- 1Department of Fisheries and Wildlife, Oregon State University, Corvallis, OR, United States
- 2Department of Natural Resources and the Environment, University of Connecticut, Storrs, CT, United States
Changing patterns of precipitation and drought will dramatically influence the distribution and persistence of lentic habitats. Pond-breeding amphibians can often respond to changes in habitat by plastically shifting behavioral and developmental trait response. However, fitness tradeoffs inherent in life history strategies can carry over to impact development, behavior, and fitness in later life stages. In this experiment, we investigated carryover effects of hydroperiod permanence on the movement behavior of newly-metamorphosed juvenile Northern Red-legged Frogs (Rana aurora). Frogs were raised through metamorphosis in mesocosms under either permanent or ephemeral hydroperiod conditions. After metamorphosis, individuals were removed from the mesocosms, measured, uniquely tagged with elastomer, and moved to holding terrariums. Movement behavior was quantified under two terrain conditions: a physiologically-taxing, dry runway treatment, or a control, moist runway treatment. Individuals were given 30 min to move down the 1 × 20m enclosed structure before distance was measured. We applied a hurdle model to examine two distinct components of movement behavior: (1) the probability of moving away from the start location, and (2) movement distance. We found that hydroperiod condition had an indirect carryover effect on movement via the relationship between individual size and the propensity to move. Individuals from ephemeral mesocosm conditions metamorphosed at a smaller size but showed increased growth rates as compared to individuals from permanent hydroperiod conditions. Individual snout-vent length and runway condition (moist or dry) were significant predictors of both aspects of movement behavior. Larger individuals were more likely to move down the runway and able to move a farther distance than smaller individuals. In addition to the influence of size, dry runway conditions reduced the probability of individuals moving from the start location, but increased the distance traveled relative to the moist runway. The demonstrated cumulative impact of stressors suggests the importance of addressing direct, indirect, and carryover effects of stressors throughout ontogeny.
Introduction
Climate change is projected to influence many aspects of the environment, including temperature and storm intensity (Hughes, 2000). Critical to lentic organisms, climate change also alters precipitation patterns, which are key in the predictability and variability of water cycling and storage (Williams et al., 2015; Smith et al., 2018). For animals such as aquatic insects and amphibians, with complex life histories that require movement between aquatic and terrestrial habitats, this variation can determine the success of individual development and survival (Robson et al., 2011; Suislepp et al., 2011; Cayuela et al., 2016).
Life history theory suggests that a dynamic yet predictable environment would select for a flexible life history strategy, such as life-cycle staging or phenotypic plasticity (Meyers and Bull, 2002; Roff, 2002). Developmental plasticity has allowed some amphibian species with complex life histories to adjust trait response, such as development rate, antipredator behavior, and metamorphosis timing in response to a variety of aquatic environmental stressors (desiccation—Denver et al., 1998; competition and predation—Relyea, 2004; multiple predators—Vonesh and Warkentin, 2006; temperature—Thurman and Garcia, 2017). However, plasticity has physiological costs and trade-offs that can constrain the degree of response both immediately and across ontogeny (Relyea, 2002). The true price of plasticity may only be understood when considering the entire life cycle.
Carryover effects, also termed latent effects, have been increasingly apparent in biological systems (Pechenik, 2006; O'connor et al., 2014). Carryover effects of larval plasticity may be important in determining response to changing environmental conditions (Johansson et al., 2010). Early life stages can be more susceptible to shifting environmental conditions than adults, an effect that has been observed in diverse taxa from reptiles to butterflies (Radchuk et al., 2013; Levy et al., 2015). Several carryover effects to larval stress in anurans have been observed: for example, drying conditions experienced by developing Túngara frog tadpoles reduced leg length and jumping performance (Charbonnier and Vonesh, 2015), and high larval densities of Red-eyed treefrogs resulted in smaller juveniles with reduced livers and fat bodies (Bouchard et al., 2016).
To date, assessment of potential impacts of climate change on amphibians have focused on adult stages, with little attention to other sensitive life history stages or across ontogeny (Levy et al., 2015; Lancaster et al., 2017). Dramatic shifts in environmental conditions that are stressors during early life stages may fundamentally change the ability of individuals to respond to stress later in development. The physiological changes and energy investment in larval trait response can represent an opportunity cost that limits the ability to respond to future stressors (Weinig and Delph, 2001; Amburgey et al., 2016). As such, investments in plastic responses at the larval, aquatic stage have the potential to carry over to influence the developmental trajectory and behavior of juvenile frogs, and limit capacity to manage stress in terrestrial life stages.
We studied the combined influence of aquatic and terrestrial environmental condition across a life history transition in the Northern Red-legged Frog (Rana aurora) to examine the interaction of direct and carryover effects on juvenile movement behavior. We chose movement behavior as our response variable because of its importance in a range of ecological aspects, such as foraging, predator avoidance, refuge discovery, and population connectivity (Osbourn et al., 2014; Schwalm et al., 2016; Székely et al., 2017). We selected pond permanence as a larval stressor because of the extensive documentation of amphibian plasticity in response to drying conditions (Johansson et al., 2010; Charbonnier and Vonesh, 2015; Thurman and Garcia, 2017). Rana aurora utilizes both ephemeral and permanent lentic waters for breeding habitat, with ephemeral ponds being important for larval success (Adams, 2000; Hayes et al., 2008). After metamorphosis, we tested for carryover effects on juvenile movement behavior under two physiologically distinct conditions: a moist, low-stress terrain or a dry, high-stress terrain. Based on past research (see above), we hypothesized that individuals who (plastically) responded to drying (ephemeral) hydroperiods would be less suited to movement under stressful conditions. A trade-off between structural growth and energy stores (Morey and Reznick, 2004) suggests that larval development has inherent resource limitations. The increased density and limitations of ephemeral conditions may tax this trade-off and change the physiology of juvenile frogs and their subsequent movement behavior.
Amphibians are species that are dependent on water throughout their life cycle. Yet, to our knowledge, impacts of 2 critical aspects of amphibian habitat affected by changing precipitation—drying ponds and dry terrain—have not been directly assessed for amphibian species across metamorphosis. As climate change influences precipitation patterns and the availability of moisture in the environment, the mechanisms by which amphibians modify their development, manage the physiological stress, and respond behaviorally to these changing conditions will be important for continued survival of those species (Mazerolle and Desrochers, 2005; Watling and Braga, 2015).
