- 1Propagate, Arvada, CO, United States
- 2The Nature Conservancy, Minneapolis, MN, United States
- 3The Nature Conservancy, Arlington, VA, United States
Silvopasture—integrating trees, forage, and grazing livestock on the same piece of land—is increasingly popular, given its potential to store carbon (C) and improve farmers’ livelihoods. We examined the C and economic implications of adding different silvopastoral systems to existing pastures in historically forested areas of the eastern United States (U.S.). We assessed nine distinct systems, varying by species and product (timber, nuts, and fodder for livestock), for two market scenarios: one based on current demand and one that assumes increased demand for products from silvopasture systems. For each system, we assessed C storage (biomass) and economics (internal rates of return (IRR) with and without C payments). We find that silvopasture in the eastern U.S. could expand by 5.6–25.3 million hectares under base case and full adoption scenarios (equaling a 6% increase in the global footprint of silvopasture), and could capture up to 4.9 or 25.6 Tg CO2e yr.−1, respectively. Expansion of silvopasture in these scenarios would come largely from demand for fodder as a supplemental feed, as well as specialty timber products. Per ha mitigation potential varied widely (0.5–6.5 tCO2e ha−1 yr.−1), due to species differences in C accumulation rates. Economics differed too, with some systems offering short break-even timelines (e.g., 7–9 years for fodder systems), and others costing more up front but having greater long-term returns (e.g., Chestnut). Furthermore, while some systems are profitable without any price on C (e.g., fodder-based silvopasture offers 6–14% 10-year IRRs without a price on C), higher payments for C would likely be necessary to unleash broad investment in timber and nut-based silvopasture. Our analysis included planting, maintenance, and harvest costs and tree product revenue. Future work is needed to fully incorporate additional considerations, like loss of grazing use during establishment, shade-induced effects on forage production, and livestock productivity. Furthermore, specific economic, ecological, site- and operation-level considerations are critical to evaluate the appropriateness of silvopasture systems for a given setting. This analysis suggests that across the eastern U.S., silvopasture could offer both climate change mitigation and enhanced profitability for farmers, with notable differences in the system-specific magnitude of opportunity.
1. Introduction
Regenerative agriculture is increasingly promoted as a necessary component of climate change mitigation (Griscom et al., 2017; Fargione et al., 2018; Drever et al., 2021; Roe et al., 2021). Greater use of trees in agriculture (i.e., “agroforestry”), such as through windbreaks, alley cropping, or silvopasture (Udawatta and Jose, 2012; Fargione et al., 2018), is a promising way to increase the amount of carbon (C) on the landscape, as well as generate other ecosystem services such as shade for livestock (Garrett et al., 2004), reduced cold and wind stress (Brandle et al., 2004), erosion control (Torralba et al., 2016), as well as enhanced soil fertility (Torralba et al., 2016), biodiversity (Jose, 2012; Torralba et al., 2016), water quality (Salceda et al., 2023), and wildlife habitat (Jose, 2012). Agroforestry can also provide additional income for farmers through timber and non-timber wood products and services, as well as C revenue.
Mitigation potential generally depends on the area of opportunity (i.e., the number of hectares where adoption is possible) and the amount of C accumulated per acre. However, the area of opportunity for agroforestry in the United States (U.S.) has only been coarsely estimated based on rough estimates of the percentage of agricultural lands that could be suitable for agroforestry, rather than detailed spatial analyses (Udawatta and Jose, 2012; Chapman et al., 2020). Furthermore, different management practices, such as species choice, will impact the C sequestration (Dold et al., 2019), but those C outcomes are often poorly quantified and/or have high uncertainty (Cardinael et al., 2018). Moreover, the potential market size and economic returns of different agroforestry products and services is necessary to evaluate how much mitigation potential could feasibly be realized, but detailed analyses of costs and benefits for specific species are infrequent in the literature (although see Brown et al., 2018 for details by agroforestry system).
Here we focus on silvopasture, the deliberate integration of trees, forage, and grazing livestock on the same piece of land (Nair et al., 2021), and its associated C and economic outcomes. Silvopasture can either be implemented by incorporating livestock grazing into wooded areas or by adding trees to existing pasture lands. Incorporating livestock into wooded areas is a common form of silvopasture, but often involves some conversion and loss of tree cover (Orefice et al., 2017), and therefore carbon loss. As a result, we focus here specifically on silvopasture that adds trees to existing pasture, as it is likely to offer more climate mitigation benefit while also providing additional revenue for farmers already grazing livestock (Smith et al., 2022a). Silvopasture can be quite adaptable as well, employing different species for different products, including timber, nuts, and fodder for livestock.
To assess the potential for silvopasture in the eastern U.S., we first identified current pastureland that was historically forested. We then applied literature-based allometric equations to estimate C sequestration rates for nine different silvopastoral systems. We calculated the costs and revenues for each system, and how economic outcomes would change if landowners were paid for C accumulation. Finally, we considered two adoption scenarios: a base case scenario and a full adoption scenario that modeled what is possible with current demand versus expanded market demand, respectively, for different timber, nut, and fodder products from silvopastoral systems.
2. Materials and methods
2.1. Silvopastoral system types
We examined nine silvopastoral systems for the eastern U.S., with different tree species and yield timelines (Table 1 and Supplementary Appendix S1). These included Robinia pseudoacacia (Black Locust), Populus x canescens and Populus x canadensis (Hybrid Poplar), Carya illinoinensis (Pecan), Castanea mollissima (Chinese Chestnut; hereafter Chestnut), Juglans nigra (Black Walnut), Pinus palustris (Longleaf Pine), Salix spp. (Willow), and Morus rubra. (Red Mulberry; hereafter Mulberry). Products included timber (Black Locust, Hybrid Poplar, Black Walnut, Longleaf Pine), nuts (Pecan, Chestnut), and fodder for livestock (Mulberry and Willow). These include native and non-native tree species and were chosen based on potential for use in agricultural systems and ability to meet existing market demands. Given that Black Locust, while native, is considered invasive in some places (see Supplementary Table S1), extra care would need to be taken to assess whether it is appropriate to plant in a specific location.
This is not intended to be an exhaustive list of potential silvopastural systems, but rather is meant to capture variation across systems. For the fodder systems, we assume the fodder to be grazed on site, as opposed to cut-and-carry systems where forage is removed to be used elsewhere. Furthermore, in this analysis we focus on the climate and economics implications of the tree component of these systems. Additional work is needed to characterize impacts on livestock. The use of each silvopastoral system in any specific farm would require individual management and place-based planting considerations, including impacts on biodiversity and ecological functions. While each system is suited for specific hardiness zones, collectively these silvopasture systems are well suited for most of the continental U.S. east of the 98th meridian, and span hardiness zones 3–11.
2.2. Available hectares
We focused this analysis on exploring the potential for new silvopasture through adding trees to existing pasture. We estimated available hectares by hardiness zone (using 10° zones) and current land use, based on Cook-Patton et al. (2020) estimate of reforestable pasture, grassland, and marginal cropland (Table 2). We limit our analysis to areas east of the 98th meridian since many grazing lands in the West occur on natural grasslands that are not ecologically suitable for tree planting. Locations in the western portion of our study area include places where the natural land cover would be savannah, which is consistent with the silvopasture planting densities investigated here. States fully included were Alabama, Arkansas, Connecticut, Delaware, Florida, Georgia, Illinois, Indiana, Iowa, Kentucky, Louisiana, Maine, Maryland, Massachusetts, Michigan, Minnesota, Mississippi, Missouri, New Hampshire, New Jersey, New York, North Carolina, Ohio, Pennsylvania, Rhode Island, Tennessee, South Carolina, Vermont, Virginia, West Virginia, and Wisconsin. States with partial land area included were North Dakota, South Dakota, Nebraska, Kansas, Oklahoma, and Texas. Given that some species included may not be appropriate to plant in certain locations (e.g., Black Locust is native to the regions included, but is considered weedy or invasive in some states), we cross-referenced state-based invasive species lists (mostly from state-based Department of Natural Resources and state invasive species council sites; Supplementary Table S1) to identify which states should have available acres excluded from the applied planting area for each species. In addition, to inform productivity estimates (both for crop yields and carbon storage), we evaluated the land capability classes of these available hectares.
2.3. Adoption scenarios
To date, silvopasture adoption remains low in the U.S. (i.e., less than 1.5% of U.S. farms employ an agroforestry practice, of which silvopasture is just one example; Smith et al., 2022b), despite being viewed by practitioners as an important way to provide shade for livestock (Orefice et al., 2017) and diversify income (Smith et al., 2022a). We therefore developed adoption scenarios based on potential demand for timber, nut, and fodder products as informed by practitioner experience and input (Figure 1).
