- 1Animal Ecology Group, J.F. Blumenbach Institute of Zoology and Anthropology, University of Göttingen, Göttingen, Germany
- 2Groningen Institute for Evolutionary Life Sciences, University of Groningen, Groningen, Netherlands
- 3Population and Evolutionary Ecology Group, Institute of Ecology, University of Bremen, Bremen, Germany
The establishment of a collective defense is an important means of controlling the spread of harmful microbes in group-living animals. Collective defenses are associated with costs resulting from the investment in resources and the risk taking of infections or the exposure to microbial toxins for the performing individual and are often assumed to have evolved in (eu)social insects, like bees and ants, as a result of close contact and pathogen transmission between nestmates. We hypothesize that collective antimicrobial defense mechanisms are potentially also found in insects that exhibit simpler forms of sociality or even mere aggregation behavior. The larvae of the saprophagous fruit fly Drosophila melanogaster develop in high-density aggregations on rotting fruits, which are often colonized by insecticidal filamentous fungi. Here we show that fruit fly larvae suppress the invasion of a harmful fungus not only by the summative effect of individuals at high densities but also because larger groups of larvae at the same density can control fungal growth more efficiently. We achieved the necessary manipulation of the group size by increasing the number of larvae in proportion to an increase in habitat size, thereby excluding the effect of density changes on fungal growth as a confounding factor. We found evidence that part of the variation in the ability to suppress the fungus in this group size-dependent manner can be explained by genetic variation at the insects’ foraging (for) locus. Group size therefore influences the extent to which the larval aggregates suppress the spread of a harmful fungus. This indicates a potential collective defense against habitat invasion by pathogenic fungi. The selection pressure on the efficiency of this potential defense strategy may contribute to the evolution of aggregation behavior in non-(eu)social insects.
Introduction
Allogrooming, application of antimicrobial substances, “weeding,” and removal of diseased broods are traits that enable group-living insects—dependent on their social organization—to defend themselves collectively against the invasion of harmful microbes (Cremer et al., 2007; Meunier, 2015; van Meyel et al., 2018). “Social” or “collective” imply that antimicrobial behavioral and physiological strategies are performed jointly and/or toward each other. Therefore, collective defense constitutes an additional shield that complements the protection achieved by individual defenses.
The individual-level processes mediating the collective defense are thought to have evolved in response to increased pathogen transmission in eusocial insects—the eusocial framework (Cremer et al., 2007; Cotter and Kilner, 2010; Kappeler et al., 2015; Nuotclà et al., 2019). For this reason, collective antimicrobial defense is often seen in the context of social immunity (Cremer et al., 2007). However, as highlighted by Meunier (2015) and van Meyel et al. (2018), antimicrobial defense strategies, such as release of self-produced antibiotics, hygienic behavior, or allogrooming, are found not only in eusocial but also in non-(eu)social and even solitary insects. If these strategies are performed collectively and result in positive feedback on individual fitness, such a feedback may be a selective force favoring group living and the social complexity of such groups—the group living framework (Meunier, 2015; Biedermann and Rohlfs, 2017; van Meyel et al., 2018; Nuotclà et al., 2019). According to the group living framework, one would expect to observe collective defense strategies in insects that are non-eusocial or in those that form only semi-social aggregations (Nuotclà et al., 2019). In such aggregations, resistance against harmful microbes can be achieved passively due to density-dependent effects (Figure 1). However, this seemingly improved control of microbes is a mere numerical summation of the effects of otherwise competing individuals, i.e., an effect that would not be considered as collective defense or social immunity (Cotter and Kilner, 2010; Meunier, 2015; van Meyel et al., 2018). Thus, if an antimicrobial trait is important for a possible collective defense in a given system, one would expect that the efficiency of the expression of this trait increases with increasing group size, independent of the actual density of individuals (Figure 1). To test this, careful experimental manipulation is required to control for the confounding effect of density.
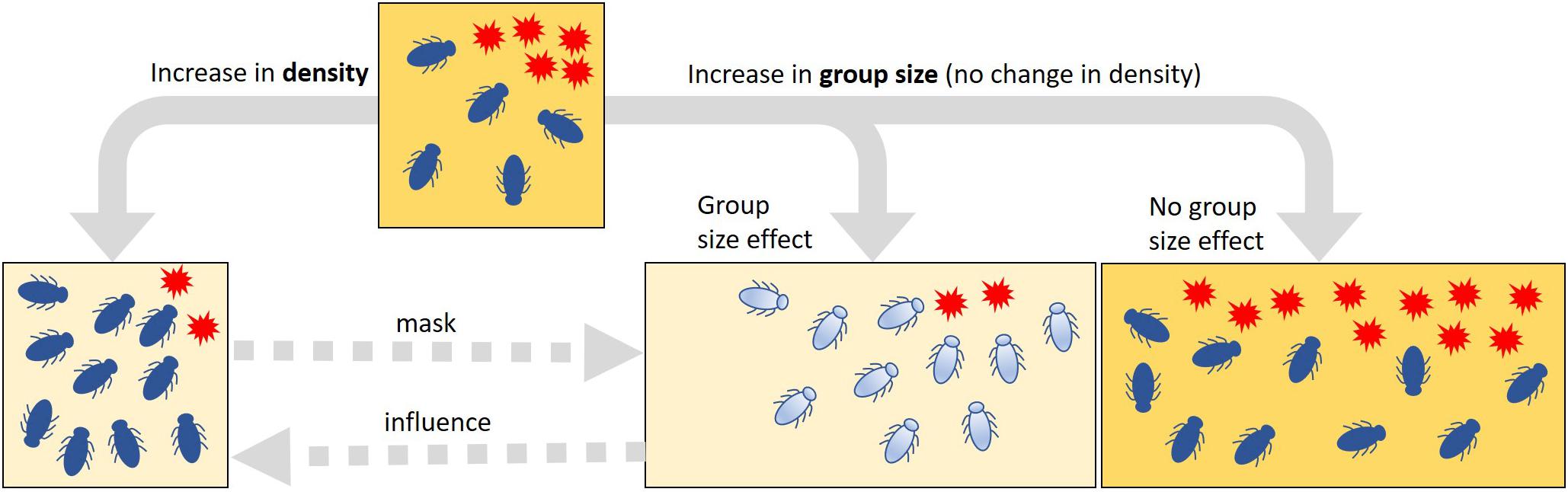
Figure 1. Summative vs. collective effects of variation in the density or group size of insects on the spread of harmful microbes. Increased suppression of harmful microbes (red stars) can be the result of an increase in both density and group size in a habitat (squares). In a pure density effect, this change in suppression occurs only passively through the additive effects of the antimicrobial activity of the individuals; an increase in density (i.e., changes in the number of insects, but no change in habitat size as indicated by the square size) does not change the quality or the intensity of the antimicrobial properties. If there are elements of a collective defense in the control of harmful microbes that is based on an active change in the quality or the intensity of antimicrobial properties (depicted by the change in insect and habitat coloration), it is to be expected that the suppression of microbes is stronger in large than in small groups. If there is no such group size effect, the harmful microbes would spread in proportion to habitat size. To detect such group size effects and thus the potential collective actions, it is important to carefully control the effects of density changes experimentally. Density effects may mask potential group size effects, but group size effects can be part of and possibly amplify density effects (dashed arrows).
Within this group living framework, we hypothesize that an ancestral stage in the early evolution of collective antimicrobial defense is due to the advantages of simple semi-social aggregation behavior (Biedermann and Rohlfs, 2017). Semi-social aggregations are widespread in insects that breed in ephemeral resources, e.g., dung, carrion, fruits. On such resources, the developing insect larvae have to cope with numerous saprotrophic and often harmful microorganisms, insecticidal bacteria, and fungi (Janzen, 1977). Aggregative behaviors of non-social insects have frequently been observed in association with such microbes [numerous examples described in Wertheim et al. (2005)]. In the fruit-inhabiting Drosophila model system, the harmful effect of molds on larval development is based on a constitutive and inducible formation of insecticidal secondary metabolites (Caballero Ortiz et al., 2013), which trigger several disease symptoms or even kill the larvae (Wölfle et al., 2009; Trienens et al., 2010). However, fruit fly larvae in high-density aggregations can successfully suppress the spread of insecticidal mold fungi, e.g., Aspergillus sp. and Penicillium sp., in their feeding habitat and thus achieve higher per capita fitness (Rohlfs, 2005). The formation of fungus-controlling aggregations not only seem to matter in Drosophila (Hodge et al., 1999; Wertheim et al., 2002; Rohlfs and Hoffmeister, 2003) but also contribute to the management of detrimental fungi in facultative eusocial ambrosia beetles, for example (Biedermann and Taborsky, 2011; Nuotclà et al., 2019). The insects probably achieve the destruction of hyphal tissue by using their mouth parts, e.g., by chewing the mycelium. In addition, chemical components such as the release of antimicrobial peptides could also be involved in the inhibition of fungal growth.
