- Oberlin College Environmental Studies Program, Oberlin, OH, United States
Positive relationships have been documented between the amount of biochar added to soils and various aspects of plant growth and fertility such as root, shoot, and fruit production. However, these effects depend on biochar source materials, soil characteristics and species of plant examined. This makes it impossible to systematically compare and generalize findings across previous studies that have used different soils and biochar. We conducted a novel investigation to assess the effects of a single source of biochar (hazelnut wood), in a constructed organic soil, on the different plant tissues in three functionally distinct species: tomatoes (Solanum lycopersicon), green beans (Phaseolus vulgaris), and willow (Salix sp.). Five levels of biochar soil amendment were assessed: 0% (control), 3, 9, and 26% by dry weight. We found a highly significant positive relationship between biochar concentration and total plant biomass (roots + shoots + fruits) in all species, with no significant difference in total biomass response among species. Fruit production increased with increased biochar in both beans and tomatoes. However, tomatoes exhibited significant differences in response among plant tissues; fruit production and shoot biomass increased significantly with biochar, but root tissue did not. Bean germination success increased significantly with biochar concentration. Date of first flowering was earlier with increasing soil biochar in beans but not in tomatoes. Control over both sources of biochar and soil composition in this experiment enables us to conclude that biochar addition can have different impacts on different plants and, in some cases, species-specific impacts on different plant tissues and other measures of fertility. Our results are contrary to prior research that found inhibiting effects of biochar at levels comparable to our 26% treatment. Biochar impacts on soil properties such as CEC and percent base cation saturation do not explain our findings, leading us to conclude that microbial interaction with biochar is an important factor that may explain the positive impacts of soil biochar on plant fertility observed. Further research that repeats this experiment in other soil types, with other biochar sources, and with other plant species is necessary to determine the generalizability of these important findings.
1 Introduction
Biochar is promoted as a soil amendment that can concurrently sequester carbon to address climate change and increase soil fertility, thereby addressing the food needs and of a growing population. In regards to soil fertility, biochar has generally been shown to: increase cation exchange capacity (CEC); increase base cation saturation, decrease bulk density, increase moisture retention, and increase pH (Zhang Y. F. et al., 2021; Kumar et al., 2023). In addition to the direct effects of biochar on the physical and chemical properties of soil, literature suggests that the positive impact of biochar on plant fertility may also result from the habitat and metabolic resources that biochar provides for beneficial microbial communities (Lehmann et al., 2011; Zhu et al., 2017; Tan et al., 2022; Xu et al., 2023) and that microbial inoculation of biochar can positively impact fertility (Castro et al., 2018). A positive relationship has often been observed between the amount of biochar added to soil and plant growth (Graber et al., 2010; Břendová et al., 2015; Haider et al., 2024). However, saturating and even negative effects have also been reported at high levels of biochar (Rondon et al., 2007; Upadhyay et al., 2014; Fornes et al., 2017). An improved understanding of biochar’s impact on individual species and on plant tissues within species may be critical to the future of sustainable agriculture and carbon sequestration.
Fortunately, there is a prodigious and growing body of research on the impacts of biochar on different species of plants and different plant tissues. Significant positive effects of soil biochar have been observed for a broad range of different plant types including: woody plants (Lebrun et al., 2017; Lefebvre et al., 2019); broadleaved herbaceous plants (Thomas et al., 2013); grains such as corn (Rajkovich et al., 2012) and wheat (Vaccari et al., 2011); and vegetables ranging from lettuce (Upadhyay et al., 2014) to onions (Khan et al., 2019). However, studies that control for biochar source and soil type have found significant differences in the impact of biochar on different plant species (Hairani et al., 2016; Lefebvre et al., 2019). Prior research likewise reveals that soil biochar can impact different plant tissues differently and that these impacts can differ among species. For example, Li et al. (2022) observed that biochar resulted in an increase in root:shoot ratio in rice (Oryza sativa). In contrast, Yang et al. (2015) documented a decrease in root:shoot ratio in sugarcane (Saccharum officinarum). Graber et al. (2010) found that biochar increased fruit yields in peppers (Capsicum annuum) but had no impact on shoot height. In contrast, these same researchers documented that biochar significantly increased tomato (Lycopersicum esculentum) stem length but not fruit production. Understanding the potentially distinct impacts of biochar on crops that are economically important for food and fiber is critical to advancing sustainable agricultural practices. However, there is a significant barrier to understanding these differences.
In spite of clear and compelling evidence that biochar can increase soil fertility, there are at least two important challenges to drawing general inferences from the literature about the differential effects that biochar might have on different plants and different plant tissues. One is that biochar is not a uniform material. Different feedstocks result in biochar with distinct chemical (Haider et al., 2022) and physical (Xu et al., 2023) properties that affect plants differently (Güereña et al., 2015). Likewise, different production processes affect biochar chemistry (Kumar and Bhattacharya, 2021; Bo et al., 2023). For example, increased temperature of biochar production (pyrolysis) has been found to increase: pH, ash content, surface area, and CEC (Elad et al., 2011), H:C ratio (Ronsee et al., 2012) and porosity (Ghorbani et al., 2024). Unsurprisingly, biochar produced with a uniform feedstock but different production processes has been found to result in differences in microbial communities, earthworm preferences, and plant biomass (Chan et al., 2008). These studies indicate the necessity of controlling for biochar source and production process when comparing biochar impacts on plant fertility. A second problem is that the impact of biochar on fertility may be dependent on the soil to which it is applied (Vanapalli et al., 2021; Singh et al., 2022). For example, Manolikaki and Diamadopoulos (2016) found that biochar addition increased ryegrass growth in sandy loam but not in loam soil. Other studies have likewise demonstrated that impacts of biochar vary depending on soil texture (Butnan et al., 2015; Manolikaki and Diamadopoulos, 2018). These studies suggest that it is simply not possible to directly compare the impact of biochar among species or among tissues from reports in the literature if the studies being considered differ in either biochar source or soil type. In this paper we describe what we believe to be a unique controlled experiment to compare the impact of different levels of biochar on different plant tissues in three functionally distinct species of plant.
We compared how different plant tissues in three functionally and economically distinct species of plant responded to a single source of biochar applied to a single soil type. Specifically, we designed an experiment to examine the impact of biochar produced from coppiced hazelnut trees on cherry tomatoes (Solanum lycopersicum var), green beans (Phaseoulous vulagris var. green bean), and hybrid willows (Salix spp.). Beans and tomatoes were selected for two reasons: they are important vegetable crops and they are functionally distinct, as beans are in the legume family and have different nutritional requirements. Hybrid willow was chosen because it is a fast-growing woody plant, often used for remediating damaged land and as a source of biofuel (Karp and Shield, 2008). Each plant type was grown in the same organic planting mix (peat moss and vermiculite) subjected to four distinct levels of biochar (0, 3, 9 and 26% by dry weight). We chose these levels based on reviewing literature on other biochar experiments. The 26% level was chosen because several prior experiments have shown saturating or inhibitory effects at this level (Upadhyay et al., 2014) or other high levels (Rondon et al., 2007; Fornes et al., 2017). We choose these quasi-logarithmic treatment levels with the goal of fitting a hyperbolic saturation curve to define the relationship between biochar concentration and growth parameters. This would have allowed us to assess and compare how different plants and tissues might saturate at different biochar levels. We examined multiple growth parameters within and among the different plant species to assess differences in the impact of a single source of biochar on different species and on different tissues in these species. Different authors refer to plant tissues differently. For the purposes of consistency within this paper we will use: “roots” to refer to all below ground tissue production (biomass); either “aboveground biomass,” or “shoots” to refer to both stems and leaves, excluding fruit and seed production; and “fruits” to refer to fruit and seed biomass.
Prior studies have documented a variety of impacts of biochar soil amendment on the three species of plants selected for our experiment. Positive effects of biochar observed for beans include increases in: the biomass of roots and shoots (Güereña et al., 2015; da Silva et al., 2017); total plant biomass (Melo et al., 2018); fruit biomass; and the number of pods and seeds (da Silva et al., 2017). However, some experiments have also documented negative impacts of biochar on bean shoot and fruit biomass (Velez et al., 2018). Saxena et al. (2013) found that biochar significantly increased: percent germination, root length, shoot length, flowers per plant, pods per plant, number of seeds, seed biomass, and tolerance of high temperatures and extreme light conditions. Increases in root:shoot ratio in response to biochar addition have also been observed in beans (Torres et al., 2020). Conflicting results have been observed regarding the impact of biochar on bean root nodules, with some studies showing a decrease in abundance (Castro et al., 2018) and others showing increases (Güereña et al., 2015). Several studies have observed increases in nutrient levels in bean tissues grown in soils augmented with biochar, including P, Fe, Mg (Gao et al., 2016), N, K, Ca, Zn, Cu, Mn (Inal et al., 2015), and B (Rondon et al., 2007). Several experiments demonstrate that benefits saturate or are even inhibited with increasing soil biochar. For example, Rondon et al. (2007) found bean biomass increased up to 6% soil biochar and decreased after 9%.
A considerable body of literature exists on the effects of biochar on growth and fertility of tomatoes. The addition of soil biochar has been shown to increase many growth parameters including aboveground biomass, total biomass, fruit yield, and plant height (Ronga et al., 2020; Guo et al., 2021). Tartaglia et al. (2020) found that biochar addition resulted in an increase in: early growth, the number of flower buds and the average number and weight of fruit. Akhtar et al. (2014) reported that biochar improved fruit quality as well as yield. Documented impacts are not entirely positive or consistent. Two studies documented a negative impact of biochar on tomato seedling growth (Yu et al., 2019; Vaughn et al., 2021). Two other studies found positive impacts on aboveground and root tissues, but no increase in tomato fruit yield (Vaccari et al., 2015; Velli et al., 2021). Other experiments have reported no significant impact on biomass production (Liao et al., 2021) or fruit yield (Dunlop et al., 2015). Research has also investigated the combined effects of fertilizer, microbial inoculation, and biochar application. Several studies have found that the positive impact of soil biochar on tomato plant height, shoot and root biomass, and fruit production is enhanced when it is augmented with either microbial inoculant (Castro et al., 2018) or compost (Sani et al., 2020). However, Nzanza et al. (2012) observed no effects of the combined addition of biochar and inoculation on tomato root biomass.
Fewer studies have examined biochar impacts on willow and the impacts observed have been more equivocal. Several studies have documented positive impacts on willow growth. Saletnik and Puchalski (2019) found that biochar addition increased growth in Salix viminalis L. Similarly, Kuttner and Thomas (2017) documented increased diameter, height, and drought resistance in S. exigua Nutt. Seehausen et al. (2017) documented increases in root:shoot ratio in S. purpurea. Several studies have documented positive impacts of the addition of soil biochar on willow biomass in contaminated soils (Lebrun et al., 2017, 2019; Mokarram-Kashtiban et al., 2019). Břendová et al. (2015) demonstrated a positive correlation between soil biochar and aboveground biomass of the hybrid Salix × Smithiana. Lebrun et al. (2017) observed differing effects on different tissues; in S. alba and S. purpurea, root and leaf biomass increased with soil biochar, while stem dry weight was not affected. Kuttner and Thomas (2017) found that biochar addition did not significantly influence biomass and growth in Salix exigua Nutt but did mitigate drought stress. Detrimental effects have also been observed. A mix of biochar and compost treatment caused Salix triandra x Salix viminalis to grow more slowly than a control group (von Glisczynski et al., 2016). A study conducted with S. purpurea documented negative effects of soil biochar on aboveground biomass, and mean total branch length (Seehausen et al., 2017).
As stated, the challenge of interpreting the literature that we have summarized for beans, tomatoes and willows is that the experiments discussed were conducted using a wide range of biochar in a wide range of soils. This makes it essentially impossible to clearly identify whether the impacts observed in different experiments might have resulted from differences in biochar and soil or represent generalizable results. We designed an experiment to assess the impacts of varying levels of one type of biochar used in one type of soil on different plant species and different tissues within those plant species. Given the important functional and physiological differences between beans, tomatoes, and willows we hypothesized the plants and the individual tissues of these plants would exhibit distinct responses to biochar. Our research objective was to test this hypothesis by addressing several specific questions that could only be assessed through experimental conditions that control for biochar source and soil type. Specifically, we asked:
1. How do three functionally distinct plant species (green beans, tomatoes, and willows) differ in their response to different levels of soil biochar?
a. Do these species differ in response with respect to: root growth, shoot growth, fruit production, root:shoot ratio, fruit:(root+shoot ratio), percent moisture content of fruit, and individual fruit weight?
b. Are certain tissues more affected by biochar than others and does this response differ among the species examined?
c. How does biochar level affect germination rate of beans, and date of first flower for beans and tomatoes?
2. Does the response of willows to biochar change during a second season of growth?
a. Does additional fertilization of willows impact second year growth?
3. How does biochar affect the properties of an organic-rich soil and how is this mediated by plants and microbial inoculation?
a. Does biochar addition affect soil properties differently when different species are grown in that soil?
b. Independent of biochar addition, do tomatoes and bean plants impact soil properties differently?
c. Does enhanced fungal and bacterial inoculation improve or alter biochar’s impact on plant growth?
2 Materials and methods
2.1 Plant preparation and management
Four plant types were used: cherry tomatoes (Solanum lycopersicum var. “Supersweet 100”), bush beans (Phaseoulous vulgrais var. Burpee’s “Stringless Green Pod”), willows (Salix sp), and a no plant control. Tomato starts approximately 10 cm in height were acquired from Thome Farms Greenhouse in Elyria, Ohio in early June of 2021. One plant was added to each treatment pot. Cages were later added for support.
