- 1Szczecin Faculty of Chemical Technology and Engineering, Department of Nanomaterials Physicochemistry, West Pomeranian University of Technology, Szczecin, Poland
- 2Kirchhoff Institute of Physics, Heidelberg University, Heidelberg, Germany
- 3Centre for Advanced Materials (CAM), Heidelberg University, Heidelberg, Germany
Three-dimensional (3D) graphene-based nanocomposites have received considerable attention in both fundamental research and industrial applications, as they combine the functionalities of well-controlled nano-architectures and the integrity of bulk materials. Actually, among these materials, spherical structures are attracting more and more attention worldwide due to their excellent performance in various fields such as drug delivery, heterogeneous catalysis, encapsulation of support, and electrode materials for lithium-ion batteries. Herein, a facile route to fabricate a three-dimensional hierarchical graphene/MoS2 nanocomposite is presented. The molecular heterostructure is derived from graphene oxide flakes and precursors of molybdenum ((NH4)2Mo7O4·4H2O) and sulfur (L-cysteine). Spherical morphology (GO/MoS2) is obtained via self-assembly of the precursor. This 3D nanocomposite exhibits MoS2-nanosheets strongly linked to graphene oxide flakes, which renders it particularly suited to exploit the conversion reaction of MoS2 for electrochemical energy storage. When assembled into an electrode in lithium-ion batteries, as-prepared GO/MoS2 electrodes indeed deliver a high initial charge capacity of 783 mA h g−1 at a current density of 100 mA/g and Coulombic efficiency of more than 96% from the second cycle on exceeding the theoretical capacity of the pristine 2D MoS2 and graphene. Overall, the study sheds some light on the design of 3D heterostructure as a promising anode material in Li-ion batteries.
Introduction
Motivated by recent rapid advancements and considering the constraints of two-dimensional (2D) graphene, the research on graphene-based materials has been directed toward the exploration of different graphene-like morphologies, with appropriate and attractive contributions from physics, chemistry, and materials science, among others (Novoselov et al., 2004; Novoselov et al., 2005; Zhu et al., 2014). The actual application performance achieved using 2D materials is always less than that anticipated based on its ideal properties such as high surface area, high charge carrier mobility, premier mechanical strength, and excellent thermal conductivity.
Functional carbon nanospheres with controllable size, surface area, pore diameter, surface morphology, and chemical composition are an appealing topic. They reveal enhanced performance in a wide variety of applications, such as electrode materials for electrochemical energy storage, absorbents, or catalysts (Zheng et al., 2014; Wang et al., 2021). Graphene spheres and reshaped structures with good chemical and mechanical properties are among the most promising candidates to overcome the obstacles associated with 2D nanosheets. They exhibit plentiful porous channels with enhanced electrical conductivity and superb structural stability (Liu et al., 2020a; Ji et al., 2020).
Various nanostructured MoS2 and carbon composites have triggered intensive interest due to their intriguing physicochemical properties. Hou et al. reported molybdenum disulfide nanoparticles with spherical structures prepared via a hydrothermal method of about 190 nm in diameter (Koroteev et al., 2021). Cui and co-workers prepared a novel carbon-coated MoS2 nanobowl structure by a solvothermal method, followed by an annealing process (Cui et al., 2015). Zhou et al. reported on carbon nanofibers decorated with molybdenum disulfide sheets (Liu et al., 2020b). To optimize links between MoS2 and carbon, a variety of approaches have been explored, such as exploiting the high wettability of N-doped graphitic surface and electrostatic attraction between thiomolybdate precursor anion and N-doped sites to fabricate amorphous molybdenum sulfide layers directly bound at vertical N-doped carbon nanotube forest surface (Li et al., 2014; Long et al., 2014). Further approaches have yielded MoS2@C nanotubes (Zhang et al., 2016a), C/MoS2/C sandwiched hollow spheres (Li et al., 2017), carbon-sheathed MoS2 on CNT (Zhang et al., 2018), and MoS2/N-doped carbon porous nanorods.
A particularly promising application of MoS2/C composite heterostructures is testing them as anode material in lithium-ion batteries (LIB). While their unique and layered structure and high theoretical lithium storage capacity of 670 mA h g−1 imply great prospects of MoS2 for LIB in general (Gao et al., 2013; Hu et al., 2016), semi-conductivity and large volume changes associated with the conversion reaction process are severe drawbacks for the actual application (Stephenson et al., 2014). Increasing conductivity and mechanical strength by fabrication of 3D MoS2/C composite structures have, however, resulted in various materials with good cycling stability in LIB (Zhang et al., 2016a; Zhang et al., 2018; Li et al., 2019a) and sodium-ion batteries (David et al., 2018; Xiong et al., 2018; Li et al., 2019b). In particular, structures are expected to offer several benefits (Li et al., 2009; Wu et al., 2018). The proposed 3D heterostructures exhibit a high contact area with the electrolyte providing more active sites for de-/lithiation and favorable Li+ transport kinetics. Also, it is proposed that the internal cross-linkage of nanosheets can prevent their aggregation and ensure good structural stability.
