- 1State Key Laboratory for Biology of Plant Diseases and Insect Pests, Institute of Plant Protection, Chinese Academy of Agricultural Sciences, Beijing, China
- 2School of Biological Sciences, The University of Queensland, St. Lucia, QLD, Australia
- 3Institute of Applied Ecology, Fujian Agriculture and Forestry University, Fuzhou, China
- 4Chrysalis Consulting, Hanoi, Vietnam
- 5Departamento de Zoología y Antropología Física, University of Murcia, Murcia, Spain
- 6Terrestrial Ecology Group (TEG-UAM), Departamento de Ecología, Universidad Autónoma de Madrid, Madrid, Spain
- 7Centro de Investigación en Biodiversidad y Cambio Global (CIBC-UAM), Universidad Autónoma de Madrid, Madrid, Spain
- 8Facultad de Ingenieria Agronomica, Universidad del Tolima, Ibague, Colombia
- 9Plant Production and Protection Module, United Nations Food and Agriculture Organization (FAO), Bangkok, Thailand
- 10Ce3C - Centre for Ecology, Evolution and Environmental Changes, Azorean Biodiversity Group, CHANGE - Global Change and Sustainability Institute, University of the Azore, Angra do Heroísmo, Portugal
For centuries, islands and mountains have incited the interest of naturalists, evolutionary biologists and ecologists. Islands have been the cradle for biogeography and speciation theories, while mountain ranges have informed how population adaptation to thermal floors shapes the distribution of species globally. Islands of varying size and mountains’ altitudinal ranges constitute unique “natural laboratories” where one can investigate the effects of species loss or global warming on ecosystem service delivery. Although invertebrate pollination or seed dispersal processes are steadily being examined, biological control research is lagging. While observations of a wider niche breadth among insect pollinators in small (i.e., species-poor) islands or at high (i.e., colder) altitudes likely also hold for biological control agents, such remains to be examined. In this Perspective piece, we draw on published datasets to show that island size alone does not explain biological control outcomes. Instead, one needs to account for species’ functional traits, habitat heterogeneity, host community make-up, phenology, site history or even anthropogenic forces. Meanwhile, data from mountain ranges show how parasitism rates of Noctuid moths and Tephritid fruit flies exhibit species- and context-dependent shifts with altitude. Nevertheless, future empirical work in mountain settings could clarify the thermal niche space of individual natural enemy taxa and overall thermal resilience of biological control. We further discuss how global databases can be screened, while ecological theories can be tested, and simulation models defined based upon observational or manipulative assays in either system. Doing so can yield unprecedented insights into the fate of biological control in the Anthropocene and inform ways to reinforce this vital ecosystem service under global environmental change scenarios.
Introduction
Since the early 1800s, islands and mountain ranges have served as model systems for nascent evolutionary and ecological theories (Warren et al., 2015; Birks, 2019). Wallace’s and Darwin’s observations on islands in the Pacific and Indomalaya spawned revolutionary ideas on natural selection. Similarly, von Humboldt’s landmark vertical stratification maps helped to decipher the climate-based distribution patterns of biodiversity (Schrodt et al., 2019). During the second half of the twentieth century, interest was reinvigorated with the equilibrium theory of island biogeography (ETIB; MacArthur and Wilson, 1967), which led to a multitude of empirical and theoretical studies in subsequent decades. Islands, representing facts of distribution in their simplest form (Wallace, 1880), thus constitute “natural laboratories” for ecology (Santos et al., 2016b). Each island offers a uniquely replicated unit with varying species complements, as defined by its size and geographical isolation (MacArthur and Wilson, 1967). Similarly, interest in how altitudinal gradients shape species distribution patterns has recently been rekindled (Dangles et al., 2008; Peters et al., 2016; Moret et al., 2019), with work confirming the role of temperature as a core determinant in these processes (Peters et al., 2016; Steinbauer et al., 2018). Research in either setting has historically centered on richness metrics, often treating species as stand-alone entities rather than constituent components of dynamic, interlinked networks (Massol et al., 2017; Galiana et al., 2022). Only in recent years have topics such as niche theory, trophic ecology or ecosystem functioning found places within ETIB-inspired theorems or mountain ecosystem science (Allouche et al., 2012; Rasmann et al., 2014a; Warren et al., 2015; Peters et al., 2016; Massol et al., 2017; Holt et al., 2020). So far, few have attempted to relate community assemblage processes to ecosystem functioning in these settings (Warren et al., 2015).
Both consumptive and non-consumptive ecological interactions constitute the fabric of natural communities and shape ecosystem services (ES), e.g., pollination or biological control (Dainese et al., 2019; Guimarães, 2020). These interaction networks are being rewired or even eliminated through global warming or biodiversity loss (Valiente-Banuet et al., 2015; Bartley et al., 2019). As the resulting impacts on insect-mediated ES are complex, grave and likely irreversible (Decourtye et al., 2019; Lehmann et al., 2020), it is challenging to predict them through empirical work in simplified laboratory conditions or “real-world” farm settings. Islands and mountain ecosystems, however, are “systems of choice” for ES-related observational studies, experimental trials, or further simulation modeling. The isolated, simplified and poor biotas of islands make them ideal settings to investigate the consequences of species loss, and how these losses may cascade and affect ES. Meanwhile, microclimate ranges along altitudinal gradients provide the myriad of conditions required to improve our understanding of the pervasive effects of global change on biodiversity. Exploratory work has only recently started on invertebrate pollination in island and mountain settings (Traveset et al., 2016; Baumann et al., 2021), exposing a higher niche overlap within the species-poor communities of small islands and elevated altitudes (Miller-Struttmann and Galen, 2014; Traveset et al., 2016; Lara-Romero et al., 2019). Yet, no comprehensive research has been done on invertebrate biological control in either setting and only few (largely) observational studies have gauged the abundance and diversity of biological control agents (BCAs). In this Perspective piece, we show how work in these settings can pick up early warning signals of an eventual collapse of trophic regulatory processes, predict the fate of biological control under global environmental change and scientifically guide the use of BCAs against invasive or endemic pests.
