- 1Division of Soil Science and Agricultural Chemistry, ICAR-Indian Agricultural Research Institute (ICAR-IARI), New Delhi, India
- 2School of Public Health, University of California, Berkeley, Berkeley, CA, United States
- 3Department of Plant Production, College of Food and Agriculture Sciences, King Saud University, Riyadh, Saudi Arabia
- 4Division of Grassland and Silvopasture Management, ICAR-Indian Grassland and Fodder Research Institute (ICAR-IGFRI), Jhansi, India
Massive amounts of rice straw (RS) provide a significant problem for in situ and instant management at a low cost. On the other hand, mobilizing soil phosphorus (P) from inorganically fixed pools may increase its effectiveness over time. To address both of these issues at once as well as to decide the optimum rate of RS for P mobilization, this study was carried out to determine whether the use of RS + P solubilizing microbes (PSMs) could solubilize a sizable portion of the soil's fixed P and affect P transformation, silicon (Si) concentration, organic acid (OA) concentration, and enzyme activity to increase wheat yield. Depending on the soil temperature, the application of RS (at 12 Mg ha−1) + PSM could solubilize 3.40–3.66% of the inorganic P. Over control, it minimized the hysteresis impact by 6–8%. The soils of wheat included the acids oxalic acid, citric acid, formic acid, malic acid, and tartaric acid. At maturity stage of wheat, application of RS (at 12 Mg ha−1) + PSM + 75%P raised the activity of dehydrogenase, alkaline phosphatase activity, cellulase, and peroxidase by 1.77, 1.65, 1.87, and 1.82 times above control in soil, respectively. It also boosted Si concentration in the soil increased by 58% over control. Wheat grain yield was 40 and 18% higher under RS (at 12 Mg ha−1) + PSM + 75%P application than under control and 100% P application. Additionally, it increased root volume, length, and P uptake by 2.38, 1.74, and 1.62 times above control, respectively. According to path analysis, P solubilisation by Si and OAs considerably increased P availability in the wheat root zone. Therefore, cultivators could be advised to use RS (at 12 Mg ha−1) + PSM + 75% P of mineral P fertilizer to save 25% P fertilizer without reducing yield.
1. Introduction
Burning field crop waste has become a yearly occurrence in the heavily populated rural regions of Southeast Asia, China, and India (Shyamsundar et al., 2019). Farmers have been compelled by the constant need to boost agricultural output to utilize methods that frequently contribute to the ecological imbalance in the agroecosystem. This is especially true in North-Western India, where agricultural residue burning is extensive. Although the adoption of the “National policy for management of crop residues” (NPMCR, 2014), combustion of crop wastes persists. In India, field burning accounts for over 16% of all agricultural waste, with rice straw (RS) making up 60% of that trash (Manna et al., 2021). This is a significant source of atmospheric aerosol and gas emissions, potentially affecting the chemistry of the environment and the quality of the world's air. However, if managed properly, crop leftovers could restore soil organic matter, a crucial factor in determining the quality of the soil, and, when mineralized, deliver vital plant nutrients (Modak et al., 2019; Ghosh et al., 2023).
For the wheat crop, phosphorus is a crucial macronutrient to get the highest yields (Kruse et al., 2015). P shortage in highland crops like wheat occurs mostly because of changes in the inorganic components, such as iron phosphate, aluminum phosphate, and calcium phosphate complexes (Kruse et al., 2015). P accumulates in the soil when P input from various sources is higher than P depletion due to crop removal. The surplus P from fertilizer tends to build iron phosphate, aluminum phosphate, and calcium phosphate complexes in semi-arid Inceptisols of India. Even while inorganic and organic P are abundant throughout most croplands, they are immobile and mostly inaccessible to crops. Only a very low concentration of P (0.1% of the total P) is accessible to plants due to the strong reactivity of P with specific metals, such as calcium (Ca), iron (Fe), and aluminum (Al), and the creation of metal complexes that cause 75–90% of applied P to precipitate or adsorb in the soils causing P acute deficiency in many agricultural soils and plants (Adesemoye and Kloepper, 2009). To increase P availability for plants regularly, a need to find an alternative method using naturally available resources is urgent.
The sorption-desorption properties of the soil regulate the availability of applied or solubilised P. According to studies, adding organic residues to soils increases P availability by preventing the formation of exposed hydroxyls on the surface of Fe and Al minerals (Kruse et al., 2015), as well as the competition between organic acids and phosphate ions for adsorbing sites (Grybos et al., 2009). In addition to this, upon decomposition of RS, silicate ions () are released. Silicate and phosphate ions compete with one another in solution for adsorbing sites on Al or Fe complexes, leading to the formation of Fe/Al-silicate and liberation of phosphate in the soil (Wang, 2021). According to Nwoke et al. (2004), the impact of organic residues on P availability varied and was influenced by both the composition of the organics and the soil's properties. Relative to no residue, corn stover enhanced resin-P; however, the amplitude of the increase was higher in soils with low P sorption capacity than in soils with high P sorption capacity. The organic wastes can also solubilise native soil P to its available form (Nwoke et al., 2004; Alori et al., 2017). The impact of crop residues on soil P availability and transformation is not entirely clear. This is a compelling researchable issue because the contribution of rice residues to the P nutrition of the wheat crop in the IGP of South Asia could be significant.
However, the ideal RS application rate for P solubilisation is yet unknown. Depending on the soil type, climate, agricultural pattern, and management technique, the rate would differ. An extremely high RS application rate might, because of the priming effect, immobilize the soil nutrients. On the other hand, a little amount of RS might not be adequate to release enough Si and OAs to significantly mobilize fixed P (Menezes-Blackburn et al., 2018). It may be possible to solubilize the adsorbed or fixed inorganic P and diminish RS combustion by using RS combined with P solubilizing microorganisms (PSMs). PSMs would hasten RS breakdown as well as soil acidification to solubilize resistant P compounds in soils. By reducing P deficiency, promoting plant absorption of P, and increasing Si availability to plants in the soil, the application of RS could improve crop yield (Hu et al., 2018; Li et al., 2019).
Another important area of research is understudied and concerns the effect of RS treatment on P transformation in the soil during crop development. There is a lack of knowledge on how applying RS + PSM impacts P mobilization, the concentration of organic acids, the activity of soil enzymes, and the root architecture of plant. We postulated that using RS + PSM would speed up the solubilisation of inorganic fixed P by lowering the hysteresis effect and modifying the soil environment by increasing the level of OAs and competitive ions like silicate. The objectives of the study was to (a) identify appropriate combinations of RS + PSMs for solubilisation of fixed P and (b) develop a fertilizer management strategy for utilizing RS as Si source. The objective of this study was to understand the effects of crop residue and P management strategies on crop yield, transformation, solubilisation, and mineralization pattern of P in addition to P availability in soil–plant systems.
Consequently, this study's design included two experiments. The first trial was carried out in the lab to determine how much RS should be applied to (a) considerably solubilize a considerable proportion of the fixed P from soil; and (b) significantly desorb the adsorbed P by minimizing the hysteresis impact. The two best RS+PSM combinations were used in the second experiment to assess their effects on the test crop, wheat, as well as the soil's P transformation, Si concentration, organic acid concentration, and enzyme activity.
2. Materials and methods
2.1. Soil collection and rice straw preparation and their properties
The soil used in this experiment was rich in total phosphorus sourced from the ICAR-Indian Agricultural Research Institute's research farm inNew Delhi, India. The soil of the experimental field is classified as Typic Haplustept, which is an Indo-Gangetic plains alluvial association (28°37′-28°39′N, 77°9′-77°11′E, 220 m above mean sea level). The soil is a non-calcareous alkaline sandy loam with low cation exchange capacity. Specifically, the study area has a semi-arid, subtropical climate characterized by hot, dry summers (May–June) and cold winters (December–January). The mean minimum and maximum temperatures of summer are 26.4 and 44.1°C, respectively, while the mean minimum and maximum temperatures of winter are 5.6 and 23.7°C, respectively. Meanwhile, the annual average maximum and minimum temperatures are 40.5 and 6.5°C, respectively. The average annual rainfall is about 760 mm, while 80% of rainfall is received from July–September. Rice straw was collected, air dried, and chopped into pieces before using it in the experiment. The detailed properties of soil and RS are described in the Table 1.
2.2. Experiment 1: Incubation experiment and measurement of P mineralization and hysteresis for screening of efficient combination of RS and PSMs
For experiment 1, the soil was treated using various amounts of RS and PSMs. The soils (100 g, oven-dried weight) were re-equilibrated to 80% of their water-holding capacity after being pre-incubated for 5 days at 27°C (Reynolds et al., 2017). The previously incubated soils were treated with PSMs and varied RS concentrations. The soil was inoculated with liquid cultures of Pseudomonas striata (108CFU mL−1) and Aspergillus niger (108 spores mL−1). P striata was selected for its P solubilizing ability and A niger was selected for its ability to degrade RS. After that, RS was applied to the soil with and without PSMs at concentrations of 3,570 (i.e., 8 Mg ha−1), 4,465 (i.e., 10 Mg ha−1), 5,355 (i.e., 12 Mg ha−1), and 6,250 mg kg−1 (i.e., 14 Mg ha−1). Ten combinations were achieved, namely, T0 (control: no PSMs and no RS), T1 (3,570 mg RS kg−1 soil), T2 (3,570 mg RS kg−1 soil + PSMs), T3 (4,465 mg RS kg−1 soil), T4 (4,465 mg RS kg−1 soil + PSMs), T5 (5,355 mg RS kg−1 soil), T6 (5,355 mg RS kg−1 soil + PSMs), T7 (6,250 mg RS kg−1 soil), T8 (6,250 mg RS kg−1 soil + PSMs), and T9 (PSMs only). The treated soils were stored in incubators for 90 days in three replications, preserving moisture content at 0.033 MPa (measured using a pressure plate apparatus) and temperatures at 25 and 35°C. By extracting soil P with 0.5 M NaHCO3 (pH = 8.5) the plant-available forms of P were determined after the 2nd, 7th, 15th, 30th, 45th, 60th, and 90th days of incubation (Olsen, 1954), representing the active growth duration of wheat. After 90 days of incubation, using the modified P fractionation scheme of Kuo (1996), different forms of inorganic soil P, including saloid bound P, Al bound P, Fe bound P, reductant soluble P, and Ca bound P, were measured. These P forms included ammonium chloride extractable P (NH4Cl-P), ammonium fluoride extractable P (NH4F-P), sodium hydroxide extractable P (NaOH-P), citrate-bicarbonate extractable P (CBD-P), and sulfuric acid extractable P (H2SO4-P). As soil P mobilization occurs by solubilisation of inorganically bound P and mineralization of organic P, we selected the following two-pool model to study its kinetics (Ghosh et al., 2023):
P denotes P that is still accessible in Equation 1 after t days of incubation. P0 is mineralisable organic P. The values of k1 and k2 represent the rates of inorganic P solubilisation and organic phosphorus mineralization, respectively.