We present a facile and highly reproducible route to prepare a spherical 3D structure based on graphene and MoS2 in a solution phase. MoS2 and graphene oxide were self-assembled from structures to spherical heterostructures at a high-temperature reaction in a line-Teflon autoclave. A mechanism for reshaping the spherical structure is proposed. Furthermore, we report on the electrochemical performance of designed GO/MoS2-based when used as electrodes in Li-ion battery.
Experimental section
Preparation of graphene oxide
The graphene oxide was synthesized by a modified Hummers method (Hummers and Offeman, 1958). Shortly, 1 g of graphite was mixed with 6 g of KMnO4 in a flask. Concentrated sulfur acid and orthophosphoric acid (90:10 ml) were added to the flask and then heated to 50°C while stirring. After 24 h, the mixture was poured into ice (100 ml) and H2O2 (30%, 1 ml) and then filtered using a polycarbonate membrane. The solid product was washed twice with water, 10% HCl, and ethanol and, finally, vacuum-dried for 12 h.
Preparation of MoS2 and GO-based heterostructures (GO/MoS2)
A total of 30 mg of GO was introduced to 820 mg of ammonium molybdate tetrahydrate (NH4)2Mo7O4•4H2O mixed with 120 ml of DI water and sonicated for 30 min. In the next step, a source of sulfur (2.4 g of l-cysteine) was added. The mixture was transferred into a Teflon-lined steel autoclave and heated to 240°C for 24 h. Afterward, the sample was washed with ethanol and dried at 60°C for 24 h.
Characterization
The crystallographic information regarding the as-synthesized samples was established by X-ray diffraction (XRD), performed using Philips X'Pert PRO X-ray diffractometer a Cu Ka radiation. The morphology of the samples was obtained using transmission electron microscopy (Tecnai F20-based at 200 kV) and scanning electron microscopy (TESCAN VEGA 3). For monitoring the microstructural changes of the GO/MoS2-electrode after cycling, ex situ SEM studies were performed using a JEOL JSM-7610F scanning electron microscope. The cycled electrodes were disassembled in an Argon glove box, washed with ethylene carbonate, and then dried overnight. Atomic Force Microscopy (AFM NTEGRA Aura (NT-MTD) microscope) was employed to obtain the thickness and number of layers of GO. Thermogravimetric analysis (TGA) was carried out using an SDT Q6000 thermoanalyzer instrument (TA Instruments Inc.) under airflow of 100 ml/min. The samples were heated from room temperature to 900°C at a linear heating rate of 10°C/min. Raman spectroscopy was applied with a microscope mode (InViaRenishaw) with a 785 nm laser. N2 adsorption/desorption isotherms were obtained at liquid nitrogen temperature (77 K) using a Micromeritics ASAP 2010M instrument. The Brunauer–Emmett–Teller (BET) and Barrett–Joyner–Halenda (BJH) methods were adopted to calculate the specific surface area and pore size distribution.
Electrochemical studies on GO/MoS2 have been performed by means of Swagelok-type cells (Ottmann et al., 2015) at 25°C. Working electrodes were prepared by mixing 80 wt% active material, 10 wt% carbon black (Super C65, Timcal), and 10 wt% polyvinylidene fluoride (PVDF, Sigma-Aldrich, 99%) in anhydrous 1-methyl-2-pyrrolidinone (NMP, Sigma-Aldrich, 99%) for 12 h. After pasting the slurry on a circular copper mesh of 10 mm diameter, the electrodes were vacuum dried overnight at 65°C and pressed subsequently. The procedure resulted in an active mass loading of about 4 mg cm−2. The cells were assembled in an Ar-Glovebox using the as-prepared working electrodes, two layers of glass fiber (Whatman GF/D) as a separator and lithium foil pressed on a nickel plate as a counter electrode. A total of 200 μL of a 1 M LiPF6 salt solution in 1:1 ethylene carbonate (EC) and dimethyl carbonate (DMC) (Merck Electrolyte LP30) was used as the electrolyte. Electrochemical measurements were performed between 0.01 and 3.0 V versus Li/Li+ at a scan rate of 0.05 mV/s for cyclic voltammetry and 100 mA/g as the current rate for galvanostatic cycling using a VMP3 multichannel potentiostat (Bio-Logic SAS). The carbon content was examined by elemental analysis using Vario MICRO Cubes (Elementar).