Green bean seeds were acquired from Tractor Supply Company. Beans were soaked overnight, drained, rolled in rhizobia bean inoculant, and planted in treatment pots at a depth of ~1.5 cm with 6 seeds per pot. Once bean sprouts reached an average height of 8 cm, they were thinned to 3 sprouts per pot. In late August, beans were thinned to two plants per pot. Green bean plants were attacked by bean leaf beetles (Cerotoma trifurcata) throughout the course of the experiment. A natural pesticide consisting of 1 L of water, 8 mL of neem oil, and 3 mL of 1% Liquinox dish soap solution was regularly applied to the beans to control damage through the end of the experiment.
Cuttings of hybrid willows were taken in early June from an existing tree that was originally obtained from Gurney’s Seed and Nursery Company.1 Cuttings were 30 cm length and 1–3 cm in diameter. Three willow cuttings were directly rooted in each treatment pot. Labels were fixed around the base of each cutting to track growth of individual plants.
Each experimental unit consisted of a 2-gallon (7.6 Liters) fabric pot (PHYEX brand, Dongguan, CN) containing the same planting mix with four different levels of biochar: 0, 3, 9 and 26% by biochar and soil dry weight. In addition to the plants, we included a no-plant control. Our 4×4 design thus consisted of 4 plant types (including the no-plant control) and 4 biochar levels. We used 8 replicates per treatment resulting in 128 experimental units for the main experiment. As described below, we also included an additional “low inoculation” treatment for each of the four plant types at the 9% biochar level to which we applied only a single microbial inoculation with 8 replicates resulting in an additional 32 experimental units.
2.2 Site and management
The experiment was conducted at Oberlin College’s Adam Joseph Lewis Center for Environmental Studies (41.2959° N, 82.2211° W) from June–October 2021, and extended to November of 2022 for the willow plants. Oberlin is in a temperate climate in USDA hardiness zone 6a. An experimental area of 700 square feet was covered in a black weed block fabric, covered in straw to combat heat island effects, and fenced to prevent unwanted herbivory from humans and other animals. Plants were watered by hand until July 26 when an automatic irrigation system was installed to ensure water was not a limiting factor.
Figure 1 shows how treatments were generally arranged in the experimental area. As depicted, pots within a treatment were not randomized by treatment level, rather treatment levels were kept together to avoid confusion and cross contamination. The main uncontrolled variation in the experimental area was variability in shading by buildings, trees, and other structures. To minimize the impact of this variability, once a week, plants were rotated by 10 units to negate any effects of placement within the experimental area. Plants were moved in an s-shaped rotation so that every plant was periodically in every row and therefore experienced similar variation in shading.
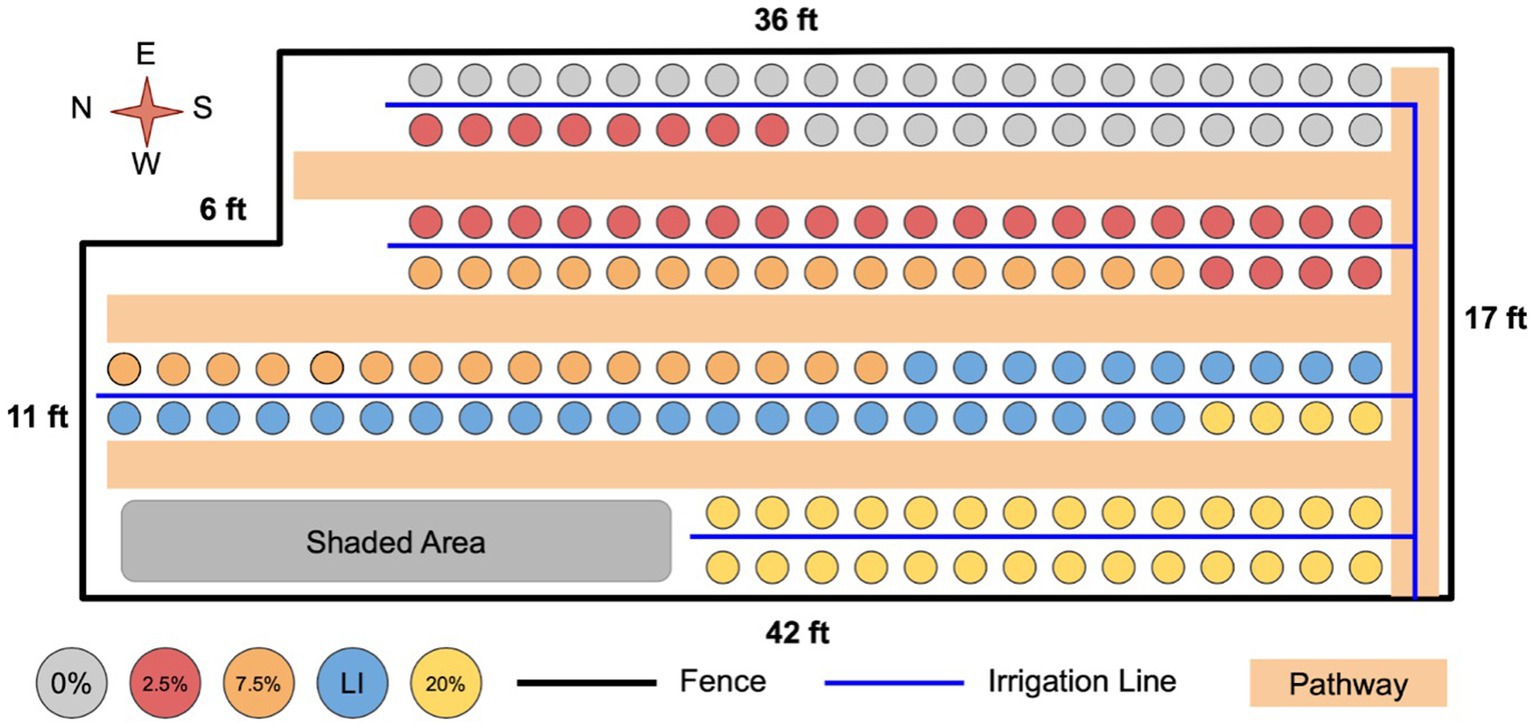
Figure 1. Layout of the experimental system. Plants were placed in the order: bean, willow, no plant, tomato. Experimental units were placed in the order of replicate number. Plants were moved regularly as described in text to minimize the impact of variability in shading.
2.3 Biochar source and preparation
Biochar was created from local coppiced hazelnut wood. Harvested branches, ranging in diameter from approximately 1–10 cm were combusted in a retort oven that was fabricated for this experiment. Specifically, a 120-gallon (450 L) propane tank was converted into a rocket stove; the top was cut off; air holes were cut into the bottom and a 3-m chimney was added to the top. A standard 50-gallon (190 L) steel drum was used as the inner chamber. The overall size and design of the inner chamber was similar to the biochar retort furnace used by Wijitkosum (2021). The hazelwood was packed into this chamber and capped with a lid that did not form a complete airtight seal and placed upside down into the rocket stove. The space between the two containers was then filled with scrap wood, the top and chimney were placed back on, and a fire was lit from below. The outer fire then heated the hazelnut wood in the absence of an oxygen source to the point that pyrolysis released combustible gasses that heated the unit until the run was completed. A complete run from ignition through termination of combustion was typically approximately 4 h. Although we did not directly measure internal temperature, given similarities in design to the apparatus described by Wijitkosum (2021), we estimate combustion at 450–600°C. The product of pyrolysis in the retort oven contained charcoal with no evident ash production of any kind. Material from multiple combustion runs was processed through a woodchipper and then homogenized resulting in biochar that ranged in size from fine powder to 5 mm in diameter.
2.4 Soil preparation
In order to achieve a uniform and controlled soil for the experiment, a soil mixture was created from a 1:1 volumetric ratio of perlite and shredded peat moss. To create the mix, 1 gallon (3.8 L) of perlite and 1 gallon (3.8 L) of peat moss were added to a hand concrete mixer and homogenized. The wet weight of 1 gallon (3.8 L) of perlite was 370 grams (g) and the wet weight of 1 gallon (3.8 L) of peat moss 500 g. The moisture content of both assessed and determined to be low (<8%). Biochar was then added in addition to the 870 g mass of soil per pot. For the four treatment levels, 0 g, 22.3 g, 70.5 g, and 217.5 g of dry biochar were added, respectively, to achieve the 0, 3, 9, and 26% by dry weight. The soil mix was added to the fabric pots. The chipped biochar was heterogeneous in particle size. In order to control for this and ensure uniformity in biochar treatments, we passed the chipped biochar through a 2 mm soil sieve to separate fine and coarse particle sizes. The same ratio of course and fine particles was added to every experimental unit that received biochar.
All experimental units were inoculated with two separate commercial sources of microbial inoculant. Manufacturers of the “Mikro-Myco” (4655 Waterford Dr., Suwanee, GA) state that it contains a combination of plant growth promoting rhizobacteria (PGPR) and phosphate solubilizing fungi (PSF) with 4 species of Endo Mycorrhizae and 7 species of Ecto Mycorrhizae. We also used “Wildroot Organic” (PO Box 4800, Horseshoe Bay, TX). An inoculant solution including both products was applied three times over the course of the summer: July 1st, July 22nd, and August 8th. The inoculant was prepared according to instructions, and a total of 100 mL of both inoculants were applied to each experimental unit at each inoculation. As mentioned, a side experiment was conducted to assess the impact of inoculation. One set of plants of each species grown at the 9% biochar level were inoculated like all other treatments. A second set of low inoculant (LI) plants were also grown at the 9% biochar level. The LI group was only inoculated once near the start of the experiment.
Every experimental unit was fertilized once, shortly after planting, using Osmocote 14–14-14 N:P:K time release fertilizer granules.2 Based on the manufacturer’s recommended application rate; 15 mL of this fertilizer was sprinkled on the soil surface in each pot.
2.5 Measurements
A variety of different growth parameters were measured for different plants at different times in the experiment. For example, we measured the germination rate for green beans and the flowering timing for both beans and tomatoes. Ripe tomato and bean fruits were harvested and immediately frozen throughout the growing season. Frozen fruits were cleaned of condensate, weighed, oven-dried and then reweighed. As common comparison measurements for all plants, we assessed the final root and shoot biomass for tomatoes, green beans, and willows, as well as the total fruit biomass for tomatoes and green beans. All reported weights for plant tissues are oven-dry weights unless otherwise specified. We also assessed total biomass, the sum of root, shoot, and fruit biomass for the species that produce fruit. Total biomass for willows was the sum of root and shoot biomass as there was no flower or seed production in these young plants. Root:shoot ratio was calculated by dividing the root biomass by the shoot biomass. The fruit:(root+shoot) ratio was calculated by dividing the fruit biomass by the sum of the root and shoot biomass. Fruit percent moisture content was measured after collecting all fruit produced from a plant throughout the season and measuring both wet and oven-dried weight (Equation 1). The equation used to determine percent moisture content was:
The average dry weight of the fruit was calculated by dividing total fruit biomass dry weight by the total number of fruits produced.
We measured the germination rate in beans by simply counting how many of the six bean seeds planted in each pot produced green shoots that broke the soil. In green beans and tomatoes, we also measured the day of first flowering for each plant (there were two plants per pot for the green beans and each plant was measured). Date of the first flower was recorded beginning on July 29. On this date, several plants had already had multiple flowers for several days, but we counted this as the first flowering date for these.
At the end of the 2021 growing season in October, any remaining fruit on the green bean and tomato plants was harvested and the remaining shoot tissue was cut where it met the soil. Aboveground shoot biomass was placed in a paper bag, oven dried and then weighed. No attempt was made to separate leaf from stem tissue in either beans or tomatoes; these were collectively treated as “aboveground biomass” or “shoots.” Tomato and bean roots were carefully separated from the soil by hand. Root material greater than 1 cm in length was captured, smaller root fragments were not. The aboveground tissues from the two individual bean plants in each experimental unit for beans were processed and weighed and analyzed as a single unit. The root tissue of the two bean plants was inseparable and also treated as a single unit.
Root material was rinsed with water, to further remove soil particles, and placed in paper bags for drying. Both root and shoot material were dried in a drying oven at 100°C until they no longer lost weight. A subset of root samples was incinerated in a muffle furnace for 24 h at 400°C. On average, mineral content of roots was less than 20% (Table 1). Mineral content of roots was not significantly larger than mineral content of shoots and fruits. We therefore concluded that we had effectively removed the vast majority of the soil particles from the roots. Final dry weights of roots, fruits, and aboveground biomass that we report in the results section include the mineral content.
Since our intention was to continue growing a subset of the willows for a second season, experimental units in the willow treatment were processed differently from the beans and tomato units. Each experimental willow unit contained three plants. Following the first season, the roots of these three plants in each unit were carefully separated from each other. Of these three plants in each unit, the one of intermediate height was processed to measure above and belowground biomass. The other two plants were retained for a second growing season. The intermediate height plant was cut at soil level, and root and shoot material was processed and dried using the same procedures described for tomato and beans. However, in contrast to beans and tomatoes, the dry weight reported for aboveground plant tissue in willows includes only stem tissue and not leaf tissue.
The willows that were retained for a second growing season were transplanted from 2-gallon (7.6 L) to 4-gallon (15.1 L) pots. We planted each of the two remaining willow plants from each experimental unit in its own individual pot, effectively doubling the number of experimental units from 8 to 16 at each biochar level. We reused the soil retained from the beans and tomato treatments for repotting the willows. Soil from each biochar level for the beans and willows was homogenized prior to using it for this purpose. During the second year, the willows were grown in the same plot (Figure 1) as the previous year and rotated in the same manner as described earlier. Additional microbial inoculant was not applied to the willows during the second season.
Willows were not fertilized at the start of the 2nd growing season. However, in response to leaf yellowing, we decided to fertilize half the willows at the end of July of the 2nd season. The decision to fertilize only half of the plants was made so that we could assess whether biochar level influenced the willows response to the fertilization. Each of the willows fertilized received 30 mL of the Osmocote sprinkled on this soil surface as in the first year (doubled from the first year to account for the doubling of pot volume).
