), or habitat enhancement to improve ecosystem services (“AV-habitat”). These types of AV systems have been shown to improve efficiencies in land-use, water use, and energy generation, as well as demonstrate the feasibility of these dual land uses to mutually benefit various ecosystem goods and services (Barron-Gafford et al., 2019; Hernandez et al., 2019; Proctor et al., 2021). For example, research on crop production in AV systems has shown the feasibility of producing lower-growing shade-tolerant crops such as lettuces, tomatoes, kale, and peppers between and under the partially-shaded rows of PV arrays (Marrou et al., 2013; Barron-Gafford et al., 2019; Weselek et al., 2019; Hudelson and Lieth, 2021; Al Mamun et al., 2022). In addition, there is growing evidence on the benefits of AV strategies to enhance onsite habitat, such as the establishment and management of native grasses and forbs, and the related potential ecosystem services of these strategies for agricultural production, biodiversity conservation, and regulation services related to carbon sequestration and erosion control (Semeraro et al., 2018; Walston et al., 2018, 2021; Siegner et al., 2019; Randle-Boggis et al., 2020).
In 2015, the United Nations (UN) defined 17 Sustainable Development Goals (SDGs) as a universal call to action to end poverty, protect the planet, and improve the lives and prospects of all people [United Nations General Assembly (UN), 2015]. Renewable energy development can meet many of these goals and represents an important driver to the transition toward sustainable energy production globally (Hernandez et al., 2020). There are multiple potential ecosystem service benefits of AV systems that align with these various UN SDGs – including those related to energy and food production, biodiversity, and society. While previous attempts have been made to align AV systems with SDGs and other development goals (for example, see Agostini et al., 2021 and Proctor et al., 2021), a comprehensive synthesis of ecosystem services under our broadened definition of AV systems has not yet been performed, nor has there been a systematic overview of the research priorities in need of further study to better understand future opportunities of AV systems toward meeting SDGs.
The purpose of this paper to is to review and synthesize the potential ecosystem services of AV systems and summarize how these development strategies could address the UN SDGs. Our review will focus on the four broad categories of potential ecosystem services of AV systems (Figure 1): (1) energy and economic benefits; (2) agricultural provisioning services: food production and animal husbandry; (3) biodiversity conservation; and (4) regulating ecosystem services such as carbon sequestration and water and soil conservation. In particular, we synthesize the state of the science, challenges and tradeoffs, and knowledge gaps that represent opportunities for further study to optimize the ecosystem service outputs of AV systems. Finally, we present a summary of how solar energy deployment can address SDGs such as SDG15 (integration of solar with terrestrial ecosystem preservation). Where applicable, we distinguish specific ecosystem services among the three AV types:
• AV-cropping systems: the co-location of solar energy and crop production.
• AV-animal systems: the co-location of solar energy and animal husbandry and livestock grazing.
• AV-habitat systems: the co-location of solar energy and habitat restoration.
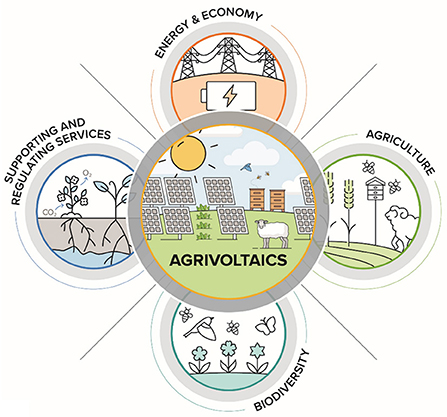
Figure 1. Ecosystem Services of Agrivoltaic Systems. Agrivoltaic (AV) systems have the potential to influence many different ecosystem services depending on priorities and implementation goals. Most clearly, AV systems produce electricity and thus contribute directly to energy and economy (top circle, Section Energy and economy). AV system configurations can also support other services. Crops and livestock can be grown or supported on land co-located with AV systems (right circle, Section Agricultural production); natural habitat implemented at AV systems can support plant and animal biodiversity to help achieve conservation goals (bottom circle, Section Biodiversity); thoughtful management can also result in beneficial supporting and regulating services, including net primary production, carbon sequestration and water and soil conservation (left circle, Section Supporting and regulating services). These different ecosystem services are not mutually exclusive, and thoughtfully designed AV systems could result in multiple co-benefits.
Review strategy
Following the Preferred Reporting Items for Systematic Reviews and Meta-analyses (PRISMA) guidelines for scoping reviews (Tricco et al., 2018), we conducted literature searches through June 2022 using Google Scholar with no publication date restriction. This was supplemented by searching reference lists and citations of included studies and relevant reviews. Our search criteria included terms such as “agrivoltaics” and “ecosystem services,” [OR] “solar energy” and “agriculture,” [OR] “solar energy” and “livestock,” [OR] “solar energy” and “honeybees,” [OR] “solar energy” and “grazing,” [OR] “solar energy” and “habitat,” [OR] “solar energy” and “ecosystem services,” [OR] “solar energy” and “pollinator habitat,” [OR] “solar-pollinator habitat.” All authors were involved in reviewing papers for eligibility. This protocol was not registered with an online registration platform.
From the search terms and resulting number of articles on Google Scholar, we excluded records that were not peer reviewed and did not directly address the co-location of solar and agriculture as defined above. Additional filtering was applied to select only those articles that were in the English language. The remaining articles were assessed for relevant information including year of publication, geographic location of the study, type of AV system, crop types (if applicable), livestock species (if applicable), habitat types (if applicable), keywords, and summary of results as determined from the abstract.
Ecosystem services of agrivoltaic systems
In this section, we review the various types of AV systems and summarize the potential ecosystem services they may provide. Ecosystem services are the direct and indirect benefits that ecosystems provide to humans (Millennium Ecosystem Assessment, 2005). There are four broad categories of ecosystem services: provisioning services such as energy and food production, regulating services such as carbon sequestration and erosion control, cultural services such as recreation, and supporting services such as photosynthesis and net primary production. A summary of the ecosystem services of AV systems is provided in Table 1. In general we consider all AV systems to implement one of the following dual land use strategies as presented in Table 1: (1) the co-location of solar development and on-site agricultural production (AV-cropping or AV-animal), or (2) the co-location of solar development and habitat enhancement (AV-habitat) to improve pollination services to adjacent agricultural fields and other ecosystem services on the solar site.
Agrivoltaic systems have the potential to support all four categories of ecosystem services. Below, we summarize the ecosystem services of AV systems: provisioning services of energy and food production and related economic benefits, and the regulating, supporting, and cultural services that are provided by AV systems (Figure 1). The alignment of these ecosystem services with the UN SDGs is summarized in Table 2.
Energy and economy
Significant improvements in solar technologies have fueled the continued growth of solar in the global electric sector. The best-known service of photovoltaic systems (including AV systems) is the low-carbon energy production these systems can provide. While in operation, a solar energy facility represents a carbon-free source of electricity [U.S. Department of Energy (DOE), 2021a]. Estimates of projected solar capacity growth suggest up to 22 TW of installed capacity by 2050 globally in a scenario where 100% of the power sector is supplied by renewable energy (Fischer et al., 2022). The associated land area (estimated as about 165 million acres or 67 million ha) is <0.5% of global land mass, but also represents a significant opportunity for AV systems to provide ecosystem services in addition to energy production.
