- 1Faculty of Mechanical and Automotive Engineering Technology, University Malaysia Pahang Al-Sultan Abdullah, Kuantan, Malaysia
- 2Centre for Research in Advanced Fluid and Process, University Malaysia Pahang Al-Sultan Abdullah, Kuantan, Malaysia
- 3Research Centre for Nano-Materials and Energy Technology (RCNMET), School of Engineering and Technology, Sunway University, Petaling Jaya, Malaysia
- 4CoE for Energy and Eco-Sustainability Research, Uttaranchal University, Dehradun, India
Introduction: The increasing global demand for sustainable energy solutions highlights the urgency of exploring renewable resources, particularly sunlight, which is abundant and virtually limitless. This study reviews innovative technologies like solar trees, wind trees, and hybrid solar-wind trees, which are emerging as efficient structures for harnessing renewable energy.
Methods: A comprehensive SWOT analysis was conducted to evaluate the strengths, weaknesses, opportunities, and threats associated with solar, wind, and hybrid trees. The analysis also considered sustainability aspects, focusing on the efficiency and practicality of these technologies in various settings.
Results: Solar trees mimic natural foliage, utilizing solar modules to convert sunlight into electricity, while wind trees incorporate micro-wind turbines and solar panels, effectively harnessing both wind and solar energy. Hybrid solar-wind trees combine these technologies to provide a consistent energy supply. These structures are compact, cost-effective, and adaptable to urban landscapes.
Discussion: Challenges such as land use, aesthetic considerations, and public perception were identified. The review emphasizes the need for future research to optimize configurations and address these challenges, ensuring the successful integration of these technologies into sustainable urban landscapes.
Conclusion: This review provides critical insights for renewable energy researchers, particularly in the development of hybrid wind and solar power systems, promoting energy security and climate resilience.
1 Introduction
Energy, manifesting in diverse forms, delineates a system’s inherent ability to perform work. The gamut of energy resources spans both non-renewable and renewable varieties notably, except for nuclear, tidal, and geothermal energies, all other energy sources ultimately derive from the Sun (Arunachalam and Fleischer, 2008). In the past decade, the urgency to drive down the per-watt cost of solar energy devices has intensified amid dwindling renewable energy resources. Solar power stands out as a frontrunner among promising renewable energy sources. The Earth is showered daily with sunlight emitting over 1366W, representing a substantial energy potential (Pérez-Lombard et al., 2008). Solar energy represents an inexhaustible and cost-free power source, boasting immense potential due to the abundant solar radiation reaching the Earth. Approximately 30% of this solar radiation is reflected in space, while the oceans, clouds, and land masses absorb the remaining portion. Solar power is a fantastic choice for generating electricity because it's widely available and sustainable (Premier, 2020), (Rajamony et al., 2024). It is clean, abundant, and sustainable, making it an ideal choice for a world struggling to transition to a low-carbon economy.
Technological advances in solar heating, photovoltaic cells, thermal power, solar fuels, solar buildings, and synthetic photosynthesis can utilize the free energy provided by sunlight. Concerning a natural energy source, solar energy originates from the radiant light and warmth of the sun. Many advanced technologies capture this, including solar thermal energy, solar architecture, solar heating, molten salt power plants, and artificial photosynthesis (Choifin et al., 2021), (Thakur et al., 2022). Creating highly efficient solar cells, panels, and modules has led to several research endeavors that have improved solar energy utilization. Photovoltaic (PV) systems are the most widely utilized technique for producing energy from solar power. The quantity of sunshine obtained in a given region is the primary factor determining how well solar photovoltaics operate. The ability of solar energy to convert sunlight into usable electricity without any intervention is another significant advantage associated with it, thanks to small photovoltaic cells (Liu et al., 2019).
The accurate assessment and prediction of solar radiation is critical to developing solar energy conversion systems. One of the main benefits of solar power is its widespread availability and affordability for the typical person compared to the expenses of fossil fuels and oils in the past and present. Additionally, compared to conventional energy production techniques, solar energy has significantly reduced labor costs (Shaikh, 2017). The installation of solar panels in both residential and commercial establishments is commonly seen as an ecologically conscientious move that also saves money on energy bills. This tendency has experienced a substantial increase in recent years and is anticipated to continue as technology developments reduce expenses. The solar business is expanding rapidly worldwide, creating more jobs than many other industries. This trend continues as countries establish laws encouraging renewable energy worldwide. With further developments and increased costs, solar energy is expected to become an even more appealing alternative for consumers across numerous applications. (Dey and Pesala, 2020).