In 2021 an attempt was made to capture willow leaves with netting prior to leaf fall. Unfortunately, this proved ineffective and willow leaves were not captured. We successfully captured leaves in the fall of 2022. We determined that leaves accounted for only a small fraction of total aboveground biomass in willows. In the results section, aboveground biomass for willows therefore includes only the woody tissue, not leaves.
Since all the willows were fertilized in 2021, the results presented in section 3.7 compares fertilized 2022 willows with 2021 willows. In section 3.8 we assess differences between fertilized and unfertilized willows in 2022.
2.6 Soil analysis
Our experiment focused on the impact of biochar soil amendment on plant fertility. Nevertheless, we thought it important to assess differences in soil chemistry resulting from biochar addition. Due to constrained resources, we were unable to conduct soil analysis for all biochar levels for all species. Soil taken from beans, tomatoes, and no plant pots were analyzed from the 0% biochar level and 26% biochar level. Soil from the willow plants was not analyzed. After all biomass was removed from tomato and green bean pots, the soil from each was re-homogenized and samples were sent for soil analysis. Soil was analyzed by Logan Labs (620 North Main Street, Lakeview, OH 43331) using standard methods of soil analysis (NCR-13 Soil Testing and Plant Analysis Committee, 1998). Analyses included: pH, organic matter, cation exchange capacity (CEC), S, P, Ca, Mg, K, Na, B, Fe, Mn, Cu, Zn, Al, and percent base saturation.
2.7 General statistical model
As stated, we selected quasi-logarithmic treatment levels, with our highest biochar level to be within the range in which prior experiments have shown saturating or inhibitory impacts on plant growth. Our intent was to fit a hyperbolic saturation curve to data on biochar level vs. growth parameter. However, initial examination of our data provided no evidence for this expected non-linear response. We therefore applied linear statistical models to quantify the impact of biochar on the various growth parameters examined. In particular, in order to answer our research questions, we assessed whether the slope of the relationship between each growth parameter measured and biochar was greater than zero. In other words we assessed whether biochar concentration had a significant positive relationship on each growth parameter.
As indicated in the introduction, a key goal of our analysis was to be able to compare the overall effects of biochar among species and among tissues within and between species. In order to be able to do this, we standardized data for each growth parameter by calculating z-scores for that variable (Equation 2). The magnitude of the slope calculated by regressing z-scored response variables against biochar then provides a non-dimensional measure of the strength of the impact of biochar on each growth parameter examined. This standardization allows slopes (essentially effect size) to be directly compared both among tissues within a plant species and among species. Specifically, a significantly larger slope indicates a stronger response to biochar application. We assessed whether slopes for different growth parameters differed within each species (for example, are the roots of tomato plants more affected by biochar than shoots?). We also assessed whether slopes differed among species (for example, is the response of root tissue to biochar greater in tomatoes than beans?). Unless otherwise noted, all p-values reported in the results section are the p-values assessing whether slopes are different from zero (i.e., testing for a positive or negative impact of biochar) and whether slopes differ from each other (more impact on one growth parameter and or species than another).
We used Analysis of Variance (ANOVA) as an additional model for assessing whether responses differed among individual treatment levels for a given species. Although we compared all the individual treatment levels, for the sake of simplicity, our results section (Table 2) only reports on ANOVA comparisons between the 0 and 26% treatment levels. The comparisons we examined among other treatment levels did not lead to conclusions that differ from simply comparing 0 and 26% levels.
RStudio was used to conduct both linear regressions, and ANOVA using the mosaic package. When only comparing two conditions, ANOVAs were run using RStudio to test for significant differences between the two conditions.
2.8 Standardizing data for comparison among tissues and among species
Different tissues within a plant can be expected to accumulate different amounts of biomass. Standardization of data was therefore necessary to be able to directly compare the impact of biochar among plant tissues within each plant species (for example, are the roots of tomato plants more affected by biochar than shoots?). Similarly, different species of plants also accumulate different amounts of biomass from each other. Standardization of the data was therefore also necessary to compare impacts of biochar among plant species (for example, is the response of root tissue to biochar greater in tomatoes than beans?). Z-scores were used for standardizing data for each variable that was compared among tissues and among species (Equation 2). The equation used for calculating the z-score was:
where z is the z-score, x is the observed value for one growth parameter for one experimental unit, μ is the mean of all values for that growth parameter for that plant species across all treatments within the biochar treatment experiment, and σ is the standard deviation of all values for that growth parameter for that plant species. All reported data on plant growth and biomass is reported as z-scores. z-scores are not used to analyze seed germination rate, number of flowers, timing of flowering and soil variables.
We conducted a concurrent experiment to assess the impact of low versus higher levels of biochar inoculation. Calculations of statistics, including z-scores for the main experiment (the effect of biochar on growth parameters in three species) completely exclude experimental units in the low inoculant group. Statistical analysis conducted on the high versus low inoculation assessment include only experimental units in the 9% biochar level (the level at which this assessment was conducted).
3 Results
3.1 How does biochar affect plant growth in different species?
3.1.1 Total biomass
Biochar had a highly significant impact on total biomass (for beans and tomatoes: root + shoot + fruit and for willows just root + shoot) for all three species considered (p = <10E-15, Table 2 and Figure 2A). The impact of biochar on total biomass was similar among species, with no significant differences in impact at the alpha = 0.05 level. Beans exhibited a marginally stronger response to increasing biochar than tomatoes (p = 0.08, Table 2) and were not different from willows (p = 0.1).
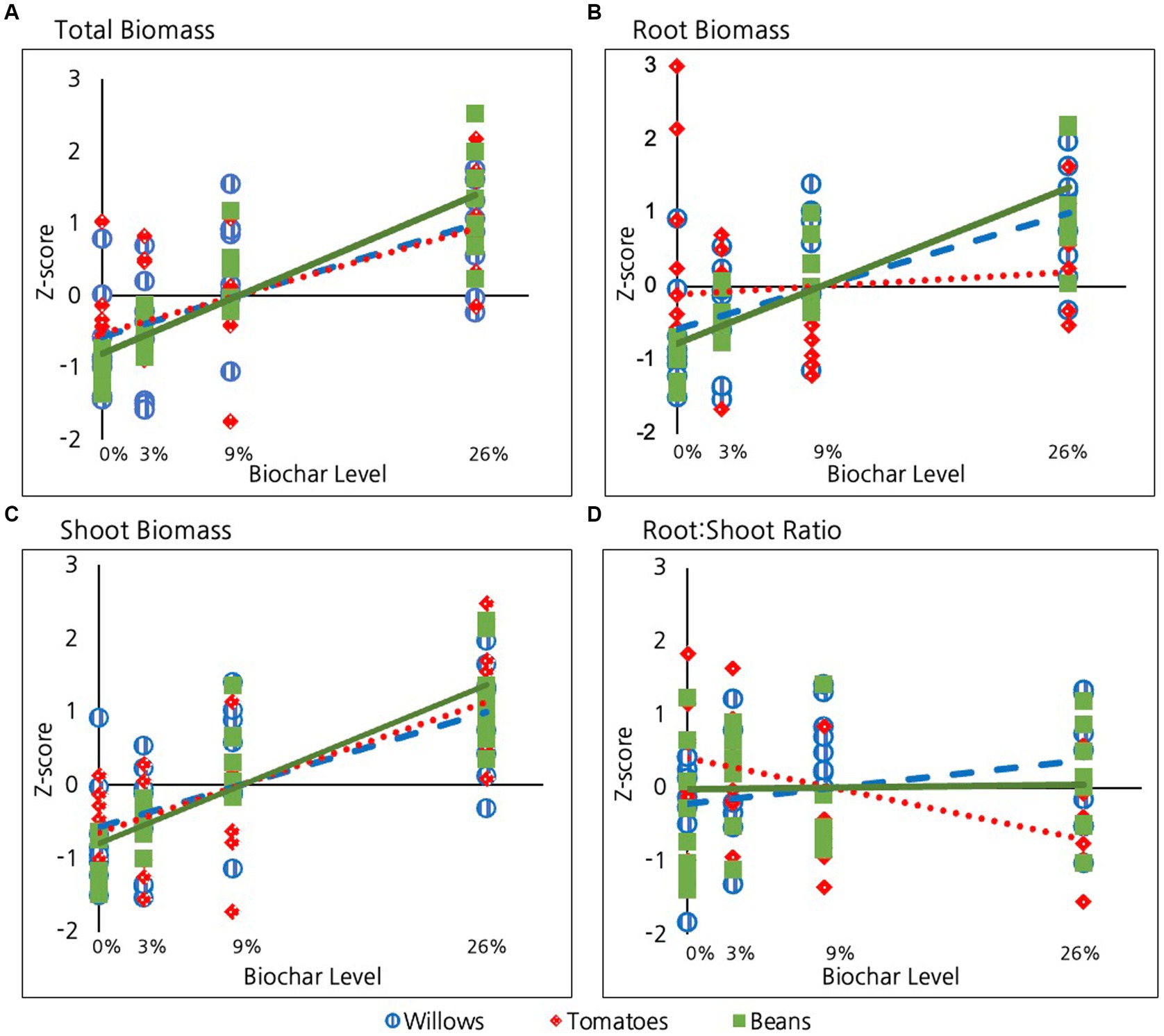
Figure 2. Effect of biochar on: (A) Total plant biomass, (B) Root biomass, (C) Shoot biomass and (D) Root:shoot ratio in the three species examined.
3.1.2 Root and shoot biomass
Biochar had a highly significant positive impact on root biomass in all three species (p = <10E-4, Table 2 and Figure 2B). However, the strength of this impact differed among species. Bean roots exhibited a significantly stronger response than the roots of tomatoes (p = 0.001, Table 2). Though not as significant as beans, willows also exhibited a stronger response than tomatoes (p = 0.05, Table 2). Bean and willow roots did not differ from each other in their response to biochar treatment (p = 0.2).
Similar to roots, Biochar had a highly significant positive effect on shoot production for all three species (p = <10E-9, Table 2 and Figure 2C). The strength of this relationship did not, however, significantly differ among species (p = 0.2).
3.1.3 Root:shoot ratio
In contrast to the significant positive impact of biochar on roots and shoots in all three species, biochar did not have a significant and definitive overall impact on root:shoot ratios when the three species were considered together (p = 0.9, Table 2 and Figure 2D). Considered individually, the root:shoot ratio was not significantly impacted by biochar in either willow or bean plants. Tomatoes, however, exhibited a marginally significant decrease in root:shoot ratio with increasing biochar (p = 0.07, Table 2). To investigate this effect further, we conducted a pairwise comparison of the root:shoot ratio in tomatoes between the 0 and 26% treatment groups and found that the 26% groups had a significantly lower root: shoot ratio than tomatoes in the 0% treatment group (p = 0.04).
3.1.4 Fruit biomass
Biochar had a highly significant positive overall impact on total fruit production (dry weight of total harvest) in beans and tomatoes when considered collectively (p = <10E-6, Table 2 and Figure 3A) and individually (p = 0.001, Table 2). The strength of this relationship did not, however, differ significantly between these two species (p = 0.4).
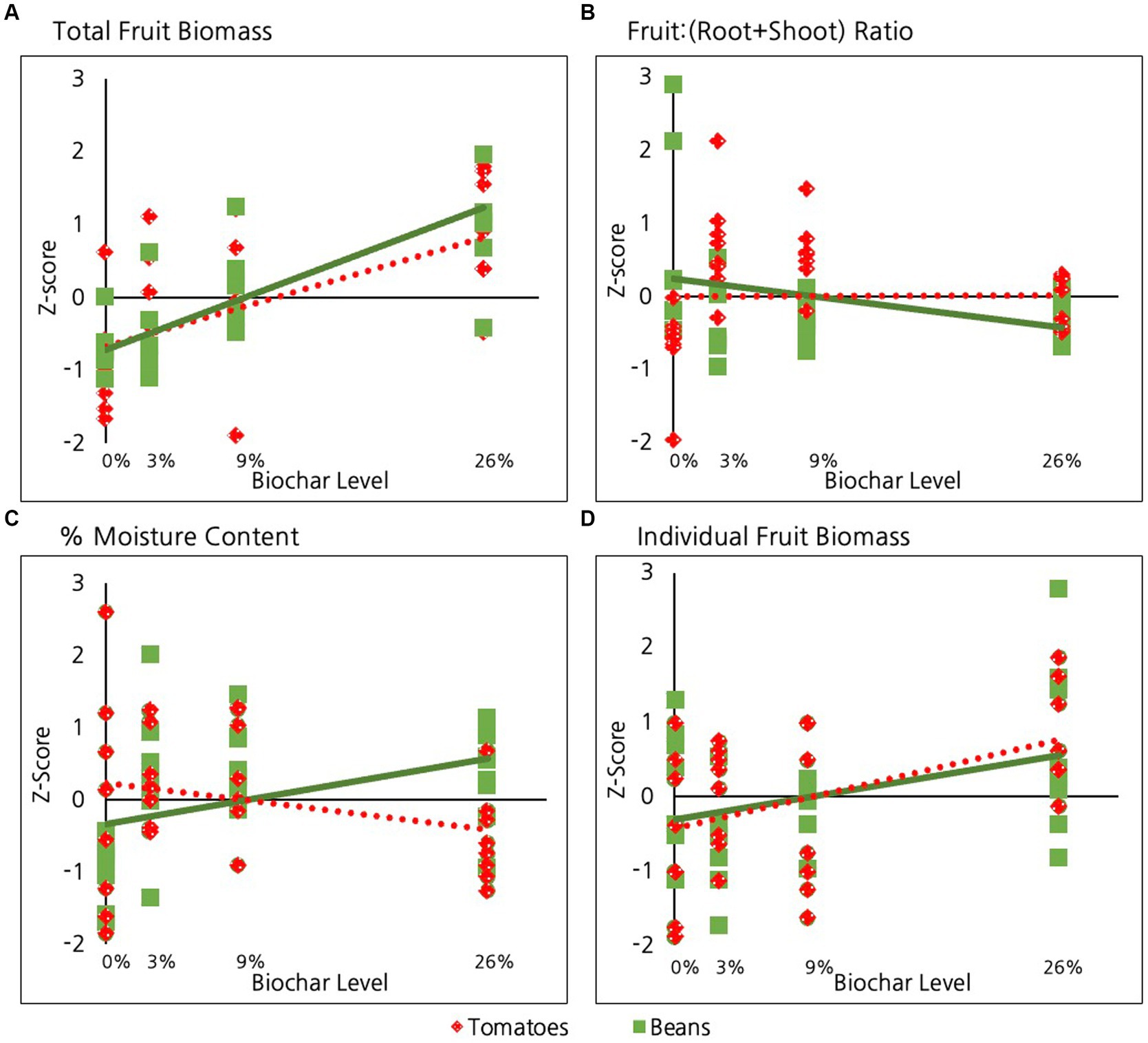
Figure 3. Effect of biochar on different aspects of fruit production in tomatoes and beans including: (A) Total fruit biomass, (B) Fruit:(root+shoot) ratio (C) Percent moisture content and (D) Individual fruit biomass.
