- Department of Animal Behaviour, Bielefeld University, Bielefeld, Germany
Physiological costs from parasites arise from host colonization and defence activation and can vary according to the interactions of host and parasite traits and states. Parasite-induced costs crucially differ between stages of infection, but this is difficult to assess in wild vertebrates. To evaluate the effects of blood parasite infection in juvenile birds, we compared physiological measures of common buzzard nestlings (Buteo buteo) between stages of infection with Leucocytozoon toddi, a Plasmodium-like pathogen. We related proxies of infection damage to experimentally manipulated infection intensity. We expected infection costs to be higher for the hosts at the onset of infection and during peak parasitemia than hosts with decreasing parasitemia and uninfected ones. We found body condition to be initially negatively correlated to infection intensity, but this relationship disappeared by the late stages of infection. Furthermore, there was no difference in growth rate and other physiological measures among infection stages. This indicates negligible costs of parasitism and transient virulence of Leucocytozoon in the nestling stage of the host. To diminish infection-driven mortality, juveniles may evolve to be particularly parasite-tolerant, further enhancing parasite transmission in the population. Our results demonstrate the necessity of including infection courses rather than point estimates in models of fitness costs of infection.
Background
Parasites are defined by the exploitation of foreign resources, resulting in negative short- and long-term fitness effects on hosts (Bermejo et al., 2006; Foley et al., 2011; Stockdale et al., 2015; Simonsen and Viboud, 2021). Parasite infections often directly affect host survival and reproduction through diverse lethal and sub-lethal effects on host physiology, behaviour and ecology (Poulin, 1998; Knowles et al., 2010; la Puente et al.,2010; Dunn et al., 2013). They can also exert indirect effects by exacerbating the adverse impacts of the abiotic environment (Brown and Pascoe, 1989; Martinez and Merino, 2011), or influencing intra- or interspecific interactions (Hatcher et al., 2006), and other symbionts (Ramsay and Rohr, 2021). Such combined detrimental effects may impose particularly high fitness costs on juvenile animals (Panter and Jones, 2002; Kubi et al., 2006; Cowman et al., 2015; Ashby and Bruns, 2018). During the early stages of host development, adaptive immunity has not fully matured yet. This may reduce the risk of pervasive autoimmunity but leaves juveniles more susceptible to intense infections than adults (Herman et al., 1975; World Health Organization, 2016; Townsend et al., 2018). Additionally, young hosts often rely on parental care and are less mobile (Grew et al., 2019); which makes them particularly accessible to vectors. This provides a particularly suitable ecological niche for parasites, whose probabilities of transmission and successful establishment in hosts are enhanced under these conditions. Correspondingly, some infections are particularly common and intense during “childhood” (Simon et al., 2015; Ashby and Bruns, 2018). Early life host-parasite interactions can, therefore, have important consequences for host survival and parasite transmission. On the other hand, because severe fitness costs to juveniles in pre-reproductive age would lead to the dying out of susceptible host demes, a weaker juvenile immunity combined with high infection probabilities may also force parasites to become less virulent and evolve toward benign forms of symbiosis approaching commensalism. However, such costs in developmental stages remain poorly understood in the wild, mainly due to the hardships of longitudinal samplings in wild juveniles.
Regardless of the severity of parasitic damages, parasitemia (i.e. the proportion of infected host tissue, used interchangeably with infection intensity) usually (1) first increases following host invasion, (2) reaches a plateau/peak and then (3) decreases rapidly (Figure 1) (Valkiūnas, 2005; Valkiūnas and Atkinson, 2020). When the adaptive immunity succeeds in mounting a specific response, parasitemia decreases and (4) stabilizes at low chronic levels, usually followed in the long term by relapses or effective clearance (Figure 1) (Snounou et al., 1989; Valkiūnas, 2005; Valkiūnas and Atkinson, 2020). Further on, we term these time periods of individual infection progressions “infection stages” (in contrast to tissue-specific parasite developmental stages). The physiological costs of infection, tissue damage, parasite-driven resource depletion and inflammation, are expected to be immediate, i.e. most pronounced during the pre-patent and acute stages of infection - shortly before and during peak parasitemia -, rather than during chronic infection when parasitemia is low and gradual recovery ensues (Hayworth et al., 1987; Williams, 2005; Valkiūnas and Iezhova, 2017). However, an open question remains whether the physiological costs of the infection are short-lived and immediate, delayed and associated with recovery, or a combination of both.
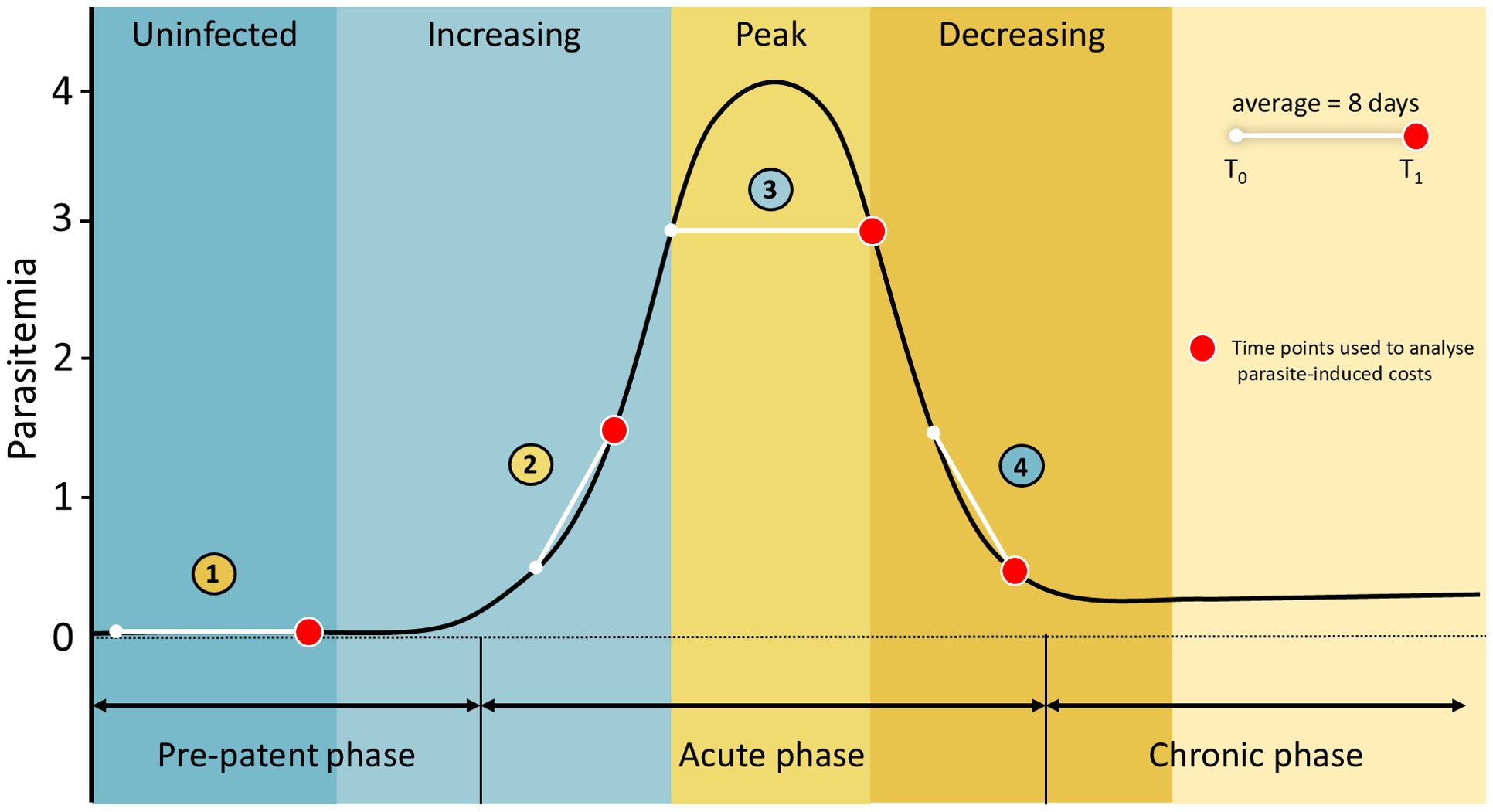
Figure 1. Theoretical dynamics of parasitemia in a host. The y-axis is parasitemia score. The x-axis represents time with the different phases of parasitemia. The corresponding infection stages at resampling (1 = uninfected, 2 = increasing, 3 = peak and 4 = decreasing) are shown as vertical colour bands. The top right diagram shows the average interval between first (T0) and second (T1) sampling. The red points represent the time points used in models testing for costs of infection on physiological parameters.