Three grams of soil from each 90-day incubated sample were obtained, together with 30 mL of CaCl2 solution (concentration: 0.01 M and ionic strength: 0.03) containing P, 0, 5, 10, 20, 30, 50, 70, and 100 mg L−1, to evaluate the effects of various hydrothermal regimes on phosphate sorption. After centrifugation and decantation, the soil was again exposed to the same CaCl2 solution and mixed for the same amount of time as in the sorption kinetics in order to investigate phosphate desorption kinetics. For the sorption and desorption study, P concentration was estimated. The P concentrations during adsorption and desorption were fitted to Langmuir and Freundlich isotherms.
The parameters x denoted the adsorbed anion concentration (mg kg−1), c stands for the equilibrium anion concentration (mg L−1), kL the adsorption energy parameter, and Sm the maximal adsorption capacity (mg kg−1). The adsorption constant, abbreviated kF, denotes the energy of adsorption on a uniform surface, whereas the parameter n has a value between 0 and 1. The difference between the center regions underneath the sorption (As) and desorption (Ad) lines was used to determine the hysteresis index (H) (Deng et al., 2010),
Si was extracted from soils using CaCl2 solution (concentration: 0.01 M) (1:10 ratio and shaking time 16 h) following 90 days of incubation (Berthelsen et al., 2003) and measured spectrophotometrically using the molybdate blue color technique (Kilmer, 1965).
2.3. Experiment 2: Pot experiment using efficient combinations of RS and PSMs
To evaluate the efficacy of RS + PSMs to lower P fertilizer needs while maintaining crop production, a pot experiment research was undertaken. Based on the findings of the laboratory investigation, six fertilizer management strategies were chosen for the trial. They were control (M0), RS at 12 Mg ha−1 + PSMs + 50% recommended dose of P (M1), RS at 12 Mg ha−1 + PSMs + 75% recommended dose of P (M2), RS at 14 Mg ha−1 + PSMs + 50% recommended dose of P (M3), RS at 14 Mg ha−1 + PSMs + 75% recommended dose of P (M4), and 100% recommended dose of P (M5). In all treatments recommended doses of nitrogen and potash were applied. The recommended optimum doses of nitrogen, phosphorus and potassium doses were 267, 45 and 135 mg pot−1, respectively for the maximum yield of wheat under irrigation in India (Biswas et al., 2022). The nutrient elements were applied through urea, single superphosphate, and muriate of potash. Wheat (cv HD 2967) was chosen as a test crop because it is cultivated in a large area immediately after harvesting rice (middle of October) in India. Wheat was sown in the first week of November and harvested in the first week of April. To collect plant and soil samples, during crown root initiation (CRI), flowering (FLO), and maturity (MAT) stages of wheat, separate pots with these treatments were maintained for each stage. Three replicates were tested for each treatment in each crop growth stage. Hence, a total of fifty-four (6 treatments × 3 replication × 3 growth stages) pots were filled with soil columns (33 cm long and 15 cm diameter) containing semi-arid subtropical Inceptisol (5 kg soil in each pot).
2.4. Soil and crop sampling and their analyses
Soils from the pots were collected during crown root initiation (CRI), flowering (FLO), and maturity (MAT) stages of wheat after uprooting the whole plants from the pot. The concentrations of OAs in the soil were estimated using an HPLC. The amount of inorganic P fractions were measured (Kuo, 1996) and organic P was quantified by the ignition method (700°C in presence of air). Si concentration (Berthelsen et al., 2003) and soil pH were also measured in the soil. Activities of enzymes, such as dehydrogenase, alkaline phosphatase, cellulase, and peroxidase were estimated in soil using tetrazolium chloride (Casida et al., 1964), p-nitro phenyl-phosphate (Tabatabai and Bremner, 1969), carboxymethyl cellulose (Deng and Tabatabai, 1994), and 2,2′-azino-bis(3-ethylbenzothiazoline-6-sulfonic acid) (ABTS) (Bach et al., 2013) as substrates, respectively. At each stage of the sampling process, whole plant samples were carefully taken from each pot. Roots from the plant samples were then cleaned twice—once with tap water and once with distilled water—and then root length and volume were measured using a root scanner. Individual mass of straw and grain shoot was measured at the maturity stage. The Wiley mill was used to grind the oven-dried (65°C) plant samples, which were then digested with di-acid (HNO3:HClO4 at 9:4 ratio) and spectrophotometrically measured for total P content using the vanadomolybdophosphate yellow color technique (Jackson, 1973).
2.5. Statistical analyses
All the data were analyzed using the Analysis of Variance (ANOVA) technique, and the Tukey's Honestly Significant Difference (HSD) test was employed to distinguish between significantly different mean values for every variable. We developed a path model (also known as a structural equation model) for the wheat using various latent variables to evaluate the direct and indirect effects of several factors, including soil chemical environment (pH and Si), microbial environment (activities of dehydrogenase, alkaline phosphatase, cellulase, and peroxidase), and plant-microbial factor (OAs) on P mobilization in the root zone of wheat. Strong model fits were indicated by high GFI (>0.9) and low RMSEA (< 0.08). The magnitude of the arrows determines the latent variables' overall influence.
3. Results
3.1. P mineralization and transformation during incubation
The use of RS + PSM improved P mobilization significantly. T6 mobilized the most P under both 25 and 35°C. For T6, cumulative P mobilization at 90 days after incubation (DAI) was 58, 35, and 20% greater at 25°C than T2, T4, and T8. Gross P mobilization at 90 DAI was 49, 30, and 12% higher for T6 at 35°C than that of T2, T4, and T8, correspondingly (Table 2). Despite having greater RS, T6 had considerably higher cumulative P mobilization than T8 at 25 and 35°C. When RS was supplied alone at various doses, P mobilization increased in comparison to control (Table 2). However, at all temperatures, RS application was less successful than the RS + PSM combination for P mobilization. The rate of organic P mineralisation was similar under T2, T4, and T6, and was 4 and 2.5 folds greater than under T0 and T9, correspondingly. T1, T3, and T5 exhibited identical rates of organic P mineralisation, which became 2.21 and 1.39 times greater than T0 and T9, correspondingly, at 25°C. At 35°C, similar outcomes were observed. At 250°C, the rate of inorganic P solubilisation was 2.22, 1.59, and 1.11 folds greater under T6 than under T2, T4, and T8. However, at 35°C, the rate of inorganic P solubilisation under T6 was 2.13, 1.73, and 1.04 times higher than under T2, T4, and T8, correspondingly (Table 2).
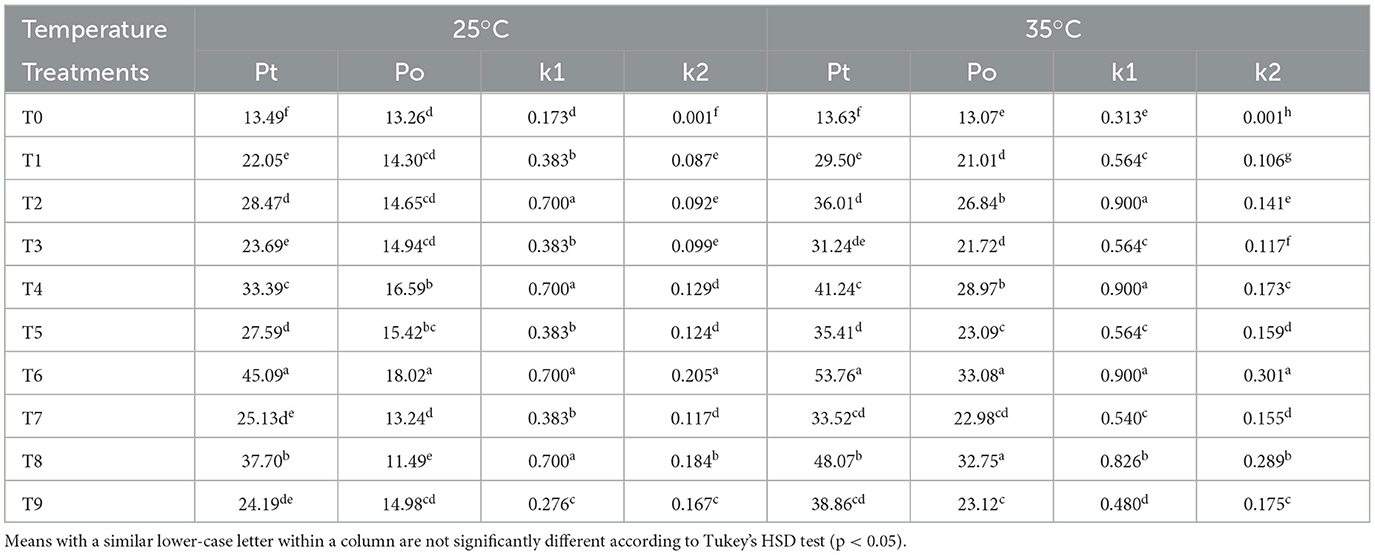
Table 2. Impact of rice straw and PSM application on cumulative P mineralization (Pt; mg kg−1), organic P mineralization (Po; mg kg−1), rate of solubilisation of inorganic P (k1; day−1), and rate of solubilisation of organic P (k2; day−1) at 25 and 35°C temperature in a semi-arid Inceptisol.
