- 1UK Centre for Carbon Dioxide Utilisation, Chemical & Biological Engineering, The University of Sheffield, Sheffield, United Kingdom
- 2The University of Sheffield, Sheffield, United Kingdom
To stand a chance of achieving net zero greenhouse gas emissions and in the implementation of UN Sustainable Development Goals society must move away from being consumers of carbon to being custodians. While SDG 7 focuses on clean energy, chemicals and materials are themselves energy, energy stored in chemical bonds. Behavioural change is needed to appreciate the societal value of carbon and to recycle carbon already present in the environment, so extracting more fossil carbon from the Earth is avoided. Society needs to develop new technologies such as carbon capture and utilisation to create value-added products from what is otherwise waste. To do this effectively, the social impact of change must be considered, its effect on the environment, and whether this transition makes economic sense. If there is social injustice, then new approaches are needed. If there is no environmental benefit, then interventions must be reconsidered. This becomes an iterative process seeking to achieve the best-balanced scenarios. As new technologies develop, interventions by governments providing aid to subsidise and accelerate new technologies will be needed. Care must be taken to ensure fiduciary duty is applied so the best possible use of public money is delivered. In this paper a systems approach is taken in developing a new circular carbon economy, where models are developed to include lifecycle, techno-economic, and social impact assessment studies into any policy development and commercialisation plans. It is vitally important to develop this methodological thinking early in that planning phase to avoid serious errors that could be costly financially, socially and environmentally. In early stages of development, a coarse-grained approach is required focusing on hotspot analysis. Once hotspots have been identified, finer grained analysis can be undertaken to develop rational approaches to process and policy development. It is vitally important that all disciplines are included within the development of such models, relying not only on engineers and scientists, but also social scientists, psychologists and financial experts. If such an approach is developed now, there is a good chance of identifying acceptable pathways to achieving sustainable development goals. This paper addresses gaps in the CO2 utilisation where social and fiscal issues are often overlooked.
Introduction
The planet is suffering environmentally and financially for many reasons. These include the Climate Emergency, as a consequence of increasing global greenhouse gas emissions (NOAA Global Monitoring Laboratory, 2021); financially due to worldwide recession; increasing socio-political instabilities and crises. As these issues evolve, there is a growing concern this will have significant social impacts, including health, food and energy poverty, transport inequalities and an ever-widening gap between high and low socio-economic groups. Policy for carbon capture, utilisation and storage (CCUS) was developed within the Mission Innovation recommendations (Mission Innovation, 2018) which explicitly included carbon dioxide utilisation, or conversion, as a key feature of climate change mitigation strategy (IPCC, 2021). This Hypothesis and Theory paper considers interventions and change needed to embed circular methodologies within an extended carbon supply chain. While technological development is needed to create a vehicle for change, we will look at factors including the sustainability of carbon without using fossil reserves, security of supply and the balance between the environment, the economy and society. It is recognised that the cost of recycled carbon chemicals will be greater than current fossil derivatives and so will need subsidies to levelise the costs to the end user. This is likely to initially come from fiscal interventions by governments and so their fiduciary duty is discussed. Furthermore, a degree of behavioural change is required to adopt circularity, so this is also considered in the full system. Each is discussed in separate sections to build possible future scenarios.
Sustainable carbon within a new carbon cycle
There has been considerable debate regarding the characterisation of carbon in a sustainable environment. Issues have been blurred with the drive towards biomass as a sustainable fossil alternative. It is worth remembering that policies look at carbon cycles. Fossil carbon is derived from biomass, formed over millions of years. Likewise, biomass can be formed from fossil carbon, through burning of fossil fuels then photosynthetic conversion of the CO2 produced together with a source of hydrogen, in this case protons from water splitting using solar energy. Likewise, synthetic fuels can be produced from captured CO2 and water in Power to X (PtoX) technologies, where the conversion energy comes from sustainable low carbon sources such as wind or solar, and in this case molecular hydrogen as the CO2 reductant over a catalyst.
Where is the distinction made between biomass carbon and fossil carbon? Distinction cannot be made as we are looking at carbon, albeit in different regions of multiple related cycles. For sustainable fuels, the main concern is the cycle size: the time to complete one cycle. The formation of fossil carbon from biomass has taken millions of years. However, it only takes minutes to take it back to CO2 through combustion. Similarly, crop-based biomass will take several months to grow, and in the case of trees typically 50–100 years. PtoX fuels on the other hand can be produced in hours. If CO2 is captured and reduced catalytically back to PtoX-fuel the cycle is shortened and can make a bigger impact on mitigating against CO2 emissions. This will require energy and so will add process costs. These costs can be reduced by creating excess low-carbon energy and using this surplus to produce e-fuels, which are much easier to store long-term than electricity.
In Figure 1, there is no chemical distinction between fossil carbon and biomass carbon after combustion. Carbon, in the form of CO2 is emitted to the atmosphere, delivering the same global warming potential (GWP) independent of the combustion source. Where the distinction is made is that nth-generation carbon can be reused in a new carbon cycle and so can be distinguished as renewable carbon (r-C). If r-C is used to produce new carbon-based fuels, materials or biomass the carbon becomes part of the cycle and so no new fossil carbon needs to be extracted. New fossil carbon is avoided, so does not enter the already carbon-saturated supply chain. While energy is needed to create these new products, the carbon intensity is reduced on the second and subsequent uses and significantly decreases the more times the cycle is completed. It is important to understand that in terms of net zero it is not necessary for the same carbon atom to be incorporated into the cycle, as for carbon accounting it can be another equivalent of carbon emitted through other means (CO2eq rather than CO2).
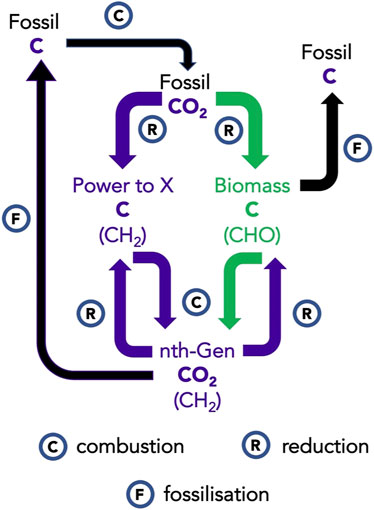
FIGURE 1. A new carbon cycle. Once nth-generation carbon is achieved there is no distinction between fossil carbon and biomass carbon: it is simply recycled carbon (r-C).
PtoX-fuels will allow us to make a transition towards Net Zero significantly faster than relying on process electrification or by storing emitted and captured carbon. This approach produces value-added carbon and new beneficial fuel types. Direct air capture (DAC) can be achieved but the expense will be such that it will likely make storage and even utilisation prohibitively expensive. It is now becoming well-recognised that the cost of e-fuel will increase relative to the counterfactual fossil fuels. However, PtoX-fuels can contribute to creating an energy economy. We will need to reduce our reliance on energy and this can only be achieved through behavioural change, discussed later.
Moving to a circular carbon economy
Sustainability and the transition to net zero will fundamentally require that existing linear economies (resources being consumed and eventually discarded along with their by-products) are redeveloped and redesigned towards circularity. Within a new increasingly circular economy, recycling and reusing of all the resources found within all products, and their by-products, must dominate the overall supply chain. Society needs to transition from recycling a fraction of total waste to recycling nearly all of it.
While reuse and recycling of precious and commodity metals, glass and some plastics are mature processes, carbon dioxide is invariably considered as a waste, if considered at all. It can be considered that practically all manufactured goods, save those made of wood or paper, have a carbon footprint with a kgCO2eq emission that will outweigh the product itself, often by a factor of 5 or more (Meinrenken et al., 2020). Given that common fundamental base materials like steel and hard plastics have embodied kgCO2eq/kgproduct factors commonly reported between 1.4 and 1.9 and 1.9–3.8 respectively perhaps this is unsurprising (Biron, 2020; World Meteorological Organisation, 2010). Some high intensity products like laptop computers are outweighed by a CO2 footprint 100–200-fold, depending on make and model (Circular Computing, 2021).
While these CO2eq figures naturally include other gases such as methane and nitrogen oxides, most of the footprint is CO2 itself, so achieving sustainability and circularisation of major economies in a new sustainable carbon cycle must involve using this CO2 wherever it is released. CO2 must be recognised as a resource rather than a waste, with its use prioritised over the consumption and exploitation of new fossil carbon.
If CO2 is used as a resource to contribute to circularising future economies, three conditions need to be met. The CO2 must be made available, be used or otherwise recycled, and must be accounted for with full and sensible tracking of wider social, economic and climate impacts. For the first part, CO2 must be collected or otherwise processed into a form which is usable and valuable. This will involve carbon capture where carbon dioxide is separated from a mixed gas stream that would otherwise be considered waste (flue gas). For utilisation, the focus should be on parts of the future economy that cannot be easily decarbonised, and that could use a significant proportion of net CO2 emissions. Care must be taken when considering also what energy inputs may be required for the utilisation, including hydrogen, and the associated knock-on effects including both emissions associated with those resources and changes to the complete supply chain as linear resource use is reduced (von der Assen et al., 2015). Finally, to properly account for the CO2 and sustainability of any approach involves looking at the entire impact pathway that the carbon has. Full, transparent Life Cycle Assessment (LCA) must be integrated with Techno-economics and Sustainability Analysis. In addition, cost, responsibility, fiduciary duty and supply chain considerations must be made with appropriate systemic thinking, and a clear choice of realistic boundary conditions. Only with such considered approaches can the existing linear economies be flexed and finally remade as circular.
Energetics and practicalities of CO2 capture
Direct air capture (DAC)
Full circularisation is impossible without the capture of CO2, particularly that which must be accounted as resulting from industries and processes that cannot be fully decarbonised within climate-relevant timescales (Rissman et al., 2020). Carbon capture, in all its forms, is also constrained by both theoretical and practical limitations. Separating any mixture of compounds requires the consumption of energy to counteract the reduction in entropy resulting from the separation. In the context of carbon dioxide, this energy will be further dependent on the concentration of the carbon dioxide in the gas stream. The lower the concentration of CO2, the higher the overall energy cost and the larger the volume of air required to be treated. This makes the capture of carbon dioxide directly from air (DAC) the most fundamentally energy-intensive method of carbon capture despite being the simplest way to directly offset emissions and the only way to capture emissions without proximity to, and direct interaction with, an emitter.
One way this can be shown is by comparing the energy generated by the formation of one tonne of CO2 from carbon against the minimum and expected energy costs of DAC, which must be sourced from low carbon energy. In effect, this calculates the net energy released or used in combustion, and capture of the CO2 before even considering how the CO2 could be reused, recycled or disposed. This method calculates at what point more energy is spent cleaning up the carbon dioxide than the production of the carbon dioxide through combustion.
The full free energy of formation of CO2 under ambient conditions is 8.96 GJ/tCO2, reducing to 2.96–4.48 GJ/tCO2 when the thermal efficiency of typical power generation, as the most common form of emission, is considered (33%–50%) (Nakagaki, 2021). While the theoretical minimum energy cost to separate CO2 from air is even lower than these figures, just 0.47 GJ/tCO2, even the best-performing laboratory-scale air capture processes operate with several times that energy cost at 1.5–2.1 GJ/tCO2 often measuring only the simple sorption/desorption energy and not including any other process cost or process inefficiency (Nagasawa et al., 2009; Lackner, 2013; Sanz-Pérez et al., 2016; Meng et al., 2021). This then leaves little overall energy budget once the additional costs associated with handling huge quantities of air to yield even relatively small quantities of CO2 (a minimum of 1.3 million cubic metres of air per tonne of CO2) and the residual carbon emissions associated even with the low carbon energy that may be used are included. This typically raises the capture energy requirements above 5 GJ/tCO2 meaning a net energy loss is made on each tonne of carbon captured (McQueen et al., 2021; NREL, 2021).
The practical result is the energy needed to achieve DAC would frequently be better used to supplant existing fossil fuel-based energy generation, avoiding emission in the first place, apart from in situations where the energy cannot otherwise be used due to practical limitations, or the hypothetical future scenario where fossil fuel combustion has completely ended. One example of waste energy utilisation would be through regenerative braking systems on North American transcontinental rail car systems as reported by Bachman, et al. (2022), where this otherwise-unrecoverable energy is used by a compact air capture process mounted on an additional car as detailed further in the extensive Supplementary Material. The limitation of this approach, and others involving such marginal energy sources, such as curtailed or isolated renewable generation, is that these energy sources are inherently niche both in location and quantity, and also are often intermittent, which significantly impacts the potential viability.