Vertebrate haemosporidian parasites, one of the parasites that mostly follow the model of infection progression outlined above is mostly known because of several group members, which are significant disease and mortality agents. Five species of Plasmodium cause substantial pathology and mortality in humans, particularly in children. Such plasmodium-induced host damages have also been recorded in birds, both in captivity and in the wild (Hayworth et al., 1987; Williams, 2005; Atkinson et al., 2008). The sister clades Haemoproteus and Leucocytozoon have been suggested to play similar roles in wildlife (Valkiūnas, 2005; Martinez and Merino, 2011). Parasitic cells, merozoites released into the blood invade different types of blood cells (Valkiūnas, 2005; Zhao et al., 2015) and blood parasitemia increases until it reaches peak infection, which can last several days. While it has been shown that these parasites can cause tissue and organ dysfunction in hosts, the frequency and severity of these problems among wild hosts, as well as factors which modulate them in nature, are still unknown (Valkiūnas, 2005; Valkiūnas and Iezhova, 2017).
In this study, we performed a field experiment to examine the effects of blood parasite infection and used physiological measures as informative proxies of costs of infection in nestlings of wild common buzzards, Buteo. Common buzzards are long-lived (up to 29 years in the wild) and the most common accipitriform in Europe. They have the highest prevalence of blood parasites of the genus Leucocytozoon among sympatric raptor species (Wiegmann et al., 2021). In our study population, the prevalence of Leucocytozoon toddi before the fledging of nestlings surpasses 50% (Chakarov et al., 2017; Wiegmann et al., 2021). We recorded and manipulated the levels of parasitemia at two-time points to evaluate how they affect physiological states and development and thus to reveal potential immediate infection- and immunity-related costs to hosts. We predicted immediate parasitic costs in nestlings, i.e. higher infection costs during challenging infection stages (increasing and peak) compared with nestlings with decreasing parasitemia and uninfected ones. Additionally, we expected that the parasitic costs will positively correlate with host infection intensity, as the number of infected blood cells increases.
Methods
(a) Host-parasite system
The study was performed in a 300-km² study area in North Rhine-Westphalia, Germany (8°25’ E and 52°06’ N) as part of a long-term monitoring study that started in 1989. In this study area, breeding and reproductive success of Common buzzards (Buteo buteo) as well as their blood parasite prevalence have been yearly recorded. Common buzzards are accipitriform birds of prey that breed in temperate Eurasian forests, in tree nests at heights between 10 to 30 m. Leucocytozoon toddi (Eukaryota, Protista, Haemosporidiae, lineages MILANS04, MILVUS01, BUTBUT03) is by far the most common blood parasite in our study population of common buzzard (Chakarov, unpublished). The vector-borne transmission of Leucocytozoon has been suggested to mostly occur in a quasi-vertical direction (Chakarov et al., 2015). Black fly vectors (Simuliidae) may first suck blood from infected parents at the nest site and predominantly transmit the same genetic pool of parasites to their offspring (Chakarov et al., 2015). Prevalence of infection varies substantially across years, with a minimum of 13.6% in 2014, a maximum of 68.2% in 2020 and a mean of 44.2% from our 2005-2020 dataset.
(b) Data collection
From 2016 to 2020, 276 common buzzard nestlings (n = 32, 65, 71, 108 in 2016, 2018, 2019, 2020 respectively) were sampled. All individuals were resampled on average eight days after first sampling (T0 thereafter, mean ± s.d. = 8.36 ± 4.21 days, Figure 1). At both sampling points, nestling age was estimated using a sex-specific polynomial regression between age and wing length, based on growth data for buzzards of known age (Bijlsma, 1998). The average estimated age of nestlings at T0 was 19.47 days (± s.d. 5.01) and 27.84 days (± s.d. 5.36) at second sampling (T1 thereafter), an age (T0) where most nestlings have already encountered Leucocytozoon and hence infections are microscopically visible (Chakarov et al., 2015). Leucocytozoon toddi was identified in the blood samples by screening with PCR using specific primers. Blood smears were also screened by microscopy for Leucocytozoon toddi infection at T0 and T1. Approximately 10.000 erythrocytes were scanned in thin blood smears and parasitemia at T0 and T1 was categorized as follows: not infected (no detectable parasites), parasitemia 1 (1–10 parasites per 10.000 erythrocytes), parasitemia 2 (>10–100 parasites per 10.000 erythrocytes); parasitemia 3 (>100–1000 parasites per 10.000 erythrocytes) and parasitemia 4 (>1000 parasites per 10.000 erythrocytes). Categorization of parasitemia allowed us to test for potential non-linear relationships between parasitemia and physiological parameters. Among the sampled nestlings, 66% (N=183) were infected during at least one of the two time points, whereas 34% (N=93) were not infected at neither time points; only 23 nestlings naturally displayed decreasing parasitemia between both sampling points. An artificial treatment aiming at mimicking decreasing parasitemia was achieved by giving 11 mg of antimalarial medicine (Malarone™; Atovaquone and Proguanil Hydrochloride, GlaxoSmithKline, UK, range: 7-21 mg/kg) diluted in 0.5mL of distilled water to a subset of sampled nestlings in 2018 (n = 14), 2019 (n = 41) and 2020 (n = 36). Within-brood randomization of treatments was performed to avoid nest effect in the downstream analyses. This uniform dose was non-toxic but higher than the usual weight-adjusted dosing thus a uniform efficiency on parasites was assumed (Palinauskas et al., 2009; Wiegmann et al., 2022). Control nestlings represent two groups: nestlings that did not receive antimalarial medicine (n = 84) and nestlings that were orally given 0.5 mL of distilled water (n = 49). Both groups did not differ in average physiological parameters nor in Leucocytozoon intensity, hence these individuals were pooled together in the control group, sampled in 2016 (n = 32), 2018 (n = 51), 2019 (n = 30) and 2020 (n = 72). Treated and control groups were, on average, 28.38 days (± s.d. 4.23) and 27.57 days (± s.d. 5.80) old at T1, respectively. Similar treatments have been previously shown to substantially reduce the prevalence of Plasmodium in bird populations (Palinauskas et al., 2009; Knowles et al., 2010; Schoenle et al., 2017). According to the change in parasitemia between both sampling points, the 276 nestlings were separated into four groups (Figure 1): (i) uninfected nestlings (no apparent infection at both samplings, n = 93), (ii) increasing infection (n = 92), (iii) peak infection (i.e., constant parasitemia and >10 parasites per 10.000 erythrocytes at both samplings, n = 24) and (iv) decreasing infection (n = 67).
To test whether two sampling events produce robust estimates, we resampled a small subset of individuals for the third time. Between 2016 and 2020, 17 nestlings (4, 5, 2, 6 respectively for 2016, 2018, 2019, 2020) were resampled for a third time. In 2022, three additional nestlings were sampled three times and were included in this analysis. All twenty nestlings were resampled at an average of 6.1 days (s.d. ± 3.6 days) after second sampling.
(c) Cost-indicative physiological parameters
At T0 and T1, we measured the body weight (to the nearest 5 g) with a spring scale, the wing length (to the nearest mm) with a wing ruler and the respiratory rate (duration of 30 breaths in seconds) of each nestling. The breathing rate serves as a proxy for parasitic-induced anemia during parasite invasion of red blood cells, observed in many bird hosts during avian malaria infections (Cardona et al., 2002; Remple, 2004; Townsend et al., 2018). The cloacal temperature (henceforth body temperature, measured with an electronic thermometer) was recorded in 2019 and 2020. The repeatability of the temperature measures was high: R²= 0.91 (CI = 0.81 – 0.96, P < 0.001), calculated from 27 paired measures taken 30s apart on both adults (> 2 calendar years, with unknown precise age) and nestling common buzzards (age: 20- 30 days, mean: 40.35°C, s.d. ± 0.39°C). To control for an ambient temperature effect, the average daily temperatures of the sampling days were obtained from the NASA POWER Project (Sparks, 2022). The growth rate per day was calculated as the weight change between T0 and T1 divided by the difference in days separating the two measurements. The body condition index of a nestling was estimated for each sampling event as the residual variance of the sex-specific linear regression between weight and wing length (log-transformed), based on standard growth data of common buzzard nestlings (Bijlsma, 1998). To account for the state-dependent development of individual body condition, we used either body condition at T1 or change in body condition (later ΔBC) between T1 and T0 as a proxy of costs in our models.
