- 1Department of Environmental Sciences, College of the Environment, Western Washington University, Bellingham, WA, United States
- 2The Evergreen State College, Olympia, WA, United States
Introduction: The increasing number of dams approaching obsolescence drives a need for knowledge about riparian restoration associated with dam removal. Restoring woody vegetation on exposed reservoir beds following dam removal is essential to stabilizing sediment, reconnecting riverine and terrestrial systems, and providing future sources of shade, nutrients, and wood. Revegetation after dam removal on many rivers can be challenging due to rapidly drying sediment, low sediment nutrient content, and heavy ungulate browse pressure. Revegetation in Elwha River restoration, the largest dam removal to date, used large woody debris (LWD) to mitigate moisture and nutrient limitation but ungulate browsing has constrained woody plant growth in many coarse sediment deposits. We evaluated potential for LWD to reduce ungulate browsing following Elwha dam removal.
Methods: We studied LWD mitigation of browsing in the largest former reservoir and a comparable valley upriver with a natural floodplain. We measured browse intensity in randomly located plots stratified by four levels of LWD extent, from no LWD to complete LWD enclosure.
Results: LWD reduced browse intensity four-fold in the former reservoir, but only in plots fully surrounded by LWD. Partial LWD enclosure provided little browse reduction. We obtained similar results in the upriver valley, where browse intensity was somewhat lower except within wood clusters. Wood-mediated browse reduction was slightly greater in the former reservoir than in the upriver valley. Protection from browse was greatest for plant species preferred by ungulates.
Discussion: These results suggest forest restoration after dam removal can be expedited by surrounding young trees with large logs. Planting within LWD clusters or placing LWD clusters in restoration sites can facilitate establishment of forest islands in strategic locations. These forest islands can support dispersal of seeds and marine derived nutrients, reconnect established forest to the river, and potentially advance restoration by decades.
1 Introduction
Rivers are vital to humanity and biodiversity (Lynch et al., 2023), but rivers are among the most imperiled systems on Earth (Dudgeon et al., 2006; Reid et al., 2019). Dams and impoundments are pervasive threats, impacting 60% of large rivers on Earth (World Commission on Dams, 2000) and all large river basins in the contiguous US (Graf, 1999). Dam removal is becoming widely recognized for efficacy in river restoration (O’Connor et al., 2015). Dam removal restores longitudinal connections in river systems, facilitating rapid responses by aquatic biota (Duda et al., 2021). Restoring lateral terrestrial–aquatic connections is slower and more challenging. Many dams sever connections between rivers and terrestrial systems by replacing lotic reaches with slackwater impoundments (Hjältén et al., 2016). After dam removal, reservoirs become open sediment deposits separating rivers from mature terrestrial habitats. Restoring mature habitats and vegetation on those sediments is at least challenging due to absence of structural legacies, rapid desiccation (Chenoweth et al., 2021), low sediment nutrient concentrations (Cavaliere and Homann, 2012), missing mycorrhizae (Cook et al., 2009; Cortese and Bunn, 2017), and herbivore browsing (Osei et al., 2015; McCaffery et al., 2020).
Restoring vegetation, habitat, and terrestrial–river connectivity is substantially more difficult for large dam removals than smaller projects because large dams and reservoirs create disproportionately large impacts. While small narrow impoundments may remain under the influence of adjacent stands of mature vegetation, large reservoir beds impose large distances separating active channels from mature vegetation. Restoring connections in small vs. large reservoir beds involves different processes and time scales. For example, the gap between an active channel and mature forest could be bridged by a single treefall on a narrow impoundment. Reconnecting river and forest across large reservoir beds requires growing forest on sediment deposits, which may take up to a century in boreal and temperate regions. Limited shade on large reservoir beds renders sediments prone to desiccation (Chenoweth et al., 2021), which further slows revegetation. Large reservoir sediment deposits are more distant from agents of ecological functions that support restoration, including litterfall, seed dispersers, and marine derived nutrient dispersers (McCaffery et al., 2018). Large rivers and river valleys support more complex channel dynamics, where extensive areas can be scoured and re-set to early successional seres (Collins et al., 2012). Similarly, revegetation in large reservoir beds may be impacted by disturbance regimes and patch dynamics that do not occur in smaller restoration projects. Relative effects of browse on revegetation following small versus large dam removals are unknown, due to limited study and complexity of confounding factors. Restoration site area, browser population abundance and seasonal distribution, adjacent habitat characteristics, alternative browser food sources, and exposure to predators may affect browse intensity in complex ways related to or independent of dam size. These issues are compounded by uncertainty: system responses to large expanses of sediment are unknown (Prach et al., 2019), with few prior large dam removals to serve as models. In summary, restoration following large dam removal is qualitatively distinct from small dam removals. Some lessons from small dam removals do not scale up simply to large projects, compelling a need to study large dam removals directly.
Restoring habitats and vegetation to dewatered reservoirs involves multiple challenges at several plant life stages (Table 1). Challenges at each stage must be overcome, culminating with browse mitigation during plant establishment and maturation stages addressed in this paper. First, plants must reach appropriate locations via active planting (Chenoweth et al., 2021) or seed dispersal by wind, water (Cubley and Brown, 2016), or animal vectors (Wang and Smith, 2002). Germination of dispersed seeds requires suitable sites and adequate moisture, which may be mediated by sediment texture (Osei et al., 2015), timing of reservoir drawdown (Muldavin et al., 2017; Chenoweth et al., 2021), or structures used by animal dispersers (McLaughlin, 2013). Plant establishment and growth require consistent sediment moisture, adequate nutrients, moderate temperatures, and protection from excessive herbivory (Maschinski et al., 2004; Heneghan et al., 2008; Osei et al., 2015). These needs can be addressed in part by planting adjacent to logs (Calimpong, 2014; Marangon et al., 2022), translocating large wood (McHenry and Chenoweth, 2015; Neilly and Cale, 2020), seeding nitrogen-fixing plants (Chenoweth et al., 2021), and inoculating with mycorrhizae (Hoeksema et al., 2010; Hawkins et al., 2015). Plant growth to maturity requires resources similar to establishment, with protection from herbivory becoming particularly important in systems with abundant browsers (Opperman and Merenlender, 2000; Peinetti et al., 2001; Zeigenfuss et al., 2002; Osei et al., 2015; Averett et al., 2017). Where intense browsing is not mitigated, plants can remain in an arrested growth form (Keigley, 1997) or become eliminated entirely (Opperman and Merenlender, 2000; Whyte and Lusk, 2019). Impacts of unrestrained browsing can persist for decades (Reed et al., 2021; Woodward et al., 2021).
Several interventions to mitigate vertebrate browsing have documented efficacy, including fencing, tree shelters, apex predator restoration, and large woody debris. Fencing can exclude herbivores (Opperman and Merenlender, 2000; Brookshire et al., 2002; Maschinski et al., 2004; Monks et al., 2023), but only if fences are maintained (Woodward et al., 1994). Fences can create undesirable impacts to connectivity (Jakes et al., 2018; Xu et al., 2021), and may contradict land management policies in some restoration sites. Tree shelters can increase woody plant survival rate substantially (Stange and Shea, 1998), but plastic residue may not be desirable in some sites. Reintroducing predators can restore riparian shrubs and forests by restoring “landscapes of fear” that reduce browse in riparian zones (Beschta and Ripple, 2008; Beschta and Ripple, 2010). Social and political factors may determine where this approach is practicable (Chapron et al., 2014; Richardson, 2023; Wakeling, 2023). Large wood can reduce ungulate access to plants, facilitating growth to maturity where otherwise browsing would exclude or suppress woody plants (Rooney, 1995; Schreiner et al., 1996).
Despite consistent efficacy of natural or placed large wood in mitigating browse impacts, this strategy has received limited attention in the restoration literature. Managing browse with large wood has been reported in fewer than 25 articles in the last 30 years, based on work on four continents. Just two articles addressed wood-mediated browse reduction in riparian systems (Matney et al., 2005; Muldavin et al., 2017). By comparison, more than 500 articles were published in the same period on limiting browse by other means or measuring its impacts in sites on six continents. Similarly, only 22 of the 306 unduplicated sources in the USGS Dam Removal Science Database (Duda et al., 2018) addressed vegetation responses to dam removal. Of those 22, just 5 considered large wood, and only one (McCaffery et al., 2020) mentioned the potential for large wood to reduce browse. The discrepancy between efficacy and attention to wood-mediated browse reduction may reflect a dearth of large wood in most forests and rivers, following centuries of large wood removal (Rooney, 1995; Wohl, 2014). We worked to address this discrepancy in forest restoration associated with dam removal.
We studied ungulate browse on woody plants in recently exposed sediments in broad valleys along the Elwha River, site of humanity’s largest dam removals to date (Ritchie et al., 2018). Our goal was to determine whether large woody debris (LWD) can protect young woody plants from ungulate browsing. Initial observations suggested six hypotheses regarding effects of browsing and large wood on riparian restoration (Table 2). We focused on two in work reported here (Table 2, hypotheses 3 and 4). Our primary hypothesis was that browse intensity would be inversely proportional to extent of large wood enclosure. Our secondary hypothesis was that LWD-mediated browse reduction would be proportional to species preferences by ungulate browsers. We evaluated these hypotheses in two valleys along the Elwha River: the recently exposed reservoir bed directly above the largest dam removal and a comparable valley upstream of both of the Elwha’s former dams and reservoirs. The two valleys represent opposite ends in a riparian restoration continuum. Vegetation in the drained reservoir was early successional, growing in substrates recently exposed to a receding water table, dispersing seeds, desiccating wind and insolation, and herbivory. The upstream valley contained a diverse successional vegetation mosaic resulting from long exposure to those factors and episodic flooding throughout the Holocene (Acker et al., 2008; Warrick et al., 2011; Brown et al., 2022). By working in the two valleys, we hoped to derive insights regarding browse impacts and LWD mediation from early years to late stages in riparian restoration.
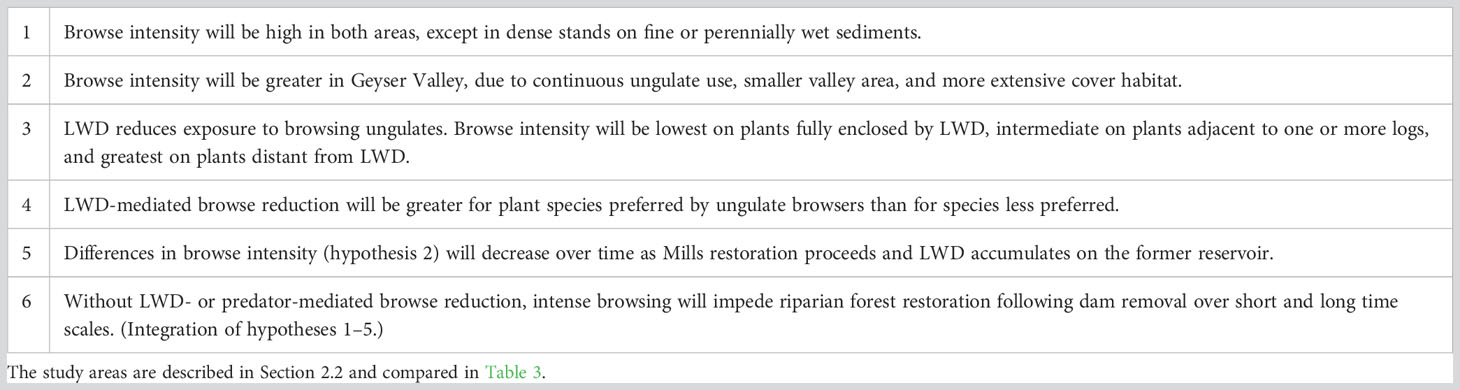
Table 2 Hypotheses regarding browse pressure on woody plants growing in coarse sediments in the two study areas, drained Mills reservoir and Geyser Valley floodplain.
2 Study area and restoration background
2.1 Study system
The Elwha River drains the largest basin in the Olympic Mountains, a coastal range in northwestern Washington State (Figure 1). The river flows north 72 kilometers from snowbound headwaters to the Strait of Juan de Fuca. Eighty-three percent of the Elwha’s 833 km2 basin lies within Olympic National Park (Duda et al., 2008), which has protected it from many anthropogenic stressors and simplified interpretation of ecosystem responses to restoration. The lower basin contains a mosaic of public, private, and Lower Elwha Klallam Tribal lands. The basin has a maritime climate, characterized by wet mild winters and warm dry summers. Conifers dominate most of the basin, particularly Douglas fir (Pseudotsuga menziesii), western hemlock (Tsuga heterophylla), and western red cedar (Thuja plicata). Mixed conifer–hardwood stands occur in many areas, where red alder (Alnus rubra), bigleaf maple (Acer macrophyllum), and black cottonwood (Populus balsamifera) intersperse with conifers. Floodplains contain vegetation mosaics (Kloehn et al., 2008), including mature forests and young stands of alder, cottonwood, and willows (Salix spp.; National Park Service (NPS), 1996). More information about the Elwha basin is in Duda et al. (2008).
