- 1Graduate School of Integrated Sciences for Life, Hiroshima University, Higashihiroshima, Japan
- 2Department of Agriculture, Higher Technical Teachers’ Training College, University of Buea, Yaounde, Cameroon
- 3Department of Agronomy, Hajee Mohammad Danesh Science and Technology University, Dinajpur, Bangladesh
- 4VIT School of Agricultural Innovations and Advanced Learning (VAIAL), Vellore Institute of Technology (VIT), Vellore, India
- 5Department of Plant Production, College of Food and Agriculture Sciences, King Saud University, Riyadh, Saudi Arabia
- 6Department of Agronomy, Faculty of Agriculture, Kafrelsheikh University, Kafrelsheikh, Egypt
- 7Department of Field Crops, Faculty of Agriculture, Siirt University, Siirt, Türkiye
Oat (Avena nuda L.) is a globally important cereal crop grown for its nutritious grains and is considered as moderately salt-tolerant. Studying salinity tolerant mechanisms of oats could assist breeders in increasing oat production and their economic income in salt-affected areas, as the total amount of saline land in the world is still increasing. The present study was carried out to better understand the salt tolerance mechanism of the naked oat line Bayou1. A soil experiment was conducted on 17 days-old Bayou1 seedlings treated with varying concentrations of NaCl for a period of 12 days. Bayou1 plants grew optimally when treated with 50 mM NaCl, demonstrating their salinity tolerance. Reduced water uptake, decreased Ca2+, Mg2+, K+, and guaiacol peroxidase activity, as well as increased Na+ concentration in leaves, all contributed to a reduction in shoot growth. However, the damage to ionic homeostasis caused by increased Na+ concentrations and decreased K+ concentrations in the roots of Bayou1 did not inhibit its root growth, indicating that the main salt-tolerant mechanism in Bayou1 existed in its roots. Further, a hydroponic experiment found that increasing Na+ concentration in root cell sap enhanced root growth, while maintaining the integrity of root cell membranes. The accumulated Na+ may have facilitated the root growth of Bayou1 exposed to NaCl by effectively adjusting cellular osmotic potential, thereby ensuring root cell turgor and expansion.
1 Introduction
Saline land is increasing in arid, semi-arid, and coastal regions due to the use of medium or high-saline water for irrigation. It is important to search for salinity-tolerant crops to meet the food demand of a growing population and to enhance the economic and ecological values of these regions, where crop production and food safety face significant challenges (Gupta and Huang, 2014). In plants, salinity causes osmotic stress, followed by ionic stress, which induces secondary stresses including oxidative stresses (Wang et al., 2022). Osmotic stress inhibits water assimilation and ionic stress causes ionic toxicity in salt-stressed plant tissues due to excessive accumulation of sodium (Na+) ions (Negrão et al., 2017).
In general, Na+ ions are not essential for plant growth, and excessive concentrations of these ions are toxic to the most plant species. The increased concentration of Na+ in salt-stressed plant tissues frequently inhibits the uptake of other nutrients, including K+, Ca2+, and Mg2+, resulting in nutrient deficiency (Keutgen and Pawelzik, 2009; Assaha et al., 2017b). Thus, the maintenance of ionic homeostasis in plants by modulating ion transporters, channels, and H+ pumps is essential for plant tolerance to salinity (Almeida et al., 2017). Plants regulate Na+ uptake through transporters or channels to prevent excessive accumulation in the cytosol, returning Na+ to growth medium or apoplast, or compartmentalizing it into vacuoles (Lv et al., 2012). Cytosolic Na+ ions are compartmentalized into vacuoles for efficient Na+ detoxification, reducing cytosol toxic effects and maintaining osmotic potential, ensuring water uptake in cells (Apse and Blumwald, 2007; Lv et al., 2012). Na+ are employed as a cost-effective osmolyte in plant tissues subjected to salt stress (Hariadi et al., 2011). Oxidative stress disrupts the balance between reactive oxygen species production and scavenging, leading to cell membrane damage and ion leakage (Gill and Tuteja, 2010; Huang et al., 2019). Plants utilize both non-enzymatic and enzymatic antioxidant defense systems, primarily catalase (CAT), glutathione peroxidase (GPX), and guaiacol peroxidase (POX), to prevent oxidative damage.
The naked oat (Avena nuda L.), which originated in Mongolia and northern China, differs from the common oat (Avena sativa L.) in morphology, nutritional value, and growth environment (Tang et al., 2019). Because of its rich supply of vitamins, minerals, carbohydrates, lipids, and oil, naked oats constitute an essential cereal crop that is grown all over the world and utilized for both human and livestock food (Carlson and Kaeppler, 2007; Daou and Zhang, 2012; Boczkowska and Tarczyk, 2013; Diao, 2017). In recent years, oat has received more attention due to its various grain bioactive compounds, which have been linked to a lower risk of cardiovascular disease, type 2 diabetes, gastrointestinal disorders, and cancer (Martínez-Villaluenga and Peñas, 2017). This traditional Chinese cereal is gaining popularity due to its cold weather tolerance, adaptation to short growing seasons and poor soil fertility (Daou and Zhang, 2012; Boczkowska and Tarczyk, 2013; Bai et al., 2018). On the contrary, the growth of naked oats is significantly impeded and its efficient utilization and development are restricted by abiotic stresses such as salinity and drought (Liu et al., 2020a,b; Zhang et al., 2022). When treated to drought stress, Huazao2 had lower yield, grain per panicle, grain weight per thousand grains, and transpiration rate (Zhang et al., 2022). Due to an imbalance in ionic homeostasis in its leaves, the growth of a 34 days-old Mengnongda1 seedling is inhibited when exposed to 100 mM NaCl (Liu et al., 2020a). The application of 50 mM sodic-alkalinity caused a reduction in the activities of antioxidant enzymes in a 20 days-old Yanke1 seedling, which affected its growth (Liu et al., 2020b). The study of salinity-tolerant naked oat lines and their mechanisms can be beneficial for crop breeding in salt-affected areas due to increasing agricultural difficulties. Figure 1 depicts the mechanisms of salinity tolerance in oats. Enhanced salt tolerance in oats is achieved through the induction of adaptive stress mechanisms. The upregulation of NHX1 compartmentalize Na+ into vacuole while the upregulation of SOS1 returns Na+ to growth media, resulting in Na+ detoxification and ionic equilibrium in the cytosol. Superoxide dismutase (SOD) and glutathione reductase (GR) catalyze the conversion of O2− to H2O2, which is further detoxified to water and oxygen by GPX and CAT. By maintaining ion homeostasis and detoxifying oxidative damage within its cellular environment, these mechanisms enable the plant to effectively endure and survive in environments with high salinity. The utilization of this adaptation mechanism confers benefits and exhibits discernible impacts in different growing regions of naked oats. Depending on the specific target organ, these mechanisms have different effects on different plant organs, such as flowers, fruits, leaves, roots, and cellular components.
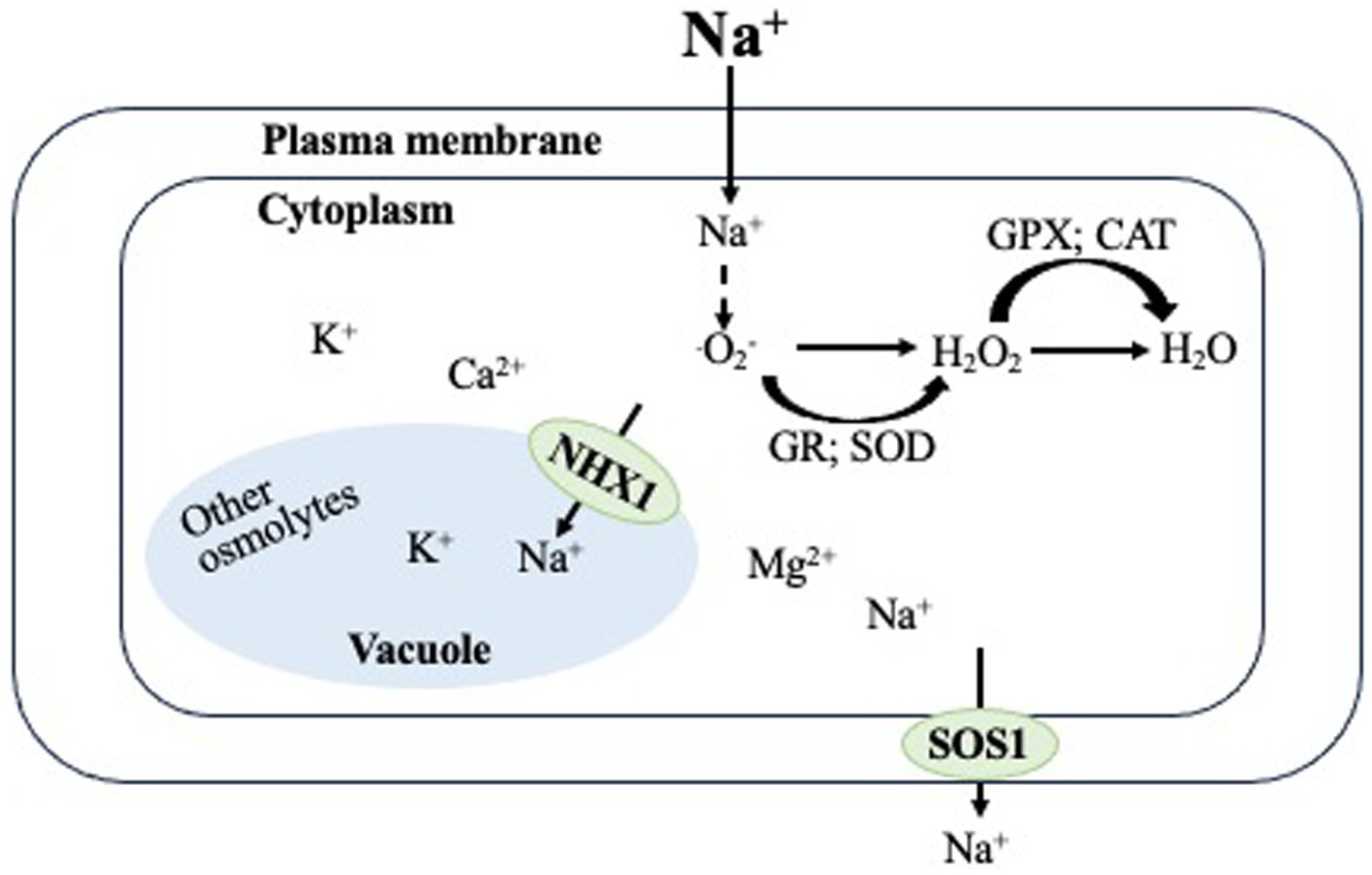
Figure 1. The oat plant’s response mechanism to salt tolerance initiates adaptive stress mechanisms, allowing it to adapt and endure high salinity conditions by effectively managing and balancing ions within its cells. This adaptive mechanism is advantageous and manifests different effects in various plant organs. These superior mechanisms influence various plant organs, including flowers and fruits, leaves, roots, and cellular components, in a variety of ways, depending on the target organ. In addition, it upregulates a number of mechanisms, including HKT1, SOS1, and NHX1, leading to an increase in abscisic acid (ABA) levels.