(d) Statistical analyses
We fitted a linear mixed model to estimate the effect of antimalarial treatment on the parasitemia of nestlings, adding year and interval between samplings as covariates and nest ID as a random factor. As suggested by Råberg et al (2007)) the comparison of slopes of a regression between host health and parasite load is a measure of tolerance, e.g. the steeper the slope, the steeper the health proxy decreases per unit of parasite load - the less tolerant the host. We hence estimated, at each infection stage (increasing, peak and decreasing parasitemia), the slope between a fitness-informative trait (ΔBC) and parasitemia. Slopes were compared among infection stages and to zero using the emmeans (Lenth, 2022) package in R 4.0.2 (R Core Team, 2020).
To examine the relationship of different cost-related physiological parameters to (i) host infection stages and (ii) parasitemia, we used linear mixed models fitted by REML, implemented in lme4 (Bates et al., 2014). We fitted four models with breathing rate and body temperature at T1 as well as body condition at T1 and growth rate between T0 and T1 as the response variables, respectively. As fixed factors, we specified either (i) the infection stage or (ii) the parasitemia, as well as sampling interval (in days), year of sampling, sex and age. To account for a potential effect of the anti-malarial treatment on the response variables, we included treatment (antimalarial treatment versus control) as a fixed factor in all models, although a previous analysis showed no effects of Malarone administration on body condition, growth rate and blood chemistry of the same population of buzzard nestlings (Wiegmann et al., 2022). Numerical explanatory variables were standardized using a z-transformation (Schielzeth, 2010). Nest ID was fitted as a random factor in all models to consider nestling relatedness. All models are described in detail in Supplementary Table S1.
Results
(a) Efficiency of the antimalarial treatment
The proportion of infected nestlings remained stable in the antimalarial treatment group, while in control nestlings it increased between T0 and T1 (Figure 2A). The antimalarial treatment significantly reduced the parasitemia of treated nestlings (Treatment [Malarone]: est. = -1.77, s.d. = 0.28, df = 177, t = -6.45, P = 1.03e-9, Figure 2B). Initial parasitemia in treated nestlings was higher than in control nestlings (permutation two sample t-test, mean ± s.d.: Control = 0.86 ± 1.34, Malarone = 1.58 ± 1.57, perm.: 999, t = -3.97, P = 0.002, Figure 2B). This corresponded to an age difference between treated nestlings compared to control nestlings (mean = 21.4 days and 18.5 days, resp., t = 5.21, df = 225.44, P = 4.30e-07). At T1, the age difference was not significant anymore (t = 1.31, df = 223.28, P = 0.191).
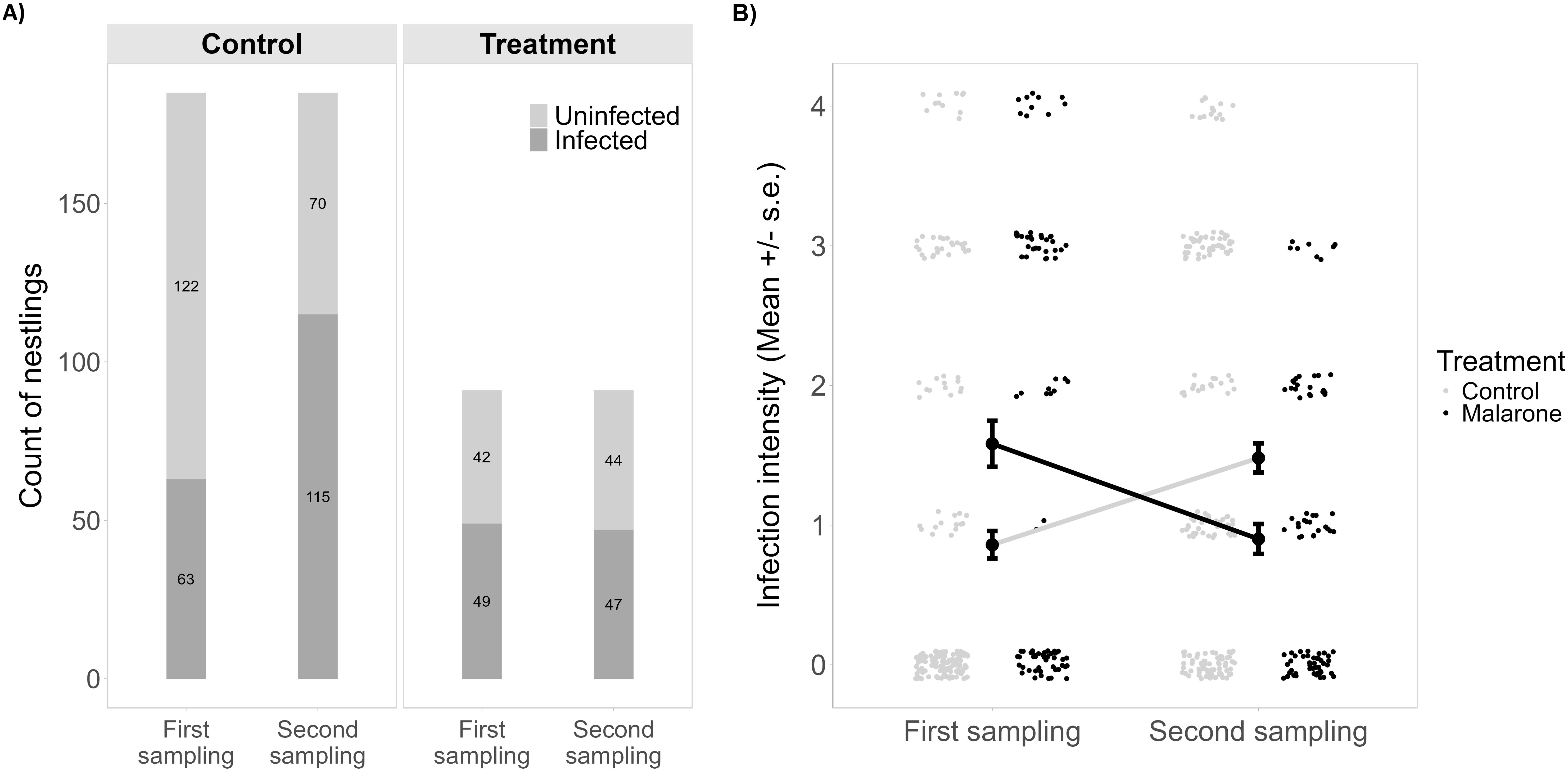
Figure 2. (A) First-to-second sampling count of uninfected and infected nestlings between treatments (control versus antimalarial treatments). (B) First-to-second sampling mean parasitemia between control and Malarone-treated nestlings. Error bars represent standard errors.
(b) Robustness of infection stages
Across the study, 20 nestlings were resampled a third time (Supplementary Figure S6). A consistent infection trend was observed for 65% of them when comparing the infection trend from the first with either the second or third sampling point. Five remained uninfected, six remained with increasing parasitemia, one remained at peak parasitemia, and one remained with decreasing parasitemia. The last seven individuals (35%) of these 20 nestlings slightly differed in their infection development between the first and either second or third sampling. The trend of two nestlings changed from increasing to stable parasitemia, one uninfected nestling changed into increasing parasitemia, two nestling with stable parasitemia changed respectively to increasing and decreasing parasitemia, another one changed from decreasing to peak parasitemia and one from decreasing parasitemia to stable, low parasitemia.
(c) Transient effect of parasitemia on host health throughout infection cycle
Overall, nestling Δ body condition (ΔBC, change between T0 and T1) correlated negatively with parasitemia (est. = -7.02, s.d. = 3.16, df = 274, t = -2.22, P = 0.027). This correlation changed, however, depending on the stage of infection - it was negative in nestlings with increasing (est. = -24.08, s.d. = 5.99, df = 177, t = -4.02, P < 0.001) and peak infections (est. = -41.80, s.d. = 23.47, df = 177, t = -1.78, P = 0.054) but did not correlate in nestlings with decreasing parasitemia (Figure 3; Supplementary Table S2). Moreover, the slopes of ΔBC against parasitemia tended to be different between increasing and decreasing infection (Supplementary Table S2).