Usage of RS alone strived to transform inorganic soil P considerably in all cases. T4, T6, and T8 enhanced NH4Cl-P by 52, 33, and 40%, respectively, over T0 at 25°C. Over T0, T6, and T8 mobilized 13.4 and 10.3% of NH4F-P, 17.7 and 12.7% of NaOH-P, and 2.28 and 1.74% of H2SO4-P, correspondingly (Figure 1). The CBD-P, on the other hand, did not differ significantly. Nonetheless, net inorganic P solubilisation was 27.1 and 26.2 mg kg−1 under T6 and T8, respectively. Organic P mineralization was 56% greater under T6 than under T8. T4, T6, and T8 increased NH4Cl-P by 50, 35, and 54%, respectively, over T0 at 35°C. Compared to T0, T6, and T8 mobilized 8 and 21% of NH4F-P, 25 and 15% of NaOH-P, and 2.43 and 2.11% of H2SO4-P, correspondingly (Figure 1). Nonetheless, net inorganic P solubilisation was 20.7 and 15.3 mg kg−1 under T6 and T8, respectively. Organic P mineralization for T6 was fairly comparable to T8. At 35°C, cumulative P mobilization was substantially higher than at 25°C (Table 2).
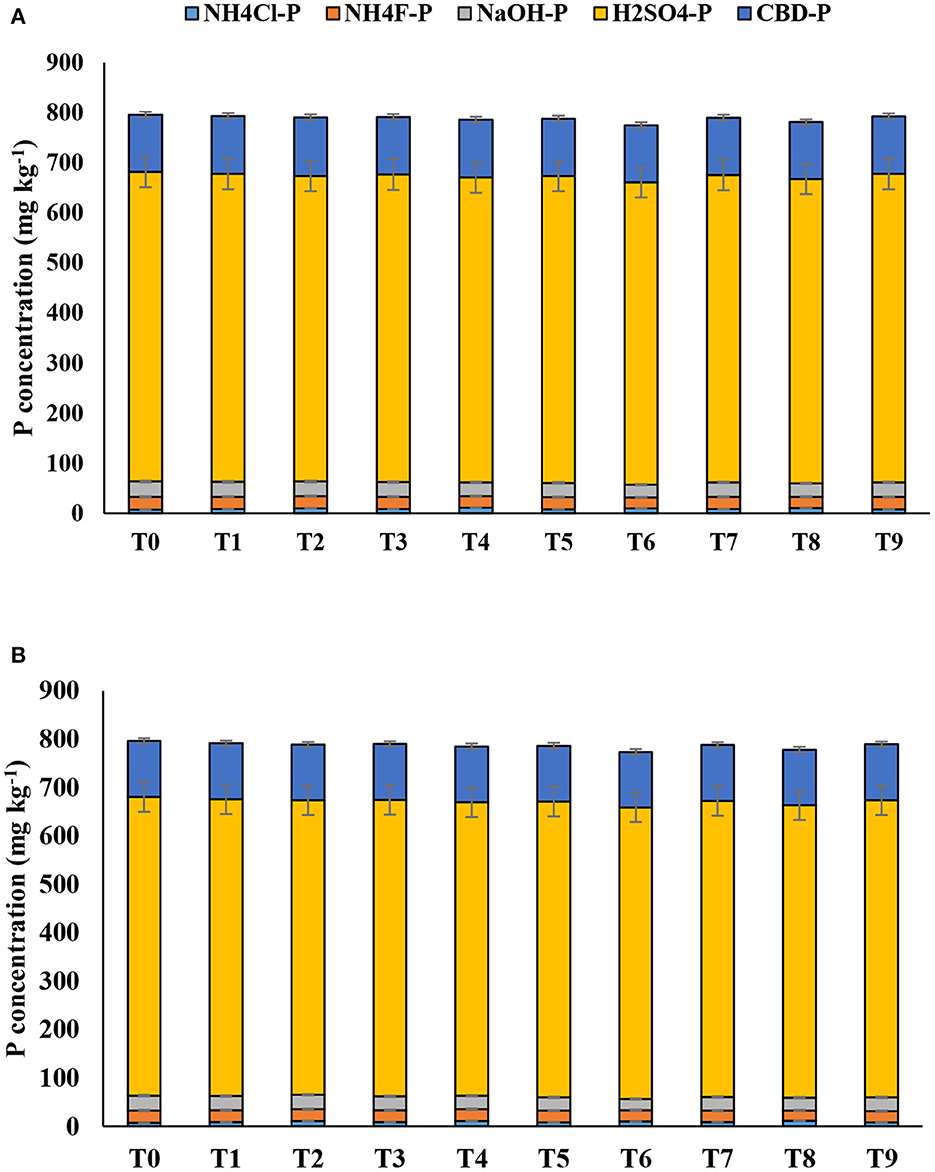
Figure 1. Changes in ammonium chloride extractable P (NH4Cl-P), ammonium fluoride extractable P (NH4F-P), sodium hydroxide extractable P (NaOH-P); citrate-bicarbonate extractable P (CBD-P), and sulfuric acid extractable P (H2SO4-P) as impacted by varying doses of rice straw application and phosphorus solubilising microbes' application at (A) 25 and (B) 35°C in a semi-arid subtropical Inceptisol. Error bars indicate LSD (p = 0.05).
3.2. P hysteresis and Si availability during incubation
The combination of RS and PSM had a significant effect on the adsorption isotherms, but neither RS nor PSM separately could. T6 and T8 greatly minimized hysteresis and adsorption parameters compared to T0 and T9. T6 and T8 reduced hysteresis by 6.5 and 8.6%, kF value by 8 and 11%, n value by 15 and 20%, kL by 13 and 13%, and Sm by 29 and 37% compared to control at 25°C (Table 3). The very same features were observed at 35°C. Because the modifications in isotherm attributes resulted in a comparable drop in hysteresis and adsorption properties, T6 and T8 might be efficient in decreasing the adsorption of supplied P while simultaneously boosting the desorption of previously adsorbed P. Soil Si content was significantly improved by utilizing RS and RS+PSM. At 25°C, T6 and T8 soil exhibited 64 and 73% greater Si contents than T0. A similar trend was seen at 35°C (Table 3). Because the T6 and T8 transformed a bigger quantity of P, they may be convincing for P solubilisation and assist plants for P intake. When compared to the control, solitary RS administration at various dosages had a substantial influence on P transformation. Because P transformation with RS + PSM was more successful than RS application based on Si release and hysteresis effect, T6 and T8 were chosen for further investigation with the test crop in the subsequent stage.
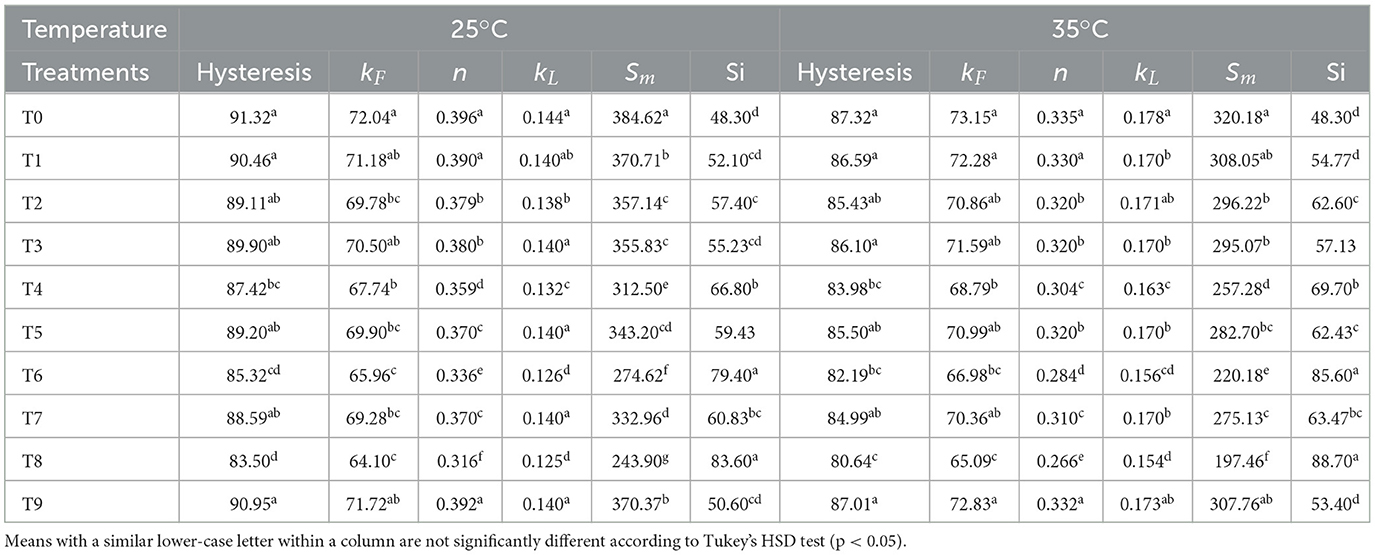
Table 3. Impact of rice straw and PSM application on the parameters of Freundlich and Langmuir adsorption isotherms, phosphorus hysteresis (%), and silicon availability (Si; mg kg−1) in a semi-arid Inceptisol.