Information on the total global amount of electricity generation from AV systems is lacking but recent estimates suggest there could be >2.8 GW of global electricity generation from AV-cropping systems (Gorjian et al., 2022), corresponding to ~21,000 ac (8,500 ha) using land use assumptions from Heath et al. (2022). There could be far greater global electricity generation from AV systems co-located with native habitat restoration. Despite recent research examining the opportunities for habitat restoration within solar facilities in the U.S. and U.K (for example see Blaydes et al., 2021 and Walston et al., 2021), there is currently little quantitative information on the amount of solar energy development with co-located native habitat restoration activities. In the U.S. state of Minnesota, it is estimated that about 1,400 ac (570 ha) of land operated for solar energy production is co-located with native habitat restoration, representing ~175 MW of electricity production [Minnesota Board of Water and Soil Resources (MN BWSR), 2021].
There is a growing body of evidence suggesting that some AV designs may improve the efficiency of solar PV panels and therefore improve energy production. Temperature has a significant impact on solar PV panel operating efficiency. A 1°C increase in temperature can decrease panel efficiency by 0.6% when temperatures exceed 25°C (Barron-Gafford et al., 2019). During hot summer months when PV panel efficiency is known to plateau and become less productive, AV systems vegetated with cropping systems or native habitat can create cooler microclimates under the PV panels, improving PV panel life and performance (Adeh et al., 2018; Barron-Gafford et al., 2019). In addition, advancements in solar technologies can also act synergistically with AV systems to improve energy efficiency. For example, in AV systems utilizing bifacial PV modules, the undersides of the PV panels may utilize greater reflectance from vegetation, leading to increased energy production (Rodríguez-Gallegos et al., 2020). In addition, a recent study in Saudi Arabia described how running water through the frame of the PV panel functioned as a heat exchanger improving the efficiency of the PV panel by nearly 10% (Li et al., 2022).
In addition to creating plant-friendly and more PV-efficient cooler microclimates, some AV systems may also lower the net energy requirements for a solar facility by improving the site's water use efficiency for plant irrigation by either (a) repurposing water used for cleaning panels for irrigating plants for agricultural production, and/or (b) reducing evapotranspiration due to shading from the PV panels (Hernandez et al., 2019).
Agrivoltaic systems have the potential to not only help meet global decarbonization goals for the electricity grid, but to also do so sustainably by simultaneously achieving other environmental, economic, and social objectives (Hernandez et al., 2019; Proctor et al., 2021; Al Mamun et al., 2022). Solar PV jobs are expected to reach 22.2 million by 2050 (Ram et al., 2020). As agricultural areas are ideal locations for solar energy development, AV developments may benefit rural economies through jobs, tax revenues for local programs, and by providing income diversification for farmers and landowners [Moore et al., 2021; Electric Power Research Institute (EPRI), 2022]. Globally, this may be most positively impactful in rural arid landscapes facing the most urgent needs for sustainable agriculture and energy production and where the benefits of PV shading for crop production and water use efficiency are highest (Parkinson and Hunt, 2020).
Agricultural production
A variety of dual use opportunities have emerged to combine AV systems with crop production, livestock grazing (Andrew et al., 2021; Electric Power Research Institute (EPRI), 2022), and other forms of animal husbandry such as beekeeping and rabbit farming (Dolezal et al., 2021; Lytle et al., 2021). Worldwide, farmland is being lost due to conversion to other land uses (Hu et al., 2020) or becoming less productive as a result of climate change (Ortiz-Bobea et al., 2021). These factors place greater pressure to intensify agricultural production on remaining farmland or to convert other natural areas for agricultural purposes. Paradoxically, solar energy development often competes with agriculture for land (Dupraz et al., 2011) and represents one form of land use conversion of former agricultural fields (Walston et al., 2021). As more land for solar development is needed, there are concerns regarding land use changes from converting agricultural land from food production to energy production. AV-cropping and AV-animal systems may mitigate these concerns by allowing food production to occur at these sites (Marrou et al., 2013; Dinesh and Pierce, 2016; Barron-Gafford et al., 2019; Sekiyama and Nagashima, 2019). In addition, these multifunctional AV systems can be mutually beneficial to both the solar industry and the agricultural communities. For example, AV-cropping and AV-animal systems may reduce operations and maintenance (O&M) costs associated with water needs and vegetation maintenance while also providing land access for agricultural producers (Proctor et al., 2021). AV-habitat systems may also be managed in a manner that reduces O&M costs while also providing pollination services to nearby (offsite) agricultural fields (Walston et al., 2018). Lower O&M costs may have positive economic implications for the solar industry while the land access and habitat restoration may positively impact agricultural communities directly, by providing land for crop production, and indirectly, by providing agricultural services to adjacent offsite fields.
Co-locating crop production with solar energy development has been deployed in different configurations throughout Europe, Asia, Australia, and recently, the United States (Marrou et al., 2013; Elamri et al., 2018; Barron-Gafford et al., 2019; Toledo and Scognamiglio, 2021; Al Mamun et al., 2022). Currently, most AV systems with co-located crop cultivation are planted with high value and hand harvested crops (i.e., lettuces, tomatoes, saffron, kale, broccoli, eggplant, peppers, etc.). A number of AV demonstrations have suggested that crop cultivation at solar facilities may be particularly useful in arid regions where shading by PV panels can improve microclimate conditions, such as temperature and soil moisture, and improve the site's water use efficiency for irrigation (Barron-Gafford et al., 2019; Parkinson and Hunt, 2020). Although the majority of AV systems have focused on crop cultivation at relatively small solar sites (<3 MW) using shade-tolerant specialty crops that can be cultivated manually or with little equipment needs, emerging research is examining how AV systems can be integrated with more traditional shade-intolerant commercial crops such as corn, soybeans, and wheat. For example, a small-scale experimental field study in Japan suggests that corn production could be achieved at solar PV facilities with raised PV panels (Sekiyama and Nagashima, 2019). While this study is limited in scale and replication, it is suggestive of the potential feasibility of commercial crop production at AV facilities and is being followed by current research in the United States and elsewhere (e.g., U.S. Department of Agriculture SCAPES Study, https://cris.nifa.usda.gov/cgi-bin/starfinder/0?path=fastlink1.txt&id=anon&pass=&search=R=94424&format=WEBLINK). In these applications, there may be additional considerations for designing solar systems that could accommodate these traditional cropping systems (i.e., increasing PV panel height and the spacing between rows of PV panels) or altering agricultural operations to facilitate row crop agriculture with solar energy infrastructure (i.e., modifying agricultural equipment to reduce risk of damage to solar infrastructure). Future research is needed examine the feasibility and cost implications of scaling AV systems to larger, commercial cropping systems.
Several other demonstrations of AV systems have shown that livestock grazing or other forms of animal husbandry can be compatible with solar energy development (Table 1). Livestock such as sheep may be particularly compatible with solar infrastructure due to their relatively small size and docile nature compared to other livestock [Al Mamun et al., 2022; Electric Power Research Institute (EPRI), 2022]. For example, Andrew et al. (2021) found lamb growth and production at a 1.4 MW (2.4 ha) solar facility in Oregon comparable to growth and production on open pastureland, demonstrating that land productivity can be improved through AV systems that co-locate livestock grazing and solar energy development. This could be particularly beneficial for the sheep industry, which has been in decline in the United States (The National Academies, 2008). While concerns have been raised about the compatibility of AV systems and other forms of livestock such as cattle [Electric Power Research Institute (EPRI), 2022], future research may help identify which solar development designs and types of livestock might be most compatible for future AV-grazing systems.