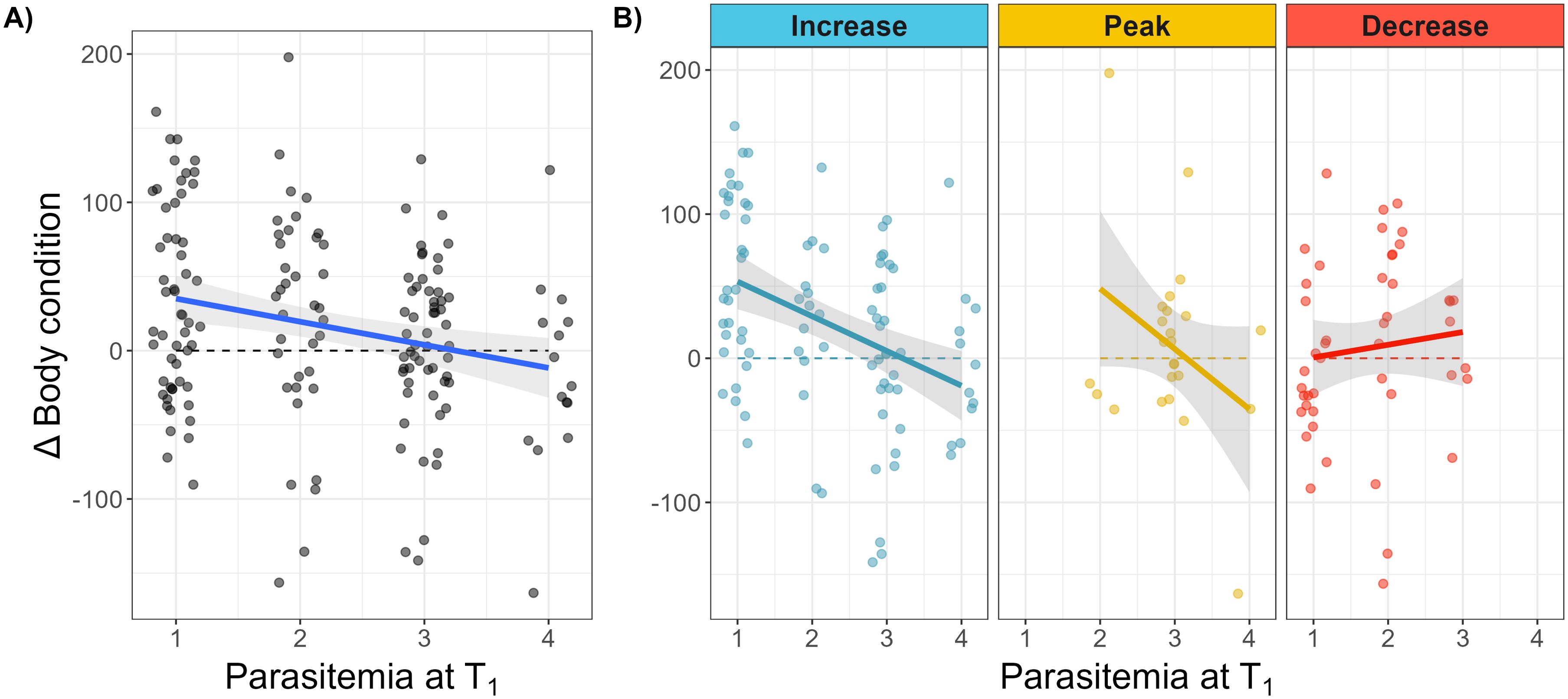
Figure 3. (A) Relationship between body condition change (proxy of host health) and parasitemia at second sampling (parasitic load as a score from 1 to 4) from the raw dataset. (B) Reaction norms are displayed for each of the infection stages (blue: increasing, yellow: peak and red: decreasing parasitemia). Comparisons of reaction norms among infection stages and in comparison to a null slope are presented in Supplementary Table S2. A ΔBC value of 0 means no change of body condition between T0 and T1. P-values of comparisons of regression slopes to zero are displayed in boxes (see Supplementary Table S2).
(d) Unchanged breathing rate across infection stages and intensities
Breathing rate did not correlate with infection stage or parasitemia (Tables 1A, 2A respectively, Supplementary Figure S4A). Heavier nestlings were breathing more slowly, regardless of their infection status and age (est. = -2.62, CI = -4.20 – -1.04, df = 252, t = -3.27, P = 0.001). The breathing rate of nestlings correlated negatively with ambient temperature (est. = -2.97, CI = -4.15 – -1.79, df = 126, t = -4.98, P < 0.001, Supplementary Table S3A). Breathing rates in 2018 and 2020 were significantly lower than in 2016 (Supplementary Table S3A). There was no significant effect of the antimalarial treatment on the breathing rate (Supplementary Table S3A).
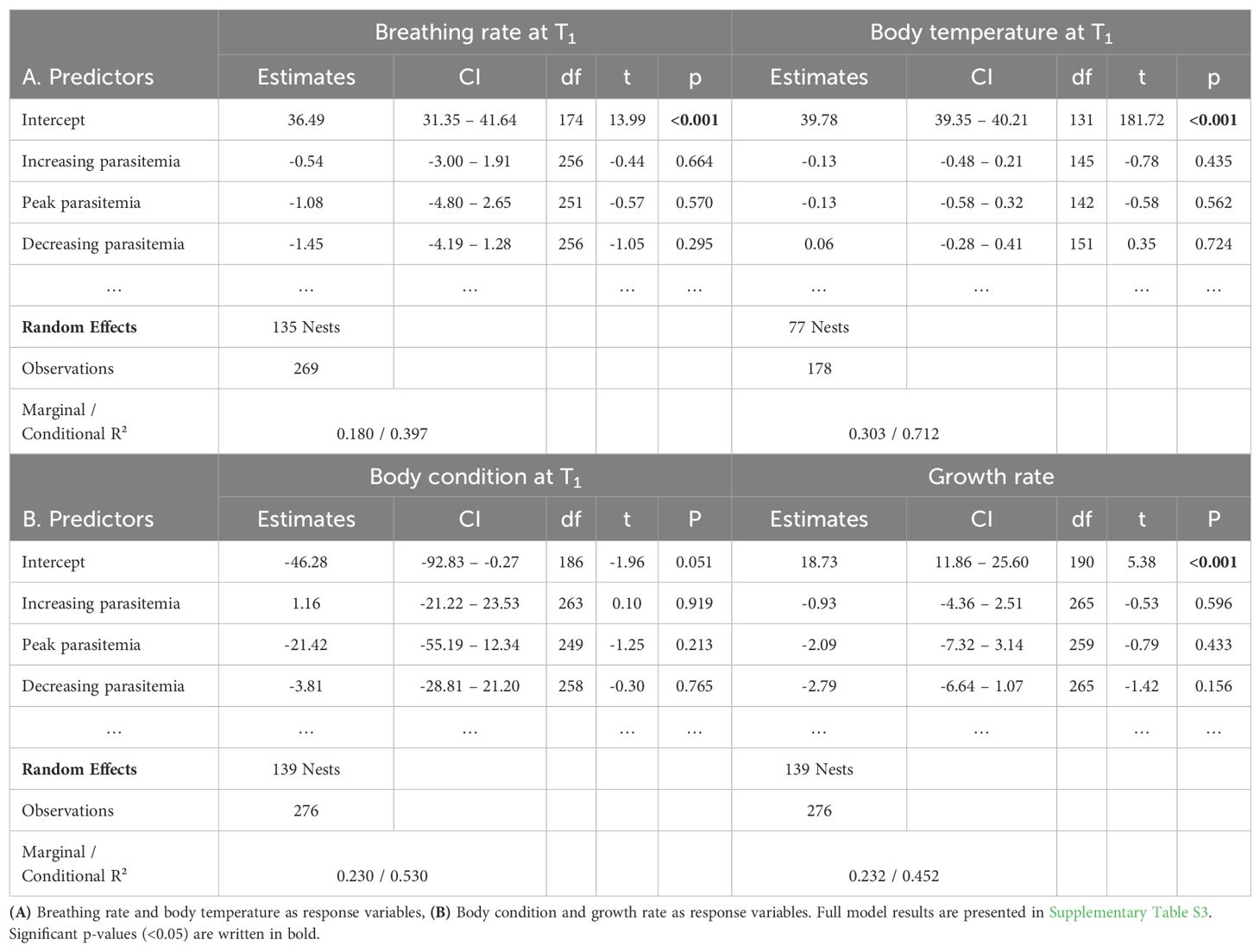
Table 1. Results of four linear mixed models described in Supplementary Table S1, testing the effect of infection stages on the cost-related physiological parameters of Common Buzzard nestlings.