Results and discussion
The morphology of GO investigated by SEM and AFM microscopes is presented in Figures 1A,B, respectively. Figure 1A shows that few-layered GO was successfully obtained by a modified Hummers method. The Tapping-mode of AFM used to determine the thickness of GO (Figure 1C) implies a thickness of ∼4.3–5.4 nm of the resulting sheets corresponding to 7–9 layers of GO (Wang et al., 2017).
The schematic illustration of synthesis and the application for GO/MoS2 is present in Scheme 1.
Figure 2 shows TEM and SEM images of the obtained spherical material. Specifically, Figures 2A,B present the TEM images of structure based on GO and MoS2 (GO/MoS2). The SEM image (Figure 2C) reveals that the composite is in the form of irregular spheres with diameters of ∼800 ± 70 nm. Further investigations by means of TEM (Figures 2A,C) allow determining the morphology in greater detail. The irregular spheres are formed by flowerlike structures. A high-resolution transmission electron microscopy image (Figures 2D,E) enables reading off the distance between the layers to be 0.69 nm which is slightly larger than the (002) plane spacing of 0.62 nm in bulk MoS2. As shown in Figure 2F, EDS line scans taken across the wall of the sphere (see red line in Figure 2E clearly prove the elemental composition: C, Mo, and S are evenly distributed throughout the wall.
TGA studies performed in the temperature range from 25 to 1,000°C under air gas flow (Figure 3A) observed a gradual weight loss occurring in three steps. A first weight loss of 9% observed in the range of 320–400°C is associated with oxidation of MoS2 to MoO3. The next significant weight loss of 58% appears above 680°C and corresponds to graphite burning. After burning at around 900°C, the ash content in the ceramic cap is about 1.5%, corresponding to MoO3.
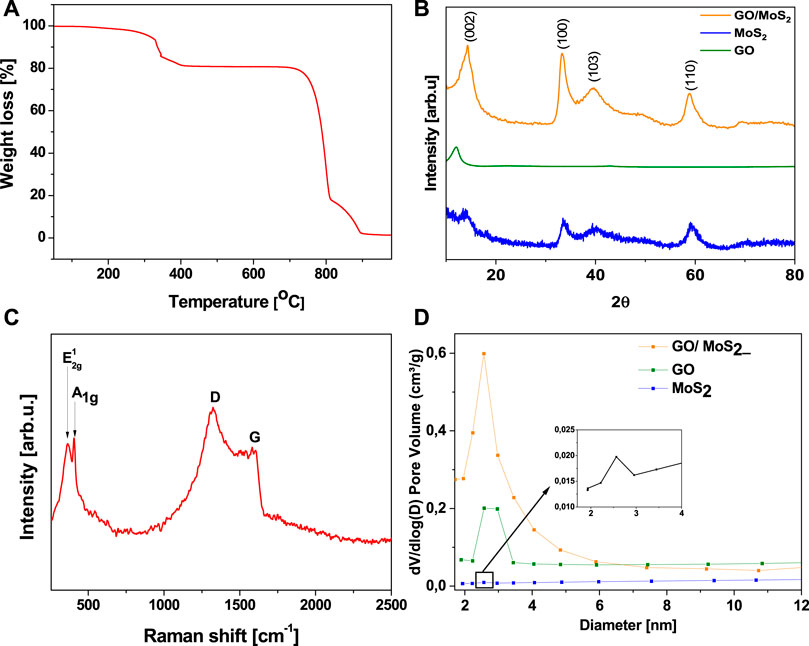
FIGURE 3. TGA profile (A), XRD pattern (B), Raman spectrum (C) and BJH pore size distribution of GO, MoS2, and GO/MoS2 (D).
The presence of crystalline MoS2 in GO/MoS2 is revealed by powder XRD as shown in Figure 3B. The diffraction peaks at 2ϴ of 14°, 33°, 39°, and 59.8° can be unambiguously assigned to the (002), (100), (103), and (110) planes in hexagonal MoS2, respectively (Zhang et al., 2016b). The (002) peak indicates the formation of the well-stacked layered structure of MoS2 (Chhowalla and Amaratunga, 2000).
No obvious XRD peaks from a carbon phase are detected, which might be due to its lower content (<10 wt%), its rather amorphous nature, and the high background signal. The presence of carbon is, however, confirmed via Raman spectroscopy (Figure 3C), which is one of the most sensitive techniques to characterize carbon materials. The spectrum shows the G-mode at about 1,583 cm−1 arising from the stretching of the C-C bond in graphitic materials, and it is common to all sp2-carbon systems (Novoselov et al., 2004), whereas the D band at about 1,353 cm−1 is assigned the so-called breathing vibrations, which are mainly enabled by defects and disorder. Raman data of GO/MoS2 present strong signals of E2g1 and A1g modes of MoS2 (Wieting and Verble, 1971).