Methods
Animal Collection and Rearing
On 31 January 2015, we collected eight recently laid R. aurora egg masses from three separate populations in the Willamette Valley, Oregon. Egg masses were immediately transported to Oregon State University and placed in de-chlorinated water in individual 75 L glass aquaria. Individuals were reared in the laboratory through hatching until Gosner developmental stage 25 (Gosner, 1960) and fed a mixture of ground fish flakes and rabbit chow ad libidum. Water changes were performed every 10 days. On 3 March 2015, tadpoles were combined into a common pool and randomly assigned to a mesocosm tub. Each mesocosm was populated with 50 randomly selected larvae, with 10 replicates per hydroperiod treatment (permanent or ephemeral). Mesocosms were held outdoors at the Lewis Brown Horticultural Farm (44.551346, −123.215831) under a mixed oak canopy. Mesocosms (120 L HDPE stock tanks) were prepared with 100 grams of dried and autoclaved oak leaves and 5 grams of rabbit chow in 100 liters of well-water. Each mesocosm tub was then inoculated with algae and microorganism communities 2 weeks prior to the introduction of tadpoles using 4 L of water from an onsite source-mesocosm. Mesocosm tubs were then fitted with mesh screen lids to prevent predation or introduction of other species.
Two weeks after the introduction of R. aurora larvae, water levels in the ephemeral treatment mesocosms were drawn down by 4 liters every week until water levels reached a total 30 liters. At this point, water levels were maintained for the remainder of the experiment. Permanent hydroperiod treatments were maintained at 100-liter levels with additional well-water every week as needed. As individuals neared metamorphosis, two mesh floats were added to mesocosms to allow individuals to emerge. Individuals were removed from the mesocosms at Gosner developmental stage 45 (Gosner, 1960), measured (snout-vent length, total length, and mass), and marked with a unique color tag. Color tags consisted of 3 subcutaneous injections of visible implant elastomer (Northwest Marine Technology, Inc.) in the belly, right leg, and left leg (Govindarajulu et al., 2006; Swanson et al., 2013). Individuals were then transferred to outdoor terrariums adjacent to the mesocosm array. Treatment groupings were maintained throughout this process. Terrariums (120 L HDPE tubs) contained moistened coconut husk bedding, well-water pools, and dried grass and leaves for refuge. Individuals in terrariums were fed an excess of wingless fruit flies (Drosophila melanogaster) 3 times a week. Emerging individuals were collected starting on 25 June and continued until we collected at least 200 individuals which occurred on 14 September. Logistical seasonal weather constraints of our runway assays prevented the inclusion of individuals that metamorphosed after our collection window, which does limit our inference to those early emerging individuals. This is an important consideration as emergence date has shown to change the size and dispersal of individuals (Chelgren et al., 2006).
Behavioral Assay- Runway Dispersal
Four 1 × 20 m runways were constructed at the Lewis Brown Horticultural Farm. Each runway was constructed from a single piece of 7-millimeter white plastic sheet draped across parallel wires suspended 75 centimeters above the perimeter of the runways. This plastic sheeting prevented individuals from escaping the runways and maintained moisture conditions within assays. The runway substrate consisted of smoothed and compacted topsoil to a depth of 7 cm. The 4 runways were arranged in pairs and placed on level ground under the dappled shade of several surrounding trees on an angle from northeast to southwest. Runways were paired, with each pair containing a dry and wet treatment runway.
Immediately prior to each assay, all runways were prepared according to their assigned moisture treatment. Wet condition runways had the entire length of substrate moistened with well-water to the point of near saturation to minimize standing pooled water. Dry condition runways had no added water except for the release point. In each runway, a release point was constructed by placing a 15 cm disk of moist sphagnum moss in the northeast end of the runs. The immediate area around this release point was gently moistened in both the wet and dry conditions to provide a place of hydrologic refuge. Runways were cleaned of detritus prior to each assay and the release point moss was replaced each day.
Individuals from a single terrarium were ordered by age and alternately assigned to runway condition to provide equal representation across ages and terrariums. Each animal was measured (snout-vent length, mass, etc.) and then allowed to acclimate within the runway under a clay pot cover in the center of the release point. Individuals were anointed with a small application of high visibility fluorescent powder (ECO Aurora Pink® Pigment, DayGlow Color Corp.) on their back. After a 5-min acclimation period, the clay pot was remotely removed, and the animal was given 30 min to freely move in the runway. Runway assays occurred during daylight-hours; average start time was at 13:25 h (SD = 1:45 h) and ranged from 08:25 to 15:40h. At the end of the assay, animals were located, recaptured, and returned to their terrarium. In between each assay, the moss disk at the release point was rinsed and immediate area remoistened. Wet runways were also remoistened as needed in between each assay. Each animal was run through the assay twice with at least 14 days between each run. Animals assigned to a runway condition were maintained in the same runway condition for their second assay. At the end of the second runway assay, individuals were humanely euthanized with a solution of Tricaine Methanesulfonate (Leary et al., 2013).
Statistical Analysis
We examined the relationship between larval hydroperiod and frog body size using two models to assess the responses of individual length at two time points: once upon metamorphic emergence from the mesocosms, and once at the time of the runway assay (juvenile growth). To assess potential carryover effects of hydroperiod conditions on frog size, we fit a linear mixed-effects regression model of metamorphic emergence snout-vent length (SVL) based on mesocosm hydroperiod, day of emergence, and a random effect of mesocosm tub. To assess carry over on juvenile growth, we built a second linear mixed-effects model that examined SVL growth after metamorphic emergence and before the first the runway assay. For this model, we fit data from the first assays with predictor variables of mesocosm hydroperiod, day of emergence, days since emergence, and a random effect of enclosure. We also included the total number of animals per enclosure (container density) as a predictor variable. The measure of density provided a continuous classification of terrarium density outside of the random effect of enclosure but was not unique for each individual. Despite feeding an excess of fruit flies, the density of animals in a terrarium could have had other effects on growth (Altwegg, 2003; Harper and Semlitsch, 2007).
We tested two distinct movement aspects of each individual: the decision to attempt a movement, and how far to travel during a movement event. A hurdle regression model was used to assess these two aspects of an individual response (Falke et al., 2013). Individuals that were within 20 cm from the start location (a 15 cm disk of moss) were not considered to have moved since they were near the moss refuge of the release point; this created a binomial response variable of a movement attempt. This analysis simultaneously fits a binomial model for the decision to leave or stay in the start location with a zero-truncated count regression model of distance for individuals that move down the runway. The models were fit using the “glmmTMB” library in R, which allowed for inclusion of random effects of experimental enclosure (Brooks et al., 2017). Only animals that completed both trials were included in the final analysis.
