- 1Department of Biology, Advanced Facility for Avian Research, University of Western Ontario, London, ON, Canada
- 2Department of Psychology, Advanced Facility for Avian Research, University of Western Ontario, London, ON, Canada
Birds are often able to cope with, and respond to, inclement weather with physiological and behavioral responses. As storms become more severe or frequent as a result of climate change, the adaptive coping responses of many species may be pushed beyond current tolerance limits. We investigated the effects of experimental recurrent inclement winter weather cues on body composition, glucocorticoid hormones, and behavior of white-throated sparrows (Zonotrichia albicollis). We used a hypobaric climatic wind tunnel to simulate storms by transiently decreasing barometric pressure and temperature, and measured behavioral responses, body composition, and baseline corticosterone levels in birds exposed, or not exposed (control), to different frequencies of simulated storms. In study 1, experimental birds were exposed to one storm per week over 9 weeks. In study 2, experimental birds were exposed to two storms per week over 12 weeks. Birds exposed to one simulated storm per week had higher fat and lean masses than control birds, with no differences in the amount of time groups spent feeding. This change in body composition suggests that birds were coping by increasing energy stores. In contrast, birds exposed to two simulated storms per week had lower fat masses compared to control birds, even though they spent more time feeding. Experimental birds in study 2 also had lower baseline corticosterone levels than controls. These changes suggest that the coping response observed in study 1 was not possible in study 2. These findings provide novel experimental evidence that birds detect and respond to changes in temperature and barometric pressure independent of other storm-related cues. One simulated storm per week resulted in potentially adaptive responses of increased mass. However, increasing the frequency of storm exposure to twice per week exceeded the birds’ capacity to maintain these energy reserves. These results also experimentally demonstrate that repeated exposure to inclement weather cues can directly affect birds’ energy reserves, even in the absence of a storm itself, with strong implications for survival as severe weather events continue to become more prevalent.
Introduction
Birds are exposed to seasonal and daily fluctuations in environmental conditions. Predictable fluctuations are typically accommodated through biological rhythms in physiology and behavior. However, less predictable fluctuations such as rapid decline in resource availability, disease exposure, predator interactions and inclement weather can vary across temporal and spatial scales, requiring coping responses with the potential of entering an emergency life history stage (Wingfield et al., 1998). Weather conditions, specifically, can be a major factor affecting individual survival and reproductive success, both directly and indirectly (Newton, 1998). Many of the environmental factors associated with storms can pose challenges with respect to foraging behavior (Boyle et al., 2010; Breuner et al., 2013), migration (Newton, 2007; James and Abbott, 2014), territorial defense (Breuner and Hahn, 2003) and mate acquisition (Wingfield, 1985; Vitousek and Romero, 2013). Inclement weather and storms can create life threatening conditions that may continue to negatively impact individuals after the storm passes.
Although there are no universally accepted definitions of stress, many define a stressor, in part, as an unpredictable challenge (Romero, 2012). However, unpredictability alone may not induce a stress response if it does not pose a threat to an organism. For example, if an unpredictable severe weather event occurs, it may not be perceived as a stressor to birds if they have maintained energy reserves and there are ample food resources. Alternatively, a storm may be perceived as a stressor if food availability and energy reserves are low. This may result in the animal entering an emergency life-history stage since the current metabolic demands of the birds cannot be met (Wingfield et al., 1998). When birds encounter a stressor and the emergency life-history stage is induced, physiological and behavioral responses are activated, including corticosterone secretion, reallocation of energy towards self-maintenance, and increased feeding behavior (Wingfield et al., 1998; Reneerkens et al., 2002). Factors including food availability and storm severity can thus influence whether a storm will be perceived as a stressor. Additionally, the frequency of unpredictable inclement weather may influence this response. Frequent acute stressors can cumulatively create a condition of chronic stress (Busch et al., 2008). Thus, repeated storms may act cumulatively as a chronic stressor and induce longer-term endocrine responses. Though corticosterone levels typically rise rapidly in response to an acute stressor, chronic stress may induce increased or decreased baseline corticosterone levels (Dickens and Romero, 2013).
Birds’ responses to environmental perturbations can be deployed over shorter time scales to cope with inclement weather, or over longer time scales to cope with prolonged winter conditions (Carey and Dawson, 1999). These coping responses include increasing foraging activity (Metcalfe et al., 2013), facultative migrations (Boyle et al., 2010), temporarily abandoning territories (Streby et al., 2015) or nests (Thierry et al., 2013), or ceasing normal life-history stages altogether (Wingfield et al., 1998). Food availability affects how a bird will behaviorally respond, particularly during energetically demanding life-history stages or in response to energetically demanding perturbations (Carey and Dawson, 1999). Food availability can also dictate whether a bird continues with, or ceases, a life-history stage.
Rapid but transient changes in the abiotic environment, particularly in inclement weather events, are common in most terrestrial habitats. Species have evolved adaptations to cope with such events, however, climate change may be pushing some species to their tolerance limit (Freeman and Class Freeman, 2014), depending on the degree of variation in adaptive traits that currently exist in the population (Charmantier and Gienapp, 2014). As climate change advances, rapid fluctuations in abiotic factors such as temperature, precipitation, wind, and storms are increasing in severity and frequency across the globe (IPCC, 2014). It is thus important to explore both how organisms respond to individual storms, and how they cope with repeated exposure to storms.
To fully understand birds’ responses to inclement weather cues, we cannot solely rely on correlational field observations. A full understanding of the responses to storms requires experimental studies where researchers can have some form of control over environmental conditions. The responses or coping mechanisms of birds to inclement weather have only recently been studied in experimental settings (Breuner et al., 2013; Metcalfe et al., 2013). Prior research has focused on how birds cope with cold winter weather (Rogers et al., 1993; Vézina et al., 2006) and their ability to predict oncoming inclement weather using cue detection of barometric pressure and temperature (Breuner et al., 2013; Metcalfe et al., 2013), but little to no research has assessed how birds respond to repeated storms over long-term periods. As well, most prior research on birds’ responses to storms has focused on the breeding season and/or migration, and we have fewer data on how birds cope with storms during winter.
In this study, our objective was to determine if birds differentially respond to lower or higher rates of repeated exposure to inclement winter weather cues and whether increased exposure frequency would cause cumulative effects. Thus, we tested the hypothesis that an increased rate of exposure to inclement weather cues would elicit greater physiological and behavioral responses. We predicted that birds exposed to storm cues would exhibit changes in body composition, baseline corticosterone levels, overall movement, and feeding behavior. Birds exposed to frequent repeated winter storm cues may respond to these repeated acute stressors as chronic stressors and increase baseline corticosterone levels. We also predicted that high-frequency exposed birds should increase fat and overall body mass, as accumulating fat reserves is a main coping technique employed by wintering birds (Carey and Dawson, 1999; Kelly et al., 2002). However, if repeated storms occur more quickly than the birds’ ability to deposit fat we may not see this increase in mass. Repeated exposure to simulated storm cues may not be stressful to a bird if there are excess food and energy reserves available, but if a storm occurs when food availability and energy reserves are low (i.e., birds receiving limited food access), there may be a higher perceived threat to survival and subsequent stress response, and eventually changes in baseline corticosterone levels. Thus, we also manipulated food availability (fixed diet or ad libitum) to determine if responses to storm cues are modulated by limited food availability.
Materials and Methods
We assessed the physiological and behavioral responses of white-throated sparrows (Zonotrichia albicollis) to either low-frequency (once per week over nine weeks; study 1) or high-frequency (twice per week over 12 weeks; study 2) winter storm cues. These cues were simulated low-pressure systems with an associated cold front, representing inclement winter weather conditions. In the northern hemisphere, some of the most severe winter storms are associated with a low-pressure system and an accompanied cold front (Ahrens, 2012). We used a hypobaric climatic wind tunnel to experimentally control barometric pressure, temperature, and relative humidity. For each simulated storm cue exposure, we manipulated barometric pressure and temperature over approximately a 24 h period. For the first 6 h, barometric pressure and temperature would both steadily decrease until a setpoint was reached, conditions would remain constant for about 13–14 h overnight, and then increase back to housing conditions for the remainder of the 24 h. These manipulations were meant to simulate a rapid, yet realistic, storm front. Within the high-frequency experiment (study 2), half of the birds received unlimited food availability and the remaining half received limited food.
Study Species
White-throated sparrows are short-distance migrants that breed in the North American boreal forest, but still experience inclement cold winter weather conditions throughout most of their wintering range (Falls and Kopachena, 1994). Although winters in this region are becoming milder overall, there will likely be more intense and more frequent winter storms in the future (Francis and Vavrus, 2012; IPCC, 2014). Thus, the presence of inclement winter weather conditions on their wintering and breeding grounds, and their ability to adjust particularly well to captivity, make white-throated sparrows an excellent species to study the effects of inclement weather cues.
Environmental Control System; Hypobaric Climatic Wind Tunnel
We used a hypobaric climatic wind tunnel at the Advanced Facility of Avian Research, University of Western Ontario, London, Ontario, to mimic inclement weather conditions. Although the wind tunnel is normally used to study bird flight under different environmental conditions, we used the tunnel to experimentally simulate weather events (as in Metcalfe et al., 2013). The wind tunnel can control temperature between −15 and 30°C and barometric air pressure from ambient (average ∼101 kPa) down to ∼37 kPa (equivalent to ∼7000 m in altitude), and thus can be used to simulate weather-related changes in temperature and pressure (Metcalfe et al., 2013; Pellegrino et al., 2013). We housed birds in the hypobaric room (hereafter plenum) within the wind tunnel structure and did not fly birds within the tunnel section (Figure 1).