Point source capture (PSC)
Capturing carbon dioxide from a point source emitter with CO2 concentrations of 5%–40% significantly reduces both the thermodynamic minimum separation energy cost and the practical energy costs by reducing the volume of gas needed to be treated. At 40% CO2 concentration, a minimum of just 1,400 cubic metres must be processed per tonne of CO2. For flue gas emissions at 12% CO2 the thermodynamic minimum energy cost falls to just 0.12 GJ/tCO2, although as with the air capture scenarios above, real capture processes typically require significantly more energy than this minimum. While traditional liquid amine capture processes require energy consumptions of 3.1–5.4 GJ/tCO2, more recent solid-sorbent processes of various types are approaching an energy cost of around or even below 1 GJ/tCO2 (Dowson et al., 2016) with far lower additional process costs, due to the smaller gas volumes than associated with DAC (Romeo et al., 2020; Sadiq et al., 2020; Styring et al., 2021). The specific capture processes that are envisioned for these plants will vary across different sectors depending on available services, such as heat, power and water, footprint space and downstream CO2 logistics, which need to be rapidly developed to allow carbon capture to have an impact on emissions.
For any near-future timescales, relevant to efforts to limit climate change, there will remain an abundance of these point sources, both in terms of absolute CO2 emissions and high CO2 concentrations, even as renewable and low carbon energy capacity is expanded. This is especially important in hard-to-decarbonise sectors such as steel and cement manufacturing (Rissman et al., 2020). Point sources will therefore likely require carbon capture to fundamentally operate in a future “net zero” environment, which reduces the importance of the energetics considerations as emissions may be seen as otherwise unavoidable and should therefore be captured wherever the costs are lowest, both in terms of energy and process. As an additional consideration relevant to the future supply chain, many of these of industries are crucial for downstream construction to allow the future energy transition in other sectors, providing the basis for new housing stock, new energy generation facilities and transport infrastructure.
With point source capture, the captured CO2 may retain some or all of the original carbon footprint, depending on the accounting practice used. For example, if the carbon was captured from a steel production site the carbon accounting can either declare the manufactured steel from that part of the process to be carbon neutral, or the captured carbon dioxide itself as a feedstock (which may go on to be utilised as a product) is carbon neutral, but not both simultaneously. This is especially important in the context of synthetic carbon transport fuels, where captured CO2 will be released to the atmosphere after combustion. While this complicates the tracking and accounting of net emissions, there still is a net carbon benefit to allowing even new fossil carbon to be used twice before it is released to the atmosphere in these necessary processes, effectively halving the total combined footprint of the steel and the fuel individually. In the case of iron/steel manufacturing, as traditional blast furnaces turn to using carbon neutral biochar for their iron smelting, the 50% initial footprint reduction by carbon capture and recycling could be applied on top of the 18%–40% reduction through biochar usage and a new industrial symbiosis could be developed that allows for net zero to be approached (Feliciano-Bruzual, 2014).
Utilisation/carbon recycling
The second of the three conditions for circularisation is use or recycling of the captured carbon dioxide as a priority above storage and direct sequestration. This mirrors the classical simple Lansink waste hierarchy (Lansink, 2018) which must now be applied to carbon as well as other reclaimable wastes: Avoid > Reduce > Reuse > Recycle > Dispose. This approach will be returned to in a later section, considering a modern-day approach.
For carbon dioxide, this hierarchy can be seen as applying to reduction in use of fossil-powered energy in the wider economy. Re-use of CO2 would include incorporation of the carbon into long-life, value-added products such as accelerated mineralisation to carbonate construction materials, certain polymers and fine chemicals that do not involve the incorporated carbon returning to the atmosphere within a short timeframe, and do not require added energy (exothermic processes). Recycling would include the generation of synthetic fuels and other valuable polymers and fine chemicals, where the carbon utilisation requires significant energy input, often in the form of sustainably sourced hydrogen. Finally, disposal represents long-term sequestration and storage of the CO2 (CCS) (Choptiany, et al., 2014), where no further value is extracted from the carbon dioxide except direct emissions mitigation (Roh, et al., 2016).
While the “reuse” of carbon dioxide in building aggregates, fine chemicals and polymers such as polyurethane and polycarbonates represents the best mitigation strategy that can be applied to captured CO2 from industries and sectors that cannot be reasonably decarbonised within the climate-relevant timeframe, the total capacity of these products is relatively low and even in idealised scenarios will not make a large impact on total carbon emissions (Dowson and Strying, 2017). Furthermore, these approaches do not help to address the continuing need for hydrocarbon transport fuels for heavy vehicles, including haulage, maritime and aviation sectors. Synthetic carbon fuels within this “recycle” branch should therefore also be considered. This is despite such fuels being less ideal, from a net-emissions perspective, than either “reuse” or “disposal”, since they have a greater role in ensuring the financing and supply security of a “just transition” (Newell and Mulvaney, 2013).
Synthetic carbon fuels
Since the total scale of emissions that can be captured by the production of fine and commodity chemicals are limited to approximately 300 Mt/y CO2, by comparison transport fuels could potentially absorb billions of tonnes of total CO2 emissions, making realistic inroads towards decarbonisation of that otherwise challenging sector (Aresta et al., 2013). New synthetic fuels will offer both challenges in their compatibility with the existing supply chain infrastructure, but also opportunities to narrow and refine fuel performance characteristics.
Synthetic fuels do not need to be poorly defined mixtures of thousands of different compounds, but instead can be created from a small number of tightly-controlled compounds, allowing completely reliable combustion performance. In the extreme case, synthetic fuel could be a single-component fuel, where every molecule will combust in a completely predictable fashion. New fuels like dimethyl ether (DME) can be used in converted diesel engines, where they can reduce harmful nitrogen oxide emissions, and practically eliminates particulate emissions (Styring, et al., 2021). Similarly, fuels like butanol in petrol engines can achieve higher fuel mileages than the standard fuels, despite having lower energy density, due to coincidentally high combustion efficiency (Ramey and Ramey, 2005; Han et al., 2017; Putrasri and Lim, 2022). This allows new fuels to not only be less carbon-intensive but also better-performing than existing fuels, meaning quality and performance does not need to be sacrificed for sustainability. It is recognised that in terms of emissions, internal combustion engines are not the problem as their efficiency has been optimised over many decades. Rather the problem is the fossil-based fuels. In this way, synthetic fuels offer the benefit of emissions reduction in use while, if manufactured with the existing supply infrastructure in mind, requiring no or only modest modification to the engines. This means that long-life vehicles such as those found in aviation and maritime industries can be used without the need to decommission serviceable equipment as low emissions zones are established.
Significant challenges remain. Fuel production from CO2, while allowing low carbon electrification-by-proxy of otherwise unavoidable emissions, requires enormous amounts of low carbon energy to produce useful quantities of fuel. In addition to this, the use of these fuels and their associated carbon footprints within the whole lifecycle and their place inside future supply chains needs to be clearly calculated and defined.
Security of supply and supply chain
The discussion of decarbonised or circular supply chains is one that runs deeply through the production of nearly all products, services, and industries, independent of the form in which the product is found. The scope, definition, and security of these new decarbonised supply chains must all be considered thoroughly before implementing industrial change. Once a product’s supply chain is defined, decarbonised alternatives of both energy and mass balances can be considered. Quantifying how these changes will impact the waste and emission profiles of each supply chain constituent. This also allows for the granular assessment of supply chain security, both in the proposed and benchmark scenarios; ultimately facilitating deeper understanding around the viability of decarbonised supply chains in the context of extended time periods.
Supply chain clarification - Scope
When considering the security of a decarbonised, circular carbon supply chain, many characteristic challenges arise. A significant number of these are inconsequential within linear alternatives. These can vary based on the scope of assessment in question. The GHG protocol defines three emission scopes, each building upon the prior with respect to the breadth of the supply chain’s system boundaries. These are summarised in Table 1.

TABLE 1. Definitions of the three emission scopes outlined by the GHG protocol (Barrow et al., 2013).
Inventory breakdown
In order to identify carbon hotspots, whether considering scope 1, 2 or 3 emissions, the theory of supply chain breakdown remains consistent with the breadth of system boundaries being the only key variable. As a means of illustration, an inventory analysis of an example product is outlined in Figure 2, with the aim of understanding which section(s) of the supply chain hold the greatest barriers with respect to production circularity. By taking a subject product which is to be assessed, a practitioner can work backwards from the product to form a “tree diagram”, displaying the overall supply chain and its constituent material and energy flows. This method is outlined in greater detail by Platt and Styring (2022) with a focus on feedstock defossilisation.
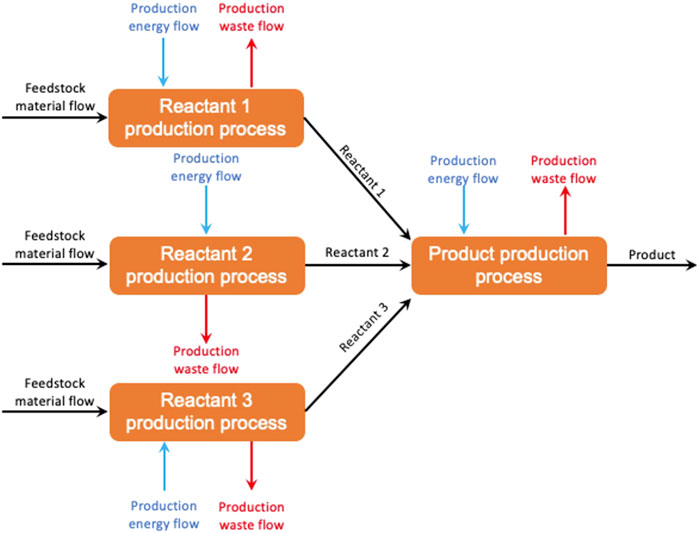
FIGURE 2. A sample supply chain tree diagram for an undefined product, with simplified material and energy flows (reproduced with permission from Platt and Styring, (2022)).
The layout of material and energy flows (Figure 2) allows each individual process to be treated as a “black box”, meaning each process can be considered independently in order to find any carbon hotspots, whether in the form of carbon-containing feedstocks or energy sources. Within a real-world scenario, the inventory for each material and energy flow will be broken down into individual components, providing an overall inventory for the subject product. With a supply chain tree diagram and accompanying inventory data, the detection of carbon hotspots should be viable based on process data scaled to a defined functional unit. Once hotspots have been sourced, the substitution of carbon intense processes or flows can be approached, implementing circular or decarbonised alternatives, easing the overall supply chain towards a fully decarbonised state. The resulting supply chain can then be assessed against the original, either through life cycle assessment or counterfactual screening methods.
Supply chain security
With any scenario, the understanding and assessment of security is vital for the implementation of circular supply chains. When assessing security, the timeframe in which materials technologies are available is one of the most important factors. An example within a linear, present-day setting could be the sourcing of hydrogen through steam methane reforming, exhibiting a strong reliance on fossil-derived natural gas. Through a variety of factors, whether geopolitical, economic, or simply resource depletion, the security of supply is almost always under some form of threat. As supply chains progress towards circularity, security will inevitably increase; a consequence of adopting greater internal recycle and re-use rates and moving away from materials with detrimental, “outside” influences. Circular supply chains also harness the benefits of a shorter chain than the linear alternatives, with spent material repurposing usually requiring far fewer raw materials, offshore manufacture, and subsequent distribution. This in turn reduces the overall threats on the supply chain, with a shorter supply chain acting as a smaller target for compromise.
As circular routes to platform chemicals are becoming ever apparent, the understanding that threats to supply chain security based on location is also a key consideration. It should therefore be noted that a shift towards distribution-based supply will likely be required for global chemical demands to be met. This will allow for the variability of feedstocks for platform chemicals, based on the most locally ubiquitous, non-fossil materials.
Whilst these changes propose an attractive future, where the production and consumption of products can be innately intertwined and constructively reliant on one another, there are inevitable trade-offs and roadblocks to address. Regarding material quality, the well-documented issue of downcycling must be addressed if circular supply chains are to remain in perpetuum (Gallego-Schmid et al., 2020). This will likely come in the form of a complete redesign of sections or entire supply chains in order to stop the decline of product quality as cycles continue.
For energy, the transition to a circular economy can in turn benefit supply chain security, though the demand for energy is still present within circular alternatives. Fortunately, the decarbonisation of energy sources has been shown to increase energy platform resilience, with a renewable energy portfolio providing a “silver buckshot” scenario. With a renewable energy supply comes a supply which is sourced through a variety of methods, a reliable energy supply can be provided as different technologies can take the slack if required. This was described by the National Renewable Energy Laboratory as providing a “smoothing effect” that “decreases vulnerability to disasters” (Cox et al., 2016).
To best reap the benefits of these energy supplies however, issues of energy storage must also be addressed. Whilst multi-source grid mixes go a considerable way in addressing the “smoothing effect” of intermittency, it cannot necessarily guarantee a sustainable, consistent energy supply. Energy storage looks to address this issue by storing excess energy at times of relatively low demand, for later use at times of higher demand (or lower supply). Whilst research efforts into energy storage are substantial, a lack of commercial viability is currently inhibiting it from full deployment (Koohi-Fayegh and Rosen, 2020).