As this experiment ranged across a metamorphic life history transition, there were a suite of individual measurements that could be included as covariates in this modeling framework. To reduce the likelihood of an over fit model, predictor covariates were grouped into three sets: environmental conditions (day of assay, time of assay, ambient temperature), individual characteristics (SVL, body condition, days since emergence), and individual traits at metamorphic emergence (emergence SVL, emergence body condition, day of emergence). Humidity is likely an important aspect of the environmental conditions in shaping movement (Chan-McLeod, 2003), but is integrally determined by temperature which had a finer resolution measurement and was used in place of humidity. Correlation between covariates within each group were assessed to check for multicollinearity. In addition to our experimental treatments (hydroperiod, runway condition, run number, random effect of enclosure), these covariate groups were used to develop four candidate models (Table 1). Body condition was calculated using the scaled mass index proposed by Peig and Green (2009), which incorporates allometric scaling of body size to better represent an individual's energy stores. Predictor covariates were standardized by subtracting the mean and dividing by their standard deviations to make them more directly comparable in the model and subsequently back transformed onto their original scales for interpretation. These four candidate models were then compared using AIC (Symonds and Moussalli, 2011). The best fit model (lowest AIC) was based on individual characteristics including SVL, body condition, and days since emergence. We assessed the presence of interaction with the best fitting model and adding interactions with and between all experimental factors and covariates. The AIC values from all interaction models showed no significant improvement (ΔAIC > 2) in model fit over the additive model. In the interest of parsimony, we used the additive individual characteristic model for interpretation (Aho et al., 2014). All statistical analyses were performed in R (version 3.5.0) using RStudio (version 1.1.447, RStudio Team, 2016).
Results
We tested 204 animals that emerged within our experimental window and successfully completed both runway assays. These animals emerged from 18 of our mesocosms (9 Ephemeral and 9 Permanent) with an average emergence of 11.3 (SD = 4.8) animals per mesocosm. Two of the mesocosms did not have any emergence within our experimental window (1 Ephemeral and 1 Permanent). The 82 individuals from Ephemeral mesocosms were subsequently divided evenly into Wet and Dry runway treatments. Permanent mesocosms had 122 individuals emerge, resulting in 63 and 59, respectively in Wet and Dry runway treatments.
Our analysis on size at emergence found that both day of emergence (z = −5.16, p < 0.001) and hydroperiod (z = 2.98, p = 0.003) had a significant effect on emergence SVL. Snout-vent length at emergence decreased by 0.12 mm for each additional week (95% CI = 0.08 to 0.17), meaning that individuals emerging earlier were relatively larger than later-emerging individuals. Individuals from permanent hydroperiods had 0.66 mm longer SVL (95% CI = 0.23 to 1.10) than individuals from ephemeral hydroperiods (Figure 1A). In addition to this effect of hydroperiod on size at emergence, we also detected a significant difference in juvenile growth rates based on larval hydroperiod. Juvenile SVL was significantly affected by day of emergence (z = −5.09, p < 0.001), days since emergence (z = 2.65, p = 0.008), and hydroperiod (z = −2.84, p = 0.005). Individuals from ephemeral hydroperiods emerged at a smaller size relative to their counterparts from permanent hydroperiods but had faster growth post-emergence. Enclosure density did not have a significant effect on SVL growth (z = −0.78, p = 0.437). Like the above analysis, day of emergence had a negative effect on SVL growth after emergence with 0.19 mm less growth for each additional week (95% CI = 0.12 to 0.27). Increased time elapsed since emergence resulted in increased SVL growth, with an additional 0.14 mm for each week post-emergence (95% CI = 0.04 to 0.24). Further, we found the effect of mesocosm hydroperiod was opposite of the previous analysis, with individuals from permanent hydroperiods increasing SVL 0.61 mm less than ephemeral conditions (95% CI = 0.19 to 1.04) after emergence (Figure 1B).
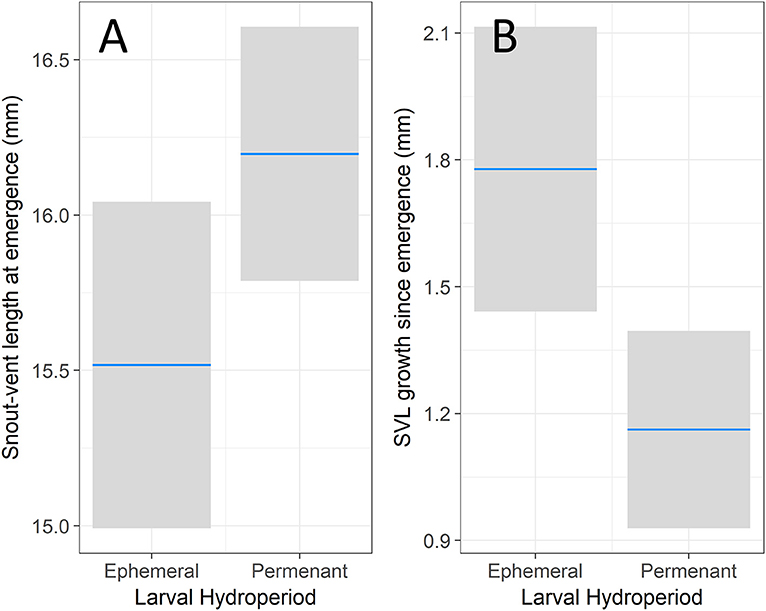
Figure 1. The effect of hydroperiod on snout-vent length. The left (A) shows the effect of hydroperiod on emergence SVL after controlling for the emergence day. The right (B) shows the effect of hydroperiod on juvenile SVL growth during the first assay after controlling for emergence day, days since emergence, enclosure density. The blue bar represents the modeled response of an average individual with the 95% confidence interval in the shaded bar.
In our analysis of individual movement behavior, the best fit model included individual characteristics at the time of the runway assay, including SVL, body condition, and days since emergence, as covariates with experimental factors of larval hydroperiod, runway condition, and runway assay number (Table 1). The hurdle model allows the movement of each treatment group to be separated into the decision to leave the release point and the subsequent decision of how far to move. These two aspects of the model were fit with identical predictors but have distinct results. In order to distinguish between these two aspects of the behavior, they are referred to as the movement hurdle (decision to move, zero-inflated model component) and the movement distance (distance undertaken after movement decision, conditional model component).