As outlined above, in such aggregations, group size-specific effects could be masked by insect density-dependent suppression of harmful fungi or not exist at all, i.e., there may be no collective behavior resulting from interactions between group members that contributes to the suppression of harmful microbes. For this reason, we investigate here the hypothesis that larger groups of fruit fly larvae have a higher capacity to suppress the growth of a harmful fungus, which would indicate the involvement of an antimicrobial collective action. To test this, we manipulated the group size of Drosophila melanogaster larvae while not altering their density and quantified the suppression of the insecticidal fungus Aspergillus nidulans by these groups. This fungus species is representative of various taxa of widespread molds that negatively influence insect development in different decomposer systems by the formation of insecticidal secondary metabolites (Janzen, 1977; Hodge et al., 1999; Drott et al., 2017; Künzler, 2018). Additionally, in order to gain a first insight into how selection might favor different behaviors in the face of microbial invasion, we also investigated the extent to which genetic variation in movement behavior, determined by allelic variation in the foraging locus (Anreiter and Sokolowski, 2019), contributes to the suppression of fungal growth in the breeding substrate of Drosophila.
Materials and Methods
Organisms
Experimental D. melanogaster larvae were obtained from an outbred lab population that was established from field-caught flies in 2006 (Wölfle et al., 2009). The population has been kept since with non-overlapping generations, where larvae were reared under moderate densities in several flasks containing breeding substrate. Subsequently, the enclosed flies have been joined as one population in a cage provided with food and water. A flask with breeding substrate was attached to the cage to allow egg laying. This strain was used in Experiment 1 and Experiment 2.
Aspergillus nidulans (strain RDIT2.3) colonies were grown on malt extract agar (30 g malt extract, 5 g peptone, and 20 g agar Kobe I, filled up to 1 L with purified water). Fungal conidiospores for inoculation of experimental setups were harvested from 7-day-old colonies by rinsing the colony with saline solution (8.6 g NaCl, 300 mg KCl, 350 mg CaCl2 per liter of demineralized water). Conidiospore titer was estimated using a hemocytometer (Neubauer improved). Experiment preparations were conducted in a laminar flow cabinet. We used heat-sterilized tools and substrate. Eggs and plastic frames were treated with sodium hypochlorite to eliminate adherent microbes.
General Experimental Setup
To manipulate insect group size rather than insect density, we designed experimental arenas of different sizes. For this, we created rectangular plastic frames that consisted of plastic strips (polyester strengthened with paper inlay, 15 mm height, 250 μm thickness, the length depends on the arena size), which were pleated at three folding edges and fastened at the fourth to obtain frames of respective sizes (Figure 2A). The plastic frames were filled with fruit agar (50/50 v/v% mashed banana, water, and 36 g agar/L) and placed in 50- and 90-mm Petri dishes, respectively. We created a clearly defined fungal growth zone by forcing the unidirectional growth of the fungi. Filter paper strips (3 mm × arena length) were soaked in a suspension of conidiospores (1 million conidia per microliter); this conidia titer was used to ensure the development of a homogeneous growth front of the fungus. The air-dried filter strips were then placed flush with the frame on the fruit agar (Figure 2B). All setups were pre-incubated at 25°C and constant darkness for 48 h. Then, the larvae were transferred to the arenas and further incubated at 25°C and constant darkness.
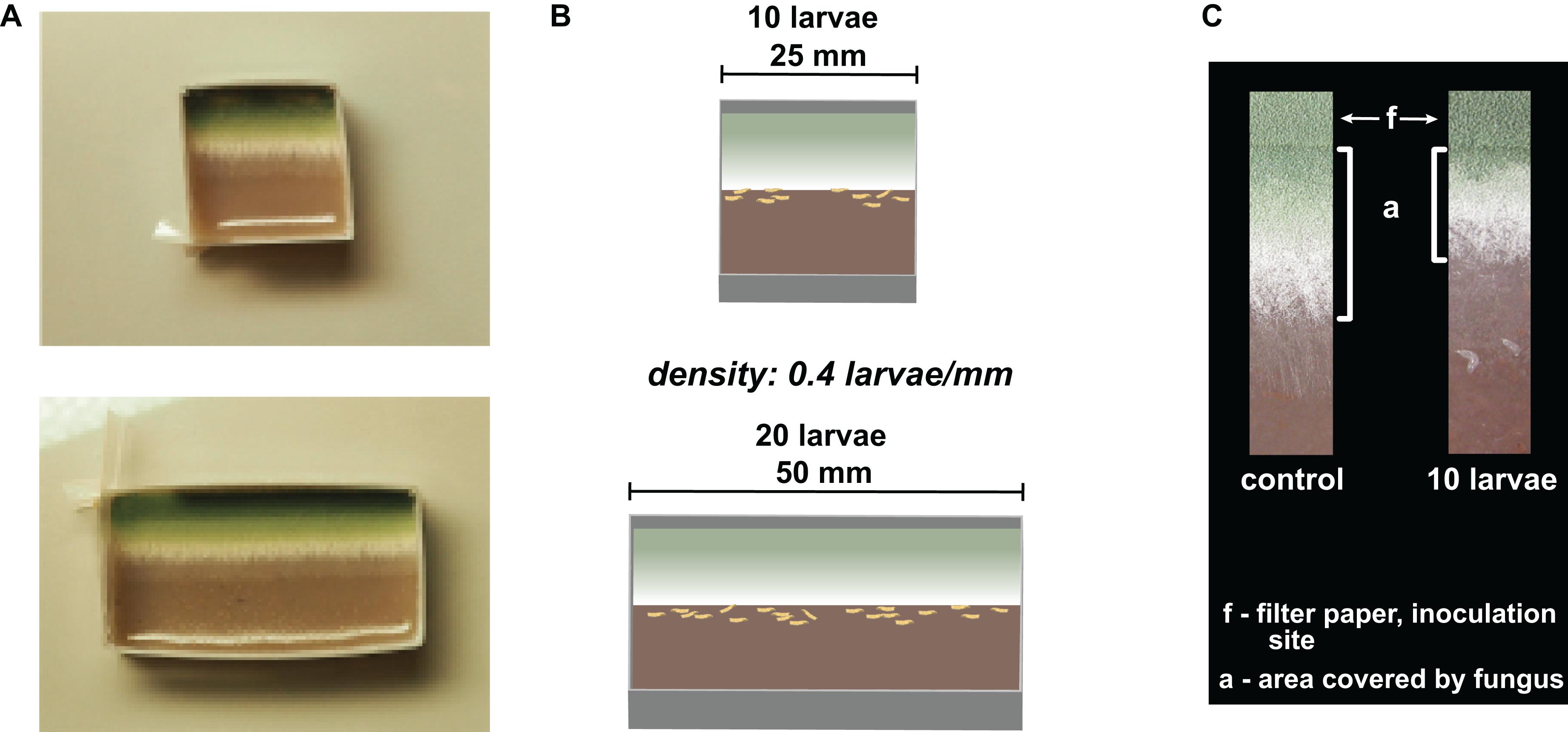
Figure 2. Illustration of experimental setup. (A) Pictures of experimental arenas with unconfronted Aspergillus nidulans colonies. (B) Schematic drawing of the experimental arenas. (C) Sections of unidirectionally growing mold (from top to bottom) without (control) and with 10 larvae. The aging and the fruiting zones (top) are characterized by the development of increasingly greener conidiospores. The younger productive zone can be recognized by the formation of aerial whitish hyphae, while the hyphae of the outer zone lie flat on the fruit agar or are slightly embedded in it. The feeding activity of Drosophila larvae is largely restricted to this outer zone of the mycelium, which makes the mold colonies less invasive. “a” is the area of undamaged fungal tissue that we quantified; “f” is the area covered by the filter paper inoculated with conidia.