A triple helix approach: environment, economics, and society
Increasing public demands have led to investment and shifts towards the responsible sourcing of goods, services, and by extension, chemicals feedstocks within supply chains. As previously mentioned, sustainability goes beyond purely environmental assessment; rather, it can be viewed as a multi-faceted problem. Three distinct, yet interconnected aspects are present. Consequently, this necessitates a holistic approach to process design and selection, considering the environment, economics, and society. In order for the chemical industry, to establish a truly sustainable status quo, none of these can be neglected. While recognition of such an approach is not novel, being suggested from 2007 onwards (Remmen et al., 2007; Kloepffer, 2008), the field has largely stagnated in its infancy. Improved practicality and harmonisation of methodologies offers a powerful tool in the transition towards sustainable societies and widespread utilisation of carbon dioxide. Numerous methodologies have been presented for individual ‘strands’. However, many diverging schools of thought are present, resulting in a deep lack of consensus around methodological approach (Remmen et al., 2007; Kloepffer, 2008).
Carbon dioxide utilisation (CDU) technologies require holistic assessment in order to manage and quantify burden shifts; occurring when improvements in one strand of sustainability catalyse detrimental effects in another. A pertinent example from another field being the transition to EVs for personal transport. While benefits can be seen through environmental metrics (European Environment Agency, 2008; Ricardo Inc., 2022), there is risk of producing a ‘mobility underclass’ through high prices and access to private off-street charging facilities. Lower income households, usually commanding no off-street parking, could expect to pay £20 per charge or ‘tank’ via commercial charging points; almost a threefold increase on the £7 realised by private charging (Witchalls, 2018). In this example, holistic consideration of road transport’s sustainability profile may provide compelling arguments for transition towards CO2 derived synthetic fuels (Newman and Styring, 2022).
Beyond burden shifts, holistic assessment delivers broader insights into the impacts of processes or value chains, highlighting otherwise overlooked issues. McCord et al. (2021) identify an example of this in gold value chains. Certifiably ‘sustainable’ gold from the Peruvian Highlands was subsequently assessed holistically, revealing large social issues such as organized crime, human trafficking, and underage sex work.
Robust sustainability assessment of CDU technologies is a requirement if CO2 is to realise its potential as a key commodity within circular economies. Favourable economics of capture and utilisation must be verified, while simultaneously ensuring associated environmental impacts are less severe than those of relevant benchmarks, and in a manner that does not jeopardise a socially ‘just-transition’.
Independent assessments
The publication rate within independent assessment strands gives useful insights to methodology maturity and perceived utility. Web of Science is used to quantify these rates by year between 2000–2021. Review papers are not removed owing to the quantity of publications returned. Searches required either the full assessment name or abbreviation listed as a keyword (e.g., Lifecycle Assessment or LCA).
Large bodies of work are seen around each aspect of the triple helix in abstraction. Life Cycle Assessment (LCA), the oldest of the three strands, emerged in the 1960s (Guinée, 2012), generating what would nowadays be considered partial LCAs; following comparative approaches with no broadly accepted methodology (Newman & Styring, 2022). In 2006, ISO released the 14 K series of standards (ISO Technical Committee 207, 2006), introducing a rigorous approach to assessments. Despite further development upon ISO 14 K (European Commission Joint Research Centre Institute for Environment and Sustainability, 2010), CDU specific iterations proved elusive until the Global CO2 Initiative (GCI) published guidelines specific to the sector in 2018 (Zimmermann, et al., 2018), succeeded by a second iteration in 2022 (Müller, et al., 2022). LCA publications grew from 11% of sustainability assessment publications to 28% between 2000 and 2021 based on the data collected for Figure 3. This is likely explained by growing emphasis on environmental protection seen in the period, owing partially to reports from bodies such as IPCC and the World Meteorological Organisation revealing record high annual and decadal temperatures (World Meteorological Organisation, 2010; IPCC, 2021). International treaties such as the Paris Agreement added additional legislative pressure internationally (United Nations/Framework Convention on Climate Change, 2015), pushing industry to quantify and reduce their impacts through LCA.
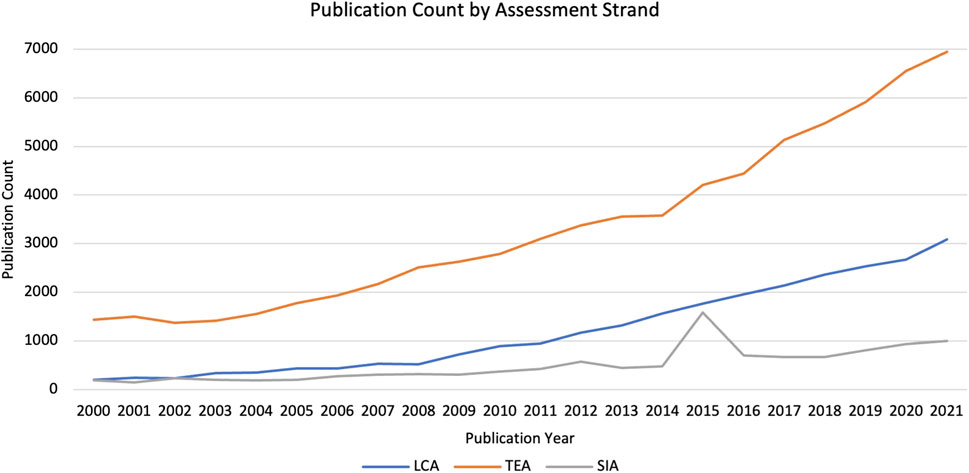
FIGURE 3. Number of publications listing each independent assessment type or its abbreviation as a keyword, collated by year. Data collected using Web of Science.
Techno-economic assessment (TEA) is historically less standardised than LCA, lacking broad consensus on best practice (Zimmermann, et al., 2020; Roh, et al., 2016). This variation in approach delivered assessments based on diverse, and sometimes erroneous, methods and assumptions. However, this did not prevent the publication of large numbers of studies, as shown in Figure 3; likely a consequence of the importance of results within industrial decision making. GCI approached the TEA standardisation problem through the adoption of a now mainstream four phase assessment structure, originally proposed within ISO 14040 (ISO Technical Committee 207, 2006); forming CDU oriented guidelines aligned with those for LCA (Zimmermann, et al., 2018). Within Figure 3, TEA sees a drop in share of sustainability assessment publications, falling from 79% in 2000 to 63% in 2021.
The third societal strand, SIA or social-LCA (S-LCA), maintains a 10% share of sustainability assessment publications between 2000–2021; remaining in its infancy when compared to both LCA and TEA (Klöpffer, 2003; Jørgensen, et al., 2007; Traverso, 2018; Huarachi, et al., 2020; Pollok, et al., 2021). This could be attributed to its relatively recent first literature appearance in 1996 (O'Brien, et al., 1996), making it LCA’s junior by approximately 3 decades. Many organisations evaluate and report social impacts using the Global Reporting Initiative (GRI) standards (Stiching Global Reporting Initiative, 2021) or UN Sustainable Development Goals (SDGs) (United Nations, 2015). However, this is typically limited to the assessment of deployed activities, restricting its use around low the TRL processes seen in CDU. The United Nations Environment Programme (UNEP) and the Society of Environmental Toxicology and Chemistry (SETAC) jointly developed general guidelines for SIA. While adopting an ISO derived structure, many discrepancies persisted around more granular procedures, most notably impact characterisation (Newman & Styring, 2022). No standards are present for CDU specific application, leaving a single framework, intended for implementation alongside the GCI’s LCA and TEA guidelines (McCord, et al., 2021). A similar but smaller increase in publication rate can be seen for SIAs from 2012 onwards (Figure 3), reaching one thousand publications in 2021.
The pursuit of harmonisation
Harmonisation of assessment strands primarily targets the homologation of methodological structure and procedure, resulting in more comparable and reproducible assessments. Such an approach within the field has been called for in numerous papers (Zimmermann & Schomäcker, 2017; McCord, et al., 2021; Wunderlich, et al., 2021), driving increased efforts in the latter half of the 2010s.
There has been debate of the relative importance of social, environmental, and economic aspects of decision making in assessing low carbon technologies. While the process should be economically viable (although not necessarily cheaper than the counterfactual petrochemical process), it must be environmentally advantageous, otherwise change becomes unnecessary. If the new process results in detriment to social structure, then it cannot be accepted. The transition to electric vehicles (EVs) is an example. While the move to EVs may seem beneficial at face value, as discussed earlier, there is a fear that the transition will be beyond the reach of low-income groups and so a new social underclass may arise where travel becomes the beyond their means. It is proposed that the order of assessment should be in the sequence of SIA → LCA → TEA with iterative decision making at each stage, arriving at the best outcome. Figure 4 shows the rational decision process that should be employed.
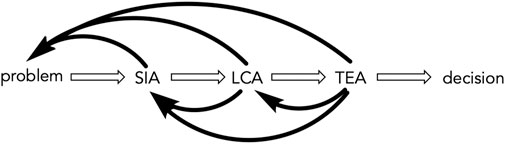
FIGURE 4. The proposed decision-making process in a triple-helix approach to process development in a circular carbon economy.
The most significant step towards harmonisation is the inter-strand adoption of the ISO four phase structure. GCI deliver this for CDU applications through both their LCA and TEA guidelines (Zimmermann, et al., 2018) (Müller, et al., 2022). The GCI TEA guidelines extend to the provision of various characterisation methods for indicators, a first attempt within the strand.
SIA, being the least mature of the methodologies, presents numerous barriers to alignment, persisting into UNEP and SETAC’s offering (UNEP, 2020). Where LCA and TEA impact indicators are quantified though the use of characterisation models, these are not yet developed for SIA. Furthermore, the presence of mixed data types is a significant challenge when targeting an objective numerical result.
McCord et al. (2021) approached the issue of data handling within the triple helix framework. A method was proposed of quantifying indicators against a practitioner derived scoring scale. While failing to allow for comparisons between assessments owing to differentiated scales, it does enable comparative evaluation within a given study. This facilitates adoption of the ISO pioneered four-phase methodology.
When considering the GCI guidelines and triple helix framework, the assessment strands can be considered harmonised in terms of structure and procedure. Additionally, practitioner guidance around setting of system boundaries and application of assumptions has further harmonised assessments within the CDU space. Key pitfalls seen in literature, such as the misconceptions of zero cost hydrogen and surplus green electricity, are also recognised and tackled by GCI.
Integrating strands
The assessment strands have historically been evaluated in isolation. However, more recently value has been recognised in the development of integrated methodologies, allowing for the parallel evaluation of the strands under a single assessment format. Meaningful integration relies heavily upon the success of precursing harmonisation efforts. If the methodological phases and approaches exhibit large variation, cross-linkages are difficult to establish. Common data inventories should be targeted within integrated frameworks, improving both results quality and required workload.
GCI promote the integration of their independent assessment, offering detailed practitioner guidance (McCord, et al., 2018). Methodological decisions are supported, such as the setting of a combined goal and scope, aggregating the components present in the independent strands. Several options for deeper integration are examined, notably through ‘eco-enviro’ indicators. These allow for examination of trade-offs between strands, e.g., cost of CO2 avoided.
With the triple helix largely harmonising SIA to the GCI guidelines, integration can be approached. Common LCA and TEA data inventories can be augmented to include data-points required for social indicators. Deployment of scoring scales as ‘pseudo-characterisation models’ delivers quantitative social impact results, allowing comparison and analysis alongside the LCA and TEA strands. The integrated results handling enables use of both aggregation and MCDA techniques, valuable tools in terms of both decision-making support and communicability.
While the foundations for integration are present, several details continue to divide opinion. For example, assessment of competing indicators adds subjectivity to the results. At what point are improved environmental impacts negated by increased OpEx or CapEx? Or balancing high working wages with OpEx? Integration generates multivariate problem spaces that are complex to optimise. While this unavoidably adds subjectivity to the field, it is reflective of the reality of the transition to a sustainable society and industrial ecosystem.
State of the art
For holistic approaches to sustainability assessment of CDU projects, the triple helix framework is the currently the only CDU focussed holistic sustainability assessment methodology. Its development can be traced back to the ISO standards around which strands are harmonised (Figure 5). Building upon the already tested GCI LCA and TEA guidelines for CDU, a robust methodological core is delivered. Practitioners are supported through decisions that are highly sensitive in CDU applications; examples including functional unit selection, feedstock pricing and CCU system boundaries. Enough flexibility is left to ensure the frameworks applicability to a vast range of CDU projects and scopes, spanning low to high TRLs.
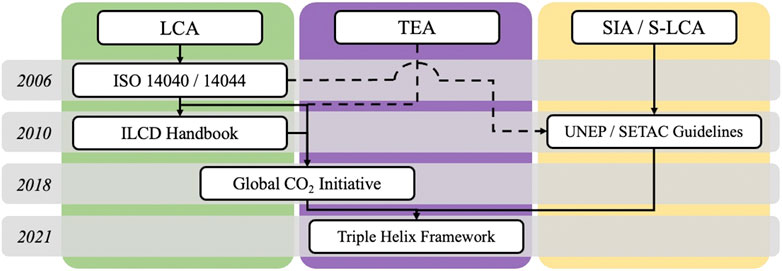
FIGURE 5. Taxonomy of standards, guidelines, and frameworks leading to a holistic sustainability assessment for CDU applications (Newman & Styring, 2022).