From our model, two variables were significant to both the movement hurdle and movement distance: runway condition and SVL. Runway condition was a significant predictor of a frog crossing the movement hurdle (z = −4.16, p < 0.001). An individual in dry runway conditions is 60% less likely to move than in wet runway conditions (95% CI = 38 to 74%). After controlling the other variables, the probability an average individual moves away from the start position was 0.24 (±0.05 SE) in a dry runway compared to 0.44 (±0.06 SE) in a wet runway. Snout-vent length significantly influenced an individual's willingness to cross the movement hurdle (z = −2.86, p = 0.004). With each millimeter increase in SVL at the time of the assay, the odds of crossing the movement hurdle and leaving the start position increased by 16% (95% CI = 0.05 to 25%). The probability of crossing the movement hurdle increased in wet runway condition and as SVL increased (Figure 2). However, after crossing the movement hurdle, the impact of runway condition changed. Runway condition still significantly influenced the distance an individual moved down the runway (z = −3.01, p = 0.003), but now wet runway condition reduced the distance moved by 36% (95% CI = 14% to 52%) compared to the dry runway condition. As in the movement hurdle, SVL was a significant predictor of movement distance (z = 2.94, p = 0.003). An increase of 1 mm SVL increased the movement distance by 12% (95% CI = 4 to 21%). Movement distance was highest for large individuals in dry runway conditions (Figure 3).
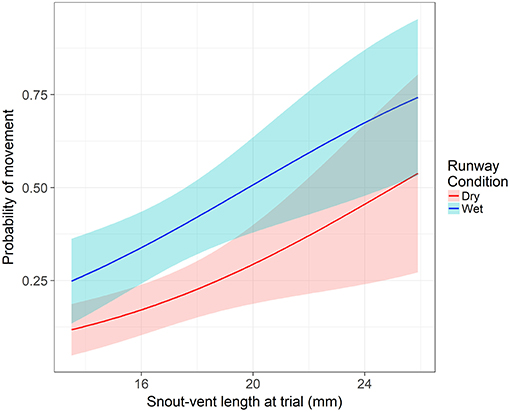
Figure 2. The impact of snout-vent length and runway condition on probability of movement across the movement hurdle. Shaded areas represent the 95% confidence interval. Response is modeled off an individual from the permanent hydroperiod in their first runway assay with average body condition and time since metamorphosis.
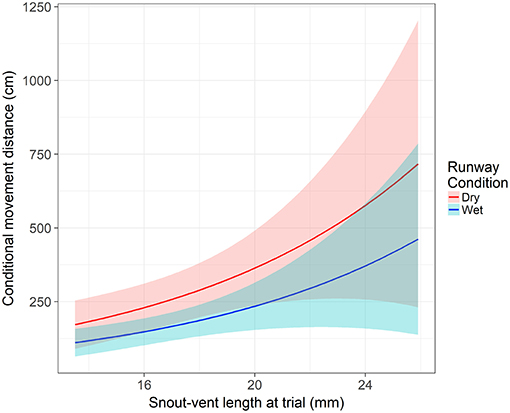
Figure 3. The impact of snout-vent length and runway condition on movement distance after crossing the movement hurdle. Shaded areas represent the 95% confidence interval. Response is modeled off an individual from the permanent hydroperiod in their first runway assay with average body condition and time since metamorphosis.
Neither body condition nor time since emergence (age) were significant in our model for movement hurdle (body condition: z = 0.20, p = 0.840; age: z = −0.28, p = 0.773) and movement distance (body condition: z = 0.81, p = 0.416; age: z = 0.40, p = 0.688). Repeatability of behavior by an individual across assays was not strongly correlated (R2 = 0.0283 distance, R2 = 0.0193 hurdle). Snout-vent length and runway condition were the only important factors in both the ability and behavior around movement, yet hydroperiod was not significant in either the movement hurdle (z = 1.25, p = 0.212) or movement distance (z = 0.23, p = 0.816). The opposite effect of ephemeral conditions with smaller size at emergence and subsequent increased growth rates likely reduced the significance of hydroperiod in the behavioral assay because of the spectrum of ages included (Range = 2–83 days after emergence, mean = 35 ± 19 days SD).
Discussion
The ability of an amphibian species to respond plastically to changing hydroperiods in seasonally-variable habitats is an essential life history adaptation, particularly in the face of changing climate. However, if an appropriate larval trait response carries over to fundamentally influence an individual's ability to contend with stressors later in life, this response could be maladaptive. Our objective was to assess the carryover effects of larval hydroperiod permanence onto juvenile growth and movement in low- and high-stress terrestrial conditions. We found that 2 factors, individual R. aurora size and terrain condition, were the central predictors of individual movement behavior. The carryover effect of hydroperiod was expressed in both the size at emergence and compensatory growth of individuals soon after metamorphosis. We identified no direct correlation between hydroperiod and movement behavior as hypothesized. However, hydroperiod is directly related to the size of juveniles, which is the main factor determining movement behavior. Thus, larval conditions, including hydroperiod, indirectly influence movement behavior.
Our experiment found that R. aurora from permanent hydroperiod conditions metamorphosed at a larger size than individuals reared in ephemeral hydroperiods (Figure 1). This pattern is consistent in species that can plastically modify larval developmental rates under stressful conditions (Denver et al., 1998; Morey and Reznick, 2004). Changes in larval duration has been one suggested explanation for the effects of hydroperiod (Morey and Reznick, 2004). We did not observe any difference in larval duration between treatments, which is supported by other observation of reduced size in drying conditions independent of changes in larval duration (Brady and Griffths, 2000; Mogali et al., 2017). However, these smaller sized individuals from ephemeral conditions then demonstrated increased growth rates immediately after emergence, possibly to compensate for suboptimal size at metamorphosis. Compensatory growth of smaller individuals exposed to stressful larval conditions has been observed in other frog species (Boone, 2005; Bouchard et al., 2016). The increased growth rates of the individuals from ephemeral conditions may explain why larval hydroperiod was not significant in our analysis of individual movement. Since our experiment included individuals from a wide emergence window (i.e., emergence dates ranged from 25 June to 24 September), the opposing effect of hydroperiod on emergence size and terrestrial growth created difficulty in deciphering the carryover effect directly. It should also be acknowledged that the relative different in sizes between ephemeral and permanent conditions is only ca. Four percentage after accounting for emergence timing, which match the magnitude of other studies (Semlitsch, 1987; Searcy et al., 2014). What is clear from our analysis is that larval hydroperiod has effects on movement of juvenile R. aurora by means of a trade-off in growth between the aquatic and terrestrial environment. This may be common for many species with multiple life history stages to escape drying conditions but requires long-term experiments to uncover fully.