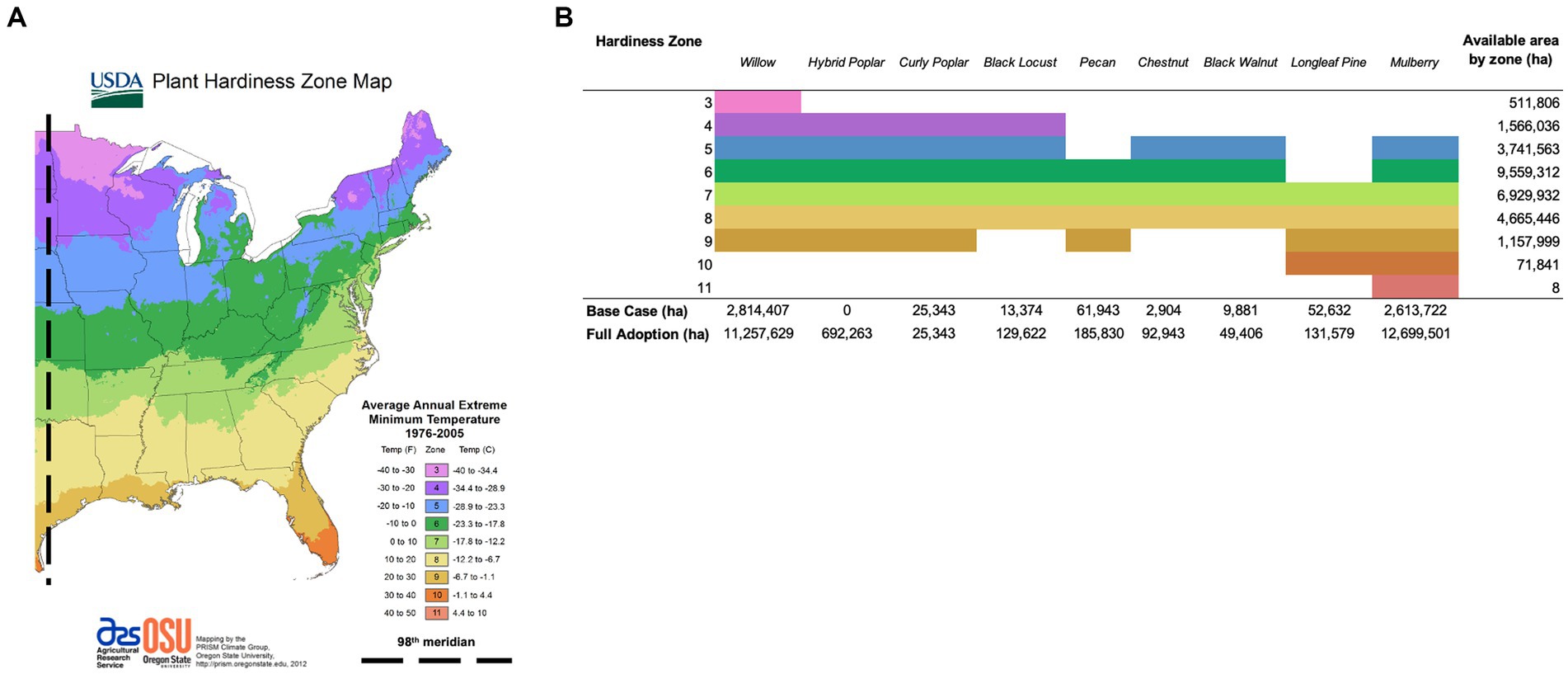
Figure 1. Potential distribution of silvopastoral systems by species. The study area for this analysis is shown with hardiness zones (A) (image adapted from the USDA Plant Hardiness Zone Map, 2012; https://planthardiness.ars.usda.gov/). Species investigated here are shown in (B) with their corresponding planting ranges by hardiness zones, as well as land area potential by adoption scenario (Base Case and Full Adoption) and total available land area for new silvopasture by hardiness zone (see Available hectares for methods details).
We explored two adoption scenarios—a “Base Case” and a “Full Adoption” scenario. For each scenario, we estimated potential market caps independently for each species and their products, based on current domestic consumption, along with import and export data for hardwood and softwood lumber, nuts, and beef (see Supplementary Appendix S1). For the Base Case, we estimated potential market caps based on what could be a serviceable obtainable market (i.e., the customer base that a product could easily reach without costs of customer acquisition exceeding profits from sale to those customers), or what the private sector would likely implement given readily available information, moderate producer interest, and socialization of silvopasture practices with producers. In the Full Adoption scenario, we estimated the potential market cap for each species based on what might occur given assumptions on the increased market share each species could achieve with producers’ complete familiarity with silvopasture and keen interest on the part of producers and their supply chains, lenders/investors, agencies, and legislators (see Supplementary information for species-level assumptions). For both scenarios, we estimated fodder system use based on a percentage of available area, ramping up from the Base Case to Full Adoption scenarios to reflect potential growth of browsed fodder as a practice for on-site supplemental feed in the U.S. (as outlined in Supplementary Appendix S1).
Once we identified potential market caps by species for each non-fodder scenario, we calculated the hectares necessary to satisfy those market caps assuming dense, but feasible planting densities (Table 1). Rotation ages were selected based on tree growth rate, median annual increment, and end-product characteristics. For both scenarios, fodder crop production area was based on a percentage of available hectares by hardiness zone (see Supplementary Appendix S1 for more details). We then assumed adoption on available hectares equivalent to the estimated market cap by each species. We checked to ensure that there were enough hectares available for each species in suitable planting areas (e.g., across their hardiness zone range), while removing hectares from states where species may be flagged as inappropriate for planting based on its potential to spread (Figure 2 and Supplementary Table S1). This resulted in silvopasture adoption on a total 20% of the available hectares in the Base Case scenario and 90% of available hectares in the Full Adoption scenario. This approach was designed to estimate the broad potential for each species across its hardiness zone range, and is not a prescription for where individual species should be planted.
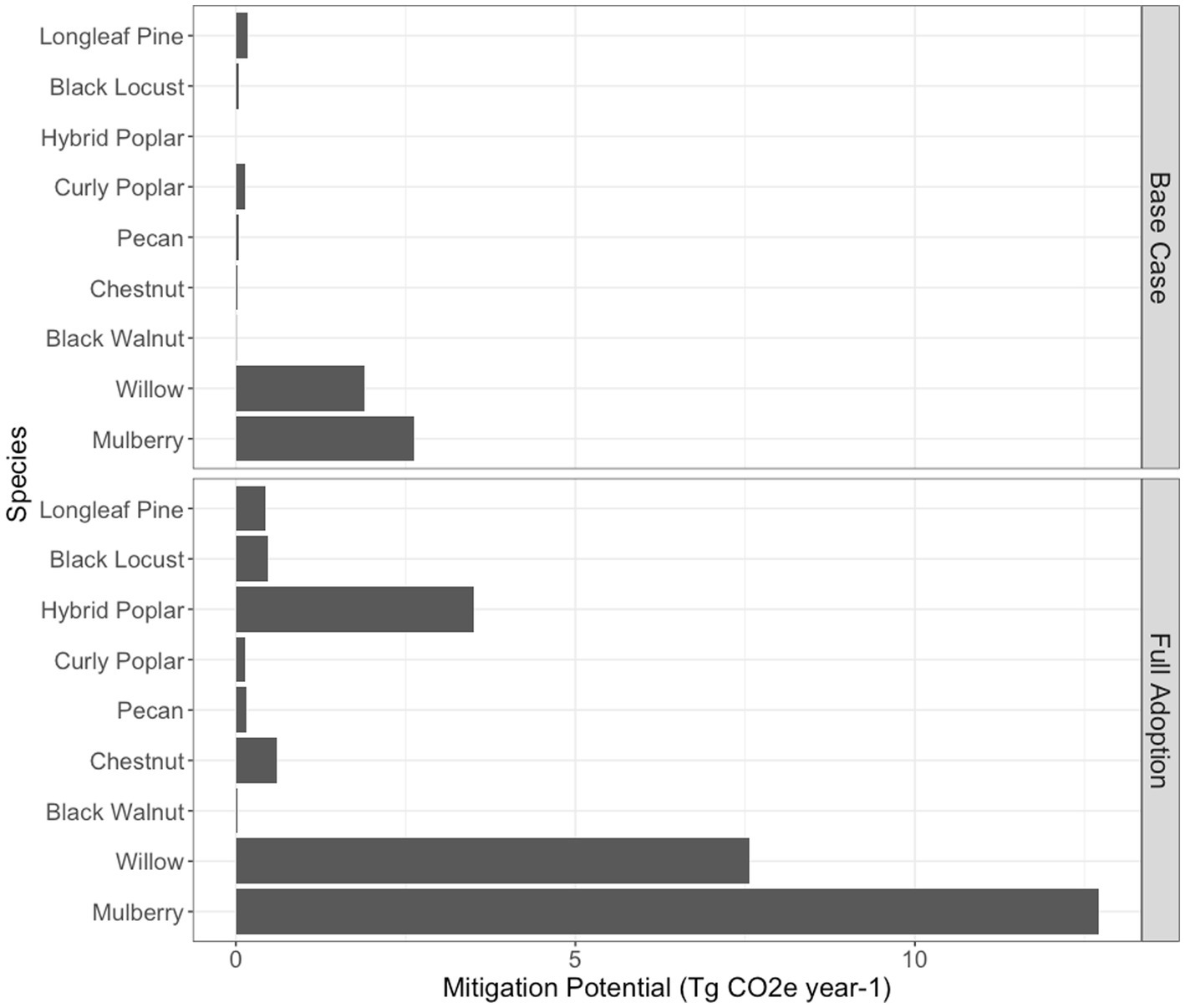
Figure 2. Mitigation potential by species and market scenarios. Total annual sequestration potential (Tg CO2e yr.−1) by system based on both C accrual rates and market demand assumptions for the Base Case and Full Adoption market scenarios.