The surface area and pore volume of GO/MoS2 andthe intermediate products, i.e., the pure GO flakes and exfoliated MoS2 are presented on Figure 3D. The nanocomposite GO/MoS2 features the largest surface area of 68 m2/g. For the pure GO flakes and exfoliated MoS2, much smaller surface areas of 10 and 11 m2/g were observed, respectively. The pore volume in GO/MoS2 is strongly enhanced to 0.52 cm3/g, whereas GO and the exfoliated MoS2 exhibit pore volumes of 0.002 and 0.01 cm3/g, correspondingly. According to the pore distribution of the three materials calculated by means of the Barrett–Joyner–Halenda (BJH) analysis, GO/MoS2 is characterized by a uniform mesostructured with narrow pore size distribution with a diameter of ∼2.5 nm (Figure 3D).
We believe that GO flakes might serve as building blocks in the self-assembly process triggered by reducing agent L-cysteine. This effect was already observed previously by Xu et al. (2021). During this process, GO is functionalized by sulfur and molybdenum. In the next step, at the temperature of 240°C, the sulfur and molybdenum deposited on the spherical structure of GO reacted, forming MoS2 sheets evenly distributed in the bulk heterostructure.
Electrochemical measurements
The electrochemical properties of GO/MoS2 are investigated by means of cyclic voltammetry (CV) and galvanostatic cycling with potential limitation (GCPL). Figure 4A shows the first, second, and tenth cycles of the CV of GO/MoS2-based electrodes. The initial reductive sweep shows an extended region of activity R1 in the potential range from 1 to 2 V corresponding to the intercalation of Li+ into the layered structure of MoS2 (Li et al., 2016) accompanied by a phase transition from trigonal prism structure (2H) into the octahedral structure (1T) (Chang and Chen, 2011; Teng et al., 2016). The absence of a well-defined redox peak agrees with the low crystallinity of MoS2 in the material, which is also inferred from the XRD data (Figure 3B). The following peak R2 at 0.5 V represents the subsequent conversion reaction of LixMoS2 into metallic Mo and Li2S (Li et al., 2016):
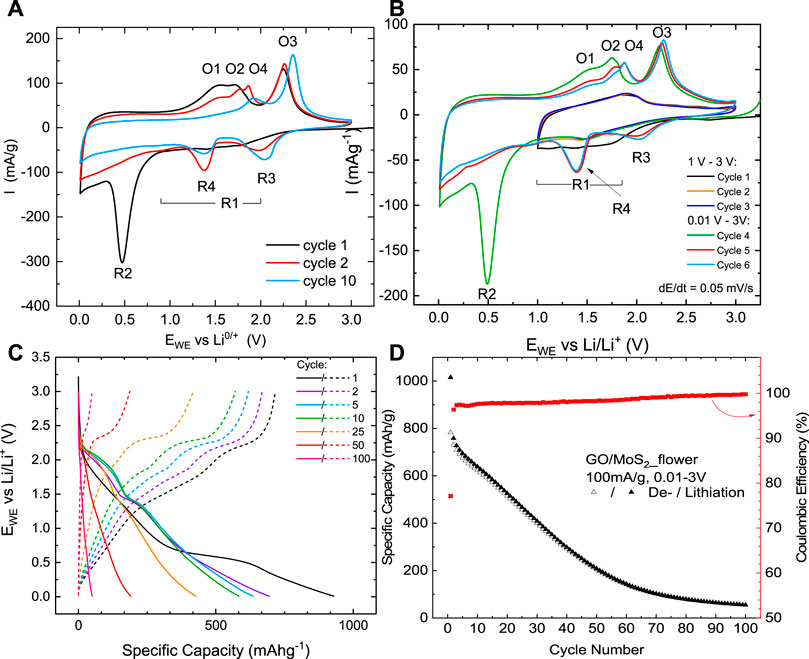
FIGURE 4. (A) Cyclic voltammograms of GO/MoS2-type electrodes recorded at a scan rate of 0.05 mV/s, (B) CV of GO/MoS2 at a scan rate of 0.05 mV/s in the potential range from 1.0 to 3 V in the first three cycles and subsequently in the potential range of 0.01 to 3 V of cycles 4, 5 and 6. (C) Potential profile of galvanostatic cycling of GO/MoS2 at 100 mA/g between 0.01 and 3 V at specific cycles. (D) Specific charge/discharge capacities and associated Coulombic efficiencies during the first hundred cycles studied by GCPL at 100 mA/g.