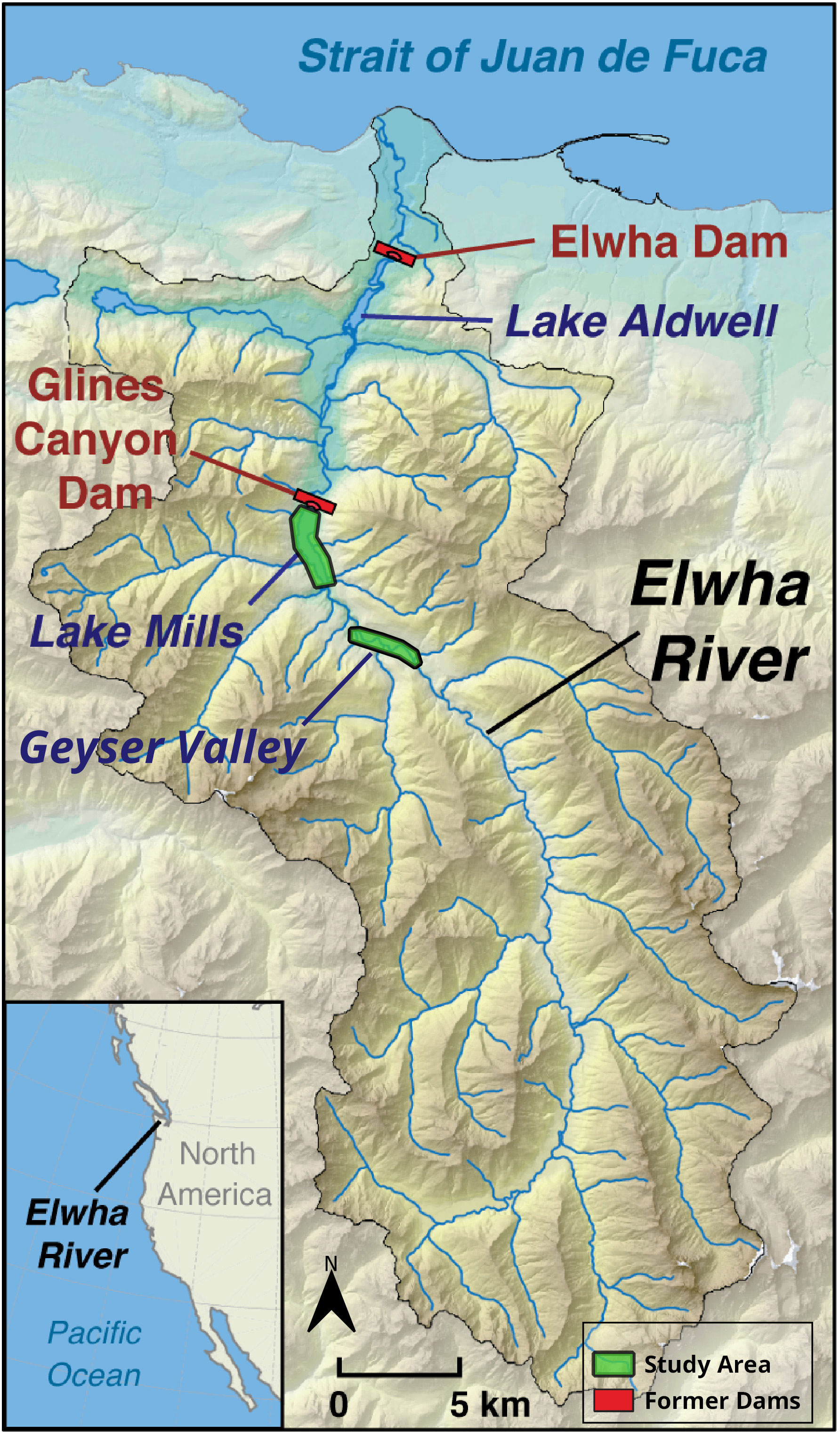
Figure 1 Map of the Elwha River basin, including locations of former dams and study areas in Geyser Valley and the former Mills reservoir. Map by CJ, modified from original at: https://www.usgs.gov/media/images/map-elwha-river-state-washington.
Two large hydroelectric dams on the Elwha obstructed passage of sediment, wood, and aquatic biota for nearly a century, during which 21 million m3 ( ± 3 million m3) of sediment accumulated in the two reservoirs (East et al., 2015). The 32 m tall Elwha Dam was built in 1910–1913 at river kilometer (rkm) 7.9 and impounded the 120-ha Aldwell reservoir. The 64 m tall Glines Canyon Dam was built in 1927 at rkm 21.4, impounding the 172-ha Mills reservoir. Both dams were in the traditional territory of the Lower Elwha Klallam Tribe, who opposed the dams, suffered from their impacts, and initiated the process leading to dam removal (Mapes, 2013; Brewitt, 2019; Mauer, 2021). Most (76%) of the impounded sediment was in the larger and upper Mills reservoir (East et al., 2015). Both dams were removed in a phased process from 2011 to 2014. In the five years following the start of dam removal, 65% of the impounded sediment eroded from the former reservoirs (Ritchie et al., 2018). The residual sediment deposits provide substrates targeted for forest restoration (Prach et al., 2019).
2.2 Study areas
Our two study areas share similar environmental and contextual factors, but differ in several characteristics relevant to browse exposure and large wood distribution. Table 3 summarizes these similarities and differences.
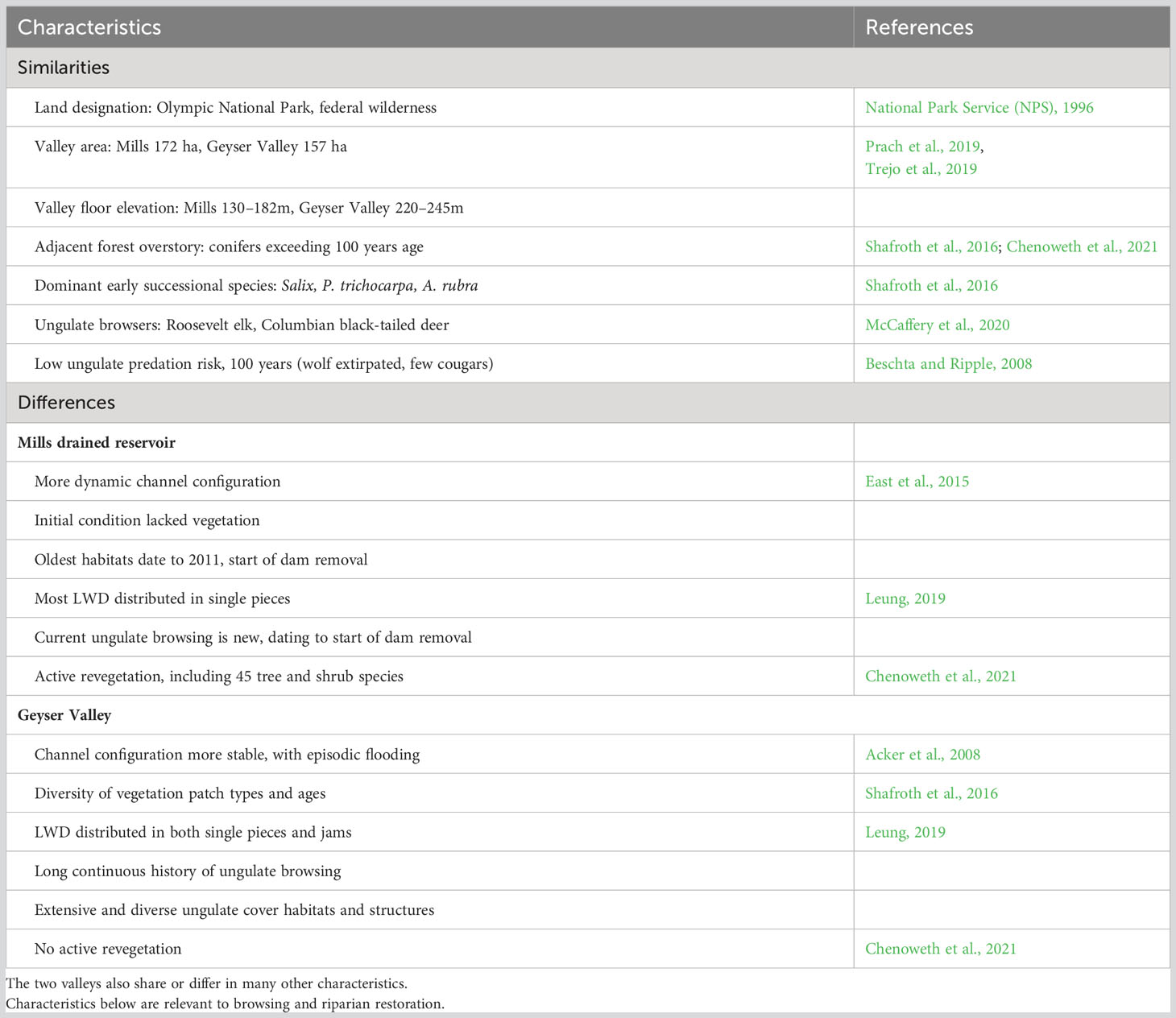
Table 3 Comparison of the two study areas, the drained Mills reservoir and Geyser Valley floodplain.
The dewatered Mills reservoir bed is the largest restoration site associated with the Elwha River Ecosystem Restoration project (Figure 1). The Mills reservoir accumulated 16 million m3 (± 3 million m3) of sediments during the 84 years following dam construction (East et al., 2015). The river eroded most (65%) of this sediment in the five years since dam removal started, of which 90% was transported to the coast and 10% was redistributed within the reservoir bed and downstream river locations (Ritchie et al., 2018). The river and its active floodplain occupy 37% of the Mills reservoir bed (Prach et al., 2019), where chronic disturbance impedes woody plant establishment. One fifth (19%) of the Mills reservoir bed consists of steep slopes along the valley wall, covered by fine lacustrine sediments that now support dense stands of young cottonwoods, alders, and willows (Prach et al., 2019). Water retention by fine sediments supported growth of woody plants, and those stands rapidly exceeded the reach of ungulate browsers. The remaining 44% of the former Mills reservoir bed consists of valley bottom and terraces covered by coarse sediments (Prach et al., 2019). Poor moisture retention in the coarse sediments causes drought stress in woody plants, which occur sparsely and grow slowly during the dry growing season (Prach et al., 2019; Chenoweth et al., 2021). Most large wood in the valley is distributed as single logs or stumps, resulting from upriver transport, hydraulic excavation from eroding sediments, or active translocation (McHenry and Chenoweth, 2015; Leung, 2019).
Geyser Valley is the largest unconstrained reach above the dams and reservoirs (Figure 1). Although the dams prevented anadromous fish from reaching Geyser Valley for a century, it was not otherwise directly impacted by the dams. The valley extends from rkm 27.3 to 31.1, and is separated from the dewatered Mills reservoir by the 1.2 km long Rica Canyon. Geyser Valley spans 1.57 km2, an area comparable to the former Mills reservoir. The valley’s geomorphology and vegetation have been shaped by a history of disturbance by high flow events (Acker et al., 2008). High flows influenced valley sediment distributions, disturbed riparian vegetation, and deposited or redistributed large wood. Similar to other Pacific Northwest rivers with “natural wood regimes” (Wohl et al., 2019), large wood in Geyser Valley is distributed as both individual logs and in multi-log jams. Log jams and spatial variation in disturbance history have generated a mosaic of successional seres (Abbe and Montgomery, 1996; Collins et al., 2012). Geyser Valley is considered a model for post-dam removal restoration of the dewatered Elwha reservoir beds, with particular relevance to the first restoration goal stated below.
Elwha valleys support large populations of Roosevelt elk (Cervus elaphus roosevelti) and Columbian black-tailed deer (Odocoileus hemionus columbianus; McCaffery et al., 2020). Elk winter in valleys and migrate to higher elevations as snow melts in late spring (Jenkins et al., 2015). Deer remain in valleys throughout the year. Ungulate browsing strongly affects valley forest patch structure and dynamics, tree recruitment, and woody plant architecture in Olympic National Park (Schreiner et al., 1996; Beschta and Ripple, 2008), including Elwha riparian forests (Woodward et al., 1994; McCaffery et al., 2020). Browse intensity, measured as fraction of the previous year’s growth, was high in both study areas. Annual browse intensity (years 2015–2018) on all woody species averaged 48.1% on the Mills reservoir and 84.2% in Geyser Valley (calculation based on data in McCaffery et al., 2020, Supplementary Material).
2.3 Reservoir revegetation
The Elwha revegetation and restoration program was designed to achieve three goals on the dewatered reservoir beds: restore native forest, stabilize residual sediment, and minimize spread of invasive exotic species (Chenoweth et al., 2011). The revegetation program lasted six years beginning concurrently with dam removal, using a combination of active seeding, active planting of woody species, passive approaches, and untreated control sites (Chenoweth et al., 2021). Early monitoring showed rapid growth of naturally dispersed cottonwood, alder, and willows in dense stands on fine sediments (Prach et al., 2019; Chenoweth et al., 2021). Subsequently, active planting focused on coarse sediments where low moisture retention slowed early plant establishment (Chenoweth et al., 2021). Recently, natural establishment of cottonwood and willow seedlings on some terraces has been extensive. The planting program included more than 205,000 woody plants of 64 native species (Chenoweth et al., 2021). Active revegetation was not conducted in the active floodplain.