3.3. P transformation in the soil during different growth stages of wheat
M2 and M4 possessed 75 and 77% higher NaHCO3-P, and 36 and 56% higher NH4Cl-P, respectively, at the CRI stage as compared to control (Figures 2A, B). Similar patterns were seen during the flowering and maturation periods of wheat. M2 and M4 solubilised only a small quantity of NH4F-P during the CRI phase. Under M2 and M4, there was net NH4F-P fixation, however, during this flowering period, they solubilized around 7.3 and 7.9% NH4F-P (Figure 2C). At mature stage, M2 and M4 dissolved 21 and 17% of NH4F-P, respectively. M2 and M4 dissolved 16 and 10% NaOH-P at the flowering stage of wheat, and 21 and 16% NaOH-P during the maturity stage, correspondingly (Figure 2D). Following earlier fixation in the CRI stage of wheat, progressive solubilisation of H2SO4-P was detected during the flowering and maturation stages (Figure 2E). The CBD-P, on the other hand, did not vary appreciably throughout crop growth, even though some P was fixed as CBD-P at the CRI and flowering stages of wheat (Figure 2F). After initial P entrapment at the CRI stage of wheat, consistent hydrolysis of organic P was detected during the flowering and maturity stages (Figure 3A).
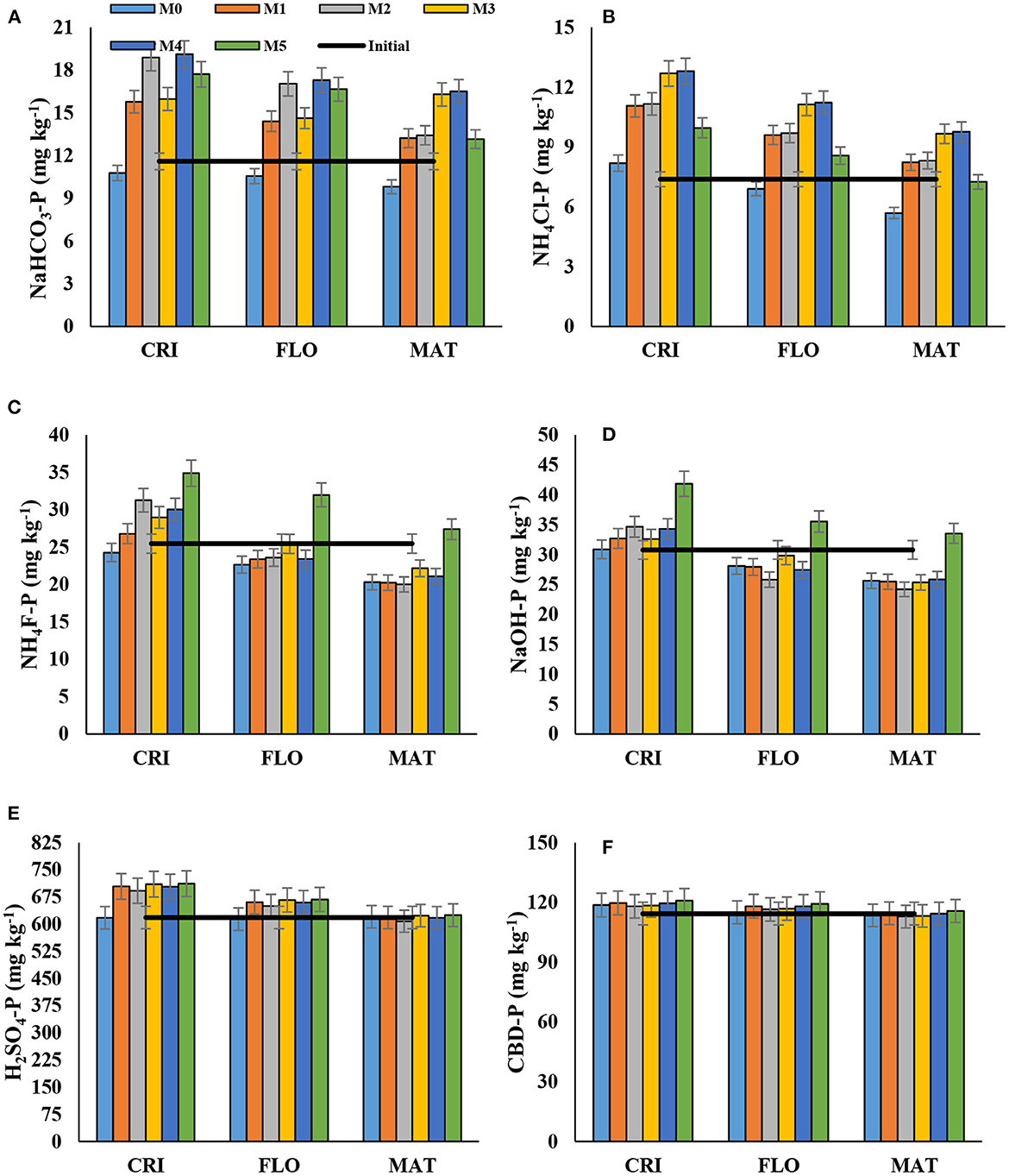
Figure 2. Changes in (A) plant available P (NaHCO3-P), (B) ammonium chloride extractable P (NH4Cl-P), (C) ammonium fluoride extractable P (NH4F-P), (D) sodium hydroxide extractable P (NaOH-P), (E) sulfuric acid extractable P (H2SO4-P), and (F) citrate-bicarbonate extractable P (CBD-P) in the soil during crown root initiation (CRI), flowering (FLO), and maturity (MAT) stages of wheat in a semi-arid subtropical Inceptisol. Error bars indicate LSD (p = 0.05).
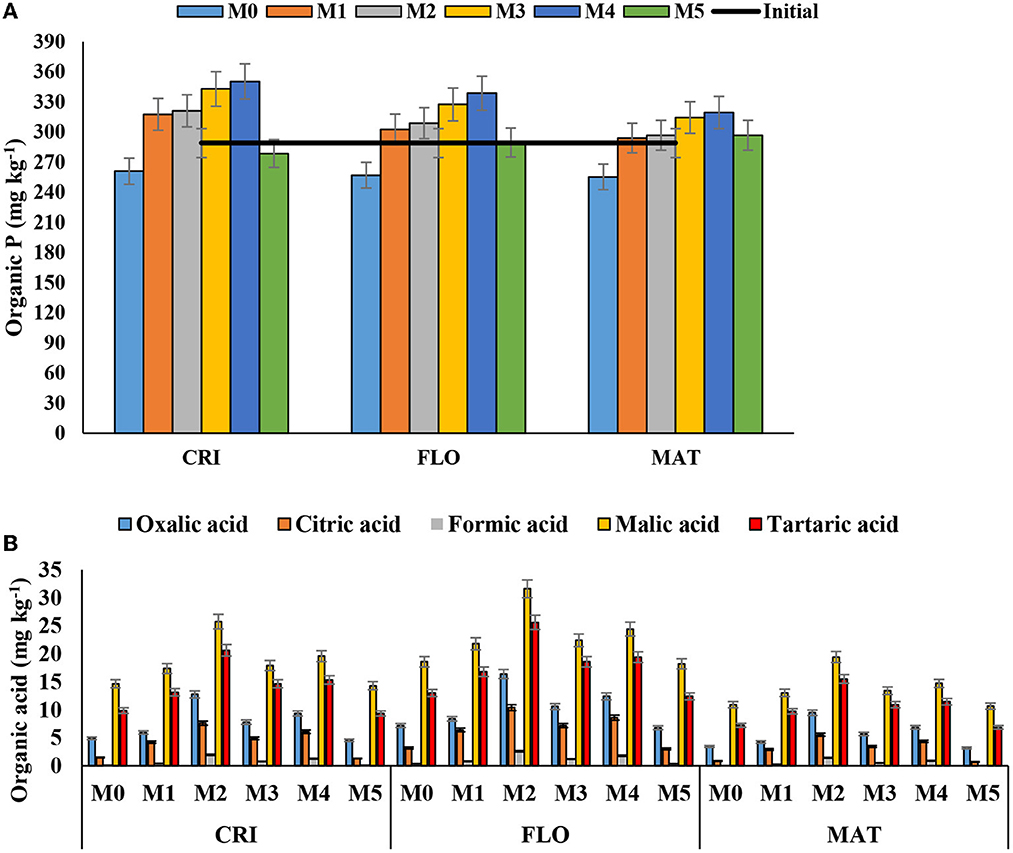
Figure 3. Changes in (A) soil organic P and (B) organic acid concentrations in the soil during crown root initiation (CRI), flowering (FLO), and maturity (MAT) stages of wheat in a semi-arid subtropical Inceptisol. Error bars indicate LSD (p = 0.05).
3.4. Activities of soil enzymes and organic acids in the soil during different growth stages of wheat
There were five organic acids, found in the soil, such as oxalic acid, citric acid, formic acid, malic acid, and tartaric acid. The maximum level of every OA was found during the flowering period of wheat, followed by the CRI and mature stages (Figure 3B). Under M2, the quantity of OAs was the greatest in all stages of wheat. However, in all stages of wheat, M3 and M4 exhibited considerably higher OA contents than M5 and M0 (Figure 3B). At the CRI stage, oxalic acid, citric acid, formic acid, malic acid, and tartaric acid levels were 2.8, 5.8, 21.1, 1.8, and 2.2 times higher than M5. Similar patterns were seen during the flowering and maturation periods of wheat.
During the flowering stage of wheat, the maximum level of activity for every enzyme was observed. The addition of RS + PSM considerably increased the activity of soil enzymes over M0 and M5. During the CRI stage of wheat, the activities of DHA, alkaline phosphatase, cellulase, and peroxidase enhanced by 1.59, 1.50, 1.25, and 1.62 times, correspondingly, under M2 over M5 (Figures 4A–D). However, the activities of DHA, alkaline phosphatase, cellulase, and peroxidase improved by 1.87, 1.75, 1.48, and 1.91 times, respectively, when M4 was used over M5 during the CRI stage of wheat. Similar patterns were identified during the flowering and maturation periods of wheat (Figures 4A–D).