2.4. Carbon estimates
We calculated C storage potential for each silvopastoral system using species-specific C accrual rates per tree and system-specific planting densities (Tables 1, 3, respectively). Both C accrual rates and planting densities were assumed to be constant across hardiness zones.
We used a generalized allometric equation to estimate average mature aboveground biomass per tree (Eq. 1; Reid, 2017), using species-specific parameter values collected from the literature (Table 3). The average aboveground biomass (AGB) of a particular species depends on its diameter at breast height (DBH), total height (h), and wood density (ρ). For the two fodder species (Mulberry and Willow), AGB was estimate solely for the primary trunk, excluding stems that would be consumed as fodder. We used AGB values directly from the literature (Willow: Pietrzykowski et al., 2021; Mulberry: Varsha et al., 2019), as opposed to using allometric equations. We assumed that the fodder trees would be sufficiently large to survive and grow after browsing after one to two growing seasons (see Supplementary Table S2). For each species, we estimated belowground biomass (BGB) as a percentage of AGB, using species-specific data that ranged from 25 to 35% for timber and nut species and 125–310% for fodder species (Table 3):
(1)
To convert from biomass to tons of C per tree, for all species we assumed that 50% of all dry-weight biomass was C (Ma et al., 2020). Annual C accrual rates were determined by assuming linear growth for 30 years to reach adult tree size, accounting for multiple harvests when applicable (Table 3). We used assumptions of dense, but feasible silvopasture planting densities specific to each species (Table 1) to estimate annual tons of C per hectare, which was then applied to planted hectares using the two scenarios (i.e., the Base Case and Full Adoption scenarios; see Supplementary Appendix S1 for more detailed assumptions for these adoption scenarios).
2.5. Economic evaluation
Silvopastoral systems are much more likely to be adopted if they generate sufficient economic returns. To quantify returns, we forecasted system-wide costs and revenues for each silvopastoral system type to calculate the rate of return over various time horizons, with and without C payments. In addition to publicly available literature on agroforestry and silvopasture (Supplementary Table S2), we consulted 19 practitioners to inform the market scenarios and economic evaluation. These practitioners had expertise in: cattle nutrition, management intensive grazing, multi-species grazing, silvopasture design, silvopasture establishment and management, Black Locust supply and economics, Black Walnut economics, Longleaf Pine economics, Chestnut agroforestry, Pecan management, Poplar establishment and economics, Mulberry as a feed source, and Willow biomass in ruminant nutrition. In generating our estimates, published research was prioritized, followed by information from government agricultural agencies, university extension, and private industry. This approach enabled us to supplement the limited data available in published research on system costs, revenues, and returns and supplement it with information based on practitioner experience.
2.5.1. Costs
We cataloged operational expenditures (OPEX; variable costs) and capital expenditures (CAPEX; fixed costs) for each silvopastoral system based on a combination of available literature and input from working farmers managing these tree systems (Supplementary Table S2). OPEX was cataloged for years 1–30, assuming site prep in year 1 and planting in year 2. Expenditures fell into three categories: management (labor, material inputs, and equipment use), harvest (labor, infrastructure use), and marketing (hours and costs necessary to achieve a certain sale price). For some agroforestry systems, this does not capture the full costs of transitioning, such as lost grazing access in pastures that require fencing to protect seedlings from cattle during establishment years (Lehmkuhler et al., 2003). Our economic analysis also does not attempt to capture any of the negative effects of shade on forage or positive effects of shade on animal performance. Forage levels did not decline under 30% shade, but did under 50 and 70% shade (Mercier et al., 2020). Shade can increase performance in beef cattle (Edwards-Callaway et al., 2021) and dairy cattle (Polsky and von Keyserlingk, 2017). Additional research is required to estimate the net economic effects of these potentially offsetting effects of shade in agroforestry systems. We calculated OPEX per tree and per acre, separate from per-farm CAPEX. Because CAPEX depends on farm size, we used a model farm size of 40.5 hectares under silvopastoral production for all of our economic modeling. Using system-specific C sequestration rates (see “Carbon estimates” above), we then calculated the capital required per ton of C for each system. We also calculated the amount of time required to “break even.” Break even is the point when accumulated revenue exceeds accumulated costs, i.e., when the business has made more money than it has spent.
2.5.2. Revenues
We forecasted yield based on existing operations and available technology and germplasm. Forecasts were informed by the literature, author experience, and consultation with agroforestry and silvopasture practitioners with first-hand knowledge of implementing these systems in the U.S., Colombia, Costa Rica, Argentina, France, and Hungary. Our revenue estimates are based on the installation of a silvopasture system in an existing, treeless pasture, with best available technology and germplasm options. Yield forecasts included assumptions of standard crop losses due to crop-specific weather responses (e.g., late frosts or drought), pests, and operational risks (e.g., management errors; see Supplementary Table S2). We estimated revenue by system type using estimated yields and current market prices. Forecasted sales and pricing were weighted to wholesale markets where available for a given species, and to retail or value-added markets where wholesale markets were unavailable, uncommon, or infeasible. Fodder crop yields (Mulberry and Willow) and their associated values were derived from the offset cost of buying hay for livestock, at a price of $65 per 1,000-lb delivered round bale. Round bales were assumed to be 50% dry matter (Henning and Wheaton, 1993).
2.5.3. Income estimation and return metrics
Costs and revenues were used to calculate return metrics (Supplementary Table S3). We calculated annual and cumulative Earnings Before Interest and Taxes (EBIT), which is one way to understand what is commonly thought of as “profit” in managerial accounting. We calculated Internal Rate of Return for 10-year, 20-year, and 30-year timeframes. We also calculated Net Present Value (NPV) at 30 years and the number of years to break even. The Internal Rate of Return (IRR) is a measure of the interest rate that would render the system unprofitable, whereas NPV indicates the value of future income (difference between revenues and expenditures over a given time period) in today’s dollars. IRR and NPV were calculated in Excel using default equations. Discount rate was standardized at 5% across all crop types. Overyield software (proprietary software from Propagate, Boulder, Colorado) was used to calculate cost, revenue, yield, and returns.
We also explored the impact of C payments on return metrics. We applied a conservative C price of $10/ton (World Bank, Ecofys, and Vivid Economics, 2017) to make comparisons of IRRs and years to break even with and without a price on C. Payments were applied annually based on linear accumulation of C through year 30. We also estimated the C price necessary to unlock a 10-year 7% IRR, a common cutoff that firms use to make investment decisions1. Finally, we estimated each silvopastoral system’s profit with and without C payments.
3. Results
Silvopasture in the eastern U.S. could expand by 5.6 million hectares today, under the Base Case scenario, and up to 25.2 million hectares under highly favorable market conditions in the Full Adoption scenario (Table 4). The spatial analysis identified up to 28.2 million hectares of pasture, grassland, and marginal cropland that would be suitable for silvopasture in the eastern U.S. (Table 2). Thus, these silvopasture adoption estimates are constrained by assumed market demand, rather than land availability. The expansion potential of different systems (timber, nut, and fodder) and individual species differ due to unique market constraints and opportunities. For example, fodder systems make up 97 and 95% of the total new silvopasture hectares in the Base Case and Full Adoption market scenarios, respectively because the demand for fodder was assumed to exceed the demand for nut and timber products.