While solar energy has many advantages, there are also a few drawbacks. One significant barrier is the upfront cost of installing solar panels, which can still be too expensive for many homes and businesses, even with recent price decreases. There is also the added difficulty of weather variability impeding the use of solar energy (Shaikh, 2017). Because solar panels need sunshine to produce power, they perform less well on gloomy or foggy days. When sunshine is scarce, energy storage devices can store the sun’s excess electricity for later use. Solar tree technology addresses challenges such as land use, aesthetics, and efficiency. A study compares a traditional flat PV module with a sunflower-shaped solar tree across different tilt angles to assess energy output variations. Results indicate the sunflower module generates 16%–23% more energy and operates cooler (41°C vs. 51°C). It also saves 85% in land use and lowers ambient temperatures by 3°C in shaded areas, potentially benefiting agriculture. Solar tree technology offers a solution to the visual and spatial challenges of traditional solar systems in urban settings, promoting sustainable development. These innovative structures vary in design and utilize diverse methodologies, from basic to advanced algorithms. The choice of PV modules depends on the tree's design, location, and local weather conditions. Maximum Power Point Tracking (MPPT) optimizes solar panel efficiency by adjusting panel angles to follow sunlight. Inspired by natural trees, solar panels are arranged using phyllotaxy patterns like 3/8 and 2/5, akin to Fibonacci sequences, enhancing energy capture compared to conventional unidirectional panels under similar irradiance conditions. Nevertheless, solar photovoltaic (PV) technology has geographical limitations, much as ground-mounted systems (Gangwar et al., 2019a). The cost of installing solar PV modules is the need for land, which is always at a premium, especially in urban areas with limited space for development. The amount of energy in the form of solar irradiance is shown in Figure 1 below.
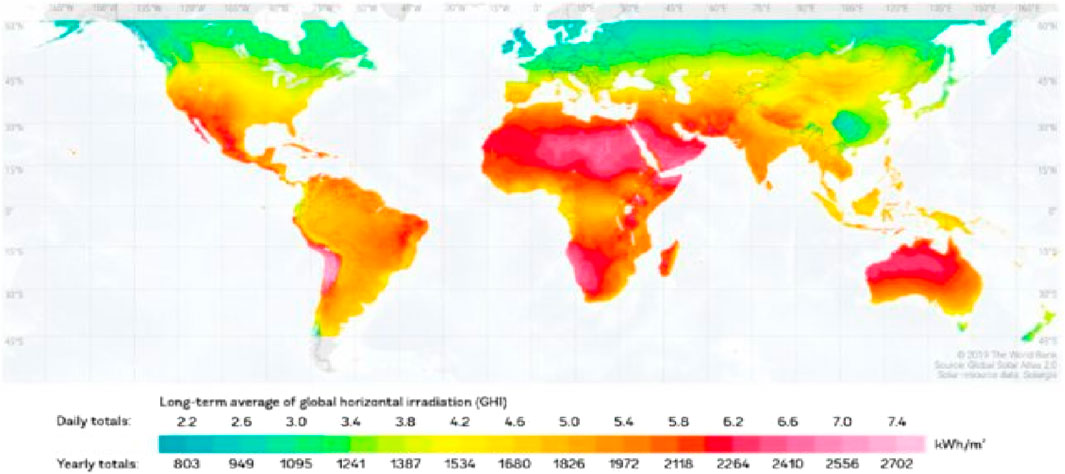
Figure 1. Global solar irradiance (Gürel et al., 2023).
The concept of the wind tree represents a groundbreaking approach to wind energy, drawing inspiration from the elegance and effectiveness of natural processes. These sophisticated structures are designed to harness the wind’s kinetic energy and convert it into usable electricity. Resembling the graceful form of a tree, they feature slender branches adorned with small-scale wind turbines. This innovative design serves a practical purpose and adds to the aesthetic appeal of renewable energy solutions.