Bayou1, a widely grown line in Inner Mongolia, Hebei, Shanxi, and Gansu, China, has a high recovery ability from high salinity due to its ionic homeostasis and osmolyte production in its leaves (Diao, 2017; Liu and Saneoka, 2022). This high recovery capacity from high salinity reveals its high salinity tolerance. However, its salinity tolerance mechanism remains unclear. The study aims to identify the adaptive mechanisms of Bayou1 in response to low and moderate salinity levels using soil and hydroponic experiments. We investigated various parameters including leaf antioxidative enzyme activities, growth, assimilation of Na+ and K+ in all organs, water status, malondialdehyde (MDA) and Mg2+ and Ca2+ ions, in the leaves of soil experiment. Furthermore, we assessed the vigor of the roots in the hydroponic experiment, osmotic potential (Ψπ), Na+ assimilation, and MDA concentration.
2 Materials and methods
2.1 Experiment 1: growth conditions of Bayou1 in a soil experiment
The Inner Mongolia Agricultural University in China provided assistance with the cultivation of oat line Bayou1 (Avena nuda L.) seeds, which were stored at 4°C -until germination. Seeds were incubated at room temperature for 24 h before being sowed directly in 350 cm3 pots filled with commercial healthy soil and a plastic mesh at the bottom of pots. Pots were irrigated twice daily for 7 days, then five uniform seedings were selected and irrigated twice with a nutrient solution including 4.2 mM NO3-N, 0.3 mM NH4-N, 1.1 mM K2O, 1.0 mM CaO, 0.4 mM MgO, 0.2 mM P2O5, 12.1 μM Fe, 5.4 μM B2O3, 5.3 μM MnO, 0.3 μM Zn, 0.1 μM Cu, and 0.08 μM Mo. Seedlings were treated with a nutrient solution containing varying concentrations of NaCl [0 mM (control), 50 mM, and up to 100 mM NaCl] in increments of 50 mM to reach a final concentration on day 17 for an additional 12 days in order to prevent seedling mortality of young oat seedlings exposed to salinity. The treatment consisted of four pots, with each pot having five plants deemed a replication to ensure statistical analysis accuracy. The soil experiment was carried out in a vinyl house with natural light, with 61% humidity, 17°C–25°C day/10°C–17°C night temperature.
2.1.1 Growth analysis and relative water content in the leaves of Bayou1 under soil experiment
After 12 days of soil experiment treatment, tiller numbers of the seedlings were counted and the seedlings were washed with tap water. Seedlings were cleaned, separated into leaves, sheaths, and roots, and oven-dried at 70°C for 3 days to determine their dry weights. The young leaf, sheath, and root tissues were flash-frozen in liquid nitrogen and stored at −80°C until use for analyses. The second fully expanded young leaves were frozen in liquid nitrogen and stored at −80°C for further analysis. Mekawy et al. (2015) provide a comprehensive account of the methodology employed to ascertain the relative water content (RWC). The fresh weight of leaves was recorded before soaking in fresh distilled water for 24 h under light. After gently removing extra water from the wet surface, the turgor weight of leaves was measured. The leaves were then oven-dried for 3 days at 80°C and the dry weight was recorded. The leaf relative water content was determined using the following formula: 100 × (fresh weight-dry weight)/(turgor weight-dry weight).
2.1.2 Na+ and K+ in the different organs, and Ca2+ and Mg2+ concentrations in the leaves of Bayou1 under soil experiment
Approximately 500 mg of oven-dried organs were digested with HNO3 at 100°C for 12 h and then with HNO3− H2O2 (1:1 v/v) at 200°C for 20 min for cations. After dilution with distilled water, The Na+ and K+ concentrations in leaves, sheaths, and roots were measured using an ANA-135 flame photometer (Eiko Instruments Inc., Tokyo, Japan), while the Ca2+ and Mg2+ concentrations in leaves were measured using an AA-6200 atomic absorption flame emission spectrophotometer (Shimadzu Corporation Industrial Company, Kyoto, Japan). The Na+, K+, Ca2+, and Mg2+ standards were used to calculate their concentrations in the organs.
2.1.3 Malondialdehyde analysis in the leaves of Bayou1 under soil experiment
The MDA concentration in the second fully expanded young leaves was evaluated to assess leaf lipid peroxidation (Draper and Hadley, 1990). Young leaves were finely pulverized in liquid nitrogen using a mortar and pestle. MDA was extracted from the powder using a 10 mM 4-(2-hydroxyethyl)-1-piperazineethanesulfonic acid (HEPES) buffer solution containing thiobarbituric acid, tricarboxylic acid, butylated hydroxytoluene, 2% ethanol, and 0.25 N HCl. After heating at 100°C for 30 min, the absorbance of the reaction supernatant was measured spectrophotometrically (U-3310; Hitachi, Tokyo, Japan) at 532 and 600 nm. The MDA concentration was determined using its extinction coefficient (1.55 mM−1 cm−1).
2.1.4 Antioxidative enzyme activities in the leaves of Bayou1 under soil experiment
Soluble antioxidant enzymes were extracted from finely crushed powder in liquid nitrogen using a potassium phosphate buffer (pH 7.8) containing 1 mM ascorbic acid, 0.5 mM ethylenediaminetetraacetic acid, and 2% polyvinylpolypyrrolidone. Antioxidant enzyme activities in soluble protein and the concentration of soluble protein in crude extract were evaluated using the supernatant obtained after centrifugation (10,000 g, 4°C, 15 min). CAT activity was determined by measuring the initial rate of H2O2 disappearance at 240 nm, GPX activity by measuring tetraguaiacol absorbance at 470 nm, and GR activity by measuring the absorbance of oxidized NADPH at 340 nm in their respective reaction mixtures (Foyer and Halliwell, 1976; Aebi, 1984; Velikova et al., 2000; Liu et al., 2017). The reaction mixture for CAT activity contained 50 mM potassium phosphate buffer (pH 7.0), 10 mM hydrogen peroxide (H2O2), and 1% enzyme extract, and CAT activity was calculated using the molar extinction coefficient for H2O2 (0.04 mM−1 cm−1). The reaction mixture for GPX activity contains 70 mM potassium phosphate buffer (pH 7.0), 15 mM guaiacol, 10 mM hydrogen peroxide (H2O2), and 2% enzyme extract, and GPX activity was determined using the molar extinction coefficient for tetraguaiacol (26.6 mM−1 cm−1). The reaction mixture for GR activity contained 40 mM potassium phosphate buffer (pH 7.5), 0.78 mM glutathione, 0.4 mM EDTA, 0.02 mM NADPH, and 2% enzyme extract, and GR activity was estimated using the molar extinction coefficient for NADPH (6.22 mM−1 cm−1). The DC protein assay kit (BioRad Laboratories, Inc.) was used to determine the amount of soluble protein in the enzyme extraction.
2.2 Experiment 2: growth conditions of Bayou1 in a hydroponic system
The seeds were germinated on damp towel paper for 3 days after being incubated at room temperature for 24 h. The seedlings were transferred to a hydroponic system comprised of plastic mesh covering 1.3 L plastic containers with tap water. After 5 days of germination, tap water was replaced with a nutrient solution according to section 2.1. Twelve-days-old seedlings were cultivated hydroponically for 6 days under 0 (control), 25 and 50 mM NaCl concentrations. Throughout the experiment, the pH of the nutrient solution was maintained between 5.9 and 6.2 with 1 N KOH. The study consisted of four containers in each treatment, with four seedlings grown in each container, representing one replicate. The tap water and nutrition solution were replaced twice a day and aerated continuously with pumps. The experiment was conducted under natural light conditions in the greenhouse, which was kept at a temperature range of 23°C–31°C during the day and 15°C–23°C at night.