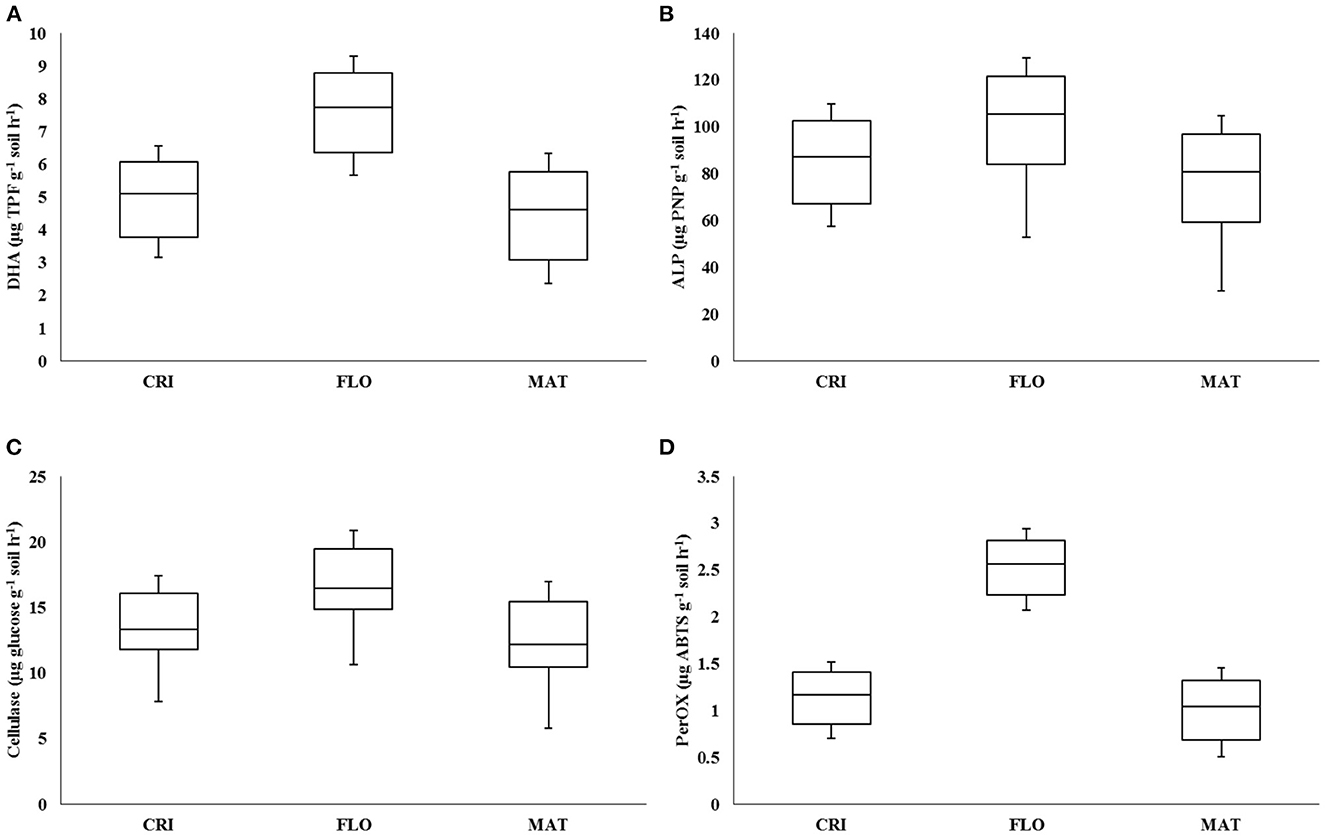
Figure 4. Changes in (A) dehydrogenase (DHA), (B) alkaline phosphatase (ALP), (C) cellulase, and (D) peroxidase (PerOX) activity in the soil during crown root initiation (CRI), flowering (FLO), and maturity (MAT) stages of wheat in a semi-arid subtropical Inceptisol.
3.5. Si availability and pH in the soil during different crop growth stages
The use of RS+PSMs over control and M5 substantially enhanced Si levels in the soil. It also grew steadily in CRI, blooming, and maturity stages (Table 4). M2 and M4 contained 17 and 21, 43 and 47, and 58 and 63% higher Si contents at CRI, flowering, and maturation stages of wheat, respectively, than M0. During different phases of wheat growth, the Si content in the soil under M0 and M5 did not vary appreciably. RS + PSMs drastically decreased soil pH compared to control and M5 throughout crop growth. M2 and M4 retained a 10% lower soil pH than M0 and a 7% lower soil pH than M5. The concentrations of OAs were positively correlated with the reduction in soil pH.
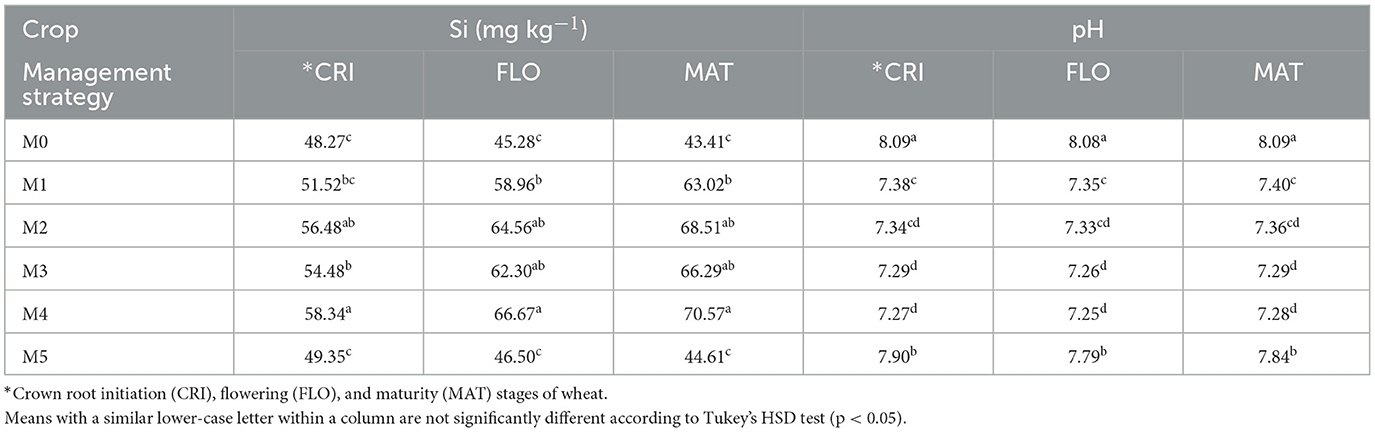
Table 4. Impact of rice straw and PSM application on silicon availability [Si; (mg kg−1)] and pH in the soil during different growth stages of wheat in a semi-arid Inceptisol.
3.6. Root traits, yield and P uptake of wheat
M2 resulted in the greatest grain output of wheat. Wheat grain production under M2 and M4 was 40 and 27% greater than M0, respectively, and 18 and 8% greater than M5. Wheat straw output under M2 and M4 was 1.39 and 1.26 times greater than M0, respectively, and 1.18 and 1.07 times greater than M5 (Table 5). P acquisition by wheat grain under M2 and M4 increased by 91 and 32% over M0, and 72 and 19% over M5, correspondingly. Total P absorption by wheat improved by 36 and 19% under M2 and M4, respectively, over control (Table 5). Wheat root characteristics exhibited a close linkage with P acquisition. Wheat root length with M2 and M4 was 1.78 and 1.22 times larger than M0 at the CRI stage, 1.88 and 1.37 times bigger than M0 at the flowering phase, and 1.74 and 1.32 times higher than M0 at the maturity stage (Table 5). Identical to this, at the CRI stage, the root volume of wheat under M2 and M4 was 2.68 and 2.14 times higher than M0, respectively. At the flowering phase, root volume was 2.50 and 2.17 times higher than M0, respectively. At the mature stage, root volume was 2.38 and 2.13 times greater than M0, respectively. In all three development phases, M2 and M4 developed larger root lengths and volumes of wheat than M5.
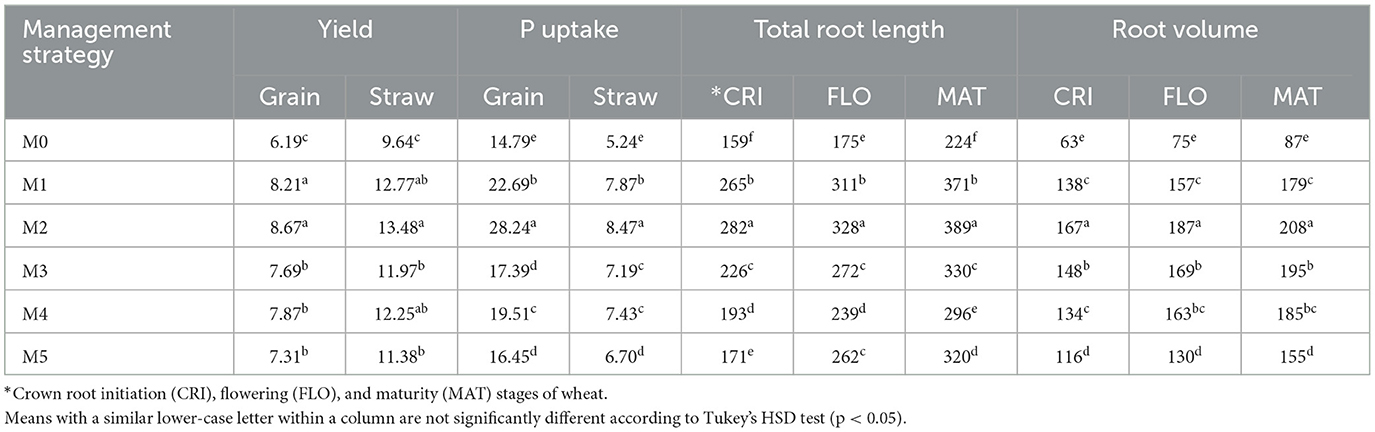
Table 5. Impact of rice straw and PSM application on the grain and straw yield (mg pot−1), P uptake (mg pot−1), and root length (cm plant−1) and volume (cm3 plant−1) of wheat in a semi-arid Inceptisol.