Wind trees offer a harmonious blend of beauty and functionality, making them valuable to the renewable energy landscape. Their ability to seamlessly integrate into urban and rural environments is particularly noteworthy. Wind trees provide a compelling alternative in areas where traditional wind farms may not be viable due to space constraints or visual impact. Their modest footprint and visual appeal offer communities a means of embracing clean energy without sacrificing visual harmony or compromising efficiency. A study investigates the feasibility of implementing a novel wind power generation system, termed tree-shaped wind turbine (TSWT), in urban areas of Hormozgan Province, Iran. Through techno-economic evaluation using HOMER software, eight urban locations were assessed for optimal placement of 25 TSWTs. Jask city exhibited the highest electricity production potential at 529,450 kWh/yr, while Bandar Abbas showed the lowest at 339,275 kWh/yr. Multi-criteria decision-making methods, including data envelopment analysis and fuzzy TOPSIS, ranked Sirik as the most suitable location based on criteria such as electricity output, cost-effectiveness, population density, land value, environmental impact, and natural disaster frequency.
In addition to adding visual impact to landscapes, wind trees offer a decentralized method of electricity generation. By relieving strain on centralized electrical grids, this decentralization improves the robustness of the energy infrastructure. Since wind trees may be positioned to maximize energy production and reduce transmission losses, this dispersed concept also promotes greater energy independence. The hybrid tree that combines solar and wind power is an inventive way to combine two powerful renewable energy sources. This innovative idea offers a comprehensive approach to sustainable energy generation by combining wind turbines’ predictable energy output with solar photovoltaic technology’s efficiency. The solar wind hybrid tree was designed with the organic structure of trees in mind. It has small wind turbines incorporated into its tall branches to collect wind energy and solar panels on its large leaves to collect sunlight. It is appropriate for both urban and rural environments because of the smooth integration of solar and wind technologies, which increases energy output while minimizing land consumption. The solar wind hybrid tree offers a versatile option for communities looking for sustainable energy solutions since it blends into various environments. Its capacity to produce power from wind and solar energy sources guarantees a consistent and reliable flow of renewable energy, boosting resilience and energy security (Ghadge, 2023).
A recent research introduces a 3 kW hybrid tree design located in Vaddeswaram, Andhra Pradesh (16.26°N and 80.36°E), integrating 2 kW of solar and 1 kW of wind power. The structure mimics natural trees, supporting solar modules and wind turbines to supply energy for mobile devices, laptops, electric vehicles, and household appliances. It employs a two-axis tracking system to maximize energy production. The study includes P–V and I–V characteristics of solar panels under varying conditions, and power characteristics of the wind turbine at different wind speeds. Structural optimizations ensure durability, with the hybrid tree yielding 4709 kWh/year compared to 3763 kWh/year from fixed solar panels at an 18.25° tilt angle. Moreover, combining wind and solar technology into one building promotes energy autonomy by reducing dependency on centralized power grids and promoting the decentralization of energy generation. This decentralized strategy positions the solar wind hybrid tree as an appealing asset in renewable energy, aligning with innovation and environmental preservation objectives. Its capacity to concurrently utilize two renewable energy sources and mix them with the environment highlights its dedication to promoting clean, effective, and environmentally responsible power generation (Torres-Barrán et al., 2019).
Solar power harnesses the radiant energy emitted by the sun through photovoltaic cells or solar thermal systems, effectively converting it into either electricity or heat. Wind energy, on the other hand, capitalizes on the kinetic force of wind to set turbines in motion, thereby generating electrical power. Hybrid systems, a fusion of both solar and wind technologies, have emerged as a strategic approach to optimize energy production. By integrating these two renewable sources, hybrid systems exploit their complementary nature, thereby enhancing overall efficiency. These sustainable energy solutions offer environmentally sound alternatives to traditional fossil fuels, thereby playing a significant role in combating climate change. Moreover, they contribute to greater energy independence and security, reducing reliance on finite resources. In summary, solar, wind, and hybrid technologies represent pivotal advancements in the global pursuit of sustainable energy, heralding a future where renewable sources dominate the energy landscape.
This review delves deeply into the innovative aspects of solar and wind tree technology, offering a captivating exploration of this emerging field. It provides a comprehensive overview of these unique arboreal structures, showcasing operational examples and meticulously examining the underlying concepts and requisite materials. Through a meticulous comparison with conventional solar, wind, and hybrid systems, the paper underscores the significance of solar and wind trees. Moreover, it addresses the challenges associated with implementing this technology and presents viable solutions. The study ventures into the forefront of solar and wind tree technology, presenting a fresh perspective on renewable energy generation. These tree-like installations harness solar and wind energy by emulating natural processes and employing inventive engineering, resulting in a harmonious blend of aesthetic appeal and practicality. The essay endeavors to underscore the distinctive advantages of solar and wind trees while acknowledging any potential limitations, shedding light on the promise of these renewable energy sources.