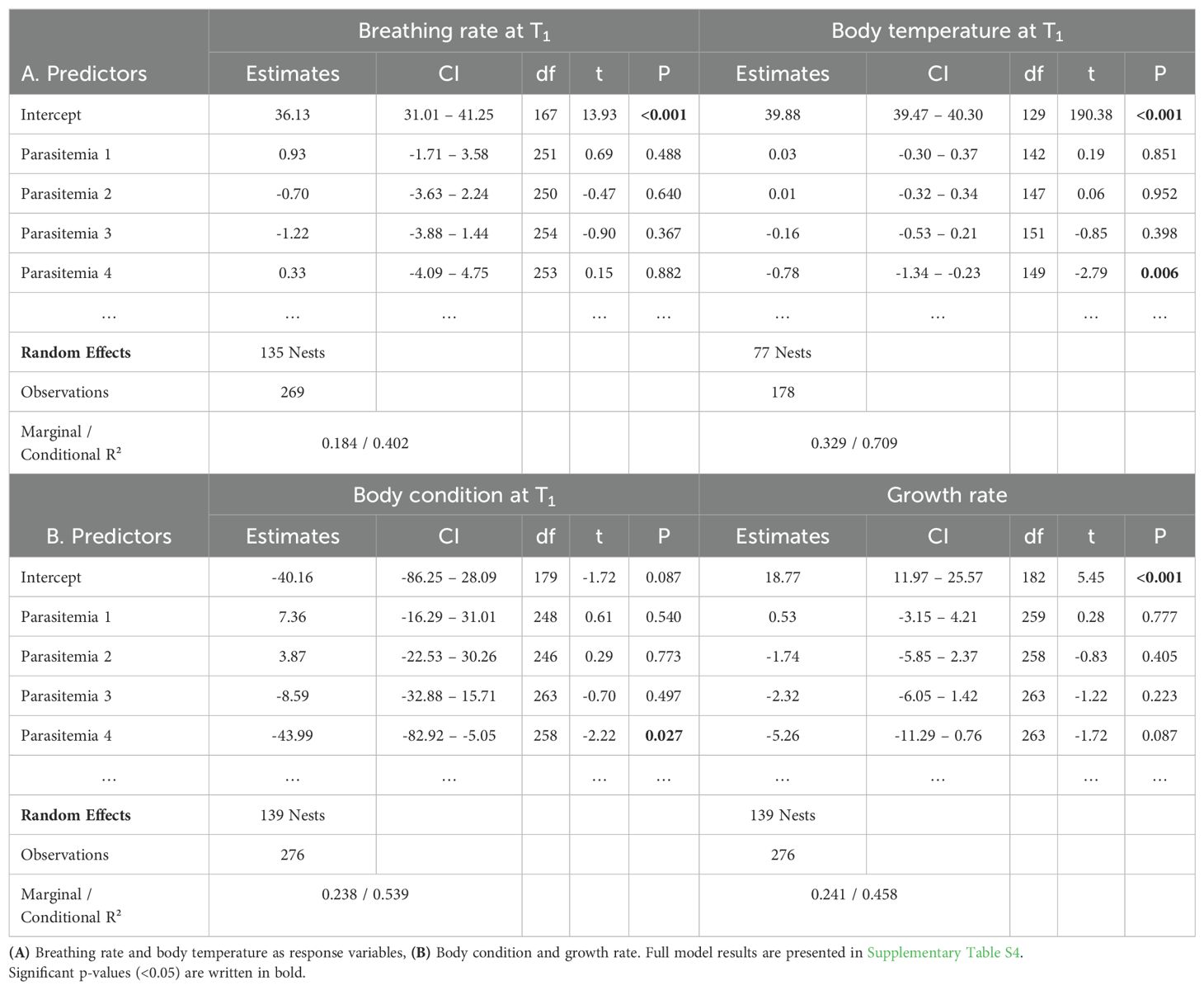
Table 2. Results of four linear mixed models described in Supplementary Table S1, testing the effect of parasitemia at T1 on cost related physiological parameters in common buzzard nestlings.
(e) Lower body temperature at highest infection intensity
Body temperature did not differ among infection stages (Table 1A). However, body temperature was significantly lower in the nestlings with acute parasitemia (level 4) compared with uninfected ones (est. = -0.78, CI = -1.34 – -0.23, df = 149, t = -2.79, P = 0.006, Table 2A, Figure 4B). Body temperature of nestlings increased with ambient temperature and decreased with increasing time between both samplings. There was no effect of the antimalarial treatment on the body temperature (Supplementary Table S3A).
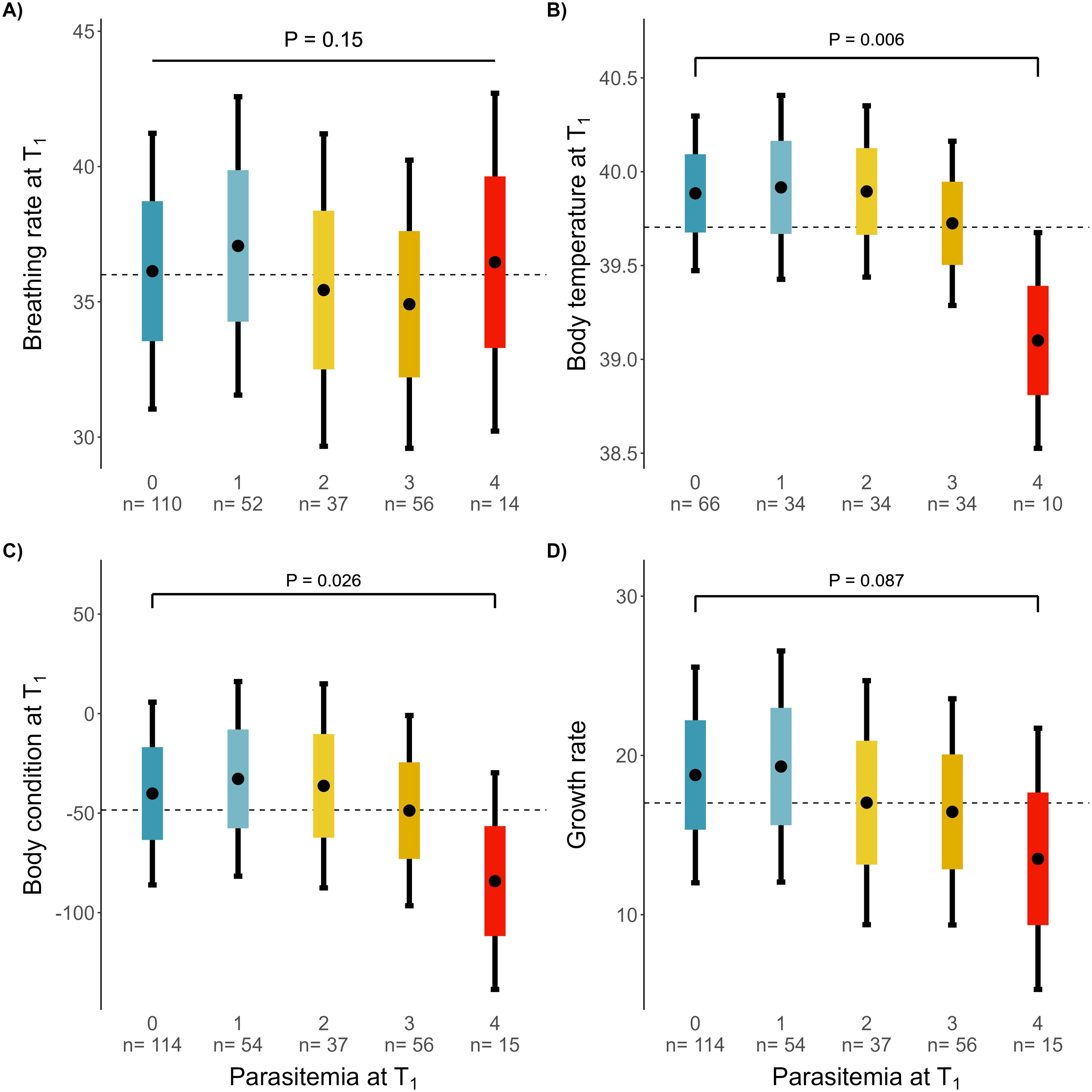
Figure 4. Comparison of predicted resistance-related physiological parameters among parasitemia (ranging from 0, uninfected, to 4, highest parasitemia) from each of the four linear models. Points are predicted means, coloured boxes the standard errors and 95% CI as the error bars. The black dashed lines represent the predicted mean value across groups of a given parameter. (A) comparison of breathing rate, (B) comparison of body temperature, (C) comparison of body condition and (D) comparison of growth rate among infection intensity groups at T1.