Increasing the adoption of silvopastoral systems in the eastern U.S. would result in meaningful climate change mitigation. Successful implementation of silvopasture on the scale identified through the Base Case scenario would sequester 4.9 Tg CO2e yr.−1 (Table 4). In the Full Adoption scenario, 25.6 Tg CO2e yr.−1 could be sequestered (Table 4). Mitigation potential ranges dramatically across the silvopastoral systems: ranging from 0.0–2.6 Tg CO2e yr.−1 in the Base Case scenario (for Hybrid Poplar and Mulberry, respectively) and 0.03–12.7 Tg CO2e yr.−1 (for Black Walnut and Mulberry, respectfully) in the Full Adoption scenario (Figure 2). In the Base Case scenario, 91% of the total mitigation potential comes from fodder systems alone (38% from Willow and 53% from Mulberry), despite their low rates of per tree C accrual, due to the high number of hectares the market was estimated to support (Figure 3).
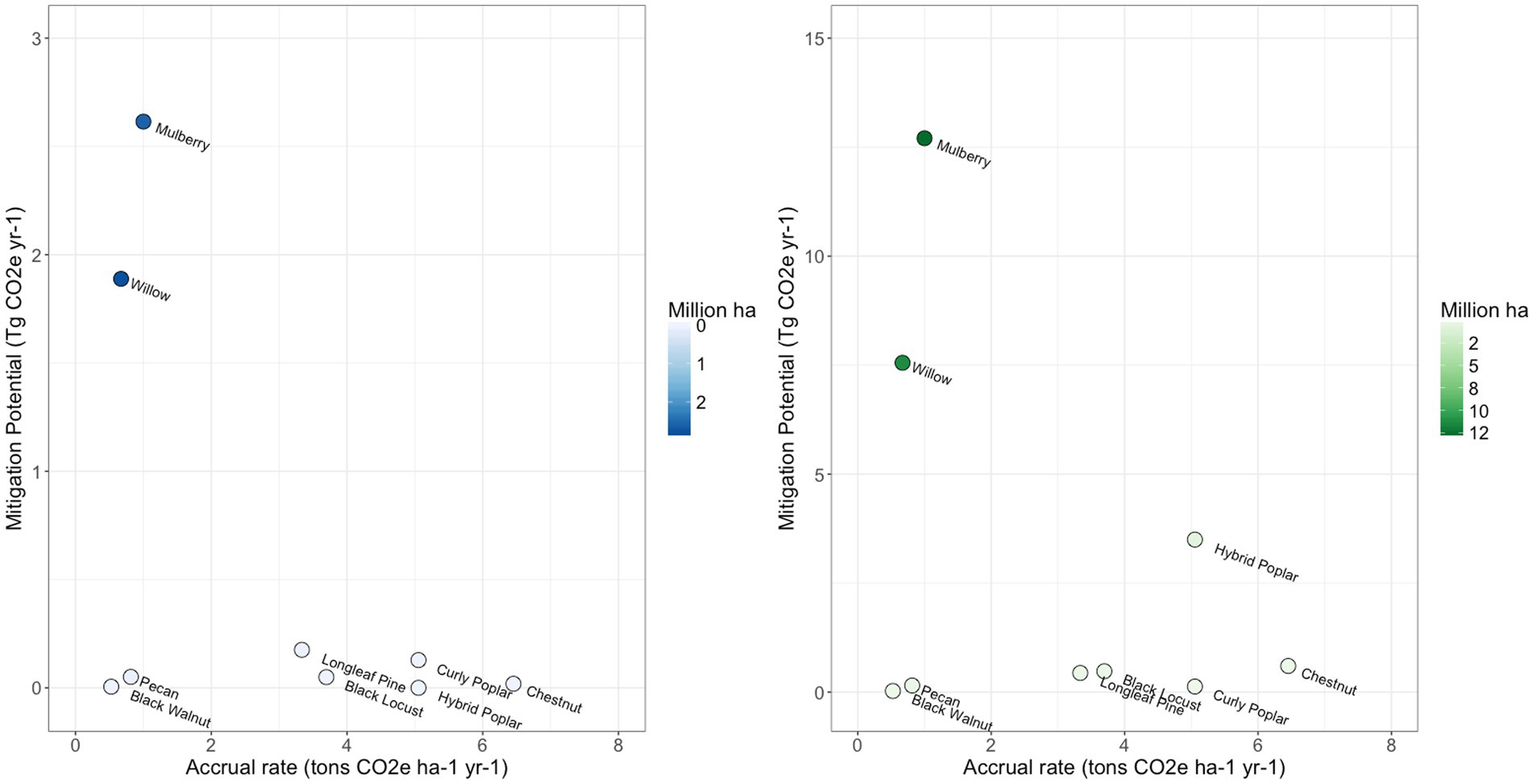
Figure 3. Relationship between total mitigation potential, accrual rates, and total planting area. Total mitigation potential (Tg CO2e yr.−1) is shown against per hectare accrual rates (tons CO2e ac−1 yr.−1). The points represent each of the nine silvopastoral systems, shown here for the Base Case scenario (left panel) and the Full Adoption scenario (right panel). Points with darker blue or green are planted in more hectares (e.g., Willow and Mulberry) than lighter green or blue points (e.g., Black Locust) based on estimated demand for the scenario. Extent of adoption of different systems (i.e., number of hectares) were modeled based on estimated market demand for products (see Supplementary Appendix S1 for more information).
A price on C would accelerate adoption of silvopasture practices, although profitability could be achieved in the near-term for some systems without C payments (Figure 4). The fodder systems (Willow and Mulberry) can break even in under 10 years and Mulberry could achieve a 7% IRR, a common investment threshold, without a price on C (Table 5). These fodder systems show a 6–14% 10-year IRR without a price on C and a 6.4–15% 10-year IRR with a price of $10/ton CO2e (Figure 4). Silvopastoral systems using slow-growing hardwood species take longer to break even (11–30 years for Chestnut, Pecan, Longleaf Pine, Black Locust, and Curly Poplar, with Black Walnut requiring twice as long- 60 years). These slower growing species also see modest increases in IRRs with a price of $10/ton CO2e (Table 5 and Figure 4). These systems would require a price between $86/ton CO2e to $505/ton CO2e to achieve a 7% IRR within 10 years (Figure 5). Despite taking longer to break even than fodder crops, some timber species have lower upfront capital requirements per ton of CO2e sequestered. For example, Black Locust requires just $31 capital/ton CO2e whereas Mulberry and Willow require $105 and $312 capital/ton CO2e (Table 5).
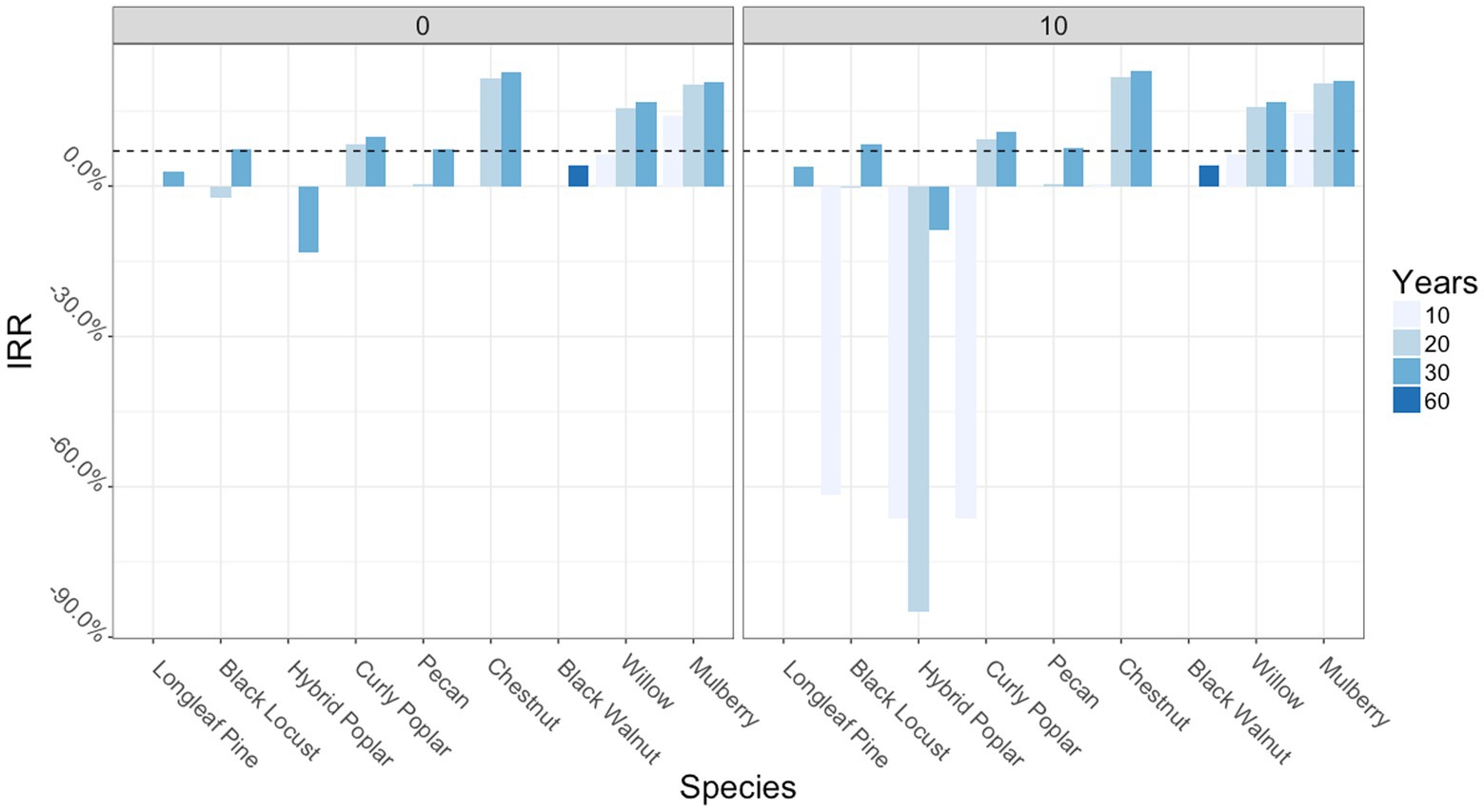
Figure 4. Internal Rates of Return (IRRs) by species, timelines, and with and without a modest price on C. For each species, IRRs are shown for 10-, 20-, and 30-year intervals assuming no price on C (0) and a modest $10/ton C (10). Some species (i.e., Black Locust, Hybrid Poplar, and Black Walnut) do not have IRRs for shorter time frames because harvests had yet to occur. The Black Walnut IRR is a 60-year IRR due to longer time to harvest.