We found body size to be a strong predictor of movement behavior. Size is a critical trait in amphibian biology from physical abilities to individual fitness (Walton, 1988; Van Allen et al., 2010; Earl and Whiteman, 2015), with snout-vent length being a simple measurement that encompassed much of the variation in size. In many amphibian species, larger individual size is often correlated with increased performance (Chelgren et al., 2006; Gomes et al., 2009; Yagi and Green, 2017), as observed in our results (Figure 3). Yet increases in snout-vent length were also correlated with increased movement probability (Figure 2). This result indicates that an individual's behavior is also influenced by its physical size. One possible explanation for this effect is a change in the perceived cost of a condition relative to body size. Larger individuals can retain moisture more efficiently than small individuals (Levy and Heald, 2016), thereby making movements in dry conditions less risky. Another possibility is inherent differences in behavior that result in differences in size. Individuals with bold personalities can be more aggressive in resource acquisition resulting in differences in body size and these personality traits can also increase predisposition to explore new areas (Kelleher et al., 2018). Whatever the cause of these behavioral differences across a gradient of snout-vent length, this physical trait may be a useful metric for understanding individual movement potential in field contexts.
Terrain moisture is an important aspect of environmental condition that shapes the movement strategies of organisms that are sensitive to dehydration, such as amphibians and invertebrates. With our experimental design, we were able to examine the critical aspects of this behavior: initial decision to move and distance traveled (Martin et al., 2005). The effect of runway condition had predictable effects, such as wet conditions increasing the probability of movement over dry conditions (Figure 2). However, dry conditions that can pose significant physiological hazards to young amphibians increased movement distances. Movement may be more effective if individuals quickly traverse the drier terrain to find more hospitable habitat. In contrast, wet terrain conditions are less risky and have the effect of reducing the distance moved (Figure 3). Most research has found reduced movement in non-habitat (Eycott et al., 2012) with only occasional evidence for increased movement in inhospitable areas (damselfly—Pither and Taylor, 1998; planthopper—Haynes et al., 2006). However, as indicated by our results, there were several instances of amphibians moving more efficiently over simple and inhospitable habitat (Natterjack toads—Stevens et al., 2004; Northern green frog—Birchfield and Deters, 2005). While there is limited information about the terrestrial movement and habitat of R. aurora, there is anecdotal evidence that they are associated with aquatic habitat and moist forests while also moving substantial distances (Haggard, 2000; Hayes et al., 2001; Chan-McLeod, 2003). Amphibians with different habitat requirements or life-history could be expected to respond differently.
Changes in precipitation pattern and droughts likely will also influence the distributions of aquatic habitat and, by extension, amphibian populations and their connectivity (Robson et al., 2011; Jaeger et al., 2014; Cayuela et al., 2016). Insights into carryover and direct effects of stress on juvenile movement behavior provide opportunities to inform management strategies. Beaver canals have been observed to influence the location and emergence of amphibians, making waterway modifications a potential strategy for spatial management of populations (Anderson et al., 2015). Amphibians' likely rely on rare long-distance movements for population connectivity (Semlitsch, 2008). Even small changes in the success of dispersing individuals or distances traversed will likely have great implication for population connectivity (Stevens et al., 2012). Our study informs this pattern by relating hydroperiod permanence with juvenile amphibian body size and growth rates with dispersal probability. The behavioral and developmental trait connections across this life history transition likely influence population connectivity.
This project looked at movement behavior in a simplified setting. However, amphibians have shown to change their movement behaviors based on ground cover and context (Cline and Hunter, 2014, 2016; Osbourn et al., 2014). Accounting for the accumulated impacts of stressors across an individual's development, including terrain complexity, is a key step in providing a complete understanding of these animals. How other stressors, such as terrestrial density, predator presence, or prey availability interact with hydroperiod and terrain conditions could add additional consideration to the movement decisions of these animals (Vonesh and Warkentin, 2006; Rittenhouse et al., 2007; Patrick et al., 2008). Evidence from other amphibian species have also found reduced growth rates in dry conditions creating potential multiplicative effects of environmental conditions, as size was the central determinant of movement (Gomez-Mestre and Tejedo, 2005; Charbonnier et al., 2018). Other research on carry-over effect in amphibians has supported the need for longitudinal studies (Johansson et al., 2010; Charbonnier and Vonesh, 2015; Yagi and Green, 2018). With this broader understanding, we would be better able to combine aquatic structure with terrestrial habitat management to provide for essential habitat components of threatened species (Fellers and Kleeman, 2007; Bartelt and Klaver, 2017).
Amphibian movement has been understudied despite its importance for population connectivity and dispersal of vulnerable species (Pittman et al., 2014; Howell et al., 2018; Zamberletti et al., 2018). Our study details some of the drivers of movement assessed in isolation which can be incorporated into predictions of in-situ movement, but there is still need for such knowledge to be explored and validated in field and population studies. There are still important aspects such as directionality and habitat settlement that would further our understanding of movement. In short, assessing movement as a holistic, ontogenetic system, will improve our understanding of this complicated behavior and the myriad factors influencing how an animal moves through life.
Data Availability
The raw data supporting the conclusions of this manuscript will be made available by the authors, without undue reservation, to any qualified researcher.
Ethics Statement
This work was performed under Oregon State University Institutional Animal Care and Use Committee with Animal Care and Use Protocol 4536 and the Oregon Department of Fisheries and Wildlife Service for Special Use Permit no. 043-15 for animal collections.
Author Contributions
EB, TG, and AM conceived the research question. EB and JU collected animals and performed data collection. EB performed the analysis. EB wrote the manuscript with significant assistance from JU, AM, and TG. All authors contributed critically to the drafts and gave final approval for publication.
Funding
This work was supported by the National Science Foundation Graduate Research Fellowship under Grant No. 1314109-DGE. The award provided tuition and stipend support with a small amount of research funds.
Conflict of Interest Statement
The authors declare that the research was conducted in the absence of any commercial or financial relationships that could be construed as a potential conflict of interest.
Acknowledgments
We would like to thank Hyslop Field Laboratory for their access and use of space. We would also like to thank Jennifer Rowe for statistical consultation, Nicolas Soria and Angie Soken for their assistance with construction and data collection.