3.1.5 Fruit:(root+shoot) ratio
In contrast to the significant impacts of biochar on fruit, roots and shoots, biochar did not have a significant overall impact on the ratio of fruit:(root+shoot) when bean and tomatoes were considered together (p = 0.5, Table 2 and Figure 3B). Likewise, considered individually neither species showed a significant response in this ratio in response to biochar addition. Not surprisingly, there was no significant difference in this ratio between tomatoes and beans (p = 0.3). Nevertheless, a pairwise comparison to test if there was a difference in ratio of beans between the 0 and 26% biochar levels indicated that the 26% groups exhibited a lower fruit:(root+shoot) ratio than beans in the 0% treatment group (p = 0.04, Table 2).
3.1.6 Fruit percent moisture content
Biochar did not have a significant overall impact on the percent moisture content of bean and tomato fruits considered together (p = 0.6, Table 2 and Figure 3C). However, this lack of general pattern is attributable to the fact that the two species exhibited distinct responses. The moisture content of beans increased significantly with biochar addition (p = 0.04, Table 2). In contrast, the moisture content in tomato fruits was not significantly impacted by biochar addition (p = 0.2). Not surprisingly, the strength of the relationship between percent fruit moisture and biochar addition (Table 2 and Figure 3C) differed significantly for the two species (p < 10E-3).
3.1.7 Individual fruit biomass
When considered together, biochar had a significant positive impact on the average dry weight of individual fruit (p = 0.02, Table 2 and Figure 3D). There was, however, no significant difference in the strength of response between tomatoes and beans (p = 0.4).
3.2 How does biochar affect plant growth in different tissues?
3.2.1 Across species
As discussed, when data are combined for all species, biochar has a highly significant positive impact on root, on fruit, and on shoot production (p < 0.01 in all cases, Table 2 and Figure 4A). When we combine root and shoot biomass together, we observe a significant increase in total plant biomass with increasing biochar (p < 10E-15). As reported, we saw small differences in the strength of the relationships among species, with total bean biomass showing a marginally stronger response than tomato biomass. No other differences were evident.
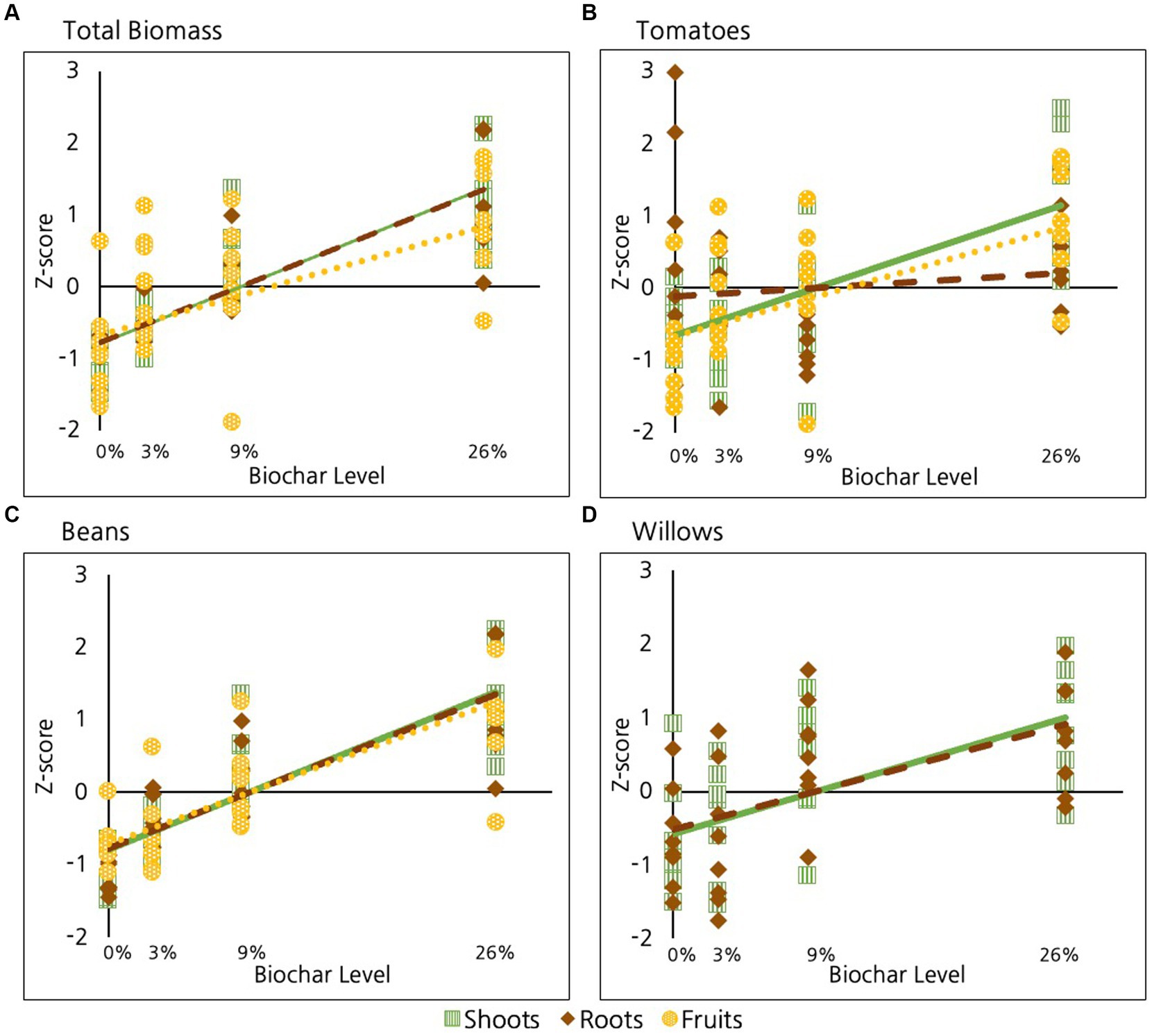
Figure 4. Effect of biochar on different plant tissues compared for: (A) All species, (B) Tomatoes, (C) Beans, and (D) Willows.
3.2.2 Within species
Of the three species examined, tomatoes were the only ones to exhibit significant difference in response among root, shoot and fruit tissues (Figure 4B); Specifically, shoot tissue in tomatoes exhibited a significantly stronger response than fruit and root tissues (p = 0.01, Table 2). Fruit tissues in tomatoes also exhibited a marginally greater response than root tissues (p = 0.08, Table 2). However, no difference was evident between the response of fruit and shoot to biochar addition in tomatoes (p = 0.6). No differences were evident in the response of different plant tissues to biochar in beans or willows (Figures 4C,D respectively, p > 0.6 in all cases).
3.3 Does biochar affect fertilized willow growth over a two-year period?
As mentioned before, only half of the willows were fertilized in 2022. Since all the willows were fertilized in 2021 the analysis presented in this section compares fertilized 2022 willows with 2021 willows. In a subsequent section we assess differences between fertilized and unfertilized willows in 2022. As described in the methods section, because we were unsuccessful in our attempt to collect willow leaves in 2021, we operationally defined willow “shoots” to include only stem material. We were somewhat successful in our efforts to isolate willow leaves in the 2022 growing season. Some leaf tissue may have been lost due to strong winds, but most of the leaf tissue was captured. However, in order to systematically compare data among years, we continued to define willow “shoots” as stem material and total biomass as the sum of this stem material and root biomass (Figure 5). We never-the-less assessed willow leaf biomass in 2022. A negative trend is evident between leaf biomass and biochar addition (Figure 5D), however, the strength of this relationship is only very marginally different from zero (p = 0.1).
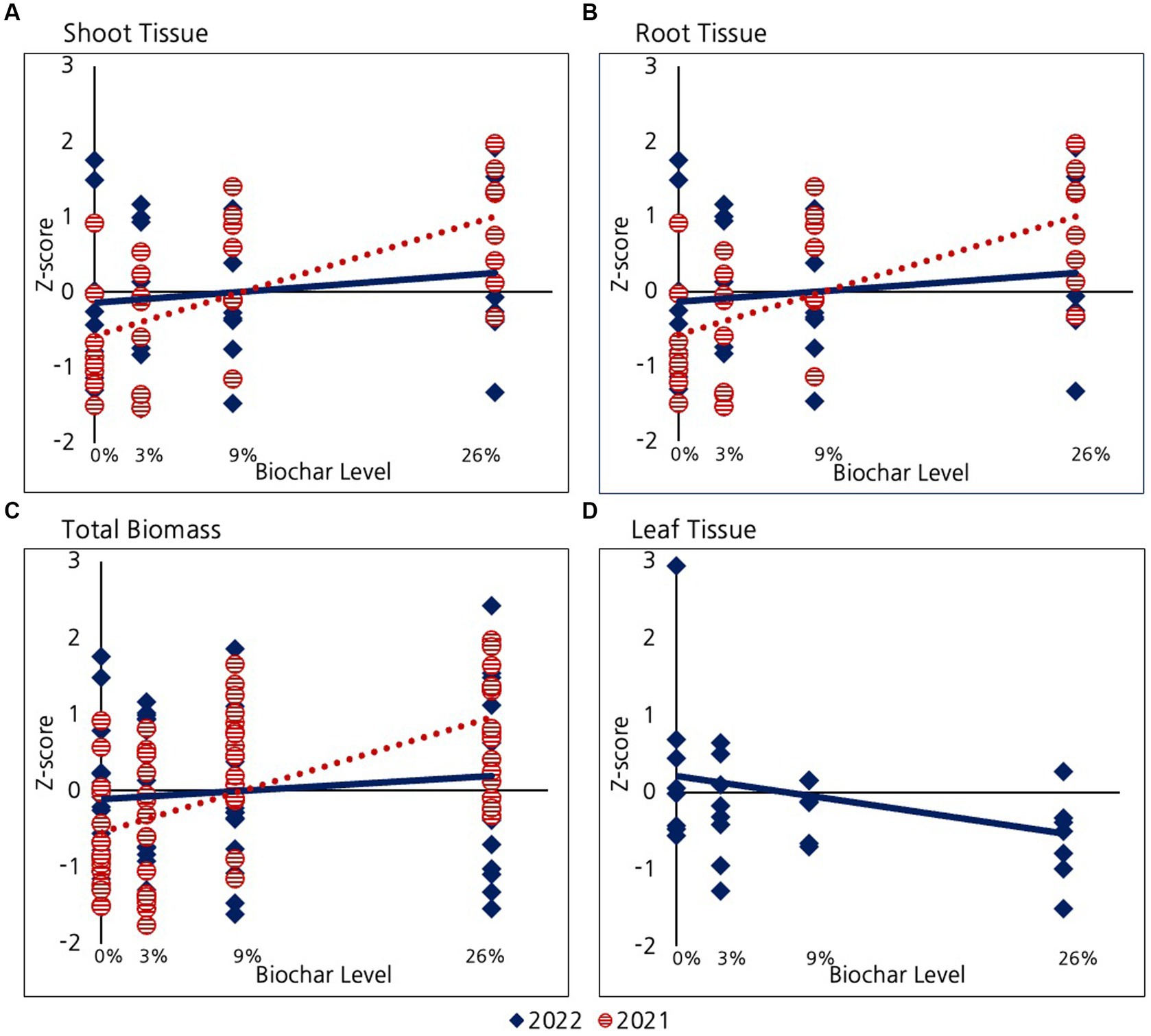
Figure 5. Comparison of the effect of biochar on Willows in their first and second growing season for: (A) Shoot tissue including only stems, (B) Root tissue, (C) Total biomass (shoot+root), and (D) Leaf tissue in 2022. Leaves were only successfully collected and measured in 2022.
As reported previously, during the first growing season (2021) willows exhibited a significant positive response to biochar with respect to shoot growth (stems only), root growth, and the combined total biomass of these two tissues (total biomass). Although the trend of positive response of roots, shoots, and total biomass remained in the second year of growth (Figure 5), the strength of this relationship decreased and was no longer significantly different from zero for any of the variables examined (p > 0.2 for biomass, root, shoot and leaves). A different way of assessing the changing impact of biomass in year two is by considering changes in the strength of the relationship between biochar and willow growth parameters (i.e., slope of the lines). This slope decreased significantly between 2022 and 2021 for root tissue (p = 0.03), shoot tissue (marginal decrease p = 0.07), and biomass (p = 0.03).