Other recent publications have argued for the utility of AV systems as sites for other forms of animal husbandry such as beekeeping (Armstrong et al., 2021; Dolezal et al., 2021). The utilization of AV systems as apiaries for honey production could have several ecosystem service benefits for honeybees and food production. Honey, a specialty crop in its own right, is valued at over $300,000,000 USD annually in the United States [United States Department of Agriculture (USDA), 2021], and honey production is reliant upon access to areas with high quantities of nectar availability. Land use changes such as agricultural intensification have led to reductions in land available to support apiaries and resulting declines in the quality and quantity of honey yields (Otto et al., 2016). As solar installations become more common in agricultural settings, there is growing opportunity for these sites to be co-managed with apiaries, which could improve honey yields and the agricultural services provided by managed honeybees (Dolezal et al., 2021). For example, a study across solar facilities in the United Kingdom found that if honeybee hives were installed at all existing solar parks in 2017, the total pollination service benefit to surrounding agricultural production would have been £5.9 million (Armstrong et al., 2021).
Biodiversity
Biodiversity provides several ecosystem services critical to human wellbeing, such as assisting in food production, pest control, and the purification of fresh water (Millennium Ecosystem Assessment, 2005). Worldwide, biodiversity is threatened from the loss of native habitat as a result of the expansion of human land uses (Ekroos et al., 2016). In many areas, land conversion associated with agricultural intensification has contributed to the loss of native grassland systems and declines in biodiversity – most notably pollinator populations (Kremen and Ricketts, 2000). In these agricultural landscapes, land management approaches that focus on providing diverse high-quality pollinator habitat may have an important role in safeguarding pollinator populations and the agricultural services they provide.
Recent attention has been placed on the role of AV-habitat systems that integrate native habitat enhancement as a means to conserve biodiversity and restore associated ecosystem services [Walston et al., 2018, 2021; Hernandez et al., 2019; Dolezal et al., 2021; Graham et al., 2021; Electric Power Research Institute (EPRI), 2022]. In these configurations, most of the focus has been placed on the creation and maintenance of pollinator-friendly seed mixes of regionally native grasses, forbs, and other wildflowers, within the solar infrastructure footprint, that attract and support native insect pollinators by providing food sources, refugia, and nesting habitat (“solar-pollinator habitat”; Walston et al., 2018, 2021). The establishment of solar-pollinator habitat could benefit biodiversity and be an important conservation mechanism for some imperiled species (Hernandez et al., 2019). In addition, increased insect abundance and diversity associated with solar-pollinator habitat could restore ecosystem services such as crop pollination and pest control that may maintain or enhance production on nearby agricultural lands. These potential benefits are inferred by years of field research associating native habitat restoration in agricultural landscapes with increases in pollinator populations and their agricultural services. For example, habitat restoration near agricultural fields can increase insect pollination services and resulting crop yields of fruits such as strawberries and blueberries (Blaauw and Isaacs, 2014; Ganser et al., 2018), and there is additional evidence that habitat restoration around other commercial crop fields, such as soybeans, could improve insect pollination services (Levenson et al., 2022) and provide habitat for natural enemies that feed on crop pests (Gardiner et al., 2009).
While there is an abundance of evidence on the benefits of habitat restoration in agricultural landscapes for biodiversity, the concept of solar-pollinator habitat restoration is still relatively new and there are questions regarding vegetation management, compatibility with solar infrastructure, and ability to achieve certain ecological objectives. Emerging research suggests that in some areas, the partial shading of solar panels can benefit the growth of native vegetation and increase insect diversity (Graham et al., 2021). In addition, modeling studies have demonstrated the important role solar facilities may serve in the conservation of native insects through the establishment of solar-pollinator habitat (Walston et al., 2018, 2021; Blaydes et al., 2022).
Supporting and regulating services
Along with the provisioning services of energy and food production and benefits to biodiversity, AV systems also have the potential to positively influence other ecosystem services, such as the regulation of climate, air quality, water, and soil quality. Solar PV energy is one of the lowest emitting sources of greenhouse gases (GHG) of all electricity generating types [Hernandez et al., 2019; Agostini et al., 2021; U.S. Department of Energy (DOE), 2021a], in large part because carbon-containing fossil fuels are not combusted to produce energy. Analysis of greenhouse gas emissions from solar facilities showed that these systems have life-cycle greenhouse gas emissions from 10 to 50 times lower than fossil-fuel based power (Turconi et al., 2013; Ludin et al., 2018; Bosmans et al., 2021). The range of lifecycle greenhouse gas emissions for solar PV facilities has been found to be most dependent on the panel type, with mono-crystalline silicon panels associated with the largest emissions due to high energy requirements of production (Muteri et al., 2020; Bosmans et al., 2021). Still, more than 99% of solar PV facilities have lifecycle emissions lower than 100 g CO2 eq/kWh (Bosmans et al., 2021). Creating AV-habitat systems can offset these relatively small greenhouse gas emissions (through vegetative capture of carbon), and improve air quality. As sites of agricultural production or habitat restoration, all types of AV systems can influence flows of other supporting and regulating ecosystem services such as net primary production, soil carbon sequestration, soil stabilization, and water retention. For example, AV systems can be designed to improve the site's water retention potential, as a result of increased shading from vegetation and PV panels that reduces evaporation and plant transpiration (Adeh et al., 2018; Barron-Gafford et al., 2019; Parkinson and Hunt, 2020).
AV-habitat systems co-located with native habitat restoration (“solar-pollinator habitat”) can also improve the supporting services of photosynthesis and net primary productivity (Huang et al., 2013) and regulating services of carbon sequestration, water retention, and erosion control. As shading by solar panels may create more favorable microclimates for the growth of native vegetation, the above- and below-ground growth of these onsite plant communities can better stabilize soil and provide greater carbon sequestration potential compared to conventional solar vegetation management practices (e.g., turfgrass) (Walston et al., 2021). Native grasses and forbs typically have deeper root systems than turfgrass (Schenk and Jackson, 2002), and these deeper root systems can facilitate the accumulation of soil organic carbon (Yang et al., 2019), improve soil stabilization to minimize erosion, and reduce water runoff (Schulte et al., 2017). Recent modeling results have demonstrated the potential for AV systems co-located with native habitat restoration to improve the site's carbon sequestration potential and reduce erosion and runoff (Walston et al., 2021). However, empirical evidence on the regulating services of AV systems is sparse and the limited available information suggests it may take several years for some of these service benefits to be realized. For example, soil organic carbon accumulates at former row-crop agricultural fields that have been restored to native prairie grasses at a rate of ~0.68 Mg C*ha−1*year−1 (McLauchlan et al., 2006). Therefore, it may take several years for statistically distinguishable changes in soil organic carbon to be detected once habitat restoration activities have started at AV systems. At one solar site in the U.S., for example, the solar site's soil carbon content remained lower than reference soils in a nearby natural area seven years after solar site construction and revegetation (Choi et al., 2020).
Relating agrivoltaic systems to Sustainable Development Goals
The potential ecosystem service benefits of AV systems can be translated into numerous alignments with the UN's Sustainable Development Goals (SDGs). Our assessment revealed potential alignments with 15 out of the 17 total SDGs (Table 2). The strengths of AV systems that help them meet these SDGs lie in the potential of these systems to (a) provide food and energy security to poor or developing communities, (b) efficiently utilize water and other resources, (c) mitigate climate change, and (d) benefit biodiversity. Some of the SDGs may be addressed by any of the three AV types, whereas some SDGs may be addressed by only one or two AV types. There are 11 SDGs that can by addressed by any of the three AV types, including SDG 1 (No Poverty), SDG 3 (Good Health and Wellbeing), and SDG 7 (Affordable and Clean Energy). The underlying mechanisms for the alignment between AV systems and these SDGs is provided in Table 2.