Anticipating Functionalities of Species-Poor Communities
Sixty years ago, influential entomologists emphasized how islands might help to uncover “common denominators” of biological control success (De Bach, 1962). As hundreds of predators and parasitoids have been (purposely or accidentally) released in island ecosystems over the past century (Hajek et al., 2007), a methodical assessment of the resulting outcomes could raise success rates in classical biological control (Mason, 2021). It can pinpoint which taxa or guilds of BCAs are more successful—acting singly or concurrently—or which herbivores are more amenable to biological control (De Bach, 1962). Likewise, islands provide unique settings to sharpen practices such as pre-release host range testing or ecological risk assessment (Van Driesche and Hoddle, 2000; Kaser and Heimpel, 2015). Further, an in-depth quantification of biological control in island settings is crucial to confidently gauge insularity effects on herbivore pressure or plant defense evolution (Moreira and Abdala-Roberts, 2021; Moreira et al., 2021). Lastly, as per ETIB colonization-extinction rules, biological control introductions in smaller islands play out in shorter food chains with smaller subsets of species and a disproportionate presence of generalists (Gravel et al., 2011; Roslin et al., 2014; Massol et al., 2017; Galiana et al., 2018)—discomfiting impressions of a future under progressive defaunation (Borges et al., 2020). The fate of these introductions may mirror how trophic regulatory forces act in simplified agro-ecosystems or, eventually, on a less-biodiverse Planet. Recent years have seen several studies of richness, trophic breadth (i.e., generalism), and functional diversity of island parasitoid communities (Santos and Quicke, 2011; Santos et al., 2011b,2016a) and multiple observational studies of predator-prey interactions (Holt, 2009; Cirtwill and Stouffer, 2016; Holt et al., 2020). However, aside from the work in Hawaii (Funasaki et al., 1988; Messing and Wright, 2006), a systematic analysis of biological control outcomes (i.e., parasitism or predation rate, herbivore suppression or ultimate crop yield) in insular settings is long overdue.
To stimulate further thought and illuminate islands’ potential to inform biological control practice, we plot three different diversity patterns (Figure 1). First, for historic biological control introductions (insect BCA vs. insect pest) on 49 islands (Mason, 2021; Figure 1A), we detect no statistically significant relationship between the overall BCA establishment rate or the odds of biological control success and island size (Figure 1A). Hence, when lumping different BCA guilds and taxa, species richness (i.e., as set by island size) does not impact these dynamics. Second, for biological control introductions in the Mariana Islands over 1911–88 (Nafus and Schreiner, 1989) and in Easter Island over 1980–1995 (Ripa et al., 1995; Figure 1B), specialist BCAs attained slightly higher establishment rates than generalists, but these differences were not statistically significant. However, for 108 separate biological control cases, island size did not exhibit any apparent effects (Figure 1B). Third, by extracting data from GloBI,1 we document parasitoid-host linkages for six target herbivores across the above set of 49 islands of varying size (Figure 1C). Herbivores comprise both large-bodied, mobile taxa [i.e., Lymantria dispar L., Plutella xylostella (L.), Spodoptera exigua (Hübner); Lepidoptera], as well as small-sized ones [Bemisia tabaci (Gennadius), Trialeurodes vaporariorum (Westwood), Myzus persicae (Sulzer); Hemiptera, Sternorrhyncha]. While the number of parasitoids per host consistently increases with island area, regression lines tend to be steeper for small-sized hosts such as B. tabaci and T. vaporariorum (Figure 1C). For these hosts, the associated (minute) parasitoids may possess limited dispersal capability (Santos et al., 2011a) and do not engage in phoresy to the same extent as aphid parasitoids (Zhang et al., 2009). Also, for P. xylostella, anthropogenic movement of infected agricultural commodities may have facilitated parasitoid colonization especially in insular settings with high human population density or tourism. Yet, the above patterns are drastically reversed when standardizing for sampling effort (i.e., total number of island biota in the Global Biodiversity Information Facility GBIF) or for human population numbers. This could mirror how parasitoid biota are considerably under-researched in small island settings (e.g., Eisenhauer et al., 2019) or how anthropogenic forces exert a negative pressure on their island-level occurrence (Tylianakis et al., 2008; Valiente-Banuet et al., 2015). Below, we assess these patterns and their relevance for biological control first from a trophic island biogeography perspective (Holt, 2009; Massol et al., 2017; Holt et al., 2020) and subsequently more broadly.
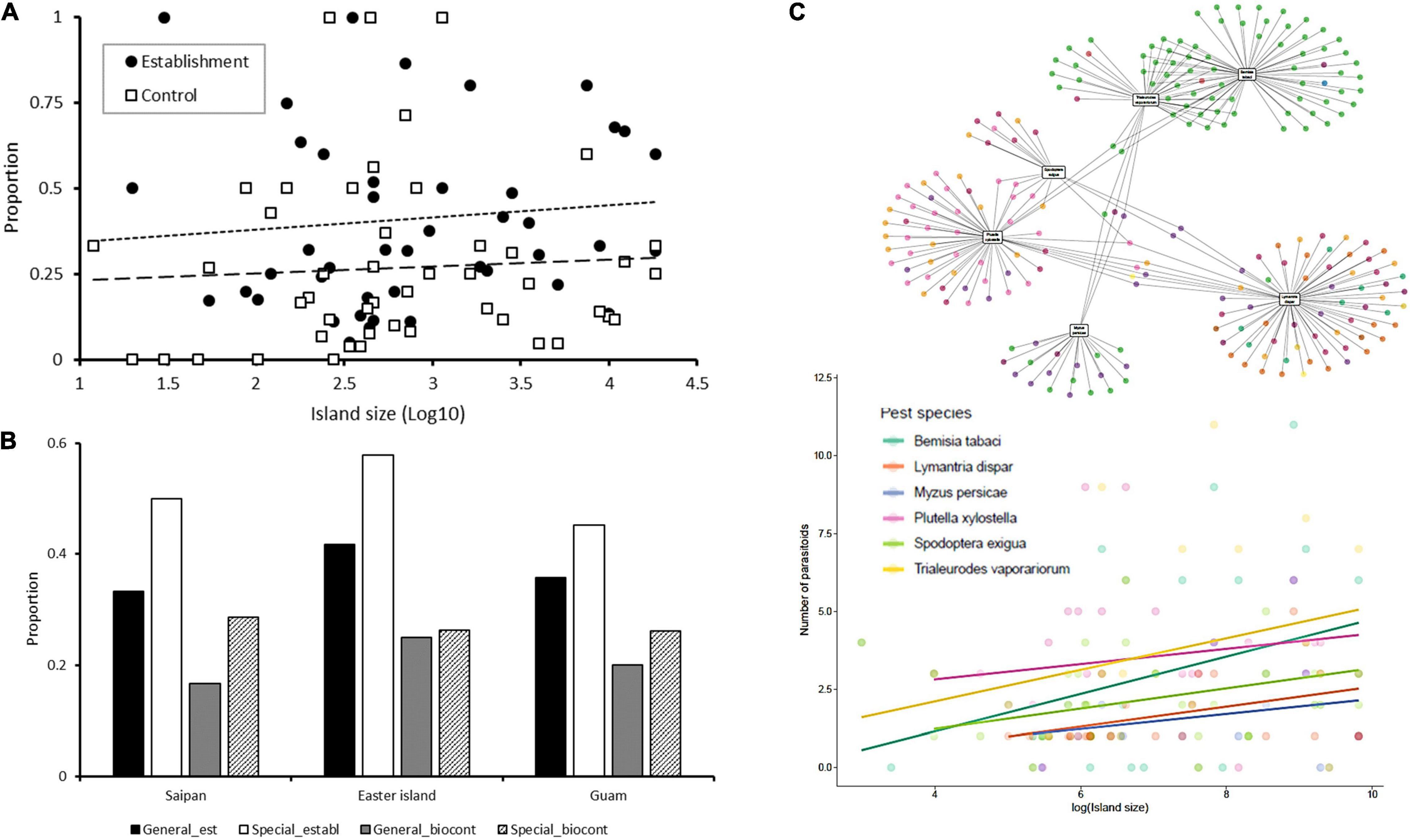
Figure 1. Natural enemy establishment rates and the ensuing biological control outcomes on islands of different sizes. (A) Coarse-grained patterns show how neither insect biological control establishment [F(1, 47) = 0.300, p = 0.587] nor success rate [F(1, 47) = 0.882, p = 0.352] are affected by island size (12–18,280 km2 range). Patterns are plotted for 49 islands harboring 14.9 ± 16.8 target insect pests and receiving 28.1 ± 34.3 insect BCAs ( ± SD). Islands and archipelagos are treated as comparable units (from Santos et al., 2010). (B) The proportion of generalist and specialist (i.e., parasitoids with less than 10 known hosts) BCAs that successfully established and provided (partial to good) control of target insect pests on Saipan (119 km2), Easter Island (164 km2), and Guam (549 km2). (C) For six target herbivores, the number of parasitoids per herbivore host varies with island size (Santos et al., 2010). Parasitoid occurrence records are extracted from the GloBI interface for each island and target herbivore, and trophic linkages are plotted. Parasitoid families are represented by differently colored dots in the food web; trendlines are shown per target herbivore: B. tabaci (p = 0.047, R2 = 0.130), L. dispar (p = 0.038, R2 = 0.174), M. persicae (p = 0.452), P. xylostella (p = 0.441), S. exigua (p = 0.036, R2 = 0.164), T. vaporariorum (p = 0.178).