To quantify the expansion of the fungi, we photographed the arenas 12, 24, 36, and 48 h after larval transfer and measured the substrate area covered by fungal tissue (“a” in Figure 2C) growing out of the filter paper (“f” in Figure 2C; using ImageJ; //imagej.net for measurement). In the insect treatment, the peripheral growth zone of the fungi was severely damaged by the feeding activity of the larvae. There were still few hyphal fragments visible in this growth zone, but these were not quantifiable, so we did not consider these fragments to be part of an intact colony (Figure 2C). To reduce variation due to observational errors, we performed three independent measurements of each colony and used their means for statistical analysis. The expansion of fungi on the substrate was quantified after 12, 24, 36, and 48 h of confrontation with the larvae as the average expansion per millimeter of growth front (in mm).
Experiment 1: Suppression of the Insecticidal Fungus Aspergillus nidulans by Differently Sized Groups of Drosophila melanogaster Larvae
To test the effect of larval group size difference on the expansion of a harmful fungus, in this first experiment, we used plastic frames of 25 × 25 mm and 50 × 25 mm, i.e., the latter was twice as large as the former. The plastic frames were filled with 4 and 8 ml fruit agar, respectively, inoculated, and incubated as described above. Before the transfer of larvae, the arenas were randomly assigned to the larval treatment. We transferred 10 first-instar larvae into the small arenas and 20 of them into the large arenas, resulting in a larval density of 0.4 larvae per millimeter of fungal growth front (larvae/mm) or 1.6 larvae per cm2 arena in both arena types. In total, there were 10 replicates per treatment, with an additional 10 larval-free control colonies per arena type. Due to the aberrant growth of the fungus in one small arena, we were forced to reduce the number of the previously assigned replicates for the larval treatment to nine. The arenas were prepared and incubated, and fungal growth was quantified as described in “General Experimental Setup”. The expansion of the fungi on the substrate was quantified after 12, 24, 36, and 48 h of confrontation with the larvae as average expansion per millimeter of growth front (in mm).
Experiment 2: Suppression of the Insecticidal Fungus Aspergillus nidulans by Differently Sized Groups of Drosophila melanogaster Larvae at Two Levels of Larval Density
The aim of this second experiment was twofold: first, to verify the previous observation made in Experiment 1 by using a wider range of group sizes and, second, to test whether the effect of group size can be observed at different larval densities. For this purpose, arenas with a size of 25, 50, or 75 × 25 mm were filled with 4, 8, or 12 ml fruit agar, respectively. The filter paper strips inoculated with conidia were adapted to the size of the arena. By reducing and increasing the number of larvae, we changed the differences between the groups compared to Experiment 1. We transferred groups of 5, 10, and 15 larvae, resulting in a constant larval density of 0.2 larvae per millimeter growth front of the fungus, or 20, 40, and 60 larvae, resulting in a constant larval density of 0.8 larvae per millimeter growth front of the fungus (Supplementary Figure S1). We conducted 20 replicates per combination; however, in individual cases, the final replicate number was reduced due to contaminations of the substrate. The arenas were prepared and incubated, and fungal growth was quantified as described in “General Experimental Setup.”
Moreover, to corroborate an overall density-dependent effect on fungal pathogen expansion in the insects breeding sites, we additionally transferred larvae in all group sizes to all arena sizes. This resulted, over all arena types, in a density range of 0.06 to 2.4 (that is, five larvae in 75-mm arenas to 60 larvae in 25-mm arenas; a full list of combinations is provided in Supplementary Tables S1, S2 and Supplementary Figure S1). The results of this density range experiment can be found in the Supplementary Material.
Experiment 3: Effect of Larval Drosophila melanogaster Foraging Phenotype on the Suppression of Insecticidal Aspergillus nidulans
Since the fly larvae actively seek out the fungus, the containment of the fungus is largely based on the behavior of the larvae. Based on this, we have analyzed whether genetic variation in the locomotor activity of D. melanogaster larvae contributes to differences in the suppression of fungal expansion. We used two D. melanogaster strains, “rover” and “sitter,” which differ in allelic variation at the foraging (for) locus and express two different behavioral phenotypes: the larvae of the “rover” phenotype travel longer distances during foraging compared to the “sitter” larvae (Sokolowski, 1985; de Belle et al., 1989). We used arenas of 25 and 50 × 25 mm and transferred groups of 10 and 20 larvae, respectively, with 10 replicates each. Fungal growth was quantified at 12, 24, 36, and 48 h after larval transfer.
To test whether there is indeed a variation in larval behavior that can be correlated with the variation in fungal suppression, we used the images taken after 12, 24, 36, and 48 h to count the number of larvae that were in contact with the fungal colonies. The arenas were prepared and incubated, and fungal growth was quantified as described in “General Experimental Setup”.
Statistics
In Experiment 1, we first tested whether the arena size had an impact on the expansion of unconfronted fungal colonies and, further, the null hypothesis that differences in larval group size do not significantly affect fungal expansion by applying generalized estimating equations (GEE) and fitting marginal generalized linear models (GLM), which take the repeated measures into account. We specified the model with a Gaussian distribution and identity link function, an auto-regressive correlation structure (on the basis of equally spaced cluster), and a fully iterated jackknife estimator. After model selection, fitting was estimated with Q-Q plots.
In concordance, we tested in Experiment 2 whether larval group size and larval density contributed to the variance in the data using the GEE. Different to the above-stated model specification, here we fitted a Gaussian distribution with a log link function (all other settings were the same as above). The effect of differences in the locomotion behavior of Drosophila larvae on fungal expansion (Experiment 3) was likewise analyzed using the GEE procedure with the same model settings as for Experiment 2.
The aggregation of larvae at the rim of the fungal colony was analyzed with a glm specifying a quasibinomial distribution of larvae counts in contact with fungal colony to account for overdispersion. In model specifications, larval group size was nested within foraging type, and time was specified as a random factor.
All statistic procedures were performed in R (R 3.6.1, R Core Team, 2019; “geepack” 1.2-1, Højsgaard et al., 2005; “car” 3.0-3).
Bioethics Statement
All aspects of the present research have been conducted in compliance with national and international legislation and fundamental ethical principles. Experimental work with the invertebrate D. melanogaster does not require specific measures regarding the Animal Welfare Act.
Results
Experiment 1: Suppression of the Insecticidal Fungus Aspergillus nidulans by Differently Sized Groups of Drosophila melanogaster Larvae
In our first experiment, the group size of D. melanogaster was 10 or 20 larvae with a constant density of 0.4 larvae per millimeter fungal growth front. Colony expansion was recorded 12 to 48 h post-larval transfer (Figure 3). This early phase of interaction of Drosophila larvae with filamentous mold fungi—about 25% of the insects’ development time—determines whether the fly larvae are able to suppress the growth of insecticidal mold fungi sustainably and survive until pupation (Rohlfs et al., 2005). In unconfronted colonies, the arena size had no effect on fungal expansion (analysis of Wald statistic χ2 = 0.031, p = 0.86; Figure 3, Table 1, Q-Q plot Supplementary Figure S2A). Compared to these unconfronted controls, the colonies of fungi exposed to fruit fly larvae expanded more slowly. In addition, the spread of the fungus was more suppressed by the larger group, with the effect of group size increasing over time (analysis of Wald statistic, time × group size: χ2 = 28.94, p < 0.001; Figure 3; Table 1, Q-Q plot Supplementary Figure S2B).