A SWOT (Strengths, Weaknesses, Opportunities, Threats) analysis forms an integral part of the examination to thoroughly evaluate the feasibility and potential ramifications of solar and wind tree technology. Notable strengths include their compact design, adaptability, and ability to seamlessly integrate into urban landscapes. Conversely, weaknesses may encompass fluctuations in energy production and initial investment costs. Technological advancements and the escalating demand for sustainable energy solutions present opportunities, yet regulatory hurdles and competition from conventional energy sources pose threats. Furthermore, sustainability emerges as a central theme throughout the research. As alternatives to fossil fuels, solar and wind power play a pivotal role in mitigating greenhouse gas emissions and reducing reliance on finite resources. Their integration into urban environments can foster environmentally conscious cities and enhance public awareness of environmental issues. However, achieving long-term sustainability necessitates addressing challenges such as sourcing materials, managing end-of-life disposal, and ensuring equitable access to renewable energy sources.
It is found from the literature, that there is no review paper based on the SWOT analyses of power generation technologies. Hence, this study delves into the groundbreaking realm of wind and solar tree technology, shedding light on its pivotal significance in steering us toward sustainable energy solutions. Through an intricate analysis of sustainability principles and SWOT (Strengths, Weaknesses, Opportunities, Threats) factors, the research strives to deepen our comprehension of renewable energy technology and its seamless integration into an increasingly environmentally conscious society.
2 Overview of solar tree
2.1 Theory of solar power
The solar energy system’s photovoltaic (PV) cell is essential because it effectively transforms sunlight into electrical power. This cell determines the difference between n-type and p-type materials by acting as a p-n junction device. Donor impurity atoms provide electrons to n-type materials, whereas acceptor impurity atoms produce holes in p-type materials. Solar cells function fundamentally through the photovoltaic effect, comprising three basic processes.
The p-n junction is essential in semiconductor physics, and photon absorption is essential to its functioning. Electron-hole pairs are produced by photons interacting with a semiconductor material at energy levels higher than the energy gap. This event causes a hole in the valence band as electrons are lifted from the band to the conduction band. The kinetic energy of the electron or hole is increased by any extra energy carried by the photon over the minimal threshold needed for this electron-hole pair production. This excess energy usually escapes the semiconductor material as heat.
As a result, when light interacts with semiconductor material, charge carriers separate. The positively charged “holes” can wander the p-region, contributing to the external solar circuit. In contrast, negatively charged electrons can leave the n-region and travel around the circuit, performing electrical functions before recombining with the holes. This consecutive process eventually leads to the release of electrons capable of powering the electric circuit. Following their travel through the circuit, these electrons return to the holes.
This process elucidates how light energy is converted into electrical energy within a semiconductor, providing insights into the fundamental workings of photovoltaic devices (Balaji and Rao, 2020). In semiconductor design, ensuring that the n-type layer is thinner than the p-type layer is imperative. This strategic choice facilitates swift electron flow through the circuit, promoting efficient current generation before the electrons recombine with the holes. Applying an anti-reflective coating on the n-layer also initiates surface reflection, amplifying light transmission within the semiconductor material (Wadaskar et al., 2020).
2.2 Classification of solar panels for solar tree
Solar panels can be classified into three primary categories based on their materials and technologies: monocrystalline, polycrystalline, and thin film. Monocrystalline panels are crafted from single-crystal silicon, granting them superior efficiency. Conversely, polycrystalline panels utilize multiple silicon crystals, providing a more economical alternative. Thin-film panels of amorphous silicon or cadmium telluride prioritize flexibility and a lightweight build. Each type boasts unique advantages and is tailored for specific applications in solar energy generation. Each classification has distinct advantages and considerations, as shown in Table 1, allowing for tailored applications in diverse solar energy systems.
2.3 Working principle and Components of Solar Tree
In a photovoltaic (PV) system, three crucial elements work harmoniously: the solar panel, supercapacitor, and inverter. The solar panel captures solar energy and converts it into electrical power using photovoltaic technology. Accompanying this, the supercapacitor is a backup, storing excess energy when sunlight is abundant. As a result, the generated direct current (DC) is converted into alternating current (AC) by the inverter, which is more suitable for powering household appliances. This synchronized operation of the components optimizes energy utilization in the PV system, ensuring a reliable electricity supply for household needs (Kumar et al., 2022a).