Vegetation monitoring results showed that woody plant establishment was slower on coarse sediments, attributed to moisture and nutrient limitation (Calimpong, 2014; Schuster, 2015; Prach et al., 2019). Moisture limitation presumably resulted from rapid water percolation through coarse sediments, likely compounded by high wind exposure on reservoir terraces. The revegetation program attempted to mitigate drought stress and reduce wind exposure by translocating LWD from accumulation sites along the reservoir perimeter to coarse sediment terraces (McHenry and Chenoweth, 2015). Most translocated logs were placed individually in an east–west orientation, to maximize shaded sediment area on the north side (McHenry and Chenoweth, 2015). Although potential impacts of browsing were acknowledged (Chenoweth et al., 2011), the revegetation program did not implement measures to limit browsing.
3 Materials and methods
3.1 Sampling design and methods
We measured browse intensity on young woody plants on coarse sediment terraces in the former Mills reservoir and coarse floodplain sediments in Geyser Valley. We restricted sampling to early successional habitats with woody plants growing within reach of ungulate browsers. We collected data during late April–May in 2018 (Mills) and 2019 (Geyser Valley). We measured recent browse on plants within or adjacent to four LWD configurations: no LWD, adjacent to single logs, between two parallel logs, and fully enclosed within LWD clusters.
We sampled woody plants using a stratified random design with strata defined by the four LWD configurations. Large woody debris was abundant in the Elwha valleys (Ritchie et al., 2018), but log clusters were less numerous than individual logs. To ensure balanced sampling among LWD configurations, we used a stratified design anchored on LWD cluster locations. Relative to each LWD cluster, we located the nearest single log, the nearest pair of parallel logs, and the nearest area free of LWD within a 10 meter radius. Although LWD usually is defined as any wood exceeding 10 cm diameter and 1 m length (Gurnell, 2013; Gregory et al., 2017; Wohl, 2017), we restricted sampling to logs at least 50 cm above the ground and 5 m long to ensure wood structures were large enough to function as partial barriers to ungulates. At each sampling site, we delineated a 5 m × 1 m plot oriented parallel to the largest log. We used an elongated plot shape to ensure all plants in LWD plots were close to LWD. We placed plots without LWD in random orientations. In the Mills valley, we sampled 140 plots: 42 plots in areas without wood, 35 plots adjacent to individual logs, 33 plots between parallel logs, and 30 plots within wood clusters. In Geyser Valley, we sampled 128 plots: 32 plots in each of the four LWD configurations. Within each plot, we recorded the total number of stems and the number of recently browsed stems on each woody plant.
3.2 Data analysis
We calculated aggregate browse intensity for plants in each plot as the ratio of browsed stems to total stems. We normalized browse percentage data using an arcsine transformation. Then we compared mean browse intensity among the four LWD configurations within each valley using analysis of variance on transformed browse intensity values. We identified configuration-specific differences in browse intensity using a Tukey multiple comparisons test.
We compared effects of study area on LWD mediation of browse using two-factor analysis, with site and LWD plot type as factors. We fit the model without an intercept to obtain site-specific browse means. We included a site × plot interaction term to evaluate whether LWD-mediated browse effects differed between the two study areas.
We compared browse intensity among the plant species selected by ungulates with high, medium, and low intensities. We classified plant species into one of the three browse selection categories using Supplementary Data in McCaffery et al. (2020). We rated species in McCaffery et al. (2020) with annual browse intensities exceeding 66% as strong selection: bigleaf maple (Acer macrophyllum), Douglas maple (Acer glabrum), Nootka rose (Rosa nutkana), red-osier dogwood (Cornus sericea), thimbleberry (Rubus parviflorus). We rated species with annual browse intensities between 34% and 66% as moderate selection: black cottonwood, willows, western red cedar, bitter cherry (Prunus emarginata), oceanspray (Holodiscus discolor), and serviceberry (Amelanchier alnifolia). We rated species with annual browse intensities of 33% or less as low selection: Douglas fir, western hemlock, grand fir (Abies grandis), salmonberry (Rubus spectabilis), and western white pine (Pinus monticola). We evaluated ungulate selection preference in the context of LWD configuration using two-factor analysis of variance with two LWD levels: LWD clusters and sites without LWD. We evaluated proportionality of wood-mediated browse reductions using the selection × LWD interaction term. We conducted all analyses using R (version 4.3.1; R Core Team, 2023).
4 Results
Our plots on the Mills reservoir bed contained 931 plants of 19 tree and shrub species. Our Geyser Valley plots contained 784 plants of 14 species. Woody plant densities were similar among plots in the two valleys: 1.4 plants/m2 in Mills and 1.3 plants/m2 in Geyser Valley.
In both valleys, browse rates differed substantially and significantly among LWD configurations (Mills: F3,136 = 20.5, p < 10−10; Geyser Valley: F3,124 = 8.8, p < 10−4). Differences in browse rates among LWD configuration types were largely due to lower browse rates within clusters than other wood configurations. Mean browse rate in LWD cluster plots was several-fold lower than in plots without LWD. In Mills, mean browse rate within clusters was four times lower than in open plots (0.135 vs. 0.541, p < 10−7; Figure 2). In Geyser Valley, mean browse rate within clusters was 2.5 times lower than in open plots (0.16 vs. 0.40, p < 10−4; Figure 3). Mean browse rates within wood clusters also were substantially lower than plots associated with single logs or two parallel logs (Tukey’s q: p ≤ 0.0004 for Mills, Figure 2; p ≤ 0.001 for Geyser Valley, Figure 3).
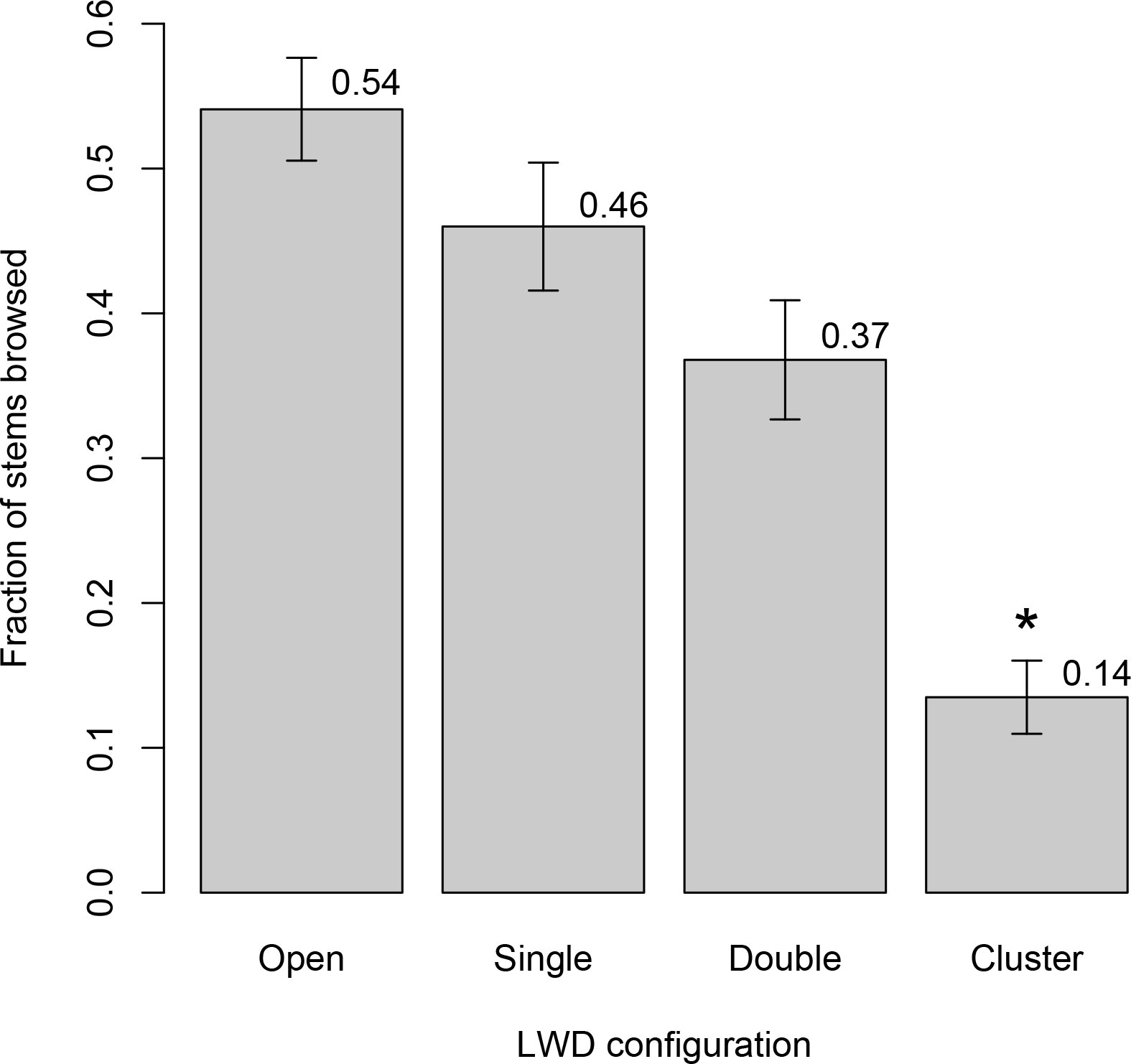
Figure 2 Browse intensity on woody plants growing in coarse sediment terraces on the drained Mills reservoir bed. Labels on the horizontal axis refer to large wood characteristics associated with each of four plot types. “Open” plots were at least 10 meters from any LWD. “Single” plots were adjacent to one log. “Double” plots were aligned between two parallel logs. “Cluster” plots were fully surrounded by LWD. All woody debris used to determine plot types stood at least 50 cm above ground level. Values on the vertical axis are mean browse rates, expressed as fraction of stems browsed relative to all stems produced within the previous year. Bar heights are mean per-plot browse rates, and error bars are +/− one standard error. The asterisk (*) indicates mean browse rate in cluster plots was significantly lower than all other wood categories. Mean browse rate in “Double” plots was significantly lower than “Open” plots, but the difference with “Single” plots was not significant.
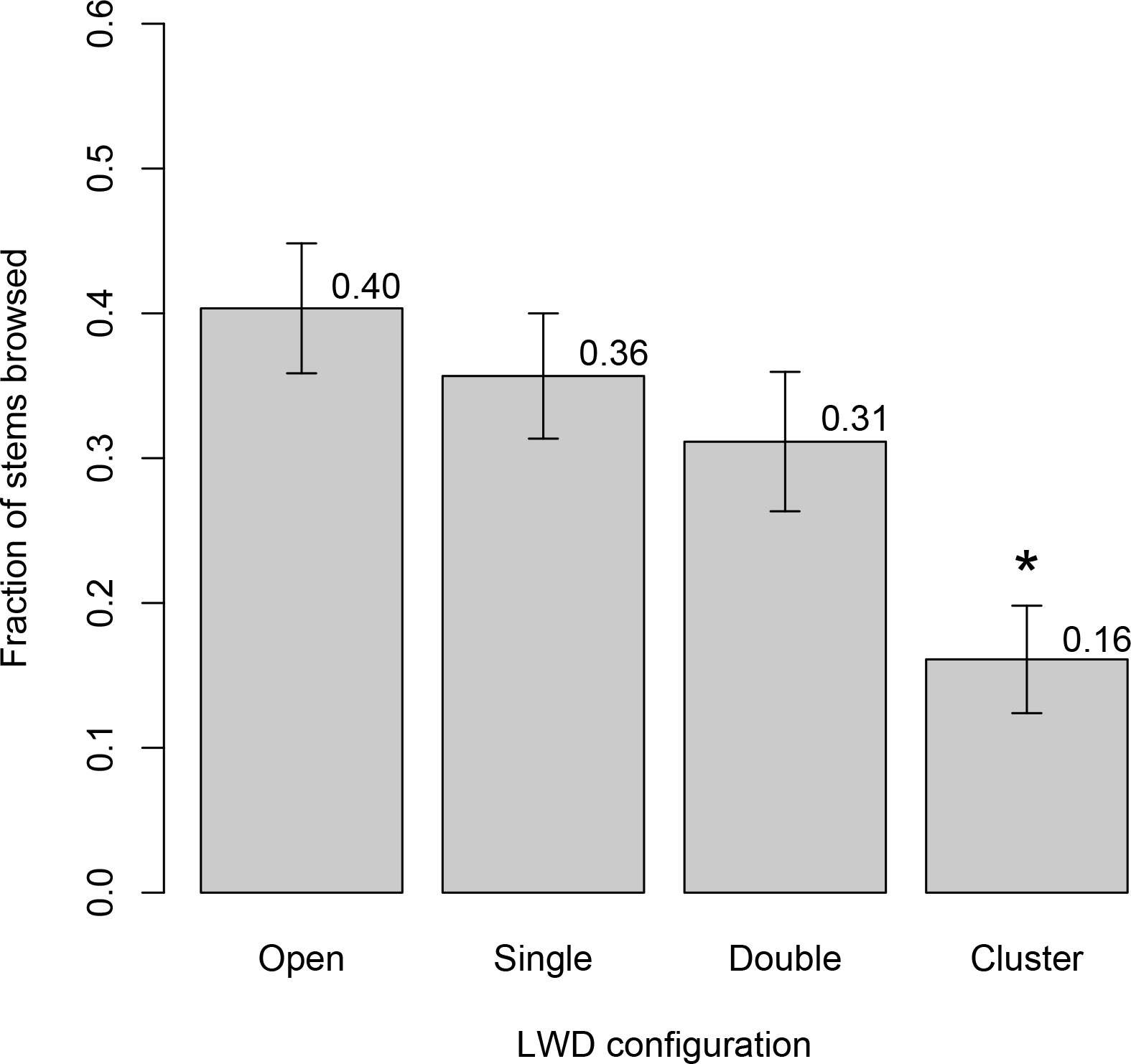
Figure 3 Browse intensity on woody plants growing in coarse sediments on the Geyser Valley floodplain. Labels on the horizontal axis refer to large wood characteristics associated with each of four plot types. “Open” plots were at least 10 meters from any LWD. “Single” plots were adjacent to one log. “Double” plots were aligned between two parallel logs. “Cluster” plots were fully surrounded by LWD. All woody debris used to determine plot types reached at least 50 cm above ground level. Values on the vertical axis are browse rates, expressed as fraction of stems browsed relative to all stems produced within the previous year. Bar heights are mean per-plot browse rates, and error bars are +/− one standard error. The asterisk (*) indicates mean browse rate in cluster plots was significantly lower than all other wood categories. Mean browse rate in other wood categories did not differ significantly from each other.