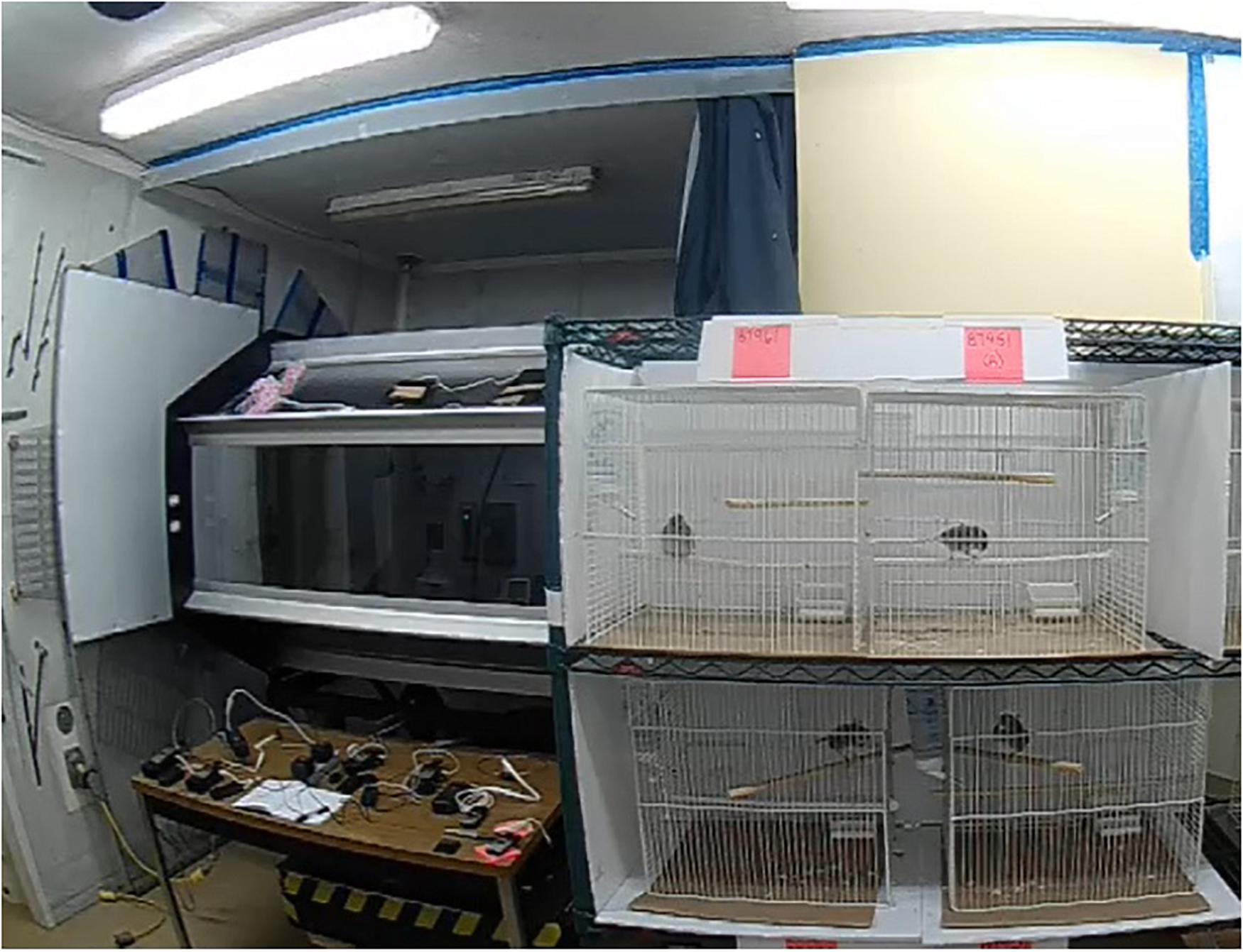
Figure 1. Experimental caging in the wind tunnel plenum. Sparrows were held individually in cages on racks adjacent to the working (flight) section of the wind tunnel that can be observed to the left. A baffle (white panel) ensured air mixing between the plenum and working section so that temperature, humidity, and pressure in the plenum was identical to the air flow in the tunnel.
Study 1: Low-Frequency Exposure
For study 1, we caught 24 white-throated sparrows during their autumn migration in London, Ontario, Canada in October 2013. Birds were housed in individual cages (33 cm × 36 cm × 38 cm) in an environmental chamber at 11°C and provided a 50:50 mixture of ground Mazuri Small Bird Maintenance Diet (catalog number 56A6, Brentwood, MO, United States) and premium fortified budgie seed (Hagen). After being held in captivity for ∼14 weeks, birds were pseudorandomly assigned to two groups [experimental (n = 12) and control (n = 12)] counterbalanced based by wing length and plumage characteristics in an attempt to have age class and plumage morph evenly distributed between groups. All birds received the same fixed amount of 8 g of food per day. Food amounts of 8 g per day were previously verified to provide birds enough energy to maintain stable body condition. Providing a fixed diet was done so that birds had sufficient food to not be food restricted, but so that they would not perceive food availability to be unlimited.
Each experimental group was housed in a separate environmental chamber, with birds in individual cages within. All birds were held at a constant 11°C under ambient barometric pressure and kept under a winter photoperiod (∼10 h light:14 h dark) that was adjusted weekly to natural outdoor conditions. Across their wintering grounds in the eastern United States, 11°C is a realistic average temperature during the wintering months for white-throated sparrows (NOAA National Centers for Environmental Information, 2015), however, this temperature can fluctuate widely depending on storm systems passing through the region.
Study 1 Procedure
Once per week over a 9-week period beginning in January 2014, we simulated inclement winter weather cues using the hypobaric climatic wind tunnel. Experimental birds were transferred from their home environmental chamber into the wind tunnel plenum in their individual cages for a 24 h period (Figure 1). Once in the wind tunnel plenum, experimental birds experienced a decrease in temperature from 11 to 1°C, a decrease in barometric pressure from ambient to 96 kPa, and a consistent shift in air water content to maintain ∼60% relative humidity (Figure 2). Temperature decreased at an average rate of 1.9°C per hour. The average rate of decreasing air pressure varied depending on ambient pressure conditions that day. Temperature was held at 1°C for ∼14 h, following which temperature increased at ∼3°C per hour until the holding temperature of 11°C was reached. Air pressure was also increased after 14 h at an average rate of ∼1 kPa per hour until ambient pressure was reached (Figure 2), but this varied daily. Following this manipulation, birds were transferred back to their home environmental chambers and remained undisturbed for the remainder of the day.
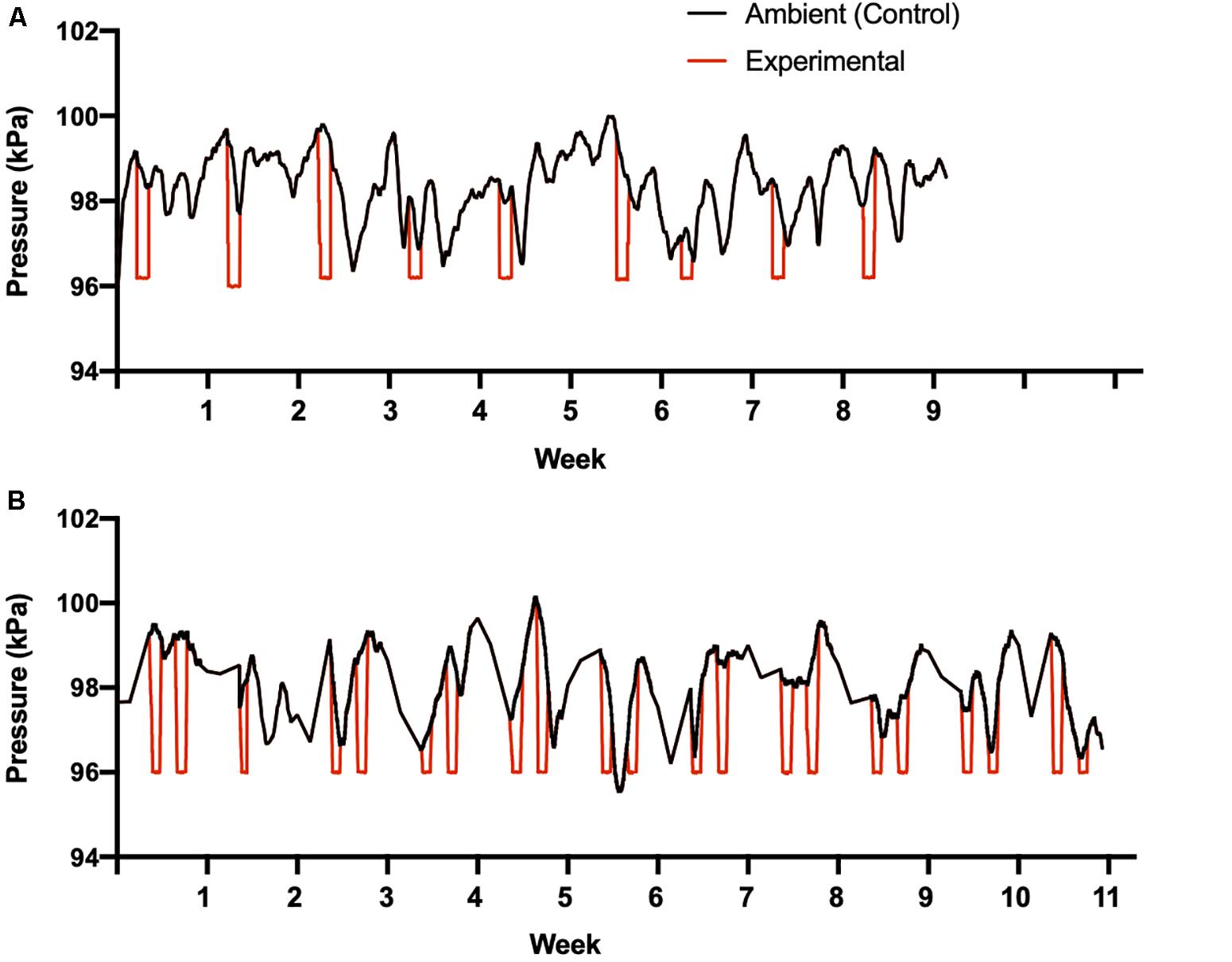
Figure 2. Pressure manipulations for study 1 (A) and study 2 (B). Black lines indicates natural changes in ambient barometric pressure during the studies, to which control birds were exposed while held at 11°C. The red lines indicates the changes in barometric pressure when experimental birds entered the wind tunnel plenum once (study 1) or twice (study 2) each week. Temperature was also decreased from 11 to 1°C for experimental birds during the times they were exposed to lowered barometric pressure.