McCord et al. (2021) modify the indicators selected by UNEP and SETAC to generate a more CDU specific approach. Several stakeholder groups are dismissed due to irrelevance to CDU technologies. As a result, a more streamlined and practical assessment strand is delivered. Despite this, the practitioner led approach to SIA impact characterisation leads to less robust and reproducible results. As a result, the SIA outlined in the triple helix framework generates a ‘hot spot’ analysis rather than a full assessment. Due to the inherent uncertainties around social impact pathways, it is unlikely a directly comparable approach will be found to the characterisation methods seen in LCA and TEA; therefore, should act as part of a ‘go-no-go’ call for a technology.
A primary strength of the approach is its ability to identify burden shifts in three dimensions. When used to inform investment or development effort, it is important that negative impacts are not just optimised to a minimum in a single area; often sustainability requires trade-offs and compromise.
With qualitative results present for indicators across all strands, the triple helix is an ideal application for multi-criteria decision analysis (MCDA) which is discussed later. The triple helix framework offers a well harmonised and integrated approach to the holistic sustainability assessment of CDU projects. These key differentiators from alternative methodologies contribute to safeguarding the idea of a ‘just transition’, ensuring progression around sustainability is available to all. Inclusion of the social pillar is key in preventing negative side-effects of environmental sustainability.
Application
Triple helix-based assessments have many applications within CDU. Validation of proposed circular economies is a significant potential use. Moving industry away from traditional linear economies will require verification that environmental impacts are actually reduced, rather than transposed to another strand. Secondly, novel supply chains must be assessed for economic viability before gaining acceptance by organisations.
Green energy use is likely to be a key factor in the adoption of CDU technology. Its strategic utilisation will be necessary as many direct or secondary processes, such as electrolytic H2 production, require large quantities of energy. Consideration of green energy allocation via a triple helix approach would support its effective use within industrial ecosystems. Suitable application would be characterised by large GHG emission savings relative to benchmarks, low impact to local communities through factors such as access to energy or materials, and sustainable prices per functional unit.
Multi criteria analysis making within CDU
Multi Criteria Decision Making (MCDM) is a historically rich field, with the first recorded example dating to circa 1,011–931 BC and the biblical story of the ‘Judgement of Solomon’ (Köksalan, et al., 2013). In its more modern analytical sense, MCDM is employed to aid stakeholders in structuring decision making problems, identifying their preferences, and formulating aligned recommendations (Cinelli, et al., 2020). Prior to the rapid methodological developments of the 1960s, industry had used simple additive methods for the evaluation of competing ‘alternatives’, the theory of which was not greatly understood (Fishburn & Lavalle, 1999).
CDU as a field is, by definition, attempting to utilise carbon dioxide, combatting the still growing atmospheric concentrations which reached 414.35 ppm in August 2022 (UNEP, 2020), while generating useful commodities. Ambitious climate targets such as the Paris Agreement (United Nations/Framework Convention on Climate Change, 2015) require that the allocation of capital and R&D efforts be effective. Such complex problems necessitate the careful handling of trade-offs and non-uniform performance metric weightings. With finite capital and time available in the pursuit of climate targets such as the Paris Agreement, technologies must be vetted and their viability confirmed. This CDU oriented application effectively utilises the strengths of MCDM, handling complex trade-offs and multiple stakeholders (Cegan, et al., 2017; Gibari, et al., 2018; Katsikopoulos, et al., 2018; Greco, et al., 2019).
Without analytical approaches, decision makers would primarily rely on ‘gut feel’ or similarly unsophisticated methods. Despite introducing subjective value choices, the mathematical nature of MCDM provides a repeatable and justifiable decision-making process; ‘quietening the noise’ generated by criteria that are either unimportant or potentially rectifiable at a later stage. Previous literature reviews have highlighted the significant use of MCDM within environmental subject areas, growing four-fold from ca. 0.2% to ca. 0.8% of the field’s publications between 2000 and 2015 alone (Cegan, et al., 2017).
MCDM can greatly enhance the communicability of sustainability assessment results to non-practitioners. Results are often complex to interpret, delivering many indicator scores with incongruent units. While this granularity and specificity is invaluable to environmental scientists, how can they be effectively presented to the public or policymakers? A stakeholder with no LCA or sustainability assessment expertise can more meaningfully interpret results when presented through MCDM, often allowing for the comparison of alternatives in terms of a single score, incorporating all assessed criteria. With policymakers wielding significant influence over the pace at which climate mitigation proceeds, such a communication tool should not be undervalued.
Two fundamentally different approaches to MCDM have been precipitated; Multi Objective Decision Making (MODM) and Multi Attribute Decision Making (MADM) (Chen & Hwang, 1992; Zimmermann, 2001; Zavadskas, et al., 2019). MADM methods incorporate four main components: alternatives, criteria, the relative importance of each criterion, and measure of performance of an alternative relative to a particular criterion (Pohekar & Ramachandran, 2004). These approaches target problems that contain a finite set of possible alternatives. Conversely, MODM approaches are suitable when evaluating undefined, or continuous, alternatives; it requires users to characterise constraints in the form of vectors reflecting decision variables (Ribeiro, 1996). Within CCU applications, alternatives represent processes or supply chain structures; consequently, they are finite in number and objectively defined, necessitating the use of MADM approaches. Due to its general inapplicability to the CCU field, MODM will not be considered further within this work. While many MADM methodologies use complex mathematical principles, the overall process can be summarised in Figure 6.
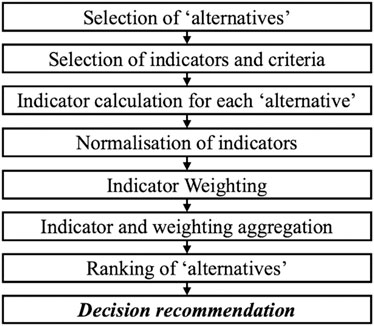
FIGURE 6. Flow diagram of a typical MCDA application (adapted from (Volkart, et al., 2016)).
CDU relevant MADM approaches
A literature review of MADM within sustainability assessments focusing on CO2 and CDU was conducted, aiming to examine current practices and methodological preferences. Web of Science was selected as the bibliographical database. Searches filtered for publication dates between 2015 and 2022, as well as identified keywords. Figure 7 shows the keywords used for each of the four rounds of searching, and the classification of each identified publication. A total of 72 publications were obtained, of which 44 are excluded. The following analysis focusses on the approaches most frequently utilised; for this reason, review papers and novel methodological proposals are excluded. Duplicates and papers with no named methodology are also removed from the process. Finally, relevance of scope is used to exclude those that do not focus on carbon management, CDU, or sustainability assessment. This reveals 28 publications for examination (a table of the literature search results can be found in the Supplementary Material).
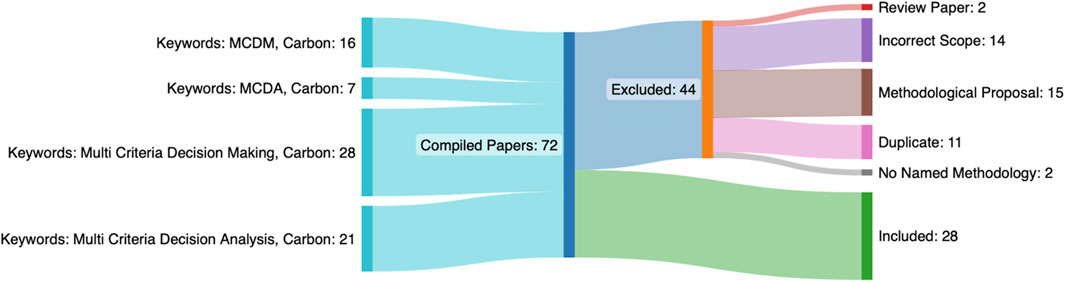
FIGURE 7. Sankey diagram showing the quantity and usage of publications returned by the systematic literature search.
Methodologies employed by the included papers were then analysed, tabulating the frequency of use. A small number of papers used hybridised approaches, using part but not all of two or more methods without further modification. In these cases, each of the incorporated methodologies are given credit for usage. The results are shown by Figure 8, with methodologies including; data envelope analysis (DEA) (Charnes, et al., 1978), quality function deployment (QFD) (Akao, 2004), weighted sum model (WSM) (introduced by Fishburn and Lavalle, (1999) and MacCrimmon (1968)), evaluation based on distance from average Solution (EDAS) (Ghorabaee, et al., 2016), stepwise weight assessment ratio analysis (SWARA) (Keršuliene, et al., 2010), complex proportional assessment (COPRAS) (Zavadskas, et al., 1994), decision making trial and evaluation laboratory (DEMATEL) (Fontela & Gabus, 1972), analytic network process (ANP) (Saaty & Vargas, 2013), élimination et choix traduisant la realité (ELECTRE) (Roy, 1968), preference ranking organization method for enrichment of evaluations (PROMETHEE) (Brans & Mareschal, 2005), viekriterijumsko kompromisno rangiranje (VIKOR) (Opricovic & Tzeng, 2004), analytic hierarchy process (AHP) (Saaty, 1980), and technique for order of preference by similarity to ideal solution (TOPSIS) (Hwang & Yoon, 1981). To allow for meaningful analysis and comparisons of methodologies, only those with more than one application case will be examined further; representing 83% of included publications.
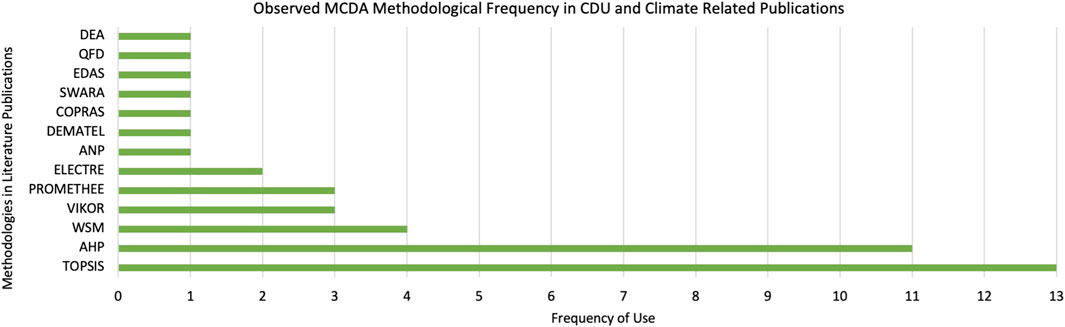
FIGURE 8. Graph to show the frequency in which MCDM methodologies were observed through literature search.
AHP and TOPSIS are the clear workhorses of CDU related MADM applications, appearing in 55.8% of publications and approximately three times more frequently than the third most common method. When the distribution in Figure 8 is compared to that in Figure 9, given by Sabaei, et al., 2015, differences are observed. It is noted that Sabaei examined MADM more broadly, choosing not to focus on applications within a specific field. However, this allows for comparison between general and CDU related applications. AHP is a frequent choice in both cases, whereas TOPSIS descends from most common in CDU related work to the least common generally, suggesting possession of characteristics advantageous within this field. Each examined method presents characteristic advantages and disadvantages, supporting rejection of a ‘one size fits all’ MADM approach. General principles, advantages, and disadvantages of each are discussed briefly before comparisons are drawn.

FIGURE 9. Number of publications for various MADM techniques (Sabaei, et al., 2015).
TOPSIS finds ideal solutions based on the closest distance to the positive ideal and farthest distance from the negative ideal. However, the relative importance of these distances is not considered through weighting (Opricovic & Tzeng, 2004; Kuo, 2017). It is capable of handling very large numbers of alternatives with high computational efficiency while possessing a clear and intuitive logic (Roszkowska, 2011). However, the issue of rank reversal is present (Çelikbilek and Tüysüz, 2020); occurring not only when duplicating an alternative, but also when a new alternative is added or an existing alternative is removed (Wang & Luo, 2009).
AHP focusses primarily on hierarchical decision problems (Sabaei, et al., 2015), using decision maker value choices to mathematically determine criteria weights through matrices. Through this it allows for finer control and monitoring of decision-making consistency (Saaty, 1986; Saaty, 1994), while facilitating group decision making (Lee, et al., 2013; Tsai, et al., 2013). Due partially to this capability, it is suited to the allocation of resources and business effort (Mu, 2006), problems in which many stakeholders are usually present. Furthermore, it is appropriate for the integration of qualitative data (Saaty, 1977; Sabaei, et al., 2015) using practitioner derived scoring scales. While weighting derivation is a characteristic strength of AHP, it can have significant influence on final scores or recommendations (Baker, et al., 2001), potential mitigation strategies are explored in literature (de Jong, 1984; Marcus & Minc, 1988). Large criteria counts can also cause issues within AHP, effecting both workloads and consistency. AHP includes the calculation of a consistency ratio, quantifying how aligned successive practitioner inputs are; as the number of criteria, and therefore pairwise comparisons increase, it is harder to keep the consistency ratio within the acceptable range (C.R. < 0.1 as prescribed by Saaty, (1987)).