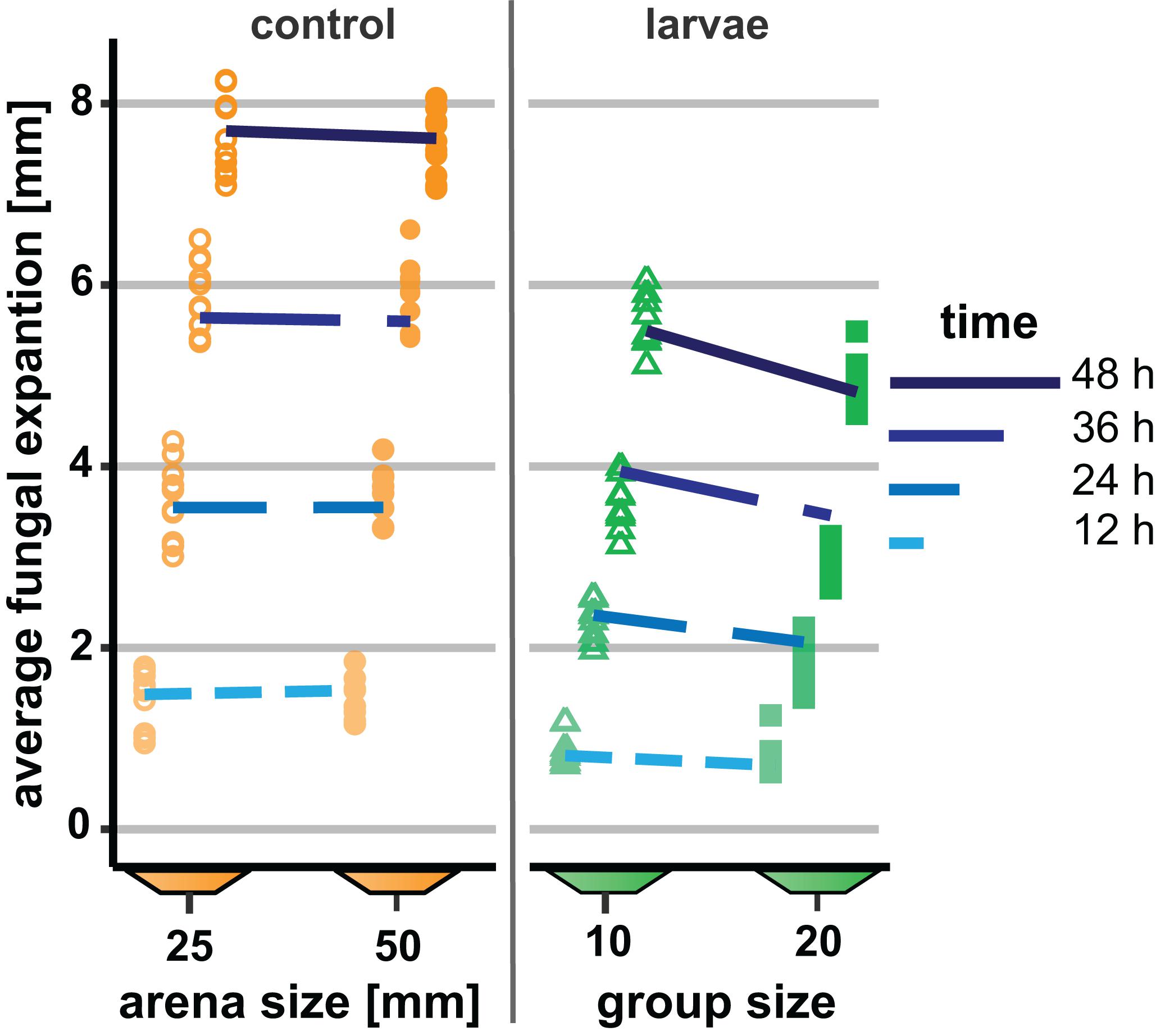
Figure 3. Effect of Drosophila melanogaster larvae at two different group sizes on the expansion of the insecticidal filamentous fungus Aspergillus nidulans. Expansion of the fungus across the substrate in small (open symbols) and large (filled symbols) arenas without larvae (“control,” left-hand side) and as affected by the presence of Drosophila larvae with 10 or 20 larvae (“larvae,” right-hand side). Dashed lines depict the fitted model estimates for the four time points. For the insect treatment, the density of larvae was 0.4/mm fungal growth front and 1.6/cm2 arena in both the small and the large arenas. The GEE was applied separately for arenas with and without larvae, while larva-free fungal colonies showed no significant difference between arena sizes; confronted colonies were more suppressed over time by larger larval groups (Table 1).
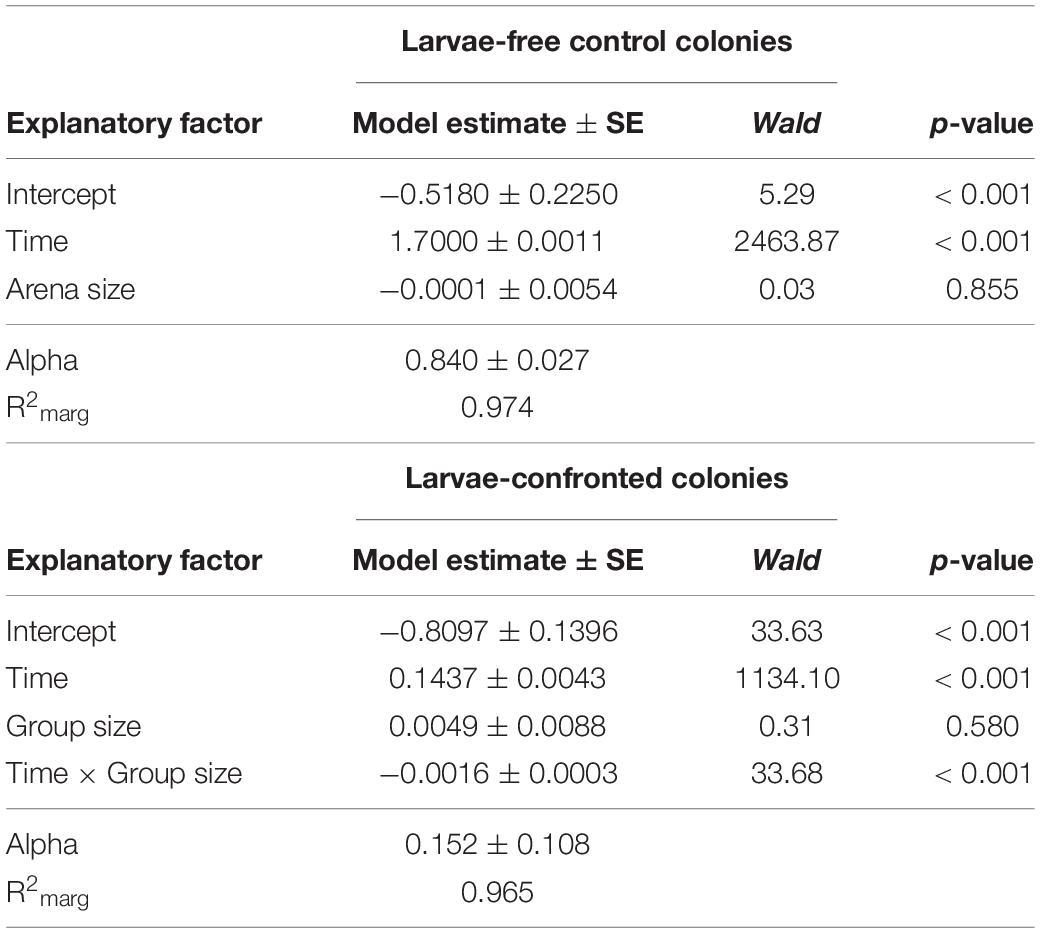
Table 1. Factors influencing expansion of Aspergillus nidulans in Experiment 1. Results of the most parsimonious GEE model (after backward elimination of non-significant factors) for larvae-free and larvae-confrontedfungal colonies are shown.