The mean browse intensity was greater in Mills than Geyser Valley (F2,260 = 701.7, p < 10−15), and differed among LWD configurations as described above (F3,260 = 30.4, p < 10−15). There was a small but significant interaction effect between site and LWD plot type (F3,260 = 2.675, p = 0.048), implying LWD-mediated browse reduction was greater in Mills than Geyser Valley.
On the Mills reservoir bed, reduction in browse intensity within wood clusters was disproportionately greater for species preferred by ungulates than for less preferred species, mostly conifers (species × LWD interaction term: F6,890 = 2.55, p = 0.0308; Figure 4). Reduction in browse intensity was intermediate for species with moderate selection. In Geyser Valley, differential reduction in browse intensity relative to ungulate preference could not be concluded with confidence. Analysis of browse intensity on plants with intermediate and low ungulate preference was equivocal (species × LWD interaction term: F1,376 = 0.020, p = 0.58; Figure 5). Plant species preferred by ungulates were almost entirely absent (0.2%) from Geyser Valley samples.
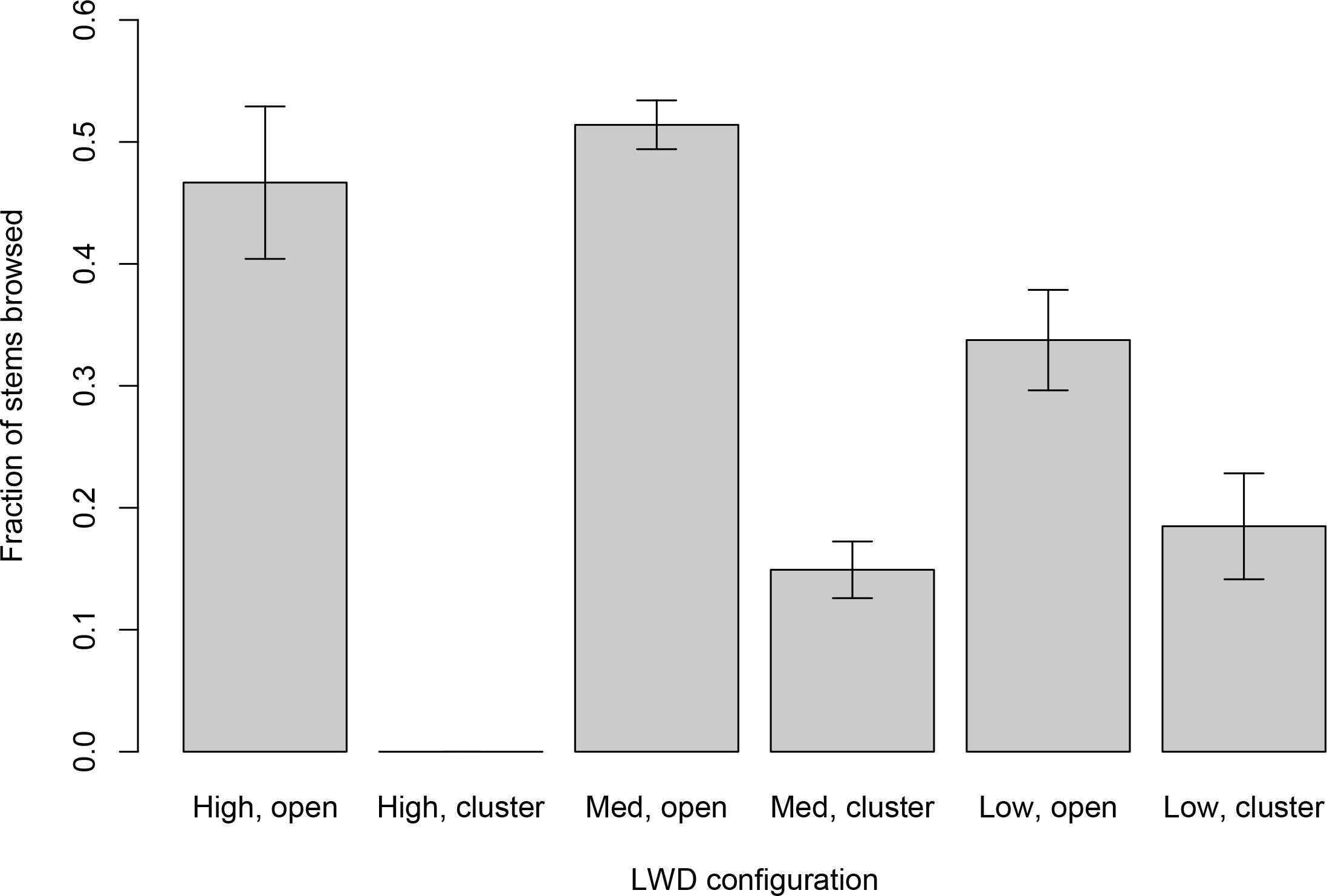
Figure 4 Browse intensity on Mills reservoir bed sorted by wood plot type and ungulates’ plant selection intensity. Labels on the horizontal axis refer to combinations of large wood characteristics and plant selection intensity. “Open” plots were at least 10 meters from any LWD. “Cluster” plots were fully surrounded by LWD. “High” intensity plants were species reported in McCaffery et al. (2020) as exceeding 66% of previous year’s stems browsed. “Med” were species reported in McCaffery et al. (2020) with browse values between 34% and 66%. “Low” were species reported in McCaffery et al. (2020) with less than 34% annual browse intensity. Values on the vertical axis are browse rates, expressed as fraction of stems browsed relative to all stems produced within the previous year. Bar heights are mean per-plant browse rates, and error bars are +/− one standard error.
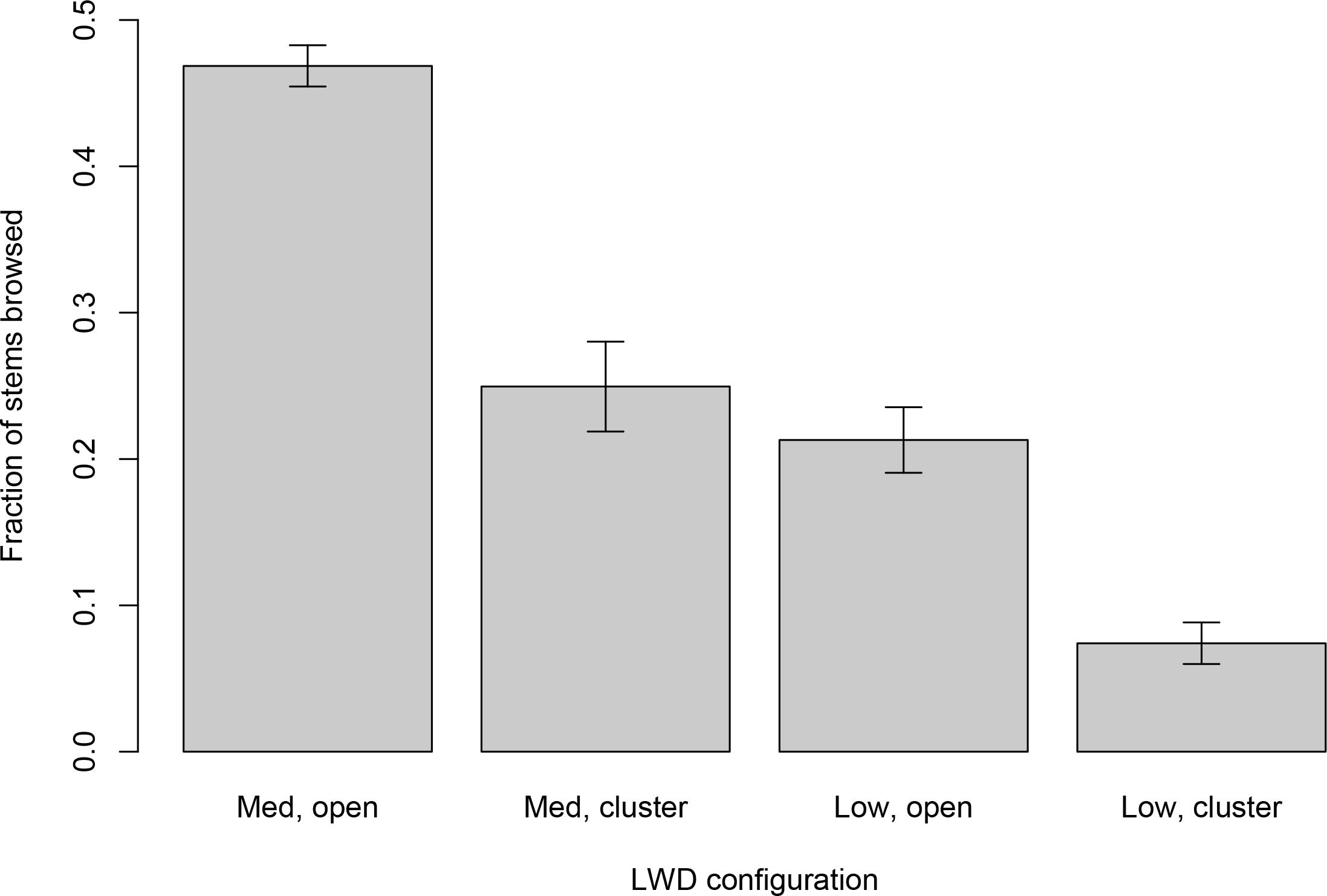
Figure 5 Browse intensity on the Geyser Valley floodplain, sorted by wood plot type and ungulates’ plant selection intensity. Labels on the horizontal axis refer to combinations of large wood characteristics and plant selection intensity. “Open” plots were at least 10 meters from any LWD. “Cluster” plots were fully surrounded by LWD. “High” intensity plants were species reported in McCaffery et al. (2020) as exceeding 66% of previous year’s stems browsed. “Med” were species reported in McCaffery et al. (2020) with browse values between 34% and 66%. “Low” were species reported in McCaffery et al. (2020) with less than 34% annual browse intensity. Values on the vertical axis are browse rates, expressed as fraction of stems browsed relative to all stems produced within the previous year. Bar heights are mean per-plant browse rates, and error bars are +/− one standard error.
5 Discussion
5.1 Key results
We found that large downed wood can substantially reduce browse intensity on young trees and shrubs, but only when wood surrounds the plants. Relative to plants without LWD protection, mean browse intensity on plants enclosed by wood was lower by a factor of 4 and 2.5 on the former Mills reservoir and Geyser Valley, respectively. Browse intensity was slightly but significantly lower where wood sheltered plants on two sides. Mean browse intensity was marginally lower adjacent to single logs, but not significantly different from areas without LWD. In areas with heavy browse pressure, wood clusters can facilitate woody plant growth to maturity instead of arrested growth forms (Keigley, 1997) that do not achieve restoration objectives (Figure 6).