Control birds were held in consistent environmental conditions throughout the duration of the experiment that matched the conditions experienced by experimental birds in their home environmental chamber (11°C, ambient barometric pressure, winter photoperiod). Control birds were also transferred to the hypobaric wind tunnel plenum once per week for a 24 h period over nine weeks, but they were held at the control temperature of 11°C and under ambient barometric pressure. After 24 h, birds were brought back to their environmental chambers and left alone for the remainder of the day.
Study 2: High-Frequency Exposure
For study 2 we caught 24 white-throated sparrows during their autumn migration in London, Ontario, Canada and near Long Point, Ontario, Canada in October 2015. All birds were brought to the Advanced Facility for Avian Research and housed in individual cages (33 cm × 36 cm × 38 cm) at 11°C under ambient barometric pressure and kept on a winter photoperiod (∼10 h light: 14 h dark) that was adjusted weekly to natural outdoor conditions. Birds were also pseudorandomly assigned to two groups [experimental (n = 12) and control (n = 12)], with half of the birds in each group receiving limited or unlimited food availability. The housing conditions, environmental conditions, and food availability was identical to conditions in study 1.
Study 2 Procedure
Over a 12-week period beginning in January 2016, inclement winter weather cues were simulated twice per week for 24 h within the hypobaric climatic wind tunnel, increasing both the frequency of manipulations per week and the overall duration of the study compared to study 1. Wind tunnel manipulations occurred over 11 weeks and the final week involved only data collection (see below; Figure 2). Due to an equipment failure only a single pressure manipulation occurred during the second week of this study (Figure 2). Identical methodologies and manipulation values were used between study 1 and the present study, with the exception of the number of times birds entered the wind tunnel plenum per week (i.e., once vs. twice per week) and an additional food treatment, with birds in both groups receiving either limited food access (8 g per day, as in study 1) or unlimited food access. As in study 1, control birds were transferred into the wind tunnel the same number of times but were not exposed to storm cues.
Body Composition Analysis
For both studies 1 and 2 we used Quantitative Magnetic Resonance (QMR) scans to non-invasively obtain body composition data, including fat mass and lean mass content from each bird (Guglielmo et al., 2011) every second week (fortnight). QMR scans occurred 5–6 days (study 1) or 2–3 days (study 2) after a wind tunnel exposure. Before each scan, we weighed each bird with a spring scale to measure total body mass. Once each scan was completed, birds were immediately returned to their individual cages and left them undisturbed for the remainder of the day. We scanned half the birds on either odd weeks (i.e., week 1, 3, 5, 7, 9, 11) or even weeks (i.e., week 2, 4, 6, 8, 10, 12). Since we scanned each individual bird only once every other week, the results were condensed into fortnight (2 week period), rather than analyzing the data by week.
Corticosterone Analysis
We collected blood samples from each bird every 2 weeks, on alternating weeks when birds had not been scanned with QMR. Each blood sample was collected 5–6 days (study 1) or 2–3 days (study 2) after birds had last been exposed to new housing conditions or to environmental manipulations in the wind tunnel plenum. This timing allowed birds to recover from potential acute stress experienced from being moved in and out of the wind tunnel that may influence baseline glucocorticoid levels.
All blood samples were obtained within 3 min of entering the birds’ environmental chamber. Approximately 75 μL of blood was taken from the alar vein using a 26-gauge needle and collected in heparinized microhematocrit tubes. Samples were immediately placed on ice and centrifuged within 30 min of collection. Plasma was separated from red blood cells by a microhematocrit centrifuge at 13,000 g for 11 min. Plasma was stored at −30°C until assays were run. Corticosterone levels were quantified using an enzymeimmunoassay (EIA) kit that has been previously validated in sparrows (Wada et al., 2007). We used Enzo kit ADI-901-097 and followed the manufacturer’s instructions, except that plasma was treated with 1% steroid displacement buffer and was diluted 1:40 with assay buffer prior to the assay. For study 1 the intra-assay coefficient of variation was 8.6% for a low control and 3.3% for a high control. Any value outside of the standard curve (n = 4) was set to the corrected sensitivity (1.37 ng/ml). For study 2 the intra-assay coefficient of variation was 9.1% for a low control and 3.2% for a high control.
Behavioral Analysis
Video cameras (Supercircuits, model PC182XS) were placed in the wind tunnel plenum to record feeding behavior (feeding duration as time spent at the food cup) and overall movement (total distance moved, time spent moving). Behavior was recorded during the time birds were housed in the plenum, but not when birds were housed in their environmental chambers. We recorded birds weeks 5 through 9 for study 1 and weeks 3 through 12 for study 2. Recording occurred for the first 5–6 h when birds were in the wind tunnel. To extract behavioral data, we used live tracking with Noldus EthoVision XT software using center-point detection settings to track each individual with static subtraction. The software analyzed total distance moved (cm) and cumulative duration of movement (s). The start velocity of movement was set to 3.01 cm/s which was independently verified for this project. We also analyzed cumulative duration spent in zone (s), and latency to first approach to zone (s), with the zone representing each individual food cup.
DNA Extraction and Genetic Sexing
We obtained a blood sample from each bird to genetically determine sex. Blood was smeared on filter paper and left to dry. Filter paper was individually stored in plastic card holders and frozen until further use. DNA was extracted using an ammonium-based protocol to salt out proteins, modified from Griffiths et al. (1998). The DNA concentration was quantified using a NanoDrop 2000 Spectrophotometer (Thermo Fisher Scientific) and diluted with 1 × TE to make a usable stock of 60 and 30 ng/ml for subsequent polymerase chain reactions (PCRs). In birds, females are the heterogametic sex (ZW) and males are the homogametic sex (ZZ). Detection of the W sex chromosome will determine the sex of the individual, thus the DNA sexing technique among birds targets the chromobox-helicase-DNA binding gene (CHD-W). We used P2 and P8 primers to amplify portions of the CHD-W and CHD-Z genes. The PCR reaction mixture consisted of 3 μl of genomic DNA, 1x PCR buffer, 2 mM MgCl2, 0.2 mM dNTP, 0.2 μM P2 and P8 primers, and 1 unit of Taq DNA polymerase. PCR thermal cycle conditions were 94°C for 1 min (initial denaturing), 40 cycles of 94°C for 30 s, 48°C for 45 s, and 72°C for 45 s, and 72°C for 5 min (final extension). PCR products were separated on a 3% agarose gel pre-stained with Sybersafe (S33102 Thermo Fisher Scientific) in 1x TAE buffer. The final gel electrophoresis showed two bands for females and one band for males.
Food Consumption
Food consumption was measured in study 2 only. When the birds entered the wind tunnel plenum, we weighed each food cup for each bird to the nearest 0.1 g. After the birds were inside the wind tunnel for 24 h, we immediately weighed each food cup to determine the amount of food ingested. We assumed spillage of seed between each food cup would be small because the food cups had steep walls, and we observed very little food on the cage bottoms.
Statistical Analysis
Data were analyzed using linear mixed models in SPSS (IBM, Version 25.0). Fortnight (2 week period), experimental group, and their interactions were entered as fixed effects. To control for repeated measures, bird ID was entered as a random effect. Sex was omitted as only 4 individuals were female in the entire sample study (2 females in each study). Because the two studies were conducted a year apart, we analyzed their data separately.
Results
Body Composition
There were different body composition responses between studies 1 and 2. In study 1, birds exposed to storm cues had higher fat mass content (Figure 3A and Table 1), lean mass content (Figure 4A and Table 2) and overall body mass (Table 3) compared to control birds.
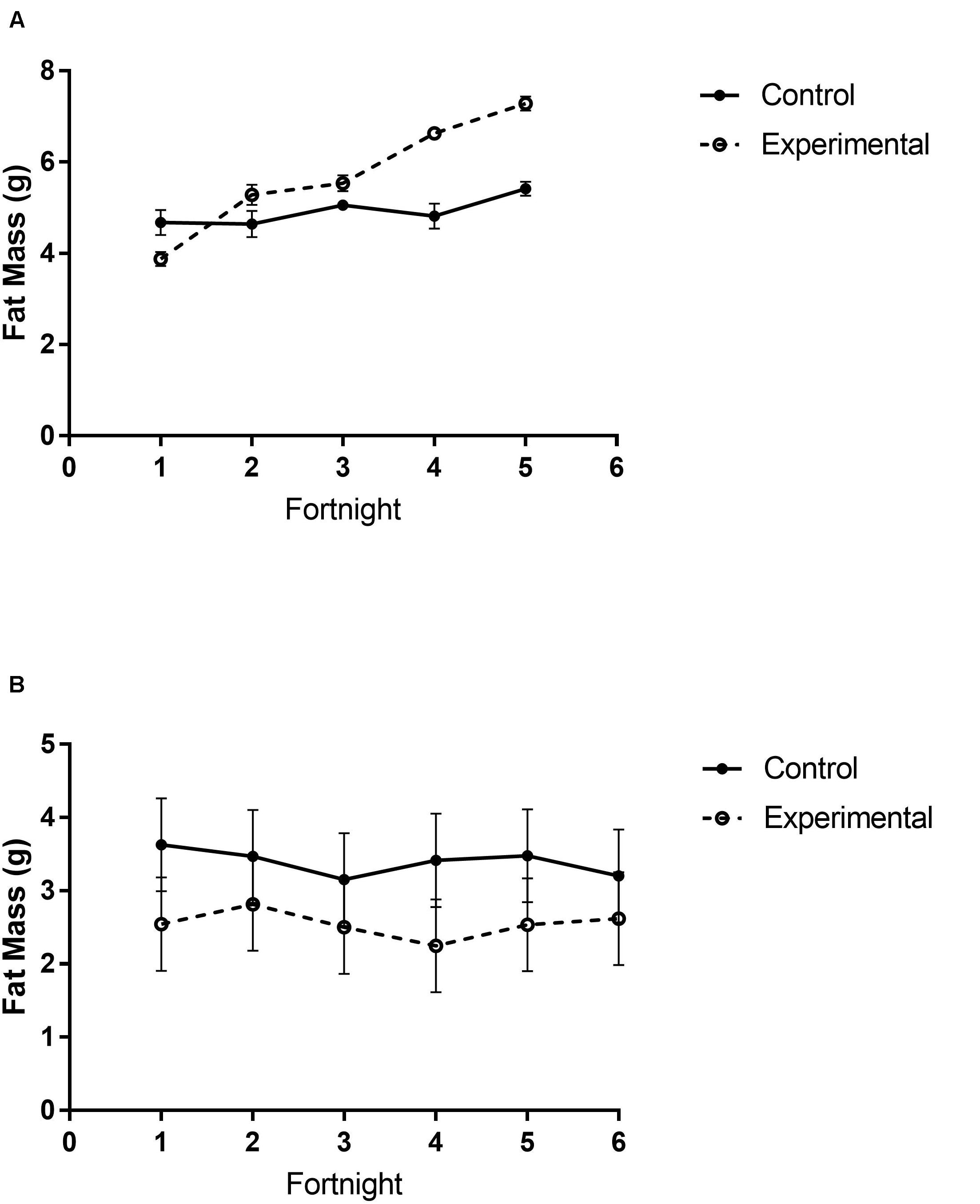
Figure 3. Fat mass content of white-throated sparrows exposed to (A) low-frequency inclement weather conditions (study 1) or (B) high-frequency inclement weather conditions (study 2). (A) Experimental birds had higher fat mass content. (B) Control birds had higher fat mass content. Points indicate mean total mass values and error bars indicate SEM.