Experiment 2: Suppression of the Insecticidal Fungus Aspergillus nidulans by Differently Sized Groups of Drosophila melanogaster Larvae at Two Levels of Larval Density
To verify the group size effect observed in Experiment 1, we repeated and extended this experiment by increasing the number of arena types and thus the number of possible group sizes. Moreover, group size variation was tested at two different density levels, 0.2 and 0.8 larvae per millimeter growth front of the fungus. As in Experiment 1, the mycelial expansion was significantly reduced, with increasing group size as a function of time (analysis of Wald statistic, time × group size: χ2 = 36.56, p < 0.001; Figure 4 and Table 2), thus verifying the previously made observation. Moreover, density also contributed significantly to the reduction of fungal expansion in a time-dependent manner (analysis of Wald statistic, time × density: χ2 = 67.40, p < 0.001; Figure 4, Table 2, Q-Q plot Supplementary Figure S2C). This means that while the effect of group size is apparent at later points in time, the effect of larval density on fungal expansion is stronger at earlier points in time. However, the absence of a statistically significant three-way interaction between time × density × group size (analysis of Wald statistic χ2 = 1.48, p = 0.22, Table 2) suggests that the overall effect of larval group size on fungal control is not different at the two densities.
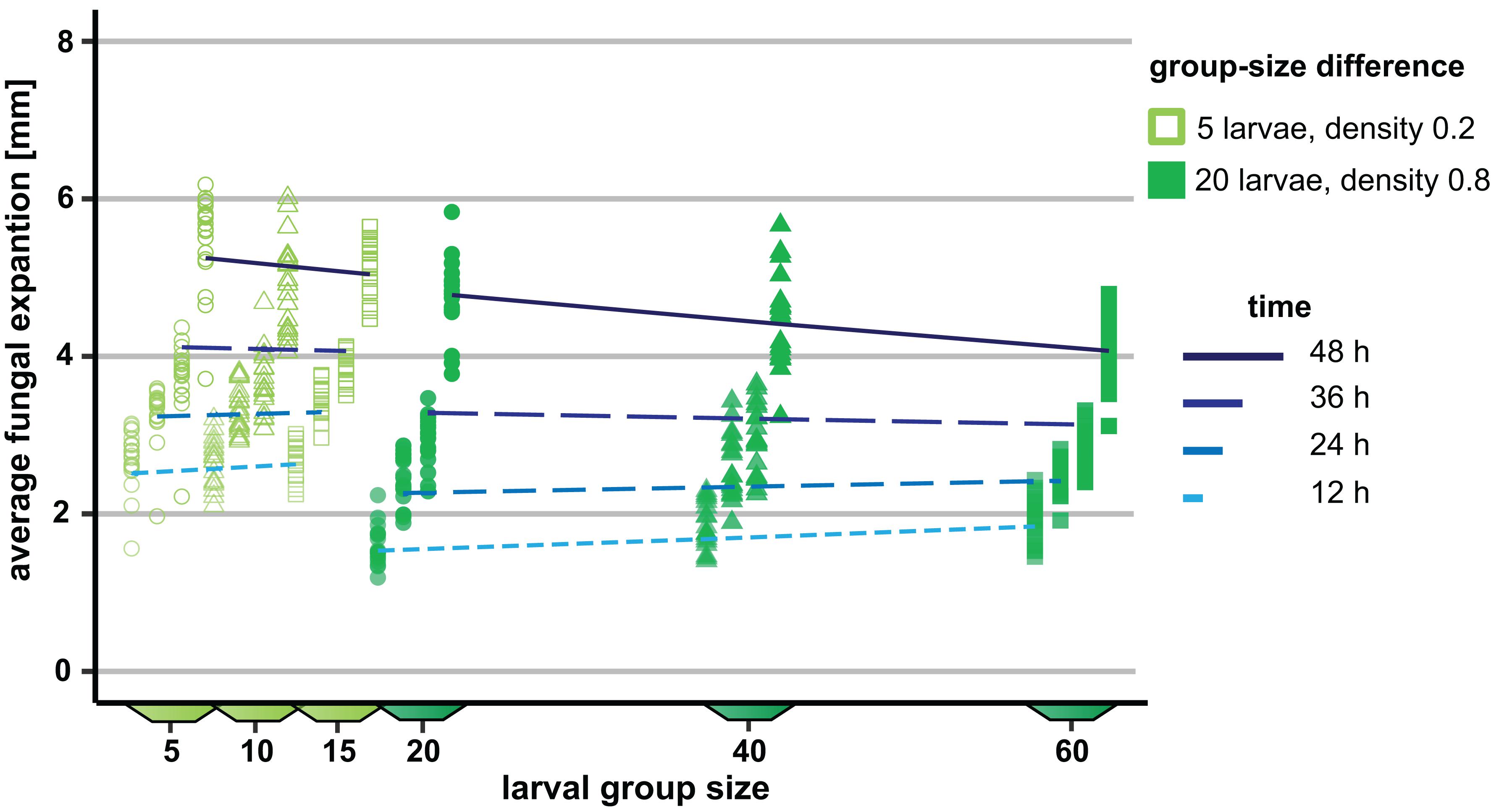
Figure 4. Influence of variation in the group size of Drosophila melanogaster larvae on the spread of the insecticidal filamentous fungus Aspergillus nidulans. The influence of the three different group sizes on the expansion of the fungus was quantified at two different densities (0.2 and 0.8 larvae per millimeter of fungal growth front) at four different points in time. The differences in group size were 5 and 20 at the two density levels, respectively. For the sake of clarity, the data points within one group size per time are shown slightly shifted along the x-axis. Lines represent the adjusted model estimates for the four time points (generalized estimation equation).
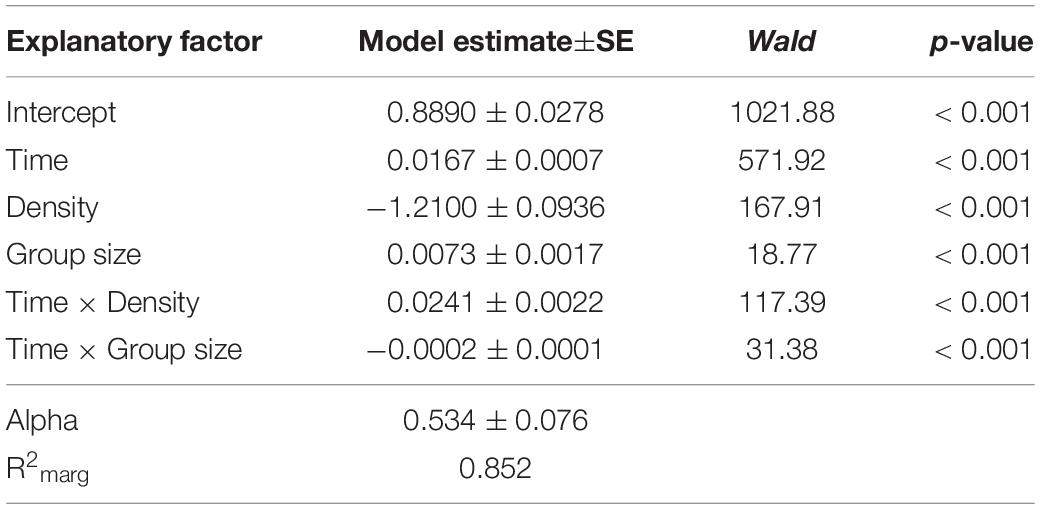
Table 2. Factors influencing the expansion of Aspergillus nidulans in Experiment 2. Results of the most parsimonious GEE model (after backward elimination of non-significant factors) are shown.
The density–range experiment revealed highly significant differences in fungal growth expansion. With increasing larval densities, fungal growth was increasingly strongly suppressed (analysis of Wald statistic, time × group size difference × density–range: χ2 = 65.20, p < 0.001; Supplementary Figure S3, Supplementary Table S3, Q-Q plot Supplementary Figure S2D).
Experiment 3: Effect of Larval Drosophila melanogaster Foraging Phenotype on the Suppression of Insecticidal Aspergillus nidulans
To explore the possible contribution of genetic variation in larval locomotor activity to the suppression of A. nidulans, we quantified the effect of allelic variation at the for locus on the spread of the mold fungus. The sitter phenotype turned out to suppress the expansion of A. nidulans more than the rover phenotype (analysis of Wald statistic, rover vs sitter: χ2 = 8.0, p = 0.004; Figure 5A and Table 3, Q-Q plot Supplementary Figure S2E). Generally, larger groups suppressed fungal expansion more effectively than smaller groups in a time dependent manner (analysis of Wald statistic, time × group size: χ2 = 6.0, p = 0.017; Figure 5A and Table 3).