Data gaps and research needs
AV systems represent a promising approach to improving land use efficiency that will help achieve global sustainability goals for energy, food, and environmental security. As an emerging strategy in the development of multifunctional landscapes, however, there are challenges and information gaps associated with all AV systems that need to be addressed. Here, we summarize tradeoffs, data gaps, and research needs associated with AV systems that warrant further investigation to implement AV systems at larger scales and further address SDG goals.
As a land sharing strategy, AV systems (especially AV-cropping systems) are often viewed as a compromise between agriculture and energy production (Proctor et al., 2021; Al Mamun et al., 2022) where these co-located outputs would not be as great as their respective individual outputs of a single land use (i.e., strictly solar development or strictly agriculture). This may be particularly evident in AV-cropping systems that require tradeoffs in shared space for crop cultivation and PV panel infrastructure, which may result in (a) lower crop yields due to loss of planting area and shading or (b) lower energy production efficiency due to lower panel density from increased spacing between PV arrays to accommodate cropping systems. Technological modifications to PV panel height or spacing (width) to accommodate AV vegetation (whether crops, livestock feed, or habitat) can result in additional costs for the solar system and effect the price of electricity (e.g., Schindele et al., 2020).
There are land sharing tradeoffs between solar energy development and vegetation management for all types of AV systems – whether for onsite cropping systems, livestock grazing and animal husbandry, or habitat restoration. To be commercially feasible, many solar PV facilities are engineered to require minimal racking materials and, as such, PV arrays mounted close to the ground [e.g., <91 cm (<36 in) clearance] are often constructed [Electric Power Research Institute (EPRI), 2022]. Low mounted PV panels limit the types of vegetation that can be grown under and around the panels (vegetation cannot grow tall enough to shade the PV panels and reduce panel performance). The extent of vegetation management for AV systems varies, and vegetation management requires relatively small equipment to be compatible with solar energy infrastructure. Also, prescribed fires commonly used in the management of native grassland habitats is not a compatible vegetation management activity at solar sites (Walston et al., 2018) and vegetation management practices often specifically aim to minimize fire risk at solar sites (e.g., regularly removing thatch and dead vegetation).
The constraints of vegetation height and management practices limit the types of crops that may be effectively grown at AV-cropping systems and the types of vegetation that may be grown for other AV systems used for livestock grazing, animal husbandry, and biodiversity conservation (e.g., solar-pollinator habitat). The vast majority of AV-cropping systems that have been demonstrated are at relatively small solar sites (<3 MW) with low-growing shade tolerant crops such as lettuces, peppers, and tomatoes (Barron-Gafford et al., 2019; Hudelson and Lieth, 2021). Additional research is needed to determine the feasibility of AV systems with taller crops and other crop types that may require more sunlight such as corn, soybeans, and wheat. This would require additional studies examining the technical and commercial feasibility of raised solar panels and increased panel spacing to accommodate the co-location of these commercial grain crops. Although some evidence suggests these types of AV-cropping systems may be feasible at small scales (e.g., Amaducci et al., 2018; Sekiyama and Nagashima, 2019), additional research is needed to understand the interactions between crop production and energy development, equipment needs, and costs of these AV-cropping systems at larger, more commercial scales. Improving the feasibility of solar energy development and cultivation of taller grain crops could create opportunities for novel AV systems such as AV-bioenergy production through the co-location of switchgrass and other biofuel crops.
Livestock grazing practices at AV systems are also influenced by the site's PV infrastructure. For example, most AV-grazing systems have demonstrated the compatibility of sheep grazing at solar facilities [Campos Maia et al., 2020; Andrew et al., 2021; Electric Power Research Institute (EPRI), 2022]. Solar grazing can benefit livestock, with vegetation providing for food requirements and shade decreasing livestock water needs (Hernandez et al., 2014; Campos Maia et al., 2020). Grazing may also decrease solar facility O&M costs by decreasing the need for mowing, and decrease fire hazards. Well-managed grazing has proven to benefit plant diversity through a natural process of winter and spring sheep grazing (Montag et al., 2016). Most studies to date have used sheep grazing as a model to demonstrate the compatibility of solar development and livestock grazing. Additional research is needed to understand the feasibility of other livestock grazing practices at AV systems and determine the types of livestock most compatible with existing solar design configurations. Although larger livestock such as cattle pose a greater risk of damaging PV infrastructure, the U.S. Department of Energy has recently funded research on the feasibility of developing solar facilities that will support cattle grazing [U.S. Department of Energy (DOE), 2021b]. Research such as this will help identify the required modifications to solar development designs to strengthen racking systems and increase the height of panels to support cattle grazing [Electric Power Research Institute (EPRI), 2022].
The tradeoffs between energy output and onsite vegetation management also extend to several techno-ecological considerations for biodiversity conservation. Most AV systems with solar-pollinator habitat are limited to planting lower-growing native grasses and forbs and are selected based, in part, on an assessment of the financial costs of seeding and management [Electric Power Research Institute (EPRI), 2022]. These project designs, while likely to provide some amount of ecological benefit, may not be optimal to achieve certain ecological objectives (Dolezal et al., 2021). The conservation of rare or at-risk species often requires specific habitat restoration activities, and such activities will need to be allowed at solar facilities if these sites are to be effective in focal species conservation. For example, North American AV systems with solar-pollinator habitat may not effectively work to conserve populations of monarch butterfly (Danaus plexippus) – a candidate species for listing under the U.S. Endangered Species Act – without the onsite establishment of milkweed (Genus Asclepias), the larval host plant (Lukens et al., 2020).
In the U.S., one approach to increasing the establishment and ecological function of solar-pollinator habitat is through the use of pollinator-friendly scorecards. The concept of these scorecards is to assess the quality of vegetation planted and managed at solar sites with respect to supporting pollinators, by providing criteria to designate solar sites as pollinator friendly. Sixteen states have developed scorecards through partnerships between state agencies, universities, and conservation consultants [Electric Power Research Institute (EPRI), 2021; Fresh Energy, 2021]. These scorecards provide guidance on enhancing the ecological services of solar developments by providing considerations for increasing native plant diversity (e.g., planting seed mixes of differing flowering plant species, flower density, and diverse flowering phenologies). Other criteria on the score cards include the use of environmentally friendly pest and weed control methods such as avoiding the use of pesticides on the sites. While the scorecards are resulting in more AV systems utilizing habitat-enhancement, they are not without criticism or shortcomings. For example, there is limited attention to maintenance and evaluation of the habitat quality over time, with only a few states including requirements for maintenance reviews, generally at 2- to 5-year intervals [Electric Power Research Institute (EPRI), 2021]. Establishing standardized long-term data collection systems, therefore, could help address information gaps on the ecological performance of solar-pollinator habitat. Additionally, field research examining the establishment of different plant species and the broader ecological responses to solar-pollinator habitat is necessary to understand the potential opportunities and limits of solar-pollinator habitat as a biodiversity conservation tool (Dolezal et al., 2021). This research should also focus on the selection of appropriate goals and indicators for evaluating the ecological success of solar-pollinator habitat (Prach et al., 2019). Doing so could assist in the identification of regionally appropriate and cost-effective seed mixes and vegetation management strategies that can perform optimally in AV systems to benefit biodiversity.