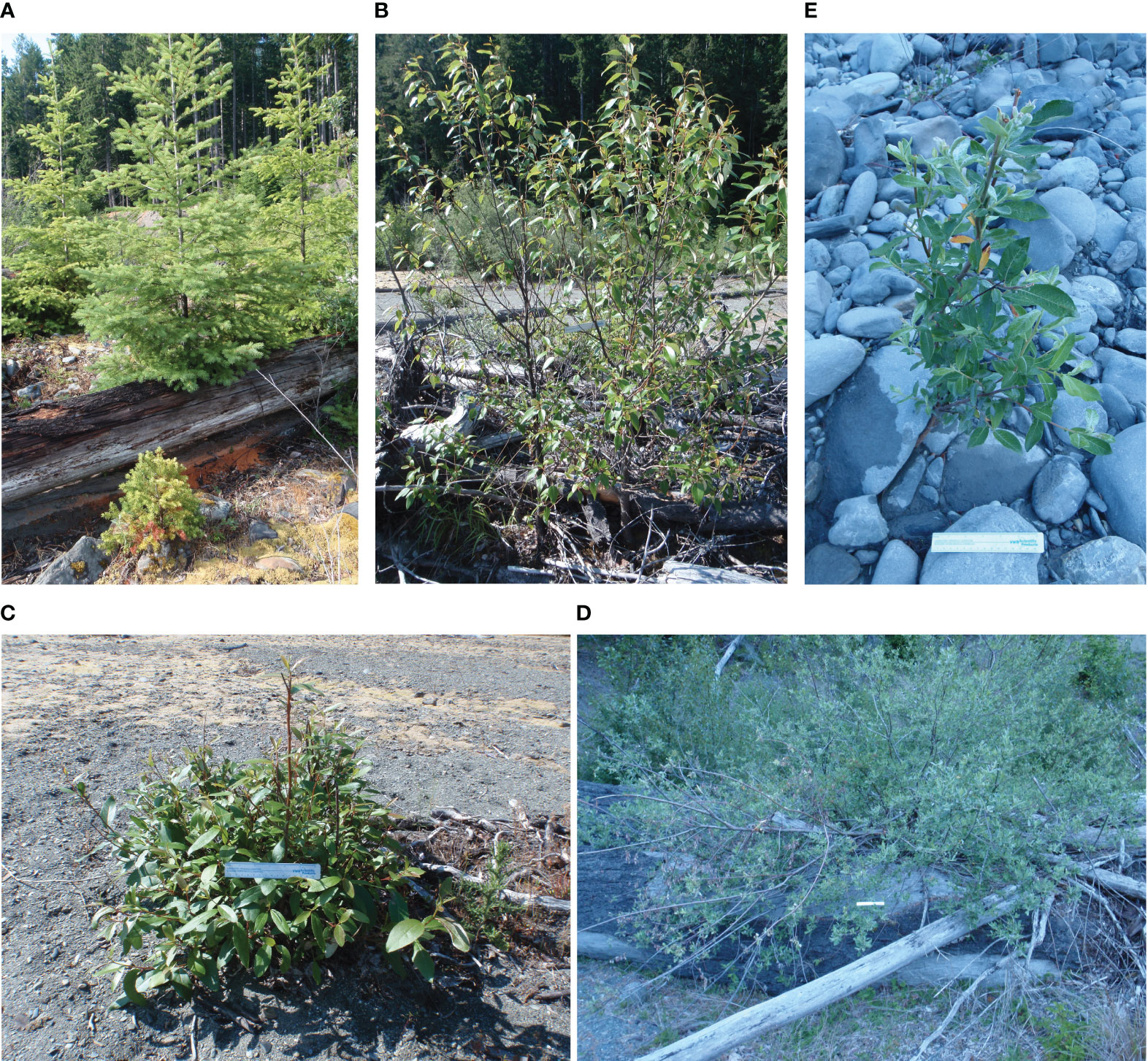
Figure 6 Growth forms of plants within large wood clusters vs. open sites. For each of the three species shown, plants within wood clusters grew rapidly to heights exceeding the reach of ungulate browsers. All apical meristems of plants in open sites were browsed, which impeded increases in plant height. (A) Douglas fir (Pseudotsuga menziesii): trees behind the log in the foreground are surrounded by large logs. The tree in the lower foreground is exposed to browsing on all sides except the log behind it. All trees in the photo had the same number of branch whorls, and likely germinated in the same year. Photo taken in Geyser Valley. (B) Black cottonwood (Populus balsamifera) saplings growing within a large wood cluster in Geyser Valley. The white ruler in the middle of the photo is 16 cm long. (C) Black cottonwood (Populus balsamifera) growing in sediment distant from large wood, in Geyser Valley, within 100 m of (B). The white ruler in the middle of the photo is 16 cm long. The arrested growth form indicates chronic browsing (Keigley, 1997). (D) Willows (Salix spp.) growing within a large wood cluster on the former Mills reservoir. The white ruler in the lower center of the photo is 16 cm long. (E) Sitka willow (Salix sitchensis) growing in open coarse sediments on the former Mills reservoir. The white ruler at the bottom of the photo is 16 cm long.
Wood-mediated browse reduction was similar in both study areas, but overall browse intensity was greater in Mills than Geyser Valley. This result contradicted our second hypothesis (Table 2) and results in McCaffery et al. (2020). These differences merit confirmation with further study. If correct, they suggest browsing is likely to slow plant growth throughout post-dam removal restoration, but browse intensity may decrease slightly as restoration proceeds. Conversely, these results suggest wood-mediated browse reduction may be greatest in the early years following dam removal, when rapid plant growth is most important. Strong reduction in browse within LWD clusters throughout the restoration process appears to be unequivocal.
Wood-mediated protection was greater for plant species preferred by ungulates. On the former Mills reservoir, the magnitude of browse reduction within wood clusters increased disproportionately with strength of ungulate plant selection. In Geyser Valley, this protection-preference interaction was not significant, but preferred species were almost entirely absent from our Geyser Valley samples. Low abundances of ungulate-preferred species in Geyser Valley were unlikely due to effects of elevation, climate, or plant species pools, which are similar to those in the Mills valley (Table 3). Future work could evaluate more plausible factors of Geyser Valley successional history, recent flooding (Acker et al., 2008; Brown et al., 2022), and heavy browse pressure during the century following wolf extirpation (Beschta and Ripple, 2008). Such work should consider that species preferred by ungulates were planted in Mills, but no seeding or planting occurred in Geyser Valley (Chenoweth et al., 2011; Chenoweth et al., 2021).
Our results are consistent with patterns reported from old-growth forests in Olympic National Park (Schreiner et al., 1996). They found large wood clusters provided refugia from ungulate browsing, enabling growth of trees and shrubs that influenced structural and functional characteristics of the forest. Our results demonstrate that large wood refugia also function during early seral stages and in the new context of large dam removal.
Browse reduction within large wood clusters in Elwha valleys supported riparian forest restoration comparable to mechanisms reported from other regions. These included logs in a montane conifer forest in Patagonia (Relva et al., 2009) and a subalpine forest in Poland (Milne-Rostkowska et al., 2020), windthrow following fire in a Swedish national park (de Chantal and Granstrom, 2007), fenced ungulate exclosures in northern California (Opperman and Merenlender, 2000) and northeast Oregon (Brookshire et al., 2002), felled trees in southeastern Oregon (Matney et al., 2005), and wolf reintroduction in Greater Yellowstone (Beschta and Ripple, 2008; Beschta and Ripple, 2019). In Biaowiea National Park, Poland, large wood magnified wolf-mediated browse reduction by impeding wolf detection and ungulate escape routes (Kuijper et al., 2013; van Ginkel et al., 2019). With each mechanism, browse reduction allowed trees and shrubs to increase in density, cover, and survival to maturity. Large wood clusters can support forest restoration where fencing would not be appropriate or where wolves and other apex predators are rare or absent.
5.2 Caveats
Our work was restricted to areas where plants grow at low to intermediate density, at heights within reach of ungulates. These areas occurred in both study areas on coarse sediments where moisture limitation reduced plant density and growth rates. On substrates where moisture was less limiting, including fine sediment deposits and stream edges, woody plants established dense stands that grew rapidly above the reach of ungulates. Our results do not apply to such stands, where herbivory was minor and where active revegetation was not needed (Prach et al., 2019; Chenoweth et al., 2021). Similarly, our results may not be as relevant to areas where apex predators limit ungulate browse intensity (Beschta and Ripple, 2010). Our study areas were within a national park with abundant large riparian wood. In regions with smaller or younger trees, wood clusters formed by simple log polygons may not provide adequate browse reduction. In those regions, stacking logs or root balls could provide functional ungulate barriers. Extensive wood in continuous alignments could be counterproductive, by providing cover and connectivity for small mammals (McCaffery et al., 2020) that girdle saplings (Keeton, 2008). Finally, we collected our data within five years of dam removal. A more comprehensive assessment of wood roles in revegetation will require monitoring over time scales comparable to forest maturation (e.g., Reed et al., 2021; Woodward et al., 2021).
5.3 Cross-disciplinary Insights
(1) Cross-disciplinary approaches can enhance forest restoration. Riparian forest restoration is influenced by geomorphic drivers, including reservoir drawdown schedules, river channel distributions and dynamics, and resultant sediment composition and distribution. Revegetation progress is constrained by climatic factors and plant physiology. Plant distributions and growth also are affected by wildlife (McLaughlin, 2013; McCaffery et al., 2020). These considerations led Elwha revegetation staff to adopt an adaptive approach to respond to uncertainties (Chenoweth et al., 2011). Although climatic uncertainty necessitates flexibility, restoration would be more effective if dam removal planning and associated restoration put greater emphasis on inclusive cross-disciplinary coordination. Reservoir drawdown could be scheduled to coincide with seed dispersal and germination requirements of riparian plants (Chenoweth et al., 2021). Log translocations to enhance plant survival could be configured to mitigate browse impacts and facilitate greater wildlife connectivity. Woody plant installation could give priority to locations within wood clusters. Revegetation and other restoration projects in future dam removals would benefit from cross-disciplinary approaches, which can leverage restoration resources to improve outcomes.
(2) Cross-disciplinary perspectives are required to understand large wood distributions, dynamics, and restoration roles. Ecological processes create large wood. Geomorphological processes determine large wood distribution and dynamics, wood influences on river structure and function, and wood-mediated distribution of water and sediment (Wohl, 2017). Fisheries science describes large wood as habitat structures providing eddies, cover, thermal refugia, and food essential to fish populations (Maser and Sedell, 1994; Roni et al., 2015). Integrating geomorphology and plant ecology is required to understand wood-mediated riparian vegetation establishment on LWD aggregations and sediment deposits (Fetherston et al., 1995; Pettit and Naiman, 2005; Montgomery and Abbe, 2006; Pettit and Naiman, 2006; Collins et al., 2012; Nakamura et al., 2012). Results in this paper and McCaffery et al. (2018) imply that restoration efficacy requires adding wildlife knowledge to geomorphology and plant ecology.
Effects of large wood often are underappreciated due to missing wood baselines. Anthropogenic removal of riparian wood and wood sources has reduced river wood quantities on most rivers by orders of magnitude (Wohl, 2014; Wohl, 2017). Nevertheless, large wood exerts strong influences on many rivers (Gurnell, 2013; Wohl, 2017) and can play important roles in restoration following dam removal. Large wood creates enduring structures (Hyatt and Naiman, 2001), which may pre-date dam construction on some rivers. These pre-dam structural legacies can catalyze restoration after dam removal by shaping local sediment distributions, providing fish habitat structures, and facilitating revegetation.
(3) Many non-human restoration partners function across disciplines. Rivers dredge sediment (Randle et al., 2015) in ways relevant to all restoration disciplines. Large wood affects and is affected by factors relevant to multiple disciplines, summarized above. Wildlife, including ungulate browsers and avian seed dispersers, respond to structures of concern to plant ecologists, attract study by wildlife ecologists, and ultimately influence vegetation with relevance to sediment stability and geomorphology. Restoration will be more effective when human efforts and resources consider and support non-human restoration partners.
5.4 Lessons learned and restoration applications
(1) Clusters of large downed wood can provide browse refugia that support woody plant growth to maturity. Wood clusters can reduce browse pressure where fencing or tree shelters would not be appropriate. Wood clusters can hasten woody plant growth by decades. Trees that escaped browsing by growing in dense stands in fine sediments on the Elwha’s former reservoirs exceeded ungulate browse height within five years. Trees exposed to chronic browsing in Geyser Valley remain suppressed in arrested growth forms after several decades. Browse protection is particularly important in coarse sediment deposits on drained reservoirs, where slower growth rates prolong exposure to ungulate browsers. By facilitating growth of forest islands (Figure 7), wood clusters can play a restoration role similar to nucleation sites used to catalyze tropical forest restoration (Corbin and Holl, 2012).