2.2.1 Plant growth, and oxidative damage and vigor in the root of Bayou1 under hydroponic system
The fresh weight of shoots and roots was measured after being washed with tap water and placed on a wiper to remove any water. MDA concentration in the roots was measured in the same manner as in the soil experiment to determine oxidative damage.
Fresh root samples (0.1 g) were incubated for 10 min at room temperature in 5 mL of 0.1 M phosphate buffer (pH 7.0) containing 125 μg of -naphthylamine (Zhang et al., 2017). After incubating for one hour at 25°C, 0.2 mL of the resulting solution was combined with 1 mL of distilled water, 0.1 mL of 1% sulfanilic acid, and 0.1 mL of 0.1% sodium nitrite. After being incubated for 5 min, the mixture was once more diluted with 1.1 mL of distilled water, and results were recorded using a spectrophotometer at 510 nm. Root vigor was measured in micrograms of α -naphthylamine per gramme of FW per hour (μg α-NA g−1 FW h−1).
2.2.2 Osmotic potential and Na+ concentration in the roots of Bayou1 under hydroponic system
Fresh roots were filled into microtubes, then placed in a 5 mL microtube and centrifuged at 1,500 × g for 10 min to collect cell sap. The Wescor 5,500 vapor pressure osmometer (Wescor Inc., Logan, UT, United States) was used to measure the Ψπ of the cell sap after centrifugation. Using a flame photometer (ANA-135; Eiko Instruments Inc., Tokyo, Japan), the Na+ concentrations of 100-fold diluted root cell fluid were then determined. The Ψπ of Na+ was subsequently computed by applying the Van’t Hoff equation derived by Liu et al. (2020a). The contribution of Ψπ for Na+ to the total Ψπ was calculated using the equation: Contribution of Ψπ for Na+ = 100 × Ψπ of Na+/ Ψπ.
2.3 Statistical analysis
All collected data were analyzed using one-way analysis of variance (ANOVA) with IBM SPSS version 25 (IBM Corp., Armonk, NY, United States). Duncan’s multiple range test was used to perform multiple comparisons at p ≤ 0.05. The values are the means (standard errors; SE) of four replicates. The values are the means (± standard errors; SE) of four replicates. The association between root biomass and other root characteristics was examined by a visual exploratory tool for correlation matrix using the corrplot package in the R programming language.
3 Results
3.1 Experiment 1
3.1.1 Effect of NaCl on biomass accumulation in soil experiment
At a concentration of 50 mM, NaCl greatly accelerated the growth of leaves and sheaths while inhibiting it at a concentration of 100 mM. When exposed to 50 mM NaCl, the DW of Bayou1 leaves and sheaths increased by 13% and 16%, respectively, but decreased by 10% and 9% when exposed to 100 mM NaCl. In contrast, neither 50 mM nor 100 mM NaCl concentrations changed root DW (Figure 2). When the concentration of NaCl was increased from 0 mM to 50 mM and then to 100 mM, the number of tillers in Bayou1 decreased by 16% and 28%, respectively (Table 1).
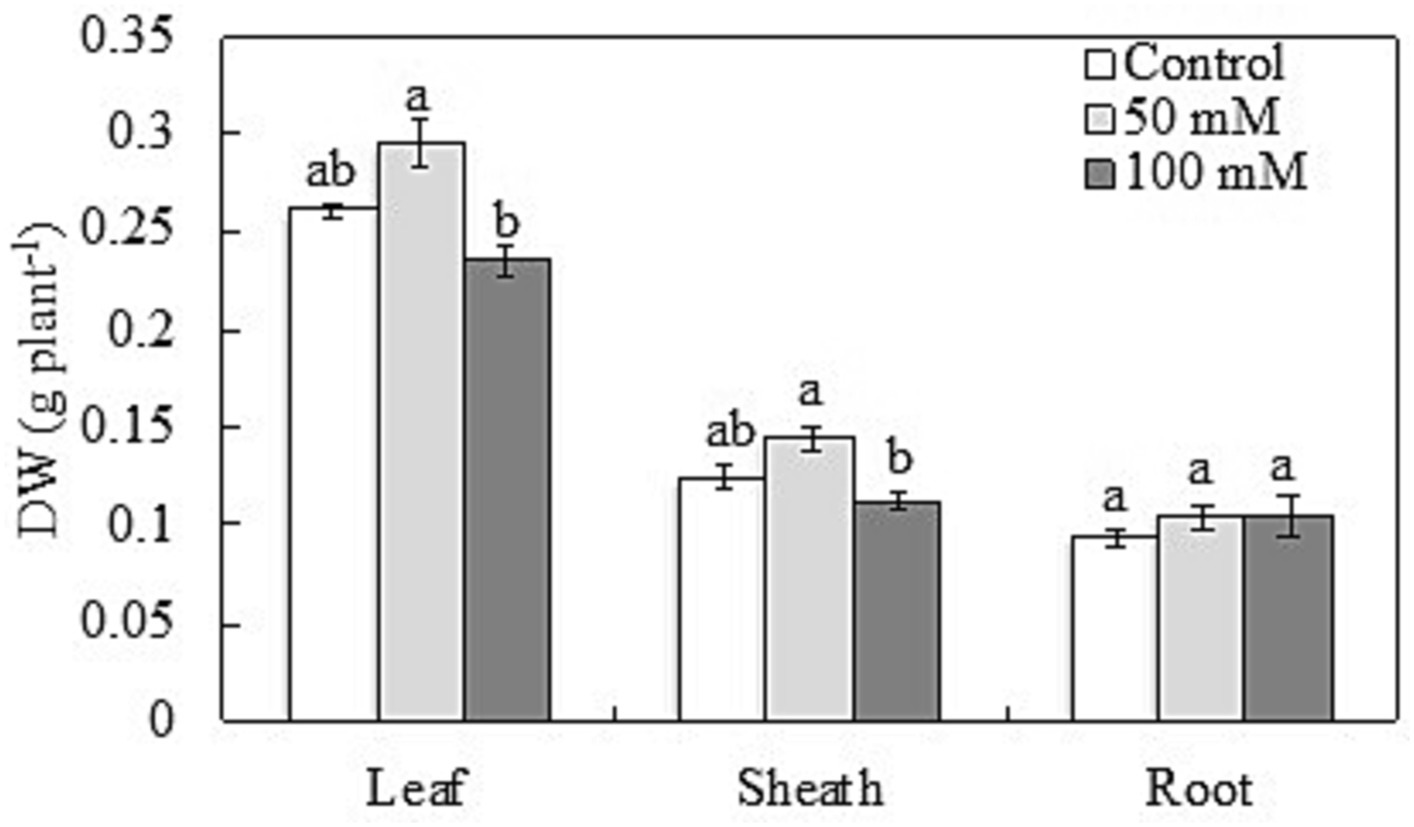
Figure 2. Plant dry weights in the leaves, sheathes, and roots of naked oat (Avena nuda L.) line Bayou1 under control conditions and 12 days of soil salinity. Values are means of four replicates ± standard error. Different lower-case letters indicate significant differences among treatments at p ≤ 0.05.

Table 1. Tiller number, Mg2+ concentration, and activities of catalase (CAT), glutathione reductase (GR), and guaiacol peroxidase (GPX) in the leaves of naked oat (Avena nuda L.) cultivar Bayou1 soil grown with nutrient solution with 0 (control), 50, and 100 mM NaCl for 12 days.
3.1.2 Water uptake capacity, and Mg2+ concentrations in the leaves of Bayou1 in soil experiment
The RWC in leaves was determined to investigate the water status of oat seedlings under salinity. When compared to control plants, RWC in the leaves was unaffected at 50 mM NaCl and considerably decreased (13%) at 100 mM NaCl (Figure 3). The Mg2+ in leaves was measured to examine the nutrient deficiency induced by salinity. The Mg2+ concentration in the leaves decreased by 13% at 100 mM NaCl, compared to 6% at 50 mM NaCl (), and by 0.15 mg g−1 DW (Table 1).