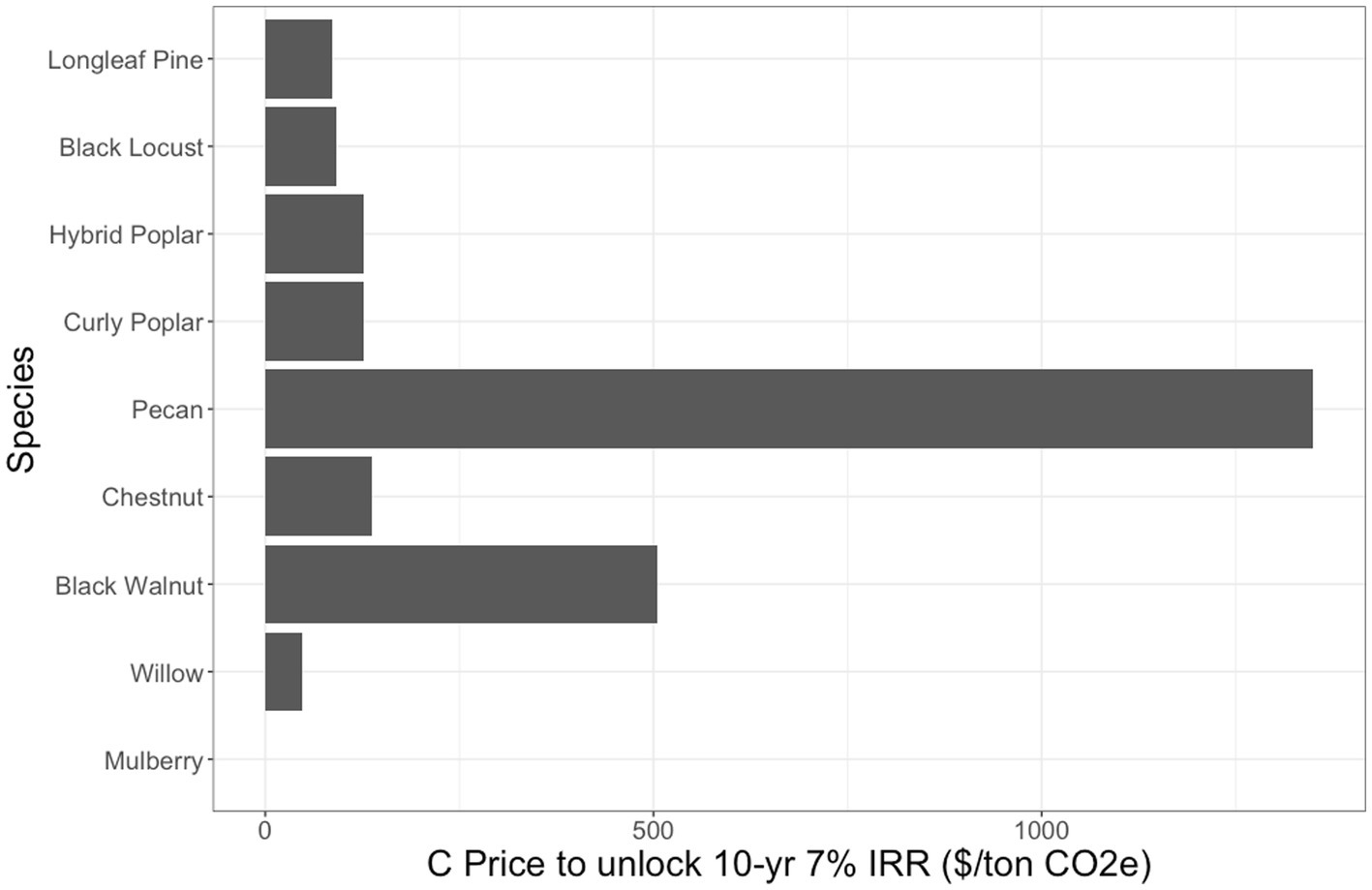
Figure 5. Price on C required to unlock a 10-year 7% IRR by species. Mulberry does not have a bar because a price on C is not necessary to unlock a 10-year 7% IRR.
4. Discussion
Here we identify the potential for 4.9 Tg CO2e yr.−1 to be sequestered from new silvopasture in the eastern U.S. under current market conditions, and up to 25.6 Tg CO2e yr.−1 if market conditions improved, which is less than what others have suggested (1,702.9 Tg CO2e yr.−1: Udawatta and Jose, 2012). This difference in total mitigation potential is likely due to both differences in the spatial extent of opportunity estimated here, as well as the rate of C accrual by system and demand for those products.
4.1. Spatial extent of opportunity
We estimate 5.6–25.3 million hectares for potential expansion of silvopasture in the eastern U.S. (under the Base Case scenario and Full Adoption scenario, respectively), the larger of which would expand the global footprint of silvopasture by about 6% (current global footprint is estimated at 450 Mha; Nair, 2012). This estimate of potential for expansion of silvopasture is on par with other recent estimates. For example, Udawatta and Jose (2012) estimate the potential for 25 Mha of new silvopasture in the U.S. from adding trees to existing pastureland (however, they estimate an additional 51 Mha from new grazing in existing forests). Our scenarios were designed to illustrate a range of estimated market opportunity. Additional economic analysis of domestic and export demand for these silvopasture products (timber, nuts, and meat) would refine these projections.
For the study area explored here, this level of increased adoption resulted in 20% of the available hectares in the Base Case scenario and 90% of available hectares in the Full Adoption scenario. Following theory of innovation adoption and assumptions about average farm size, this suggests that producers across a spectrum of innovation profiles (e.g., innovators, early adopters, early majority, etc.; Rogers, 1995) would need to adopt silvopasture practices to meet the potential outlined here, particularly in the Full Adoption scenario. Although access to information on silvopasture is seen as a primary barrier to adoption and ongoing management (Smith et al., 2022a), technical assistance is also likely a constraint for silvopasture adoption (Stutzman et al., 2020) and ongoing technical assistance has been shown to lead to greater adoption of improved practices in livestock production generally (Bragança et al., 2022). Availability of trees to accommodate expansion of silvopasture on the scale modeled here, could also be a barrier to realizing this potential (Fargione et al., 2021).