3.4 Does fertilization affect biochar’s impact on willow growth during a second growing season?
As discussed, in the 2nd year we divided our experimental units in half to examine whether the level of biochar addition mediated how willow plants responded to a late season fertilization treatment. We found that the shoots (stem material) in fertilized willow plants exhibited a non-significant positive response to biochar while the unfertilized willows actually exhibited a negative response, with the slopes of these lines differing significantly (Figure 6A, p = 0.03). A similar pattern of response was evident for roots, but differences between slopes were only marginally significant (Figure 6B, p = 0.1). The pattern of a marginally significant decrease in growth with increasing biochar in unfertilized shoots, roots, leaf tissue, and total biomass was further supported when we used ANOVA to directly compare the 0% treatment with the 26% treatment for the unfertilized root tissue (p = 0.06), shoot tissue (p = 0.06), leaf tissue (Figure 6C, p = 0.3), and total biomass (Figure 6D, p = 0.05).
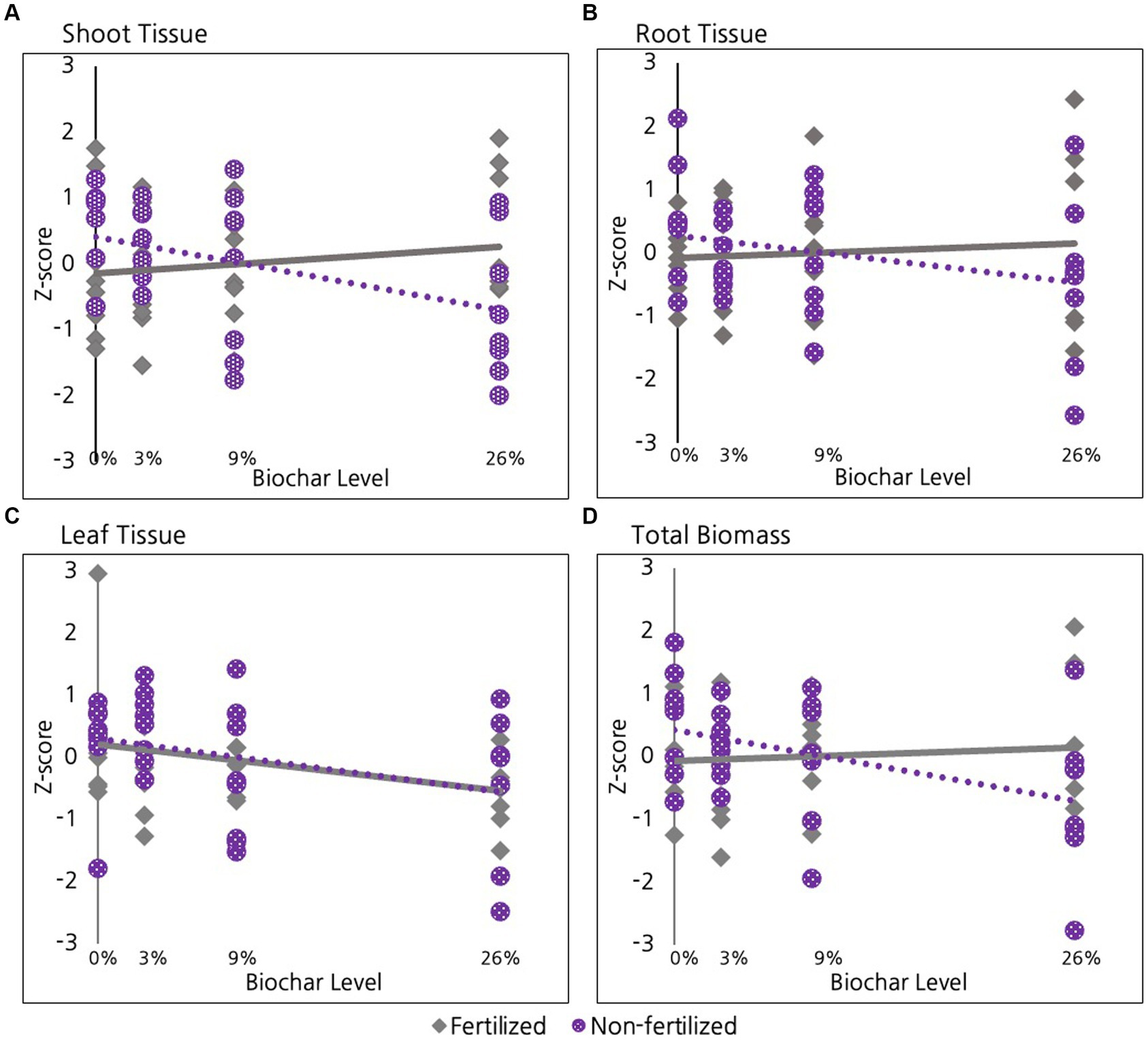
Figure 6. Effect of fertilization on Willows in their second growing season for (A) Shoot tissue and (B) Root tissue (C) Leaf tissue and (D) Total Biomass.
3.5 How does biochar affect seed development and first flowering?
As described in methods, we assessed germination rates for green bean seeds in the different treatments. We found increases in germination with increasing biochar with rates of 79, 88, 94 and 100% for the 0, 3, 9, and 26% treatments, respectively. More specifically, we found a significant linear relationship between biochar level and bean seed germination (p = 0.003), with an enhancement of 21% for the 26% biochar amendment level germination rate compared to the rate at the 0% amendment.
We also observed significantly earlier first flowering in bean plants with increasing soil biochar (p < 10E-6), with first flowering occurring 8 days earlier in the 26% level than the 0% level. In contrast to beans, in tomato plants we observed no significant impact of biochar addition on the date of first flowering (p = 0.6).
3.6 Does biochar affect soil properties?
As discussed, our primary goal was to assess the impact of biochar on plant fertility. A secondary goal was to assess whether biochar addition had a detectable impact on soil parameters and whether these impacts were mediated by the plants grown in that soil. Because this was a secondary goal, in this case we considered only two of the plant species and only the 0 and 26% biochar soils. More specifically, select properties of soil were compared in experimental units that included beans, tomatoes, and no plant treatment at the 0 and 26% biochar levels.
Table 3 reports on the percent difference in soil parameters to document increases in measured soil parameters that might be attributable to biochar addition. P-values resulting from pairwise comparisons among treatments are also included to indicate the significance of differences between 0 and 26% biochar level across soils used to grow tomatoes and beans at both 0 and 26% biochar levels. A dash stands for not significant in the table when p-values were greater than 0.1.
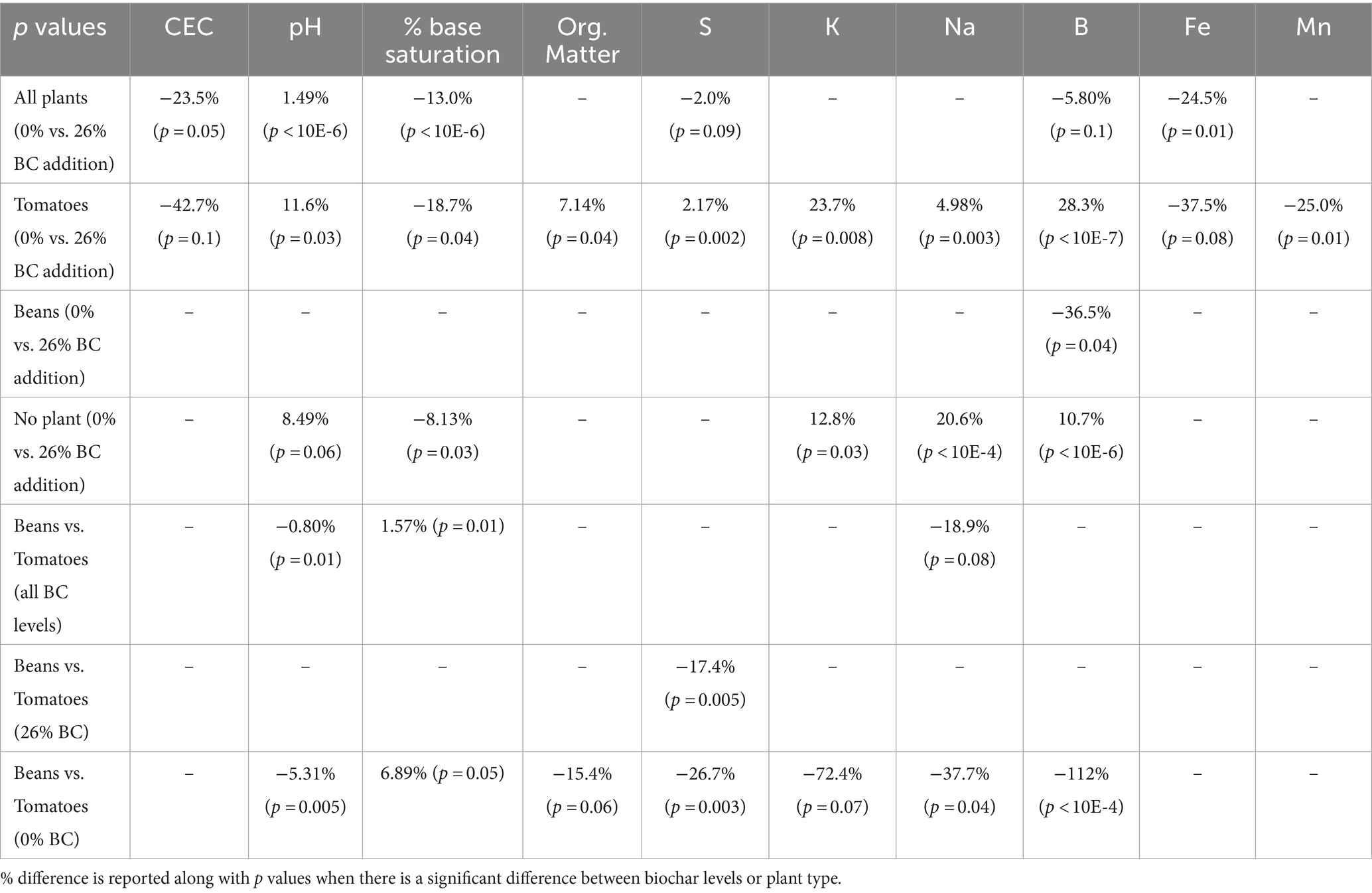
Table 3. Effect of biochar addition on soil properties as well as effect of plant type on soil properties.
The percent difference used to compare impacts on different soils was calculated as indicated below (Equation 3). Here we use cation exchange capacity (CEC) as an example:
In Table 3, positive values in rows 1–4 indicates that the soil property was higher at 26% biochar compared to 0%. Negative values in these rows indicate that the soil property was lower at 26% biochar compared to 0% biochar. For rows 5–7, negative values indicate that the soil property was lower for tomatoes than beans. Values were only reported if significant. Although soils were analyzed for P, Ca, and Mg, no significant differences attributable to either biochar or plants were evident for these elements and so they are not reported in Table 3.
When the soil results were combined for beans, tomatoes, and the no plant controls to examine if there was a general trend in soil properties with the addition of biochar, it was found that CEC, percent base saturation, and Fe all exhibited significant differences between the two biochar levels. As is evident in the table, CEC, percent base saturation, and Fe all decreased between 0 and 26% biochar, while pH increased.
Next, we examined how plant species mediated the effects of biochar on soil properties. The no plant treatment was designed to serve as something of a control. In this control five soil properties, pH, percent base saturation, K, Na and B were significantly different between 0 and 26% biochar treatments (Table 3). Tomato plants appeared to slightly enhance the degree of difference in soil properties between the two biochar levels; when tomatoes were grown in the soil, significant differences between the two soil biochar levels were evident in seven soil properties including percent base saturation, organic matter, S, K, Na, B, and Mn. In contrast, beans appear to have decreased differences in soil properties between the two biochar levels; only B was different between 0 and 26% biochar. We compared the properties of the combined values for the 0 and 26% biochar amended soil grown with tomatoes against that grown with beans and found that there were differences between pH and percent base saturation (Table 3). We compared the tomato and bean soils at the 26% level to examine if biochar addition has different effects on soils grown with different species and observed a significant difference in S (Table 3). We then compared the two species’ soils at the 0% level to examine if the species have an impact on soil properties independent of biochar addition and observed a significant difference in S (Table 3).
3.7 Is there a difference between low inoculant and high inoculant treatments?
As discussed, a concurrent experiment was conducted to assess whether multiple microbial inoculations mediated the impact of biochar on plant growth in the 9% biochar treatment. Repeated inoculation had a positive impact on beans. Compared to the low inoculation treatment, the high inoculation treatment yielded increases in bean biomass (p = 0.008), bean shoots (p = 0.003), and fruit production (Figure 7, p = 0.07). In tomatoes the impacts were less pronounced and in the reverse direction. Compared to the low inoculation treatment, the high inoculation treatment yielded marginally significant decreases in total biomass (p = 0.09) and root biomass (p = 0.05), with no differences evident in tomato production (Figure 7). No significant differences were found for root:shoot ratio or any willow biomass measures between the low and high inoculant treatments.