In the first oxidative scan, a broad double peak O1/O2 around 1.5/1.7 V is visible. The corresponding reaction processes are controversially discussed in the literature. On the one hand, O1/O2 has been associated with partial oxidation of Mo, including possible re-formation of MoSx (Yang et al., 2013; Tang et al., 2014; Wu et al., 2018). However, our CV studies in a restricted potential range (Figure 4B) indicate that peak O1 is linked to the conversion reaction (Eq. 2). The oxidative peak O3 at 2.3 V corresponds to the reversible conversion reaction of Li2S to elemental S (Li et al., 2016):
The associated reduction peak R3 emerges from the second cycle on at 2.0 V. Figure 4B shows that the reversible de-/lithiation process with the participation of the electrochemical active couple Li2S/S is enabled by the conversion reaction (Eq. 2), which provides the reactant Li2S. The main lithium storage mechanism for the following cycles is the reversible sulfur conversion reaction (Eq. 3). This is also visible up to the 100th cycle in the potential profiles (Figure 4C). Starting from the second cycle on, another pronounced reduction peak R4 (1.4 V) and an oxidation peak O4 (1.9 V) emerge. These peaks were also observed by Li et al. (2016), but the underlying processes remain unclear.
The cycling performance of GO/MoS2-type electrodes studied by GCPL measurements at a current density of 100 mA/g over 100 cycles is displayed in Figure 4D. The dis-/charge capacities in the first cycle are 1,015/783 mA h/g. The capacity loss in the first cycle is mainly attributed to the partially irreversible conversion reaction (Eq. 2) and the formation of a solid electrolyte interface (SEI). Based on the reaction mechanism of Li2S/S (Eq. 3) with a theoretical capacity of 1,675 mA h/g, for MoS2, a maximal capacity of 672 mA h/g is expected. Initially, the measured capacities of GO/MoS2 even exceed this value, suggesting that the complete MoS2 is involved in the Li+-ion storage process. The dis-/charge capacities start to decrease constantly from 758/730 mA h/g in the second cycle and stabilize at around 55 mA h/g after 100 cycles. Strong fading effects are expected to arise from mechanical stress associated with the conversion reaction and the dissolution of polysulfides (shuttle effect) during electrochemical cycling. SEM images of a GO/MoS2-type electrode extracted after the 100th cycle (Figure 5) show that the structure seems to be destroyed after cycling. The same observation has been reported in previous works (Das et al., 2012; Ren et al., 2017a; Ren et al., 2017b), where it is attributed to aggregation of MoS2 during electrochemical cycling. Moreover, SEM studies confirm that a coating, for example, with TiO2 or carbon, can prevent structural degradations and thus enhances the cycling stability.
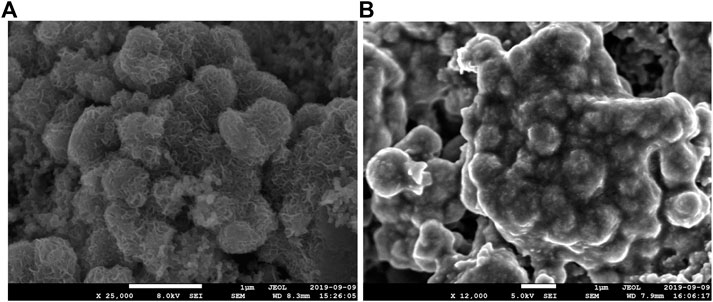
FIGURE 5. SEM images of GO/MoS2-based electrodes before (A) and after 100 times, galvanostatically cycled at 100 mA/g (B).
By combining spherical structured MoS2 nanosheets with graphene oxide, a composite with a large surface area and conductive network is obtained, enabling high accessibility of the active material. The GO/MoS2 composite initially reaches the full theoretical capacity, but degradation effects lead to poor cycle stability. Comparison with the electrochemical performance of other reported MoS2/C-composites shows promising options to optimize the cycling stability. The GO/MoS2 composite studied by Jiao et al. exhibits similar electrochemical properties as the composite studied at hand. N-plasma treatment improves the cycling stability. Another effective strategy to inhibit structural degradation and thus capacity fading is coating MoS2 with carbon, for example. Das et al. showed that while uncoated MoS2/C composite delivers a performance like that of the material presented at hand, C-coated MoS2/C exhibits superior cycling stability. (Das et al., 2012).
Conclusion
In summary, this study presents a facile and reproducible fabrication route of 3D graphene and molybdenum disulfide heterostructure with spherical morphology and their potential application as electrode material in Li-ion batteries. The mechanism of spherical structure formation was proposed. As anode material for Li-ion batteries, the spherical GO/MoS2 initially delivers an excellent capacity by complete conversion. The high surface area attained by the proposed heterostructure and the presence of graphene oxide, providing a conductive network, enable the high theoretical capacity of MoS2. Therefore, we believe this material shows considerable promise from both a scientific viewpoint and practical applications.