3.7. Interrelationship of available phosphorus with other soil parameters
Soil available P (NaHCO3-P) was positively correlated (R2 > 0.768, p < 0.05) with saloid bound P during all stages of wheat. Among other fractions of inorganic soil P, NH4F-P (R2 > 0.678, p < 0.05) and NaOH-P (R2 > 0.713, p < 0.05) were significantly correlated with NaHCO3-P at the flowering and maturity stage of wheat. However, H2SO4-P and CBD-P were not correlated with available P in any stage of crop growth. The concentration of Si in soil was positively correlated (R2 > 0.793, p < 0.05) with available P during all stages of wheat. However, the concentrations of OAs were not correlated with available P in any stages of crop growth. Interestingly, concentrations of OAs were significantly correlated with root length (R2 > 0.729, p < 0.05) and volume (R2 > 0.716, p < 0.05) of wheat in all stages. The dehydrogenase activity in soil was positively correlated (R2 > 0.847, p < 0.05) with available P during all stages of wheat. The ALP activity in soil positively contributed (R2 > 0.821, p < 0.05) to available P during all stages of wheat. Cellulase and peroxidase activities in soil were positively correlated (R2 > 0.868 and R2 > 0.846, respectively; p < 0.05) with available P during all stages of wheat.
4. Discussion
4.1. Impact of RS application on P solubilisation and transformation during incubation study: Screening of efficient combination of RS + PSM
Due to the greater stability of H2SO4-P at the soil's initial pH value (8.2), a higher amount of NaOH-P and NH4F-P could be mobilized by RS + PSMs at 25°C. Furthermore, the introduction of RS + PSMs led to a significant substitution of by in the Fe-phosphate and Al-phosphate minerals, causing a release of in the soil solution. The NaOH-P and NH4F-P could be mobilized by ligand replacement of by , dissolving of Fe/Al-phosphate minerals by OAs, and other processes. The H2SO4-P is liberated during the breakdown of Ca-phosphate minerals by OAs. The surge in NH4Cl-P could be attributed to the sorption of solubilised and hydrolysed P on soil particles. Previous research suggested that PSM and Si might also help plants flourish in low-P environments (Kostic et al., 2017; Emami et al., 2019). PSMs worked successfully in the cycles of dissolution-precipitation, mineralization-immobilization, and sorption-desorption for transforming insoluble phosphates bioavailable (Etesami and Jeong, 2021). Organic acids, through ligand-induced dissolution, might remove P from mineral surfaces. Organic acids either competed with or displaced adsorbed phosphates for fixation sites on soil clay particles such as amorphous aluminum oxides, goethite, kaolinite, and montmorillonite (Etesami and Jeong, 2021). OAs helped in the liberation of NH4F-P, NaOH-P, and H2SO4-P by increasing the chelation with P-bound to Al3+, Ca2+, and Fe3+ or by forming soluble metal ion complexes and inhibiting P precipitation. The inclusion of RS + PSM enhanced the quantities of ions in soil solution. ions and OAs contended for adsorbent surface with phosphate ions in the solution, resulting in a significant decrease in kF (for Freundlich isotherm), kL, and Sm (for Langmuir isotherm) for P sorption. At a greater temperature, the quantity of ions and OAs increased, and RS + PSMs substantially reduced the adsorption behavior (such as kF and Sm) to enhance the amount of NaHCO3-P (Table 3).
The central atoms' removal from the structural configuration of the metal-ion complex might be more energy-intensive than its synthesis. This could impose hindrance on the desorption route during the P holding operations by the oxides. Organic anions, according to Afif et al. (1995), could compete with phosphorous for a similar adsorbent surface, reducing P sorption. This emphasized the necessity for and OAs to impede the P adsorption process. The use of RS raised the concentration of ions as well (Table 3). The adsorbent surface could also exchange ions for ions. This was understandable given that P adsorption was could fast fall while Si adsorption might be nearly at its peak in the pH range of 6–11. These outcomes indicated that the soil pH needed to be relatively high (>7.0) in for silicate treatment to properly restrict phosphate binding. Indeed, the utilization of RS+PSMs liberated OAs and Si, as well as aided in the mobilization of inorganic P. As indicated by increasing NH4Cl-P at 35°C, phosphate adsorption decreased with increasing silicate concentration and temperature in the solution (Figures 1A, B). At all temperatures, there was a significant relationship between the amount of phosphate mobilized from NaOH-P and NH4F-P and silica content (R2 > −0.823; P < 0.05), but OA concentration was strongly related to H2SO4-P (R2 > −0.769; P < 0.05). This obviously demonstrated that NaOH-P and NH4F-P mobilization was influenced by the liberation of ions by , whereas H2SO4-P solubilisation was impacted by the solvation of Ca-phosphate minerals through OAs. According to the incubation experiment, T6 and T8 could boost inorganic P mobilization to enhance availability by solubilizing up to 3.40–3.70% of fixed P (Figures 1A, B). As a result, they might be employed for agricultural production. T6 and T8 have been identified for the second experiment following the outcomes of the incubation study to confirm their performance during crop production.
4.2. Impact of RS application on soil environment
Greater activity of soil enzymes such as DHA, ALP, and cellulase under M1, M2, M3, and M4 compared to M0 and M5 could be attributed to higher substrate accessibility due to RS supply (Figures 4A–D). Cellulose generated through RS breakdown served as a substrate for cellulase enzymes in the soil (Ghosh et al., 2020). Greater cellulose concentrations in M2 and M4 as a result of RS treatment may have boosted their activity when compared to M0 and M5 (Becerra-Castro et al., 2015). According to reports, the peroxidase enzyme transformed resistant aromatic molecules like lignin into more labile substrates (Ghosh et al., 2019). Undoubtedly, enhanced peroxidase enzyme activity in M2 and M4 over M0 and M5 were related to increased substrate availability by RS + PSM treatment. According to Ghosh et al. (2019), crop leftovers can influence phosphatase activity in the soil. There was a strong connection between residue application and phosphatase activity in the soil of the current investigation. Increased enzyme activity resulted in quicker breakdown of RS, resulting in a considerable increase in OA concentration in the soils of the wheat root zone. Increased OA concentrations resulted in a decrease in pH in the soil under M2, M3, and M4 compared to M0 and M5. The breakdown of RS further resulted in an increase in Si concentration in the soil under M2, M3, and M4 over M0 and M5. Si increased soil P availability and plant P uptake by mobilizing or desorbing organic P from soil particles or mineral binding sites (e.g., goethite) via competitive exchange and P-Si sorptive interaction (Alam et al., 2022). OA mediated decomposition process assisted in the liberation of Al/Fe/Ca bound P. Mobilization of P from Al/Fe/Ca-phosphate compound resulted in a significant increase in saloid bound P in M2, M3, and M4 than M0 and M5.
4.3. Impact of RS application on yield and P uptake by crops
Higher P bioavailability resulted in its increased acquisition by wheat straw and grain. The structural equation modeling of soil parameters clearly highlighted changes in the mechanistic routes for enhancing P availability. The SEM revealed that soil pH and Si concentration dominated the soil chemical environment (Figure 5). The chemical environment of the soil had a substantial impact on the microbiological ecology, and vice versa. Furthermore, the chemical and microbiological environments of the soil had a substantial influence on the release of organic acids. The SEM demonstrated that the chemical environment of the soil, enzyme activity, and OAs all had a significant influence on P transformation in the soil (Figure 5). Higher soil enzyme activity resulted in quicker degradation of RS, increasing the amounts of Si and OAs in the soil under M2, M3, and M4 (Meena and Rao, 2021). The higher the Si content in the soil, the more competitive desorption of phosphate by silicate took place to liberate previously absorbed P. The pH of the soil was regulated at 7–8. Si adsorption inhibits phosphate adsorption in the pH range of 6–11. Furthermore, the OAs developed complexes with ionic species of Fe, Al, or Ca, releasing phosphate from iron, aluminum, and calcium phosphate compounds. This is substantiated by the decrease in NH4F-P, NaOH-P, and H2SO4-P in the soil under M2, M3, and M4 (Figures 2C–F). These mechanisms may also have minimized P hysteresis, enabling the release of adsorbed P, as seen in the first experiment. This is substantiated by the fact that NH4F-P, NaOH-P, and H2SO4-P levels increased significantly at the CRI stage and subsequently reduced during wheat flowering and maturity under M2, M3, and M4 (Figures 2C–F). By looking at the higher coefficients, it is apparent that the solubilized P moved mostly to NH4Cl-P and NaHCO3-P (Figure 5). Importantly, the significant negative coefficient for organic P indicated that the influence of soil organic P on NH4Cl-P and NaHCO3-P was also considerable (Figure 5). The transformation of P had a considerable influence on wheat production by improving P absorption.