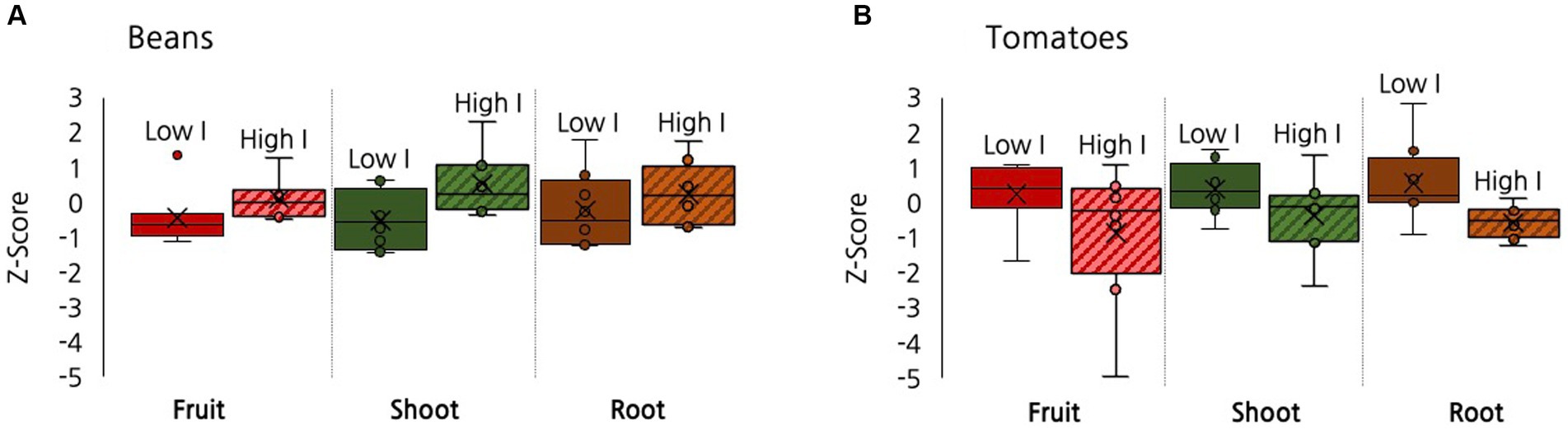
Figure 7. A box and whisker plot showing the effect of inoculation, low versus high, on root, shoot, and fruit production when comparing (A) Beans and (B) Tomatoes. All data is graphed using z-scores as points with an interval between −5 and 3. ‘Low I’ stands for low inoculation and ‘High I’ stands for high inoculation.
We also assessed whether different plants responded differently from each other to inoculation. We compared the results at the low inoculant level between species to examine if some species were affected more than others. We found that beans and tomatoes demonstrated significantly different responses in their biomass production under low inoculation in total biomass, root, shoot, and fruit biomass but in opposite directions. Beans had a moderately significant increase in the high inoculant treatment in fruit (p = 0.07), a significant increase in shoot (p = 0.003) and total biomass (p = 0.02) production. In contrast, the high inoculant tomatoes exhibited a marginally significant decrease in root production and in total biomass (p = 0.09). Among the root, fruit, and shoot tissues there were significantly different responses in root biomass (p = 0.04), moderately significant differences in fruit biomass (p = 0.07), and moderately significant differences in shoot biomass (p = 0.06). Comparisons between tomato and willows and willows and beans were not significantly different (p > 0.1).
4 Discussion
Our experiment examined and compared the impact of adding different amounts of biochar to the soil for three functionally distinct plants. By using the same soil and biochar from a single source processed in the same manner, we were able to assess the impacts of biochar on different attributes of plant growth among plant types. Our results showed that biochar had a significant positive effect on root, shoot, and fruit biomass in all three species examined. We also documented improved bean germination and earlier date of first flower in green beans. Although all three species responded positively to biochar, we found important and significant differences in response among the growth parameters examined and among the species. This discussion is organized around addressing the questions posed in the introduction of this manuscript.
4.1 How do three functionally distinct plant species differ in their response to different levels of soil biochar?
Our results are consistent with numerous prior studies that that have documented a positive impact of biochar on total biomass in green beans (Melo et al., 2018), tomatoes (Ronga et al., 2020), and willows (Saletnik and Puchalski, 2019), and inconsistent with the smaller number of studies documenting negative or null impacts (Velez et al., 2018). As with total biomass, we observed a significant positive effect of biochar on shoot growth in all three species. These results agree with past studies where others also found increases in shoot biomass in beans and tomatoes (Güereña et al., 2015; Ronga et al., 2020) and showed increases in other aboveground growth parameters, such as stem diameter and plant height in willows (Kuttner and Thomas, 2017).
Our experiment was distinct from prior studies in that it was explicitly designed to compare the impact of biochar among species and among plant tissues. In spite of the fact that we chose three functionally distinct species, we did not find a significant difference among these species in the strength of response of total biomass or stem biomass to biochar additions. Furthermore, in contrast to prior experiments (Rondon et al., 2007; Upadhyay et al., 2014; Fornes et al., 2017), we saw no evidence of a saturating impact of biochar, even at our highest treatment level of 26% biochar. These results suggest that, at least for the particular biochar, soil, and plant species examined, the benefits of biochar are robust.
While the pattern and strength of relationships between biochar addition and total plant biomass and stem biomass was similar among species, important differences were evident when we considered root tissues. Although null impacts of biochar on tomato root growth have been observed (Nzanza et al., 2012), the significant increase in root growth with biochar we observed in all three species is generally consistent with the findings of prior studies that we identified in our review of the literature (Güereña et al., 2015; Vaccari et al., 2015; Lebrun et al., 2017; Velli et al., 2021; Wan et al., 2023). What is distinct about our experimental design is that we were able to document differences in the strength of this relationship among the species examined. Specifically, we found that root tissue in beans and willows exhibited a significantly stronger positive response to biochar addition than did root tissue in tomatoes. Although prior studies have documented increases in root:shoot ratio with biochar application in green beans (Torres et al., 2020) and willows (Seehausen et al., 2017), we observed no significant impact of biochar on root:shoot ratio in beans and willows, and a significant decrease in root:shoot ratio for tomatoes with increasing levels of biochar.
Root production is critical to plants’ capacity to access nutrients and water. One might therefore expect that differences in the root response among the species examined might lead to parallel differences in shoot and fruit production (Wan et al., 2023). This was not, however, the case in our experiment; as already stated, no differences were evident in biochar impacts on shoot production among the three species. Furthermore, no differences were evident in the impact of biochar on total harvested fruit biomass between beans and tomatoes. This lack of difference in shoot and fruit response occurred in spite of the significant decreases in root:shoot ratio of tomatoes with increasing biochar. This is interesting because it indicates that similar benefits of biochar addition on shoot and fruit production in tomatoes versus beans occur in spite of differences in the response of tomato root tissue. The design of our study does not allow us to directly assess physiological mechanisms. Nevertheless, our results suggest that biochar may enhance the capacity of tomato plants to access water and/or nutrients such that at high biochar levels tomato plants are able to access resources necessary to support elevated stem growth and fruit production with a relatively smaller root system. The fact that this same pattern of reduced relative root growth is not evident in beans and willows indicates that the physiological mechanisms associated with the benefits of biochar differ for these different species.
The goal of annual crop production is obviously to produce as much fruit as possible. Although null effects have been documented for soil biochar impact on tomatoes (Dunlop et al., 2015), most prior research reveals beneficial effects of soil biochar on fruit production in beans (Saxena et al., 2013; da Silva et al., 2017) and tomatoes (Ronga et al., 2020; Guo et al., 2021). Our results show that biochar addition had a significant positive impact on fruit production in both beans and tomatoes, but no significant difference in the magnitude of effect between these two plants. At least under our experimental conditions, biochar addition improves fruit yield, but does not affect total fruit production differently in these two species.
One possible explanation for enhanced fruit production in response to biochar would be if biochar resulted in increases in the ratio of fruit:(root+shoot). For example, one study demonstrated an increase in reproductive tissue of oak trees with biochar addition (Ohtsuka et al., 2021). However, we found no overall enhancement of fruit:(root+shoot) ratio with biochar addition in either beans or tomatoes. It appears that the positive impact of biochar on fruit production scales with the increases in overall plant biomass rather than resulting in disproportionate carbon allocation to fruit tissue.
In addition to documenting impacts of biochar on root, shoot and fruit production, we considered biochar impacts on germination in beans and flowering in both beans and tomatoes. Previous research has demonstrated an increased germination rate in green beans with biochar application (Saxena et al., 2013; Velez et al., 2018). Our results are consistent with these findings; we documented a linear increase in bean germination from 79% in the 0% biochar treatment up to a 100% germination rate in our 26% biochar treatment. These results are inconsistent with the findings of Murtaza et al. (2023) that higher rates of biochar application could restrict germination in a variety of plants. We did not identify prior research on the impacts of biochar on the timing of flowering in beans or tomatoes. We observed significantly earlier first flowering in bean plants with increasing soil biochar, with first flowering occurring 8 days earlier in the 26% level than the 0% level. In contrast to beans, in tomato plants we observed no significant impact of biochar addition on the date of first flowering. These results further contribute to our conclusion that while biochar exhibited similar overall positive impacts on the species examined in terms of total biomass and fruit biomass, there were also species-specific differences in the impact of biochar.
4.2 Does the response of willows to biochar change during a second season of growth?
A touted feature of biochar is that it does not decompose as rapidly as other organic soil amendments such as compost and thus could provide a longer term benefit to soil fertility as well as carbon sequestration potential (Kumar et al., 2022). There are two important considerations related to the long term impacts of biochar on fertility. One is the shifting impact of biochar as the material ages and changes in chemical, physical and ecological composition (for example through microbial colonization). The second is how plants rooted in soil containing this biochar change in their response to biochar as the plants themselves mature through various growth stages.
With respect to the aging of the biochar itself, previous studies have shown that positive effects of biochar on soil fertility can either increase over time (Kätterer et al., 2019; Jiang et al., 2022; Wali et al., 2022) or decrease over time (Olszyk et al., 2020). We were not able to identify literature that documented changes in how plants respond to biochar as the plants themselves mature. With annual plants, such as the beans and tomatoes we examined, a full evaluation of the impact of biochar on all life phases can take place over a single growing season. Repeating an experiment such as ours in the same soil with multiple crops of the same species over multiple years would provide a valuable direct assessment of the impact of biochar aging on plant growth. Separating the impacts of biochar aging and shifts in response resulting from changes in plant life stage is more challenging for perennial plants. It is clear that for woody plants such as willows, the longer term impacts are an important consideration that requires multiple seasons to assess.
We conducted a two-year study of willows specifically because we wanted to assess whether response during a second year might differ from response during the first year. During the first year of growth, willows exhibited a highly significant positive response to biochar with respect to shoot growth (stems only), root growth and the combined total biomass of these two tissues (total biomass). Although the trend of positive response of roots, shoots, and total biomass to biochar remained in the second year of growth, the strength of this relationship decreased significantly from year one to year two and was no longer significantly different from zero for any of the variables examined in year two. Thus we observed a significant decrease in the positive impact of biochar between the first and second growth season in willows. Because of the design of our experiment, we are unable to determine whether this decrease in response might be appropriately attributed to aging of the biochar or changes in how the more mature willows were responding to this biochar.
While the decreased response of willows to biochar in year two is an evocative and potentially important finding, we note that three experimental conditions beyond the biochar treatment itself may also play a role in explaining this reduced response. First, it is possible that the stress of having their roots separated as they were transplanted into larger pots for the second year may have reduced the willow’s response. Second, we did not apply microbial inoculation during the second growing season as we had in the first. Our inoculation experiment in the first growing season demonstrated that repeated inoculations had a significant impact on growth in biochar enhanced soil; it may be that additional inoculation in the second year would have enhanced the response to biochar. Third, during the second growing season we fertilized the willows late in July (i.e., middle to late in our growing season) when we noticed that willow leaves were yellowing. In spite of this late fertilization, we documented that willow shoots exhibited significant differences in their response to biochar addition in fertilized vs. fertilized treatments. Specifically, willow shoots in the fertilized group exhibited a significantly more positive response to biochar addition than those in the unfertilized group. This suggests that ample fertilization of all treatments in year one may have been an important factor determining the strong overall response that first year. So, it is possible that the reduction in response to biochar in year two is a result of nutrient limitation and not a change in response to biochar. Further research is definitely warranted to confirm whether the reduced impact of biochar on willow growth during a second season is reproducible as well as the role of microbial inoculation and fertilization in mediating plant response to biochar.
4.3 How does biochar affect the properties of an organic-rich soil and how is this mediated by plants and microbes?
Since our experiment focused on adding different amounts of biochar to soil, the dramatic and consistent positive relationships between biochar addition and plant growth and fertility parameters must be attributed to interactions that occurred within the soil itself. As discussed in our introduction, physicochemical benefits of soil biochar generally include enhanced CEC; increased base cation saturation; decreased bulk density; increased moisture retention; and increased pH (Zhang Y. F. et al., 2021). These direct effects are mediated and potentially augmented by enhanced habitat and resources for a beneficial soil microbial community (Zhu et al., 2017; Tan et al., 2022).
It is important to note that some of the general physicochemical benefits of biochar may have been less important in the soil we constructed for this experiment. For example, the shredded peat moss which made up 50% of our mix already resulted in a soil with a low bulk density, high water retention capacity, and high total cation exchange capacity, even in the absence of biochar. The 50% perlite in our mix ensured high porosity, good drainage and high moisture retention. Furthermore, the application of a time release fertilizer at the start of the experiment was intended to provide soil nutrients throughout the first growing season. We also applied two different sources of microbial inoculants three times over the course of the first growing season. The inoculant suppliers indicated that these contained multiple species of endo- and ecto-mycorrhizae designed to promote plant growth and phosphate solubilization. Thus we generated what we hoped would be a highly fertile organic-rich soil even in the absence of biochar addition.
The soil analysis we conducted must therefore be considered in light of the particular nature of our constructed soil. Some of the changes in soil chemistry resulting from the addition of biochar are as expected. For example, the fact that pH increased in our 26% biochar soils is consistent with the alkaline nature of biochar and most prior literature (Zhang Y. F. et al., 2021; Zhang M. et al., 2021). We were, however, surprised to find a decrease in cation exchange capacity CEC and reduction in percent base saturation in the 26% versus 0% biochar treatments. A partial explanation is that with the very high background CEC provided by the peat moss and high cation concentrations provided by fertilization, the addition of biochar may have made a relatively minor overall contribution to these soil attributes.
Since biochar is a type of organic matter, most studies find that additions of biochar result in an increase in soil carbon (Juriga and Šimanský, 2018). In our experiment we only documented a significant increase in organic matter with biochar addition in the tomato treatment. This finding is likely also attributable to the fact that our experiment was conducted in a soil that was already dominated by organic matter in the form of the peat. We found no overall difference in the plant macronutrients P, Ca, Mg or K in 0 and 26% biochar levels. While significant differences were evident in certain elements among treatments, we believe that the use of a time release fertilizer generally overwhelmed most direct impacts of biochar on soil nutrients.