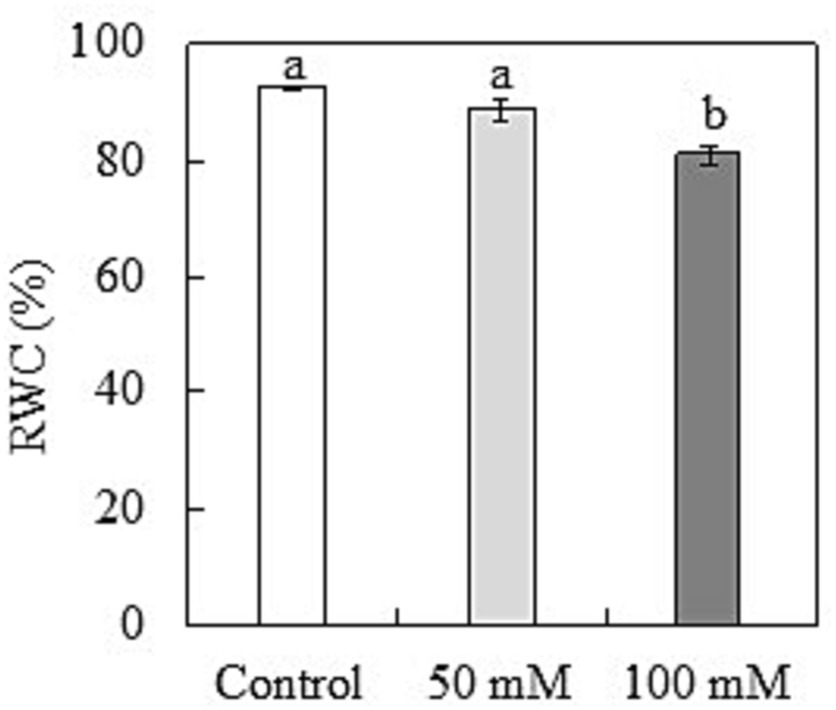
Figure 3. Relative water content (RWC) in the leaves of naked oat (Avena nuda L.) line Bayou1 under control conditions and 12 days of soil salinity. Values are means of four replicates ± standard error. Different lower-case letters indicate significant differences among treatments at p ≤ 0.05.
3.1.3 Na+ and K+ homeostasis in the different plant organs of Bayou1 in soil experiment
Ionic homeostasis in plant tissues is frequently disrupted as a result of the accumulation of excess Na+ and depletion of other ions. When compared to controls, NaCl significantly increased Na+ assimilation in all plant organs examined (Figure 4A). With an increase in salinity from 50 to 100 mM NaCl, leaf and root Na+ concentrations did not differ significantly; Na+ concentrations in the sheaths increased from 50 mM to 100 mM as NaCl. Compared to the controls, NaCl significantly decreased K+ concentrations in the leaves, sheaths, and roots (Figure 4B). The K+ concentration in the leaves and roots was reduced by 61% and 48% more at 100 mM than at 50 mM NaCl (51% and 16%). The K+ concentration in the sheaths was reduced by 59% and 60% at 50 mM and 100 mM NaCl, respectively. All NaCl treatments increased the Na+/K+ ratio in the leaves, sheaths, and roots comparable to the control, with the highest ratios recorded at 100 mM (Figure 4C).
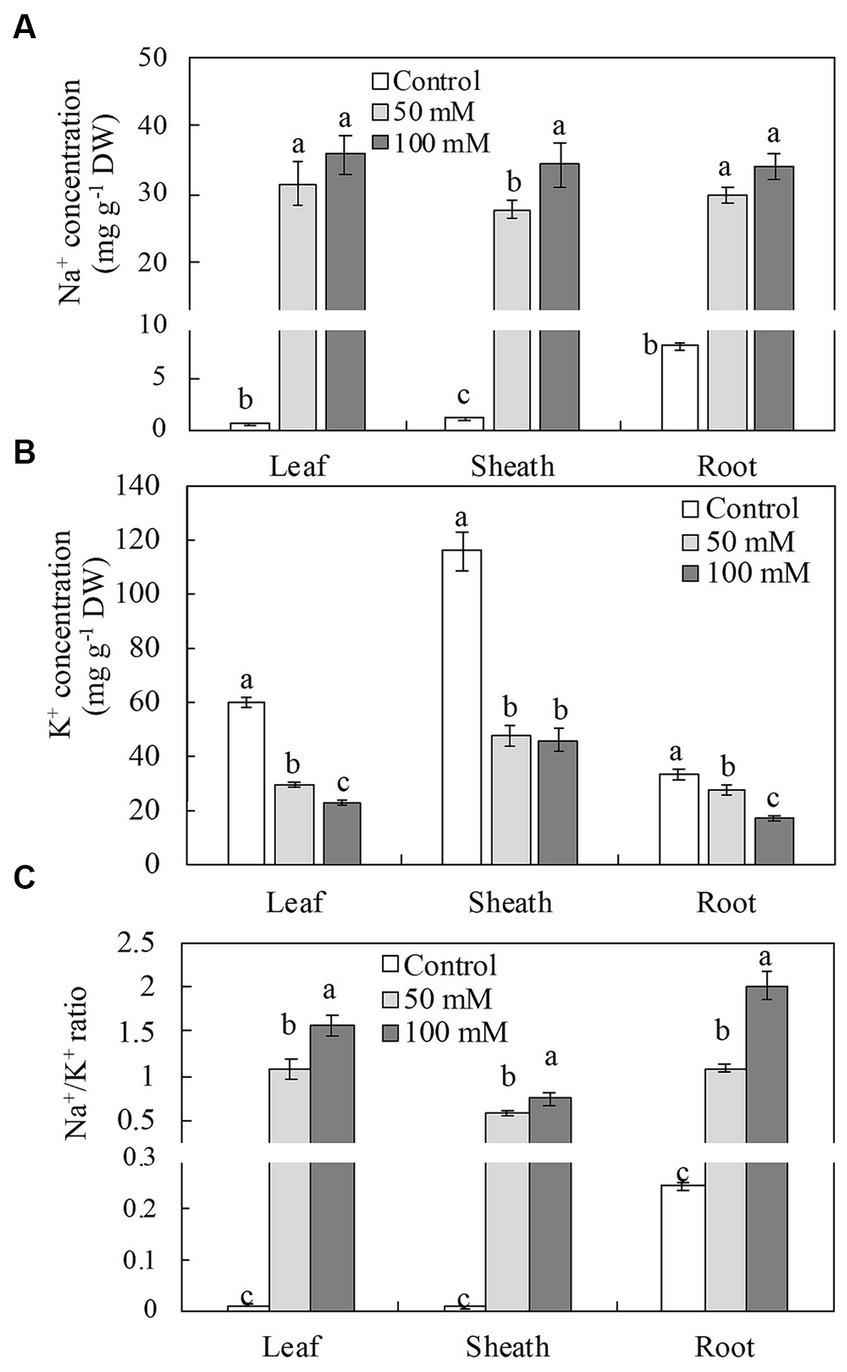
Figure 4. Concentrations of Na+, (A) K+ (B) and Na+/K+ ratio (C) in leaves, sheathes, and roots of naked oat (Avena nuda L.) line Bayou1 under control conditions and 12 days of soil salinity. Values are means of four replicates ± standard error. Different lower-case letters indicate significant differences among treatments at p ≤ 0.05.
3.1.4 Effect of NaCl on the parameters related to cell membrane integrity in the leaves of Bayou1
The MDA concentration was measured to determine leaf damage under salinity (Figure 5A). MDA concentrations in the leaves increased significantly with increasing NaCl concentrations, with the highest level measured at 100 mM NaCl. However, there were no significant differences between 50 mM and 100 mM NaCl in terms of MDA concentration. Regulates A number of cell membrane functions that are associated with cell membrane integrity are regulated by Ca2+. The Ca2+ concentration in the leaves decreased by 36% and 46% at 50 mM and 100 mM NaCl, respectively, with no significant difference between the two concentrations (Figure 5B). To investigate the antioxidative capacity of oat seedlings to salinity, antioxidant enzyme activities were measured. The activities of CAT and GR in the leaves were not affected at either by 50 mM or 100 mM NaCl. However, 50 mM NaCl had no effect on GPX activity, whereas 100 mM NaCl reduced it significantly (62%) (Table 1).
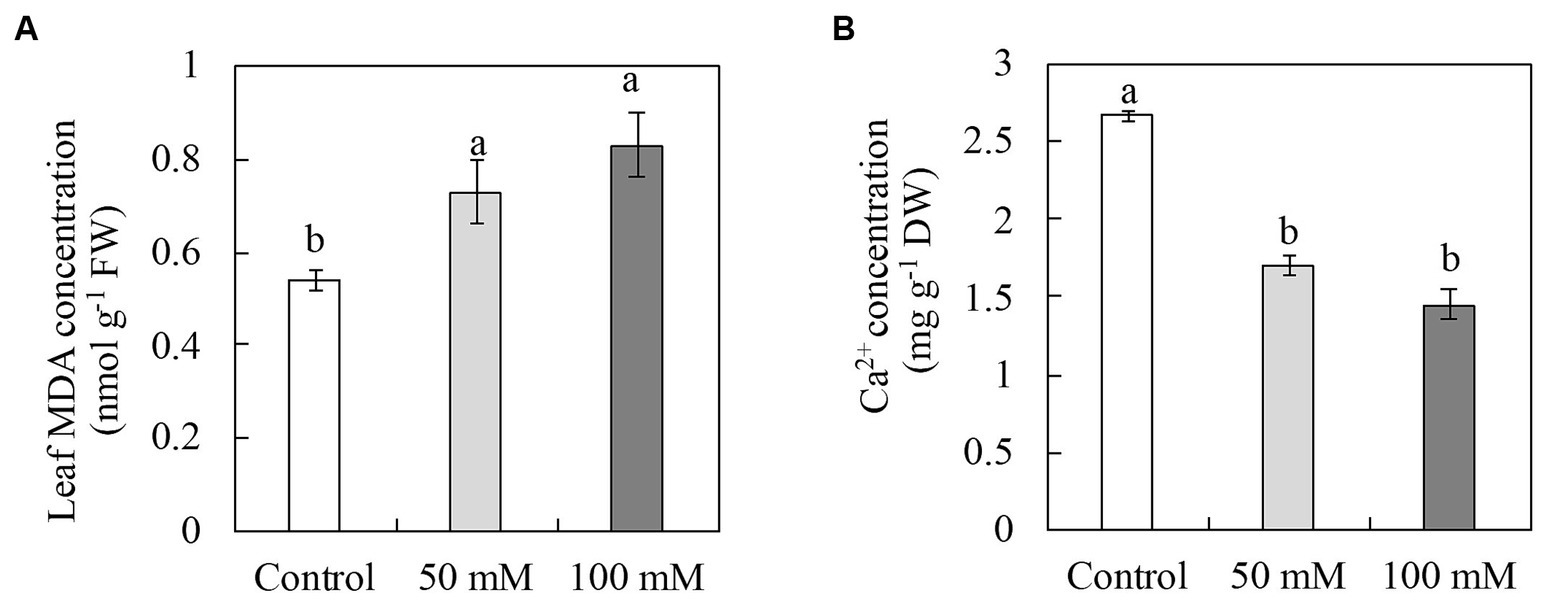
Figure 5. Concentrations of malondialdehyde (MDA) (A) and Ca2+ (B) in the leaves of naked oat (Avena nuda L.) line Bayou1 soil grown with nutrient solution with 0 (control), 50, and 100 mM NaCl for 12 days.