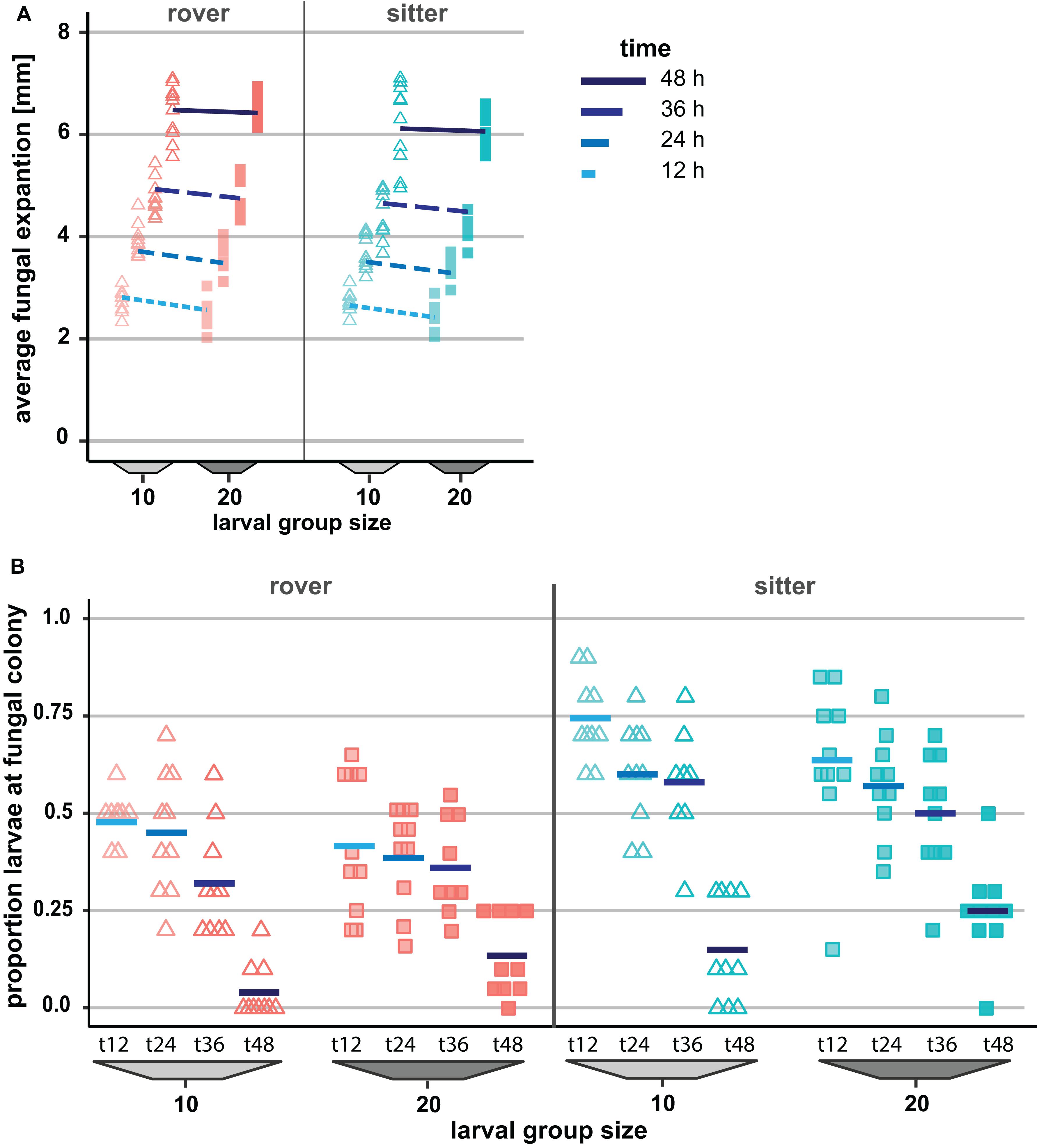
Figure 5. Effect of genetically determined variation in the locomotion behavior of Drosophila melanogaster larvae on the spread of the insecticidal filamentous fungus Aspergillus nidulans. (A) Inhibition of the fungal expansion by rover and sitter Drosophila larvae in different group sizes (constant density = 0.4 larvae/mm). Fungal growth was determined for four time points after larval transfer as the average expansion of the fungus into the substrate surface per millimeter of growth front. Lines represent the fitted model estimates for the four time points (GEE; Table 4). (B) Proportion of larvae in direct contact with the fungal colonies at four different time points after larval transfer (Table 4).
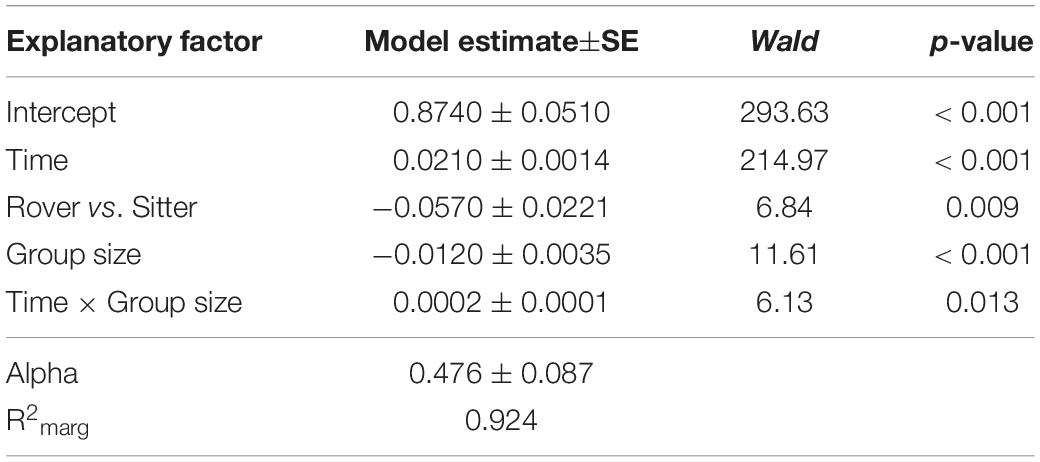
Table 3. Factors influencing expansion of Aspergillus nidulans in Experiment 3. Results of the most parsimonious GEE model (after backward elimination of non-significant factors) are shown.
Variation in the foraging phenotype possibly leads to differences in larval behavior directed against the fungus, which might provide an explanation for why the genotypes differ in their quantitative effect on the fungus. To test this possibility, we calculated the proportion of larvae found to aggregate at the growth front of the fungus and are in touch with the mycelium. Based on the analysis of the images taken 12 to 48 h post-larvae transfer for fungal growth measurements, we found that a higher proportion of larvae of the sitter phenotype was in direct contact with the fungal colony than the larvae of the rover phenotype (analysis of deviance, type II: rover vs. sitter F1,155 = 51.656, p < 0.001; time: F1,155 = 126.40, p < 0.001; time × group size: F1,155 = 6.449, p = 0.0121; Figure 5B, Table 4, Q-Q plot Supplementary Figure S2F). Further, the decline of larvae in contact with the colony at time point 48 h coincides with the generic behavior of D. melanogaster larvae to leave the substrate surface around the beginning of the third larval stage, where they start digging deeper into the substrate.