2.3.1 Formation of the depletion region
A solar cell can be defined as a specialized semiconductor device designed to harness sunlight and convert it into electrical energy. A diode, constructed from a single crystal semiconductor material, typically silicon, lies at its heart. One side of this semiconductor material is infused with pentavalent impurities, creating an n-type region. Conversely, the other side is infused with trivalent impurities, establishing a p-type region. This distinct arrangement enables the solar cell to effectively capture photons from sunlight and transform them into usable electricity (Ahmed and Mohamed, 2021). The doping process involves introducing extra mobile carriers, primarily majority carriers, into each semiconductor region. When n-type and p-type semiconductors are brought together, a phenomenon occurs where electrons from the n-region diffuse into the p-region, generating a zone near the junction with positively charged donor atoms. At the same time, holes from the p-region migrate into the n-region, leaving an area near the junction with negatively charged acceptor atoms. This process forms a p-n junction characterized by the redistribution of charge carriers and the establishment of an electric fie (Choifin et al., 2021) ld. The subsequent shifts of valence electrons can be observed as movements of holes within a material. This movement of electrons and holes leads to the generation of a current known as diffusion current, denoted as “Idiff,” and creates a region depleted of charge carriers, termed the depletion region or space charge region. An induced electric field arises, segregating electrons and holes from this depletion region, thereby hindering any further flow of charge carriers (Bista et al., 2018).
Photovoltaic (PV) energy conversion relies on electronic semiconductor devices, primarily crystalline silicon (c-Si) or thin-film semiconductor materials. A c-Si solar system typically comprises two primary types of crystalline materials: monocrystalline and multi-crystalline solar modules. Monocrystalline semiconductors exhibit superior electrical characteristics, achieving approximately 20% efficiency, comparable to polycrystalline materials (Patil and Madiwal, 2016). Despite the widely held belief that monocrystalline PV modules are uneconomical due to the high cost of crystalline wafer-based technology, thin-film technology provides an alternative. Thin-film solar cells, which use materials like amorphous silicon (a-Si), cadmium telluride (CdTe), copper indium gallium selenide (CIGS), or gallium arsenide (GaAs), are a possible option. These cells are much thinner than traditional crystalline silicon cells, ranging in thickness from a few nanometers to tens of micrometers. Because of their thinness, thin-film modules are lightweight and flexible, making them suitable for various applications. Furthermore, thin-film technology is widely acknowledged for its cost-effectiveness compared to traditional wafer-based techniques (Dey et al., 2018).
2.3.2 Various configurations of solar cells
Over the years, various types of solar cells have been developed, each with different materials and technologies, impacting their efficiency and suitability for different applications. Here’s a comparative analysis focusing on some key types of solar cells:
2.3.2.1 Crystalline silicon solar cells
Types:
• Monocrystalline Silicon: Made from single-crystal silicon, giving it a uniform appearance. Higher efficiency due to better electron flow.
• Polycrystalline Silicon: Made from multiple crystals, which can result in lower efficiency compared to monocrystalline.
Efficiency:
• Monocrystalline: Typically achieves efficiencies of 20% to 25%.
• Polycrystalline: Efficiency ranges from 15% to 20%.
Advancements:
• Improved manufacturing techniques have increased efficiency and reduced production costs.
• Bifacial panels capture sunlight from both sides, increasing overall efficiency.
• PERC (Passivated Emitter Rear Cell) technology improves light absorption and reduces electron recombination.
2.3.2.2 Thin-film solar cells
Types:
• Amorphous Silicon (a-Si): Non-crystalline form of silicon deposited on a substrate. Flexible and lightweight.
• Cadmium Telluride (CdTe): Uses cadmium and tellurium as the semiconductor material. Lower cost but efficient.
Efficiency:
• Amorphous Silicon: Efficiency typically ranges from 7% to 12%.
• Cadmium Telluride: Efficiency can reach up to 22%.
Advancements:
• Enhanced deposition techniques have improved efficiency and reduced material usage.
• Tandem structures combining different materials (like perovskites or silicon) promise higher efficiencies.
• Improved stability and longevity through better encapsulation and substrate materials.
2.3.2.3 Perovskite solar cells
Types:
• Organic-Inorganic Hybrid Perovskites: Lead halide-based materials with organic cations.