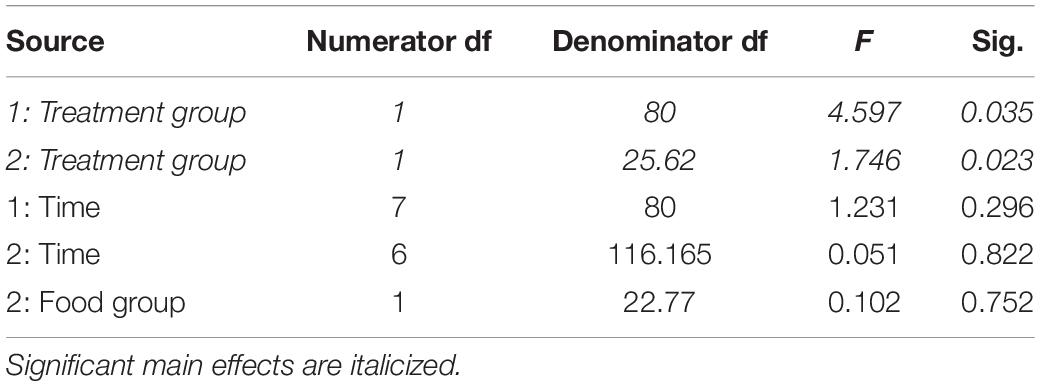
Table 1. Fixed effects from the linear mixed effects model of fat mass in study 1 (indicated by 1) and study 2 (2) between treatment groups, food groups and across time.
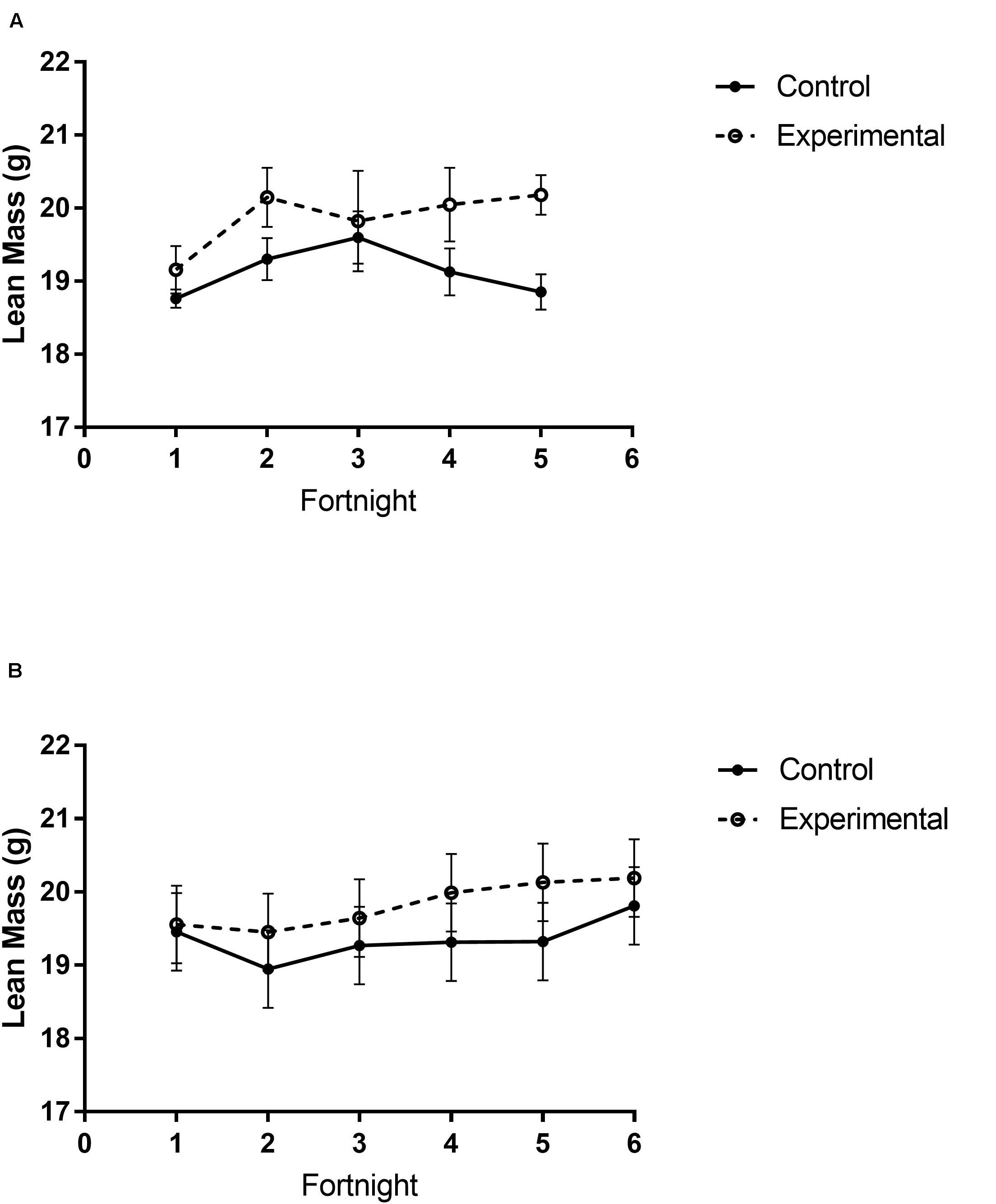
Figure 4. Lean mass content of white-throated sparrows exposed to (A) low-frequency inclement weather conditions (study 1) or (B) high-frequency inclement weather conditions (study 2). (A) Experimental birds had higher lean mass content. (B) There were no differences in lean mass between groups. Points indicate mean total mass values and error bars indicate SEM.
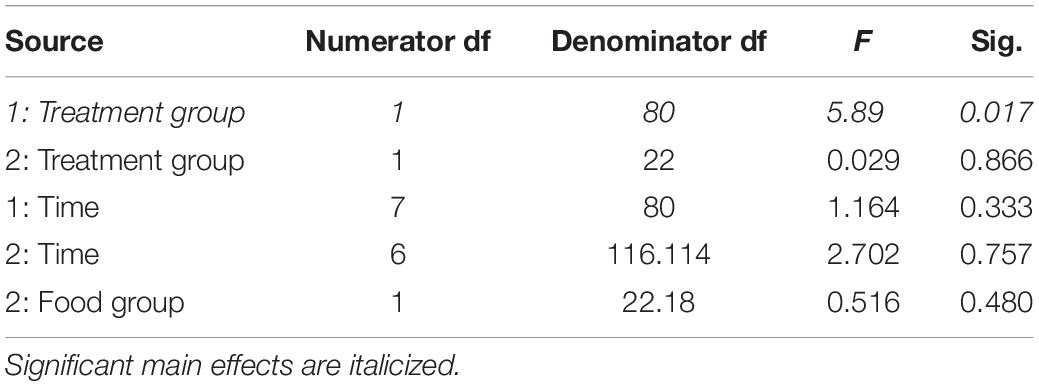
Table 2. Fixed effects from the linear mixed effects model of lean mass in study 1 (indicated by 1) and study 2 (2) between treatment groups, food groups and across time.
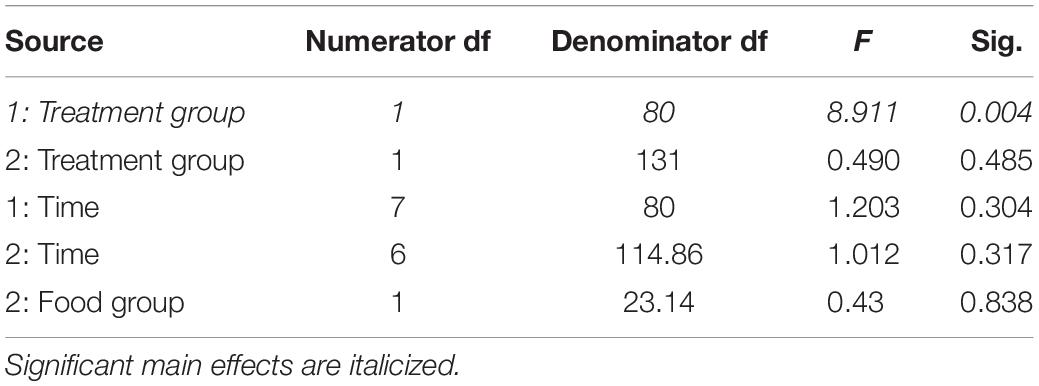
Table 3. Fixed effects from the linear mixed effects model of total body mass in study 1 (indicated by 1) and study 2 (2) between treatment groups, food groups and across time.