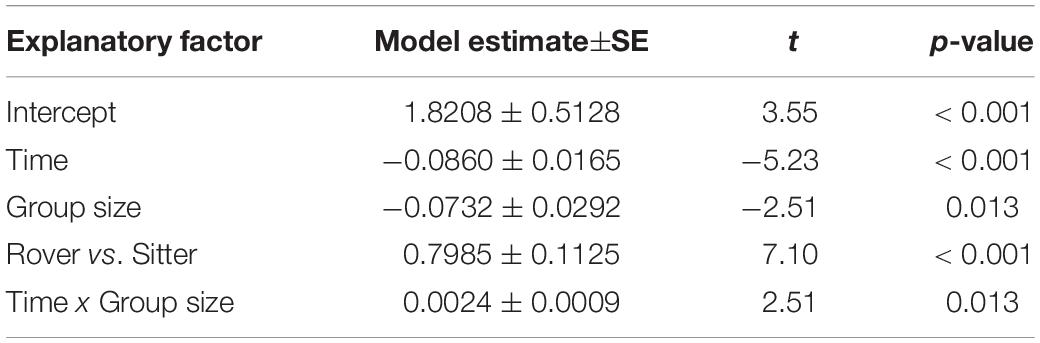
Table 4. Factors influencing the proportion of Drosophila melanogaster larvae found in contact with Aspergillus nidulans colonies in Experiment 3. Results of the most parsimonious GLM (after backward elimination of non-significant factors) are shown.
Discussion
The existence and effect size of collective actions, such as defense, can be inferred from the extent to which the efficiency of performing a particular fitness-related “task” increases with increasing group size, while the density of individuals involved remains constant (Cotter and Kilner, 2010; Dornhaus et al., 2012). In a series of independent experiments, we tested whether larvae of the fruit fly D. melanogaster can collectively increase the efficiency of such a “task” that is directed against an insecticidal fungus invading the insects’ habitat. While insect density clearly is, as expected, a significant factor in the suppression of the harmful fungus A. nidulans, we repeatedly found that larvae in larger groups, regardless of these density effects, suppressed the invasion of this fungus into their habitat more sustainably. This indicates that the emergence of a collective action in larger larval groups may constitute a central yet overlooked component of the suppression of harmful microbes in fruit fly larvae.
The aggregation of larvae on colonies of filamentous fungi probably results from a sensory response evolved in the context of the detection of food microbes, e.g., yeast fungi (Cooper, 1960), because both yeast and mold fungi emit volatile metabolites to which fruit fly larvae are attracted (Stötefeld et al., 2015). In previous observations, we found that a mold–fruit substratum can indeed serve as a benign dietary environment when A. nidulans is genetically (Trienens et al., 2010; Caballero Ortiz et al., 2013) or chemically (Caballero Ortiz et al., 2018) impaired in the production of toxic metabolites that otherwise deter, weaken, or even kill fly larvae. It therefore remains to be seen to what extent the aggregation of larvae on harmful fungi is due to an ancestral attraction to food microbes.
When D. melanogaster larvae reach the fungal growth front, they reduce their roving activity and display intensive mouth hook movements on the mycelium without crawling further to the center of the fungal colony (Figure 2C). This response leads to visible disruption of young exploitative hyphae, reduced formation of aerial hyphae, and impaired development of reproductive organs, i.e., a smaller fruiting zone (Figure 2C) (see also Trienens et al., 2010). To identify the exact mechanisms underlying the collectively caused suppression of the fungus, it will be important to test whether variation in group size induces changes in the expression of individual larval behavioral or physiological traits (e.g., release of antimicrobial peptides; Rolff and Schmid-Hempel, 2016). As it has been proposed for group size-dependent emergence of collective behaviors in ants (Dornhaus et al., 2012), we hypothesize that group size-dependent changes in the antimicrobial traits of fruit fly larvae would render larger larval interaction networks more effective in gaining control of their habitat.
Understanding how antimicrobial traits contribute to the formation of collective patterns from the interaction of individuals requires a combination of detailed observation of individual behavior and physiological approaches. Given that we found variation in larval locomotor activity—as determined by variation in the foraging locus—to differentially influence fungal growth suggests that behavior may indeed be a crucial factor in building up collective defense actions. This is corroborated by our observation that the larvae of the sitter phenotype appear to have more intensive contact with the fungus than that of the rover phenotype. That is, there seems to be genetic variation in how the insects respond to a harmful fungus at the level of behavior, which has consequences for the efficiency of microbial control. Whether this phenotypic variation has a function in the emergence of a collective defense in mixed genotype populations (Jolles et al., 2020) remains to be investigated for the Drosophila system.
Explaining the emergence of social complexity in insect communities is one of the chief problems in evolutionary ecology. By separating group size from density effects, our results suggest that non-(eu)social D. melanogaster fruit fly larvae harbor the potential to collectively suppress the invasion of a harmful filamentous fungus. It has been repeatedly stated that collective actions that keep harmful microbes in check are not limited to eusocial insects or those with sophisticated parental care in small family groups (Biedermann and Taborsky, 2011; Hwang and Lin, 2013; Meunier, 2015). On the contrary, it is assumed that collective actions have their evolutionary origin in comparatively simple semi-social aggregation behaviors, such as the Drosophila system, which lack comparatively complex behavioral structuring, nest building, and altruism (Meunier, 2015; Biedermann and Rohlfs, 2017).
Our finding thus supports the suggestions made by Meunier (2015) or van Meyel et al. (2018) that, in tracing the origin of complex collective defenses, such as social immunity, we need to better understand the extent to which non-(eu)social insects have evolved group-based strategies to manage the microbial environment and thereby stabilize habitat conditions to the benefit of their fitness (Biedermann and Rohlfs, 2017). Several recent studies have started revealing the mechanisms underlying social interactions, incl. collective actions, between Drosophila larvae, as related to food choice (Lihoreau et al., 2016), spatial foraging (Dombrovski et al., 2017), microbiome- and pheromone-mediated mutual attraction (Mast et al., 2014; Venu et al., 2014), and physical contact (Otto et al., 2016). Drosophila fruit flies and their interactions with microbes could thus be a suitable model system that would allow us to better understand how social complexity in insects can evolve from behavior in simple social precursor systems.
Data Availability Statement
Raw data are available as Supplementary Material in Table 1. XLSX and additional information in Data Sheet 1.docx.
Author Contributions
MR and MT designed the study, did the statistical analyses, and wrote the manuscript. MT performed the experiments and generated the data.
Funding
This work was funded by DFG (German Research Foundation) research grants to MR (RO3523/3-1 and 3-2).
Conflict of Interest
The authors declare that the research was conducted in the absence of any commercial or financial relationships that could be construed as a potential conflict of interest.
Acknowledgments
We thank Mark J. Fitzpatrick, University of Toronto, for providing the Drosophila rover/sitter strains.
Supplementary Material
The Supplementary Material for this article can be found online at: https://www.frontiersin.org/articles/10.3389/fevo.2020.00079/full#supplementary-material
References
Anreiter, placeI., and Sokolowski, M. B. (2019). The foraging gene and its behavioral effects: pleiotropy and plasticity. Annu. Rev. Genet. 53, 373–392. doi: 10.1146/annurev-genet-112618-043536
Biedermann, P. H. W., and Rohlfs, M. (2017). Evolutionary feedbacks between insect sociality and microbial management. Curr. Opin. Insect Sci. 22, 92–100. doi: 10.1016/J.COIS.2017.06.003
Biedermann, P. H. W., and Taborsky, M. (2011). Larval helpers and age polyethism in ambrosia beetles. Proc. Natl. Acad. Sci. U.S.A. 108, 17064–17069. doi: 10.1073/pnas.1107758108
Caballero Ortiz, S., Trienens, M., Pfohl, K., Karlovsky, P., Holighaus, G., and Rohlfs, M. (2018). Phenotypic responses to microbial volatiles render a mold fungus more susceptible to insect damage. Ecol. Evol. 8, 4328–4339. doi: 10.1002/ece3.3978
Caballero Ortiz, S., Trienens, M., and Rohlfs, M. (2013). Induced fungal resistance to insect grazing: reciprocal fitness consequences and fungal gene expression in the Drosophila-Aspergillus model system. PLoS One 8:e74951. doi: 10.1371/journal.pone.0074951
Cooper, D. M. (1960). Food preferences oflarval and adult Drosophila. Evolution 14, 41–55. doi: 10.2307/2405921
Cotter, S. C., and Kilner, R. M. (2010). Personal immunity versus social immunity. Behav. Ecol. 21, 663–668. doi: 10.1093/beheco/arq070
Cremer, S., Armitage, S. A. O., and Schmid-Hempel, P. (2007). Social immunity. Curr. Biol. 17, R693–R702. doi: 10.1016/J.CUB.2007.06.008
de Belle, J. S., Hilliker, A. J., and Sokolowski, M. B. (1989). Genetic localization of foraging (for): a major gene for larval behavior in Drosophila melanogaster. Genetics 123, 157–163.