There are other considerations associated with converting solar facilities into suitable habitat to drive biodiversity conservation. Positive ecological outcomes of solar-pollinator habitat could be region specific. For example, native vegetation restoration in arid regions is often challenging and time consuming (Lovich and Bainbridge, 1999), making it difficult to establish solar-pollinator habitat in these areas to offset the negative impacts of solar development on native vegetation and insect communities, such as those observed in the southwestern U.S. (Grodsky et al., 2021). Depending on various factors such as previous land use and the scale and configuration of development, AV-habitat systems with solar-pollinator habitat could provide a net benefit for biodiversity conservation (Blaydes et al., 2021; Walston et al., 2021). However, there are concerns in some areas that solar panels can act as ecological traps for some wildlife such as aquatic insects, resulting in females attempting to oviposit eggs onto the surface of PV panels that inevitably perish (Horváth et al., 2010). While some of the negative effects on aquatic insects can be mitigated by altering the design of the solar panels to make them less attractive to insects (Horváth et al., 2010), more research is needed on the biodiversity impacts of solar-pollinator habitat and elucidating the potential role of these sites as ecological traps. In addition, there is need for research that examines these solar-wildlife interactions beyond insect communities to include birds, mammals, and other vertebrate wildlife. Finally, further consideration needs to be placed on proper siting for solar developments if AV strategies are to optimally address SDGs. The siting of any energy system is one of the most important measures to understanding and minimizing environmental impacts (Jager et al., 2021); therefore, AV siting should consider factors such as the site's previous land use and its ecosystem service values.
There is also a lack of field data on the ecosystem services of AV systems in terms of their ability to sequester carbon and support sediment and water retention. To date, most studies of the regulating ecosystem services of AV systems have relied on models using fixed assumptions and inputs from other studies (e.g., Walston et al., 2021). Future research is needed to directly link specific vegetation management activities at AV systems with quantifiable measures of their regulating ecosystem services (e.g., carbon storage potential). This knowledge can be used to guide future decisions on seed mixes, planting strategies, and other management activities to optimize the ecological performance of AV-habitat systems. For example, this research could help industry and regulators better quantify the amount of carbon that could be offset or sequestered under certain AV system designs, which could be used in setting and achieving sustainable development objectives.
Conclusions
There are numerous opportunities for AV systems to synergize the ecosystem service outputs of solar energy production and other compatible land uses. AV systems also align with several UN SDGs that could contribute to the global transition to renewable energy and sustainable development. The environmental benefits of AV systems are primarily created by methods to optimize land productivity. Land productivity can be optimized to meet a number of objectives for energy and food production, biodiversity conservation, and climate change mitigation. These objectives may not be mutually exclusive. For example, climate change is an increasing threat to agriculture and food security (Ortiz-Bobea et al., 2021). Paradoxically, conventional agricultural practices are energy-intensive and contribute to climate change by accounting for about one-third of global GHG emissions (Gilbert, 2012). AV systems can help mitigate the negative interactions between climate change and agriculture by simultaneously improving agricultural production in arid regions and offsetting declines in food production from areas that have been impacted by climate change, while also mitigating the impact of agricultural land use practices toward climate change through lower GHG emissions and improved energy and water use efficiency.
AV systems can directly and positively impact SDG 7 “Ensure access to affordable, reliable, sustainable, and modern energy for all” by expanding and reducing the costs of solar energy through reducing O&M costs over the life of the project, creating new revenues streams, keeping agricultural land in production, and enhancing ecosystem services on these solar sites. While the advantages of AV systems align positively with many of the other SDGs (as shown in Table 2), we believe the strongest contributions could be made in SDG 9, 11, 13, and 15. For SDG 9, AV systems can help ensure rural areas have consistent access to renewable energy, which will be important for future energy and food needs. Similarly for SDG 11, AV systems can be sited close to communities and cities that have need for energy and nutritional food sources. For SDG 13, AV systems will produce energy to offset fossil fuel sources, but also provide a source of carbon and methane sequestration from vegetation establishment. For SDG 15, AV systems can enhance natural habitats to provide refuge for plant, animal, and insect populations. These few examples highlight the relevance and impact AV systems can have on UN SDG goals and the need for more research to make these systems an established construction method for the solar industry.
Finally, as larger-scale AV systems are being considered to address the energy and food security needs of larger communities, there is greater need for partnerships between solar developers, operators, and members of the agricultural and natural resource conservation communities. These partnerships can be useful in facilitating the scaling of AV systems by identifying cost-effective methods to integrate solar energy development and agricultural activities early in project conceptualization and design. In addition, these partnerships may be useful in providing recommendations to policymakers for the creation of incentives for AV systems, such as tax incentives and development of cost sharing mechanisms for site operation and maintenance.
Data availability statement
The original contributions presented in the study are included in the article/supplementary material, further inquiries can be directed to the corresponding author.
Author contributions
LW conceptualized and drafted the manuscript. IB and BC focused primarily on energy and economy. LW, HH, TB, and AD focused primarily on biodiversity and supporting and regulating services. JM focused primarily on agriculture production. All authors participated in literature review and contributed to writing portions of the draft manuscript. All authors contributed to the article and approved the submitted version.
Acknowledgments
The authors are thankful to Iris Caldwell for review and constructive comments on previous drafts of this article.
Licenses and permissions
This manuscript was primarily supported by the U.S. Department of Energy (DOE) Office of Energy Efficiency and Renewable Energy (EERE) Solar Energy Technologies Office (SETO) and was created by UChicago Argonne, LLC, Operator of Argonne National Laboratory (Argonne). Argonne is operated under Contract No. DE-AC02-06CH11357 for the DOE. This work was also authored in part by Alliance for Sustainable Energy, LLC, the manager and operator of the National Renewable Energy Laboratory for the DOE under Contract No. DE-AC36-08GO28308. We acknowledge support from the InSPIRE project through the DOE EERE SETO under award DE-EE00038642 and the PHASE project through the DOE EERE SETO under award DE-EE0009371. The U.S. Government retains for itself, and others acting on its behalf, a paid-up nonexclusive, irrevocable worldwide license in said article to reproduce, prepare derivative works, distribute copies to the public, and perform publicly and display publicly, by or on behalf of the Government.
Conflict of interest
The authors declare that the research was conducted in the absence of any commercial or financial relationships that could be construed as a potential conflict of interest.
Publisher's note
All claims expressed in this article are solely those of the authors and do not necessarily represent those of their affiliated organizations, or those of the publisher, the editors and the reviewers. Any product that may be evaluated in this article, or claim that may be made by its manufacturer, is not guaranteed or endorsed by the publisher.