• All-Inorganic Perovskites: More stable due to absence of organic components.
Efficiency:
• Organic-Inorganic Hybrid: Efficiency record exceeded 25%, with average efficiencies around 20%.
• All-Inorganic: Efficiency records approaching 25%.
Advancements:
• Rapid efficiency improvements since their discovery in 2009.
• Potential for low-cost, scalable production using solution-based processing.
• Stability enhancements through material engineering and encapsulation strategies.
Advancements in solar PV Technology
• Efficiency Improvements: Across all types, there's a trend towards higher efficiencies through better materials, manufacturing processes, and novel cell architectures.
• Cost Reduction: Economies of scale, improved production methods, and material innovations have significantly reduced the cost per watt.
• Durability and Longevity: Enhanced reliability and longer lifespan due to better encapsulation materials and improved understanding of degradation mechanisms.
• Integration and Application: Solar cells are increasingly integrated into building materials (building-integrated photovoltaics, or BIPV) and everyday objects (wearables, electronics).
Each type of solar cell has its strengths and weaknesses in terms of efficiency, cost, and suitability for different applications. Crystalline silicon dominates the market with steady efficiency improvements and reliability. Thin-film technologies offer flexibility and cost advantages but with varying efficiencies. Perovskite solar cells show remarkable potential for high efficiency and low-cost production but are still in the early stages of commercialization. Advancements in solar PV technology continue to focus on increasing efficiency, reducing costs, improving durability, and expanding applications, making solar energy an increasingly viable and competitive source of renewable energy globally.
2.3.3 Working principle
A solar tree appears like a genuine tree, with solar panels arranged like leaves on branches connected to a central pole. These panels are intentionally oriented to absorb as much sunlight as possible. After absorbing sunlight, the panels turn it into electrical energy by converting direct current (DC) to alternating current (AC) via an inverter. This unique design harvests solar electricity effectively and resembles the natural process of photosynthesis in plants (Sikka, 2019). Numerous infrastructures, including residences, companies, and other buildings, can be powered by alternating current (AC) electricity produced by solar panels. These solar panels are primarily photovoltaic cells made of semiconductor materials like silicon. They are frequently grouped in shapes that resemble trees. Photons from the sun’s rays interact with these panels, displacing electrons from semiconductor material atoms and starting an electric current. Notably, the quantity of power produced is closely correlated with the sun’s strength (Mishra et al., 2020). The concept of a solar tree represents a decentralized approach to power generation, aiming to produce electricity on a local scale instead of depending solely on centralized power plants. This innovative system offers potential benefits in mitigating our dependency on fossil fuels and ultimately reducing our carbon emissions. Solar trees can find application in diverse environments such as residential areas, commercial complexes, and public domains, contributing to a more sustainable energy landscape. The performance of solar photovoltaic (PV) cells is often assessed using several key parameters, including the fill factor (FF) and the power output. Understanding the relationship between these parameters is crucial for optimizing the efficiency of solar PV systems.
Fill factor
where:
• FF is the fill factor of a solar cell.
•
•
•
•
Solar power output:
where:
• P is the power output in watts (W).
• A is the area of the solar panel in square meters (m2).
• S is the solar irradiance in watts per square meter (W/m2).
• η is the efficiency of the solar panel (a fraction between 0 and 1).
2.3.4 Components of solar tree
Solar trees comprise several components, as shown in Figure 2, including solar panels, inverters, mounting systems, wiring and electrical components, battery storage, and protective casing. The details of each component are discussed below.