3.2 Experiment 2
3.2.1 Effect of NaCl on growth biomass of whole seedlings, and water cell membrane integrity, uptake ability, and role of Na+ in the roots of Bayou1
The shoot FW increased by 15% at 25 mM and was unaffected at 50 mM NaCl, while the root FW increased by 22% at 25 mM and 21% at 50 mM NaCl (Table 2). MDA concentration was used to assess root damage (Figure 6A), while-naphthylamine-oxidizing activity was used to evaluate root vigor (Table 2). In comparison to the control plants, neither 25 mM nor 50 mM NaCl had any influence on MDA concentrations or root vigor (Figure 6A and Table 2).
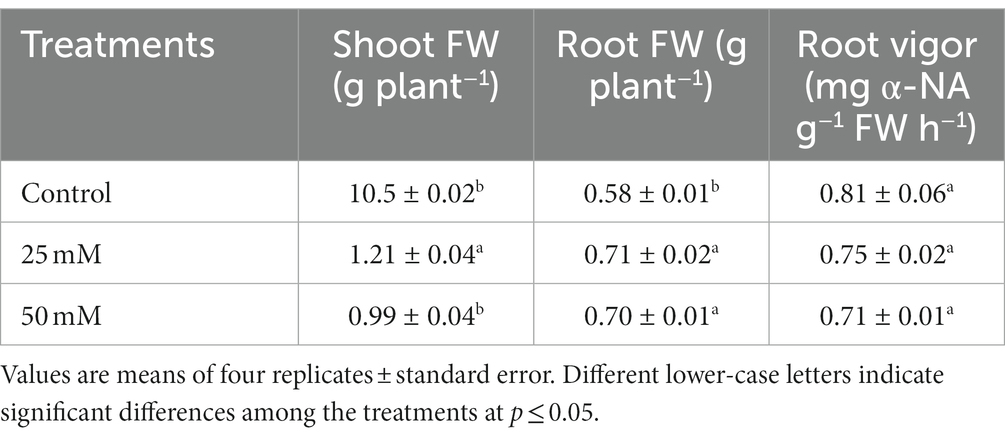
Table 2. Fresh weight (FW) in the shoot, root FW and root vigor in the roots of naked oat (Avena nuda L.) cultivar Bayou1 hydroponically grown with nutrient solution with 0 (control), 25, and 50 mM NaCl for 6 days.
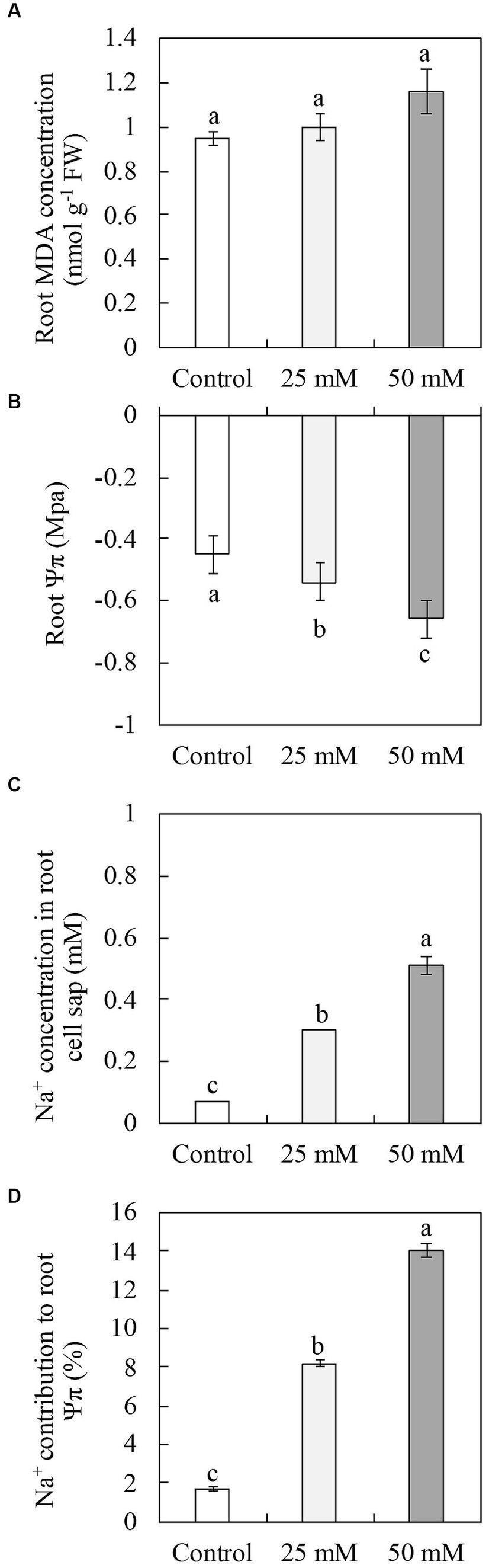
Figure 6. Malondialdehyde (MDA) concentration (A), osmotic potential (Ψπ) (B), Na+ concentration in cell sap (C), and Na+ contribution to Ψπ (D) in the roots of naked oat (Avena nuda L.) line Bayou1 hydroponically grown with nutrient solution with 0 (control), 25, and 50 mM NaCl for 6 days.
Due to osmotic stress, the assimilation of Na+ frequently causes water deficiency in plant tissues. To determine the effect of NaCl on water loss in Bayou1 roots, the root Ψπ was measured (Figure 6B). In comparison to the controls, the highest root Ψπ was observed at control, followed by 25 and 50 mM NaCl. Compared to the control plants, 25 mM and 50 mM NaCl increased Na+ concentrations in root cell sap by 428 and 728 times, respectively (Figure 6C). To investigate the possibility of Na+ sequestration in the vacuole of root cell vacuoles, the Na+ contribution to root Ψπ was determined. In comparison to the control plants, it increased at 25 mM (4.8-fold) and 50 mM NaCl (8.3-fold) (Figure 6D).
3.3 Correlation coefficient
The correlation coefficient of root biomass and other root characteristics in Bayou1 was significantly influenced by NaCl (Figure 7). The positive correlation between root biomass (DW-S and FW-H) and Na+ concentration (Na-S and Na-H) in both soil and hydroponic experiments, as well as the positive correlation between the Na+ contribution to root Ψπ (ΨNa/Ψπ-H) and FW-H, suggested that Na+ has a beneficial effect on root growth. The negative correlation between K+ concentration (K-S), root vigor (vigor-H), Ψπ-H, and root biomass indicated that reduced K+, root vigor, and Ψπ had no effect on root growth. The cell membrane integrity of roots was highlighted as the positive correlation between the MDA-H and FW-H.