4.2. Potential C accrual
Our estimates of C sequestration rates across nine distinct silvopastoral systems varied widely, from 0.53 to 6.45 tons CO2e ha−1 yr.−1. This illustrates that it can be misleading to use a single estimate for C sequestration of silvopasture, as this varies greatly based on the species due to both per tree accrual rates (Table 4) and planting density (Table 1). Carbon accrual rates for each species could be higher or lower than the rates used here, with either shifts in per-tree accruals given site-specific conditions or planting density. For example, carbon accrual rates vary as a function of site productivity. Although we did not vary our rates based on site productivity, the average land capability class (a measure of site productivity used by the USDA) in the potential silvopasture lands that we identified was 5.1. This is equivalent to relatively productive pasture lands, but less than most current croplands. With this caveat in mind, our Full Adoption scenario had an average 1.0 tons CO2 ha−1 yr.−1, given our expected mix of agroforestry systems. For comparison, in an analysis of silvopasture mitigation potential in Canada, Drever et al. (2021) estimate accrual of an average rate of 2.87 tons CO2e ha−1 yr.−1. Another analysis of U.S. silvopasture, estimated an average rate of 22.41 tons CO2e ha−1 yr.−1 (Udawatta and Jose, 2012). Other agroforestry estimates for the U.S. fall within this range at 4.87 tons CO2e ha−1 yr.−1 from alley cropping and 12.22 tons CO2e ha−1 yr.−1 for windbreaks (Fargione et al., 2018). Our lower average per hectare estimate is due to the prominence of fodder systems in our Full Adoption scenario. We find these systems to currently be the most economically viable system, but to have lower C sequestration rates than other systems.
Notably, the silvopastoral systems that generally offer the most mitigation potential in this analysis are not necessarily those with high rates of C accrual, but instead those with high estimated market demand (Figure 3). Fodder species, which were assumed not to be constrained by demand, given the high and ongoing need for feed, have the lowest C accrual rates yet offer the most potential because they could expand to cover the greatest area. In contrast, demand was considered low for some of the higher-accruing species based on estimated market demand. Consequently, if there were increased demand for products from species like Black Locust or Hybrid Poplar, the overall mitigation potential of silvopasture in the eastern U.S. could increase.
The mitigation estimates here are potentially conservative in that they do not include increases in soil C from silvopasture establishment. Given the lack of data on soil C change with specific silvopasture tree species, we chose to focus solely on the mitigation potential from aboveground and belowground biomass. Aboveground biomass is the main source of increased C in agroforestry systems, especially silvopasture (Shi et al., 2018). However, a global meta-analysis found that converting pasture or grassland to silvopasture increased soil C in the top 30 cm by 10% on average (De Stefano and Jacobson, 2018). Additional research on soil C change by silvopasture species would help clarify the full mitigation potential.
The mitigation estimates presented here are comparable to other estimates, despite a difference in approach. We estimated mitigation potential by estimating C accrual per tree using allometric equations and scaling up based on planting densities, which differs from approaches using plot-based estimates of C accrual. Allometric equations assume ample space for tree growth. Consistent with this approach, our estimated planting spacing is large enough to allow trees to grow to financial maturity. In contrast, estimates from the literature based on plot-based methods may include slightly less growth and C accrual than what allometric equations predict if trees are planted close enough together to cause crowding. Here we use initial planting densities ranging between 88 and 5,382 trees/ha depending on the specific species (with fodder species having 15 times higher density than the average planting for timber and nut species; Supplementary Table S2). Because both the Base Case and Full Adoption scenarios assume a majority of silvopasture hectares are planted using fodder crops, the distribution of systems in this study is also weighted toward the higher density plantings (although also lower C accruing species; Table 4). In contrast, other studies exploring silvopasture mitigation potential assume 111 trees/ha (Drever et al., 2021).
Overall, this analysis provides evidence that market-driven expansion of silvopasture in the eastern U.S. would make a meaningful contribution to mitigating greenhouse gas emissions from agriculture. The U.S.’s agricultural emissions in 2020 were 594.7 Tg CO2e, or 9.9% of total U.S. emissions (EPA, 2022). Annual life cycle emissions from beef cattle production in the U.S. have been estimated as 243 Tg CO2e (Rotz et al., 2019). Although we did not perform a full life cycle assessment, the gross sequestration identified here suggests that silvopasture could reduce current life cycle cattle emissions by up to 11% under favorable market conditions in the Full Adoption scenario (including through a great expansion of fodder systems, Hybrid Poplar satisfying more of the dimensional lumber market, and through expansion of specialty products such as Black Locust decking).
4.3. Economic potential
Silvopastoral systems could offer additional revenue sources for farmers and ranchers already grazing cattle on pastures and grasslands. One comparison of different agricultural systems in the southern U.S. found loblolly pine silvopasture (with timber products) had 10% higher annual income than cattle production alone when interest rates were low and 27% higher income than cattle production alone when factoring in revenue from hunting leases that were made possible by improved wildlife in the silvopastoral system) (Husak and Grado, 2002). However, in some settings, silvopasture may be less profitable than either exclusively timber or cattle production systems, depending on capital costs and market conditions (Bruck et al., 2019) and potential impacts on forage production (Lin et al., 1998), which would likely depend on the specific system in use (e.g., DeBruyne et al., 2011). Indeed, although our analysis underscores the strong potential profitability of silvopasture generally, not all systems assessed here appear profitable on a 30-year timeline (e.g., Black Walnut). A complete economic analysis, including quantifying opportunity costs, would be important for any given operation.
Our analysis is unique in comparing economics across different silvopastoral system types. In doing so, we highlight how different systems offer distinct economic considerations and benefits, including how quickly they become profitable and how profitable they become. For example, because of the ongoing needs for feed and fast growth rates, fodder systems could be particularly profitable in the short term (breaking even in less than ten years). In contrast, Chestnut requires more upfront capital and takes longer to break even than fodder systems but has a 30-year NPV that is ~14 times higher than the fodder systems, on average. In addition, species require different planting, management, and harvest activities (Supplementary Table S2), which can affect costs. For example, while many species would likely benefit from protective tree tubes, the associated cost of ~$8/tree makes them economically unviable for many species. Here we only model them for species whose returns unquestionably justify an elevated upfront cost to ensure survival (e.g., Chestnut). As a result, a farmer may need to plant unprotected species in locations with lower deer pressure, unless incentives are available to offset the cost of tree tubes. Thus, in addition to consideration of different species and systems, farm-level analysis with context-specific economics is essential producers to successfully implement agroforestry on their farm. For example, farm-scale economics of adopting different silvopasture systems could change across different farm sizes. Here we modeled economics based on a 100-acre agroforestry operation, for simplicity, however farm size varies in the modeled states from 55 acres in Rhode Island to 372 acres in Illinois, with an average of 203 acres across modeled states (USDA NASS, 2017).
A robust price on C could increase the pace and extent of silvopasture adoption in the eastern U.S. Using a 10-year 7% IRR as a threshold, which is common for investors, only two of the nine systems studied exceed this threshold without any C payments. Of the remaining seven systems, a C payment of $10/ton does not cause any of them to exceed this hurdle, and a price of $100/ton would add three additional systems (Willow, Longleaf Pine, and Black Locust). While $100/ton exceeds current prices on C, the social cost of C exceeds $100/ton, with many forecasters projecting C prices to pass this threshold (Rennert et al., 2022). For some of the systems that do not meet a 10-year 7% IRR, it is because the trees’ products mature later. For example, Chestnuts may not produce a heavy crop until year 11, highlighting the need for patient capital. Emerging ecosystem service markets or policies that support habitat creation or water filtration could additionally improve economic outlooks for certain silvopastoral species, perhaps even favoring the higher C-accruing species or even species important for ecosystem restoration that may not have market demand (e.g., Longleaf Pine in the Southeastern U.S.). Furthermore, existing programs like the USDA Natural Resources Conservation Service’s Environmental Quality Incentives Program (EQIP) could mitigate upfront costs of silvopasture establishment and shift the economics for farmers to be more favorable.
The analysis here explores the potential for silvopasture across the eastern U.S. based on a suite of market assumptions, as opposed to an assessment of where silvopasture should be adopted. Specific economic, ecological, site- and operation-level considerations are critical to address the appropriateness of silvopasture systems or species and the potential C and economic impacts for any given situation. For example, some of the species included here are non-native tree species that, while offering agricultural potential, could carry ecological challenges that would need to be considered. In particular, before planting, it is important to assess the potential aggressive, spreading, or crowding tendencies of any species or cultivar under the given environmental conditions as part of a long-term planting plan for a given property.
Additional research exploring the market potential for native species-based silvopasture systems, for example a native hybrid cross Populus x canadensis instead of Populus x canescens, would be critical to identifying solutions that support farmer economics, C storage, and conservation.
Future research could also explore the climate- and context-dependent C accrual rates for each species to further refine estimates for climate mitigation from adding trees to existing pasture. Finally, while this analysis offers insight into the carbon and economic implications of adding trees to existing pasture, additional work is needed to holistically synthesize the net climate and economic impacts of these systems. For example, our estimates of economic returns may be high because we do not consider potential shading effects reducing forage production and viable herd size. However, our economic benefits may also be underestimated since we do not consider how shade can improve livestock productivity. Growing evidence suggests that silvopasture can provide essential cooling services (Zeppetello et al., 2022) that can reduce heat stress for livestock and improve performance (Kendall et al., 2006; Smith et al., 2022a). This will likely become even more critical as extreme heat events become more common under climate change. Moreover, further work is needed to assess how climate change-induced shifts in temperature and precipitation patterns might affect silvopasture systems and their future economics (e.g., with increased need for irrigation for some systems, or reduced heat or cold stress for animals) and climate benefits (e.g., with shifting tree growth rates).