Of particular note in our soil analyses are the ways in which the different plant treatments affected the soils differently and mediated the impacts of biochar on the soil. For example, in the no plant control treatment five soil properties (pH, percent base saturation, K, Na and B) were significantly different between 0 and 26% biochar treatments. Tomato plants enhanced the degree of difference in soil properties between the two biochar levels; in soils supporting tomatoes, seven soil properties exhibited significant differences. In contrast, beans reduced differences in soil properties between 0 and 26% biochar with only a single soil variable showing differences at the two levels. Furthermore we identified significant differences in soil chemistry among plant treatments in both 0 and 26% biochar treatments. These findings are important because they indicate that the plants (or perhaps symbiotic microbes associated with these plant species) are strongly mediating soil chemistry in general as well as mediating the impact of biochar on the chemical properties of the soil.
Perhaps the most important finding related to analysis of soils in this experiment is that the significant beneficial impacts of biochar on plant growth and production can not be easily attributed to the differences in soil chemistry that we quantified. Indeed, one might expect that the decrease in CEC and percent base cation concentration we observed between the 0 and 26% biochar treatments would be associated with a decrease rather than the observed increase in plant fertility. In short, there is very little evidence that the overwhelmingly positive impacts of biochar on plant fertility observed in our experiment can be directly attributed to measured changes in soil chemistry. While it is possible that we did not measure some important direct physicochemical benefit of biochar, we think it is more reasonable to conclude that the benefits of biochar are attributable to their benefits on the microbial community.
The important role that microbes must have played in enhancing fertility is supported by the positive impact we observed in our side experiment on the impact of microbial inoculation. Previous studies have likewise found that inoculating biochar with microbes or compost improves fertility (Castro et al., 2018; Sani et al., 2020). We found that multiple inoculations enhanced shoot, fruit, and total biomass production in beans and root biomass in tomatoes. Taken together, our soil analysis and inoculation experiment both point toward the importance of microbial communities in mediating the impacts of biochar on plant fertility.
5 Conclusion and suggestions for future research
We documented significant positive impacts of increasing levels of soil biochar on root, fruit and shoot production in three distinct plant species. We further documented enhanced germination and earlier flowering in beans with increasing biochar. These findings contribute to, but are also largely consistent with prior research. The novel contribution of this research is an experimental design that allowed us to directly compare the response of plant tissues in three economically and functionally distinct plants by controlling for both soil type and biochar source. Using this approach we found that while overall impacts and effects sizes of biochar addition were generally similar among these three species, biochar addition had significantly different impacts on the different species and, in some cases, species-specific impacts on different plant tissues and other measures of fertility. The physiological and/or ecological mechanisms responsible for these differences warrant further study.
Some of our results are inconsistent with prior research. Differences may stem, in part, from the fact that our study was conducted in a highly organic soil (composed of 50% peat). For example, while numerous studies have documented inhibiting effects of biochar at high levels comparable to our 26% treatment, we documented positive effects even at this highest level. It may be that inhibitory effects are a function of the mineral content in soils. We also did not reproduce many of the enhanced physicochemical properties typically attributed to biochar such as enhanced CEC and enhanced base cation saturation. This again may be attributable to overlapping physicochemical benefits of biochar and fertilized soil that is already rich in organic matter.
In some ways, the lack of substantial differences in measured chemical properties in control soil, which contained 0% biochar, and our highest biochar treatment (26%) may serve to highlight other mechanisms by which biochar may enhance soil fertility. Specifically, the highly significant impacts of biochar observed in the absence of substantial differences in soil chemistry lead us to conclude that microbial interaction with biochar is the critical factor explaining the positive impacts of soil biochar on plant fertility in our experiment.
Our study was conducted in a highly organic, constructed soil with hazelnut wood biochar; further research that controls for soil type and biochar source is necessary to determine the extent to which our findings apply for other biochar sources in other soil types and for other species of plants. Based on our findings we suggest that future work should examine the impact of biochar on several variables that we did not measure including: stem versus leaf growth, nutritional value of fruit (sugars, nutrient concentration, antioxidant properties); microbial dynamics in the soil, changes in the chemistry of biochar and the microbial community that occur as biochar ages; changes in perennial species response to biochar at different life stages; interactions between fertilization and biochar. Future studies should also strive to better characterize the chemical properties of the biochar used as well as the microbial community present in the soil.
Data availability statement
The raw data supporting the conclusions of this article will be made available by the authors, without undue reservation.
Author contributions
SS: Conceptualization, Data curation, Formal analysis, Investigation, Methodology, Writing – original draft, Writing – review & editing. TH: Conceptualization, Formal analysis, Investigation, Methodology, Writing – original draft, Writing – review & editing. JP: Conceptualization, Formal analysis, Funding acquisition, Methodology, Resources, Supervision, Visualization, Writing – original draft, Writing – review & editing.
Funding
The author(s) declare financial support was received for the research, authorship, and/or publication of this article. This research was funded by Oberlin College’s Environmental Studies Program.
Acknowledgments
We would like to start by thanking Oberlin College Environmental Studies Program as well as Ben Hobbs for their provision of funding, space, and laboratory equipment. Hillel Hinton-Williams provided us the invaluable service of supervising the experimental system over our September break and ensuring the continuity of measurements. We would also like to thank members of Oberlin College’s Ecosystems Ecology class (ENVS316) for their aid in data collection and analysis, specifically, Grace Gao, Aidan Kirchgraber, and Stephanie Macedo for their work in plant tissue analysis and Elise Steenburgh, QiaoHui Zhang, and Sophia Cartsonis for their work in soil analysis. Many friends and colleagues helped with this project, whether through being a sounding board for ideas or holding a flashlight during an evening plant measurement. Cindy Frantz helped us to conceptualize our method of statistical analysis and Jeff Witmer provided consultation on creating and conducting this analysis in RStudio; both of their help was essential for analyzing our results and conceptualizing our findings. We greatly appreciate Roger Laushman and Ben Hobbs for serving on our honors committee and providing us with their valuable feedback.
Conflict of interest
The authors declare that the research was conducted in the absence of any commercial or financial relationships that could be construed as a potential conflict of interest.
Publisher’s note
All claims expressed in this article are solely those of the authors and do not necessarily represent those of their affiliated organizations, or those of the publisher, the editors and the reviewers. Any product that may be evaluated in this article, or claim that may be made by its manufacturer, is not guaranteed or endorsed by the publisher.
Footnotes
References
Akhtar, S. S., Li, G. T., Andersen, M. N., and Liu, F. L. (2014). Biochar enhances yield and quality of tomato under reduced irrigation. Agric. Water Manag. 138, 37–44. doi: 10.1016/j.agwat.2014.02.016
Bo, X., Zhang, Z., Wang, J., Guo, S., Li, Z., Lin, H., et al. (2023). Benefits and limitations of biochar for climate-smart agriculture: a review and case study from China. Biochar 5:77. doi: 10.1007/s42773-023-00279-x
Břendová, K., Tlustoš, P., and Száková, J. (2015). Biochar immobilizes cadmium and zinc and improves phytoextraction potential of willow plants on extremely contaminated soil. Plant Soil Environ. 61, 303–308. doi: 10.17221/181/2015-PSE
Butnan, S. J. L., Deenik, B., Toomsan, B., Antal, M. J., and Vityakon, P. (2015). Biochar characteristics and application rates affecting corn growth and properties of soils contrasting in texture and mineralogy. Geoderma 237-238, 105–116. doi: 10.1016/j.geoderma.2014.08.010
Castro, A., Batista, N. D., Latawiec, A. E., Rodrigues, A., Strassburg, B., Silva, D., et al. (2018). The effects of gliricidia-derived biochar on sequential maize and bean farming. Sustain. For. 10:578. doi: 10.3390/su10030578
Chan, K., Van Zwieten, L., Meszaros, I., Downie, A., and Joseph, S. (2008). Using poultry litter biochars as soil amendments. Aust. J. Soil Res. 46, 437–444. doi: 10.1071/SR08036
da Silva, I., Fernandes, L., Colen, F., and Sampaio, R. (2017). Growth and production of common bean fertilized with biochar. Cienc. Rural 47. doi: 10.1590/0103-8478cr20170220
Dunlop, S. J., Arbestain, M. C., Bishop, P. A., and Wargent, J. J. (2015). Closing the loop: use of biochar produced from tomato crop green waste as a substrate for soilless, hydroponic tomato production. HortScience 50, 1572–1581. doi: 10.21273/HORTSCI.50.10.1572
Elad, Y., Cytryn, E., Harel, Y. M., Lew, B., and Graber, E. R. (2011). The biochar effect: plant resistance to biotic stresses. Phytopathol. Mediterr. 50, 335–349. doi: 10.14601/Phytopathol_Mediterr-9807
Fornes, F., Belda, R. M., de Cordova, P. F., and Cebolla-Cornejo, J. (2017). Assessment of biochar and hydrochar as minor to major constituents of growing media for containerized tomato production. J. Sci. Food Agric. 97, 3675–3684. doi: 10.1002/jsfa.8227
Gao, S., Hoffman-Krull, K., Bidwell, A. L., and Deluca, T. H. (2016). Locally produced wood biochar increases nutrient retention and availability in agricultural soils of the San Juan Islands, USA. Agric. Ecosyst. Environ. 233, 43–54. doi: 10.1016/j.agee.2016.08.028
Ghorbani, M., Konvalina, P., Neugschwandtner, R. W., Soja, G., Bárta, J., Chen, W., et al. (2024). How do different feedstocks and pyrolysis conditions effectively change biochar modification scenarios? A critical analysis of engineered biochars under H2O2 oxidation. Energy Convers. Manag. 300:117924. doi: 10.1016/j.enconman.2023.117924
Graber, E. R., Harel, Y. M., Kolton, M., Cytryn, E., Silber, A., David, D. R., et al. (2010). Biochar impact on development and productivity of pepper and tomato grown in fertigated soilless media. Plant Soil 337, 481–496. doi: 10.1007/s11104-010-0544-6
Güereña, D. T., Lehmann, J., Thies, J. E., Enders, A., Karanja, N., and Neufeldt, H. (2015). Partitioning the contributions of biochar properties to enhanced biological nitrogen fixation in common bean (Phaseolus vulgaris). Biol. Fertil. Soils 51, 479–491. doi: 10.1007/s00374-014-0990-z
Guo, L. L., Yu, H. W., Kharbach, M., Zhang, W. Q., Wang, J. W., and Niu, W. Q. (2021). Biochar improves soil-tomato plant, tomato production, and economic benefits under reduced nitrogen application in northwestern China. Plants 10:759. doi: 10.3390/plants10040759
Haider, F. U., Ain, N. U., Khan, I., Farooq, M., Habiba,, Cai, L., et al. (2024). Co-application of biochar and plant growth regulators improves maize growth and decreases cd accumulation in cadmium-contaminated soil. J. Clean. Prod. 440:140515. doi: 10.1016/j.jclepro.2023.140515
Haider, F. U., Coulter, J. A., Cai, L., Hussain, S., Cheema, S. A., Wu, J., et al. (2022). An overview on biochar production, its implications, and mechanisms of biochar-induced amelioration of soil and plant characteristics. Pedosphere 32, 107–130. doi: 10.1016/S1002-0160(20)60094-7
Hairani, A., Osaki, M., and Watanabe, T. (2016). Effect of biochar application on mineral and microbial properties of soils growing different plant species. Soil Sci. Plant Nutr. 62, 519–525. doi: 10.1080/00380768.2016.1212648
Inal, A., Gunes, A., Sahin, O., Taskin, M. B., and Kaya, E. C. (2015). Impacts of biochar and processed poultry manure, applied to a calcareous soil, on the growth of bean and maize. Soil Use Manag. 31, 106–113. doi: 10.1111/sum.12162
Jiang, R. W., Mechler, M. A. A., and Oelbermann, M. (2022). Softwood biochar and greenhouse gas emissions: a field study over three growing seasons on a temperate agricultural soil. Can. J. Soil Sci. 102, 197–211. doi: 10.1139/cjss-2021-0160
Juriga, M., and Šimanský, V. (2018). Effect of biochar on soil structure – review. Acta Fytotechn Zootechn 21, 11–19. doi: 10.15414/afz.2018.21.01.11-19
Karp, A., and Shield, I. (2008). Bioenergy from plants and the sustainable yield challenge. New Phytol. 179, 15–32. doi: 10.1111/j.1469-8137.2008.02432.x
Kätterer, T., Roobroeck, D., Andrén, O., Kimutai, G., Karltun, E., Kirchmann, H., et al. (2019). Biochar addition persistently increased soil fertility and yields in maize-soybean rotations over 10 years in sub-humid regions of Kenya. Field Crop Res. 235, 18–26. doi: 10.1016/j.fcr.2019.02.015
Khan, M., Fatima, K., Ahmad, R., Younas, R., Rizwan, M., Azam, M., et al. (2019). Comparative effect of mesquite biochar, farmyard manure, and chemical fertilizers on soil fertility and growth of onion (Allium cepa L.). Arab. J. Geosci. 12:563. doi: 10.1007/s12517-019-4734-0
Kumar, A., and Bhattacharya, T. (2021). Biochar: a sustainable solution. Environ. Dev. Sustain. 23, 6642–6680. doi: 10.1007/s10668-020-00970-0
Kumar, A., Bhattacharya, T., Mukherjee, S., and Sarkar, B. (2022). A perspective on biochar for repairing damages in the soil–plant system caused by climate change-driven extreme weather events. Biochar 4:22. doi: 10.1007/s42773-022-00148-z
Kumar, A., Bhattacharya, T., Saikh, W. A., Roy, A., Cakraborty, S., Vithanage, M., et al. (2023). Multifaceted applications of biochar in environmental management: a bibliometric profile. Biochar 5:11. doi: 10.1007/s42773-023-00207-z
Kuttner, B. G., and Thomas, S. C. (2017). Interactive effects of biochar and an organic dust suppressant for revegetation and erosion control with herbaceous seed mixtures and willow cuttings. Restor. Ecol. 25, 367–375. doi: 10.1111/rec.12439
Lebrun, M., Macri, C., Miard, F., Hattab-Hambli, N., Motelica-Heino, M., Morabito, D., et al. (2017). Effect of biochar amendments on as and Pb mobility and phytoavailability in contaminated mine technosols phytoremediated by Salix. J. Geochem. Explor. 182, 149–156. doi: 10.1016/j.gexplo.2016.11.016
Lebrun, M., Miard, F., Nandillon, R., Scippa, G. S., Bourgerie, S., and Morabito, D. (2019). Biochar effect associated with compost and iron to promote Pb and as soil stabilization and Salix viminalis L. growth. Chemosphere 222, 810–822. doi: 10.1016/j.chemosphere.2019.01.188
Lefebvre, D., Román-Dañobeytia, F., Soete, J., Cabanillas, R., Corvera, R., Ascorra, C., et al. (2019). Biochar effects on two tropical tree species and its potential as a tool for reforestation. Forests 10:678. doi: 10.3390/f10080678
Lehmann, J., Rillig, M. C., Thies, J., Masiello, C. A., Hockaday, W. C., and Crowley, D. (2011). Biochar effects on soil biota – a review. Soil Biol. Biochem. 43, 1812–1836. doi: 10.1016/j.soilbio.2011.04.022
Li, X., Yao, T. X., Huang, X. X., Li, X. B., Li, P. Y., Du, S., et al. (2022). Biochar increases rice yield by improving root morphological and root physiological functions in heavily saline-sodic paddy soil of Northeast China. Bioresources 17, 1241–1256. doi: 10.15376/biores.17.1.1241-1256
Liao, H. K., Zheng, C. L., Long, J., and Guzman, I. (2021). Effects of biochar amendment on tomato rhizosphere bacterial communities and their utilization of plant-derived carbon in a calcareous soil. Geoderma 396:115082. doi: 10.1016/j.geoderma.2021.115082
Manolikaki, I., and Diamadopoulos, E. (2016). Ryegrass yield and nutrient status after biochar application in two Mediterranean soils. Arch. Agron. Soil Sci. 63, 1093–1107. doi: 10.1080/03650340.2016.1267341
Manolikaki, I., and Diamadopoulos, E. (2018). Positive effects of biochar and biochar-compost on maize growth and nutrient availability in two agricultural soils. Commun. Soil Sci. Plant Anal. 50, 512–526. doi: 10.1080/00103624.2019.1566468
Melo, T. M., Bottlinger, M., Schulz, E., Leandro, W. M., Filho, A. M. A., Wang, H., et al. (2018). Plant and soil responses to hydrothermally converted sewage sludge (sewchar). Chemosphere 206, 338–348. doi: 10.1016/j.chemosphere.2018.04.178
Mokarram-Kashtiban, S., Hosseini, S. M., Kouchaksaraei, M. T., and Younesi, H. (2019). Biochar improves the morphological, physiological and biochemical properties of white willow seedlings in heavy metal-contaminated soil. Arch. Biol. Sci. 71, 281–291. doi: 10.2298/ABS180918010M
Murtaza, G., Ahmed, Z., Eldin, S. M., Ali, B., Bawazeer, S., Usman, M., et al. (2023). Biochar-soil-plant interactions: a cross talk for sustainable agriculture under changing climate. Frontiers in environmental. Science 11:1059449. doi: 10.3389/fenvs.2023.1059449
NCR-13 Soil Testing and Plant Analysis Committee (1998). Recommended chemical soil test procedures for the north central region. Missouri Agricultural Experiment Station SB 1001, North Central Regional Research. Publication 221.