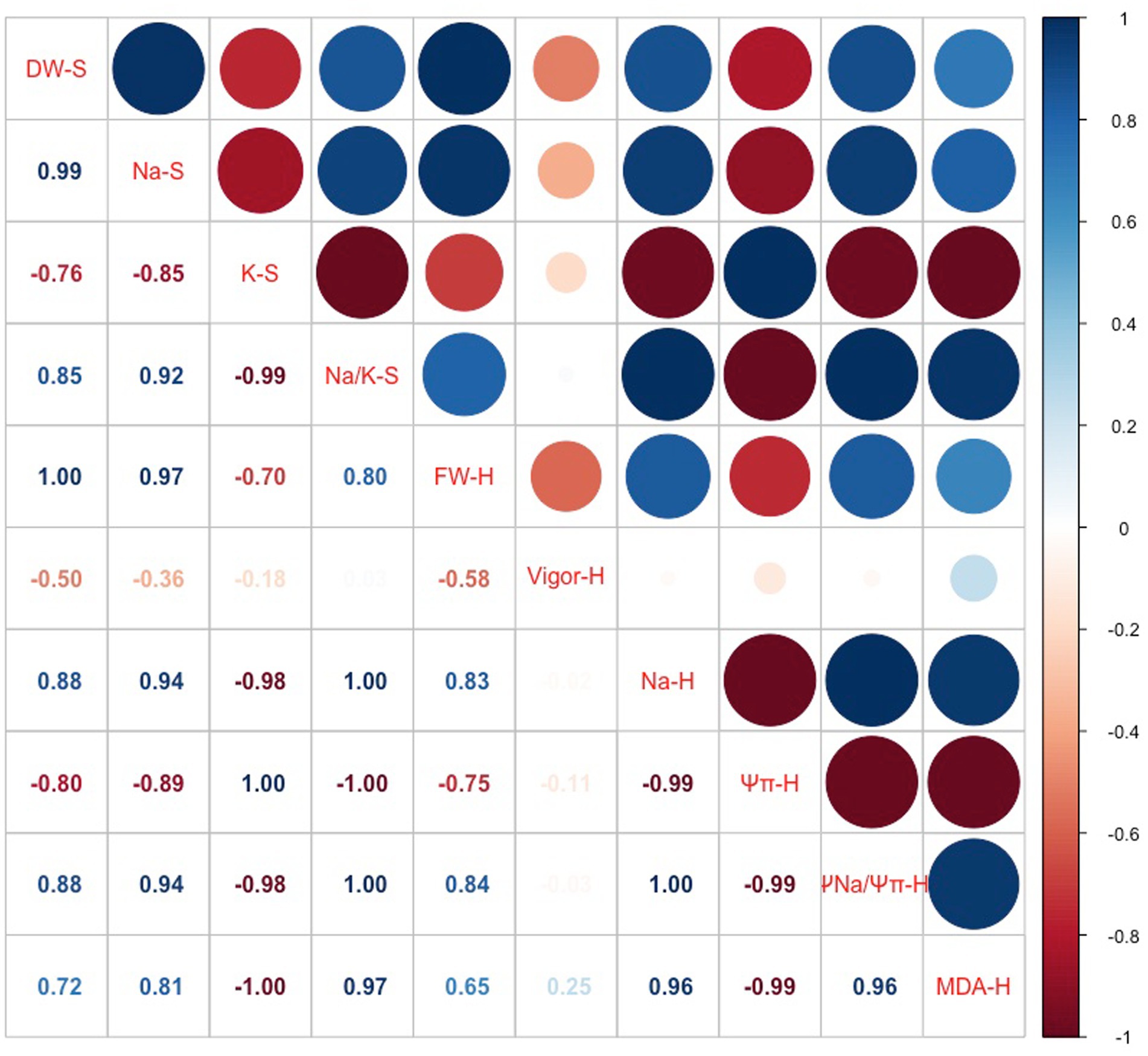
Figure 7. Correlation coefficients of root dry weight (DW-S) for soil experiment (S) and root fresh weight (FW-H) for hydroponic (H) system with Na+ concentration (Na-S), K+ concentration (K-S), and Na+/K+ ratio (Na/K-S) in the root of Bayou1 at different concentration of NaCl under soil experiment, and root vigor (vigor-H), Na+ concentration in cell sap (Na-H), osmotic potential (Ψπ-H), Na+ contribution to root Ψπ (ΨNa/Ψπ-H), and malondialdehyde (MDA-H) in the root of Bayou1 at different concentration of NaCl under hydroponic system.
4 Discussion
4.1 Greater Na+ allocation to the shoots under high salinity may be related to shoot reduction in Bayou 1
Salinity significantly decreases naked oat production, and earlier research investigated the short-term salinity tolerant mechanism in naked oats at the protein level (Chen et al., 2022). The short-term salinity tolerance mechanism in Bayou1 was determined at the physiological level in this investigation. Among plant salinity tolerance mechanisms, the exclusion of Na+ from the shoot, i.e., the ability to restrict Na+ transport and accumulation in the shoot, is of most importance, particularly in glycophytes (Munns and Tester, 2008; Assaha et al., 2017b; Wangsawang et al., 2018). The restriction of Na+ allocation to young leaves is critical to Na+ detoxification at the whole-plant level (Munns, 2002). This study found that low salinity had a positive effect on the growth and development of this line since Bayou1 did not appear to have any control over Na+ allocation to the leaves and sheaths. Furthermore, this delivery of Na+ coincided with improved growths of leaves and sheaths at 50 mM in the soil experiment (Figures 2, 4A). Mild salt stress improves the production and nutritional quality of several vegetable species (Rouphael and Kyriacou, 2018), which supports the observation of greater DW in leaves and sheaths in this study (Figure 2). In spinach, 50 mM NaCl enhances total yield, branch number, and antioxidant activity (Sogoni et al., 2021). It is possible that some of the Na+ supplied to the leaves and sheaths from roots was detoxified by some mechanisms at 50 mM NaCl. However, increasing the salinity to 100 mM NaCl inhibited the growth of leaves and sheaths due to increased Na+ buildup (Figures 2, 4A). Root Na+ retention mechanisms are typically activated at this time, however they appeared inefficient, as Na+ concentrations in roots levelled off with Na+ concentrations in leaves and sheaths (Figure 4A). It is possible that the high Na+/K+ ratio, particularly in the leaves, caused significant damage to leaf tissues and impeded cellular activities using K+ because the increase in shoot Na+ coincided with a decrease in shoot K+ (Munns and Tester, 2008). Therefore, the reduction in shoot growth may also be significantly influenced by the decrease in shoot K+ caused by high salt levels. However, the reduction in root growth was not related to the decrease in root K+ and an increase in root Na+ and the root Na+/K+ ratio (Figures 2, 4). It was hypothesized that increased Na+ in roots would be compartmentalized into the vacuole rather than K+ by NHX1 transporter, allowing roots to retain their K+-protective functions in the cytosol. This maintenance of K+-protective functions in root cytosol may aid in growth of roots under salinity. This effect is also demonstrated by the transformation of a barley (Hordeum vulgare) VP gene, HVP10, into rice (Fu et al., 2022). Transgenic rice overexpressing HVP10 exhibits greater salt tolerance than wild type, as well as Na+ sequestration in the root vacuole and K+ maintenance in roots (Fu et al., 2022).
4.2 NaCl treatments induce oxidative stress in the leaves of soil experiment
Under salinity, Na+ outside of the cytosol causes oxidative stress, resulting in the production of MDA, which is the final product of peroxidation and alters cell membrane integrity (Gill and Tuteja, 2010). Due to the application of NaCl, increased MDA concentrations were observed in the leaves of Bayou1 (Figure 5A), indicating the presence of oxidative stress in the leaves. According to the findings, decreased water status (Figure 3) and Mg2+ concentration (Table 1), as well as increased Na+ assimilation caused by NaCl may reduce photosynthetic capacity and, consequently, decrease photosynthetic electron transport activity, resulting in unfavorable dissipation of surplus light energy and overproduction of reactive oxygen species (ROS). Overproduction of ROS such as hydrogen peroxide (H2O2) in chloroplast photosystem II (PSII) induces oxidative stress in Bayou1 leaves. Plants typically utilize antioxidative enzymes such as CAT, GR, and GPX for ROS neutralization to combat oxidative stress and prevent membrane peroxidation (Couto et al., 2016; Huang et al., 2019). SOD protects against ROS-induced damage by catalyzing the conversion of O2− to H2O2, which is further catalyzed by CAT, GPX, and other peroxidases (Couto et al., 2016; Huang et al., 2019). In this instance, the maintenance of CAT activity maintained the decomposition of H2O2 to water and oxygen, thereby protecting the cells in the leaves from oxidative stress caused by H2O2. The maintenance of GR activity ensured the production of reduced glutathione, a key cellular antioxidant, and thus the protection of leaf cells against oxidative damage. However, a reduction in GPX activity decreased the capacity of the cell to eliminate excess H2O2 and had no effect on the cell’s ability to withstand oxidative stress induced by H2O2 in the leaves. Meanwhile, Ca2+ can maintain membrane integrity, resulting in a reduction of MDA concentration under abiotic stress (Li et al., 2020); thus, the reduction of Ca2+ concentration (Figure 5B) may primarily weaken the permeability of cell membrane and damage the cell membrane integrity of Bayou1 leaves.
Likewise, higher shoot FW was observed at 25 mM NaCl and was maintained at 50 mM NaCl compared to the control plants in the hydroponic system (Table 2). However, the root FW increased at both 25 mM and 50 mM NaCl (Table 2), indicating that the roots are tolerant to salinity. Further analysis revealed the same MDA concentrations in the roots regardless of salinity treatment (Figure 6A), as well as a consistently increasing Na+ concentration in root cell sap (Figure 6C), indicating that increased Na+ concentrations in roots help maintain cell membrane integrity rather than diminish it. Moreover, root vigor is a comprehensive indicator of the number of living cells and their metabolic activity in roots; consequently, root vigor is a crucial attribute for the growth of terrestrial plants under environmental stress (Kajikawa et al., 2010; Zhang et al., 2019). The decrease in root vigor is associated with a decrease in root cell metabolism and the number of living root cells (Zhang et al., 2019). Here, the maintenance of root vigor (Table 2) suggests that the roots have maintained their capacity to adapt to either low or high salinity. Together, increased Na+ concentrations did not affect the cell metabolic mechanisms in the roots of Bayou1 and may play a significant role in hydroponic root cell division and expansion.