Islands were long thought to be more conducive to successful biological control, with BCA establishment successes often ascribed to depauperate island fauna and empty niche space (De Bach, 1962; Hall and Ehler, 1979). However, this rationale has proven less apt for generalization than originally thought and the trophic theory of island biogeography (TTIB; the interplay of food web ecology and island biogeography; Holt, 2009) perspective allows examination of the underlying mechanisms. Establishment dynamics and the resulting ES outcomes are modulated by food web complexity (# species, # links), which increases with island area following a power law (Galiana et al., 2022). Specialists perform well in simple food webs (Montoya et al., 2003; Holt, 2009), which should provide them with an advantage in small oceanic islands. Meanwhile, TTIB predicts that generalists (i.e., species with broad niche breadth) are also more likely to occur on small islands, where they effortlessly encounter suitable (prey, host) resources and thus are more likely to persist (Gravel et al., 2011; Galiana et al., 2018). BCA incidence is thus proportional to the square of its degree, i.e., number of prey or host items (Massol et al., 2017). Nevertheless, as importation biological control aims to introduce known antagonists of a resident pest, these trophic constraints are only relevant for obligate generalists, i.e., those that require access to different prey or host items to complete specific development stages (Holt et al., 2020). Hence, introduced obligate generalists likely do well on islands of intermediate size, where the full breadth of host (or prey) items is more likely to be present and where intraspecific competition is moderate. The same may hold for life history omnivores, including those that rely upon non-prey resources such as pollen, (floral) nectar or honeydew (Lundgren, 2009). Conversely, colonization by specialists is inhibited in prey-rich communities, where interference competition or intraguild predation are more dominant. In the presence of their target prey or hosts, specialists are thus likely to perform best in small- to intermediate-sized islands (Holt, 2009; Schoener et al., 2016), as suggested in Figure 1. Hence, when disregarding constraints on colonization, specialist BCAs may attain substantially higher odds of success in small, species-poor settings.
In the above, the TTIB and specifically BCA’s dietary specialization only offers a first approximation of the drivers of biological control in species-poor settings. As a next step, we account for the functional differences of species, the heterogeneity of local habitats and niche partitioning (Kadmon and Allouche, 2007). Incipient work has shown how biotic filters—which act during the establishment phase—are core determinants of parasitoid functional diversity (Santos et al., 2016a). From a regional pool of potential colonists, only those with traits that allow exploitation of the resident (host, prey) resources thrive. These go far beyond dietary breadth or trophic position (e.g., primary or hyperparasitoid), but also cover fecundity, development mode, attack strategy, host biology, behavioral plasticity or species’ ability to consume allochthonous inputs (Polis and Hurd, 1995). Some variables are captured by fundamental ecological traits such as body mass (Massol et al., 2017; Holt et al., 2020), which in turn will define the overall (resident, introduced) BCA biomass distribution i.e., another core determinant of biological control (Ostandie et al., 2021). In addition to trait-based approaches (Perović et al., 2018), an understanding of food web topology and the composition of recipient host or prey communities is thus essential to make useful predictions on establishment potential (Gravel et al., 2011). Yet, this has been habitually overlooked during a century of biological control science (e.g., Heimpel and Asplen, 2011). When interpreting linkages between species richness, food web structure and biological control outcomes, connectance can possibly be a defining metric (Galiana et al., 2018; Yang et al., 2021). Habitat heterogeneity assumes an equally important role (e.g., providing refuges or climate niches), but its contribution is scale-dependent and moderated by reproduction rate—which acts negatively or positively for species with a respective slow or fast reproductive rate (Kadmon and Allouche, 2007). Hence, habitat heterogeneity cannot be omitted for example when interpreting differences in S. exigua vs. B. tabaci parasitoid loads or biological control efficacy (Figure 1C) and thus needs to feature in an inclusive, functional trait-based approach. Similarly, environmental heterogeneity shapes BCAs’ habitat domain (i.e., the spatial extent of habitat space used), defines its overlap with that of target host or prey items and can help us to anticipate the outcomes of predator-prey interactions (Schmitz et al., 2017). In addition, other confounding factors include release numbers, which determine demographic factors such as Allee effects or genetic bottlenecks (Grevstad, 1999), environmental heterogeneity (Barajas-Barbosa et al., 2020) and the on-site presence of a seasoned biological control practitioner (MJW Cock, personal comm.). Clearly, myriad ecological and anthropogenic processes shape biotic interactions and the resulting ecosystem services (Cabral et al., 2017) and the related phenomena play out in islands with varying species numbers. Exciting island biogeography research lays ahead to unravel those and guide the practice of biological control.
Prisms for Gauging Impacts of Global Warming
Climate change is expected to alter the strength and stability of biological control e.g., by affecting BCA fitness, geographical distributions and phenological mismatches (Furlong and Zalucki, 2017; Nechols, 2021). Mountain ecosystems provide a unique and prescient opportunity to view and examine the consequences of a warmer future, but mountain ecology science has made scant progress in gauging community assembly dynamics, defining explanatory models or assessing ecosystem functioning (Dangles et al., 2008; Peters et al., 2016; Steinbauer et al., 2018). Clearly, these patterns and the associated ES result from an intricate interplay of spatiotemporal mechanisms and species- or population-level processes e.g., metabolic constraints, niche ranges, host (plant) phenology (Cabral et al., 2017). While temperature shapes community-level richness in mountain settings (Peters et al., 2016), short-wave radiation, atmospheric pressure, precipitation and turbulence all act as confounding variables (Hodkinson, 2005). For example, for BCA taxa such as parasitoids or hoverflies, precipitation dictates diversity patterns in the dry savannah ecosystem of Mount Kilimanjaro (Peters et al., 2016). Similarly, while the thermal niche space of individual taxa may vary substantially (Kühsel and Blüthgen, 2015), little is known about the thermal resilience of biological control across geographies and ecological contexts.