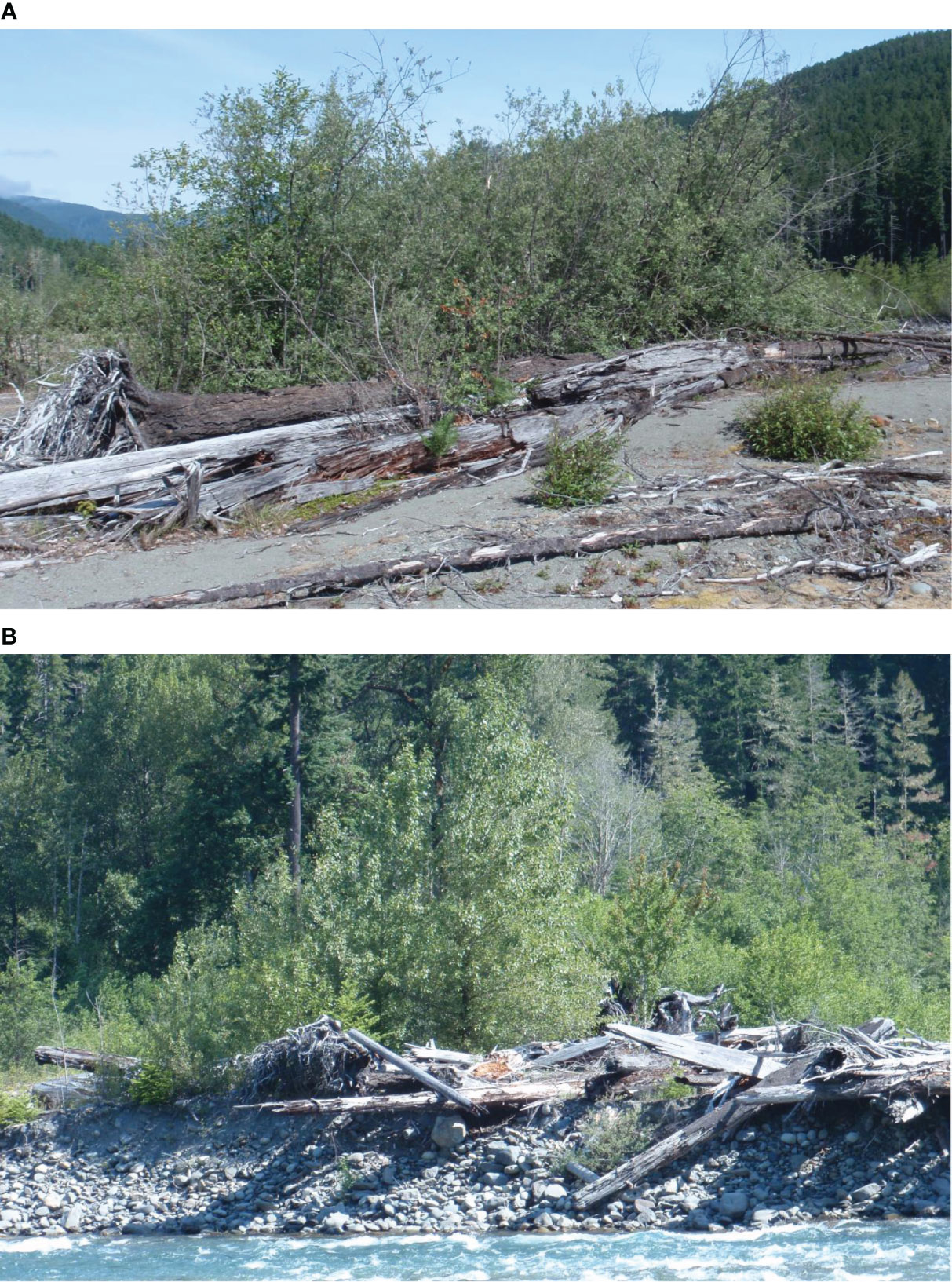
Figure 7 Young forest islands growing within clusters of large downed wood in Geyser Valley. (A) Forest island consisting of black cottonwood (Populus balsamifera), red alder (Alnus rubra), and willows (Salix spp.), surrounded by large logs in an expanse of sparsely vegetated coarse sediments. (B) Black cottonwood (Populus balsamifera) saplings growing within a log jam on the Elwha River active channel bank.
(2) Results reported here may apply to forest restoration contexts beyond dam removal. Limiting browse can support riparian forest growth to enhance habitat in fish restoration programs (Averett et al., 2017). Similarly, using LWD clusters to mitigate browse can hasten riparian forest restoration associated with Floodplains by Design projects (WDOE (Washington Department of Ecology), Bonneville Environmental Foundation, and American Rivers, 2023), levee setback initiatives (Gergel et al., 2002; Knox et al., 2022), and other riparian restoration programs (Horner et al., 2016; NWIFC (Northwest Indian Fisheries Commission), 2020). Large wood clusters also could facilitate forest restoration following wildfire, timber harvest, or conversion to cattle pasture (Holl et al., 2000; Vanha-Majamaa et al., 2007; Puerta-Piñero et al., 2010; Rost et al., 2010; but see Forester et al., 2007). Large wood and other perch structures can attract seed dispersers to restoration sites (Robinson and Handel, 2000; Corbin and Holl, 2012; McLaughlin, 2013; Guidetti et al., 2016). If that wood is clustered to reduce browse exposure, it can facilitate plant growth following seed germination. Although installation of wood clusters may be resource-intensive, they are likely to persist longer and require less maintenance than alternative interventions such as fencing (Brookshire et al., 2002; Wassie et al., 2009; Kota and Bartos, 2010; Woodward et al., 2021; Monks et al., 2023) or tree shelters (Stange and Shea, 1998; Keeton, 2008). These applications share a common theme: restoring LWD structures and functions can catalyze forest restoration in diverse systems.
(3) Revegetation planning should span entire plant lifecycles. The Elwha revegetation program accurately anticipated sediment moisture as a factor limiting plant establishment on the former reservoirs, and it implemented measures to mitigate seasonal drought (Chenoweth et al., 2021). Nevertheless, early revegetation success may not translate to long term objectives without interventions to support later life stages. Results from several riparian systems demonstrate that establishment success can stall or reverse without measures to mitigate herbivory (Opperman and Merenlender, 2000; Brookshire et al., 2002; Osei et al., 2015; Averett et al., 2017). If revegetation programs address plant needs throughout the life cycle (Table 1), they can produce mature stands that achieve long term outcomes. In systems with intense ungulate browse pressure, strategic placement of large wood clusters or planting within natural clusters would complement measures directed at earlier stages. Different approaches may be appropriate in other systems, but they should mitigate factors that limit plant growth in stages ranging from dispersal to maturation.
(4) Restoration monitoring should continue until objectives are met (Woodward et al., 2008). For revegetation, monitoring timeframes should extend to stand maturity. This timeframe should be reflected in allocation of funding and other resources, which may necessitate strategies to continue monitoring under budget restrictions after dam removal success is declared and interest shifts to other projects. Monitoring also should give priority to LWD abundances and distributions, including LWD clusters which rarely are given explicit attention (Gregory et al., 2017).
(5) For large dam removal and associated restoration, some important drivers are episodic and uncertain, such as federal funding or flood stage river flows. Other processes are regular and predictable, such as seed rain of wind-dispersed riparian trees or chronic ungulate browse pressure. Episodic processes determine the context, distribution, and time course for chronic predictable processes such as plant growth and browsing. Restoration programs should be poised for the former and plan for the latter.
(6) Strategic position or placement of multiple wood clusters can restore connections between rivers and terrestrial systems after dam removal. Just as individual wood clusters can facilitate growth of forest islands, series of clusters could generate forest archipelagos. If archipelagos are aligned to extend from the pre-dam forest to the active river channel, they can function as forest-to-river travel corridors for terrestrial wildlife. Many wildlife species perform important ecosystem functions, including dispersal of seeds and nutrients (Hobbs, 1996; McCaffery et al., 2018). At sites where girdling by small mammals causes high sapling mortality, LWD clusters should be discontinuous. Small mammal habitat connectivity would develop later as LWD-facilitated forest islands establish. For rivers with anadromous fishes, wood-catalyzed forest corridors can expedite restoration of positive feedback loops associated with marine-derived nutrient dispersal and rapid forest growth (Helfield and Naiman, 2001; McCaffery et al., 2018; Quinn et al., 2018). Individual wood clusters can reduce tree maturation time by decades. Similarly, strategic location of wood clusters in sequences potentially could advance restoration considerably in some contexts. More rapid achievement of restoration objectives may provide valuable mitigation of elevated extinction risks for aquatic species and accelerating impacts of climate change.
Data availability statement
The raw data supporting the conclusions of this article will be made available by the authors, without undue reservation.
Author contributions
CJ, CD, TM, and JM conceptualized the study. All authors coordinated the field planning. All authors participated in data collection and conducted the data analysis. CJ created Figure 1. JM wrote the manuscript. CJ, CD, TM, and JM edited and approved the submitted version.
Funding
The work was self-funded by the authors. Western Washington University loaned field sampling equipment at no charge. Olympic National Park granted a backcountry camping fee waiver as a condition of a scientific research permit.
Acknowledgments
We owe deep gratitude to the Lower Elwha Klallam Tribe for advocating, leading, and helping to implement Elwha restoration, and enduring its continuing impacts. Kim Sager-Fradkin, Rebecca McCaffery, Patti Happe, and Kurt Jenkins provided valuable discussion about Elwha ungulates and browsing in the Elwha system. Josh Chenoweth generously provided information about the Elwha revegetation program. Delaney Corcoran, Aurora Grant, and Kenjo Pollmann helped collect browse data with unwavering dedication. Ethan Duvall provided effective logistical support and thoughtful discussion. Jeff Duda helped arrange image panels in Figure 6. Thoughtful comments by Jeff Duda and two reviewers helped us improve the manuscript. Matthew Dubeau, Olympic National Park Research Coordinator, provided prompt and essential assistance with research permits. Scott Kinghorn, Olympic National Park Wilderness Information Center staff, graciously helped us obtain wilderness camping permits for surveys in Geyser Valley. We are indebted to a large diverse group of Elwha researchers for interdisciplinary communication and collaboration before, during, and after dam removals. The community of Elwha researchers gathered regularly to share their results and insights; we are grateful to those who have organized Elwha symposia over the last 15 years. In particular, Jeff Duda’s unflagging dedication has been instrumental. Support for Elwha symposia was provided by the Elwha Research Consortium, with funding from National Science Foundation grant #0443527.
Conflict of interest
The authors declare that the research was conducted in the absence of any commercial or financial relationships that could be construed as a potential conflict of interest.
Publisher’s note
All claims expressed in this article are solely those of the authors and do not necessarily represent those of their affiliated organizations, or those of the publisher, the editors and the reviewers. Any product that may be evaluated in this article, or claim that may be made by its manufacturer, is not guaranteed or endorsed by the publisher.
References
Abbe T. B., Montgomery D. R. (1996). Large woody debris jams, channel hydraulics and habitat formation in large rivers. Regulated. Rivers.: Res. Manage. 12, 201–221. doi: 10.1002/(SICI)1099-1646(199603)12:2/3<201::AID-RRR390>3.0.CO;2-A
Acker S. A., Beechie T. J., Shafroth P. B. (2008). Effects of a natural dam-break flood on geomorphology and vegetation on the Elwha River, Washington, USA. Northwest. Sci. 82 (Special Issue), 210–223. doi: 10.3955/0029-344X-82.S.I.210
Averett J. P., Endress B. A., Rowland M. M., Naylor B. J., Wisdom M. J. (2017). Wild ungulate herbivory suppresses deciduous woody plant establishment following salmonid stream restoration. For. Ecol. Manage. 391, 135–144. doi: 10.1016/j.foreco.2017.02.017
Beschta R. L., Ripple W. J. (2008). Wolves, trophic cascades, and rivers in the Olympic National Park, USA. Ecohydrology 1, 118–130. doi: 10.1002/eco.12
Beschta R. L., Ripple W. J. (2010). Recovering riparian plant communities with wolves in the northern Yellowstone, U.S.A. Restor. Ecol. 18, 380–389. doi: 10.1111/j.1526-100X.2008.00450.x
Beschta R. L., Ripple W. J. (2019). Can large carnivores change streams via a trophic cascade? Ecohydrology 12, c2048. doi: 10.1002/eco.2048
Brewitt P. (2019). Same river twice: the politics of dam removal and river restoration (Corvallis, OR: Oregon State University Press).
Brookshire E. N. J., Kauffman J. B., Lytjen D., Otting N. (2002). Cumulative effects of wild ungulate and livestock herbivory on riparian willows. Oecologia 132, 559–566. doi: 10.1007/s00442-002-1007-4
Brown R. L., Thomas C. C., Cubley E. S., Clausen A. J., Shafroth P. B. (2022). Does large dam removal restore downstream riparian vegetation diversity? Testing predictions on the Elwha River, Washington, USA. Ecol. Appl. 32, e2591. doi: 10.1002/eap.2591
Calimpong C. (2014). Elwha River revegetation 2013: A plant performance study. M.S. Thesis (Seattle: University of Washington).
Cavaliere E., Homann P. (2012). Elwha River sediments: phosphorus characterization and dynamics under diverse environmental conditions. Northwest. Sci. 86, 95–107. doi: 10.3955/046.086.0202
Chapron G., Kaczensky P., Linnell J. D. C., von Arx M., Huber D., Andrén H., et al. (2014). Recovery of large carnivores in Europe’s modern human-dominated landscapes. Science 346, 1517–1519. doi: 10.1126/science.1257553
Chenoweth J., Acker S. A., McHenry M. L. (2011). Revegetation and restoration plan for Lake Mills and Lake Aldwell (Olympic National Park and Lower Elwha Klallam Tribe). Available at: http://npshistory.com/publications/olym/elwha-river/reveg-plan-2011.pdf (Accessed 13 January 2023).