In study 2, birds exposed to storm cues twice per week had lower fat mass content (Figure 3B and Table 1) compared to control, but we detected no difference in lean mass content (Figure 4B and Table 2) or overall body mass (Table 3). We did not detect any difference in fat, lean or overall body mass between fixed and unlimited food groups (see Tables 1–3). In study 2, experimental birds consumed more food than control birds (Figure 5 and Table 4) and increased food consumption across time (Figure 5 and Table 4). Food consumption was mot measured in study 1.
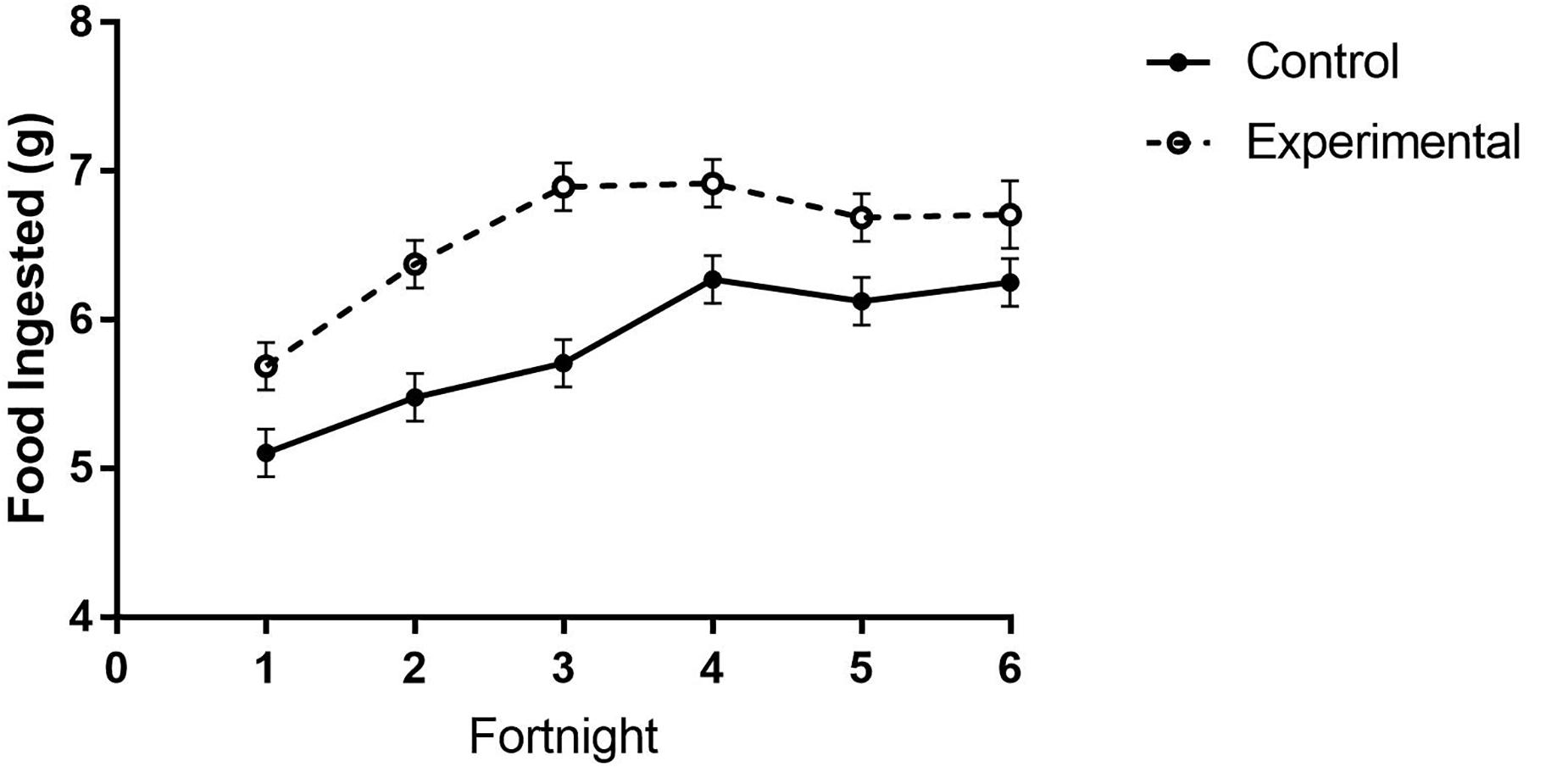
Figure 5. Food consumption of white-throated sparrows in study 2 exposed to high-frequency inclement weather cues or control conditions. Birds that were repeatedly exposed to simulated high-frequency storm cues ingested more food than control birds. Points indicate mean food amounts ingested (g) and error bars indicate SEM.
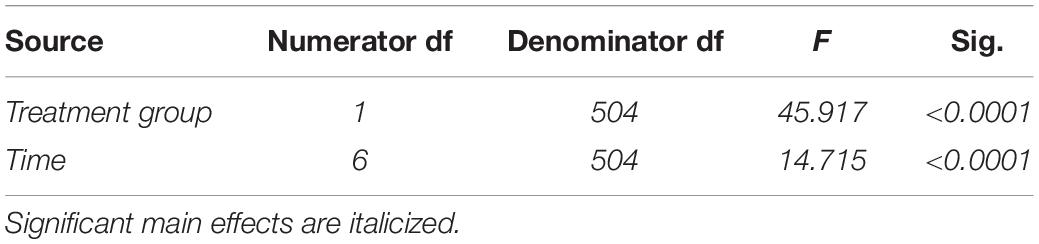
Table 4. Fixed effects from the linear mixed effects model of food ingested between experimental groups and across time for study 2.
Corticosterone
In study 1, baseline corticosterone levels significantly decreased across time (Figure 6A and Table 5), however, no differences in baseline levels were observed between treatment groups (Figure 6B and Table 5).
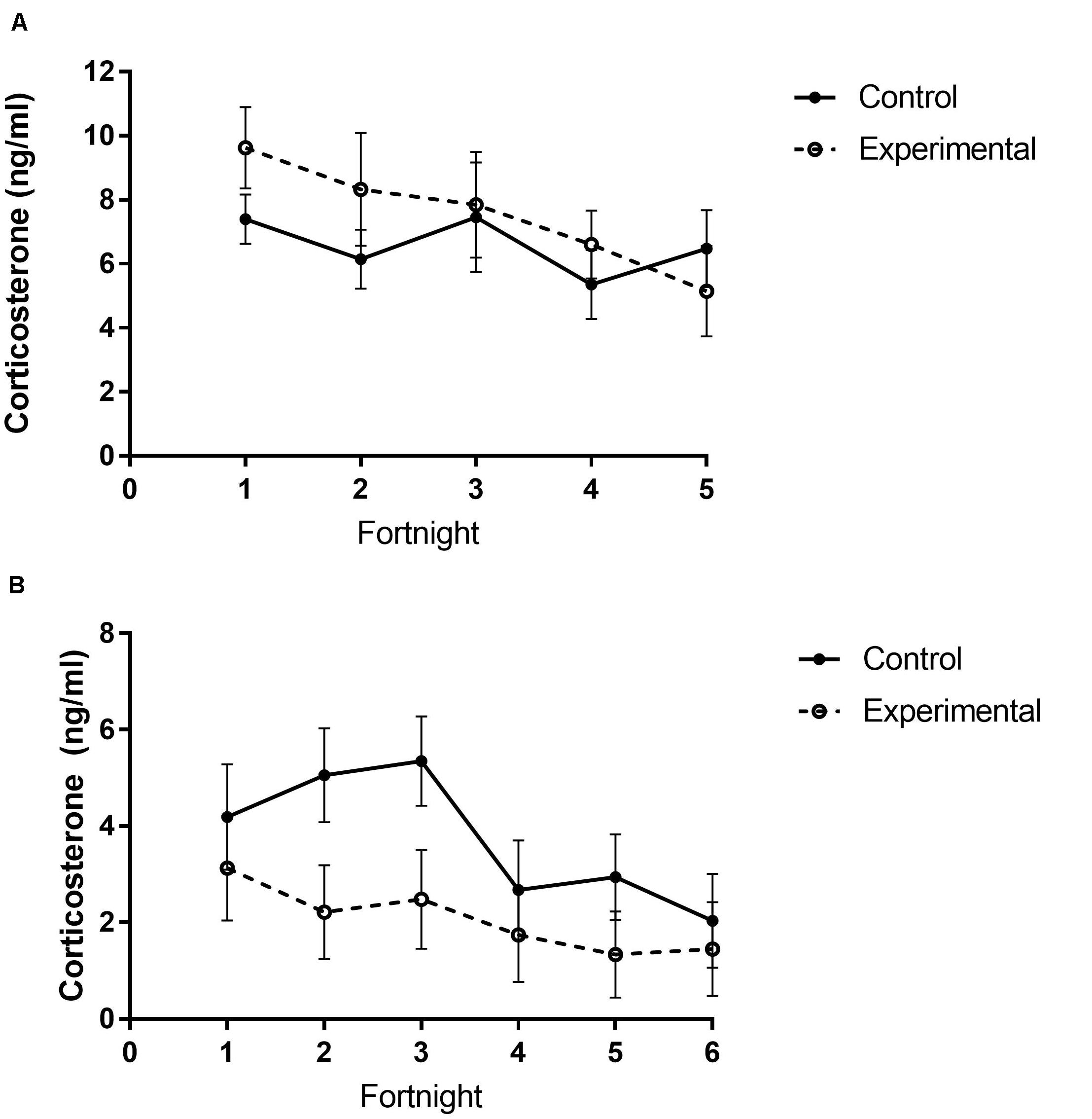
Figure 6. Total plasma corticosterone of white-throated sparrows exposed to (A) low-frequency inclement weather cues (study 1) or (B) high-frequency inclement weather cues (study 2) compared to control conditions. (A) There were no differences in plasma corticosterone levels between treatment groups, but levels did significantly decrease across time. (B) Control birds had higher corticosterone levels and decreased levels across time. Points indicate mean corticosterone levels (ng/ml) and error bars indicate SEM.