Nzanza, B., Marais, D., and Soundy, P. (2012). Effect of arbuscular mycorrhizal fungal inoculation and biochar amendment on growth and yield of tomato. Int. J. Agric. Biol. 14, 965–969.
Ohtsuka, T., Tomotsune, M., Ando, M., Tsukimori, Y., Koizumi, H., and Yoshitake, S. (2021). Effects of the application of biochar to plant growth and net primary production in an oak forest. Forests 12:152. doi: 10.3390/f12020152
Olszyk, D., Shiroyama, T., Novak, J., Cantrell, K., Sigua, G., Watts, D., et al. (2020). Biochar affects growth and shoot nitrogen in four crops for two soils. Agrosyst. Geosci. Environ. 3, 1–22. doi: 10.1002/agg2.20067
Rajkovich, S., Enders, A., Hanley, K., Hyland, C., Zimmerman, A. R., and Lehmann, J. (2012). Corn growth and nitrogen nutrition after additions of biochars with varying properties to a temperate soil. Biol. Fertil. Soils 48, 271–284. doi: 10.1007/s00374-011-0624-7
Rondon, M. A., Lehmann, J., Ramírez, J., and Hurtado, M. (2007). Biological nitrogen fixation by common beans (Phaseolus vulgaris L.) increases with bio-char additions. Biol. Fertil. Soils 43, 699–708. doi: 10.1007/s00374-006-0152-z
Ronga, D., Caradonia, F., Parisi, M., Bezzi, G., Parisi, B., Allesina, G., et al. (2020). Using digestate and biochar as fertilizers to improve processing tomato production sustainability. Agronomy 10:138. doi: 10.3390/agronomy10010138
Ronsee, F., Van Hecke, S., Dickinson, D., and Prins, W. (2012). Production and characterization of slow pyrolysis biochar: influence of feedstock type and pyrolysis conditions. GCB Bioenergy 5, 104–115. doi: 10.1111/gcbb.12018
Saletnik, B., and Puchalski, C. (2019). Suitability of biochar and biomass ash in basket willow (Salix viminalis L.) cultivation. Agronomy 9:577. doi: 10.3390/agronomy9100577
Sani, M. N. H., Hasan, M., Uddain, J., and Subramaniam, S. (2020). Impact of application of Trichoderma and biochar on growth, productivity and nutritional quality of tomato under reduced N-P-K fertilization. Ann. Agric. Sci. 65, 107–115. doi: 10.1016/j.aoas.2020.06.003
Saxena, J., Rana, G., and Pandey, M. (2013). Impact of addition of biochar along with Bacillus sp. on growth and yield of French beans. Sci. Hortic. 162, 351–356. doi: 10.1016/j.scienta.2013.08.002
Seehausen, M. L., Gale, N. V., Dranga, S., Hudson, V., Liu, N., Michener, J., et al. (2017). Is there a positive synergistic effect of biochar and compost soil amendments on plant growth and physiological performance? Agronomy 7:13. doi: 10.3390/agronomy7010013
Singh, H., Northup, B. K., Rice, C. W., and Prasad, P. V. V. (2022). Biochar applications infuence soil physical and chemical properties, microbial diversity, and crop productivity: a meta-analysis. Biochar 4:3. doi: 10.1007/s42773-022-00138-1
Tan, S., Narayanan, M., Thu Huong, D. T., Ito, N., Unpaprom, Y., Pugazhendhi, A., et al. (2022). A perspective on the interaction between biochar and soil microbes: a way to regain soil eminence. Environ. Res. 214:113832. doi: 10.1016/j.envres.2022.113832
Tartaglia, M., Arena, S., Scaloni, A., Marra, M., and Rocco, M. (2020). Biochar administration to san Marzano tomato plants cultivated under low-input farming increases growth, fruit yield, and affects gene expression. Front. Plant Sci. 11:1281. doi: 10.3389/fpls.2020.01281
Thomas, S. C., Frye, S., Gale, N., Garmon, M., Launchbury, R., Machado, N., et al. (2013). Biochar mitigates negative effects of salt additions on two herbaceous plant species. J. Environ. Manag. 129, 62–68. doi: 10.1016/j.jenvman.2013.05.057
Torres, W. G. A., Colen, F., Pandey, S. D., Frazão, L. A., Sampaio, R. A., and Fernandes, L. A. (2020). Phosphorus availability in soil amended with biochar from rice rusk and cattle manure and cultivated with common bean. Sci. Agrotechnol. 44, 1–10. doi: 10.1590/1413-7054202044014620
Upadhyay, K. P., George, D., Swift, R. S., and Galea, V. (2014). The influence of biochar on growth of lettuce and potato. J. Integr. Agric. 13, 541–546. doi: 10.1016/S2095-3119(13)60710-8
Vaccari, F. P., Baronti, S., Lugato, E., Genesio, L., Castaldi, S., Fornasier, F., et al. (2011). Biochar as a strategy to sequester carbon and increase yield in durum wheat. Eur. J. Agron. 34, 231–238. doi: 10.1016/j.eja.2011.01.006
Vaccari, F. P., Maienza, A., Miglietta, F., Baronti, S., Di Lonardo, S., Giagnoni, L., et al. (2015). Biochar stimulates plant growth but not fruit yield of processing tomato in a fertile soil. Agric. Ecosyst. Environ. 207, 163–170. doi: 10.1016/j.agee.2015.04.015
Vanapalli, K. R., Samal, B., Dubey, B. K., and Bhattacharya, J. (2021). Chapter nine - biochar for sustainable agriculture: prospects and implications. Adv. Chem. Pollut. Environ. Manag. Prot. 7, 221–262. doi: 10.1016/bs.apmp.2021.08.008
Vaughn, S. F., Byars, J. A., Jackson, M. A., Peterson, S. C., and Eller, F. J. (2021). Tomato seed germination and transplant growth in a commercial potting substrate amended with nutrient-preconditioned eastern red cedar (Juniperus virginiana L.) wood biochar. Sci. Hortic. 280:109947. doi: 10.1016/j.scienta.2021.109947
Velez, T., Moonilall, N., Reed, S., Jayachandran, K., and Scinto, L. (2018). Impact of Melaleuca quinquenervia biochar on Phaseolus vulgaris growth, soil nutrients, and microbial gas flux. J. Environ. Qual. 47, 1487–1495. doi: 10.2134/jeq2017.12.0484
Velli, P., Manolikaki, I., and Diamadopoulos, E. (2021). Effect of biochar produced from sewage sludge on tomato (Solanum lycopersicum L.) growth, soil chemical properties and heavy metal concentrations. J. Environ. Manag. 297:113325. doi: 10.1016/j.jenvman.2021.113325
von Glisczynski, F., Pude, R., Amelung, W., and Sandhage-Hofmann, A. (2016). Biochar-compost substrates in short-rotation coppice: effects on soil and trees in a three-year field experiment. J. Plant Nutr. Soil Sci. 179, 574–583. doi: 10.1002/jpln.201500545
Wali, F., Sardar, S., Naveed, M., Asif, M., Nezhad, M. T. K., Baig, K. S., et al. (2022). Effect of consecutive application of phosphorus-enriched biochar with different levels of P on growth performance of maize for two successive growing seasons. Sustainability 14:1987. doi: 10.3390/su14041987
Wan, H., Liu, X., Shi, Q., Chen, Y., Jiang, M., Zhang, J., et al. (2023). Biochar amendment alters root morphology of maize plant: its implications in enhancing nutrient uptake and shoot growth under reduced irrigation regimes. Front. Plant Sci. 14:1122742. doi: 10.3389/fpls.2023.1122742
Wijitkosum, S. (2021). Biochar derived from agricultural wastes and wood residues for sustainable agricultural and environmental applications. Int. Soil Water Conserv. Res. 10, 335–341. doi: 10.1016/j.iswcr.2021.09.006
Xu, P., Gao, Y., Cui, Z., Wu, B., Yan, B., Wang, Y., et al. (2023). Research progress on effects of biochar on soil environment and crop nutrient absorption and utilization. Sustainability 15:4861. doi: 10.3390/su15064861
Yang, L., Liao, F., Huang, M., Yang, L. T., and Li, Y. R. (2015). Biochar improves sugarcane seedling root and soil properties under a pot experiment. Sugar Tech. 17, 36–40. doi: 10.1007/s12355-014-0335-0
Yu, P., Li, Q. S., Huang, L., Niu, G. H., and Gu, M. M. (2019). Mixed hardwood and sugarcane bagasse biochar as potting mix components for container tomato and basil seedling production. Appl. Sci. 9:4713. doi: 10.3390/app9214713
Zhang, Y. F., Wang, J. M., and Feng, Y. (2021). The effects of biochar addition on soil physicochemical properties: a review. Catena 202:105284. doi: 10.1016/j.catena.2021.105284
Zhang, M., Zhang, L., Riaz, M., Xia, H., and Jiang, C. (2021). Biochar amendment improved fruit quality and soil properties and microbial communities at different depths in citrus production. J. Clean. Prod. 292:126062. doi: 10.1016/j.jclepro.2021.126062
Keywords: plant tissue, soil fertility, carbon sequestration, Salix, plant growth
Citation: Sheffield SB, Hoefer TA and Petersen JE (2024) Biochar has positive but distinct impacts on root, shoot, and fruit production in beans, tomatoes, and willows. Front. Sustain. Food Syst. 8:1346529. doi: 10.3389/fsufs.2024.1346529
Edited by:
Mohamed Ait-El-Mokhtar, University of Hassan II Casablanca, MoroccoReviewed by:
Abhishek Kumar, University of California, Davis, United StatesMohammad Ghorbani, University of South Bohemia in České Budějovice, Czechia
Fasih Ullah Haider, Chinese Academy of Sciences (CAS), China
Copyright © 2024 Sheffield, Hoefer and Petersen. This is an open-access article distributed under the terms of the Creative Commons Attribution License (CC BY). The use, distribution or reproduction in other forums is permitted, provided the original author(s) and the copyright owner(s) are credited and that the original publication in this journal is cited, in accordance with accepted academic practice. No use, distribution or reproduction is permitted which does not comply with these terms.
*Correspondence: John E. Petersen, am9obi5wZXRlcnNlbkBvYmVybGluLmVkdQ==
†These authors have contributed equally to this work