Data availability statement
The raw data supporting the conclusion of this article will be made available by the authors without undue reservation.
Author contributions
Conceptualization, KW, EM, and RK; methodology and formal analysis, ET,XC and KW; writing–original draft preparation, KW, LS, VA, and ET; writing–review and editing, EM, RK, XC, visualization, KW, ET; funding acquisition, EM, RK, XC. All authors have read and agreed to the published version of the manuscript.
Acknowledgments
The authors are grateful for the financial support from National Science Centre Poland OPUS 10 UMO-2015/19/B/ST8/00648 and Beethoven UMO-2016/23/G/ST5/04200. This work was partly supported by Deutsche Forschungsgemeinschaft DFG via KL 1824/12-1.
Conflict of interest
The authors declare that the research was conducted in the absence of any commercial or financial relationships that could be construed as a potential conflict of interest.
Publisher’s note
All claims expressed in this article are solely those of the authors and do not necessarily represent those of their affiliated organizations or those of the publisher, the editors, and the reviewers. Any product that may be evaluated in this article, or claim that may be made by its manufacturer, is not guaranteed or endorsed by the publisher.
References
Chang, K., and Chen, W. (2011). l-Cysteine-Assisted synthesis of layered MoS2/graphene composites with excellent electrochemical performances for lithium ion batteries. ACS Nano 5 (6), 4720–4728. doi:10.1021/nn200659w
Chhowalla, M., and Amaratunga, G. A. (2000). Thin films of fullerene-like MoS 2 nanoparticles with ultra-low friction and wear. Nature 407, 164–167. doi:10.1038/35025020
Cui, C., Li, X., Hu, Z., Xu, J., Liu, H., and Ma, J. (2015). Growth of MoS2@C nanobowls as a lithium-ion battery anode material. RSC Adv. 5, 92506–92514. doi:10.1039/C5RA17992K
Das, S. K., Mallavajula, R., Jayaprakash, N., and Archer, L. A. (2012). Self-assembled MoS2–carbon nanostructures: Influence of nanostructuring and carbon on lithium battery performance. J. Mat. Chem. 22 (26), 12988. doi:10.1039/C2JM32468G
David, L., Bhandavat, R., and Singh, G. (2018). MoS2/graphene composite paper for sodium-ion battery electrodes. ACS Nano 8 (2), 1759–1770. doi:10.1021/nn406156b
Gao, M.-R., Xu, Y.-F., Jiang, J., and Yu, S.-H. (2013). Nanostructured metal chalcogenides: Synthesis, modification, and applications in energy conversion and storage devices. Chem. Soc. Rev. 42 (7), 2986–3017. doi:10.1039/C2CS35310E
Hu, Z., Liu, Q., Sun, W., Li, W., Tao, Z., Chou, S.-L., et al. (2016). MoS2 with an intercalation reaction as a long-life anode material for lithium ion batteries. Inorg. Chem. Front. 3 (4), 532–535. doi:10.1039/C5QI00237K
Hummers, W. S., and Offeman, R. E. (1958). Preparation of graphitic oxide. J. Am. Chem. Soc. 80, 1339. doi:10.1021/ja01539a017
Ji, S., Kim, S. K., Song, W., Yoon, Y., Myung, S., Lim, J ., et al. (2020). Extraordinary lithium storage capacity and lithiation mechanism of partially amorphous molybdenum sulfide on chemically exfoliated graphene. Electrochimica Acta 354, 136636. doi:10.1016/j.electacta.2020.136636
Koroteev, V. O., Stolyarova, S. G., Kotsun, A. A., Modin, E., Makarova, A. A., Shubin, Y., et al. (2021). Nanoscale coupling of MoS2 and graphene via rapid thermal decomposition of ammonium tetrathiomolybdate and graphite oxide for boosting capacity of Li-ion batteries. Carbon 173, 194–204. doi:10.1016/j.carbon.2020.10.097
Li, H., Li, W., Ma, L., Chen, W., and Wang, J. (2009). Electrochemical lithiation/delithiation performances of 3D flowerlike MoS2 powders prepared by ionic liquid assisted hydrothermal route. J. Alloys Compd. 471 (1-2), 442–447. doi:10.1016/j.jallcom.2008.03.133