4.4. Conclusion
In conclusion, silvopasture offers a financially beneficial C storage opportunity for farmers and ranchers in the eastern U.S. If broadly implemented, silvopasture could result in meaningful C mitigation for the agricultural sector in the eastern U.S. Silvopastoral systems, particularly fast-growing fodder species, are feasible today and could add additional revenue for farmers and ranchers. A robust price on C could further accelerate and expand adoption of these practices, and potentially shift investment toward longer-lived timber species with greater per hectare C sequestration benefits.
Data availability statement
The raw data supporting the conclusions of this article will be made available by the authors, without undue reservation.
Author contributions
JF, KJ, HG, and JK conceived the work. HG and JK designed the study. HG compiled the data. JK and ES contributed to the analyses. CK and HG wrote the first draft. All authors contributed substantially to writing and interpretation of data.
Funding
This study was funded by the Nature Conservancy. The Bezos Earth Fund supported SC-P’s time on this manuscript.
Acknowledgments
We thank Brandon Bless, Brett Chedzoy, Colette DeGarady, Darren Doherty, Julio Domingo Garcia, Gabor Erdélyi, Jorge Esquivel, Steve Gabriel, Eliza Greenman, Will Harris, Keavin Hill, Cesar Hompart, Veronica Honfy, Amy Miller, Greg Miller, Márton Németh, Enrique Murgueitio Restrepo, Zach Rike, Grant Schultz, Norberto Serventi, Larry Smart, Steve Timmermans, and Tom Wahl for sharing their management experiences and for informing our understanding of the operational dynamics for different silvopastoral systems. We also thank Ciara Hovis, Nadia Alsadi, Alicia Calle, and Brian Campbell for their input and feedback on this article, and two reviewers whose input and suggestions greatly improved the manuscript.
Conflict of interest
HG (Chief Research Officer), JK (Chief Operations Officer), and ES (Chief Executive Officer) are co-founders of Propagate, a business that provides financial analytics, project development, and financing of agroforestry systems. Propagate is a public benefit corporation, and the provision of ecosystem services and rural livelihoods is written into the charter.
The remaining authors declare that the research was conducted in the absence of any commercial or financial relationships that could be construed as a potential conflict of interest.
Publisher’s note
All claims expressed in this article are solely those of the authors and do not necessarily represent those of their affiliated organizations, or those of the publisher, the editors and the reviewers. Any product that may be evaluated in this article, or claim that may be made by its manufacturer, is not guaranteed or endorsed by the publisher.
Supplementary material
The Supplementary material for this article can be found online at: https://www.frontiersin.org/articles/10.3389/fsufs.2023.1158459/full#supplementary-material
Footnotes
References
Bragança, A., Newton, P., Cohn, A., Assunção, J., Camboim, C., de Faveri, D., et al. (2022). Extension services can promote pasture restoration: evidence from Brazil’s low carbon agriculture plan. Proc. Natl. Acad. Sci. U. S. A. 119:e2114913119. doi: 10.1073/pnas.2114913119
Brandle, J. R., Hodges, L., and Zhou, X. H. (2004). Windbreaks in north American agricultural systems. Agrofor. Syst. 61, 65–78. doi: 10.1007/978-94-017-2424-1_5
Brown, S. E., Miller, D. C., Ordonez, P. J., and Baylis, K. (2018). Evidence for the impacts of agroforestry on agricultural productivity, ecosystem services, and human well-being in high-income countries: a systematic map protocol. Environ. Evid. 7:24. doi: 10.1186/s13750-018-0136-0
Bruck, S. R., Bishaw, B., Cubbage, T. L., and Cubbage, F. W. (2019). Modeling the financial potential of silvopasture agroforestry in eastern north carolina and northeastern oregon. J. For. 117, 13–20. doi: 10.1093/jofore/fvy065
Cardinael, R., Umulisa, V., Toudert, A., Olivier, A., Bockel, L., and Bernoux, M. (2018). Revisiting IPCC tier 1 coefficients for soil organic and biomass carbon storage in agroforestry systems. Environ. Res. Lett. 13:124020. doi: 10.1088/1748-9326/aaeb5f
Chapman, M., Walker, W. S., Cook-Patton, S. C., Ellis, P. W., Farina, M., Griscom, B. W., et al. (2020). Large climate mitigation potential from adding trees to agricultural lands. Glob. Chang. Biol. 26, 4357–4365. doi: 10.1111/gcb.15121
Cook-Patton, S. C., Gopalakrishna, T., Daigneault, A., Leavitt, S. M., Platt, J., Scull, S. M., et al. (2020). Lower cost and more feasible options to restore forest cover in the contiguous United States for climate mitigation. One Earth 3, 739–752. doi: 10.1016/j.oneear.2020.11.013
De Stefano, A., and Jacobson, M. G. (2018). Soil carbon sequestration in agroforestry systems: a meta-analysis. Agrofor. Syst. 92, 285–299. doi: 10.1007/s10457-017-0147-9
DeBruyne, S. A., Feldhake, C. M., Burger, J. A., and Fike, J. H. (2011). Tree effects on forage growth and soil water in an Appalachian silvopasture. Agrofor. Syst. 83, 189–200. doi: 10.1007/s10457-011-9376-5
Dickens, E. D., Sunday, J., and Moorhead, D. J. (2014). Series paper# 8 economics of growing loblolly, longleaf, and slash pine to various rotation ages with three stumpage price sets, four establishment cost sets, four discount rates, with and without pine straw–soil expectation value. University of Georgia and Georgia Forestry Commission.
Dold, C., Thomas, A. L., Ashworth, A. J., Philipp, D., Brauer, D. K., and Sauer, T. J. (2019). Carbon sequestration and nitrogen uptake in a temperate silvopasture system. Nutr. Cycl. Agroecosyst. 114, 85–98. doi: 10.1007/s10705-019-09987-y
Drever, C. R., Cook-Patton, S. C., Akhter, F., Badiou, P. H., Chmura, G. L., Davidson, S. J., et al. (2021). Natural climate solutions for Canada. Sci. Adv. 7:eabd6034. doi: 10.1126/sciadv.abd6034
Edwards-Callaway, L. N., Cramer, M. C., Cadaret, C. N., Bigler, E. J., Engle, T. E., Wagner, J. J., et al. (2021). Impacts of shade on cattle well-being in the beef supply chain. J. Anim. Sci. 99, 1–21. doi: 10.1093/jas/skaa375
EPA (2022) Inventory of U.S. greenhouse gas emissions and sinks: 1990-2020. U.S. Environmental Protection Agency, EPA 430-R-22-003. Available at: https://www.epa.gov/ghgemissions/draft-inventory-us-greenhouse-gas-emissions- and-sinks-1990-2020.
Fargione, J. E., Bassett, S., Boucher, T., Bridgham, S. D., Conant, R. T., Cook-Patton, S. C., et al. (2018). Natural climate solutions for the United States. Sci. Adv. 4:eaat1869. doi: 10.1126/sciadv.aat1869
Fargione, J., Haase, D. L., Burney, O. T., Kildisheva, O. A., Edge, G., Cook-Patton, S. C., et al. (2021). Challenges to the reforestation pipeline in the United States. Front. For. Glob. Change 4:629198. doi: 10.3389/ffgc.2021.629198
Garrett, H. E., Kerley, M. S., Ladyman, K. P., Walter, W. D., Godsey, L. D., Van Sambeek, J. W., et al. (2004). “Hardwood silvopasture management in North America” in New vistas in agroforestry: a compendium for 1st world congress of agroforestry, 2004, Eds. P. K. R. Nair, M. R. Rao and L. E. Buck (Springer Netherlands), 21–33.
Gonzalez-Benecke, C. A., Gezan, S. A., Martin, T. A., Cropper, W. P. J., Samuelson, L. J., and Leduc, D. J. (2014). Individual tree diameter, height, and volume functions for longleaf pine. For. Sci. 60, 43–56. doi: 10.5849/forsci.12-074
Griscom, B. W., Adams, J., Ellis, P. W., Houghton, R. A., Lomax, G., Miteva, D. A., et al. (2017). Natural climate solutions. Proc. Natl. Acad. Sci. U. S. A. 114, 11645–11650. doi: 10.1073/pnas.1710465114
Henning, J. C., and Wheaton, H. N. (1993). Making and storing quality hay University of Missouri Extension Available at: https://extension.missouri.edu/publications/g4575.