(f) Body condition decreased with higher infection intensities but not stages
Body condition did not differ among infection stages (Table 1B). Nestlings with acute peak infections (level 4) had a lower body condition compared with uninfected nestlings (Figure 4C, est. = -43.99, CI = -82.92 – -5.05, df = 258, t = -2.22, P = 0.027, Table 2B). Nestlings at T1 appeared to be leaner (i.e. apparent lower body condition) than at T0. Nestlings had a significantly higher body condition in 2018, 2019 and 2020 compared to 2016 (Supplementary Table S3B). Age and the antimalarial treatment showed no effects on ΔBC (Supplementary Table S3B).
(g) Lower growth rate associated with highest infection intensities
The growth rate of nestlings did not differ between different infection stages after accounting for potential confounding factors, despite a non-significant reduction of growth in later infection stages (Table 1B, Supplementary Figure S1D). Nestlings with the highest parasitemia (level 4) tended to have lower growth rates than uninfected nestlings (Table 2B, Figure 4D). Females had higher growth rates than males. The growth rate decreased with the age of the nestlings, as they were approaching adult size. The growth rate tentatively appeared higher during the last year of the study, and when the time interval between both samplings was longer (Supplementary Table S3B). Finally, there was no evidence for a difference in growth rate between antimalarial-treated and control nestlings.
Discussion
We found that a proxy of host health, body condition decreased with higher parasitemia levels. This finding was shown to be infection stage-dependent, as this negative correlation turned positive at the outset of the infection course (decreasing parasitemia, Figure 3B). The infection stages had no effect on the physiological parameters considered, but body temperature, body condition and growth rate were found significantly lower only at the highest infection intensity (level 4, Figure 4). Despite reducing infection intensities, the anti-malarial treatment did not improve nestlings’ physiological parameters, compared to control nestlings.
Our results suggest that young common buzzards generally do not display parasite-induced costs during the course of infection. Only in cases of acute infection (extraordinarily high levels of peak infection), nestlings appeared unable to completely maintain thermoregulation, body condition and growth rate. Substantial damage and population-wide mortality due to exceptionally high Leucocytozoon parasitemia have been reported in some populations of mostly non-coadapted hosts (Herman et al., 1975; Valkiūnas, 2005; Jia et al., 2018; Valkiūnas and Iezhova, 2023). However, our results indicate that while possible, costs of parasite exploitation and immune activation occur rarely in young birds of prey. While some species of blood parasites form large tissue stages (megalomeronts) which might be most strongly correlated with tissue damage and infection severity (Atkinson and Van Riper, 1991), others, including raptor-specific Leucocytozoon, typically do not appear to produce such stages (Himmel et al., 2019). The moderate effects of Leucocytozoon on raptor nestlings are supported by recent studies showing that these parasites do not induce blood chemistry changes indicative of tissue damage (Wiegmann et al., 2021). Future research across host-parasite interactions is needed to test whether parasitic lineages lacking such tissue stages have a longer coadaptation history and are consequently more benign for the hosts.
We found no difference in breathing rate among nestlings in different stages of infection and uninfected nestlings. This suggests that Leucocytozoon do not cause substantial anaemia in nestlings, which could reduce the physiological oxygen availability and lead to respiratory complications (Hayworth et al., 1987; Wale et al., 2019). Blood parasite genera differ in their life cycle with potential relevance in this aspect - Plasmodium multiply within red blood cells, causing them to burst (schizogony), whereas Leucocytozoon and Haemoproteus only make infected blood cells weaker and/or more susceptible to immune defences (Atkinson and Van Riper, 1991). Both comparative and experimental studies are needed to discern if breathing rate remains unchanged only in young altricial hosts, which are not challenged through flight and other intense muscle use, or if this also applies to free-ranging hosts during peak infection. However, in certain host groups such as raptors (own observations), the majority of primary infection peaks are concentrated during the immobile nestling phase, which may both open opportunities but also restrict the evolutionary paths parasites can take.
Thermoregulation failure as a typical inflammatory response is common in hosts infected by blood parasites (Hayworth et al., 1987; Williams, 2005). Such responses have been found only in bird-Plasmodium systems where fever and hypothermia appeared after parasite inoculation in captive birds (Hayworth et al., 1987; Williams, 2005). Indeed, buzzard nestlings with acute infections displayed lower body temperatures by on average 0.8°C, potentially reflecting hypothermia. However, no difference in body temperature was found among infection stages, suggesting that most hosts pass through all stages of infection without their thermoregulation being compromised.
As several different organs and tissue types may be targeted during infection with blood parasites, host body condition can be expected to deteriorate while parasites increasingly invade host cells (Commichau and Jonas, 1977; Williams, 2005). Nevertheless, body condition only appeared worst in nestlings experiencing acute infections, while there was no difference between infection stages. Thus, symptomatic costs of infection appear to be paid immediately and are only noticeable over rare and short periods when infections are extraordinarily intense. In large bird species, nestling body condition predicts juvenile survival (16, Ottensmann et al., in review). Consequently, acute infections of nestlings may contribute to delayed increase of juvenile mortality, under the condition that chicks are not able to compensate for condition loss before independence.
Growth of infected nestlings did not differ compared to uninfected nestlings, even though it appeared to slow down during consecutive stages of parasitic infection, irrespective of age. Nestlings bearing a high parasitemia tended to display lower growth rates than other groups. Thus, our results suggest that parasitic costs are not apparent at every stage of infection (Figure 3) but only at the highest levels of parasitemia (Figure 4), which occur in only a few individuals. Furthermore, body condition, our best proxy for fitness-relevant host health, decreased with parasite load but this relationship turned around in the later stages of infection (Figure 3). This indicates immediate costs for hosts bearing intense infections and symptoms of infection occurring only during poorly controlled parasitemia. Differing slopes of host health to parasitemia (Figure 3, Supplementary Table S2) between nestlings with increasing and decreasing parasitemia suggests resilience to infection, i.e. condition being regained after onset of adaptive immunity (after peak, already during decreasing parasitemia). Therefore, the overall effects of Leucocytozoon toddi infections in buzzards can be considered mild. Thus, the timing of infection in raptors and similar big birds may be adaptive, as parental care provides a buffer against potentially but rarely cost-intense, short periods of peak infection (Shutler et al., 1999; Valkiūnas, 2005).
The anti-malarial treatment decreased significantly host infection intensities without inducing detectable effects - expected positive - on host health. Our power to detect health improvements in this study seems now unlikely because infection symptoms are already minute, clustered in few peak, acute infections. An infection experiment, combined with a treatment group, may properly demonstrate host health improvements in such low damaging infections.
The apparent low virulence, together with the mostly absent physiological responses to, and relief of infection suggest that the host and parasite may have defused the co-evolutionary arms-race by reaching widespread transmission to the immunologically naïve and susceptible but tolerant nestling stage and thereby, a different equilibrium to parasites of the genus Plasmodium. Unlike generalist parasites, parasites similar to raptor-specific Leucocytozoon may achieve very high prevalence among their main host species, especially because of such relaxed selection pressure (Wiegmann et al., 2021).
Recent research shows that individual survival within the same buzzard population is not explained by Leucocytozoon infection status or intensity (manuscript in review). Because survival is explained by body condition during the nestling phase but not by parasitemia directly, acute parasitic infections could decrease the long-term survival prospects of some individuals through lowered body condition of chicks (Pigeault et al., 2018). However, other environmental factors, such as prey availability and weather conditions appear to be much more influential (Kostrzewa and Kostrzewa, 2008; Sundev et al., 2009).
We show that the year of sampling explains variation in all four physiological parameters. Body condition and growth rate are tightly linked to food availability, while annual fluctuations in field vole (Microtus arvalis) abundance as the main source of energy for common buzzards are known to have strong effects on their demography (Sundev et al., 2009). Fluctuations in vole abundance also explain variation in the breeding success in different parts of the breeding range (Chakarov and Krüger, 2010; Panek, 2021). In our study area, the proportion of voles among prey items corresponds to differences in body condition and growth rate of nestlings of the same cohorts (unpublished data). Future research should specifically address host-parasite relationships in light of the highly dynamic food availability.
Overall, our long-term field experiment resulted in finding only a few signs of infection costs on nestling physiology, suggesting overall low pathogenicity of specialist blood parasites in raptor nestlings. Potential negative effects were apparent rarely and transiently at the highest infection peaks but disappeared at later stages of the infection course. During most non-extreme infections, juveniles appear to be tolerant and robust hosts that enable parasite transmission without paying substantial fitness costs and avoid direct or indirect parasite-induced mortality. Our study demonstrates the necessity to understand in greater detail “childhood diseases” in the wild. We suggest exploration without prejudice of symbiotic and parabiotic relationships between microorganisms and the most probable host developmental stages at the time of first contact, as these interactions and their valence are most relevant for the long-term co-evolutionary dynamics.