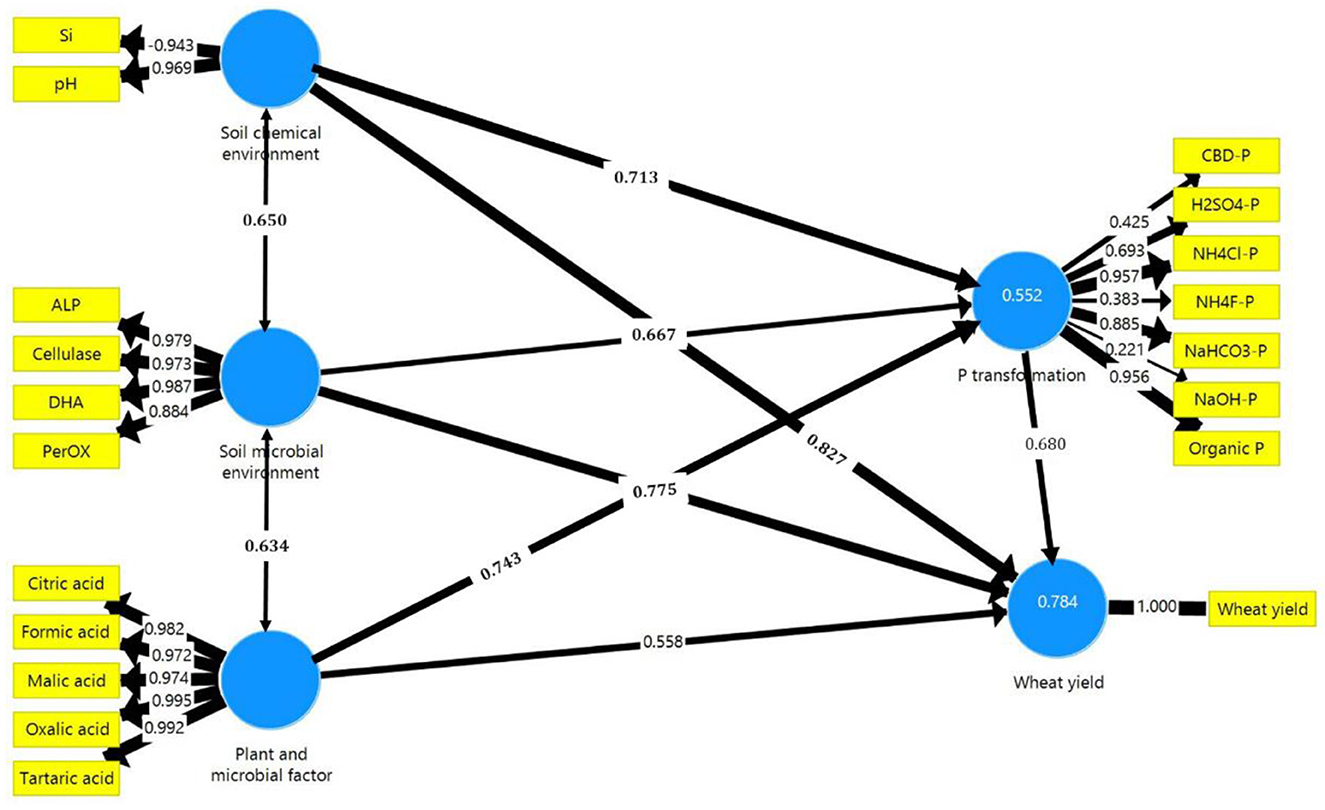
Figure 5. Path analysis to depict the impacts of soil chemical environment, soil microbial environment, and plant and microbial factors on phosphorus (P) mobilization in the soils of wheat root zone. Models satisfactorily fitted to data based on χ2 and RMSEA analyses [χ2 = 341.37, GFI = 0.92, RMSEA < 0.001]. Solid arrows represent the significant effects. The width of the arrows indicates the strength of the casual relationship. Si, silicon; pH, soil pH; ALP, alkaline phosphatase; DHA, dehydrogenase; PerOX, peroxidase; Org P, organic phosphorus mineralized; NH4Cl-P, ammonium chloride extractable P; NH4F-P, ammonium fluoride extractable P; NaOH-P, sodium hydroxide extractable P; CBD-P, citrate-bicarbonate extractable P; H2SO4-P, sulfuric acid extractable P.
P availability has a substantial influence on wheat productivity. Even though M3 and M4 had higher levels of RS, M2 delivered the best yield and the maximum P absorption by wheat. This might be related to the initial immobilization of nutrients under M3 and M4 due to the use of a high amount of RS, which impeded crop development substantially, lowering production and P absorption.
P acquisition was strongly influenced by root properties, which included root length and root volume. Greater root volume and length under M2 enabled the root system to traverse more soil and offered a higher surface area for mineral nutrient intake (Thorup-Kristensen et al., 2020). For much less mobile PO43-ion, a bigger root system provided a higher absorption surface (Sinha et al., 2018). The synchronized proliferation of roots at all growth phases might be the cause of wheat's higher root efficiency under M2. It seemed that a root system's growth facilitated improved P absorption, which supported higher biomass output. Despite M4 having a larger P concentration in the soil than M2, M2 had a stronger P uptake by wheat. P uptake was dependent on both the root system's ability to absorb nutrients and the above-ground growth that resulted in the demand for nutrients in the shoot, suggesting that P uptake was driven by increased shoot demand under M2. Our study demonstrated the functional relationships between root features and P absorption by finding significant positive associations between root volume (R2 = 0.786 and 0.739, respectively; P < 0.05) and length (R2 = 0.713 and 0.724, respectively; P < 0.05) with P concentration in shoot and grain.
5. Conclusions
According to the results of the first experiment, adding rice straw to subtropical soils at a rate of 12 and 14 Mg ha−1 with PSM could be a practical way to solubilize the inorganic resistant soil P and minimize the hysteresis impact of P. Nearly 3.1–3.5% of the soil's inorganic P may be solubilized by applying rice straw at a rate of 12 and 14 Mg ha−1. It suggests that applying rice straw at a rate of 12 and 14 Mg ha−1 would be advantageous for supplying the P requirements of crops in semi-arid subtropical Inceptisols.
The inference from the first experiment was confirmed by the second experiment. It showed that the highest wheat yield was obtained when rice straw was applied at a rate of 12 Mg ha−1 along with PSM and 75% mineral P fertilizer. Additionally, it made it easier for wheat to absorb P. Furthermore, by leaving more NaHCO3-P in the soil, it could improve the availability of P for the next crops. Nevertheless, using rice straw at a rate of 12 Mg ha−1 in conjunction with PSM allowed soil pH to be maintained in a range close to neutral and, more crucially, increased the activities of soil enzymes. Because better yield and P acquisition were found with the application of rice straw at 12 Mg ha−1 with PSM and 75% mineral P fertilizer, it could be assumed that producers may substantially minimize cultivation costs by saving 25% P fertilizer with this strategy. This might lessen India's total burden for P imports from other nations, when applied on a broader scale. Despite this information, further research is required to determine the unique mechanisms and processes occurring in the soil, the quantity of RS, and their consequences on the growth and production of crops.
Data availability statement
The original contributions presented in the study are included in the article/supplementary material, further inquiries can be directed to the corresponding authors.
Author contributions
AG, HOE, and DRB contributed to the conception and design of the study. AG and SSM organized the database. AG and RC performed the statistical analysis of data. AG and KA wrote the first draft of the manuscript. RB, SD, TKD, KL, and SS supervised the work. RC and HOE performed the interpretation of data and language editing. SSM performed data acquisition, revised it critically, and edited the revised manuscript. All authors contributed to the manuscript revision, read, approved the submitted version, approved the final version to be published, accountable for all aspects of the work in ensuring that questions related to the accuracy or integrity of any part of the work are appropriately investigated and resolved.
Funding
Researchers Supporting Project number (RSP2023R118), King Saud University.
Acknowledgments
This research was supported by Director, ICAR-IARI. We thank our scientists from ICAR-IARI, New Delhi who provided insight and expertise that greatly assisted the research. The authors would like to thank Researchers Supporting Project number (RSP2023R118), King Saud University, Riyadh, Saudi Arabia.
Conflict of interest
The authors declare that the research was conducted in the absence of any commercial or financial relationships that could be construed as a potential conflict of interest.
Publisher's note
All claims expressed in this article are solely those of the authors and do not necessarily represent those of their affiliated organizations, or those of the publisher, the editors and the reviewers. Any product that may be evaluated in this article, or claim that may be made by its manufacturer, is not guaranteed or endorsed by the publisher.
Abbreviations
RS, rice straw; PSMs, P solubilizing microorganisms; CRI, crown root initiation; FLO, flowering; MAT, maturity; DHA, dehydrogenase; ALP, alkaline phosphatase; PerOX, peroxidase; DAI, days after incubation.
References
Adesemoye, A. O., and Kloepper, J. W. (2009). Plant–microbes interactions in enhanced fertilizer-use efficiency. Appl. Microbiol. Biotechnol. 85, 1–12. doi: 10.1007/s00253-009-2196-0
Afif, E., Barron, V., and Torrent, J. (1995). Organic matter delays but does not prevent phosphate sorption by Cerrado soils from Brazil. Soil Sci. 159, 207–211.
Alam, K., Biswas, D. R., Bhattacharyya, R., Das, D., Suman, A., Das, T. K., et al. (2022). Recycling of silicon-rich agro-wastes by their combined application with phosphate solubilizing microbe to solubilize the native soil phosphorus in a sub-tropical Alfisol. J. Environ. Manag. 318, 115559. doi: 10.1016/j.jenvman.2022.115559
Alori, E. T., Glick, B. R., and Babalola, O. O. (2017). Microbial phosphorus solubilization and its potential for use in sustainable agriculture. Front. Microbiol. 8, 971. doi: 10.3389/fmicb.2017.00971
Bach, C. E., Warnock, D. D., Van Horn, D. J., Weintraub, M. N., Sinsabaugh, R. L., Allison, S. D., et al. (2013). Measuring phenol oxidase and peroxidase activities with pyrogallol, L-DOPA, and ABTS: effect of assay conditions and soil type. Soil Biol. Biochem. 67, 183–191. doi: 10.1016/j.soilbio.2013.08.022
Becerra-Castro, C., Lopes, A. R., Vaz-Moreira, I., Silva, E. F., Manaia, C. M., and Nunes, O. C. (2015). Wastewater reuse in irrigation: a microbiological perspective on implications in soil fertility and human and environmental health. Environ. Int. 75, 117–135. doi: 10.1016/j.envint.2014.11.001
Berthelsen, S., Noble, A. D., Kingston, G., Hurney, A., Rudd, A., and Garside, A. (2003). Improving yield and ccs in sugarcane through the application of silicon based amendments. Final Report, Sugar Research and Development Corporation Project CLW009.