Chenoweth J., Bakker J. D., Acker S. A. (2021). Planting, seeding, and sediment impact restoration success following dam removal. Restor. Ecol. 30, e13506. doi: 10.1111/rec.13506
Collins B. D., Montgomery D. R., Fetherston K. L., Abbe T. B. (2012). The floodplain large wood cycle hypothesis: a mechanism for the physical and biotic structuring of temperate forested alluvial valleys in the North Pacific coastal ecoregion. Geomorphology 139-140, 460–470. doi: 10.1016/j.geomorph.2011.11.011
Cook K. L., Wallender W. W., Bledsoe C. S., Pasternack G., Upadhyaya S. K. (2009). Effects of native plant species, mycorrhizal inoculum, and mulch on restoration of reservoir sediment following dam removal, Elwha River, Olympic Peninsula, Washington. Restor. Ecol. 19, 251–260. doi: 10.1111/j.1526-100X.2009.00559.x
Corbin J. D., Holl K. D. (2012). Applied nucleation as a forest restoration strategy. For. Ecol. Manage. 265, 37–46. doi: 10.1016/j.foreco.2011.10.013
Cortese A. M., Bunn R. A. (2017). Availability and function of arbuscular mycorrhizal and ectomycorrhizal fungi during revegetation of dewatered reservoirs left after dam removal. Restor. Ecol. 25, 63–71. doi: 10.1111/rec.12406
Cubley E. S., Brown R. L. (2016). Restoration of hydrochory following dam removal on the Elwha River, Washington. River. Res. Appl. 32, 1566–1575. doi: 10.1002/rra.2999
de Chantal M., Granstrom A. (2007). Aggregations of dead wood after wildfire act as browsing refugia for seedlings of Populus tremula and Salix caprea. For. Ecol. Manage. 250, 3–8. doi: 10.1016/j.foreco.2007.03.035
Duda J. J., Freilich J. E., Schreiner. E. G. (2008). Baseline studies in the Elwha River ecosystem prior to dam removal: introduction to the special issue. Northwest. Sci. 82 (Special Issue), 1–12. doi: 10.3955/0029-344X-82.S.I.1
Duda J. J., Torgersen C. E., Brenkman S. J., Peters R. J., Sutton K. T., Connor H. A., et al. (2021). Reconnecting the Elwha: Spatial patterns of fish response to dam removal. Front. Ecol. Evol. 9. doi: 10.3389/fevo.2021.765488
Duda J. J., Wagner E. J., Wieferich D. J., Johnson R. C., Bellmore J. R. (2018) USGS Dam Removal Science Database v4.0 (ver. 4.0, June 2021): U.S. Geological Survey data release (Accessed 9 July 2023). doi: 10.5066/P9IGEC9G
Dudgeon D., Arthington A. H., Gessner M. O., Kawabata Z. I., Knowler D. J., Lévêque C., et al. (2006). Freshwater biodiversity: importance, threats, status and conservation challenges. Biol. Rev. 81, 163–182. doi: 10.1017/S1464793105006950
East A. E., Pess G. R., Bountry J. A., Magirl C. S., Ritchie A. C., Logan J. B., et al. (2015). Large-scale dam removal on the Elwha River, Washington, USA: River channel and floodplain geomorphic change. Geomorphology 228, 765–786. doi: 10.1016/j.geomorph.2014.08.028
Fetherston K. L., Naiman R. J., Bilby R. E. (1995). Large woody debris, physical process, and riparian forest development in montane river networks of the Pacific Northwest. Geomorphology 13, 133–144. doi: 10.1016/0169-555X(95)00033-2
Forester J. D., Anderson D. P., Turner M. G. (2007). Do high-density patches of coarse wood and regenerating saplings create browsing refugia for aspen (Populus tremuloides Michx.) in Yellowstone National Park (USA)? For. Ecol. Manage. 253, 211–219. doi: 10.1016/j.foreco.2007.07.019
Gergel S. E., Dixon M. D., Turner M. G. (2002). Consequences of human-altered floods: Levees, floods, and floodplain forests along the Wisconsin River. Ecol. Appl. 12, 1755–1770. doi: 10.2307/3099936
Graf W. L. (1999). Dam nation: A geographic census of American dams and their large-scale hydrologic impacts. Water Resour. Res. 35, 1305–1311. doi: 10.1029/1999WR900016
Gregory S. V., Gurnell A., Piégay H., Boyer K. (2017). “Dynamics of wood, ch. 29,” in Methods in stream ecology, volume 2: ecosystem function, 3rd ed. Eds. Lamberti G. A., Hauer F. R. (San Diego, CA: Academic Press). doi: 10.1016/B978-0-12-813047-6.00007-3
Guidetti B. Y., Amico G. C., Dardanelli S., Rodriguez-Cabal M. A. (2016). Artificial perches promote vegetation restoration. Plant Ecol. 217, 935–942. doi: 10.1007/s11258-016-0619-4
Gurnell A. M. (2013). “Wood in fluvial systems,” in Fluvial geomorphology. Ed. Wohl E. (San Diego, CA: Academic Press), 163–188.
Hawkins B. J., Jones M. D., Kranabetter J. M. (2015). Ectomycorrhizae and tree seedling nitrogen nutrition in forest restoration. New Forests. 46 (Special Issue), 747–771. doi: 10.1007/s11056-015-9488-2
Helfield J. M., Naiman R. J. (2001). Effects of salmon-derived nitrogen on riparian forest growth and implications for stream productivity. Ecology 82, 2403–2409. doi: 10.2307/2679924
Heneghan L., Miller S. P., Baer S., Callaham M. A., Montgomery J., Pavao-Zuckerman M., et al. (2008). Integrating soil ecological knowledge into restoration management. Restor. Ecol. 16, 608–617. doi: 10.1111/j.1526-100X.2008.00477.x
Hjältén J., Nilsson C., Jørgensen D., Bell D. (2016). Forest-stream links, anthropogenic stressors, and climate change: implications for restoration planning. BioScience 66, 646–654. doi: 10.1093/biosci/biw072
Hobbs N. T. (1996). Modification of ecosystems by ungulates. J. Wildlife. Manage. 60, 695–713. doi: 10.2307/3802368
Hoeksema J. D., Chaudhary V. B., Gehring C. A., Johnson N. C., Karst J., Koide R. T., et al. (2010). A meta-analysis of context-dependency in plant response to inoculation with mycorrhizal fungi. Ecol. Lett. 13, 394–407. doi: 10.1111/j.1461-0248.2009.01430.x
Holl K. D., Loik M. E., Lin E. H. V., Samuels I. A. (2000). Tropical montane forest restoration in Costa Rica: Overcoming barriers to dispersal and establishment. Restor. Ecol. 8, 339–349. doi: 10.1046/j.1526-100x.2000.80049.x
Horner G. J., Cunningham S. C., Thomson J. R., Baker P. J., MacNally R. (2016). Recruitment of a keystone tree species must concurrently manage flooding and browsing. J. Appl. Ecol. 53, 944–952. doi: 10.1111/1365-2664.12601
Hyatt T. L., Naiman R. J. (2001). The residence time of large woody debris in the Queets River, Washington, USA. Ecol. Appl. 11, 191–202. doi: 10.1890/1051-0761(2001)011[0191:TRTOLW]2.0.CO;2
Jakes A. F., Jones P. F., Paige L. C., Seidler R. G., Huijser M. P. (2018). A fence runs through it: A call for greater attention to the influence of fences on wildlife and ecosystems. Biol. Conserv. 227, 310–318. doi: 10.1016/j.biocon.2018.09.026
Jenkins K. J., Griffin P. C., Happe P. J., Reid M., Vales D. J., Moeller B., et al. (2015). “Elk monitoring in Mount Rainier and Olympic national parks: 2008-2011 synthesis report,” in Natural resource report NPS/NCCN/NRR—2015/904 (Fort Collins, CO: National Park Service).
Keeton W. S. (2008). Evaluation of tree seedling mortality and protective strategies in riparian forest restoration. Northern. J. Appl. Forestry. 25, 117–123. doi: 10.1093/njaf/25.3.117
Kim S., Toda Y., Tsujimoto T. (2014). Effects of a low-head dam removal on river morphology and riparian vegetation: a case study of Gongreung River. J. Water Resour. Prot. 6, 1682–1690. doi: 10.4236/jwarp.2014.618151
Kloehn K. K., Beechie T. J., Morley S. A., Coe H. J., Duda J. J. (2008). Influence of dams on river-floodplain dynamics in the Elwha River, Washington. Northwest. Sci. 82 (Special Issue), 224–235. doi: 10.3955/0029-344X-82.S.I.224
Knox R. L., Wohl E. E., Morrison R. R. (2022). Levees don’t protect, they disconnect: A critical review of how artificial levees impact floodplain functions. Sci. Total. Environ. 837, 155773. doi: 10.1016/j.scitotenv.2022.155773
Kota A. M., Bartos D. L. (2010). Evaluation of techniques to protect aspen suckers from ungulate browsing in the Black Hills. Western. J. Appl. Forestry. 25, 161–168. doi: 10.1093/wjaf/25.4.161
Kuijper D. P. J., de Kleine C., Churski M., van Hooft P., Budnicki J., Jędrzejewska B. (2013). Landscape of fear in Europe: wolves affect spatial patterns of ungulate browsing in Bialowieza Primeval Forest, Poland. Ecography 36, 1263–1275. doi: 10.1111/j.1600-0587.2013.00266.x
Leung V. (2019). Large woody debris and river morphology in scour pool formation, dam removal and delta formation. PhD. Dissertation (Seattle: University of Washington).
Lisius G. L., Snyder N. P., Collins M. J. (2018). Vegetation community response to hydrologic and geomorphic changes following dam removal. River. Res. Appl. 34, 317–327. doi: 10.1002/rra.3261
Lynch A. J., Cooke S. J., Arthington A. H., Baigun C., Bossenbroek L., Dickens C., et al. (2023). People need freshwater biodiversity. WIREs Water 10, e1633. doi: 10.1002/wat2.1633
Marangon D., Marchi N., Lingua E. (2022). Windthrown elements: a key point improving microsite amelioration and browsing protection to transplanted seedlings. For. Ecol. Manage. 508, e120050. doi: 10.1016/j.foreco.2022.120050
Maschinski J., Baggs J. E., Sacchi C. F. (2004). Seedling recruitment and survival of an endangered limestone endemic in its natural habitat and experimental reintroduction sites. Am. J. Bot. 91, 689–698. doi: 10.3732/ajb.91.5.689
Maser C., Sedell J. R. (1994). From the forest to the sea: the ecology of wood in streams, rivers, estuaries, and oceans (Delray Beach, FL: St. Lucie Press).
Matney C. A., Boyd C. S., Stringham T. K. (2005). Use of felled junipers to protect streamside willows from browsing. Rangeland. Ecol. Manage. 58, 652–655. doi: 10.2111/04-127R2.1
Mauer K. W. (2021). Unsettling resilience: colonial ecological violence, Indigenous futurisms, and the restoration of the Elwha River. Rural Sociol. 86, 611–634. doi: 10.1111/ruso.12365
McCaffery R., Jenkins K. J., Cendejas-Zarelli S., Happe P. J., Sager-Fradkin K. A. (2020). Small mammals and ungulates respond to and interact with revegetation processes following dam removal. Food Webs. 25, e00159. doi: 10.1016/j.fooweb.2020.e00159
McCaffery R., McLaughlin J., Sager-Fradkin K., Jenkins K. J. (2018). Terrestrial fauna are agents and endpoints in ecosystem restoration following dam removal. Ecol. Restor. 36, 97–107. doi: 10.3368/er.36.2.97
McHenry M. L., Chenoweth J. (2015). Revegetation of the former Mills reservoir on the Elwha River following dam removal: moving large wood by helicopter to assist revegetation efforts (Olympia, Washington, USA: EPA/Puget Sound Partnership and the Salmon Recovery Funding Board).
McLaughlin J. F. (2013). Engaging birds in vegetation restoration after Elwha dam removal. Ecol. Restor. 31, 46–56. doi: 10.3368/er.31.1.46
Michel J. T., Helfield J. M., Hooper D. U. (2011). Seed rain and revegetation of exposed substrates following dam removal on the Elwha River. Northwest Sci. 85, 15–29. doi: 10.3955/046.085.0102
Milne-Rostkowska F., Holeksa J., Bogdziewicz M., Piechnik Ł., Seget B., Kurek P., et al. (2020). Where can palatable young trees escape herbivore pressure in a protected forest? For. Ecol. Manage. 472, 118221. doi: 10.1016/j.foreco.2020.118221
Monks L., Yen J., Dillon R., Standish R., Coates D., Byrne M., et al. (2023). Herbivore exclusion and water availability improve success across 76 translocations of 50 threatened plant species in a biodiversity hotspot with a Mediterranean climate. Plant Ecol. doi: 10.1007/s11258-023-01313-5
Montgomery D. R., Abbe T. B. (2006). Influence of logjam-formed hard points on the formation of valley-bottom landforms in an old-growth forest valley, Queets River, Washington, USA. Quaternary. Res. 65 (1), 147–155. doi: 10.1016/j.yqres.2005.10.003
Muldavin E. H., Milford E. R., Umbreit N. E., Chauvin Y. D. (2017). Long-term outcomes of natural-process riparian restoration on a regulated river site: The Rio Grande Albuquerque Overbank Project after 16 years. Ecol. Restor. 35, 341–353. doi: 10.3368/er.35.4.341
Nakamura F., Fuke N., Kubo M. (2012). Contributions of large wood to the initial establishment and diversity of riparian vegetation in a bar-braided temperate river. Plant Ecol. 213, 735–747. doi: 10.1007/s11258-012-0037-1
Nathan R., Muller-Landau H. C. (2000). Spatial patterns of seed dispersal, their determinants and consequences for recruitment. Trends Ecol. Evol. 15, 278–285. doi: 10.1016/S0169-5347(00)01874-7
National Park Service (NPS). (1996). Elwha river ecosystem restoration implementation: final environmental impact statement. U.S. National park service (Denver, CO: Denver Service Center).