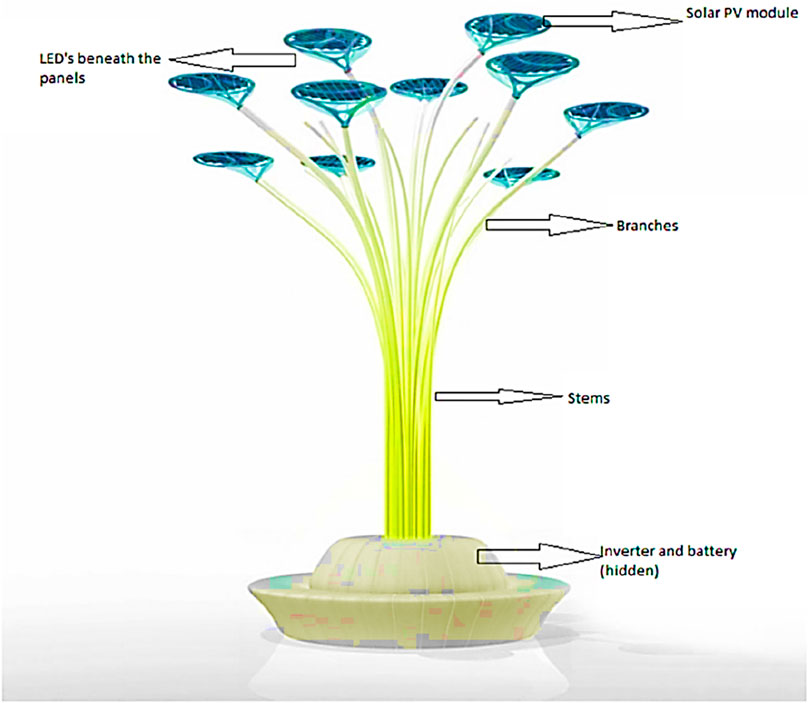
Figure 2. Components of solar tree (Hyder et al., 2018a).
Solar panels are the main component of the solar tree and are responsible for converting sunlight into electricity. Solar panels comprise photovoltaic cells made of semiconductor materials such as silicon.
The inverter is responsible for converting the solar panels’ direct current (DC) electricity generated into alternating current (AC) to power homes and buildings.
The mounting system is responsible for holding the solar panels in place and positioning them at the optimal angle to maximize their exposure to sunlight. The mounting system can be fixed or adjustable, depending on the design of the solar tree.
The central pole is the main support structure for the solar tree and is typically made of steel or another strong material.
Wiring and electrical components: The solar tree will also have wiring and other electrical components to connect the panels to the inverter and distribute the electricity generated by the system.
Battery storage: Some solar tree systems may also include battery storage, which allows excess electricity to be stored for use when the panels are not generating electricity, such as at night or on cloudy days.
Protective casing: The solar tree may also have a protective casing or enclosure to protect the panels and other components from the elements and improve the system’s overall appearance.
2.4 SWOT analysis of solar tree
Solar trees represent a promising avenue for sustainable energy, but addressing their weaknesses and seizing opportunities is essential for their successful adoption in urban landscapes. Below is Table 2 displays the detailed SWOT analysis of solar trees.
3 Overview of wind tree
3.1 Theory of wind power
Wind power, a renewable energy source, harnesses the kinetic energy of moving air to generate electricity. The underlying principle revolves around the conversion of wind energy into mechanical energy through the use of wind turbines (Tomczak et al., 2020). These turbines consist of rotor blades attached to a central hub, which rotates when exposed to the force of the wind. The kinetic energy acquired from this rotation is then transferred to a generator and converted into electrical energy.
The efficiency of wind power generation depends on various factors, including wind speed, turbine design, and the height of the turbines. Higher wind speeds typically result in increased energy production, and advancements in turbine technology have led to the development of larger, more efficient models. Wind turbines are strategically positioned in areas with consistent and substantial wind flow, such as coastal regions or open landscapes, to maximize their energy output (Cuce et al., 2022).
Despite its eco-friendly nature, the wind power theory is not without challenges. Variability in wind speed can lead to fluctuations in energy production, necessitating energy storage solutions for a stable power supply (Sachin et al., 2023). Additionally, concerns about visual and noise impacts and potential effects on wildlife must be addressed when planning and implementing wind power projects. As technology advances and the demand for clean energy grows, the theory of wind power is a pivotal component in the global shift toward sustainable and renewable energy sources (Awaze et al., 2018).
3.2 Classification of wind turbines for wind tree
Classifying wind turbines for a wind tree involves categorizing these structures based on design, size, and functionality. Wind trees typically house small-scale turbines that differ from traditional horizontal-axis wind turbines (HAWTs). One classification is based on the turbine’s axis orientation: vertical and horizontal-axis. Vertical-axis wind turbines (VAWTs) are often employed in wind trees, with their blades arranged vertically around a central axis. The Wind Tree can take advantage of all different kinds of wind, from light breezes to strong gusts of wind, and it can do so in both urban and rural settings, as shown in Figure 3. This design allows for omnidirectional wind capture, making them suitable for compact installations. Other unique designs of wind turbines with vertical axes are Savonius and Darrieus types (Janamala, 2021).