Li, D. J., Maiti, U. N., Lim, J., Choi, D. S., Lee, W. J., Oh, Y., et al. (2014). Molybdenum sulfide/N-doped CNT forest hybrid catalysts for high-performance hydrogen evolution reaction. Nano Lett. 14 (3), 1228–1233. doi:10.1021/nl404108a
Li, Z., Liu, S., Vinayan, B. P., Zhao-Karger, Z., Diemant, T., Wang, K., et al. (2019). Hetero-layered MoS2/C composites enabling ultrafast and durable Na storage. Energy Storage Mater. 21, 115–123. doi:10.1016/j.ensm.2019.05.042
Li, Z., Ottmann, A., Sun, Q., Kast, A. K., Wang, K., Zhang, T., et al. (2019). Hierarchical MoS 2–carbon porous nanorods towards atomic interfacial engineering for high-performance lithium storage. J. Mat. Chem. A Mat. 7 (13), 7553–7564. doi:10.1039/C8TA12293H
Li, Z., Ottmann, A., Thauer, E., Neef, C., Sai, H., Sun, Q., et al. (2016). A facile synthesis method and electrochemical studies of a hierarchical structured MoS2/C-nanocomposite. RSC Adv. 6 (79), 76084–76092. doi:10.1039/C6RA11214E
Li, Z., Ottmann, A., Zhang, T., Sun, Q., Meyer, H.-P., Vaynzof, Y., et al. (2017). Preparation of hierarchical C@MoS2@C sandwiched hollow spheres for lithium ion batteries. J. Mat. Chem. A Mat. 5 (8), 3987–3994. doi:10.1039/C6TA10439H
Liu, C. L., Bai, Y., Zhao, Y., Yao, H., and Pang, H. (2020). MoS2/graphene composites: Fabrication and electrochemical energy storage. Energy Storage Mater. 33, 470–502. doi:10.1016/j.ensm.2020.06.020
Liu, Y. M., Zhang, C. L., Cui, J. H., and Wei, W. (2020). Graphene enwrapped molybdenum disulfide for long life rechargeable batteries. Mat. express 10, 1358–1363. doi:10.1166/mex.2020.1748
Long, F., Chen, Y., Wu, C. H., Wang, J. L., Mo, S. Y., Zou, Z. G., et al. (2014). Unique three-dimensional hierarchical heterogeneous MoS2/graphene structures as a high-performance anode material for lithium-ion batteries. IONICS 27, 1977–1986. doi:10.1007/s11581-021-03936-y
Novoselov, K. S., Geim, A. K., Morozov, S. V., Jiang, D., Zhang, Y., Dubonos, S. V., et al. (2004). Electric field effect in atomically thin carbon films. Science 306, 666–669. doi:10.1126/science.1102896
Novoselov, K. S., Jiang, D., Schedin, F., Booth, T. J., Khotkevich, V. V., Morozov, S. V., et al. (2005). Two-dimensional atomic crystals. Proc. Natl. Acad. Sci. U. S. A. 102, 10451–10453. doi:10.1073/pnas.0502848102
Ottmann, A., Zakharova, G. S., Ehrstein, B., and Klingeler, R. (2015). Electrochemical performance of single crystal belt-like NH4V3O8 as cathode material for lithium-ion batteries. Electrochim. Acta 174, 682–687. doi:10.1016/j.electacta.2015.06.027
Ren, W., Zhang, H., Guan, C., and Cheng, C. (2017). Ultrathin MoS2Nanosheets@Metal organic framework-derived N-doped carbon nanowall arrays as sodium ion battery anode with superior cycling life and rate capability. Adv. Funct. Mat. 27 (32), 1702116. doi:10.1002/adfm.201702116
Ren, W., Zhou, W., Zhang, H., and Cheng, C. (2017). ALD TiO2-coated flower-like MoS2 nanosheets on carbon cloth as sodium ion battery anode with enhanced cycling stability and rate capability. ACS Appl. Mat. Interfaces 9 (1), 487–495. doi:10.1021/acsami.6b13179
Stephenson, T., Li, Z., Olsen, B., and Mitlin, D. (2014). Lithium ion battery applications of molybdenum disulfide (MoS2) nanocomposites. Energy Environ. Sci. 7, 209–231. doi:10.1039/C3EE42591F
Tang, Y., Wu, D., Mai, Y., Pan, H., Cao, J., Yang, C., et al. (2014). A two-dimensional hybrid with molybdenum disulfide nanocrystals strongly coupled on nitrogen-enriched graphene via mild temperature pyrolysis for high performance lithium storage. Nanoscale 6 (24), 14679–14685. doi:10.1039/C4NR05519E
Teng, Y., Zhao, H., Zhang, Z., Li, Z., Xia, Q., Zhang, Y., et al. (2016). MoS2 nanosheets vertically grown on graphene sheets for lithium-ion battery anodes. ACS Nano 10 (9), 8526–8535. doi:10.1021/acsnano.6b03683
Wang, J., Ma, F., and Sun, M. (2017). Graphene, hexagonal boron nitride, and their heterostructures: Properties and applications. RSC Adv. 7, 16801–16822. doi:10.1039/C7RA00260B