References
Adeh, E. H., Selker, J. S., and Higgins, C. W. (2018). Remarkable agrivoltaic influence on soil moisture, micrometeorology and water-use efficiency. PLoS ONE 13, e0203256. doi: 10.1371/journal.pone.0203256
Agostini, A., Colauzzi, M., and Amaducci, S. (2021). Innovative agrivoltaic systems to produce sustainable energy: an economic and environmental assessment. Appl. Energy 281, 116102. doi: 10.1016/j.apenergy.2020.116102
Al Mamun, M. A., Dargusch, P., Wadley, D., Zulkarnain, N. A., and Aziz, A. A. (2022). A review of research on agrivoltaic systems. Renew. Sust. Energ. Rev. 161, 112351. doi: 10.1016/j.rser.2022.112351
Amaducci, S., Yin, X., and Colauzzi, M. (2018). Agrivoltaic systems to optimize land use for electric energy production. Appl. Energy 220, 545–561. doi: 10.1016/j.apenergy.2018.03.081
Andrew, A. C., Higgins, C. W., Smallman, M. A., Graham, M., and Ates, S. (2021). Herbage yield, lamb growth, and forage behavior in agrivoltaic production system. Front. Sustain. Food Syst.s 5, 659175. doi: 10.3389/fsufs.2021.659175
Armstrong, A., Brown, L., Davies, G., Whyatt, J. D., and Potts, S. G. (2021). Honeybee pollination benefits could inform solar park business cases, planning decisions, and environmental sustainability targets. Biol. Conserv. 263, 109332. doi: 10.1016/j.biocon.2021.109332
Barron-Gafford, G. A., Pavao-Zuckerman, M. A., Minor, R. L., Sutter, L. F., Barnett-Moreno, I., Blackett, D. T., et al. (2019). Agrivoltaics provide mutual benefits across the food–energy–water nexus in drylands. Nat. Sustain. 2, 848–855. doi: 10.1038/s41893-019-0364-5
Blaauw, B. R., and Isaacs, R. (2014). Flower plantings increase wild bee abundance and the pollination services provided to a pollination-dependent crop. J. Appl. Ecol. 51, 890–898. doi: 10.1111/1365-2664.12257
Blaydes, H., Potts, S. G., Whyatt, J. D., and Armstrong, A. (2021). Opportunities to enhance pollinator biodiversity in solar parks. Renew. Sust. Energ. Rev. 145, 111065. doi: 10.1016/j.rser.2021.111065
Blaydes, H. E., Gardner, J. D., Whyatt, Potts, S. G., and Armstrong, A. (2022). Solar park management and design to boost bumble bee populations. Environ. Res. Lett. 17, 044002. doi: 10.1088/1748-9326/ac5840
Bosmans, J. H., Dammeier, L. C., and Huijbregts, M. A. (2021). Greenhouse gas footprints of utility-scale photovoltaic facilities at the global scale. Environ. Res. Lett. 16, 094056. doi: 10.1088/1748-9326/ac1df9
Campos Maia, A. S., Culhari, E. D., Fonseca, V. D. F. C., Milan, H. F. M., and Gebremedhin, K. G. (2020). Photovoltaic panels as shading resources for livestock. J. Clean. Prod. 258, 120551. doi: 10.1016/j.jclepro.2020.120551
Choi, C. S., Cagle, A. E., Macknick, J., Bloom, D. E., Caplan, J. S., et al. (2020). Effects of revegetation on soil physical and chemical properties in solar photovoltaic infrastructure. Front. Environ. Sci. 8:140. doi: 10.3389/fenvs.2020.00140
Dinesh, H., and Pierce, J. M. (2016). The potential of agrivoltaic systems. Renew. Sust. Energ. Rev. 54, 299–308. doi: 10.1016/j.rser.2015.10.024
Dolezal, A. G., Torres, J., and O'Neal, M. E. (2021). Can solar energy fuel pollinator conservation? Environ. Entomol. 50, 757–761. doi: 10.1093/ee/nvab041
Dupraz, C., Marrou, H., Talbot, G., Dufour, L., Nogier, A., Ferard, Y., et al. (2011). Combining solar photovoltaic panels and food crops for optimising land use: towards new agrivoltaic schemes. Renew. Energy 36, 2725–2732. doi: 10.1016/j.renene.2011.03.005
Ekroos, J., Ödman, A. M., Andersson, G. K. S., Birkhofer, K., Herbertsson, L., Klatt, B. K., et al. (2016). Sparing land for biodiversity at multiple spatial scales. Front. Ecol. Evol. 3, 1–11. doi: 10.3389/fevo.2015.00145
Elamri, Y., Cheviron, B., Lopez, J.-., M., Dejean, C., and Belaud, G. (2018). Water budget and crop modeling for agrivoltaic systems: application to irrigated lettuces. Agric. Water Manag. 208, 440–453. doi: 10.1016/j.agwat.2018.07.001
Electric Power Research Institute (EPRI) (2021). Pollinator-Friendly Solar Scorecards: Comprehensive Analysis of Scorecard Attributes. Palo Alto, CA 3002022121.
Electric Power Research Institute (EPRI) (2022). Environmental and Social Considerations of Land Conversion to Solar Generation. Palo Alto, CA. In Press.
Energy Information Administration (EIA) (2021b). Annual Energy Outlook 2021. Available online at: https://www.eia.gov/outlooks/aeo/ (accessed April 26, 2022).
Energy Information Administration (EIA) (2021a). Form EIA-860 Detailed Data for 2021. Available online at: https://www.eia.gov/electricity/data/eia860/ (accessed April 26, 2022).
Fischer, M., Woodhouse, M., Herritsch, S., and Trube, J. (2022). International Technology Roadmap for Photovoltaic, 2021 Results. Available online at: https://www.vdma.org/international-technology-roadmap-photovoltaic (accessed April 26, 2022).
Fresh Energy (2021). Pollinator-Friendly Solar Scorecards. Available online at: https://fresh-energy.org/beeslovesolar/pollinator-friendly-solar-scorecards (accessed April 26, 2022).
Ganser, D., Mayr, B., Albrecht, M., and Knop, E. (2018). Wildflower strips enhance pollination in adjacent strawberry crops at the small scale. Ecol. Evol. 8, 11775–11784. doi: 10.1002/ece3.4631
Gardiner, M. M., Landis, D. A., Gratton, C., DiFonzo, C. D., O'Neal, M., Chacon, J. M., et al. (2009). Landscape diversity enhances biological control of an introduced crop pest in the north-central USA. Ecol. Appl. 19, 143–154. doi: 10.1890/07-1265.1
Gilbert, N. (2012). One-third of our greenhouse gas emissions come from agriculture. Nature 31, 10–12. doi: 10.1038/nature.2012.11708
Gorjian, S., Bousi, E., Özdemir, O. E., Trommsdorff, M., Kumar, N. M., Anand, A., et al. (2022). Progress and challenges of crop production and electricity generation in agrivoltaic systems using semi-transparent photovoltaic technology. Renew. Sustain. Energy Rev. 158, 112126. doi: 10.1016/j.rser.2022.112126
Graham, M., Ates, S., Melathopoulos, A. P., Moldenke, A. R., DeBano, S. J., Best, L. R., et al. and Higgins, C. W. (2021). Partial shading by solar panels delays bloom, increases floral abundance during the late-season for pollinators in a dryland, agrivoltaic ecosystem. Sci. Rep. 11, 1–13. doi: 10.1038/s41598-021-86756-4
Grodsky, S. M., Campbell, J. W., and Hernandez, R. R. (2021). Solar energy development impacts flower-visiting beetles and flies in the Mojave Desert. Biol. Conserv. 263, 109336. doi: 10.1016/j.biocon.2021.109336
Heath, G., Dwarakanath, R., Ovaitt, S., Walston, L., Curtis, T., Millstein, D., et al. (2022). Environmental and Circular Economy Implications of Solar Energy in a Decarbonized U.S. Grid. Technical Report NREL/TP-6A20-80818. Available online at: https://www.nrel.gov/docs/fy22osti/80818.pdf (accessed April 26, 2022).