The fastest of the MADM approaches, WSM, takes a highly transparent approach to score calculation; simply summing the product of criteria values and weights for each alternative. However, this simplicity results in several limitations. One of the most impactful is a failure to define interrelations between criteria (Franek & Kashi, 2014); reducing the techniques efficacy in complex decision-making contexts in which the balancing of conflicts is often necessary. Such straightforward aggregation can lead to identical objective vectors for significantly different criteria weightings (Khan & Rehman, 2013), limiting the granularity of possible insights. Additionally, small variations in weightings often cause a change in the recommended alternative, usually requiring a compensatory sensitivity analysis.
VIKOR handles large numbers of alternatives, a desirable quality in many applications. The methodology is conceptually intuitive (Siregar, et al., 2017), however, the procedure often results in erroneous alternative rankings due to flawed calculation methods for the maximum group utility and the minimum individual regret of the opponent (Tzeng & Liu, 2009). Another shortcoming is revealed in criteria weighting determination, requiring hybridisation with other techniques for accurate and stable results (Wibawa, et al., 2019).
PROMETHEE is characterised by the expression of user preference functions, used to generate a partial (PROMETHEE I) or full ranking (PROMETHEE II) of alternatives (Abdullah, et al., 2019). Requiring few user inputs compared to other prominent methodologies (Macharis, et al., 2004), PROMETHEE reduces workloads and potential for bias. There is also no requirement for normalisation of alternatives’ criteria scores (Sabaei, et al., 2015), this step being included within the base methodology. Despite these advantages, the approach often exhibits loss of data or resolution if used to generate complete rankings (Macharis, et al., 2004), reducing the utility of any outputs. PROMETHEE also suffers from rank reversal phenomena (De Keyser & Peeters, 1996), a trait shared with TOPSIS.
Finally, the ELECTRE family comprises of seven sub-methodologies are present (ELECTRE I, ELECTRE Iv, ELECTRE IS, ELECTRE II, ELECTRE III, ELECTRE IV, ELECTRE TRI) (Roy, 1991; Yu, et al., 2018), tailoring the approach to different problem types. ELECTRE I and ELECTRE III are the most applicable around CDU and sustainability assessment, tacking selection and ranking problems respectively. The methods allow for the prescription of ‘veto’ criteria, serving to remove undesirable alternatives (Sahaaya Arul Mary & Suganya, 2016). However, resulting rankings depend on the size of this threshold, for which there exists no ‘correct’ value (Sabaei, et al., 2015). Literature also reports significant workload requirements (Akmaludin, et al., 2020), explaining the limited use (Figure 8).
Key attributes associated with MADM selection have been identified. Table 2 compares the performance of examined methodologies against these attributes. Both references and further methodological advantages and disadvantages can be found in the Supplementary Material. All methodologies are seen to cater to a broad variety of scopes, while remaining applicable to cases containing large numbers of alternatives. Beyond this, the methodologies exhibit often significant divergences. As stated by others, there is no perfect MADM methodology, with selection requires on a case-by-case basis (Guarini, et al., 2018); the approach chosen can influence study outputs an introduce bias. Additionally, data availability influences the selection of methodology as explored by Sabaei, et al. (2015).
Of the methodologies seen frequently in the literature search, only AHP includes a robust approach to weighting derivation. This represents a significant flaw in other methods as human perception and logic of prioritisation is prone to errors when more than four criteria are present (Saaty, 1994). Consequently, additional work is required to determine meaningful weightings, often involving hybridisation. Through this integrated weighting procedure, AHP is the only method to track the consistency of user value-choices, safeguarding against conflicting inputs.
While AHP mathematically determines weightings, it is not suited to problems with large numbers of criteria; a consequence of its pair-wise comparison approach. In a comparison matrix with n criteria present,
Implementation within CDU problems
Each MADM approach has specific advantages and disadvantages. When seeking the best method for a given field or problem type, these must be considered both in isolation and in hybridised forms. Hybridisation likely offers the best approach, retaining strengths while resolving weaknesses. Such approaches can be seen throughout literature with many different technique combinations (Zavadskas, et al., 2016). The first appeared in 1999 (Mesghouni, et al., 1999), with the term Hybrid Multi Criteria Decision Making being coined by Shyur & Shih, (2006).
To identify suitable methodologies, the requirements of the application must be defined. For use in CDU oriented sustainability assessment, the following factors are determined as important:
• Systematic and repeatable criteria weighting procedure
• User value choice consistency checking to prevent conflicting preference prescription
• Transparency of calculations
• Applicability to problems with many criteria
• Satisfactory computational and workload efficiency
After identifying these requirements, the assessed methods can be examined for suitability. AHP and TOPSIS appear ideal for hybrid application; perhaps unsurprisingly given their combined 55.8% prevalence in the earlier systematic literature search. Arslan, et al. previously reviewed the hybridization of these two methodologies, determining that their integration produces a more powerful and effective decision-making tool (Arslan, et al., 2021). This approach will now be examined further in the context of CDU applications.
As noted by Chauvy, et al. (2020) AHP, developed by Saaty, (1980), already appears within environmental decision making, consistently being noted as a common technique in the area (Baumann, et al., 2019; Martins, et al., 2020). Additionally, it is the only methodology that can fulfil the first two requirements laid out for this application: systematic and repeatable criteria weighting, and user value choice consistency checking. Furthermore, the approach’s tiered hierarchical structure aligns well with the needs of McCord, et al.‘s Triple helix framework (McCord, et al., 2021); alleviating the pairwise comparison workloads associated with high criteria counts. In addition, this approach would allow for application of a zero weighting to one or two entire strands; instead delivering a double or single stranded assessment. This is represented in a three-tier configuration by Figure 10 (analogous to the approach taken by Chauvy, et al. (2020).
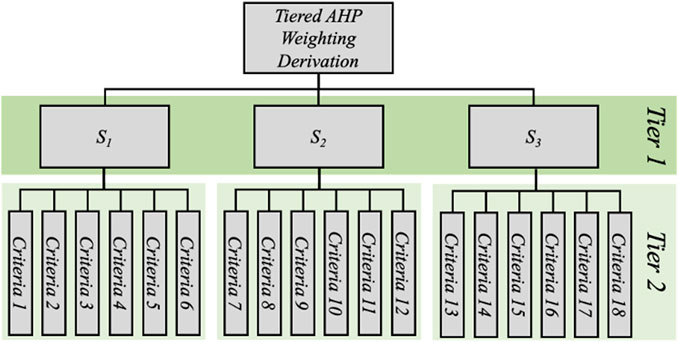
FIGURE 10. Tiered AHP structure (based on that described by Chauvy, et al. (2020)). Si represent assessment strands.
The number of pairwise comparisons required for both global and tiered AHP approaches can be derived Equations (1) and (2) respectively.
Eq. 1 - Calculation for the number of pairwise comparisons required for a global AHP approach. Where C is the number of criteria assessed.
Eq. 2 - Calculation for the number of pairwise comparisons required for a tiered AHP approach. Where S is the number of strands employed, and C is the number of criteria assessed. (Assuming criteria are distributed evenly between strands).
If calculated across varying total criteria counts, the difference in pairwise comparisons and workload can be seen visually (Figure 11). As the number of criteria assessed increases, the required pairwise comparisons diverge; consequently, efficiency increases with assessment granularity. For example, studies evaluating 18 criteria experience a 68.63% reduction in practitioner workload when using a tiered approach. With many assessments needed to evaluate growing numbers of emerging CDU technologies, an efficient methodology will prove invaluable.
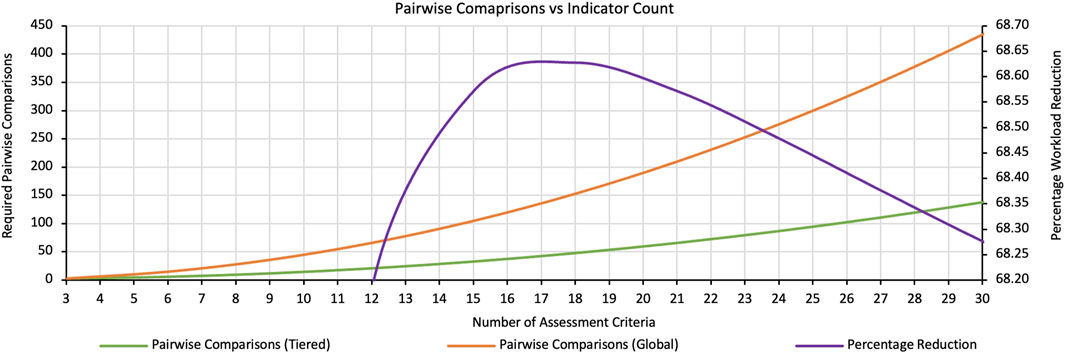
FIGURE 11. Evaluation of pairwise comparisons required for the implementation of AHP in tiered and global structures and criteria count increases (based on application to the triple helix framework (McCord, et al., 2021) with three AHP sub-tiers and an even indicator distribution). Percentage workload is overlaid using a secondary axis.
Once criteria weights have been determined using AHP, the outputs can be fed directly into TOPSIS in order to generate a ranking order of the available alternatives. This hybridisation strategy eliminates the weaknesses of both methodologies; the aggregation, scoring scale directionality, and ranking within AHP, and the weighting derivation within TOPSIS. Furthermore, TOPSIS is capable of handling very large numbers of alternatives with high computational efficiency while possessing a clear and intuitive logic (Roszkowska, 2011), facilitating more detailed assessments. This delivers a strong foundation for applications such as technology or supply chain selection, in which many structural permutations are present. However, the issue of rank reversal persists (Çelikbilek and Tüysüz, 2020). To combat this, sensitivity analysis is recommended to verify the stability of generated results.
When qualitative data is presented, such as that seen in most social impact assessments, a five or nine-point scale should be used for the assignment of quantitative values within the TOPSIS decision matrix. This is prescribed to achieve better differentiation between alternatives, as suggested by McCord et al. (2021) within the triple helix framework. Once the decision matrix is created, vector normalisation is carried out by criterion using Eq. (3).
Eq. 3 - Vector normalisation procedure for alternative criteria scores. Where n is the number of alternatives examined.
The standard TOPSIS methodology can then be applied as developed by Hwang & Yoon, (1981). For each criterion the best and worst scores are identified, Vj+ and Vj− respectively. Using these the Euclidian distance from best (Si+) and worst (Si−) performance values can be calculated for each alternative.
Eq. 4 - Calculation of the Euclidean distance from the ideal best performance
Eq. 5 - Calculation of the Euclidean distance from the ideal worst performance
The performance score for each alternative, accounting for all criteria, can then be evaluated. This provides the basis on which the alternatives are ranked. Opricovic (developer of VIKOR) and Teng suggest that the efficacy of TOPSIS may be improved by allowing the user to weight the importance of the Euclidean distance from the deal best and worst scenarios (Opricovic & Tzeng, 2004). This would allow for more problem specific application of both independent and hybridised TOPSIS. However, for a majority of problems and observed uses, including those with CDU focusses, the standard equal weighting approach is deemed satisfactory.
Eq. 6 - Calculation of the alternatives’ performance score
Fiduciary duty in the carbon economy
The transition from a linear to circular economy will not be without problems. The need to defossilise power and industry has been addressed in different policies over a number of decades. Carbon capture and storage, or in reality landfill, has been considered to be the best way to mitigate against CO2 emissions, however it has made little progress. Initial development will need incentivisation or subsidies, most likely from governments, so due diligence must be exercised to ensure correct decisions are being made. There is a fiduciary duty that is incumbent on any individual or organisation to ensure investments are used wisely. Fiduciary duties are owed when someone “has undertaken to act for or on behalf of another in a particular matter in circumstances which give rise to a relationship of trust and confidence”. This highlights an interesting feature of due diligence in respect of government funding to environmental projects. A government acts on behalf of the people, the electorate, to ensure public funds are used wisely and that they are trusted to do so in a financially, and especially fiscally sound manner.
As an example the case for carbon capture and storage in the UK is considered. In recent years there have been a number of competitions where projects competed for money to finance CCS projects. This was originally mooted by the Labour Government headed by Prime Minister Blair. However, despite the first project in Peterhead, Scotland being announced in 2007 it was subsequently cancelled in 2011. A second project was announced in 2012 and then cancelled in 2016 (BEIS, 2017) after a statement to the London Stock Exchange by Chancellor Osborn in 2015. The fiduciary duty of the government was then declared by Prime Minister Cameron in the House of Commons in a reply to a Parliamentary question asking why the latter project had been cancelled. Cameron (2016) said:
“You have to make decisions about technology that works and technology that is not working. We are spending the money on innovation, on energy storage, on small nuclear reactors, and on other things such as energy heat systems for local communities that will make a difference. To govern is to choose, and we made the right choice”.