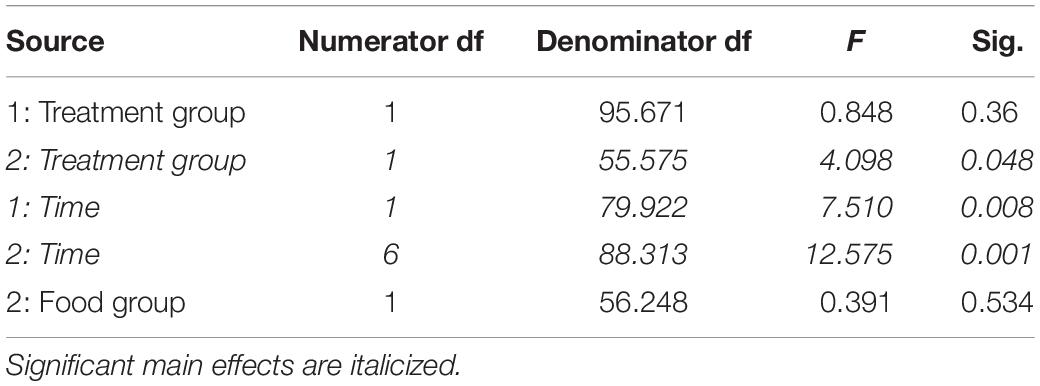
Table 5. Fixed effects from the linear mixed effects model of baseline corticosterone levels in study 1 (indicated by 1) and study 2 (2) between treatment groups, food groups and across time.
Contrary to predictions in study 2, experimental birds had lower baseline corticosterone levels than control birds (Figure 6B and Table 5). Corticosterone levels also decreased across time (Figure 6B and Table 5), but there were no significant differences between food groups (Table 5).
Feeding Duration
In study 1, the time spent at the food cup was not affected by time or treatment (Figure 7A and Table 6).
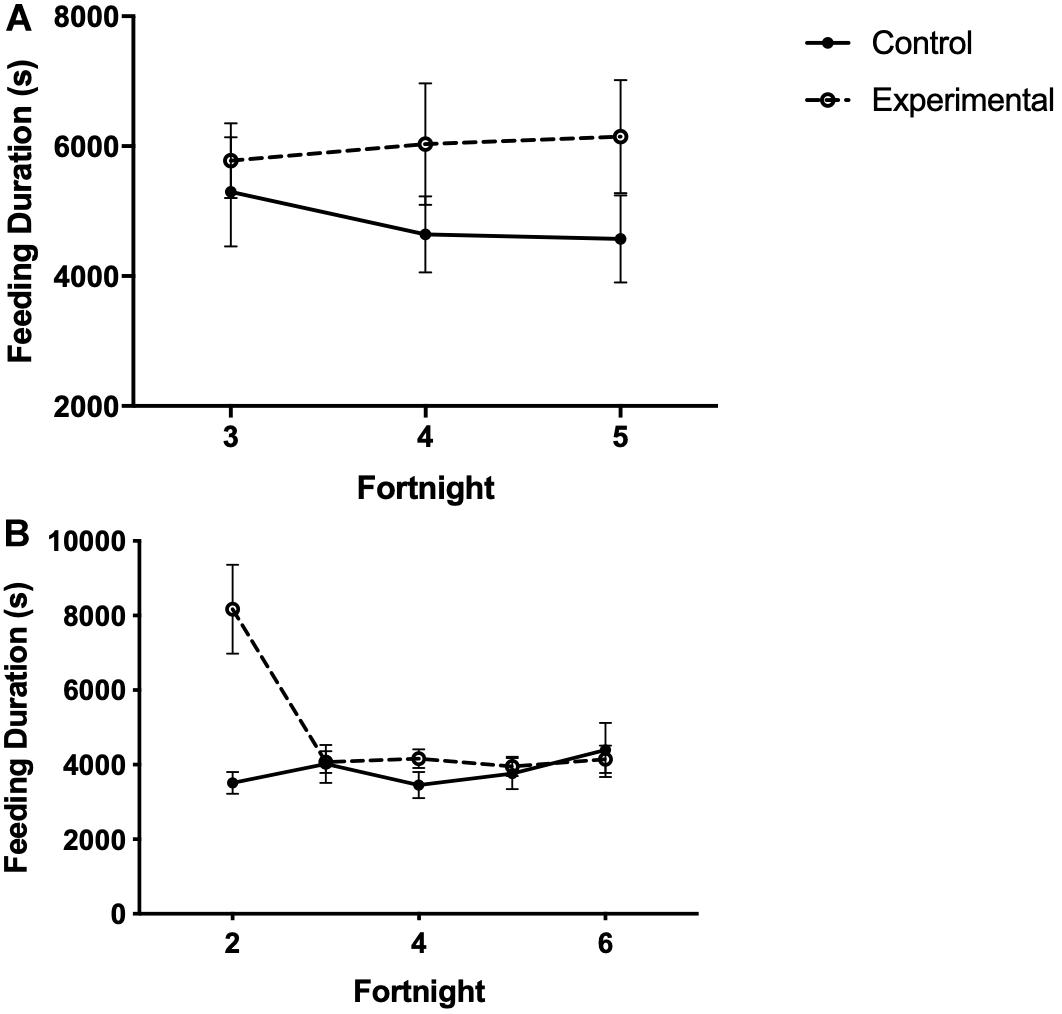
Figure 7. Feeding duration (time spent at the food cup) in seconds of white-throated sparrows exposed to (A) low-frequency inclement weather conditions (study 1) or (B) high-frequency inclement weather conditions (study 2). Points indicate total time spent feeding (s) and error bars indicate SEM.
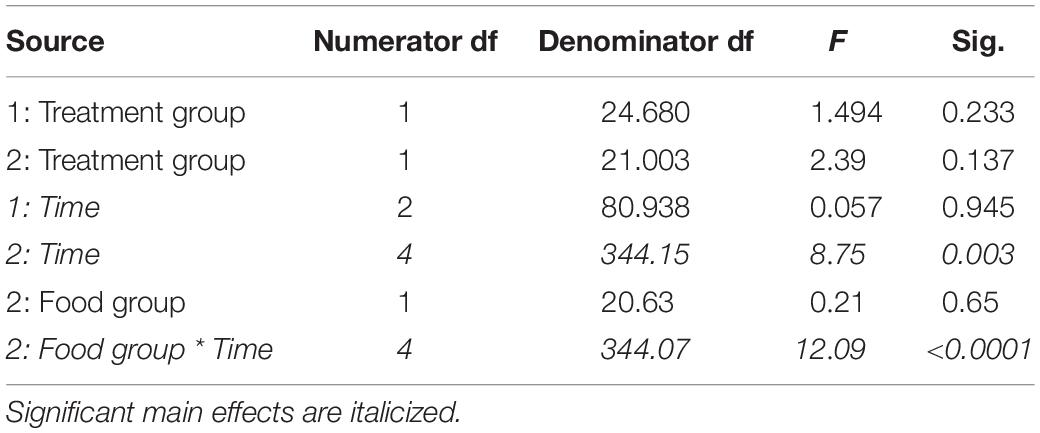
Table 6. Fixed effects from the linear mixed effects model of time spent at the food cup in study 1 (indicated by 1) and study 2 (2) between treatment groups, food groups and across time.
In study 2, there was no difference in the total time spent at the food cup between birds receiving limited or unlimited food access (Table 6). Experimental birds spent more time feeding than control birds in the first 2 weeks of the study, then quickly reduced feeding duration to levels similar to those of control birds (Figure 7B and Table 6).
Total Movement
In study 1 for fortnights 3 through 5, there were no significant effects on total distance moved across time (Table 7) or between treatment groups (Table 7). Thus, total distance moved was not affected by changes in barometric pressure or temperature.
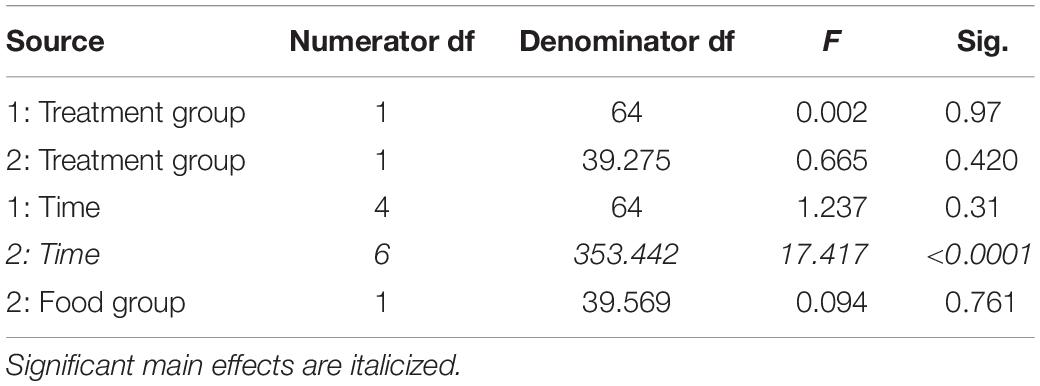
Table 7. Fixed effects from the linear mixed effects model of total distance moved in study 1 (indicated by 1) and study 2 (2) between treatment groups, food groups, across time and their interactions.
In study 2, there were no differences between food group (Table 7) or treatment group (Table 7) in the distance moved, but the distance moved did increase across time (Table 7).
Discussion
We analyzed physiological and behavioral responses, including corticosterone levels, body composition, feeding and overall behavior in white-throated sparrows exposed, or not exposed, to recurrent inclement winter weather cues once per week (study 1) or twice per week (study 2) and found noticeable differences between treatments. Birds that were repeatedly exposed to storm cues responded both physiologically and behaviorally, however, most responses did not match predictions.