Neilly H., Cale P. (2020). “Branching” with complex coarse woody debris reduces herbivory on recovering erosion scalds. Ecol. Manage. Restor. 21, 143–146. doi: 10.1111/emr.12409
NWIFC (Northwest Indian Fisheries Commission). (2020). 2020 state of our watersheds: A report by the treaty tribes in western washington (Olympia, Washington, USA: NWIFC). Available at: https://nwifc.org/publications/state-of-our-watersheds/ (Accessed 2 July 2023).
O’Connor J. E., Duda J. J., Grant G. E. (2015). 1000 dams down and counting. Science 348, 496–497. doi: 10.1126/science.aaa9204
Opperman J. J., Merenlender A. M. (2000). Deer herbivory as an ecological constraint to restoration of degraded riparian corridors. Restor. Ecol. 8, 41–47. doi: 10.1046/j.1526-100x.2000.80006.x
Orr C. H., Koenig S. (2006). Planting and vegetation recovery on exposed mud flats following two dam removals in Wisconsin. Ecol. Restor. 24, 79–86. doi: 10.3368/er.24.2.79
Orr C. H., Stanley E. H. (2006). Vegetation development and restoration potential of drained reservoirs following dam removal in Wisconsin. River. Res. Appl. 22, 281–295. doi: 10.1002/rra.891
Osei N. A., Gurnell A. M., Harvey G. L. (2015). The role of large wood in retaining fine sediment, organic matter and plant propagules in a small, single-thread forest river. Geomorphology 235, 77–87. doi: 10.1016/j.geomorph.2015.01.031
Peinetti H. P., Menezes R. S. C., Coughenour M. B. (2001). Changes induced by elk browsing in the aboveground biomass production and distribution of willow (Salix monticola Bebb): their relationships with plant water, carbon, and nitrogen dynamics. Oecologia 2001, 334–342. doi: 10.1007/s004420000593
Pettit N. E., Naiman R. J. (2005). Flood-deposited wood debris and its contribution to heterogeneity and regeneration in a semi-arid riparian landscape. Oecologia 145, 434–444. doi: 10.1007/s00442-005-0143-z
Pettit N. E., Naiman R. J. (2006). Flood-deposited wood creates regeneration niches for riparian vegetation on a semi-arid South African river. J. Vegetation. Sci. 17, 615–624. doi: 10.1658/1100-9233(2006)17[615:FWCRNF]2.0.CO;2
Prach K., Chenoweth J., del Moral R. (2019). Spontaneous and assisted restoration of vegetation on the bottom of a former water reservoir, the Elwha River, Olympic National Park,WA, U.S.A. Restor. Ecol. 27, 592–599. doi: 10.1111/rec.12915
Puerta-Piñero C., Sánchez-Miranda A., Leverkus A., Castro J. (2010). Management of burnt wood after fire affects post-dispersal acorn predation. For. Ecol. Manage. 260, 345–352. doi: 10.1016/j.foreco.2010.04.023
Quinn T. P., Helfield J. M., Austin C. S., Hovel R. A., Bunn A. G. (2018). A multidecade experiment shows that fertilization by salmon carcasses enhanced tree growth in the riparian zone. Ecology 99, 2433–2441. doi: 10.1002/ecy.2453
Randle T. J., Bountry J. A., Ritchie A., Wille K. (2015). Large-scale dam removal on the Elwha River, Washington, USA: erosion of reservoir sediment. Geomorphology 246, 709–728. doi: 10.1016/j.geomorph.2014.12.045
R Core Team. (2023). R: A language and environment for statistical computing (Vienna, Austria: R Foundation for Statistical Computing). Available at: https://www.R-project.org/.
Reed S. P., Royo A. A., Fotis A. T., Knight K. S., Flower C. E., Curtis P. S. (2021). The long-term impacts of deer herbivory in determining temperate forest stand and canopy structural complexity. J. Appl. Ecol. 59, 812–821. doi: 10.1111/1365-2664.14095
Reid A. J., Carlson A. K., Creed I. F., Eliason E. J., Gell P. A., Johnson P. T., et al. (2019). Emerging threats and persistent conservation challenges for freshwater biodiversity. Biol. Rev. 94, 849–873. doi: 10.1111/brv.12480
Relva M. A., Westerholm C. L., Kitzberger T. (2009). Effects of introduced ungulates on forest understory communities in northern Patagonia are modified by timing and severity of stand mortality. Plant Ecol. 201, 11–22. doi: 10.1007/s11258-008-9528-5
Ritchie A. C., Warrick J. A., East A. E., Magirl C. S., Stevens A. W., Bountry J. A., et al. (2018). Morphodynamic evolution following sediment release from the world’s largest dam removal. Sci. Rep. 8, 13279. doi: 10.1038/s41598-018-30817-8
Robinson G. R., Handel S. N. (2000). Directing spatial patterns of recruitment during an experimental urban woodland reclamation. Ecol. Appl. 10, 174–188. doi: 10.1890/1051-0761(2000)010[0174:DSPORD]2.0.CO;2
Roni P., Beechie T., Pess G., Hanson K. (2015). Wood placement in river restoration: fact, fiction, and future direction. Can. J. Fisheries. Aquat. Sci. 72, 466–478. doi: 10.1139/cjfas-2014-0344
Rooney T. P. (1995). Restoring landscape diversity and old-growth to Pennsylvania northern hardwood forests. Natural Areas. J. 15, 274–278.
Rost J., Pons P., Bas J. M. (2010). Building wood debris piles benefits avian seed dispersers in burned and logged Mediterranean pine forests. For. Ecol. Manage. 260, 79–86. doi: 10.1016/j.foreco.2010.04.003
Schmitz D., Blank M., Ammondt S., Patten D. T. (2009). Using historic aerial photography and paleohydrologic techniques to assess long-term ecological response to two Montana dam removals. J. Environ. Manage. 90 (Suppl.3), S237–S248. doi: 10.1016/j.jenvman.2008.07.028
Schreiner E. G., Krueger K. A., Houston D. B., Happe P. J. (1996). Understory patch dynamics and ungulate herbivory in old-growth forests of Olympic National Park, Washington. Can. J. For. Res. 26, 255–265. doi: 10.1139/x26-029
Schuster J. L. (2015). Vegetation colonization within exposed reservoirs following dam removal on the Elwha River, Washington (Cheney (WA: Eastern Washington University). Available at: https://dc.ewu.edu/theses/310/.
Shafroth P. B., Friedman J. M., Auble G. T., Scott M. L., Braatne J. H. (2002). Potential responses of riparian vegetation to dam removal. BioScience 52, 703–712. doi: 10.1641/0006-3568(2002)052[0703:PRORVT]2.0.CO;2
Shafroth P. B., Perry L. G., Rose C. A., Braatne J. H. (2016). Effects of dams and geomorphic context on riparian forests of the Elwha River, Washington. Ecosphere 7, e01621. doi: 10.1002/ecs2.1621
Stange E. E., Shea K. L. (1998). Effect of deer browsing, fabric mats, and tree shelters on Quercus rubra seedlings. Restor. Ecol. 6, 29–34. doi: 10.1046/j.1526-100x.1998.00614.x
Trejo A., Moran C., Baxter J. (2019). Habitat selection of the Spotted Sandpiper (Actitus macularius) along the Elwha River, Washington. Unpublished report, Western Washington University.
van Ginkel H. A. L., Kuijper D. P. J., Schotanus J., Smit C. (2019). Wolves and tree logs: Landscape-scale and fine-scale risk factors interactively influence tree regeneration. Ecosystems 22, 202–212. doi: 10.1007/s10021-018-0263-z
Vanha-Majamaa I., Lilja S., Ryömä R., Kotiaho J. S., Laaka-Lindberg S., Lindberg H., et al. (2007). Rehabilitating boreal forest structure and species composition in Finland through logging, dead wood creation and fire: The EVO experiment. For. Ecol. Manage. 250, 77–88. doi: 10.1016/j.foreco.2007.03.012
Wang B. C., Smith T. B. (2002). Closing the seed dispersal loop. Trends Ecol. Evol. 17, 379–385. doi: 10.1016/S0169-5347(02)02541-7
Warrick J. A., Draut A. E., McHenry M. L., Miller I. M., Magirl C. S., Beirne M. M., et al. (2011). “Geomorphology of the Elwha River and its delta,” in Coastal habitats of the Elwha River, Washington–biological and physical patterns and processes prior to dam removal, vol. 2011–5120. (U.S. Geological Survey, Reston, Virginia, USA: USGS Inv. Rep), 47–74. Available at: https://pubs.usgs.gov/sir/2011/5120/pdf/sir20115120_ch3.pdf.
Wassie A., Sterck F. J., Teketay D., Bongers F. (2009). Effects of livestock exclusion on tree regeneration in church forests of Ethiopia. For. Ecol. Manage. 257, 765–772. doi: 10.1016/j.foreco.2008.07.032
WDOE (Washington Department of Ecology), Bonneville Environmental Foundation, and American Rivers. (2023). Floodplains by design (Olympia, WA). Available at: https://floodplainsbydesign.org/ (Accessed 2 July 2023).
Whyte H. D., Lusk C. H. (2019). Woody debris in treefall gaps shelters palatable plant species from deer browsing, in an old-growth temperate forest. For. Ecol. Manage. 448, 198–207. doi: 10.1016/j.foreco.2019.06.010
Wohl E. (2014). A legacy of absence: Wood removal in US rivers. Prog. Phys. Geogr. 38, 637–663. doi: 10.1177/0309133314548091
Wohl E. (2017). Bridging the gaps: An overview of wood across time and space in diverse rivers. Geomorphology 279, 3–26. doi: 10.1016/j.geomorph.2016.04.014
Wohl E., Kramer N., Ruiz-Villanueva V., Scott D. N., Comiti F., Gurnell A. M., et al. (2019). The natural wood regime in rivers. BioScience 69, 259–273. doi: 10.1093/biosci/biz013
Woodward A., Jenkins K. J., Harmon M. E. (2021). Plant community succession following ungulate exclusion in a temperate rainforest. Ecosphere 12, 03889. doi: 10.1002/ecs2.3889
Woodward A., Schreiner E. G., Crain P., Brenkman S. J., Happe P. J., Acker S. A., et al. (2008). Conceptual models for research and monitoring of Elwha dam removal-management perspective. Northwest. Sci. 82 (Special Issue), 59–71. doi: 10.3955/0029-344X-82.S.I.59
Woodward A., Schreiner E. G., Houston D. B. (1994). Ungulate forest relationships in Olympic National Park: retrospective exclosure studies. Northwest. Sci. 68, 97–110.
World Commission on Dams. (2000). Dams and development (London, G.B. and Sterling, VA, USA: Earthscan Publications Ltd). Available at: http://www.dams.org/report/ (Accessed 3 March 2008).
Xu W. J., Dejid N., Herrmann V., Sawyer H., Middleton A. D. (2021). Barrier Behaviour Analysis (BaBA) reveals extensive effects of fencing on wide-ranging ungulates. J. Appl. Ecol. 58, 690–698. doi: 10.1111/1365-2664.13806
Keywords: forest restoration, LWD, dam removal, plant growth, forest–river connection, deer, elk
Citation: Johnson C, Douglas C, Mansmith T and McLaughlin J (2023) Large wood supports Elwha revegetation by reducing ungulate browsing. Front. Ecol. Evol. 11:1215144. doi: 10.3389/fevo.2023.1215144
Received: 01 May 2023; Accepted: 10 August 2023;
Published: 30 August 2023.
Edited by:
Jeffrey J. Duda, US Geological Survey, United StatesReviewed by:
Rebecca Brown, Eastern Washington University, United StatesRoger Del Moral, University of Washington, United States
Copyright © 2023 Johnson, Douglas, Mansmith and McLaughlin. This is an open-access article distributed under the terms of the Creative Commons Attribution License (CC BY). The use, distribution or reproduction in other forums is permitted, provided the original author(s) and the copyright owner(s) are credited and that the original publication in this journal is cited, in accordance with accepted academic practice. No use, distribution or reproduction is permitted which does not comply with these terms.
*Correspondence: John McLaughlin, am1jbEB3d3UuZWR1
†These authors have contributed equally to this work and share first authorship