References
Adams, M. (2000). Pond permanence and the effects of exotic vertebrates on anurans. Ecol. Appl. 10, 559–568. doi: 10.1890/1051-0761(2000)010[0559:PPATEO]2.0.CO;2
Aho, K., Derryberry, D., and Peterson, T. (2014). Model selection for ecologists: the worldview of AIC and BIC. Ecology 95, 631–636. doi: 10.1890/13-1452.1
Altwegg, R. (2003). Multistage density dependence in an amphibian. Oecologia 136, 46–50. doi: 10.1007/s00442-003-1248-x
Amburgey, S. M., Murphy, M., and Funk, W. C. (2016). Phenotypic plasticity in developmental rate is insufficient to offset high tadpole mortality in rapidly drying ponds. Ecosphere 7, 1–14. doi: 10.1002/ecs2.1386
Anderson, N. L., Paszkowski, C. A., and Hood, G. A. (2015). Linking aquatic and terrestrial environments: Can beaver canals serve as movement corridors for pond-breeding amphibians? Anim. Conserv. 18, 287–294. doi: 10.1111/acv.12170
Bartelt, P. E., and Klaver, R. W. (2017). Response of anurans to wetland restoration on a Midwestern Agricultural landscape. J. Herpetol. 51, 504–514. doi: 10.1670/16-113
Birchfield, G., and Deters, J. (2005). Movement paths of displaced northern green frogs (Rana clamitans melanota). Southeast. Nat. 4, 63–76. doi: 10.1656/1528-7092(2005)004[0063:MPODNG]2.0.CO;2
Boone, M. D. (2005). Juvenile frogs compensate for small metamorph size with terrestrial growth: overcoming the effects of larval density and insecticide exposure. J. Herpetol. 39, 416–423. doi: 10.1670/187-04A.1
Bouchard, S. S., O'Leary, C. J., Wargelin, L. J., Charbonnier, J. F., and Warkentin, K. M. (2016). Post-metamorphic carry-over effects of larval digestive plasticity. Funct. Ecol. 30, 379–388. doi: 10.1111/1365-2435.12501
Brady, L. D., and Griffths, R. A. (2000). Developmental responses to pond desiccation in tadpoles of the British anuran amphibians (Bufo bufo, B. calamita and Rana temporaria). J. Zool. 252, 61–69. doi: 10.1111/j.1469-7998.2000.tb00820.x
Brooks, M. E., Kristensen, K., Benthem, K. J., van Magnusson, A., Berg, C. W., Nielsen, A., et al. (2017). glmmTMB balances speed and flexibility among packages for zero-inflated generalized linear mixed modeling. R J. 9, 378–400. doi: 10.32614/RJ-2017-066
Cayuela, H., Arsovski, D., Thirion, J. M., Bonnaire, E., Pichenot, J., Boitaud, S., et al. (2016). Contrasting patterns of environmental fluctuation contribute to divergent life histories among amphibian populations. Ecology 97, 980–991. doi: 10.1890/15-0693.1
Chan-McLeod, A. C. A. (2003). Factors affecting the permeability of clearcuts to red-legged frogs. J. Wildl. Manage. 67:663. doi: 10.2307/3802673
Charbonnier, J., Pearlmutter, J., Vonesh, J., Gabor, C., Forsburg, Z., Grayson, K., et al. (2018). Cross-life stage effects of aquatic larval density and terrestrial moisture on growth and corticosterone in the spotted salamander. Diversity 10:68. doi: 10.3390/d10030068
Charbonnier, J. F., and Vonesh, J. R. (2015). Consequences of life history switch point plasticity for juvenile morphology and locomotion in the Tungara frog. PeerJ. 3:e1268. doi: 10.7717/peerj.1268
Chelgren, N. D., Rosenberg, D. K., Heppell, S. S., and Gitelman, A. I. (2006). Carryover aquatic effects on survival of metamorphic frogs during pond emigration. Ecol. Appl. 16, 250–261. doi: 10.1890/04-0329
Cline, B. B., and Hunter, M. L. (2014). Different open-canopy vegetation types affect matrix permeability for a dispersing forest amphibian. J. Appl. Ecol. 51, 319–329. doi: 10.1111/1365-2664.12197
Cline, B. B., and Hunter, M. L. Jr. (2016). Movement in the matrix: substrates and distance- to- forest edge affect postmetamorphic movements of a forest amphibian. Ecosphere 7:e01202. doi: 10.1002/ecs2.1202
Denver, R. J., Mirhadi, N., and Phillips, M. (1998). Adaptive plasticity in amphibian metamorphosis: response of Scaphiopus hammondii tadpoles to habitat desiccation. Ecology 79, 1859–1872. doi: 10.1890/0012-9658(1998)079[1859:APIAMR]2.0.CO;2
Earl, J. E., and Whiteman, H. H. (2015). Are commonly used fitness predictors accurate? a meta-analysis of amphibian size and age at metamorphosis. Copeia 103, 297–309. doi: 10.1643/CH-14-128
Eycott, A., Stewart, G., Buyung-Ali, L., Bowler, D. E., Watts, K., and Pullin, A. S. (2012). A meta-analysis on the impact of different matrix structures on species movement rates. Landsc. Ecol. 27, 1263–1278. doi: 10.1007/s10980-012-9781-9
Falke, J. A., Dunham, J. B., Jordan, C. E., McNyset, K. M., and Reeves, G. H. (2013). Spatial ecological processes and local factors predict the distribution and abundance of spawning by steelhead (Oncorhynchus mykiss) across a complex riverscape. PLoS ONE 8:e079232. doi: 10.1371/journal.pone.0079232
Fellers, G., and Kleeman, P. (2007). California red-legged frog (Rana draytonii) movement and habitat use: implications for conservation. J. Herpetol. 41, 276–286. doi: 10.1670/0022-1511(2007)41[276:CRFRDM]2.0.CO;2
Gomes, F. R., Rezende, E. L., Grizante, M. B., and Navas, C. A. (2009). The evolution of jumping performance in anurans: Morphological correlates and ecological implications. J. Evol. Biol. 22, 1088–1097. doi: 10.1111/j.1420-9101.2009.01718.x
Gomez-Mestre, I., and Tejedo, M. (2005). Adaptation or exaptation? An experimental test of hypotheses on the origin of salinity tolerance in Bufo calamita. J. Evol. Biol. 18, 847–855. doi: 10.1111/j.1420-9101.2004.00878.x
Gosner, K. L. (1960). A simplified table for staging anuran embryos larvae with notes on identification. Herpetologica 16, 183–190.
Govindarajulu, P., Price, W. M. S., and Anholt, B. R. (2006). Introduced Bullfrogs (Rana catesbeiana) in Western Canada: has their ecology diverged? J. Herpetol. 40, 249–260. doi: 10.1670/68-05A.1
Haggard, J. A. G. (2000). A Radio Telemetric Study of the Movement Patterns of Adult Northern Red-Legged Frogs (Rana aurora aurora) at Freshwater Lagoon. Humboldt County CA, thesis from Humboldt State University.
Harper, E. B., and Semlitsch, R. D. (2007). Density dependence in the terrestrial life history stage of two anurans. Oecologia 153, 879–889. doi: 10.1007/s00442-007-0796-x
Hayes, M. P., Pearl, C. A., and Rombough, C. J. (2001). Rana aurora aurora (Northern Red-legged Frog) movement. Herpetol. Rev. 32, 35–36.