Dombrovski, M., Poussard, L., Moalem, K., Kmecova, L., Hogan, N., Schott, E., et al. (2017). Cooperative behavior emerges among Drosophila larvae. Curr. Biol. 27, 2821–2826. doi: 10.1016/j.cub.2017.07.054
Dornhaus, A., Powell, S., and Bengston, S. (2012). Group size andits effects on collective organization. Annu. Rev. Entomol. 57, 123–141. doi: 10.1146/annurev-ento-120710-100604
Drott, M. T., Lazzaro, B. P., Brown, D. L., Carbone, I., and Milgroom, M. G. (2017). Balancing selection for aflatoxin in Aspergillus flavus is maintained through interference competition with, and fungivory by insects. Proc. R. Soc. B Biol. Sci. 284:20172408. doi: 10.1098/rspb.2017.2408
Hodge, S., Mitchell, P., and Arthur, W. (1999). Factors affecting the occurrence of facilitative effects in interspecific interactions: an experiment using two species of Drosophila and Aspergillus niger. Oikos 87, 166–174. doi: 10.2307/3547007
Højsgaard, S., Halekoh, U., and Jun, Y. (2005). The R package geepack for generalized estimating equations. J. Stat. Softw. 15, 1–11. doi: 10.18637/jss.v015.i02
Hwang, W., and Lin, H.-M. (2013). Carcass fungistasis of the burying beetle Nicrophorus nepalensis Hope (Coleoptera: Silphidae). Psyche 2013:162964. doi: 10.1155/2013/162964
Janzen, D. H. (1977). Why fruits rot, seeds mold, and meat spoils. Am. Nat. 111, 691–713. doi: 10.1086/283200
Jolles, J. W., King, A. J., and Killen, S. S. (2020). The role of individual heterogeneity in collective animal behaviour. Trends Ecol. Evol. 35, 278–291. doi: 10.1016/j.tree.2019.11.001
Kappeler, P. M., Cremer, S., and Nunn, C. L. (2015). Sociality and health: impacts of sociality on disease susceptibility and transmission in animal and human societies. Philos. Trans. R. Soc. Lond. B. Biol. Sci. 370:20140116. doi: 10.1098/rstb.2014.0116
Künzler, M. (2018). How fungi defend themselves against microbial competitors and animal predators. PLoS Pathog. 14:e1007184. doi: 10.1371/journal.ppat.1007184
Lihoreau, M., Clarke, I. M., Buhl, J., Sumpter, D. J. T., and Simpson, S. J. (2016). Collective selection of food patches in Drosophila. J. Exp. Biol. 219, 668–675. doi: 10.1242/jeb.127431
Mast, J. D., De Moraes, C. M., Alborn, H. T., Lavis, L. D., and Stern, D. L. (2014). Evolved differences in larval social behavior mediated by novel pheromones. eLife 3:e04205. doi: 10.7554/eLife.04205
Meunier, J. (2015). Social immunity and the evolution of group living in insects. Philos. Trans. R. Soc. B Biol. Sci. 370:20140102. doi: 10.1098/rstb.2014.0102
Nuotclà, J. A., Biedermann, P. H. W., and Taborsky, M. (2019). Pathogen defence is a potential driver of social evolution in ambrosia beetles. Proc. R. Soc. B Biol. Sci. 286:20192332. doi: 10.1098/rspb.2019.2332
Otto, N., Risse, B., Berh, D., Bittern, J., Jiang, X., and Klämbt, C. (2016). Interactions among Drosophila larvae before and during collision. Sci. Rep. 6:31564. doi: 10.1038/srep31564
R Core Team (2019). R: A Language and Environment for Statistical Computing. Vienna: R Foundation for Statistical Computing.
Rohlfs, M. (2005). Density-dependent insect-mold interactions: effects on fungal growth and spore production. Mycologia 97, 996–1001. doi: 10.1080/15572536.2006.11832749
Rohlfs, M., and Hoffmeister, T. S. (2003). An evolutionary explanation of the aggregation model of species coexistence. Proc. R. Soc. London. Ser. B Biol. Sci. 270, S33–S35. doi: 10.1098/rsbl.2003.0002
Rohlfs, M., Obmann, B., and Petersen, R. (2005). Competition with filamentous fungi and its implication for a gregarious lifestyle in insects living on ephemeral resources. Ecol. Entomol. 30, 556–563. doi: 10.1111/j.0307-6946.2005.00722.x
Rolff, J., and Schmid-Hempel, P. (2016). Perspectives on the evolutionary ecology of arthropod antimicrobial peptides. Philos. Trans. R. Soc. B Biol. Sci. 371:20150297. doi: 10.1098/rstb.2015.0297
Sokolowski, M. B. (1985). Genetics and ecology of Drosophila melanogaster larval foraging and pupation behaviour. J. Insect Physiol. 31, 857–864. doi: 10.1016/0022-1910(85)90103-90109
Stötefeld, L., Holighaus, G., Schütz, S., and Rohlfs, M. (2015). Volatile-mediated location of mutualist host and toxic non-host microfungi by Drosophila larvae. Chemoecology 25, 271–283. doi: 10.1007/s00049-015-0197-192
Trienens, M., Keller, N. P., and Rohlfs, M. (2010). Fruit, flies and filamentous fungi - experimental analysis of animal-microbe competition using Drosophila melanogaster and Aspergillus as a model system. Oikos 119, 1765–1775. doi: 10.1111/j.1600-0706.2010.18088.x
van Meyel, S., Körner, M., and Meunier, J. (2018). Social immunity: why we should study its nature, evolution and functions across all social systems. Curr. Opin. Insect Sci. 28, 1–7. doi: 10.1016/J.COIS.2018.03.004
Venu, I., Durisko, Z., Xu, J., and Dukas, R. (2014). Social attraction mediated by fruit flies’ microbiome. J. Exp. Biol. 217, 1346–1352. doi: 10.1242/jeb.099648
Wertheim, B., Marchais, J., Vet, L. E. M., and Dicke, M. (2002). Allee effect in larval resource exploitation in Drosophila: an interaction among density of adults, larvae, and micro-organisms. Ecol. Entomol. 27, 608–617. doi: 10.1046/j.1365-2311.2002.00449.x
Wertheim, B., van Baalen, E.-J. A., Dicke, M., and Vet, L. E. M. (2005). Pheromone-mediated aggregation in non-social arthropods: an evolutionary ecological perspective. Annu. Rev. Entomol. 50, 321–346. doi: 10.1146/annurev.ento.49.061802.123329
Keywords: aggregation, density dependence, Drosophila, foraging phenotype, group size, group living, pathogenic fungi, social immunity
Citation: Trienens M and Rohlfs M (2020) A Potential Collective Defense of Drosophila Larvae Against the Invasion of a Harmful Fungus. Front. Ecol. Evol. 8:79. doi: 10.3389/fevo.2020.00079
Received: 25 November 2019; Accepted: 11 March 2020;
Published: 14 May 2020.
Edited by:
Heikki Helanterä, University of Oulu, FinlandReviewed by:
Christopher Pull, Royal Holloway, University of London, United KingdomFranziska Dickel, University of Graz, Austria
Copyright © 2020 Trienens and Rohlfs. This is an open-access article distributed under the terms of the Creative Commons Attribution License (CC BY). The use, distribution or reproduction in other forums is permitted, provided the original author(s) and the copyright owner(s) are credited and that the original publication in this journal is cited, in accordance with accepted academic practice. No use, distribution or reproduction is permitted which does not comply with these terms.
*Correspondence: Monika Trienens, bS50cmllbmVuc0BydWcubmw=; Marko Rohlfs, cm9obGZzMUB1bmktYnJlbWVuLmRl
†ORCID: Monika Trienens, b3JjaWQub3JnLzAwMDAtMDAwMi0xODc0LTExNjA=; Marko Rohlfs, b3JjaWQub3JnLzAwMDAtMDAwMi04NzY3LTE2Mjk=