Biswas, S. S., Biswas, D. R., Ghosh, A., Sarkar, A., Das, A., and Roy, T. (2022). Phosphate solubilizing bacteria inoculated low-grade rock phosphate can supplement P fertilizer to grow wheat in sub-tropical inceptisol. Rhizosphere 23, 100556.
Casida, L. E., Klein, D. A., and Santoro, T. (1964). Soil dehydrogenase activity. Soil Sci. 98, 371–376.
Deng, J., Jiang, X., Hu, W., and Hu, L. (2010). Quantifying hysteresis of atrazine desorption from a sandy loam soil. J. Environ. Sci. 22, 1923–1929. doi: 10.1016/S1001-0742(09)60340-5
Deng, S. P., and Tabatabai, M. A. (1994). Cellulase activity of soils. Soil Biol. Biochem. 26, pp.1347–1354.
Emami, S., Alikhani, H. A., Pourbabaei, A. A., Etesami, H., Sarmadian, F., and Motessharezadeh, B. (2019). Effect of rhizospheric and endophytic bacteria with multiple plant growth promoting traits on wheat growth. Environ. Sci. Pollut. Res. 26, 19804–19813. doi: 10.1007/s11356-019-05284-x
Etesami, H., and Jeong, B. R. (2021). Contribution of arbuscular mycorrhizal fungi, phosphate–solubilizing bacteria, and silicon to P uptake by plant: a review. Front. Plant Sci. 12, 1355. doi: 10.3389/fpls.2021.699618
Ghosh, A., Biswas, D. R., Das, S., Das, T. K., Bhattacharyya, R., Alam, K., et al. (2023). Rice straw incorporation mobilizes inorganic soil phosphorus by reorienting hysteresis effect under varying hydrothermal regimes in a humid tropical Inceptisol. Soil Tillage Res. 225, 105531. doi: 10.1016/j.still.2022.105531
Ghosh, A., Kumar, S., Manna, M. C., Singh, A. K., Sharma, P., Sarkar, A., et al. (2019). Long-term in situ moisture conservation in horti-pasture system improves biological health of degraded land. J. Environ. Manag. 248, 109339. doi: 10.1016/j.jenvman.2019.109339
Ghosh, A., Singh, A. B., Kumar, R. V., Manna, M. C., Bhattacharyya, R., Rahman, M. M., et al. (2020). Soil enzymes and microbial elemental stoichiometry as bio-indicators of soil quality in diverse cropping systems and nutrient management practices of Indian Vertisols. Appl. Soil Ecol. 145, 103304. doi: 10.1016/j.apsoil.2019.06.007
Grybos, M., Davranche, M., Gruau, G., Petitjean, P., and Pédrot, M. (2009). Increasing pH drives organic matter solubilization from wetland soils under reducing conditions. Geoderma 154, 13–19. doi: 10.1016/j.geoderma.2009.09.001
Hu, A. Y., Che, J., Shao, J. F., Yokosho, K., Zhao, X. Q., Shen, R. F., et al. (2018). Silicon accumulated in the shoots results in down-regulation of phosphorus transporter gene expression and decrease of phosphorus uptake in rice. Plant Soil 423, 317–325. doi: 10.1007/s11104-017-3512-6
Kilmer, V. J. (1965). “Silicon,” in Methods of Soil Analysis, Part 1. Chemical Methods, ed C. A. Black (Madison, WI: American Society of Agronomy), 959–962.
Kostic, L., Nikolic, N., Bosnic, D., Samardzic, J., and Nikolic, M. (2017). Silicon increases phosphorus (P) uptake by wheat under low P acid soil conditions. Plant Soil 419, 447–455. doi: 10.1007/s11104-017-3364-0
Kruse, J., Abraham, M., Amelung, W., Baum, C., Bol, R., Kühn, O., et al. (2015). Innovative methods in soil phosphorus research: a review. J. Plant Nutr. Soil Sci. 178, 43–88. doi: 10.1002/jpln.201400327
Kuo, S. (1996). “Phosphorus,” in Methods of Soil Analysis: Part 3-Chemical Methods, ed D. L. Sparks (Madison, WI: SSSA), 869–919.
Li, Z., Unzué-Belmonte, D., Cornelis, J. T., Vander Linden, C., Struyf, E., Ronsse, F., et al. (2019). Effects of phytolithic rice-straw biochar, soil buffering capacity and pH on silicon bioavailability. Plant Soil 438, 187–203. doi: 10.1007/s11104-019-04013-0
Manna, M. C., Rahman, M. M., Naidu, R., Bari, A. F., Singh, A. B., Thakur, J. K., et al. (2021). Organic farming: a prospect for food, environment and livelihood security in Indian agriculture. Adv. Agron. 170, 101–153. doi: 10.1016/bs.agron.2021.06.003
Meena, A., and Rao, K. S. (2021). Assessment of soil microbial and enzyme activity in the rhizosphere zone under different land use/cover of a semiarid region, India. Ecol. Process. 10, 1–12. doi: 10.1186/s13717-021-00288-3
Menezes-Blackburn, D., Giles, C., Darch, T., George, T. S., Blackwell, M., Stutter, M., et al. (2018). Opportunities for mobilizing recalcitrant phosphorus from agricultural soils: a review. Plant Soil 427, 5–16. doi: 10.1007/s11104-017-3362-2
Modak, K., Ghosh, A., Bhattacharyya, R., Biswas, D. R., Das, T. K., Das, S., et al. (2019). Response of oxidative stability of aggregate-associated soil organic carbon and deep soil carbon sequestration to zero-tillage in subtropical India. Soil Tillage Res. 195, 104370. doi: 10.1016/j.still.2019.104370
NPMCR (2014). National Policy for Management of Crop Residues. New Delhi: Government of India, Ministry of Agriculture, Department of Agriculture and Cooperation (Natural Resource Management Division), Krishi Bhawan. Available online at: http://agricoop.nic.in/sites/default/files/NPMCR_1.pdf (accessed September 27, 2022).
Nwoke, O. C., Vanlauwe, B., Diels, J., Sanginga, N., and Osonubi, O. (2004). Impact of residue characteristics on phosphorus availability in West African moist savanna soils. Biol. Fertil. Soils 39, 422–428. doi: 10.1007/s00374-004-0731-9
Olsen, S. R. (1954). Estimation of Available Phosphorus in Soils by Extraction with Sodium Bicarbonate (No. 939). New York, NY: US Department of Agriculture.
Reynolds, L. L., Lajtha, K., Bowden, R. D., Johnson, B. R., and Bridgham, S. D. (2017). The carbon quality-temperature hypothesis does not consistently predict temperature sensitivity of soil organic matter mineralization in soils from two manipulative ecosystem experiments. Biogeochemistry 136, 249–260. doi: 10.1007/s10533-017-0384-z
Shyamsundar, P., Springer, N. P., Tallis, H., Polasky, S., Jat, M. L., Sidhu, H. S., et al. (2019). Fields on fire: alternatives to crop residue burning in India. Science 365, 536–538. doi: 10.1126/science.aaw4085
Sinha, S. K., Rani, M., Kumar, A., Kumar, S., Venkatesh, K., and Mandal, P. K. (2018). Natural variation in root system architecture in diverse wheat genotypes grown under different nitrate conditions and root growth media. Theor. Exp. Plant Physiol. 30, 223–234. doi: 10.1007/s40626-018-0117-2
Tabatabai, M. A., and Bremner, J. M. (1969). Use of p-nitrophenyl phosphate for assay of soil phosphatase activity. Soil Biol. Biochem. 1, 301–307.
Thorup-Kristensen, K., Halberg, N., Nicolaisen, M., Olesen, J. E., Crews, T. E., Hinsinger, P., et al. (2020). Digging deeper for agricultural resources, the value of deep rooting. Trends Plant Sci. 25, 406–417. doi: 10.1016/j.tplants.2019.12.007
Keywords: rice straw management, phosphorus transformations, silicate concentration, wheat yield, root architecture
Citation: Ghosh A, Biswas DR, Bhattacharyya R, Das S, Das TK, Lal K, Saha S, Alam K, Casini R, Elansary HO and Manjangouda SS (2023) Rice residue recirculation enhances mobilization and plant acquisition of soil inorganic phosphorus by increasing silicon availability in a semi-arid Inceptisol. Front. Sustain. Food Syst. 7:1059450. doi: 10.3389/fsufs.2023.1059450
Received: 01 October 2022; Accepted: 01 March 2023;
Published: 23 March 2023.
Edited by:
Engracia Madejon, Institute of Natural Resources and Agrobiology of Seville (CSIC), SpainReviewed by:
Rajendra Kumar Yadav, Agriculture University (Kota), IndiaPrakash Kumar Jha, Kansas State University, United States
Abhay Shirale, National Bureau of Soil Survey and Land Use Planning (ICAR), India
Copyright © 2023 Ghosh, Biswas, Bhattacharyya, Das, Das, Lal, Saha, Alam, Casini, Elansary and Manjangouda. This is an open-access article distributed under the terms of the Creative Commons Attribution License (CC BY). The use, distribution or reproduction in other forums is permitted, provided the original author(s) and the copyright owner(s) are credited and that the original publication in this journal is cited, in accordance with accepted academic practice. No use, distribution or reproduction is permitted which does not comply with these terms.
*Correspondence: Avijit Ghosh, YXZpaml0Z2hvc2gxOTg5MkBnbWFpbC5jb20=; Dipak Ranjan Biswas, ZHJiX3NzYWNAaWFyaS5yZXMuaW4=