Hayes, M. P., Quinn, T., Richter, K. O., Schuett-hames, J. P., and Shean, J. T. S. (2008). “Maintaining lentic-breeding amphibians in urbanizing landscapes: the case study of the northern red-legged frog (Rana aurora),” in Urban Herpetology, eds. J. C. Mitchell and R. E. J. Brown (Salt Lake City, UT: Society for the Study of Amphibians and Reptiles), 133–149.
Haynes, K. J., Cronin, J. T., and de Roos, A. (2006). Interpatch movement and edge effects: the role of behavioral responses to the landscape matrix. Oikos 113, 43–54. doi: 10.1111/j.0030-1299.2006.13977.x
Howell, P. E., Muths, E., Hossack, B. R., Sigafus, B. H., and Chandler, R. B. (2018). Increasing connectivity between metapopulation ecology and landscape ecology. Ecology 99, 1119–1128. doi: 10.1002/ecy.2189
Hughes, I. (2000). Biological consequences of global warming: is the signal already apparent? Trends Ecol. Evol. 15, 56–61. doi: 10.1016/S0169-5347(99)01764-4
Jaeger, K. L., Olden, J. D., and Pelland, N. A. (2014). Climate change poised to threaten hydrologic connectivity and endemic fishes in dryland streams. Proc. Natl. Acad. Sci. U.S.A. 111, 13894–13899. doi: 10.1073/pnas.1320890111
Johansson, F., Lederer, B., and Lind, M. I. (2010). Trait performance correlations across life stages under environmental stress conditions in the common Frog, Rana temporaria. PLoS ONE 5:e11680. doi: 10.1371/journal.pone.0011680
Kelleher, S. R., Silla, A. J., and Byrne, P. G. (2018). Animal personality and behavioral syndromes in amphibians : a review of the evidence, experimental approaches, and implications for conservation. Behav. Ecol. Sociobiol. 72, 72–79. doi: 10.1007/s00265-018-2493-7
Lancaster, L. T., Morrison, G., and Fitt, R. N. (2017). Life history trade-offs, the intensity of competition, and coexistence in novel and evolving communities under climate change. Philos. Trans. R. Soc. B Biol. Sci. 372:20160046. doi: 10.1098/rstb.2016.0046
Leary, S., Underwood, W., Anthony, R., Cartner, S., Corey, D., Grandin, T., et al. (2013). AVMA Guidelines for the Euthanasia of Animals. Schaumburg, IL: American Veterinary Medical Association. doi: 10.1016/B978-012088449-0.50009-1
Levy, D. L., and Heald, R. (2016). Biological scaling problems and solutions in amphibians. Cold Spring Harb. Perspect. Biol. 8:a019166. doi: 10.1101/cshperspect.a019166
Levy, O., Buckley, L. B., Keitt, T. H., Smith, C. D., Boateng, K. O., Kumar, D. S., et al. (2015). Resolving the life cycle alters expected impacts of climate change. Proc. R. Soc. B 1 282:20150837. doi: 10.1098/rspb.2015.0837
Martin, T. G., Wintle, B. A., Rhodes, J. R., Kuhnert, P. M., Field, S. A., Low-Choy, S. J., et al. (2005). Zero tolerance ecology: improving ecological inference by modelling the source of zero observations. Ecol. Lett. 8, 1235–1246. doi: 10.1111/j.1461-0248.2005.00826.x
Mazerolle, M., and Desrochers, A. (2005). Landscape resistance to frog movements. Can. J. Zool. 464, 455–464. doi: 10.1139/Z05-032
Meyers, L. A., and Bull, J. J. (2002). Fighting change with change: adaptive variation in an uncertain world. Trends Ecol. Evol. 17, 551–557. doi: 10.1016/S0169-5347(02)02633-2
Mogali, S., Saidapur, S., and Shanbhag, B. (2017). Influence of desiccation threat on the metamorphic traits of the Asian common toad, Duttaphrynus melanostictus (Anura). Acta Herpetol. 12, 175–180. doi: 10.13128/Acta_Herpetol-21016
Morey, S. R., and Reznick, D. N. (2004). The relationship between larval habitat and permanence in California toads : field and laboratory comparisons of developmental plasticity. Oikos 104, 172–190. doi: 10.1111/j.0030-1299.2004.12623.x
O'connor, C. M., Norris, D. R., Crossin, G. T., and Cooke, S. J. (2014). Biological carryover effects: linking common concepts and mechanisms in ecology and evolution. Ecosphere 5, 1–11. doi: 10.1890/ES13-00388.1
Osbourn, M. S., Connette, G. M, and Semlitsch, R. D. (2014). Effects of fine-scale forest habitat quality on movement and settling decisions in juvenile pond-breeding salamanders. Ecol. Appl. 24, 1719–1729. doi: 10.1890/13-0813.1
Patrick, D. A., Harper, E. B., Hunter, M. L., and Calhoun, A. J. (2008). Terrestrial habitat selection and strong density-dependent mortality in recently metamorphosed amphibians. Ecology 89, 2563–2574. doi: 10.1890/07-0906.1
Pechenik, J. A. (2006). Larval experience and latent effects–metamorphosis is not a new beginning. Integr. Comp. Biol. 46, 323–333. doi: 10.1093/icb/icj028
Peig, J., and Green, A. J. (2009). New perspectives for estimating body condition from mass/length data: the scaled mass index as an alternative method. Oikos 118, 1883–1891. doi: 10.1111/j.1600-0706.2009.17643.x
Pither, J., and Taylor, P. D. (1998). An experimental assessment of landscape connectivity. Oikos 83:166. doi: 10.2307/3546558
Pittman, S. E., Osbourn, M. S., and Semlitsch, R. D. (2014). Movement ecology of amphibians: a missing component for understanding population declines. Biol. Conserv. 169, 44–53. doi: 10.1016/j.biocon.2013.10.020
Radchuk, V., Turlure, C., and Schtickzelle, N. (2013). Each life stage matters: The importance of assessing the response to climate change over the complete life cycle in butterflies. J. Anim. Ecol. 82, 275–285. doi: 10.1111/j.1365-2656.2012.02029.x
Relyea, R. A. (2004). Fine-tuned phenotypes: Tadpole plasticity under 16 combinations of predators and competitors. Ecology 85, 172–179. doi: 10.1890/03-0169
Rittenhouse, T. A. G., Semlitsch, R. D., and Hall, T. (2007). Distribution of amphibians in terrestrial habitat surrounding wetlands. Wetlands. 27, 153–161. doi: 10.1672/0277-5212
Robson, B. J., Chester, E. T., and Austin, C. M. (2011). Why life history information matters: drought refuges and macroinvertebrate persistence in non-perennial streams subject to a drier climate. Mar. Freshw. Res. 62, 801–810. doi: 10.1071/MF10062
RStudio Team (2016). RStudio: Integrated Development Environment for R. Available online at: http://www.rstudio.com/ (accessed January 15, 2019).