4.3 Bayou1 uses Na+ in the roots as an osmolyte to osmotically adjust to salt stress
Low water potential in the root environment causes water loss through the roots when exposed to high salinity; therefore, plants require osmolytes to continue water absorption and maintain positive turgor and root cell growth (Puniran-Hartley et al., 2014). Among the various osmolytes, soluble organic osmolytes are essential for achieving this goal. However, organic osmolytes biosynthesis is an energy-demanding process (Munns et al., 2020), making inorganic osmolytes a more cost-effective option to organic osmolytes in the short term (Puniran-Hartley et al., 2014). Numerous plants, including halophytes, withstand salinity by sequestering Na+ in vacuoles to prevent its deleterious effects on the cytosol (Flowers et al., 2015). Here, Na+ concentrations in roots of Bayou1 steadily increased with increasing salinity, without any growth restriction in the roots (Figures 2, 4A, 6C and Table 2), indicating that Na+ accumulation was harmless to the roots as the stress increased from 50 to 100 mM NaCl in soil experiment or from 25 mM to 50 mM in hydroponic system. It is possible that sequestered Na+ into vacuoles as an inorganic osmolyte supported cell expansion in roots and lateral root development. Similarly, the salinity tolerance of Talinum paniculatum is predominantly due to its ability to retain Na+ in the roots without inhibiting root growth in both low and high salinity conditions (Assaha et al., 2017a). Here, the progressively increasing root Na+ concentrations limited the transport of this toxic ion from roots to shoots (Figure 4A), thereby partially preventing Na+ toxicity in the shoots in the soil experiment and hydroponic system.
Certain physiological mechanisms prevent the deleterious effects of Na+ on the roots of Bayou1 as evidenced by the fact that root DW was maintained in the soil experiment while root FW increased in the hydroponic system. To confirm these mechanisms, further research was conducted. Analyses demonstrated that osmotic adjustment was crucial and that the role of Na+ was extremely essential, as Na+ significantly contributed to the root Ψπ (Figures 6B,D). These findings suggest that Bayou1 has evolved mechanisms for the selective sequestration of Na+ into its root cell vacuoles for osmotic adjustment. This Na+ sequestration enables the cytoplasm to sustain significantly lower Na+ concentrations, thereby maintaining optimal cytosolic K+ required for normal metabolic processes in the roots of Bayou1 under conditions of salt stress. The ionic homeostasis between Na+ and K+ in the cytosol contributed to the maintenance of normal molecular functions in root cells, as well as root vigor and growth. Therefore, in the present investigation, it was safe to predict that the root Na+ in the cell sap was highest under 50 mM NaCl stress (Figure 6C), with a greater proportion of this Na+ sequestered in the vacuoles in the hydroponic system. This physiological mechanism can also be demonstrated by the significant correlation between Na+ concentration and root DW in the soil experiment and the positive correlation between Na+ concentration in root cell sap and root FW in the hydroponic system (Figure 7).
5 Conclusion
Despite a reduction in shoot growth under high NaCl stress due to decreased water absorption capacity, Ca2+ and Mg2+ concentrations in the leaves, Bayou1 appeared to tolerate the salinity. The optimal plant growth of Bayou1 was found under low NaCl stress in either soil experiment or hydroponic system, rather than at control, implying the existence of salinity tolerant mechanisms in Bayou1. The novel discovery was the maintenance of root growth in a soil experiment (100 mM) and enhanced root FW in a hydroponic system at the highest NaCl concentration (50 mM). As a result, Bayou1’s enhanced salinity tolerance is dependent on mechanisms that occur mostly inside the roots. Further investigation revealed that one of these processes is osmotic adjustment caused by Na+ as the principal osmolyte in the roots, with a positive association between root Na+ and root biomass in both the soil experiment and the hydroponic system. As a result, Bayou1 has a high tolerance to salinity and the ability to sequester Na+ in root cell vacuoles. This method of Na+ sequestration in the roots may be required to prevent Na+ translocation to the shoots. This study provided the initial evidence demonstrating the salt tolerance of Bayou1, as indicated by the continued growth of its root system under conditions of low salinity.
Data availability statement
The datasets presented in this study can be found in online repositories. The names of the repository/repositories and accession number(s) can be found in the article/supplementary material.
Author contributions
LL: Conceptualization, Data curation, Formal analysis, Investigation, Methodology, Project administration, Resources, Software, Writing – original draft, Writing – review & editing. DVMA: Writing – review & editing, Software, Writing – original draft. MSI: Writing – review & editing, Conceptualization, Data curation, Formal analysis. KR: Software, Writing – review & editing. PT: Formal analysis, Writing – review & editing. WS: Funding acquisition, Resources, Software, Writing – review & editing. AES: Conceptualization, Funding acquisition, Resources, Writing – original draft, Writing – review & editing. AU: Conceptualization, Supervision, Writing – original draft, Writing – review & editing.
Funding
The author(s) declare financial support was received for the research, authorship, and/or publication of this article. This research was funded by the Researchers Supporting Project number (RSP2024R390) at King Saud University, Riyadh, Saudi Arabia.
Acknowledgments
All the authors are thankful to Researchers Supporting Project number (RSP2024R390), King Saud University, Riyadh, Saudi Arabia. The authors greatly appreciate the College of Grassland, Resources and Environment, Inner Mongolia Agricultural University, Inner Mongolia, China for providing the seeds of Bayou1.
Conflict of interest
The authors declare that the research was conducted in the absence of any commercial or financial relationships that could be construed as a potential conflict of interest.
Publisher’s note
All claims expressed in this article are solely those of the authors and do not necessarily represent those of their affiliated organizations, or those of the publisher, the editors and the reviewers. Any product that may be evaluated in this article, or claim that may be made by its manufacturer, is not guaranteed or endorsed by the publisher.
References
Aebi, H. (1984). Catalase in vitro. Methods Enzymol. 105, 121–126. doi: 10.1016/S0076-6879(84)05016-3
Almeida, D. M., Oliveira, M. M., and Saibo, N. J. M. (2017). Regulation of Na+ and K+ homeostasis in plants: towards improved salt stress tolerance in crop plants. Genet. Mol. Biol. 40, 326–345. doi: 10.1590/1678-4685-GMB-2016-0106
Apse, M. P., and Blumwald, E. (2007). Na+ transport in plants. FEBS Lett. 581, 2247–2254. doi: 10.1016/j.febslet.2007.04.014
Assaha, D. V. M., Mekawy, A. M. M., Liu, L., Noori, S., Kokulan, K. S., Ueda, A., et al. (2017a). Na+ retention in the root is a key adaptive mechanism to low and high salinity in the glycophyte, Talinum paniculatum (Jacq.) Gaertn. (Portulacaceae). J. Agron. Crop Sci. 203, 56–67. doi: 10.1111/jac.12184
Assaha, D. V. M., Ueda, A., Saneoka, H., Al-Yahyai, R., and Yaish, M. W. (2017b). The role of Na+ and K+ transporters in salt stress adaptation in glycophytes. Front. Physiol. 8:509. doi: 10.3389/fphys.2017.00509
Bai, J., Yan, W., Wang, Y., Yin, Q., Liu, J., Wight, C., et al. (2018). Screening oat genotypes for tolerance to salinity and alkalinity. Front. Plant Sci. 9:1302. doi: 10.3389/fpls.2018.01302
Boczkowska, M., and Tarczyk, E. (2013). Genetic diversity among Polish landraces of common oat (Avena sativa L.). Genet. Resour. Crop Evol. 60, 2157–2169. doi: 10.1007/s10722-013-9984-1
Carlson, A., and Kaeppler, H. F. (2007). “Oat” in Transgenic crops IV: biotechnology in agriculture and forestry. eds. E. C. Pua and M. R. Davey (Berlin: Springer). 59, 151–160. doi: 10.1007/978-3-540-36752-9_8
Chen, X., Xu, Z., Zhao, B., Yang, Y., Mi, J., Zhao, Z., et al. (2022). Physiological and proteomic analysis responsive mechanisms for salt stress in oat. Front. Plant Sci. 13:891674. doi: 10.3389/fpls.2022.891674
Couto, N., Wood, J., and Barber, J. (2016). The role of glutathione reductase and related enzymes on cellular redox homoeostasis network. Free Radic. Biol. Med. 95, 27–42. doi: 10.1016/j.freeradbiomed.2016.02.028