Mountain settings do hold ample promise to resolve these questions, and biological control scientists have made cautious attempts to pick up meaningful patterns (Sivinski et al., 2000; Moya-Raygoza et al., 2012). So far, observational studies in mountain ecosystems have primarily considered simple (bi-trophic) interactions and failed to yield general rules of thumb (Hodkinson, 2005). Apparent declines in biological control have been ascribed to drops in foraging abilities of parasitoids and (comparative specialist) predators at cooler temperatures. This, however, over-simplifies the complexity of real-world communities: indeed, multiple direct and indirect as well as trophic and non-trophic interactions shape biotic interactions and the resulting biological control outcomes (Cabral et al., 2017; Thierry et al., 2019). Initial manipulative assays reveal how parasitoid-host food webs change considerably along elevational gradients (Maunsell et al., 2015), with important mediating effects of habitat diversity (Corcos et al., 2018). Biotic interactions (e.g., oviposition behavior, host availability, constitutive defenses, predator-free space) further mediate temperature effects on insect species along altitudinal ranges (Merrill et al., 2008; Rasmann et al., 2014b; Moreira et al., 2018). Altitudinal responses are also greatly modulated by functional traits of target pests and BCAs alike (Péré et al., 2013). In addition to thermal niche space, trophic niche breadth is mediated by altitude and local host or prey communities (Rasmann et al., 2014a); higher degrees of polyphagy have thus been recorded among high-altitude bumblebees (Miller-Struttmann and Galen, 2014). If the “altitude niche breadth hypothesis” also applies to BCAs, this will clearly impact biological control in mountain settings. To complicate matters further, many of the above patterns are plastic and adaptive over time (Le Lann et al., 2021). Hence, anticipating the above (direct, indirect) impacts is challenging and existing studies only detect few consistencies across crop × pest systems and farming contexts (Nechols, 2021). Evidently, few observations over space and time make it impossible to make wide-ranging predictions regarding the fate of biological control.
By plotting two different patterns in parasitism with altitude, we accentuate the promise but also the pitfalls of mountain ecological research for biological control (Figure 2A). First, we draw on published work (Molina-Ochoa et al., 2004) to assess whether parasitism of the fall armyworm Spodoptera frugiperda Smith varies with altitude across six Mexican states. A gradual decline in S. frugiperda parasitism with altitude corresponds with other work (Péré et al., 2013), although parasitoid richness is entirely unaffected. With increasing altitude, considerable species turnover is recorded among 13 parasitoid taxa. Individual parasitoid species exhibit clear altitudinal zonation and (associated) thermal niches, with Campoletis flavicincta Ashmead more prevalent at higher altitudes. Meanwhile, Chelonis insularis Cresson acts across altitudes and Euplectrus plathypenae Howard and Meteorus laphygmae Viereck dominate in lowlands. Aside from their different thermal responses, these species differ in attack strategy, development mode and/or dietary breadth. Also, the markedly lower S. fruiperda infestation levels at higher altitudes (Wyckhuys and O’Neil, 2006) restrain parasitoid search efficiency and carry implications for the overall relevance of biological control. A second exercise draws on published datasets of Tephritid fruit fly parasitoids from the tropical Andes i.e., multiple sites in Colombia and Peru (e.g., Ruiz-Hurtado et al., 2013; Saavedra-Díaz et al., 2017; Salazar-Mendoza et al., 2021; Figure 2B). For a complex of Tephritid hosts (dominated by Anastrepha striata Schiner) and a broad altitudinal range (300–2,264 m), parasitism increased with altitude. This contrasts with studies in other agricultural settings (Sivinski et al., 2000), over narrow elevational bands or with lower sample sizes (Salazar-Mendoza et al., 2021), where marked declines were recorded. While Sivinski et al. (2000) logged the highest parasitism levels (16%) at 600–800 m in central Veracruz (Mexico), values of 26.5% (max. 86.7%) were recorded in the Andes at 1,600–1,800. Despite eventual methodological differences and seasonal impacts between studies, it may prove rewarding to closely examine the underlying causal factors. One possible explanation is that local farming context (e.g., pesticide use intensity, landscape heterogeneity) can reverse some of the observed patterns. In more pristine landscapes (e.g., possibly in Colombia’s High Andes), parasitoid diversity can be retained and inter-habitat spillover of generalists thus bolsters (on-farm) biological control (Laliberté and Tylianakis, 2010). By accounting for the “habitat domain,” one can forecast the resulting host-parasitoid interactions and parasitoid-mediated ES (Schmitz and Barton, 2014). In the meantime, it is important to bear in mind that the above patterns solely consider (relatively host-specific) parasitoids and disregard a multitude of other BCAs e.g., generalist predators or entomopathogens. Some of these potentially outperform parasitoids under the comparatively humid, high-altitude conditions of the tropics. Hence, when observing drops in parasitism with altitude (Péré et al., 2013), one may be tempted to foresee a more effective biological control in a warmer world. Yet, especially when compounded by species loss and environmental degradation, the reality may prove to be entirely different.