This clearly articulates that the government did not have confidence in the technology to be used and that in light of this it would not be responsible to continue to fund the project from the public purse. The government was clearly exercising its fiduciary duty. However, in 2021 the government of then Prime Minister Johnson announced a new competition to do CCUS in 20 projects at existing clusters. These were typically using existing amine technology. So, what had changed in the intervening period? Alarmingly very little. The projects are still reliant on amine capture and storage via pipeline to the North Sea. Despite the call looking at CCUS (Mission Innovation, 2018), the U or utilisation part is largely absent.
Behavioural change as a driver towards a circular economy
Similarities between resource security, energy security and food security demonstrate the need for a whole systems approach as there are considerable inter-dependencies. Typically, government industrial resource strategies across the world look at cost and GHG mitigation as primary drivers to enable supply chain stability and maintain an acceptable standard of living. However, in the case of the United Kingdom, the BEIS Areas of Research Interest do not identify the chemicals industries as a priority (BEIS, 2020). These concentrate on the economy, climate change and decarbonisation of energy systems particularly but fail to tackle the ever-increasing demand on materials. The result is that a linear supply chain and economy are perpetuated, meaning more fossil carbon is needed to supply demand. This is driven by growing consumerism and a dependency on carbon in a disposable asset society. To reduce fossil dependence, several approaches to this problem should be addressed. Switching to renewable carbon assets is a primary driver but behavioural change should be developed and embedded in society. Energy is the crux of the problem: as well as heat and power being energy, so are materials and chemicals, and so is food.
As way of an analogy, during World War Two, allotments were of upmost importance during a period of rationing which was in place for over a decade. “Dig for victory” propaganda is strikingly appropriate today, for tackling carbon security. The strategy encouraged citizens to “dig in”, to use resources sparingly and where possible create their own. In the case of current energy issues, UK government broadcasts encouraged people to reduce the thermostat temperature, to use heating sparingly, and while not necessarily producing their own energy, encouraging them to insulate their homes to preserve energy. However, there has been little effort to promote materials security as these concepts are largely decoupled from energy considerations. Populations of many countries have become increasingly aware of the challenges of energy at affordable costs and recent global events have added to this, leading to the fear of blackout and food shortages in the United Kingdom. This can be attributed to multiple variables, including the need to limit global temperatures to 1.5°C, increasing industrial action, growing civil unrest, and the war in Ukraine. Whilst not in a World War, it could be said that society is at war with the world and that a multi-levelled, multi-disciplined approach to creating a sustainable energy is required, and consequently sustainable chemicals, that do not negatively impact those already suffering from energy insecurity. The idea of energy rationing as a driver for a more sustainable society has been proposed. While the French government is reluctant to commit to energy rationing in the near future, Germany has suggested that energy rationing may be needed over the coming winters. Households and other protected communities (care and health) would be exempted under German law but there would be serious implications for industry and in particular the chemicals supply chain (Guardian, 2022). The German energy regulator (BNA) have suggested that natural gas use may need to be reduced by 20% in 2022 to avoid rationing over the winter of 2022–3 (Financial Times, 2022) and measures are being put in place to prepare for emergency gas rationing (Bloomberg, 2022). In the UK, Climate Minister Graham Start indicated that blackouts could be possible over winter over peak periods which will affect industrial and domestic users (Sky News, 2022). Such measures may encourage a better long-term use of energy across the chemicals sector, by improving process design and intensification. However, care must be taken to ensure that a drift back to less sustainable operation does not occur.
Consumers need to be redefined as Custodians to achieve carbon circularity. As such they would then hold more power, and if they choose, could change how they approach energy and chemicals supply systems. Social behavioural change is difficult to enact as individual actors in civil society have their own autonomy and priorities, so governance is needed that is effective, efficient, and equitable. Current policies are not. Most energy policies still promote a linear approach to energy and therefore materials use. Policies need to be developed and enacted that promote the reuse of carbon in sustainable systems. These ambitions cannot be achieved without considering external factors that can impact specific areas. Chemicals production policy has been idealised and politicised to the extent it is difficult to reach the standards needed for a sustainable system, such as those defined within the Science Based Targets Initiative. An energy-rationed economy would lead subsequently to a materials-rationed economy. This may be seen by many as a retrograde step but may equally be perceived as a behavioural re-set. When rationing is effective, essentials are not forsaken, rather consumerism is scaled back. As such our expectations will become reduced society will adapt to a moderated and more circular approach to life. After the Second World War there was an attitude of ‘mend and make do’. This is one of the tenets of a circular economy as shown in Figure 12 which gives a proposed hierarchal approach to chemical circularity from a supply chain perspective. Dig for Victory could equally be described as ‘recycle for victory’.
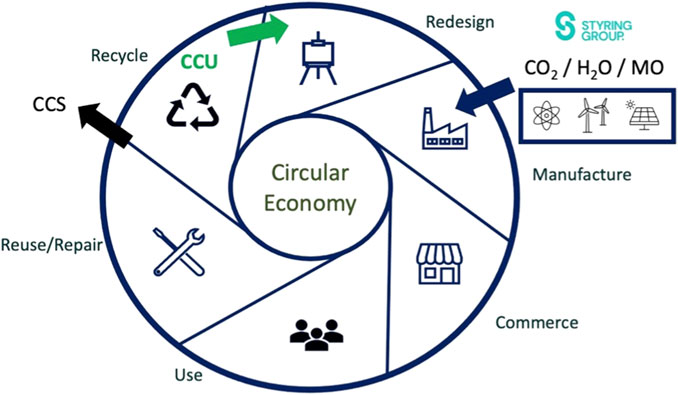
FIGURE 12. A circular approach to chemicals manufacture using CCU as a carbon vector. In this representation fossil carbon has been avoided and is so eliminated.
In Figure 12, the thick black arrows represent a linear economic approach where carbon enters the supply chain, is consumed and is then either emitted or sent for storage (CCS) where this is available. To embrace a circular approach that carbon must stay within the supply chain so that no new fossil carbon enters in an ideal situation, using CCU (thick green arrow). It may be that using the traditional linear process chemistry approaches is not possible in a circular economy. Therefore, new chemical transformations and processes may be needed through Re-design to achieve the objectives.
While rationing may give a short-term mitigation potential in the climate change emergency it is always possible that once the energy crisis is resolved there will be a bounce back once there is respite. A similar response in GHG emissions was observed post-covid lockdowns when emissions reductions observed during the pandemic quickly reversed once ‘normality’ was restored. The difference with a transition to a circular chemical economy needs to be considered in the short-medium and long-term. Benefits of a circular economy using CO2 need to be evidenced using a multi-faceted approach, especially if industry is to invest in the capital infrastructure to facilitate it. When looking at the problem from a triple helix perspective (McCord et al., 2021), a methodology needs to be embedded in product development in which data are collected at each stage of the decision-making process and that there is iterative feedback to ensure the best outcome scenarios.
The United Nations (2019), cited in Dobson et al. (2020) has predicted that, by 2050, 90% of the UK population will live in urban areas, so it is important to consider the supply chain implications when manufacturing is de-urbanised, but the workforce is not. Serious consideration is therefore needed in designing systems within potential national chemicals production policies. Distributed energy and chemicals production specifically around urban populated areas, would be beneficial for, not only the environment but also for greater commodities provisions.
Conclusion
This Perspectives Paper discusses areas of carbon dioxide utilisation that are often considered in isolation rather than within a whole system. While TEA is often reoorted in terms of the CCU process it is rarely considered over the whole system and concentrates primarily on financial rather than fiscal aspects and does not consider the role of fiduciary duty within a policy framework. Likewise, social impact is often an afterthought, whereas here it is considered within an iterative decision-making process. The aim of this article is to encourage practitioners of CCU to think of all consequences during the analysis of CCU Technology development. There is an urgency to address the climate emergency in terms of not only energy but also materials and chemicals production and use. There is a need to move from a linear to a circular carbon economy, using nth-generation recycled carbon in the production of value-added materials. This needs to be achieved within the acceptable bounds of social, environmental and economic responsibility that does not create social underclasses (Styring et al., 2021) or energy and material poverty. Governments need to apply the principles of fiduciary duty to ensure that public money is invested wisely in technology development and implementation. In all aspects of future living, a new sense of responsibility of individuals and groups is required to ensure equity in society. We need to move away from considering ourselves as consumers of carbon (as well as other chemicals) to becoming custodians of carbon: protecting it as a resource for future generations as well as also protecting the environment and quality of life.
Data availability statement
The original contributions presented in the study are included in the article/Supplementary Material, further inquiries can be directed to the corresponding author.
Author contributions
Each author contributed original content to the paper, including original ideas and manuscript preparation. Each was involved in the review of the final document. PS was additionally research supervisor and Principal Investigator on the research projects.
Funding
We are grateful the UKRI Engineering and Physical Sciences Research Council (EPSRC) for funding to PS under the UKRI Interdisciplinary Centre for Circular Chemical Economy award (EP/V011863/1) and to PS and GD under the SUSTAIN Manufacturing Hub award (EP/S018107/1). AN and EP are funded under PhD Scholarships from a Strategic Partnership with Unilever. HH-S was an undergraduate student at the University of Sheffield and was unfunded.
Conflict of interest
The authors declare that the research was conducted in the absence of any commercial or financial relationships that could be construed as a potential conflict of interest.
Publisher’s note
All claims expressed in this article are solely those of the authors and do not necessarily represent those of their affiliated organizations, or those of the publisher, the editors and the reviewers. Any product that may be evaluated in this article, or claim that may be made by its manufacturer, is not guaranteed or endorsed by the publisher.
Supplementary material
The Supplementary Material for this article can be found online at: https://www.frontiersin.org/articles/10.3389/fenrg.2023.1124072/full#supplementary-material
References
Abdullah, L., Chan, W., and Afshari, A. (2019). Application of PROMETHEE method for green supplier selection: A comparative result based on preference functions. J. Industrial Eng. Int. 15, 271–285. doi:10.1007/s40092-018-0289-z
Akao, Y. (2004). Quality function deployment (QFD): Integrating customer requirements into product design. 1st ed. New York, NY: Productivity Press.
Akmaludin, A., Hartati, T., Purwanto, H., Sukendar, T., Latifah, F., and Septiana, L. (2020). The best selection of programmers in generation 4.0 using AHP and ELECTRE elimination methods. J. Phys. Conf. Ser. 1477, 032001. doi:10.1088/1742-6596/1477/3/032001
Aresta, M., Dibenedetto, A., and Angelini, A. (2013). The changing paradigm in CO2 utilization. J. CO2Util. 3, 65–73. doi:10.1016/j.jcou.2013.08.001
Arslan, A. E., Arslan, O., and Kandemir, S. Y. (2021). AHP–TOPSIS hybrid decision-making analysis: Simav integrated system case study. J. Therm. Analysis Calorim. 145, 1191–1202. doi:10.1007/s10973-020-10270-4
Baker, D., Bridges, D., Hunter, R., Johnson, G., Krupa, J., Murphy, J., et al. (2001). Guidebook to decision-making methods. Washington, D.C: U.S. Department of Energy.
Barrow, M., Buckley, B., Caldicott, T., Cumberlege, T., Hsu, J., Kaufmann, S., et al. (2013). Technical Guidance for scope 3 emissions (version 1.0) greenhouse gas protocol. Washington D.C., USA: World Resources Institute.
Baumann, M., Weil, M., Peters, J. F., Chibeles-Martins, N., and Moniz, A. B. (2019). A review of multi-criteria decision-making approaches for evaluating energy storage systems for grid applications. Renew. Sustain. Energy Rev. 107, 516–534. doi:10.1016/j.rser.2019.02.016
BEIS (2020). BEIS areas of research interest: Interim update 2020. Available at: https://assets.publishing.service.gov.uk/government/uploads/system/uploads/attachment_data/file/862777/beis-areas-research-interest-interim-update-2020.pdf (Accessed December 07, 2022).
BEIS (2017). Carbon capture usage and storage: Third time lucky? Available at: https://publications.parliament.uk/pa/cm201719/cmselect/cmbeis/1094/1094.pdf.
Bloomberg (2022). Bloomberg. Available at: www.bloomberg.com/news/articles/2022-09-23/germany-steps-up-preparations-for-emergency-gas-rationing?leadSource=uverify%20wall (Accessed December 07, 2022).
Brans, J.-P., and Mareschal, B. (2005). “Promethee methods,” in Multiple criteria decision analysis: State of the art surveys. Editors J. R. Figueira, S. Greco, and M. Ehrogott (New York, NY: Springer), 163–168.
Cameron, D. (2016). Hansard UK parliament, transcript on the debate surrounding the Paris climate conference, volume 604. Debated on Thursday 7 January 2016. Available at: https://hansard.parliament.uk/Commons/2016-01-07/debates/16010722000015/ParisClimateConference?highlight=carbon%20capture%20storage#contribution- 16010722000056 (Accessed November 22, 2022).