Data availability statement
The datasets presented in this study can be found in online repositories. The names of the repository/repositories and accession number(s) can be found below: Raw data and associated code for analyses are available on this repository (https://github.com/TonyRinaud/MS_physiology_infection). Supplementary Materials are available on this repository (https://osf.io/4dhuv/).
Ethics statement
Sampling and drug application of common buzzards were performed in accordance with the relevant guidelines and regulations. This work followed the ARRIVE guidelines and was approved by the Animal Ethics Committee at Bielefeld University and permitted by the local authority Kreis Gütersloh, permit number: 4.5.2-723-Bussard and by the ethics committee of the Animal Care and Use Committee of the German North Rhine-Westphalia State Office for Nature, Environment and Consumer Protection (Landesamt für Nature, Umwelt und Verbraucherschutz Nordrhein-Westfalen) under permit numbers: 84-02.04.2014.A091, 84-02-04.2017.A147. The study was conducted in accordance with the local legislation and institutional requirements.
Author contributions
TR: Conceptualization, Data curation, Formal analysis, Investigation, Methodology, Visualization, Writing – original draft, Writing – review & editing. MO: Conceptualization, Data curation, Formal analysis, Investigation, Methodology, Validation, Visualization, Writing – review & editing. TR: Data curation, Writing – review & editing. HP: Data curation, Writing – review & editing. KG: Data curation, Writing – review & editing. OK: Data curation, Funding acquisition, Project administration, Resources, Supervision, Writing – review & editing. NC: Conceptualization, Data curation, Funding acquisition, Investigation, Methodology, Project administration, Resources, Supervision, Validation, Writing – original draft, Writing – review & editing.
Funding
The author(s) declare that financial support was received for the research and/or publication of this article. This study was supported by the German Science Foundation (DFG) as part of the SFB TRR 212 “A Novel Synthesis of Individualisation across Behaviour, Ecology and Evolution: Niche Choice, Niche Conformance, Niche Construction (NC3)” (project number 396780709) and a research grant for the project “Short- and long-term consequences of malaria-like infections in birds” (project number 398434413). We also acknowledge the Open Access Publication Fund of Bielefeld University for the article processing charge.
Acknowledgments
We would like to thank Thomas Grünkorn who climbed trees to deliver us precious samples for this study. We are also grateful for all the field assistants that helped during fieldwork of 2016, 2018, 2019 and 2020. We commend Öncü Maraci for helpful comments on this manuscript. We acknowledge the financial support of the German Research Foundation (DFG) and the Open Access Publication Fund of Bielefeld University for the article processing charge.
Conflict of interest
The authors declare that the research was conducted in the absence of any commercial or financial relationships that could be construed as a potential conflict of interest.
Generative AI statement
The author(s) declare that no Generative AI was used in the creation of this manuscript.
Publisher’s note
All claims expressed in this article are solely those of the authors and do not necessarily represent those of their affiliated organizations, or those of the publisher, the editors and the reviewers. Any product that may be evaluated in this article, or claim that may be made by its manufacturer, is not guaranteed or endorsed by the publisher.
Supplementary material
The Supplementary Material for this article can be found online at: https://www.frontiersin.org/articles/10.3389/fevo.2025.1581179/full#supplementary-material
References
Ashby B., Bruns E. (2018). The evolution of juvenile susceptibility to infectious disease. Proc. R Soc. B Biol. Sci. 285, 20180844. doi: 10.1098/rspb.2018.0844
Atkinson C. T., Thomas N. J., Hunter D. B. (Eds.) (2008). Parasitic diseases of wild birds (Ames, Iowa: Wiley-Blackwell).
Atkinson T. C., Van Riper III C. (1991). “Bird-parasite interactions,” in Pathology and Epizootiology of Haematozoa. Eds. Loye J. E., Zuk M. (Oxford Ornitohlogy Series). doi: 10.1093/oso/9780198577386.001.00
Bates D., Mächler M., Bolker B., Walker S. (2014). Fitting Linear Mixed-Effects Models using lme4. ArXiv14065823. doi: 10.18637/jss.v067.i01
Bermejo M., Rodriguez-Teijeiro J. D., Illera G., Barroso A., Vila C., Walsh P. D. (2006). Ebola outbreak killed 5000 gorillas. Science 314, 1564–1564. doi: 10.1126/science.1133105
Brown A. F., Pascoe D. (1989). Parasitism and host sensitivity to cadmium: an acanthocephalan infection of the freshwater amphipod gammarus pulex. J. Appl. Ecol. 26, 473–487. doi: 10.2307/2404075
Cardona C. J., Ihejirika A., McClellan L. (2002). Haemoproteus lophortyx infection in bobwhite quail. Avian Dis. 46, 249–255. doi: 10.1637/0005-2086(2002)046[0249:HLIIBQ]2.0.CO;2
Chakarov N., Krüger O. (2010). Mesopredator release by an emergent superpredator: a natural experiment of predation in a three level guild. PloS One 5, e15229. doi: 10.1371/journal.pone.0015229
Chakarov N., Linke B., Boerner M., Goesmann A., Krüger O., Hoffman J. I. (2015). Apparent vector-mediated parent-to-offspring transmission in an avian malaria-like parasite. Mol. Ecol. 24, 1355–1363. doi: 10.1111/mec.13115
Chakarov N., Pauli M., Krüger O. (2017). Immune responses link parasite genetic diversity, prevalence and plumage morphs in common buzzards. Evol. Ecol. 31, 51–62. doi: 10.1007/s10682-016-9871-2
Commichau C., Jonas D. (1977). A disease of ducklings caused by Leucocytozoon simondi with special reference to histopathological diagnosis. Zentralblatt Für Veterinärmedizin Reihe B 24, 662–667.
Cowman A. F., Healer J., Marapana D., Marsh K. (2016). Malaria: biology and disease. Cell 15, 610–624. doi: 10.1016/j.cell.2016.07.055
Dunn J. C., Goodman S. J., Benton T. G., Hamer K. C. (2013). Avian blood parasite infection during the non-breeding season: an overlooked issue in declining populations? BMC Ecol. 13, 30. doi: 10.1186/1472-6785-13-30
Foley J., Clifford D., Castle K., Cryan P., Ostfeld R. S. (2011). Investigating and managing the rapid emergence of white-nose syndrome, a novel, fatal, infectious disease of hibernating bats: white-nose syndrome in bats. Conserv. Biol. 25, 223–231. doi: 10.1111/j.1523-1739.2010.01638.x
Grew R., Ratz T., Richardson J., Smiseth P. T. (2019). Parental care buffers against effects of ambient temperature on offspring performance in an insect. Behav. Ecol. 30, 1443–1450. doi: 10.1093/beheco/arz100
Hatcher M. J., Dick J. T. A., Dunn A. M. (2006). How parasites affect interactions between competitors and predators. Ecol. Lett. 9, 1253–1271. doi: 10.1111/j.1461-0248.2006.00964.x
Hayworth A. M., van Riper C., Weathers W. W. (1987). Effects of plasmodium relictum on the metabolic rate and body temperature in canaries (Serinus canarius). J. Parasitol 73, 850. doi: 10.2307/3282431
Herman C. M., Barrow J. H., Tarshis I. B. (1975). Leucocytozoonosis in Canada geese at the seney national wildlife refuge. J. Wildl Dis. 11, 404–411. doi: 10.7589/0090-3558-11.3.404
Himmel T., Harl J., Kübber-Heiss A., Konicek C., Fernández N., Juan-Sallés C., et al. (2019). Molecular probes for the identification of avian Haemoproteus and Leucocytozoon parasites in tissue sections by chromogenic in situ hybridization. Parasit. Vectors 12, 1–10. doi: 10.1186/s13071-019-3536-2
Jia T., Huang X., Valkiūnas G., Yang M., Zheng C., Pu T., et al. (2018). Malaria parasites and related haemosporidians cause mortality in cranes: a study on the parasites diversity, prevalence and distribution in Beijing Zoo. Malar. J. 17, 1–11. doi: 10.1186/s12936-018-2385-3