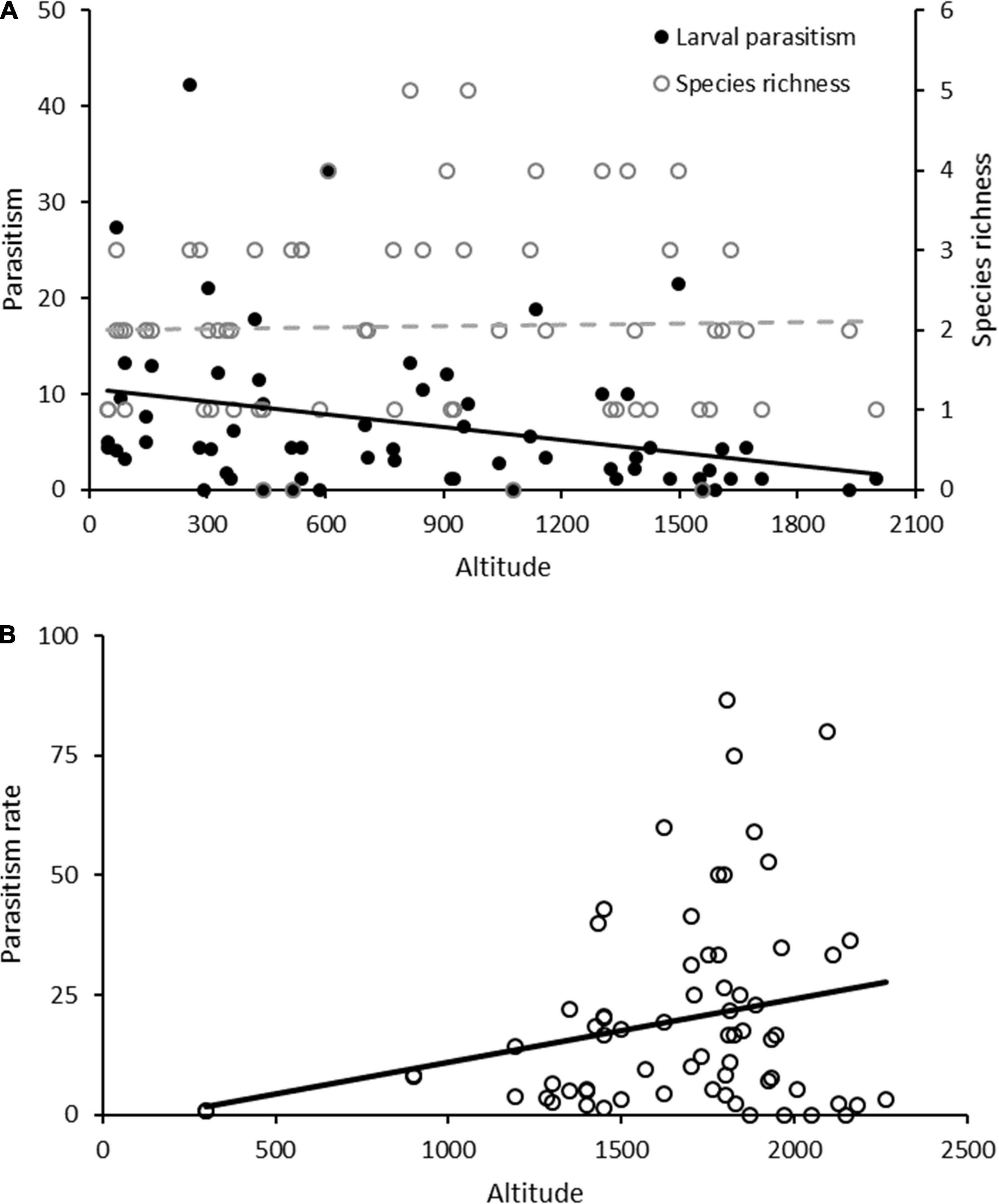
Figure 2. Parasitism rates are differentially affected by altitude, for two prime sets of agricultural pests in Neotropical mountain ranges. (A) Shows parasitism rates of the fall armyworm Spodoptera frugiperda on major cereal grains in six Mexican states, by a complex of 13 parasitoid (morpho-)species. Parasitism steadily declines with elevation [F(1, 62) = 6.231, p = 0.015, R2 = 0.091], while parasitoid richness remains unaffected [F(1, 62) = 0.047, p = 0.829]. (B) Reveals parasitism levels of a (multi-species) complex of Tephritid fruit flies in Andean mountain settings. Although overall parasitism increases with altitudinal elevation [F(1, 66) = 4.19, p = 0.045, R2 = 0.060], values appear to decline rapidly at altitudes above 2,000 m.
Moving Forward
Islands and mountain ecosystems provide complementary lenses through which scientists can examine (and anticipate) biological control outcomes under conditions of progressive biodiversity loss or global warming. In both settings, multiple roads can be pursued to relate demographic processes, (spatially defined) biotic interactions and the ultimate ecosystem service delivery (Cabral et al., 2017; Moreira et al., 2021). These include observational assays, empirical studies, e.g., manipulative trials and (mechanistic) simulation models. As a logical starting point for future work, one can examine the (2,300) island-specific records of historic biological control introductions in the BIOCAT database (Cock et al., 2016). For BCAs with varying functional traits (e.g., as deployed against common targets), conditional incidence can be examined for islands of varying size, heterogeneity or energy (Wright, 1983; Holt, 2009). Aside from yielding static, species specific attributes or inferences on specific predator x prey couplets (Massol et al., 2017; Schmitz et al., 2017), doing so can generate insights into how multiple BCAs act within dynamic communities. In the meantime, fieldwork needs to be conducted in a systematic manner -using standardized protocols—across different island and/or mountain settings (e.g., Borges et al., 2018). In insular settings, observational assays can use aggregate density (as a proxy for richness; Holt, 2009) of individual BCA taxa to reveal their TIB species-area relationships or the trophic structure of local communities as predictor of BCA establishment (Cirtwill and Stouffer, 2016). To our knowledge, BCA exclusion assays or gut content analyses, i.e., mainstays of empirical biological control science in mainland settings (Furlong, 2015)- wait to be conducted with island biota or across altitudinal ranges. These field-level data eventually can feed simulation models and thus generate much-needed insights and theories regarding the fate of biological control under progressive ecological simplification. One hereby urgently needs to look beyond richness or community assembly, and instead aim to unveil the mechanistic basis of (agro-)ecosystem functioning. Time is of the essence, because global change impacts on biological control and ecological resilience are prone to be unforgiving.
Data Availability Statement
The original contributions presented in the study are included in the article/supplementary material, further inquiries can be directed to the corresponding author/s.
Author Contributions
KW led the idea generation, writing, analytics, and editing process. FS, NC, and GP contributed to data extraction and analytics. All authors actively contributed to writing and editing.
Funding
The development of this manuscript was funded by the Food and Agriculture Organization FAO through LOA/RAP/2021/57, executed by The University of Queensland. AS was supported by the “Ramón y Cajal” program (RYC2020-029407-I), financed by the Spanish “Ministerio de Ciencia e Innovación”.
Conflict of Interest
KW is the chief executive officer of Chrysalis Consulting, a firm that provides tailored support to biological control and biodiversity-friendly agriculture.
The remaining authors declare that the research was conducted in the absence of any commercial or financial relationships that could be construed as a potential conflict of interest.
Publisher’s Note
All claims expressed in this article are solely those of the authors and do not necessarily represent those of their affiliated organizations, or those of the publisher, the editors and the reviewers. Any product that may be evaluated in this article, or claim that may be made by its manufacturer, is not guaranteed or endorsed by the publisher.
Footnotes
References
Allouche, O., Kalyuzhny, M., Moreno-Rueda, G., Pizarro, M., and Kadmon, R. (2012). Area–heterogeneity tradeoff and the diversity of ecological communities. Proc. Natl. Acad. Sci. 109, 17495–17500. doi: 10.1073/pnas.1208652109
Barajas-Barbosa, M. P., Weigelt, P., Borregaard, M. K., Keppel, G., and Kreft, H. (2020). Environmental heterogeneity dynamics drive plant diversity on oceanic islands. J. Biogeogr. 47, 2248–2260.