Cegan, J. C., Filion, A. M., Keisler, J. M., and Linkov, I. (2017). Trends and applications of multi-criteria decision analysis in environmental sciences: Literature review. Environ. Syst. Decis. 37, 123–133. doi:10.1007/s10669-017-9642-9
Çelikbilek, Y., and Tüysüz, F. (2020). An in-depth review of theory of the TOPSIS method: An experimental analysis. J. Manag. Anal. 7 (2), 281–300. doi:10.1080/23270012.2020.1748528
Charnes, A., Cooper, W. W., and Rhodes, E. (1978). Measuring the efficiency of decision making units. Eur. J. Operational Res. 2 (16), 429–444. doi:10.1016/0377-2217(78)90138-8
Chauvy, R., Lepore, R., Fortemps, P., and De Weireld, G. (2020). Comparison of multi-criteria decision-analysis methods for selecting carbon dioxide utilization products. Sustain. Prod. Consum. 24, 194–210. doi:10.1016/j.spc.2020.07.002
Chen, S.-J., and Hwang, C.-L. (1992). Fuzzy multiple attribute decision making. Heidelberg: Springer.
Choptiany, J. M. H., Pelot, R., and Sherren, K. (2014). An interdisciplinary perspective on carbon capture and storage assessment methods. J. Industrial Ecol. 18 (3), 445–458. doi:10.1111/jiec.12121
Cinelli, M., Kadziński, M., Gonzales, M., and Słowiński, R. (2020). How to support the application of multiple criteria decision analysis? Let us start with a comprehensive taxonomy. Omega 96, 102261. doi:10.1016/j.omega.2020.102261
Circular Computing (2021). What is the carbon footprint of a laptop? Available at: https://circularcomputing.com/news/carbon-footprint-laptop/(Accessed 11 24, 2022).
Cox, S., Gagnon, P., Stout, S., Zinaman, O., Watson, A., and Hotchkiss, E. (2016). Distributed Generation to support development-focused climate action. Golden, United States: National Renewable Energy Lab. (NREL/TP-6A20-66597): 30.
de Jong, P. (1984). A statistical approach to Saaty’s scaling method for priorities. J. Math. Psychol. 28 (4), 467–478. doi:10.1016/0022-2496(84)90013-0
De Keyser, W., and Peeters, P. (1996). A note on the use of PROMETHEE multicriteria methods. Eur. J. Operational Res. 89 (3), 457–461. doi:10.1016/0377-2217(94)00307-6
Dobson, M. C., Edmondson, J. L., and Warren, P. H. (2020). Urban food cultivation in the United Kingdom: Quantifying loss of allotment land and identifying potential for restoration. Landsc. Urban Plan. 199, 103803. doi:10.1016/j.landurbplan.2020.103803
Dowson, G. R. M., Reed, D. G., Bellas, J.-M., Charalambous, C., and Styring, P. (2016). Fast and selective separation of carbon dioxide from dilute streams by pressure swing adsorption using solid ionic liquids. Faraday Discuss. 192, 511–527. doi:10.1039/C6FD00035E
Dowson, G. R. M., and Strying, P. (2017). Demonstration of CO2 conversion to synthetic transport fuel at flue gas concentrations. Front. Energy Res. 5, 26. doi:10.3389/fenrg.2017.00026
European Commission Joint Research Centre Institute for Environment and Sustainability (2010). International reference life cycle data system (ILCD) handbook - general guide for life cycle assessment - detailed guidance. Luxembourg: Publications Office of the European Union.
European Environment Agency (2008). Electric vehicles from life cycle and circular economy perspectives TERM 2018: Transport and Environment Reporting Mechanism (TERM) report. Luxembourg: Publications Office of the European Union.
Feliciano-Bruzual, C. (2014). Charcoal injection in blast furnaces (Bio-PCI): CO2 reduction potential and economic prospects. J. Mat. Res. Technol. 3, 233–243. doi:10.1016/j.jmrt.2014.06.001
Financial Times (2022). Financial times. Available at: www.ft.com/content/ca215782-a3ba-4a85-997d-197769275a50 (Accessed December 07, 2022).
Fishburn, P. C., and Lavalle, I. H. (1999). Mcda: Theory, practice and the future. J. Multi-Criteria Decis. Anal. 8, 199901. doi:10.1002/(SICI)1099-1360(199901)8:1<1:AID-MCDA205>3.0.CO;2-E
Fontela, E., and Gabus, A. (1972). World problems an invitation to further thought within the framework of DEMATEL. Geneva: Battelle Geneva Research Centre.
Franek, J., and Kashi, K. (2014). A review and critique of MADM methods and applications in business and management. Int. J. Anal. Hierarchy Process 6. doi:10.13033/ijahp.v6i2.254
Gallego-Schmid, A., Chen, H. M., Sharmina, M., and Mendoza, J. M. F. (2020). Links between circular economy and climate change mitigation in the built environment. J. Clean. Prod. 260, 121115. doi:10.1016/j.jclepro.2020.121115
Ghorabaee, M. K., Zavadskas, E. K., Olfat, L., and Turskis, Z. (2016). Multi-criteria inventory classification using a new method of evaluation based on distance from average solution (EDAS). Informatica 26 (3), 435–451.
Gibari, S. E., Gómez, T., and Ruiz, F. (2018). Building composite indicators using multicriteria methods: A review. J. Bus. Econ. 89, 1–24. doi:10.1007/s11573-018-0902-z
Greco, S., Ishizaka, A., Tasiou, M., and Torrisi, G. (2019). On the methodological framework of composite indices: A review of the issues of weighting, aggregation, and robustness. Soc. Indic. Res. 141, 61–94. doi:10.1007/s11205-017-1832-9
Guardian (2022). Guardian. Available at: www.theguardian.com/business/2022/sep/15/gas-rationing-germany-basf-plant-europe-crisis (Accessed December 07, 2022).
Guarini, M. R., Battisti, F., and Chiovitti, A. (2018). Public initiatives of settlement transformation: A theoretical-methodological approach to selecting tools of multi-criteria decision analysis. Buildings 8 (1), 1. doi:10.3390/buildings8010001)
Guinée, J. B. (2012). Lifecycle assessment: Past, present and future. Nantes, France: International Symposium on Lifecycle Assessment and Construction.
Han, X., Yang, Z., Wang, M., Tjong, J., and Zheng, M. (2017). Clean combustion of n-butanol as a next generation biofuel for diesel engines. Appl. Energy 198, 347–359. doi:10.1016/j.apenergy.2016.12.059
Huarachi, D. A. R., Piekarski, C. M., Puglieri, F. M., and de Francisco, A. C. (2020). Past and future of social life cycle assessment: Historical evolution and research trends. J. Clean. Prod. 264, 1–12. doi:10.1016/j.jclepro.2020.121506
Hwang, C. L., and Yoon, K. (1981). Multiple attribute decision making: Methods and applications. New York, NY: Springer-Verlag.
IPCC (2021). Climate change 2021: The physical science basis. Contribution of working group I to the sixth assessment report of the intergovernmental panel on climate change. Cambridge, United Kingdom and New York, NY, USA: Cambridge University Press. doi:10.1017/9781009157896
ISO Technical Committee 207 (2006). ISO14040:2006 Environmental management - lifecycle assessment - principles and framework. Geneva, Switzerland: International Standards Organisation.
Jørgensen, A., Le Bocq, A., Nazarkina, L., and Hauschild, M. Z. (2007). Methodologies for social life cycle assessment. Int. J. Life Cycle Assess. 13 (2), 96–103. doi:10.1065/lca2007.11.367
Katsikopoulos, K. V., Durbach, I. N., and Stewart, J. T. (2018). When should we use simple decision models? A synthesis of various research strands. Omega 81, 17–25. doi:10.1016/j.omega.2017.09.005
Keršuliene, V., Zavadskas, E. K., and Turskis, Z. (2010). Selection of rational dispute resolution method by applying new step-wise weight assessment ratio analysis (Swara). J. Bus. Econ. Manag. 11, 243–258. doi:10.3846/jbem.2010.12
Khan, S. A., and Rehman, S. (2013). Iterative non-deterministic algorithms in on-shore wind farm design: A brief survey. Renew. Sustain. Energy Rev. 19, 370–384. doi:10.1016/j.rser.2012.11.040
Kloepffer, W. (2008). Life cycle sustainability assessment of products. Int. J. Life Cycle Ass. 13 (2), 89–95. doi:10.1065/lca2008.02.376
Klöpffer, W. (2003). Life-Cycle based methods for sustainable product development. Int. J. Life Cycle Assess. 8, 157–159. doi:10.1007/BF02978462
Köksalan, M., Wallenius, J., and Zionts, S. (2013). An early history of multiple criteria decision making. J. Multi Criteria Decis. Analysis 20, 87–94. doi:10.1002/mcda.1481
Koohi-Fayegh, S., and Rosen, M. A. (2020). A review of energy storage types, applications and recent developments. J. Energy Storage 27, 101047. doi:10.1016/j.est.2019.101047
Kuo, T. (2017). A modified TOPSIS with a different ranking index. Eur. J. Operational Res. 260, 152–160. doi:10.1016/j.ejor.2016.11.052
Lackner, K. S. (2013). The thermodynamics of direct air capture of carbon dioxide. Energy 50, 38–46. doi:10.1016/j.energy.2012.09.012
Lansink, A. (2018). Challenging changes–connecting waste hierarchy and circular economy. Waste Manag. Res, J. a Sustain. Circular Econ. 36, 872. doi:10.1177/0734242x18795600
Lee, H., Tzeng, G., Yeih, W., Wang, Y., and Yang, S. (2013). Revised DEMATEL: Resolving the infeasibility of DEMATEL. Appl. Math. Model. 37 (10), 6746–6757. doi:10.1016/j.apm.2013.01.016
Macharis, C., Springael, J., De Brucker, K., and Verbeke, A. (2004). PROMETHEE and AHP: The design of operational synergies in multicriteria analysis. Eur. J. Operational Res. 153, 307–317. doi:10.1016/s0377-2217(03)00153-x
Martins, I. D., Moreas, F. F., Távora, G., Soares, H. L. F., Infante, C. E., Arruda, E. F., et al. (2020). A review of the multicriteria decision analysis applied to oil and gas decommissioning problems. Ocean. Coast. Manag. 184, 1–20. doi:10.1016/j.ocecoaman.2019.105000
McCord, S., Armstrong, K., and Styring, P. (2021). Developing a triple helix approach for CO2 utilisation assessment. Faraday Discuss. 230, 247–270. doi:10.1039/D1FD00002K
McCord, S., Zaragoza, A. V., Langhorst, T., Strunge, T., Cremonese, L., Schomäcker, R., et al. (2018). “Part E: Integrated TEA & LCA guidelines,” in Techno-economic assessment & life cycle assessment guidelines for CO2 utilization (version 2) (Ann Arbor: Global
McQueen, N., Gomes, K. V., McCormick, C., Blumanthal, K., Pisciotta, M., and Wilcox, J. (2021). A review of direct air capture (DAC): Scaling up commercial technologies and innovating for the future. Prog. Energy 3, 032001. doi:10.1088/2516-1083/abf1ce
Meinrenken, C. J., Chen, D., Esparza, R. A., Lyer, V., Paridis, S. P., Prasad, A., et al. (2020). Carbon emissions embodied in product value chains and the role of Life Cycle Assessment in curbing them. Nature 10, 6184. doi:10.1038/s41598-020-62030-x
Meng, H., Mi, J., Guo, M., Wu, H., Lv, L., Yun, J., et al. (2021). A highly efficient and stable composite of polyacrylate and metal organic framework prepared by interface engineering for direct air capture. ACS Appl. Mat. Inter. 13, 21775–21785. doi:10.1021/acsami.1c03661
Mesghouni, K., Pesin, P., Trentesaux, D., Hammadi, S., Tahon, C., and Borne, P. (1999). Hybrid approach to decision-making for job-shop scheduling. Prod. Plan. Control 10, 690–706. doi:10.1080/095372899232768
Mission Innovation (2018). Accelerating breakthrough innovation in carbon capture, utilization, and storage. US Department of energy, Office of Fossil Energy and Carbon Management, Available at: www.energy.gov/fecm/downloads/accelerating-breakthrough-innovation-carbon-capture-utilization-and-storage.
Mu, E. (2006). A unified framework for site selection and business forecasting using ANP. J. Syst. Sci. Syst. Eng. 15, 178–188. doi:10.1007/s11518-006-5006-6
Müller, L., Langhorst, T., Kätelhön, A., Bachmann, M., Sternberg, A., and Bardow, A. (2022). Part C: LCA guidelines, techno-economic assessment & life cycle assessment Guidelines for CO2utilization (version 2). Ann Arbor, Michigan: CO2Chem Media and Publishing Ltd, 133–188.
Nagasawa, H., Yamasaki, A., Iizuka, A., Kumagai, K., and Yanagisawa, Y. (2009). A new recovery process of carbon dioxide from alkaline carbonate solution via electrodialysis. AIChE J. 55, 3286–3293. doi:10.1002/aic.11907
Nakagaki, T. (2021). Fundamentals of thermal and nuclear power generation, 5- issues in power generation and future prospects. Tokyo, Japan: JSME, 275–288.