Hernandez, R. R., Armstrong, A., Burney, J., Ryan, G., Moore-O'Leary, K., Diédhiou, I., et al. (2019). Techno-ecological synergies of solar energy for global sustainability. Nat. Sustain. 2, 560–568. doi: 10.1038/s41893-019-0309-z
Hernandez, R. R., Hoffacker, M., and Field, C. (2014). Land-use efficiency of big solar. Environ. Sci. Technol. 48, 1315–1323. doi: 10.1021/es4043726
Hernandez, R. R., Jordaan, S. M., Kaldunski, B., and Kumar, N. (2020). Aligning climate change and sustainable development goals with an innovation systems roadmap for renewable power. Front. Sustain. 1, 583090. doi: 10.3389/frsus.2020.583090
Horváth, G., Blah,ó, M., Egri, Á., Kriska, G., and Seres, I., and Robertson, B. (2010). Reducing the maladaptive attractiveness of solar panels to polarotactic insects. Conserv. Biol. 24, 1644–1653. doi: 10.1111/j.1523-1739.2010.01518.x
Hu, G., Li, X., Zhou, B. B., Ma, Q., Meng, X., Liu, Y., et al. (2020). How to minimize the impacts of urban expansion on farmland loss: developing a few large or many small cities? Landsc. Ecol. 35, 2487–2499. doi: 10.1007/s10980-020-01073-x
Huang, L., Xiao, T., Zhao, Z., Sun, C., Liu, J., Shao, Q., et al. (2013). Effects of grassland restoration programs on ecosystems in arid and semiarid China. J. Environ. Manage. 117, 268–275. doi: 10.1016/j.jenvman.2012.12.040
Hudelson, T., and Lieth, J. H. (2021). Crop production in partial shade of solar photovoltaic panels on trackers. AIP Conf. Proc. 2361, 80001. doi: 10.1063/5.0055174
International Energy Agency (IEA) (2021). Net Zero by 2050: A Roadmap for the Global Energy Sector. Available online at: https://www.iea.org/reports/net-zero-by-2050 (accessed April 26, 2022).
Jager, H. I., Efroymson, R. A., and McManamay, R. A. (2021). Renewable energy and biological conservation in a changing world. Biol. Conserv. 263, 109354. doi: 10.1016/j.biocon.2021.109354
Kremen, C., and Ricketts, T. (2000). Global perspectives on pollination disruptions. Conserv. Biol. 14, 1226–1228. doi: 10.1046/j.1523-1739.2000.00013.x
Levenson, H. K., Sharp, A. E., and Tarpy, D. R. (2022). Evaluating the impact of increased pollinator habitat on bee visitation and yield metrics in soybean crops. Agric. Ecosyst. Environ. 331, 107901. doi: 10.1016/j.agee.2022.107901
Li, R., Wu, M., Aleid, S., Zhang, C., Wang, W., Wang, P., et al. (2022). An integrated solar-driven system produces electricity with fresh water and crops in arid regions. Cell Rep. Phys. Sci. 3, 100781. doi: 10.1016/j.xcrp.2022.100781
Lovich, J. E., and Bainbridge, D. (1999). Anthropogenic degradation of the southern California desert ecosystem and prospects for natural recovery and restoration. Environ. Manage. 24, 309–326. doi: 10.1007/s002679900235
Ludin, N. A., Mustafa, N. I., Hanafiah, M. M., Ibrahim, M. A., Teridi, M. A. M., Sepeai, S., et al. and Sopian, K. (2018). Prospects of life cycle assessment of renewable energy from solar photovoltaic technologies: a review. Renew. Sustain. Energy Rev. 96, 11–28. doi: 10.1016/j.rser.2018.07.048
Lukens, L., Kasten, K., Stenoien, C., Cariveau, A., Caldwell, W., Oberhauser, K., et al. (2020). Monarch habitat in conservation grasslands. Front. Ecol. Evol. 8, 13. doi: 10.3389/fevo.2020.00013
Lytle, W., Meyer, T. K., Tanikella, N. G., Burnham, L., Engel, J., Schelly, C., et al. and Pearce, J. M. (2021). Conceptual design and rationale for a new agrivoltaics concept: pasture-raised rabbits and solar farming. J. Clean. Prod. 282, 124476. doi: 10.1016/j.jclepro.2020.124476
Marrou, H., Wery, J., Dufour, L., and Dupraz, C. (2013). Productivity and radiation use efficiency of lettuces grown in the partial shade of photovoltaic panels. Eur. J. Agron. 44, 54–66. doi: 10.1016/j.eja.2012.08.003
McLauchlan, K. K., Hobbie, S. E., and Post, W. M. (2006). Conversion from agriculture to grassland builds soil organic matter on decadal timescales. Ecol. Appl. 16, 143–153. doi: 10.1890/04-1650
Millennium Ecosystem Assessment (2005). Ecosystems and Human Well-being: Synthesis. Washington, DC: Island Press. Available online at: http://www.millenniumassessment.org/documents/document.356.aspx.pdf (accessed April 26, 2022).
Minnesota Board of Water and Soil Resources (MN BWSR) (2021). Minnesota Habitat Friendly Solar Program List of Projects that Meet the Standards. Available online at: https://bwsr.state.mn.us/sites/default/files/2021-05/List%20of%20HFS%20Sites.pdf (accessed April 26, 2022).
Montag, H., Parker, G., and Clarkson, T. (2016). The Effects of Solar Farms on Local Biodiversity. Clarkson and Woods and Wychwood. Available online at: https://helapco.gr/wp-content/uploads/Solar_Farms_Biodiversity_Study.pdf (accessed April 26, 2022).
Moore, S., Graff, H., Ouellet, C., Leslie, S., Olweean, D., Wycoff, A., et al. (2021). Developing Utility-Scale Solar Power in Michigan at the Agriculture-Energy Nexus: Stakeholder perspectives, pollinator habitat and trade-offs. Written for the Institute for Public Policy and Social Research, Michigan State University. Available at https://ippsr.msu.edu/mappr/developing-utility-scale-solar-power-michigan-agriculture-energy-nexus (accessed April 26, 2022).
Muteri, V., Cellura, M., Curto, D., Franzitta, V., Longo, S., Mistretta, M., et al. (2020). Review on life cycle assessment of solar photovoltaic panels. Energies 13, 252. doi: 10.3390/en13010252
Ortiz-Bobea, A., Ault, T. R., Carrillo, C. M., Chambers, R. G., and Lobell, D. B. (2021). Anthropogenic climate change has slowed global agricultural productivity growth. Nat. Clim. Change 11, 306–312. doi: 10.1038/s41558-021-01000-1
Otto, C. R. V., Roth, C. L., Carlson, B. L., and Smart, M. D. (2016). Land-use change reduces habitat suitability for supporting managed honey bee colonies in the Northern Great Plains. Proc. Natl. Acad. Sci. USA. 113, 10430–10435. doi: 10.1073/pnas.1603481113
Parkinson, S., and Hunt, J. (2020). Economic potential for rainfed agrivoltaics in groundwater-stressed regions. Environ. Sci. Technol. Lett. 7, 525–531. doi: 10.1021/acs.estlett.0c00349
Prach, K., Durigan, G., Fennessy, S., Overbeck, G. E., Torezan, J. M., Murphy, S. D., et al. (2019). A primer on choosing goals and indicators to evaluate ecological restoration success. Restor. Ecol. 27, 917–923. doi: 10.1111/rec.13011
Proctor, K. W., Murthy, G. S., and Higgins, C. W. (2021). Agrivoltaics align with green new deal goals while supporting investment in the US' rural economy. Sustainability 13, 137. doi: 10.3390/su13010137
Ram, M., Aghahosseini, A., and Breyer, C. (2020). Job creation during the global energy transition towards 100% renewable power system by 2050. Technol. Forecast. Soc. Change 151, 119682. doi: 10.1016/j.techfore.2019.06.008
Randle-Boggis, R. J., White, P. C. L., Cruz, J., Parker, G., Montag, H., Scurlock, J. M. O., et al. (2020). Realizing co-benefits for natural capital and ecosystem services from solar parks: a co-developed, evidence-based approach. Renew. Sustain. Energy Rev. 125, 109775. doi: 10.1016/j.rser.2020.109775
Ravi, S., Macknick, J., Lobell, D., Field, C., Ganesan, K., Jain, R., et al. (2016). Colocation opportunities for large solar infrastructures and agriculture in drylands. Appl. Energy 165, 383–392. doi: 10.1016/j.apenergy.2015.12.078
REN21 (2021). Global Status Report (Paris: REN21 Secretariat). ISBN 978-3-948393-03-8. Available online at: https://www.ren21.net/wp-content/uploads/2019/05/GSR2021_Full_Report.pdf (accessed April 26, 2022).