Bartley, T. J., McCann, K. S., Bieg, C., Cazelles, K., Granados, M., Guzzo, M. M., et al. (2019). Food web rewiring in a changing world. Nat. Ecol. Evol. 3, 345–354. doi: 10.1038/s41559-018-0772-3
Baumann, K., Keune, J., Wolters, V., and Jauker, F. (2021). Distribution and pollination services of wild bees and hoverflies along an altitudinal gradient in mountain hay meadows. Ecol. Evol. 11, 11345–11351. doi: 10.1002/ece3.7924
Birks, H. J. B. (2019). Contributions of quaternary botany to modern ecology and biogeography. Plant Ecol. Divers. 12, 189–385. doi: 10.11646/zootaxa.4878.3.2
Borges, P. A., Cardoso, P., Kreft, H., Whittaker, R. J., Fattorini, S., Emerson, B. C., et al. (2018). Global Island monitoring scheme (GIMS): a proposal for the long-term coordinated survey and monitoring of native island forest biota. Biodivers. Conserv. 27, 2567–2586.
Borges, P. A., Rigal, F., Ros-Prieto, A., and Cardoso, P. (2020). Increase of insular exotic arthropod diversity is a fundamental dimension of the current biodiversity crisis. Insect Conserv. Divers. 13, 508–518.
Cabral, J. S., Valente, L., and Hartig, F. (2017). Mechanistic simulation models in macroecology and biogeography: state-of-art and prospects. Ecography 40, 267–280.
Cirtwill, A. R., and Stouffer, D. B. (2016). Knowledge of predator–prey interactions improves predictions of immigration and extinction in island biogeography. Glob. Ecol. Biogeogr. 25, 900–911.
Cock, M. J., Murphy, S. T., Kairo, M. T., Thompson, E., Murphy, R. J., and Francis, A. W. (2016). Trends in the classical biological control of insect pests by insects: an update of the BIOCAT database. BioControl 61, 349–363.
Corcos, D., Cerretti, P., Mei, M., Vigna Taglianti, A., Paniccia, D., Santoiemma, G., et al. (2018). Predator and parasitoid insects along elevational gradients: role of temperature and habitat diversity. Oecologia 188, 193–202. doi: 10.1007/s00442-018-4169-4
Dainese, M., Martin, E. A., Aizen, M. A., Albrecht, M., Bartomeus, I., Bommarco, R., et al. (2019). A global synthesis reveals biodiversity-mediated benefits for crop production. Sci. Adv. 5:eaax0121. doi: 10.1126/sciadv.aax0121
Dangles, O., Carpio, C., Barragan, A. R., Zeddam, J. L., and Silvain, J. F. (2008). Temperature as a key driver of ecological sorting among invasive pest species in the tropical Andes. Ecol. Appl. 18, 1795–1809. doi: 10.1890/07-1638.1
De Bach, P. (1962). An analysis of successes in biological control of insects in the Pacific area. Proc. Hawaii. Entomol. Soc. 18, 69–79.
Decourtye, A., Alaux, C., Le Conte, Y., and Henry, M. (2019). Toward the protection of bees and pollination under global change: present and future perspectives in a challenging applied science. Curr. Opin. Insect Sci. 35, 123–131. doi: 10.1016/j.cois.2019.07.008
Eisenhauer, N., Bonn, A., and Guerra, C. A. (2019). Recognizing the quiet extinction of invertebrates. Nat. Commun. 10:50. doi: 10.1038/s41467-018-07916-1
Funasaki, G. Y., Lai, P. Y., Nakahara, L. M., Beardsley, J. W., and Ota, A. K. (1988). A review of biological control introductions in Hawaii: 1890 to 1985. Proc. Hawaii. Entomol. Soc. 28, 105–160.
Furlong, M. J. (2015). Knowing your enemies: integrating molecular and ecological methods to assess the impact of arthropod predators on crop pests. Insect Sci. 22, 6–19. doi: 10.1111/1744-7917.12157
Furlong, M. J., and Zalucki, M. P. (2017). Climate change and biological control: the consequences of increasing temperatures on host–parasitoid interactions. Curr. Opin. Insect Sci. 20, 39–44. doi: 10.1016/j.cois.2017.03.006
Galiana, N., Lurgi, M., Bastazini, V. A., Bosch, J., Cagnolo, L., Cazelles, K., et al. (2022). Ecological network complexity scales with area. Nat. Ecol. Evol. 6, 307–314. doi: 10.1038/s41559-021-01644-4
Galiana, N., Lurgi, M., Claramunt-López, B., Fortin, M. J., Leroux, S., Cazelles, K., et al. (2018). The spatial scaling of species interaction networks. Nat. Ecol. Evol. 2, 782–790. doi: 10.1038/s41559-018-0517-3
Gravel, D., Massol, F., Canard, E., Mouillot, D., and Mouquet, N. (2011). Trophic theory of Island biogeography. Ecol. Lett. 14, 1010–1016.
Grevstad, F. S. (1999). Experimental invasions using biological control introductions: the influence of release size on the chance of population establishment. Biol. Invasions 1, 313–323.
Guimarães, P. R. Jr. (2020). The structure of ecological networks across levels of organization. Annu. Rev. Ecol. Evol. Syst. 51, 433–460.
Hajek, A. E., McManus, M. L., and Junior, I. D. (2007). A review of introductions of pathogens and nematodes for classical biological control of insects and mites. Biol. Control 41, 1–13.
Hall, R., and Ehler, L. E. (1979). Rate of establishment of natural enemies in classical biological control. Bull. ESA 25, 280–283.
Heimpel, G. E., and Asplen, M. K. (2011). A ‘Goldilocks’ hypothesis for dispersal of biological control agents. Biocontrol 56, 441–450.
Hodkinson, I. D. (2005). Terrestrial insects along elevation gradients: species and community responses to altitude. Biol. Rev. 80, 489–513. doi: 10.1017/s1464793105006767
Holt, R. D. (2009). “Toward a trophic island biogeography,” in The Theory of Island Biogeography Revisited, eds J. B. Losos and R. E. Ricklefs (Princeton, NJ: Princeton University Press), 143–185.
Holt, R. D., Gravel, D., Stier, A., and Rosindell, J. (2020). “On the interface of food webs and spatial ecology: the trophic dimension of species–area relationships,” in The Species–Area Relationship: Theory and Application, eds T. J. Matthews, K. A. Triantis, and R. J. Whittaker (Cambridge, MA: Cambridge University Press), 289–318.
Kadmon, R., and Allouche, O. (2007). Integrating the effects of area, isolation, and habitat heterogeneity on species diversity: a unification of Island biogeography and niche theory. Am. Nat. 170, 443–454. doi: 10.1086/519853
Kaser, J. M., and Heimpel, G. E. (2015). Linking risk and efficacy in biological control host–parasitoid models. Biol. Control 90, 49–60.