Schwalm, D., Epps, C. W., Rodhouse, T. J., Monahan, W. B., Castillo, J. A., Ray, C., et al. (2016). Habitat availability and gene flow influence diverging local population trajectories under scenarios of climate change: a place-based approach. Glob. Chang. Biol. 22, 1572–1584. doi: 10.1111/gcb.13189
Searcy, C. A., Snaas, H., and Shaffer, H. B. (2014). Determinants of size at metamorphosis in an endangered amphibian and their projected effects on population stability. Oikos. 124, 724–731. doi: 10.1111/oik.01775
Semlitsch, R. D. (1987). Paedomorphosis in Ambystoma Talpoideum: effects of density, food, and pond drying. Ecology 68, 994–1002. doi: 10.2307/1938370
Semlitsch, R. D. (2008). Differentiating migration and dispersal processes for pond-breeding amphibians. J. Wildl. Manage. 72, 260–267. doi: 10.2193/2007-082
Smith, L. L., Subalusky, A. L., Atkinson, C. L., Earl, J. E., Mushet, D. M., Scott, D. E., et al. (2018). Biological connectivity of seasonally ponded wetlands across spatial and temporal scales. J. Am. Water Resour. Assoc. doi: 10.1111/1752-1688.12682. [Epub ahead of print].
Stevens, V. M., Polus, E., Wesselingh, R. A., Schtickzelle, N., and Baguette, M. (2004). Quantifying functional connectivity: experimental evidence for patch-specific resistance in the Natterjack toad (Bufo calamita). Landsc. Ecol. 19, 829–842. doi: 10.1007/s10980-004-0166-6
Stevens, V. M., Trochet, A., Van Dyck, H., Clobert, J., and Baguette, M. (2012). How is dispersal integrated in life histories: a quantitative analysis using butterflies. Ecol. Lett. 15, 74–86. doi: 10.1111/j.1461-0248.2011.01709.x
Suislepp, K., Rannap, R., and Lõhmus, A. (2011). Impacts of artificial drainage on amphibian breeding sites in hemiboreal forests. For. Ecol. Manage. 262, 1078–1083. doi: 10.1016/j.foreco.2011.06.001
Swanson, J. E., Bailey, L. L., Muths, E., and Funk, W. C. (2013). Factors influencing survival and mark retention in postmetamorphic boreal chorus Frogs. Copeia 2013, 670–675. doi: 10.1643/CH-12-129
Symonds, M. R. E., and Moussalli, A. (2011). A brief guide to model selection, multimodel inference and model averaging in behavioural ecology using Akaike's information criterion. Behav. Ecol. Sociobiol. 65, 13–21. doi: 10.1007/s00265-010-1037-6
Székely, D., Cogălniceanu, D., Székely, P., and Denoël, M. (2017). Out of the ground: two coexisting fossorial toad species differ in their emergence and movement patterns. Zoology 121, 49–55. doi: 10.1016/j.zool.2016.12.003
Thurman, L. L., and Garcia, T. S. (2017). Differential plasticity in response to simulated climate warming in a high-elevation amphibian assemblage. J. Herpetol. 51, 232–239. doi: 10.1670/16-502
Van Allen, B. G., Briggs, V. S., McCoy, M. W., and Vonesh, J. R. (2010). Carry-over effects of the larval environment on post-metamorphic performance in two hylid frogs. Oecologia 164, 891–898. doi: 10.1007/s00442-010-1728-8
Vonesh, J., and Warkentin, K. (2006). Opposite shifts in size at metamorphosis in response to larval and metamorph predators. Ecology 87, 556–562. doi: 10.1890/05-0930
Walton, M. (1988). Relationships among metabolic, locomotory, and field measures of organismal performance in the Fowler's toad (Bufo woodhousei fowleri). Physiol. Zool. 61, 107–118.
Watling, J. I., and Braga, L. (2015). Desiccation resistance explains amphibian distributions in a fragmented tropical forest landscape. Landsc. Ecol. 30, 1449–1459. doi: 10.1007/s10980-015-0198-0
Weinig, C., and Delph, L. F. (2001). Phenotypic plasticity early in life constrains developmental responses later. Evolution 55, 930–936. doi: 10.2307/2680306
Williams, C. M., Henry, H. A., and Sinclair, B. J. (2015). Cold truths: How winter drives responses of terrestrial organisms to climate change. Biol. Rev. 90, 214–235. doi: 10.1111/brv.12105
Yagi, K. T., and Green, D. M. (2017). Performance and movement in relation to postmetamorphic body size in a pond- breeding amphibian. J. Herpetol. 51, 482–489. doi: 10.1670/17-058
Yagi, K. T., and Green, D. M. (2018). Post-metamorphic carry-over effects in a complex life history: behavior and growth at two life stages in an amphibian, Anaxyrus fowleri. Copeia 106, 77–85. doi: 10.1643/CE-17-593
Keywords: plasticity, movement behavior, amphibian, latent effects, life history, ontogeny
Citation: Bredeweg EM, Urbina J, Morzillo AT and Garcia TS (2019) Starting on the Right Foot: Carryover Effects of Larval Hydroperiod and Terrain Moisture on Post-metamorphic Frog Movement Behavior. Front. Ecol. Evol. 7:97. doi: 10.3389/fevo.2019.00097
Received: 30 November 2018; Accepted: 11 March 2019;
Published: 02 April 2019.
Edited by:
Caitlin R. Gabor, Texas State University System, United StatesReviewed by:
Elizabeth A. Roznik, Memphis Zoo, United StatesIvan Gomez-Mestre, Estación Biológica de Doñana (EBD), Spain
Copyright © 2019 Bredeweg, Urbina, Morzillo and Garcia. This is an open-access article distributed under the terms of the Creative Commons Attribution License (CC BY). The use, distribution or reproduction in other forums is permitted, provided the original author(s) and the copyright owner(s) are credited and that the original publication in this journal is cited, in accordance with accepted academic practice. No use, distribution or reproduction is permitted which does not comply with these terms.
*Correspondence: Evan M. Bredeweg, ZXZhbi5icmVkZXdlZ0BvcmVnb25zdGF0ZS5lZHU=