In study 1, simulated winter weather cues once per week caused an increase in overall mass, lean mass, and fat mass. However, baseline corticosterone levels, the time spent feeding and overall movement while in the wind tunnel were not significantly different between groups. The cues associated with one simulated storm system per week were enough to elicit a physiological change in body composition, however, these cues did not appear to act cumulatively as a chronic stressor to induce a change in baseline corticosterone levels or behavior.
In contrast, in study 2 we found that simulated winter weather cues twice per week caused lower baseline corticosterone levels and lower fat mass. There was no difference in lean mass or feeding duration between groups, however, experimental birds did ingest more food despite no detectable change in feeding duration. Birds thus increased their ingestion rate per time foraging (time at the food cup), rather than extending foraging time. These studies provide further evidence that birds can respond to changes in temperature and barometric pressure, but the frequency of simulated storm exposure can specifically influence how birds will respond.
Food Ingestion and Body Composition
Increasing fat stores is a common wintertime response to the onset of inclement winter weather to prepare against unpredictable future conditions and disruptions in resource availability (Carey and Dawson, 1999). We predicted that birds exposed to simulated storm cues should increase fat as an anticipatory response. Results from study 1 were consistent with this prediction, but in study 2 body fat was reduced in response to increased storm frequency. Interestingly, despite lower levels of fat, the amount of food ingested during the storm simulation was higher in experimental study 2 birds. This suggests that although these birds were ingesting more food during simulated storm exposure, they were unable to allocate energy to creating additional fat stores. Increased energy storage is a common response to unpredictable or unfavorable conditions in birds (e.g., Kelly et al., 2002; van Berkel et al., 2018). Combined, these results suggest that in response to inclement weather cues birds will eat more and store more fat, but if the energetic demands of storms are too high, fat stores may be reduced below control conditions. Unfortunately, we did not measure food consumption in study 1. Further research could titrate the points at which increased food ingestion is insufficient to support additional fat storage.
Increased thermogenic demand can cause increased lean mass to enhance thermogenesis (Carey et al., 1989) and shivering of the large flight muscles is the primary means of thermogenesis in most birds (Carey and Dawson, 1999). Through winter acclimatizing, flight muscles are often enlarged to facilitate shivering responses (Swanson, 2001). We thus predicted an increase in lean mass in birds exposed to storm cues. Similar to fat mass data, our prediction was partially met. Birds exposed to one simulated storm per week (study 1) had higher lean mass than controls, but this trend was not significant in birds exposed to two storms per week (study 2). Though lean mass appears to increase over time for experimental birds in both studies (Figure 4) this was not statistically significant.
The effects on body composition and food consumption indicate that exposure to a higher frequency of simulated storms per week may more negatively influence the birds’ ability to store energy and exceed a threshold white-throated sparrows. Below the threshold the birds stored energy in fat and muscle tissue, but beyond the threshold energy may have been immediately invested in coping (higher metabolic rate) rather than being stored. It is possible that the birds were ‘coping’ with the higher rate of storms despite the lower fat reserves, as they did not have lower body mass in general and remained healthy. However, with lower energy stores the birds would certainly be more susceptible to future energetic challenges.
A previous study found that red knots (Calidris canutus) exposed to cold conditions increased their food ingestion, accompanied with increased body mass and lean muscle content to cope with cold conditions (Vézina et al., 2006), similar to the findings of our study 1. Captive dunlins (Calidris alpina), similarly, increased body mass following high winds and lower temperatures (Kelly et al., 2002). It appears that food ingestion, fat storage, and increased lean mass to enhance shivering are adaptive responses to inclement winter weather in birds, but that this response can be overcome by increased frequency of exposure to inclement weather cues. Although the experimental birds in study 2 remained generally healthy, they were not able to increase energy reserves as did the birds in study 1. It is also possible that our birds in study 2 switched coping strategy from energy storage to energy conservation through hypometabolism or hypothermia (McKechnie and Lovegrove, 2002). Such hypothermic responses can be modulated by food predictability (Nilsson et al., 2020). Because we did not measure metabolic rates in our study we cannot disentangle the potential mechanisms by which the birds in study 2 coped with the experimental storm cues. Regardless, because they did not increase energy stores as did the birds in study 1, they would likely be less capable of meeting future energy demands or stressors.
Corticosterone
We predicted that repeated storm cue exposure may act cumulatively to create a chronic stressor that would result in elevated baseline corticosterone levels. This prediction was not met. In both studies, corticosterone levels decreased over time, potentially indicating that birds were habituating to housing conditions throughout the study. In study 1 there were no detectable effects on corticosterone, replicating earlier studies showing that songbirds can respond to storm cues without modulating glucocorticoid levels (Breuner et al., 2013; de Bruijn et al., 2017). In contrast, in study 2 we found lower corticosterone levels in birds exposed to inclement winter weather cues twice per week. Birds exposed to two simulated storms per week may lower corticosterone levels to conserve energy.
This may represent a downregulation of the HPA axis under chronic stress conditions (de Bruijn et al., 2017). Indeed, the relationship between environmental stressors and baseline corticosterone levels is not as established as some studies suggest. HPA function, including the directional changes of glucocorticoid concentration, can differ widely across species exposed to repeated stressors and chronic stress (Dickens and Romero, 2013). A response in HPA function itself is more informative than the actual direction of that change (e.g., increasing or decreasing glucocorticoid levels; Dickens and Romero, 2013). Further work, perhaps using ACTH and dexamethasone challenges to characterize HPA function, would be required to better determine how birds are altering corticosterone regulation in response to twice weekly storm cues.
Behavioral Response
In the winter, a low pressure cold front can bring precipitation in the form of snow through most of white-throated sparrows’ wintering range. Since these birds are ground feeding species, they need to forage prior to the onset of the storm as food may become less available once the ground is covered in snow. Previous studies that experimentally decreased barometric pressure found that birds decreased their latency to feed and increased the feeding amount (Breuner et al., 2013; Metcalfe et al., 2013). We therefore predicted that foraging time should increase in birds exposed to simulated inclement weather.
We found no group differences in overall activity (as measured by total movement). However, in study 2 birds exposed to storm cues twice per week spent more time at their food cup during the first 2 weeks of the study, regardless of whether they received a fixed amount of food per day or unlimited food. This was reflected in the total mass of food consumed during the manipulation (see above; Figure 5).
Increased levels of corticosterone can lead to an increased rate of foraging (Breuner and Hahn, 2003), however, this direct relationship was not observed in this study. Experimental birds showed lower corticosterone levels but did increase their feeding duration and food ingestion, indicating that there was still a response as a result of repeated storm cues, but these repeated storm cue exposures did not act as a stressor to induce an HPA response. Interestingly, a limited or unlimited diet did not influence any behavioral or physiological responses. Captive white-crowned sparrows showed little activity around their food cups when food was unlimited, but activity at the food cup increased once food was removed (Astheimer et al., 1992). Similarly, European starlings (Sturnus vulgaris) increased foraging time when food was experimentally made unpredictable (van Berkel et al., 2018). Whether birds in study 2 had unlimited or limited food amounts, they were still consistently exposed to food, therefore there may not have been a strong response to immediately forage once environmental changes were detected due to the predictability of resources. Study 2 birds exposed to storm cues ingested more food and spent more time feeding but had lower fat mass content and corticosterone levels. This indicates that the rate of feeding may have been higher and more intense when birds were at the food cups. Although feeding duration and ingestion increased in experimental birds, we did not detect more fat mass in these individuals, indicating the frequency of storm cues occurring may have affected how food was stored and metabolized after ingestion. The increase in food consumption may be a result of immediate thermogenesis costs. Wintering songbirds can rapidly increase summit metabolic rates during exposure to chronic cold conditions (Stager et al., 2020). The sparrows in study 2 may have similarly been using energy to increase metabolic rates rather than storing energy as fat.
Conclusion
The responses of birds exposed to simulated storms twice per week for 12 weeks compared to once per week for 9 weeks were remarkably different. These results suggest birds can detect changes in temperature and barometric pressure and can adjust their response accordingly, however, there may be a threshold of storm frequency to which birds are able to cope, both metabolically and physiologically. Our findings suggest that increasing the frequency of storm exposure from once per week to twice per week may exceed a threshold for these songbirds. On one side of the threshold birds respond to storm cues by storing energy, and on the other they must immediately expend energy to maintain homeostasis such that they are unable to invest in energy storage, likely putting them at risk to future stressors.
Data Availability Statement
Data are available at Data Dryad https://doi.org/10.5061/dryad.69p8cz8zk.
Ethics Statement
All procedures were approved by the University of Western Ontario’s Animal Care Committee and followed the guidelines of the Canadian Council for Animal Care.
Author Contributions
AB conceived the idea, conducted the experiments, and wrote the manuscript. SM-S substantially contributed to the experimental design and edited the manuscript. All authors contributed to the article and approved the submitted version.
Funding
This research was funded by the NSERC Canada and an Animal Behavior Society student research grant.
Conflict of Interest
The authors declare that the research was conducted in the absence of any commercial or financial relationships that could be construed as a potential conflict of interest.
Acknowledgments
We thank A. Gould and F. Boon for technical support with wind tunnel operations; M. Rebuli, K. Erhard, and B. Azad for animal care support; K. Munoz for technical support with video analysis software; T. Farrell, T. Kelly, S. Mischler, and B. Azad for assistance with sampling; and the volunteers and staff at the Long Point Bird Observatory for assistance with capturing birds.
References
Ahrens, C. D. (2012). Meteorology Today: An Introduction to Weather, Climate and the Environment, 10th Edn. Belmont, CA: Brooks Cole.
Astheimer, L. B., Buttemer, W. A., and Wingfield, J. C. (1992). Interactions of corticosterone with feeding, activity and metabolism in passerine birds. Ornis Scand. 23, 355–365.