Daou, C., and Zhang, H. (2012). Oat beta-glucan: its role in health promotion and prevention of diseases. Compr. Rev. Food Sci. Food Saf. 11, 355–365. doi: 10.1111/j.1541-4337.2012.00189.x
Diao, X. (2017). Production and genetic improvement of minor cereal in China. Crop J. 5, 103–114. doi: 10.1016/j.cj.2016.06.004
Draper, H. H., and Hadley, M. (1990). Malondialdehyde determination as index of lipid peroxidation. Methods Enzymol. 186, 421–431. doi: 10.1016/0076-6879(90)86135-I
Flowers, T. J., Munns, R., and Colmer, T. D. (2015). Sodium chloride toxicity and the cellular basis of salt tolerance in halophytes. Ann. Bot. 115, 419–431. doi: 10.1093/aob/mcu217
Foyer, C. H., and Halliwell, B. (1976). The presence of glutathione and glutathione reductase in chloroplasts: a proposed role in ascorbic acid metabolism. Planta 133, 21–25. doi: 10.1007/BF00386001
Fu, L., Wu, D., Zhang, X., Xu, Y., Kuang, L., Cai, S., et al. (2022). Vacuolar H+-pyrophosphatase HVP10 enhances salt tolerance via promoting Na+ translocation into root vacuoles. Plant Physiol. 188, 1248–1263. doi: 10.1093/plphys/kiab538
Gill, S. S., and Tuteja, N. (2010). Reactive oxygen species and antioxidant machinery in abiotic stress tolerance in crop plants. Plant Physiol. Biochem. 48, 909–930. doi: 10.1016/j.plaphy.2010.08.016
Gupta, B., and Huang, B. (2014). Mechanism of salinity tolerance in plants: physiological, biochemical, and molecular characterization. Int. J. Genomics 2014:701596. doi: 10.1155/2014/701596
Hariadi, Y., Marandon, K., Tian, Y., Jacobsen, S. E., and Shabala, S. (2011). Ionic and osmotic relations in quinoa (Chenopodium quinoa Willd.) plants grown at various salinity levels. J. Environ. Bot. 62, 185–193. doi: 10.1093/jxb/erq257
Huang, H., Ullah, F., Zhou, D. X., Yi, M., and Zhao, Y. (2019). Mechanisms of ROS regulation of plant development and stress responses. Front. Plant Sci. 10:800. doi: 10.3389/fpls.2019.00800
Kajikawa, M., Morikawa, K., Abe, Y., Yokota, A., and Akashi, K. (2010). Establishment of a transgenic hairy root system in wild and domesticated watermelon (Citrullus lanatus) for studying root vigor under drought. Plant Cell Rep. 29, 771–778. doi: 10.1007/s00299-010-0863-3
Keutgen, A., and Pawelzik, E. (2009). Impacts of NaCl stress on plant growth and mineral nutrient assimilation in two cultivars of strawberry. Environ. Exp. Bot. 65, 170–176. doi: 10.1016/j.envexpbot.2008.08.002
Li, Z., Wang, L., Xie, B., Hu, S., Zheng, Y., and Jin, P. (2020). Effects of exogenous calcium and calcium chelant on cold tolerance of postharvest loquat fruit. Sci. Hortic. 269:109391. doi: 10.1016/j.scienta.2020.109391
Liu, L., El-Shemy, H. A., and Saneoka, H. (2017). Effects of 5-aminolevulinic acid on water uptake, ionic toxicity, and antioxidant capacity of Swiss chard (Beta vulgaris L.) under sodic-alkaline conditions. J. Plant Nutr. Soil Sci. 180, 535–543. doi: 10.1002/jpln.201700059
Liu, L., Han, G., Nagaoka, T., and Saneoka, H. (2020a). A comparative study of the growth and physiological parameters of two oat (Avena sativa L.) lines under salinity stress. Soil Sci. Plant Nutr. 66, 847–853. doi: 10.1080/00380768.2020.1820756
Liu, L., Petchphankul, N., Ueda, A., and Saneoka, H. (2020b). Differences in physiological response of two oat (Avena nuda L.) lines to sodic-alkalinity in the vegetative stage. Plan. Theory 9:1188. doi: 10.3390/plants9091188
Liu, L., and Saneoka, H. (2022). Physiological changes and subsequent recovery in seedlings of two lines of oat (Avena nuda L.) in response to salinity. J. Soil. Sci. Plant Nutr. 22, 3280–3290. doi: 10.1007/s42729-022-00886-w
Lv, S., Jiang, P., Chen, X., Fan, P., Wang, X., and Li, Y. (2012). Multiple compartmentalization of sodium conferred salt tolerance in Salicornia europaea. Plant Physiol. Biochem. 51, 47–52. doi: 10.1016/j.plaphy.2011.10.015
Martínez-Villaluenga, C., and Peñas, E. (2017). Health benefits of oat: current evidence and molecular mechanisms. Curr. Opin. Food Sci. 14, 26–31. doi: 10.1016/j.cofs.2017.01.004
Mekawy, A. M. M., Assaha, D. V. M., Yahagi, H., Tada, Y., Ueda, A., and Saneoka, H. (2015). Growth, physiological adaptation, and gene expression analysis of two Egyptian rice cultivars under salt stress. Plant Physiol. Biochem. 87, 17–25. doi: 10.1016/j.plaphy.2014.12.007
Munns, R. (2002). Comparative physiology of salt and water stress. Plant Cell Environ. 25, 239–250. doi: 10.1046/j.0016-8025.2001.00799.x
Munns, R., Passioura, J. B., Colmer, T. D., and Byrt, C. S. (2020). Osmotic adjustment and energy limitation to plant growth in saline soil. New Phytol. 225, 1091–1096. doi: 10.1111/nph.15862
Munns, R., and Tester, M. (2008). Mechanisms of salinity tolerance. Annu. Rev. Plant Biol. 59, 651–681. doi: 10.1146/annurev.arplant.59.032607.092911
Negrão, S., Schmöckel, S. M., and Tester, M. (2017). Evaluating physiological responses of plants to salinity stress. Ann. Bot. 119, 1–11. doi: 10.1093/aob/mcw191
Puniran-Hartley, J., Hartley, N., Shabala, L., and Shabala, S. (2014). Salinity-induced accumulation of organic osmolytes in barley and wheat leaves correlates with increased oxidative stress tolerance: in planta evidence for cross-tolerance. Plant Physiol. Biochem. 83, 32–39. doi: 10.1016/j.plaphy.2014.07.005
Rouphael, Y., and Kyriacou, M. C. (2018). Enhancing quality of fresh vegetables through salinity eustress and biofortification application facilitated by soilless cultivation. Front. Plant Sci. 9:1254. doi: 10.3389/fpls.2018.01254
Sogoni, A., Jimoh, M. O., Kambizi, L., and Laubscher, C. P. (2021). The impact of salt stress on plant growth, mineral composition, and antioxidant activity in Tetragonia decumbens mill.: an underutilized edible halophyte in South Africa. Hort. 7:140. doi: 10.3390/horticulturae7060140
Tang, M., Wang, L., Cheng, X., Wu, Y., and Ouyang, J. (2019). Non-starch constituents influences the in vitro digestibility of naked oat (Avena nuda L.) starch. Food Chem. 297:124953. doi: 10.1016/j.foodchem.2019.124953
Velikova, V., Yordanov, I., and Edreva, A. (2000). Oxidative stress and some antioxidant systems in acid rain-treated bean plants: protective role of exogenous polyamines. Plant Sci. 151, 59–66. doi: 10.1016/S0168-9452(99)00197-1
Wang, C. F., Han, G. L., Yang, Z. R., Li, Y. X., and Wang, B. S. (2022). Plant salinity sensors: current understanding and future directions. Front. Plant Sci. 13:859224. doi: 10.3389/fpls.2022.859224
Wangsawang, T., Chuamnakthong, S., Kohnishi, E., Sripichitt, P., Sreewongchai, T., and Ueda, A. (2018). A salinity-tolerant japonica cultivar has Na+ exclusion mechanism at leaf sheaths through the function of a Na+ transporter OsHKT1; 4 under salinity stress. J. Agro. Crop. Sci. 204, 274–284. doi: 10.1111/jac.12264
Zhang, X., Liu, W., Lv, Y., Li, T., Tang, J., Yang, X., et al. (2022). Effects of drought stress during critical periods on the photosynthetic characteristics and production performance of naked oat (Avena nuda L.). Sci. Rep. 12:11199. doi: 10.1038/s41598-022-15322-3
Zhang, H., Liu, X. L., Zhang, R. X., Yuan, H. Y., Wang, M. M., Yang, H. Y., et al. (2017). Root damage under alkaline stress is associated with reactive oxygen species accumulation in rice (Oryza sativa L.). Front. Plant Sci. 8:1580. doi: 10.3389/fpls.2017.01580
Keywords: antioxidant enzymes, ionic homeostasis, relative water content, root growth, salinity tolerance
Citation: Liu L, Assaha DVM, Islam MS, Rajendran K, Theivasigamani P, Soufan W, Ayman ES and Ueda A (2024) Effect of NaCl on physiological, biochemical, and ionic parameters of naked oat (Avena nuda L.) line Bayou1. Front. Sustain. Food Syst. 7:1336350. doi: 10.3389/fsufs.2023.1336350
Edited by:
Md. Khairul Alam, Bangladesh Agricultural Research Institute, BangladeshReviewed by:
Michela Janni, Institute of Materials for Electronics and Magnetism National Research Council, ItalyOli Fakir, Bangladesh Agricultural Research Institute, Bangladesh
Copyright © 2024 Liu, Assaha, Islam, Rajendran, Theivasigamani, Soufan, Ayman and Ueda. This is an open-access article distributed under the terms of the Creative Commons Attribution License (CC BY). The use, distribution or reproduction in other forums is permitted, provided the original author(s) and the copyright owner(s) are credited and that the original publication in this journal is cited, in accordance with accepted academic practice. No use, distribution or reproduction is permitted which does not comply with these terms.
*Correspondence: Liyun Liu, bGl1bGl5dW43OTQxOUAxNjMuY29t; Mohammad Sohidul Islam, YWcuaXNsYW0uYWduQHRjaC5oc3R1LmFjLmJk
†Present address: Liyun Liu, Graduate School of Engineering, Osaka University, Suita, Japan