Knowles S. C. L., Palinauskas V., Sheldon B. C. (2010). Chronic malaria infections increase family inequalities and reduce parental fitness: experimental evidence from a wild bird population. J. Evol. Biol. 23, 557–569. doi: 10.1111/j.1420-9101.2009.01920.x
Kostrzewa A., Kostrzewa R. (2008). The relationship of spring and summer weather with density and breeding performance of the Buzzard Buteo buteo, Goshawk Accipiter gentilis and Kestrel Falco tinnunculus. Ibis 132, 550–559. doi: 10.1111/j.1474-919X.1990.tb00278.x
Kubi C., Van Den Abbeele J., De Deken R., Marcotty T., Dorny P., Van Den Bossche P. (2006). The effect of starvation on the susceptibility of teneral and non-teneral tsetse flies to trypanosome infection. Med. Vet Entomol. 20, 388–392. doi: 10.1111/j.1365-2915.2006.00644.x
la Puente J. M., Merino S., Tomás G., Moreno J., Morales J., Lobato E., et al. (2010). The blood parasite Haemoproteus reduces survival in a wild bird: a medication experiment. Biol. Lett. 6, 663–665. doi: 10.1098/rsbl.2010.0046
Martinez J., Merino S. (2011). Host-parasite interactions under extreme climatic conditions. Curr. Zool 57, 390–405. doi: 10.1093/czoolo/57.3.390
Palinauskas V., Valkiūnas G., Križanauskienė A., Bensch S., Bolshakov C. V. (2009). Plasmodium relictum (lineage P-SGS1): further observation of effects on experimentally infected passeriform birds, with remarks on treatment with Malarone™. Exp. Parasitol. 123, 134–139. doi: 10.1016/j.exppara.2009.06.012
Panek M. (2021). Does habitat diversity modify the dietary and reproductive response to prey fluctuations in a generalist raptor predator, the eurasian buzzard buteo buteo? Birds 2, 114–126. doi: 10.3390/birds2010008
Panter S. N., Jones D. A. (2002). Age-related resistance to plant pathogens. Adv. Bot. Res. 38, 251–280. doi: 10.1016/S0065-2296(02)38032-7
Pigeault R., Cozzarolo C.-S., Choquet R., Strehler M., Jenkins T., Delhaye J., et al. (2018). Haemosporidian infection and co-infection affect host survival and reproduction in wild populations of great tits. Int. J. Parasitol. 48, 1079–1087. doi: 10.1016/j.ijpara.2018.06.007
Poulin R. (1998). Evolution and phylogeny of behaviour manipulation of insect hosts by parasites. Parasitology 116, S3–S11. doi: 10.1017/S0031182000084894
Råberg L., Sim D., Read A. F. (2007). Disentangling genetic variation for resistance and tolerance to infectious diseases in animals. Science 318, 812–814. doi: 10.1126/science.1148526
Ramsay C., Rohr J. R. (2021). The application of community ecology theory to co-infections in wildlife hosts. Ecology 102. doi: 10.1002/ecy.3253
R Core Team (2020). R: A Language and Environment for Statistical Computing (Vienna, Austria: R Foundation for Statistical Computing).
Remple J. D. (2004). Intracellular hematozoa of raptors: A review and update. J. Avian Med. Surg. 18, 75–88. doi: 10.1647/2003-008
Schielzeth H. (2010). Simple means to improve the interpretability of regression coefficients: Interpretation of regression coefficients. Methods Ecol. Evol. 1, 103–113. doi: 10.1111/j.2041-210X.2010.00012.x
Schoenle L. A., Kernbach M., Haussmann M. F., Bonier F., Moore I. T. (2017). An experimental test of the physiological consequences of avian malaria infection. J. Anim Ecol. 86, 1483–1496. doi: 10.1111/1365-2656.12753
Shutler D., Ankney C. D., Mullie A. (1999). Effects of the blood parasite Leucocytozoon simondi on growth rates of anatid ducklings. Can. J. Zool. 77, 6. doi: 10.1139/z99-121
Simon A. K., Hollander G. A., McMichael A. (2015). Evolution of the immune system in humans from infancy to old age. Proc. R. Soc. B Biol. Sci. 282, 20143085. doi: 10.1098/rspb.2014.3085
Simonsen L., Viboud C. (2021). A comprehensive look at the COVID-19 pandemic death toll 3. eLife. doi: 10.7554/eLife.71974
Snounou G., Jarra W., Viriyakosol S., Wood J., Brown K. (1989). Use of a DNA probe to analyse the dynamics of infection with rodent malaria parasites confirms that parasite clearance during crisis is predominantly strain- and species-specific. Mol. Biochem. Parasitol 37, 37–46. doi: 10.1016/0166-6851(89)90100-X
Stockdale J. E., Dunn J. C., Goodman S. J., Sheehan D. K., Grice P. V., Hamer K. C. (2015). The protozoan parasite Trichomonas gallinae causes adult and nestling mortality in a declining population of European Turtle Doves, Streptopelia turtur. Parasitology 9, 490–498. doi: 10.1017/S0031182014001474
Sundev G., Yosef R., Birazana O. (2009). Brandt’s Vole density affects nutritional condition of Upland Buzzard Buteo hemilasius on the Mongolian Grassland Steppe. Ornis Fennica 86, 9.
Townsend A. K., Wheeler S. S., Freund D., Sehgal R. N. M., Boyce W. M. (2018). Links between blood parasites, blood chemistry, and the survival of nestling American crows. Ecol. Evol. 8, 8779–8790. doi: 10.1002/ece3.4287
Valkiūnas G., Atkinson C. T. (2020). Introduction to life cycles, taxonomy, distribution, and basic research techniques. Avian Malar Relat. Parasites Trop. Ecol. Evol. Syst., 45–80.
Valkiūnas G., Iezhova T. A. (2017). Exo-erythrocytic development of avian malaria and related haemosporidian parasites. Malar. J. 24, 1–24. doi: 10.1186/s12936-017-1746-7
Valkiūnas G., Iezhova T. A. (2023). Insights into the biology of leucocytozoon species (Haemosporida, leucocytozoidae): why is there slow research progress on agents of leucocytozoonosis? Microorganisms 11, 1251. doi: 10.3390/microorganisms11051251
Wale N., Jones M. J., Sim D. G., Read A. F., King A. A. (2019). The contribution of host cell-directed vs. parasite-directed immunity to the disease and dynamics of malaria infections. Proc. Natl. Acad. Sci. 116, 22386–22392. doi: 10.1073/pnas.1908147116
Wiegmann A., Rinaud T., Ottensmann M., Krüger O., Springer A., Legler M., et al. (2022). Tolerability of atovaquone/proguanil application in common buzzard nestlings. Vet Sci. 9. doi: 10.3390/vetsci9080397
Wiegmann A., Springer A., Rinaud T., Ottensmann M., Legler M., Krüger O., et al. (2021). The prevalence of Leucocytozoon spp. in nestlings of three wild raptor species including implications on haematological and blood chemistry values. Int. J. Parasitol. Parasites Wildl. 16, 236–243. doi: 10.1016/j.ijppaw.2021.10.009
Williams R. B. (2005). Avian malaria: clinical and chemical pathology of Plasmodium gallinaceum in the domesticated fowl Gallus gallus. Avian Pathol. 34, 29–47. doi: 10.1080/03079450400025430
World Health Organization (2016). World malaria report 2015 (World Health Organization). Available online at: https://www.who.int/publications/i/item/9789241565158.
Keywords: avian malaria, bird of prey, host-parasite interactions, physiology, infection costs
Citation: Rinaud T, Ottensmann M, Rapp TM, Pereira H, Gladow K-P, Krüger O and Chakarov N (2025) Blood parasite infection causes marginal temporary costs in juvenile birds of prey. Front. Ecol. Evol. 13:1581179. doi: 10.3389/fevo.2025.1581179
Received: 21 February 2025; Accepted: 31 March 2025;
Published: 28 April 2025.
Edited by:
Pascual López-López, University of Valencia, SpainReviewed by:
Sveinn Are Hanssen, Norwegian Institute for Nature Research (NINA), NorwayElena Arriero, Complutense University of Madrid, Spain
Copyright © 2025 Rinaud, Ottensmann, Rapp, Pereira, Gladow, Krüger and Chakarov. This is an open-access article distributed under the terms of the Creative Commons Attribution License (CC BY). The use, distribution or reproduction in other forums is permitted, provided the original author(s) and the copyright owner(s) are credited and that the original publication in this journal is cited, in accordance with accepted academic practice. No use, distribution or reproduction is permitted which does not comply with these terms.
*Correspondence: Tony Rinaud, dG9ueS5yaW5hdWRAZ21haWwuY29t
†ORCID: Tony Rinaud, orcid.org/0000-0003-3811-2270