Kühsel, S., and Blüthgen, N. (2015). High diversity stabilizes the thermal resilience of pollinator communities in intensively managed Grasslands. Nat. Commun. 6, 1–10. doi: 10.1038/ncomms8989
Laliberté, E., and Tylianakis, J. M. (2010). Deforestation homogenizes tropical parasitoid–host networks. Ecology 91, 1740–1747. doi: 10.1890/09-1328.1
Lara-Romero, C., Seguí, J., Pérez-Delgado, A., Nogales, M., and Traveset, A. (2019). Beta diversity and specialization in plant–pollinator networks along an elevational gradient. J. Biogeogr. 46, 1598–1610.
Le Lann, C., van Baaren, J., and Visser, B. (2021). Dealing with predictable and unpredictable temperatures in a climate change context: the case of parasitoids and their hosts. J. Exp. Biol. 224(Suppl. 1):jeb238626. doi: 10.1242/jeb.238626
Lehmann, P., Ammunét, T., Barton, M., Battisti, A., Eigenbrode, S. D., Jepsen, J. U., et al. (2020). Complex responses of global insect pests to climate warming. Front. Ecol. Environ. 18:141–150. doi: 10.1002/fee.2160
MacArthur, R. H., and Wilson, E. O. (1967). The Theory of Island Biogeography. Princeton University Press: Princeton, NJ.
Mason, P. G. (2021). Biological Control: Global Impacts, Challenges and Future Directions of Pest Management. Clayton: CSIRO Publishing.
Massol, F., Dubart, M., Calcagno, V., Cazelles, K., Jacquet, C., Kéfi, S., et al. (2017). Island biogeography of food webs. Adv. Ecol. Res. 56, 183–262.
Maunsell, S. C., Kitching, R. L., Burwell, C. J., and Morris, R. J. (2015). Changes in host–parasitoid food web structure with elevation. J. Anim. Ecol. 84, 353–363. doi: 10.1111/1365-2656.12285
Merrill, R. M., Gutiérrez, D., Lewis, O. T., Gutiérrez, J., Díez, S. B., and Wilson, R. J. (2008).. Combined effects of climate and biotic interactions on the elevational range of a phytophagous insect. J. Anim. Ecol. 77, 145–155. doi: 10.1111/j.1365-2656.2007.01303.x
Messing, R. H., and Wright, M. G. (2006). Biological control of invasive species: solution or pollution? Front. Ecol. Environ. 4:132–140. doi: 10.1890/1540-92952006004[0132:BCOISS]2.0.CO;2
Miller-Struttmann, N. E., and Galen, C. (2014). High-altitude multi-taskers: bumble bee food plant use broadens along an altitudinal productivity gradient. Oecologia 176, 1033–1045. doi: 10.1007/s00442-014-3066-8
Molina-Ochoa, J., Carpenter, J. E., Lezama-Gutiérrez, R., Foster, J. E., González-Ramírez, M., Angel-Sahagún, C. A., et al. (2004). Natural distribution of hymenopteran parasitoids of Spodoptera frugiperda (Lepidoptera: Noctuidae) larvae in Mexico. Fla. Entomol. 87, 461–472.
Montoya, J. M., Rodríguez, M. A., and Hawkins, B. A. (2003). Food web complexity and higher-level ecosystem services. Ecol. Lett. 6, 587–593.
Moreira, X., and Abdala-Roberts, L. (2021). A roadmap for future research on insularity effects on plant–herbivore interactions. Glob. Ecol. Biogeogr. 31, 602–610.
Moreira, X., Castagneyrol, B., García-Verdugo, C., and Abdala-Roberts, L. (2021). A meta-analysis of insularity effects on herbivory and plant defences. J. Biogeogr. 48, 386–393.
Moreira, X., Petry, W. K., Mooney, K. A., Rasmann, S., and Abdala-Roberts, L. (2018). Elevational gradients in plant defences and insect herbivory: recent advances in the field and prospects for future research. Ecography 41, 1485–1496.
Moret, P., Muriel, P., Jaramillo, R., and Dangles, O. (2019). Humboldt’s tableau physique revisited. Proc. Natl. Acad. Sci. 116, 12889–12894. doi: 10.1073/pnas.1904585116
Moya-Raygoza, G., Albarracin, E. L., and Virla, E. G. (2012). Diversity of egg parasitoids attacking Dalbulus maidis (Hemiptera: Cicadellidae) populations at low and high elevation sites in Mexico and Argentina. Fla. Entomol. 95, 105–112.
Nafus, D., and Schreiner, I. (1989). Biological control activities in the Mariana Islands from 1911 to 1988. Micronesica 22, 65–106.
Nechols, J. R. (2021). The potential impact of climate change on non-target risks from imported generalist natural enemies and on biological control. Biocontrol 66, 37–44.
Ostandie, N., Muneret, L., Giffard, B., Thiéry, D., and Rusch, A. (2021). The shape of the predator biomass distribution affects biological pest control services in agricultural landscapes. Funct. Ecol. 35, 193–204.
Péré, C., Jactel, H., and Kenis, M. (2013). Response of insect parasitism to elevation depends on host and parasitoid life-history strategies. Biol. Lett. 9:20130028. doi: 10.1098/rsbl.2013.0028
Perović, D. J., Gámez-Virués, S., Landis, D. A., Wäckers, F., Gurr, G. M., Wratten, S. D., et al. (2018). Managing biological control services through multi-trophic trait interactions: review and guidelines for implementation at local and landscape scales. Biol. Rev. 93, 306–321. doi: 10.1111/brv.12346
Peters, M. K., Hemp, A., Appelhans, T., Behler, C., Classen, A., Detsch, F., et al. (2016). Predictors of elevational biodiversity gradients change from single taxa to the multi-taxa community level. Nat. Commun. 7, 1–11. doi: 10.1038/ncomms13736
Polis, G. A., and Hurd, S. D. (1995). Extraordinarily high spider densities on Islands: flow of energy from the marine to terrestrial food webs and the absence of predation. Proc. Natl. Acad. Sci. 92, 4382–4386. doi: 10.1073/pnas.92.10.4382
Rasmann, S., Alvarez, N., and Pellissier, L. (2014a). The altitudinal niche-breadth hypothesis in insect-plant interactions. Annu. Plant Rev. 47, 339–359.
Rasmann, S., Pellissier, L., Defossez, E., Jactel, H., and Kunstler, G. (2014b). Climate-driven change in plant–insect interactions along elevation gradients. Funct. Ecol. 28, 46–54.
Ripa, S. R., Rojas, P. S., and Velasco, G. (1995). Releases of biological control agents of insect pests on Easter Island (Pacific Ocean). Entomophaga 40, 427–440.
Roslin, T., Várkonyi, G., Koponen, M., Vikberg, V., and Nieminen, M. (2014). Species–area relationships across four trophic levels–decreasing island size truncates food chains. Ecography 37, 443–453.
Ruiz-Hurtado, F. M., Ramirez-Quimbayo, J. H., Rojas-Plazas, B., Galeano-Olaya, P. E., and Canal, N. A. (2013). Diversidad de parasitoides (Hymenoptera) de Moscas frugívoras (Diptera: Tephritoidea) en dos áreas cafeteras del departamento del Tolima, Colombia. Rev. Tumbaga 2, 29–54.