Husak, A. L., and Grado, S. C. (2002). Monetary benefits in a southern silvopastoral system. South. J. Appl. For. 26, 159–164. doi: 10.1093/sjaf/26.3.159
Jose, S. (2012). Agroforestry for conserving and enhancing biodiversity. Agrofor. Syst. 85, 1–8. doi: 10.1007/s10457-012-9517-5
Kendall, P. E., Nielsen, P. P., Webster, J. R., Verkerk, G. A., Littlejohn, R. P., and Matthews, L. R. (2006). The effects of providing shade to lactating dairy cows in a temperate climate. Livest. Sci. 103, 148–157. doi: 10.1016/j.livsci.2006.02.004
Lehmkuhler, J. W., Felton, E. E. D., Schmidt, D. A., Bader, K. J., Garrett, H. E., and Kerley, M. S. (2003). Tree protection methods during the silvopastoral-system establishment in midwestern USA: cattle performance and tree damage. Agrofor. Syst. 59, 35–42. doi: 10.1023/A:1026184902984
Lin, C. H., McGraw, R. L., George, M. F., and Garrett, H. E. (1998). Shade effects on forage crops with potential in temperate agroforestry practices. Agrofor. Syst. 44, 109–119. doi: 10.1023/A:1006205116354
Ma, S. H., Eziz, A., Tian, D., Yan, Z. B., Cai, Q., Jiang, M. W., et al. (2020). Size-and age-dependent increases in tree stem carbon concentration: implications for forest carbon stock estimations. J. Plant Ecol. 13, 233–240. doi: 10.1093/jpe/rtaa005
Mercier, K. M., Teutsch, C. D., Fike, J. H., Munsell, J. F., Tracy, B. F., and Strahm, B. D. (2020). Impact of increasing shade levels on the dry-matter yield and botanical composition of multispecies forage stands. Grass Forage Sci. 75, 291–302. doi: 10.1111/gfs.12489
Nair, P. K. R. (2012). “Climate change mitigation: a low-hanging fruit of agroforestry” in Agroforestry—the future of global land use. eds. P. K. R. Nair and D. Garrity (Dordrecht: Springer), 31–67.
Nair, P. K. R., Kumar, B. M., and Nair, V. D. (2021). An introduction to agroforestry: four decades of scientific developments. Springer, Cham.
Orefice, J., Carroll, J., Conroy, D., and Ketner, L. (2017). Silvopasture practices and perspectives in the northeastern United States. Agrofor. Syst. 91, 149–160. doi: 10.1007/s10457-016-9916-0
Panayotov, P., Valchev, I., Kalmukov, K., Panayotov, M., Petrin, S., and Yegorov, N. (2015). Black locust wood–perspective raw material for production of chemical pulp and glucose. For. Ideas 21, 307–316.
Pietrzykowski, M., Woś, B., Tylek, P., Kwaśniewski, D., Juliszewski, T., Walczyk, J., et al. (2021). Carbon sink potential and allocation in above-and below-ground biomass in willow coppice. J. For. Res. 32, 349–354. doi: 10.1007/s11676-019-01089-3
Polsky, L., and von Keyserlingk, M. A. G. (2017). Invited review: effects of heat stress on dairy cattle welfare. J. Dairy Sci. 100, 8645–8657. doi: 10.3168/jds.2017-12651
Reid, Rowan. Heartwood: the art and science of growing trees for conservation and profit. Melbourne: Melbourne Books (2017).
Rennert, K., Errickson, F., Prest, B. C., Rennels, L., Newell, R. G., Pizer, W., et al. (2022). Comprehensive evidence implies a higher social cost of CO2. Nature 610, 687–692. doi: 10.1038/s41586-022-05224-9
Roe, S., Streck, C., Beach, R., Busch, J., Chapman, M., Daioglou, V., et al. (2021). Land-based measures to mitigate climate change: potential and feasibility by country. Glob. Chang. Biol. 27, 6025–6058. doi: 10.1111/gcb.15873
Rotz, C. A., Asem-Hiablie, S., Place, S., and Thoma, G. (2019). Environmental footprints of beef cattle production in the United States. Agric. Syst. 169, 1–13. doi: 10.1016/j.agsy.2018.11.005
Salceda, M., Udawatta, R. P., Anderson, S. H., Mendis, S. S., and Liu, F. (2023). Agroforestry buffers on nitrogen reduction in groundwater on a grazed hillslope. Agrosyst. Geosci. Environ. 6:e20370. doi: 10.1002/agg2.20370
Shi, L., Feng, W., Xu, J., and Kuzyakov, Y. (2018). Agroforestry systems: Meta-analysis of soil carbon stocks, sequestration processes, and future potentials. Land Degrad. Dev. 29, 3886–3897. doi: 10.1002/ldr.3136
Smith, M. M., Bentrup, G., Kellerman, T., MacFarland, K., Straight, R., and Ameyaw, L. (2022b). Agroforestry extent in the United States: a review of national datasets and inventory efforts. Agriculture 12:726. doi: 10.3390/agriculture12050726
Smith, M. M., Bentrup, G., Kellerman, T., MacFarland, K., Straight, R., Ameyaw, L., et al. (2022a). Silvopasture in the USA: a systematic review of natural resource professional and producer-reported benefits, challenges, and management activities. Agric. Ecosyst. Environ. 326:107818. doi: 10.1016/j.agee.2021.107818
Smith, M. W., and Wood, B. W. (2006). Pecan tree biomass estimates. HortScience 41, 1286–1291. doi: 10.21273/HORTSCI.41.5.1286
Stutzman, E., Barlow, R. J., Morse, W., Monks, D., and Teeter, L. (2020). Natural resource professionals’ engagement with landowners on silvopasture in the southeastern United States. Agrofor. Syst. 94, 2137–2146. doi: 10.1007/s10457-020-00536-z
Torralba, M., Fagerholm, N., Burgess, P. J., Moreno, G., and Plieninger, T. (2016). Do European agroforestry systems enhance biodiversity and ecosystem services? A meta-analysis. Agric. Ecosyst. Environ. 230, 150–161. doi: 10.1016/j.agee.2016.06.002
Udawatta, R. P., and Jose, S. (2012). Carbon sequestration potential of agroforestry practices in temperate North America. Agrofor. Syst. 86, 225–242. doi: 10.1007/978-94-007-1630-8_2
USDA NASS (2017) Census of agriculture. Available at: www.nass.usda.gov/AgCensus
USDA Plant Hardiness Zone Map (2012). Agricultural Research Service U.S. Department of Agriculture Available at: https://planthardiness.ars.usda.gov/.
Varsha, K. M., Raj, A. K., Kurien, E. K., Bastin, B., Kunhamu, T. K., and Pradeep, K. P. (2019). High density silvopasture systems for quality forage production and carbon sequestration in humid tropics of southern India. Agrofor. Syst. 93, 185–198. doi: 10.1007/s10457-016-0059-0
World Bank, Ecofys, and Vivid Economics (2017). State and trends of carbon pricing 2017. Washington, DC: World Bank. Available at: https://openknowledge.worldbank.org/handle/10986/28510
Wotherspoon, A., Thevathasan, N. V., Gordon, A. M., and Voroney, R. P. (2014). Carbon sequestration potential of five tree species in a 25-year-old temperate tree-based intercropping system in southern Ontario, Canada. Agrofor. Syst. 88, 631–643. doi: 10.1007/s10457-014-9719-0
Keywords: natural climate solutions, carbon storage, sequestration, agroforestry, economics, cattle, livestock grazing, pasture
Citation: Greene H, Kazanski CE, Kaufman J, Steinberg E, Johnson K, Cook-Patton SC and Fargione J (2023) Silvopasture offers climate change mitigation and profit potential for farmers in the eastern United States. Front. Sustain. Food Syst. 7:1158459. doi: 10.3389/fsufs.2023.1158459
Edited by:
Peter Newton, University of Colorado Boulder, United StatesReviewed by:
Matthew Smith, United States Department of Agriculture (USDA), United StatesDiane Mayerfeld, University of Wisconsin-Madison, United States
Copyright © 2023 Greene, Kazanski, Kaufman, Steinberg, Johnson, Cook-Patton and Fargione. This is an open-access article distributed under the terms of the Creative Commons Attribution License (CC BY). The use, distribution or reproduction in other forums is permitted, provided the original author(s) and the copyright owner(s) are credited and that the original publication in this journal is cited, in accordance with accepted academic practice. No use, distribution or reproduction is permitted which does not comply with these terms.
*Correspondence: Clare E. Kazanski, Y2xhcmUua2F6YW5za2lAdG5jLm9yZw==