Boyle, W. A., Norris, D. R., and Guglielmo, C. G. (2010). Storms drive altitudinal migration in a tropical bird. Proc. Roy. Soc. B 277, 2511–2519. doi: 10.1098/rspb.2010.0344
Breuner, C. W., and Hahn, T. P. (2003). Integrating stress physiology, environmental change, and behavior in free-living sparrows. Horm. Behav. 43, 115–123. doi: 10.1016/S0018-506X(02)00020-X
Breuner, C. W., Sprague, R. S., Patterson, S. H., and Woods, H. A. (2013). Environment, behavior and physiology: do birds use barometric pressure to predict storms? J. Exp. Biol. 216, 1982–1990. doi: 10.1242/jeb.081067
Busch, D. S., Sperry, T. S., Wingfield, J. C., and Boyd, E. H. (2008). Effects of repeated, short-term, corticosterone administration on the hypothalamo-pituitary-adrenal axis of the white-crowned sparrow (Zonotrichia leucophrys gambelii). Gen. Comp. Endocrinol. 158, 211–223. doi: 10.1016/j.ygcen.2008.06.004
Carey, C., and Dawson, W. R. (1999). “A search for environmental cues used by birds in survival of cold winters,” in Current Ornithology, 15th Edn, eds V. Nolan, E. D. Ketterson, and C. F. Thompson (New York, NY: Plenum Publishers.), 1–31. doi: 10.1007/978-1-4757-4901-4_1
Carey, C., Marsh, R. L., Bekoff, A., Johnston, R. M., and Olin, M. (1989). “Enzyme activities in muscles of seasonally acclimatized house finches,” in Physiology of Cold Adaptations in Birds, eds C. Bech and R. E. Reinertsen (New York, NY: Plenum).
Charmantier, A., and Gienapp, P. (2014). Climate change and timing of avian breeding and migration: evolutionary versus plastic changes. Evol. App. 7, 15–28. doi: 10.1111/eva.12126
de Bruijn, R., Reed, J. M., and Romero, L. M. (2017). Chronic repeated exposure to weather-related stimuli elicits few symptoms of chronic stress in captive molting and non-molting European starlings (Sturnus vulgaris). J. Exp. Zool. A 327, 493–503. doi: 10.1002/jez.2134
Dickens, M. J., and Romero, L. M. (2013). A consensus endocrine profile for chronically stressed wild animals does not exist. Gen. Comp. Endocrinol. 191, 177–189. doi: 10.1016/j.ygcen.2013.06.014
Falls, J. B., and Kopachena, J. G. (1994). “White-throated Sparrow,” in The Birds of North America, eds A. Poole and F. Gill (Washington, DC: American Ornithological Society), 1–30.
Francis, J. A., and Vavrus, S. J. (2012). Evidence linking Arctic amplification to extreme weather in mid-latitudes. Geophys. Res. Lett. 39:L06801.
Freeman, B. G., and Class Freeman, A. M. (2014). Rapid upslope shifts in New Guinean birds illustrate strong distributional responses of tropical montane species to global warming. PNAS 20, 1–5. doi: 10.1073/pnas.1318190111
Griffiths, R., Double, M. C., Orr, K., and Dawson, R. J. G. (1998). A DNA test to sex most birds. Mol. Ecol. 7, 1071–1075. doi: 10.1046/j.1365-294x.1998.00389.x
Guglielmo, C. G., McGuire, L. P., Gerson, A. R., and Seewagen, C. L. (2011). Simple, rapid, and non-invasive measurement of fat, lean, and total water masses of live birds using quantitative magnetic resonance. J. Ornithol. 152:75. doi: 10.1007/s10336-011-0724-z
IPCC (2014). “Climate change 2014: synthesis report,” in Contribution of Working Groups I, II and III to the Fifth Assessment Report of the Intergovernmental Panel on Climate Change, eds Core Writing Team Pachauri, R. K., and Meyer, L. A. (Geneva: IPCC), 1–151.
James, A. R. M., and Abbott, K. C. (2014). Phenological and geographical shifts have interactive effects on migratory bird populations. Am. Nat. 183, 40–53. doi: 10.1086/674129
Kelly, J. P., Warnock, N., Page, G. W., and Weathers, W. W. (2002). Effects of weather on daily body mass regulation in wintering dunlin. J. Exp. Biol. 205, 109–120.
McKechnie, A. E., and Lovegrove, B. G. (2002). Avian facultative hypothermic responses: a review. Condor 104, 705–724. doi: 10.1093/condor/104.4.705
Metcalfe, J., Schmidt, K. L., Bezner-Kerr, W., Guglielmo, C. G., and MacDougall-Shackleton, S. A. (2013). White-throated sparrows adjust behaviour in response to manipulations of barometric pressure and temperature. Anim. Behav. 86, 1285–1290. doi: 10.1016/j.anbehav.2013.09.033
Newton, I. (2007). Weather-related mass-mortality events in migrants. Ibis 149, 453–467. doi: 10.1111/j.1474-919x.2007.00704.x
Nilsson, J. F., Nilsson, J. Å, Broggi, J., and Watson, H. (2020). Predictability of food supply modulates nocturnal hypothermia in a small passerine. Biol. Lett. 16:20200133. doi: 10.1098/rsbl.2020.0133
NOAA National Centers for Environmental Information (2015). State of the Climate: Global Climate Report for December 2015. Available online at: https://www.ncdc.noaa.gov/sotc/global/201512 (accessed June 28, 2020).
Pellegrino, A. C., Peñaflor, M. F. G. V., Nardi, C., Bezner-Kerr, W., Guglielmo, C. G., Bento, J. M. S., et al. (2013). Weather forecasting by insects: modified sexual behaviour in response to atmospheric pressure changes. PLoS One 8:e75004. doi: 10.1371/journal.pone.0075004
Reneerkens, J., Morrison, R. I. G., Ramenofsky, M., Piersma, T., and Wingfield, J. C. (2002). Baseline and stress-induced levels of corticosterone during different life cycle substages in a shorebird on the high arctic breeding grounds. Phys. Biochem. Zool. 75, 200–208. doi: 10.1086/340853
Rogers, C. M., Ramenofsky, M., Ketterson, E. D., Nolan, V., Wingfield, J. C., and Url, S. (1993). Plasma corticosterone, adrenal mass, winter weather, and season in nonbreeding populations of dark-eyed juncos (Junco hyemalis hyemalis). Auk 110, 279–285.
Romero, L. M. (2012). Using the reactive scope model to understand why stress physiology predicts survival during starvation in Galápagos marine iguanas. Gen. Comp. Endocrinol. 176, 296–299. doi: 10.1016/j.ygcen.2011.11.004
Stager, M., Senner, N. R., Tobalske, B. W., and Cheviron, Z. A. (2020). Body temperature maintenance acclimates in a winter-tenacious songbird. J. Exp. Biol. 6:jeb.221853. doi: 10.1242/jeb.221853
Streby, H. M., Kramer, G. R., Peterson, S. M., Lehman, J. A., and Buehler, D. A. (2015). Tornadic storm avoidance behavior in breeding songbirds. Curr. Biol. 25, 98–102. doi: 10.1016/j.cub.2014.10.079
Swanson, D. L. (2001). Are summit metabolism and thermogenic endurance correlated in winter-acclimatized passerine birds? J. Comp. Physiol. B 171, 475–481. doi: 10.1007/s003600100197
Thierry, A. M., Massemin, S., Handrich, Y., and Raclot, T. (2013). Elevated corticosterone levels and severe weather conditions decrease parental investment of incubating Adelie penguins. Horm. Behav. 63, 475–483. doi: 10.1016/j.yhbeh.2012.12.011
van Berkel, M., Bateson, M., Nettle, D., and Dunn, J. (2018). Can starlings use a reliable cue of future food deprivation to adaptively modify foraging and fat reserves? Anim. Behav. 142, 147–155. doi: 10.1016/j.anbehav.2018.06.015
Vézina, F., Jalvingh, K. M., Dekinga, A., and Piersma, T. (2006). Acclimation to different thermal conditions in a northerly wintering shorebird is driven by body mass-related changes in organ size. J. Exp. Biol. 209, 3141–3154. doi: 10.1242/jeb.02512
Vitousek, M. N., and Romero, L. M. (2013). Stress responsiveness predicts individual variation in mate selectivity. Gen. Comp. Endocrinol. 187, 32–38. doi: 10.1016/j.ygcen.2013.03.009
Wada, H., Hahn, T. P., and Breuner, C. W. (2007). Development of stress reactivity in white-crowned sparrow nestlings: total corticosterone response increases with age, while free corticosterone response remains low. Gen. Comp. Endocrinol. 150, 405–413. doi: 10.1016/j.ygcen.2006.10.002
Wingfield, J. C. (1985). Influences of weather on reproductive function in male song sparrows, Melospiza melodia. J. Zool. 205, 525–544. doi: 10.1111/j.1469-7998.1985.tb03542.x
Keywords: storms, weather, corticosterone, stress response, songbirds
Citation: Boyer AC and MacDougall-Shackleton SA (2020) High Rates of Exposure to Simulated Winter Storm Cues Negatively Affect White-Throated Sparrow (Zonotrichia albicollis) Energy Reserves. Front. Ecol. Evol. 8:222. doi: 10.3389/fevo.2020.00222
Received: 13 March 2020; Accepted: 16 June 2020;
Published: 14 July 2020.
Edited by:
Andreas Nord, Lund University, SwedenReviewed by:
David Leslie Swanson, University of South Dakota, United StatesDevin R. de Zwaan, The University of British Columbia, Canada
John C. Wingfield, University of California, Davis, United States
Copyright © 2020 Boyer and MacDougall-Shackleton. This is an open-access article distributed under the terms of the Creative Commons Attribution License (CC BY). The use, distribution or reproduction in other forums is permitted, provided the original author(s) and the copyright owner(s) are credited and that the original publication in this journal is cited, in accordance with accepted academic practice. No use, distribution or reproduction is permitted which does not comply with these terms.
*Correspondence: Andrea C. Boyer, YWJveWVyQHV3by5jYQ==