Saavedra-Díaz, J., Galeano-Olaya, P. E., and Canal, D. N. A. (2017). Ecologic relationships among host fruits, frugivorous flies and parasitoids in a fragment of tropical dry forest. Rev. Cienc. Agrícolas 34, 32–49.
Salazar-Mendoza, P., Peralta-Aragón, I., Romero-Rivas, L., Salamanca, J., and Rodriguez-Saona, C. (2021). The abundance and diversity of fruit flies and their parasitoids change with elevation in guava orchards in a tropical Andean forest of Peru, independent of seasonality. PloS one 16:e0250731. doi: 10.1371/journal.pone.0250731
Santos, A., and Quicke, D. L. (2011). Pattern and process in host-parasitoid interactions. Entomol. Sci. 14, 371–382.
Santos, A. M., Field, R., and Ricklefs, R. E. (2016b). New directions in island biogeography. Glob. Ecol. Biogeogr. 25, 751–768.
Santos, A. M., Cianciaruso, M. V., and De Marco, P. Jr. (2016a). Global patterns of functional diversity and assemblage structure of Island parasitoid faunas. Glob. Ecol. Biogeogr. 25, 869–879.
Santos, A. M., Quicke, D. L., Borges, P. A., and Hortal, J. (2011b). Species pool structure determines the level of generalism of Island parasitoid faunas. J. Biogeogr. 38, 1657–1667.
Santos, A. M., Fontaine, C., Quicke, D. L., Borges, P. A., and Hortal, J. (2011a). Are island and mainland biotas different? Richness and level of generalism in parasitoids of a microlepidopteran in Macaronesia. Oikos 120, 1256–1262.
Santos, A. M., Whittaker, R. J., Triantis, K. A., Borges, P. A., Jones, O. R., Quicke, D. L., et al. (2010). Are species area relationships from entire archipelagos congruent with those of their constituent islands? Glob. Ecol. Biogeogr. 19, 527–540.
Schmitz, O. J., and Barton, B. T. (2014). Climate change effects on behavioral and physiological ecology of predator–prey interactions: implications for conservation biological control. Biol. Control 75, 87–96.
Schmitz, O. J., Miller, J. R., Trainor, A. M., and Abrahms, B. (2017). Toward a community ecology of landscapes: predicting multiple predator–prey interactions across geographic space. Ecology 98, 2281–2292. doi: 10.1002/ecy.1916
Schoener, T. W., Spiller, D. A., and Piovia-Scott, J. (2016). Variation in ecological interaction strength with Island area: theory and data from the Bahamian archipelago. Glob. Ecol. Biogeogr. 25, 891–899.
Schrodt, F., Santos, M. J., Bailey, J. J., and Field, R. (2019). Challenges and opportunities for biogeography—What can we still learn from von Humboldt? J. Biogeogr. 46, 1631–1642.
Sivinski, J., Pinero, J., and Aluja, M. (2000). The distributions of parasitoids (Hymenoptera) of Anastrepha fruit flies (Diptera: Tephritidae) along an altitudinal gradient in Veracruz, Mexico. Biol. Control 18, 258–269.
Steinbauer, M. J., Grytnes, J. A., Jurasinski, G., Kulonen, A., Lenoir, J., Pauli, H., et al. (2018). Accelerated increase in plant species richness on mountain summits is linked to warming. Nature 556, 231–234. doi: 10.1038/s41586-018-0005-6
Thierry, M., Hrèek, J., and Lewis, O. T. (2019). Mechanisms structuring host–parasitoid networks in a global warming context: a review. Ecol. Entomol. 44, 581–592.
Traveset, A., Tur, C., Trøjelsgaard, K., Heleno, R., Castro-Urgal, R., and Olesen, J. M. (2016). Global patterns of mainland and insular pollination networks. Glob. Ecol. Biogeogr. 25, 880–890.
Tylianakis, J. M., Didham, R. K., Bascompte, J., and Wardle, D. A. (2008). Global change and species interactions in terrestrial ecosystems. Ecol. Lett. 11, 1351–1363. doi: 10.1111/j.1461-0248.2008.01250.x
Valiente-Banuet, A., Aizen, M. A., Alcántara, J. M., Arroyo, J., Cocucci, A., Galetti, M., et al. (2015). Beyond species loss: the extinction of ecological interactions in a changing world. Funct. Ecol. 29, 299–307.
Van Driesche, R. G., and Hoddle, M. S. (2000). Classical Arthropod Biological Control: Measuring Success, Step by Step. In Biological Control: Measures of Success. Dordrecht: Springer, 39–75.
Warren, B. H., Simberloff, D., Ricklefs, R. E., Aguilée, R., Condamine, F. L., Gravel, D., et al. (2015). Islands as model systems in ecology and evolution: prospects fifty years after MacArthur-Wilson. Ecol. Lett. 18, 200–217. doi: 10.1111/ele.12398
Wright, D. H. (1983). Species-energy theory: an extension of species-area theory. Oikos 41, 496–506. doi: 10.2307/3544109
Wyckhuys, K. A., and O’Neil, R. J. (2006). Population dynamics of Spodoptera frugiperda Smith (Lepidoptera: Noctuidae) and associated arthropod natural enemies in Honduran subsistence maize. Crop Protection 25, 1180–1190.
Yang, F., Liu, B., Zhu, Y., Wyckhuys, K. A., van der Werf, W., and Lu, Y. (2021). Species diversity and food web structure jointly shape natural biological control in agricultural landscapes. Commun. Biol. 4, 1–11. doi: 10.1038/s42003-021-02509-z
Keywords: climate change, global warming, insect biodiversity loss, biological control, agroecology, island biogeography, community ecology, functional ecology
Citation: Wyckhuys KAG, Sanchez Garcia FJ, Santos AMC, Canal NA, Furlong MJ, Melo MC, GC YD and Pozsgai G (2022) Island and Mountain Ecosystems as Testbeds for Biological Control in the Anthropocene. Front. Ecol. Evol. 10:912628. doi: 10.3389/fevo.2022.912628
Received: 04 April 2022; Accepted: 30 May 2022;
Published: 24 June 2022.
Edited by:
Pavel Kindlmann, Charles University, CzechiaReviewed by:
Thomas Wassmer, Siena Heights University, United StatesCopyright © 2022 Wyckhuys, Sanchez Garcia, Santos, Canal, Furlong, Melo, GC and Pozsgai. This is an open-access article distributed under the terms of the Creative Commons Attribution License (CC BY). The use, distribution or reproduction in other forums is permitted, provided the original author(s) and the copyright owner(s) are credited and that the original publication in this journal is cited, in accordance with accepted academic practice. No use, distribution or reproduction is permitted which does not comply with these terms.
*Correspondence: Kris A. G. Wyckhuys, ay53eWNraHV5c0B1cS5lZHUuYXU=