Newell, P., and Mulvaney, D. (2013). The political economy of the ‘just transition. Geogr. J. 179 (2), 132–140. doi:10.1111/geoj.12008
Newman, A. J. K., and Styring, P. (2022). The pursuit of methodological harmonization within the holistic sustainability assessment of CCU projects: A history and critical review. Front. Sustain. 3. doi:10.3389/frsus.2022.1057476
NOAA Global Monitoring Laboratory (2021). Trends in atmospheric carbon dioxide. Available at: https://gml.noaa.gov/ccgg/trends/global.html (Accessed November 18, 2022).
NREL (2021). Life cycle greenhouse gas emissions from electricity generation. Colorado, USA: Update¸ US Department of Energy.
O'Brien, M., Doig, A., and Clift, R. (1996). Social and environmental life cycle assessment (SELCA). Int. J. Life Cycle Assess. 1, 231–237. doi:10.1007/BF02978703
Opricovic, S., and Tzeng, G.-H. (2004). Compromise solution by MCDM methods: A comparative analysis of VIKOR and TOPSIS. Eur. J. Operational Res. 156, 445–455. doi:10.1016/s0377-2217(03)00020-1
Platt, E. G., and Styring, P. (2022). New olefin production routes — a review of defossilised supply chain technologies with regards to surfactant production. Front. Sustain. 3, 1057491. doi:10.3389/frsus.2022.1057491
Pohekar, S. D., and Ramachandran, M. (2004). Application of multi-criteria decision making to sustainable energy planning - a review. Renew. Sustain. Energy Rev. 8 (4), 365–381. doi:10.1016/j.rser.2003.12.007
Pollok, L., Spierling, S., Endres, H-.J., and Grote, U. (2021). Social life cycle assessments: A review on past development, advances and methodological challenges. Sustainability 13 (18), 10286–10329. doi:10.3390/su131810286
Putrasari, Y., and Lim, O. (2022). Dimethyl ether as the next generation fuel to control nitrogen oxides and particulate matter emissions from internal combustion engines: A review. ACS Omega 7 (1), 32–37. doi:10.1021/acsomega.1c03885
Ramey, S., and Ramey, D. (2005). Butanol biofuel 100% coast to coast 2005. Available at: https://www.butanol.com/(Accessed 11 24, 2022).
Remmen, A., Jensen, A. A., and Frydendal, J. (2007). Life cycle management A business guide to sustainability. New York, New York: United Nations Environment Programme.
Ribeiro, R. A. (1996). Fuzzy multiple attribute decision making: A review and new preference elicitation techniques. Fuzzy Sets Syst. 78 (2), 155–181. doi:10.1016/0165-0114(95)00166-2
Ricardo Inc (2022). Life cycle analysis comparison: Electric and internal combustion engine vehicles. Alexandria, VA, USA: The Fuels Initiative.
Rissman, J., Bataille, C., Masanet, E., Aden, N., Morrow, W. R., Zhou, N., et al. (2020). Technologies and policies to decarbonize global industry: Review and assessment of mitigation drivers through 2070. Appl. Energy 266, 114848. doi:10.1016/j.apenergy.2020.114848
Roh, K., Lee, J. H., and Gani, R. (2016). A methodological framework for the development of feasible CO2 conversion processes. Int. J. Greenh. Gas. Con. 47, 250–265. doi:10.1016/j.ijggc.2016.01.028
Romeo, L. M., Minguell, D., Shirmohammadi, R., and Andres, J. M. (2020). Comparative analysis of the efficiency penalty in power plants of different amine-based solvents for CO2 capture. Ind. Eng. Chem. Res. 59, 10082–10092. doi:10.1021/acs.iecr.0c01483
Roszkowska, E. (2011). “Multi-criteria decision making models by applying the TOPSIS method to crisp interval data,” in Multiple criteria decision making vol. 6. Editor T. Trzaskalik (Katowice: Publishing House of the University of Economics in Katowice), 200–230.
Roy, B. (1968). Classement et choix en présence de points de vue multiples. Revue française d'automatique, d’informatique de recherche opérationnelle 8, 57–75. doi:10.1051/ro/196802v100571
Roy, B. (1991). The outranking approach and the founda-tions of ELECTRE methods. Theory Decis. 31, 49–73. doi:10.1007/bf00134132
Saaty, R. W. (1987). The analytic hierarchy process—What it is and how it is used. Math. Model. 9, 161–176. doi:10.1016/0270-0255(87)90473-8
Saaty, T. L. (1977). A scaling method for priorities in hierarchical structures. J. Math. Psychol. 15, 234–281. doi:10.1016/0022-2496(77)90033-5
Saaty, T. L. (1986). Axiomatic foundation of the analytic hierarchy process. Manag. Sci. 32, 841–855. doi:10.1287/mnsc.32.7.841
Saaty, T. L. (1994). Highlights and critical points in the theory and application of the Analytic Hierarchy Process. European Journal of Operational Research 74, 426–447.
Saaty, T. L., and Vargas, L. G. (2013). Decision making with the analytic network process. New York, NY: Springer.
Sabaei, D., Erkoyuncu, J., and Roy, R. (2015). A review of multi-criteria decision making methods for enhanced maintenance delivery. Procedia CIRP 37, 30–35. doi:10.1016/j.procir.2015.08.086
Sadiq, M. M., Konstas, K., Falcaro, P., Hill, A. J., Suzuki, K., and Hill, M. R. (2020). Engineered Porous nanocomposites that deliver remarkably low carbon capture energy costs. Cell Rep. Phys. Sci. 1, 100070. doi:10.1016/j.xcrp.2020.100070
Sahaaya Arul Mary, S. A., and Suganya, G. (2016). Multi-criteria decision making using ELECTRE. Circuits Syst. 7, 1008–1020. doi:10.4236/cs.2016.76085
Sanz-Perez, E. S., Murdock, C. R., Didas, S. A., and Jones, C. W. (2016). Direct capture of CO2 from ambient air. Chem. revs 116, 11840–11876. doi:10.1021/acs.chemrev.6b00173
Shyur, H.-J., and Shih, H.-S. (2006). A hybrid MCDM model for strategic vendor selection. Math. Comput. Model. 44, 749–761. doi:10.1016/j.mcm.2005.04.018
Siregar, D., Nurdiyanto, H., Sriadhi, S., Suita, D., Khair, U., Rahim, R., et al. (2017). Multi-Attribute decision making with VIKOR method for any purpose decision. J. Phys. Conf. Ser. 1019, 012034. doi:10.1088/1742-6596/1019/1/012034
Sky News (2022). Sky news. Available at: https://news.sky.com/story/we-plan-for-all-eventualities-minister-fails-to-rule-out-energy-rationing-this-winter-12714178 (Accessed December 07, 2022).
Stiching Global Reporting Initiative (2021). Gri 1: Foundation 2021. Amsterdam, Netherlands: Global Sustainability Standards Board.
Styring, P., Duckworth, E. L., and Platt, E. G. (2021). Synthetic fuels in a transport transition: Fuels to prevent a transport underclass. Front. Energy Res. 9, 707867. doi:10.3389/fenrg.2021.707867
Traverso, M. (2018). Is social life cycle assessment really struggling in development or is it on a normal path towards harmonization/standardization? Int. J. Life Cycle Assess. 23, 199–200. doi:10.1007/s11367-017-1387-7
Tsai, W., Yang, C.-C., Leu, J.-D., Lee, Y.-F., and Yang, C. H. (2013). An integrated group decision making support model for corporate financing decisions. Group Decis. Negot. 22, 1103–1127. doi:10.1007/s10726-012-9308-4
Tzeng, G.-H., and Liu, H.-H. (2009). “A revised VIKOR model for multiple criteria decision making - the perspective of regret theory,” in Communications in computer and information science (berlin: Springer-Verlag), 761–768.
United Nations/Framework Convention on Climate Change (2015). Adoption of the Paris agreement, 21st conference of the parties. Paris, France: United Nations/Framework Convention on Climate Change.
United Nations (2019). Department of economic and social affairs, population division. World urbanization prospects, the 2018 revision. New York, United States: United Nations. ST/ESA/SER.A/420).
United Nations (2015). Transforming our world: The 2030 agenda for sustainable development. New York, USA: United Nations General Assembly.
UNEP (2020). Guidelines for Social Life Cycle Assessment of Products and Organizations 2020. Nairobi, Kenya: United Nations Environment Programme (UNEP).
Volkart, K., Bauer, C., Burgherr, P., Hirschberg, S., Schenler, W., and Spada, M. (2016). Interdisciplinary assessment of renewable, nuclear and fossil power generation with and without carbon capture and storage in view of the new Swiss energy policy. Int. J. Greenh. Gas. Con. 54, 1–14. doi:10.1016/j.ijggc.2016.08.023
von der Assen, N., V., Lafuente, A., Peters, M., and Bardow, A. (2015). “Environmental assessment of CO2 capture and utilisation,” in Carbon dioxide utilisation: Closing the carbon cycle. Editors P. Styring, E., A. Quadrelli, and K. Armstrong (London: Elsevier), 45–55.
Wang, Y.-M., and Luo, Y. (2009). On rank reversal in decision analysis. Math. Comput. Model. 49, 1221–1229. doi:10.1016/j.mcm.2008.06.019
Wibawa, A. P., Fauzi, I. S., Irsyada, R., Dhaniyar, D., and Hernandez, L. (2019). VIKOR multi-criteria decision making with AHP reliable weighting for article acceptance recommendation. Int. J. Adv. Intell. Inf. 5, 160–168. doi:10.26555/ijain.v5i2.172
Witchalls, S. (2018). Power poverty: The new paradigm for social and economic inequality of electric vehicles. Available at: https://www.stantec.com/en/ideas/power-poverty-the-new-paradigm-for-social-and-economic-inequality-of-electric-vehicles (Accessed October 24, 2022).
World Meteorological Organisation (2010). WMO Statement on the Status of the Global Climate in 2009. Geneva, Switzerland: World Meteorological Organization (WMO).
Wunderlich, J., Armstrong, K., Buchner, G. A., Styring, P., and Schomäcker, R. (2021). Integration of techno-economic and life cycle assessment: Defining and applying integration types for chemical technology development. J. Clean. Prod. 287, 125021–125114. doi:10.1016/j.jclepro.2020.125021
Yu, X., Zhang, S., Liao, X., and Qi, X. (2018). ELECTRE methods in prioritized MCDM environment. Inf. Sci. 424, 301–316. doi:10.1016/j.ins.2017.09.061
Zavadskas, E. K., Antucheviciene, J., and Kar, S. (2019). Multi-objective and multi-attribute optimization for sustainable development decision aiding. Sustainability 11, 3069. doi:10.3390/su11113069
Zavadskas, E. K., Govindan, K., Antucheviciene, J., and Turskis, Z. (2016). Hybrid multiple criteria decision-making methods: A review of applications for sustainability issues. Econ. Research-Ekonomska Istraživanja 29, 857–887. doi:10.1080/1331677x.2016.1237302
Zavadskas, E. K., Kaklauskas, A., and Šarka, V. (1994). The new method of multicriteria complex proportional assessment of projects. Technol. Econ. Dev. Econ. 1, 131–139.
Zimmermann, A. W., and Schomäcker, R. (2017). Assessing early-stage CO2 utilization technologies — comparing apples and oranges? Energy Technol. 5, 850–860. doi:10.1002/ente.201600805
Zimmermann, A., Wunderlich, J., Buchner, G. A., Armstrong, K., Marxen, A., Michailos, S., et al. (2018). Part B: TEA guidelines, techno-economic assessment & life cycle assessment Guidelines for CO2utilization. Ann Arbor, Michigan: CO2Chem Media and Publishing, 25–97.
Zimmermann, A. W., Wunderlich, J., Müller, L., Buchner, G. A., Marxen, A., Michailos, S., et al. (2020). Techno-economic assessment guidelines for CO2 utilization. Front. Energy Res. 8 (5), 1–23. doi:10.3389/fenrg.2020.00005
Keywords: circular economy (CE), carbon, CO2 utilization, fiduciary duty, policy, social impact, LCA/TEA, behavioural change
Citation: Newman AJK, Dowson GRM, Platt EG, Handford-Styring HJ and Styring P (2023) Custodians of carbon: creating a circular carbon economy. Front. Energy Res. 11:1124072. doi: 10.3389/fenrg.2023.1124072
Received: 14 December 2022; Accepted: 21 April 2023;
Published: 09 May 2023.
Edited by:
Greeshma Gadikota, Cornell University, United StatesReviewed by:
Bing Shen How, Swinburne University of Technology Sarawak Campus, MalaysiaSohaib Mohammed, Cornell University, United States
Copyright © 2023 Newman, Dowson, Platt, Handford-Styring and Styring. This is an open-access article distributed under the terms of the Creative Commons Attribution License (CC BY). The use, distribution or reproduction in other forums is permitted, provided the original author(s) and the copyright owner(s) are credited and that the original publication in this journal is cited, in accordance with accepted academic practice. No use, distribution or reproduction is permitted which does not comply with these terms.
*Correspondence: Peter Styring, cC5zdHlyaW5nQHNoZWZmaWVsZC5hYy51aw==