Wang, W. W., Guo, S. Z., Zhang, P. L., Liu, J. Z., Zhou, C. C., Zhou, J. J., et al. (2021). Interlayer expanded MoS2/nitrogen-doped carbon hydrangea nanoflowers assembled on nitrogen-doped three-dimensional graphene for high-performance lithium and sodium storage. ACS Appl. Energy Mat. 4, 5775–5786. doi:10.1021/acsaem.1c00609
Wieting, T., and Verble, J. (1971). Infrared and Raman studies of long-wavelength optical phonons in hexagonal MoS2. Phys. Rev. B 3, 4286–4292. doi:10.1103/PhysRevB.3.4286
Wu, M., Xia, S., Ding, J., Zhao, B., Jiao, Y., Du, A., et al. (2018). Growth of MoS2 nanoflowers with expanded interlayer distance onto N-doped graphene for reversible lithium storage. ChemElectroChem 5 (16), 2263–2270. doi:10.1002/celc.201800520
Xiong, P., Ma, R., Sakai, N., Nurdiwijayanto, L., and Sasaki, T. (2018). Unilamellar metallic MoS2/graphene superlattice for efficient sodium storage and hydrogen evolution. ACS Energy Lett. 3 (4), 997–1005. doi:10.1021/acsenergylett.8b00110
Xu, M., Wu, T., Qi, J., Zhou, D., and Xiao, Z. (2021). V2C/VO2 nanoribbon intertwined nanosheet dual heterostructure for highly flexible and robust lithium–sulfur batteries. J. Mat. Chem. A Mat. 9, 21429–21439. doi:10.1039/D1TA05693J
Yang, L., Wang, S., Mao, J., Deng, J., Gao, Q., Tang, Y., et al. (2013). Hierarchical MoS(2)/polyaniline nanowires with excellent electrochemical performance for lithium-ion batteries. Adv. Mat. 25 (8), 1180–1184. doi:10.1002/adma.201203999
Zhang, F., Tang, Y., Liu, H., Ji, H., Jiang, Ch., Zhang, J., et al. (2016). Uniform incorporation of flocculent molybdenum disulfide nanostructure into three-dimensional porous graphene as an anode for high-performance lithium ion batteries and hybrid supercapacitors. ACS Appl. Mat. Interfaces 8, 4691–4699. doi:10.1021/acsami.5b11705
Zhang, X., Li, X., Liang, J., Zhu, Y., and Qian, Y. (2016). Synthesis of MoS2@ C nanotubes via the kirkendall effect with enhanced electrochemical performance for lithium ion and sodium ion batteries. Small 12 (18), 2484–2491. doi:10.1002/smll.201600043
Zhang, Z., Zhao, H., Teng, Y., Chang, X., Xia, Q., Li, Z., et al. (2018). Carbon-sheathed MoS2 nanothorns epitaxially grown on CNTs: Electrochemical application for highly stable and ultrafast lithium storage. Adv. Energy Mat. 8 (7), 1700174. doi:10.1002/aenm.201700174
Zheng, L. X., O’Connell, M. J., Doorn, S. K., Liao, X. Z., Zhao, Y. H., Akhadov, E. A., et al. (2014). Ultralong single-wall carbon nanotubes. Nat. Mat. 3, 673–676. doi:10.1038/nmat1216
Keywords: molybdenum disulfide (MoS) 2, graphene, lithium-ion batteries, electrochemistry (113), anode
Citation: Wenelska K, Adam V, Thauer E, Singer L, Klingeler R, Chen X and Mijowska E (2022) Fabrication of 3D graphene/MoS2 spherical heterostructure as anode material in Li-ion battery. Front. Energy Res. 10:960786. doi: 10.3389/fenrg.2022.960786
Received: 03 June 2022; Accepted: 14 July 2022;
Published: 29 August 2022.
Edited by:
Fei Zhang, The Chinese University of Hong Kong, Shenzhen, ChinaReviewed by:
Lingqi Huang, Zhejiang University of Science and Technology, ChinaTong Liu, Hong Kong Polytechnic University, Hong Kong SAR, China
Copyright © 2022 Wenelska, Adam, Thauer, Singer, Klingeler, Chen and Mijowska. This is an open-access article distributed under the terms of the Creative Commons Attribution License (CC BY). The use, distribution or reproduction in other forums is permitted, provided the original author(s) and the copyright owner(s) are credited and that the original publication in this journal is cited, in accordance with accepted academic practice. No use, distribution or reproduction is permitted which does not comply with these terms.
*Correspondence: K. Wenelska, a3dlbmVsc2thQHp1dC5lZHUucGw=