Rodríguez-Gallegos, C. D., Liu, H., Gandhi, O., Singh, J. P., Krishnamurthy, V., Kumar, A., et al. (2020). Global techno-economic performance of bifacial and tracking photovoltaic systems. Joule 4, 1514–1541. doi: 10.1016/j.joule.2020.05.005
Schenk, H. J., and Jackson, R. B. (2002). Rooting depths, lateral root spreads ad below-ground/above-ground allometries of plants in water-limited ecosystems. J. Ecol. 90, 480–494. doi: 10.1046/j.1365-2745.2002.00682.x
Schindele, S., Trommsdorff, M., Schlaak, A., Obergfell, T., Bopp, G., Reise, C., et al. (2020). Implementation of agrophotovoltaics: techno-economic analysis of the price-performance ratio and its policy implications. Appl. Energy 265, 114737. doi: 10.1016/j.apenergy.2020.114737
Schulte, L. A., Niemi, J., Helmers, M. J., Liebman, M., Arbuckle, J. G., James, D. E., et al. (2017). Prairie strips improve biodiversity and the delivery of multiple ecosystem services from corn–soybean croplands. Proc. Nat. Acad. Sci. 114, 11247–11252. doi: 10.1073/pnas.1620229114
Sekiyama, T., and Nagashima, A. (2019). Solar sharing for both food and clean energy production: performance of agrivoltaic systems for corn, a typical shade-intolerant crop. Environments 6, 65. doi: 10.3390/environments6060065
Semeraro, T., Pomes, A., Del Giudice, C., Negro, D., and Aretano, R. (2018). Planning ground-based utility scale solar energy as green infrastructure to enhance ecosystem services. Energy Policy 117, 218–227. doi: 10.1016/j.enpol.2018.01.050
Siegner, K., Wentzell, S., Urrutia, M., Mann, W., and Kennan, H. (2019). Maximizing Land Use Benefits from Utility Scale Solar: A Cost Benefit Analysis of Pollinator Friendly Solar in Minnesota. Yale Center for Business and Environmental Policy. Available online at: https://cbey.yale.edu/research/maximizing-land-use-benefits-from-utility-scale-solar (accessed April 26, 2022).
The National Academies (2008). Changes in Sheep Industry in the United States. Washington, DC: The National Academies. Available online at: https://www.nap.edu/resource/12245/SheepFinal.pdf (accessed April 26, 2022).
Toledo, C., and Scognamiglio, A. (2021). Agrivoltaic systems design and assessment: a critical review, and a descriptive model towards a sustainable landscape vision (three-dimensional agrivoltaic patterns). Sustainability 13, 6871. doi: 10.3390/su13126871
Tricco, A. C., Lillie, E., Zarin, W., O'Brien, K. K., Colquhoun, H., Levac, D., et al. (2018). PRISMA extension for scoping reviews (PRISMA-ScR): Checklist and explanation. Ann. Intern. Med. 169, 467–473. doi: 10.7326/M18-0850
Turconi, R., Boldrin, A., and Astrup, T. (2013). Life cycle assessment (LCA) of electricity generation technologies: overview, comparability and limitations. Renew. Sustain. Energy Rev. 28, 555–565. doi: 10.1016/j.rser.2013.08.013
U.S. Department of Energy (DOE) (2021a). Solar Futures Study. Washington, DC: Department of Energy, Office of Energy Efficiency and Renewable Energy. Available online at: https://www.energy.gov/eere/solar/solar-futures-study (accessed April 26, 2022).
U.S. Department of Energy (DOE) (2021b). SETO 2020 – Solar and Agriculture. U.S. Department of Energy, Solar Energy Technologies Office. Available online at: https://www.energy.gov/eere/solar/seto-2020-solar-and-agriculture (accessed April 26, 2022).
United Nations General Assembly (UN) (2015). Transforming our world: the 2030 agenda for sustainable development. General Assembly 70 Session 2015. Available online at: https://sustainabledevelopment.un.org
United States Department of Agriculture (USDA) (2021). Honey. Available online at: https://www.nass.usda.gov/Publications/Todays_Reports/reports/hony0321.pdf (accessed April 26, 2022).
Walston, L. J., Li, Y., Hartmann, H. M., Macknick, J., Hanson, A., Nootenboom, C., et al. (2021). Modeling the ecosystem services of native vegetation management practices at solar energy facilities in the Midwestern United States. Ecosyst. Serv. 47, 101227. doi: 10.1016/j.ecoser.2020.101227
Walston, L. J., Mishra, S. K., Hartmann, H. M., Hlohowskyj, I., McCall, J., Macknick, J., et al. (2018). Examining the potential for agricultural benefits from pollinator habitat at solar facilities in the United States. Environ. Sci. Technol. 52, 7566–7576. doi: 10.1021/acs.est.8b00020
Weselek, A., Ehmann, A., Zikeli, S., Lewandowski, I., Schindele, S., Högy, P., et al. (2019). Agrophotovoltaic systems: applications, challenges, and opportunities: a review. Agron. Sustain. Dev. 39, 35. doi: 10.1007/s13593-019-0581-3
Keywords: agrivoltaics, solar energy, renewable energy, sustainable development, ecosystem services, biodiversity, climate change mitigation
Citation: Walston LJ, Barley T, Bhandari I, Campbell B, McCall J, Hartmann HM and Dolezal AG (2022) Opportunities for agrivoltaic systems to achieve synergistic food-energy-environmental needs and address sustainability goals. Front. Sustain. Food Syst. 6:932018. doi: 10.3389/fsufs.2022.932018
Received: 03 May 2022; Accepted: 15 August 2022;
Published: 16 September 2022.
Edited by:
Ngonidzashe Chirinda, Mohammed VI Polytechnic University, MoroccoReviewed by:
Frank De Ruijter, Wageningen University and Research, NetherlandsMohd Ashraf Zainol Abidin, Universiti Teknologi Mara Perlis Branch, Malaysia
Helene Marrou, Montpellier SupAgro, France
Copyright © 2022 Walston, Barley, Bhandari, Campbell, McCall, Hartmann and Dolezal. This is an open-access article distributed under the terms of the Creative Commons Attribution License (CC BY). The use, distribution or reproduction in other forums is permitted, provided the original author(s) and the copyright owner(s) are credited and that the original publication in this journal is cited, in accordance with accepted academic practice. No use, distribution or reproduction is permitted which does not comply with these terms.
*Correspondence: Leroy J. Walston, bHdhbHN0b25AYW5sLmdvdg==