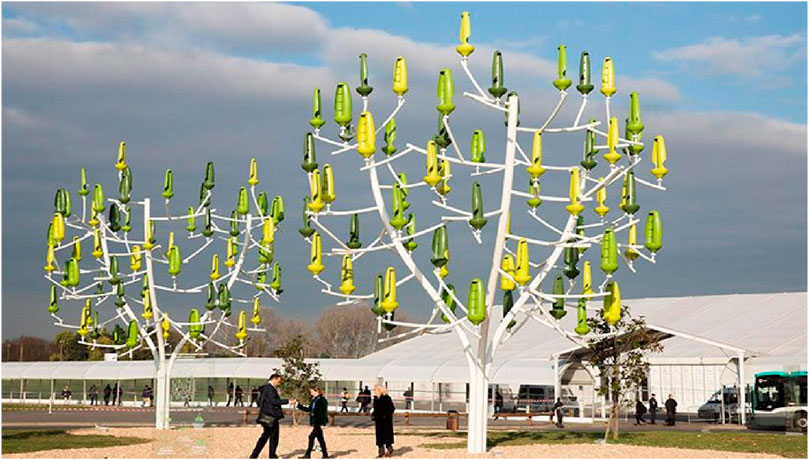
Figure 3. Wind tree (Source: https://www.startupselfie.net/2022/07/23/the-wind-tree/ and New World wind).
3.2.1 Horizontal-axis wind turbines (HAWT)
Design: HAWTs have their rotating axis oriented horizontally. They typically feature three blades designed to face the wind (Hyder et al., 2018b).
Efficiency: HAWTs operate efficiently when directly pointing into the wind. As the wind direction changes, the entire head of the turbine must turn (or “yaw”) to maintain optimal alignment.
Applications:
• Offshore wind farms.
• Onshore wind farms in flat and open areas with less turbulent wind.
Scalability: HAWTs can be built at various scales, from small-scale versions for home use to massive turbines for wind farms. Their scalability makes them cost-effective for large installations (Suliński et al., 2021).
3.2.2 Vertical-axis wind turbines (VAWT)
Design: VAWTs have their rotating axis aligned vertically. Unlike HAWTs, they harness kinetic energy in the opposite direction.
Efficiency and Size: VAWTs are less efficient and less common. They cannot be built at the large scales seen in HAWT wind farms. The largest VAWT ever built was the 110-m-tall, 3.8 MW “ÉOLE” turbine in Quebec, Canada.
Applications:
• Smaller-scale installations, such as residential or experimental setups.
• Urban environments where space constraints limit the use of HAWTs.
3.2.3 Savonius wind turbine
The Savonius wind turbine is a type of vertical-axis wind turbine (VAWT). It consists of curved aerofoil blades mounted on a rotating shaft or framework. The blades are arranged symmetrically, allowing them to capture wind from any direction without rotating. As the rotor spins, the curved blades experience differential drag due to their shape. This causes the turbine to rotate. Savonius turbines are drag-type devices extracting less wind power than lift-type turbines (Torres-Barrán et al., 2019).
The advantages are low noise levels. Savonius turbines operate quietly. Secondly, it can generate power even at low wind speeds. Thirdly, relative independence on wind direction. Unlike horizontal-axis turbines, Savonius turbines don't need to face directly into the wind. The limitations of this type of wind turbine are lower efficiency. Their average maximum efficiency is around 20%. Secondly, the ground-level energy extraction. If mounted low without an extended post, wind speeds near the ground limit overall energy extraction (Debadatta Behera et al., 2020).
3.2.4 Darrieus wind turbine
The Darrieus wind turbine is another type of vertical-axis wind turbine. It uses curved aerofoil blades mounted on a rotating shaft or framework. Unlike Savonius turbines, Darrieus turbines utilize lift forces generated by the airflow hitting the aerofoils. The curvature of the blades allows them to be stressed only in tension at high rotating speeds.
The advantages are that Darrieus turbines can harness wind regardless of its direction. Other than that, it is suitable for urban and low-wind areas. Their versatility makes them ideal for urban environments and regions with varying wind patterns. The challenges of Darrieus wind turbines are that protecting them from extreme wind conditions is difficult—secondly, they have a self-starting mechanism. Ensuring self-starting capability remains a challenge (Batiles and Culaba, 2019).
3.3 Working principle
A wind tree’s working principle revolves around converting kinetic energy from wind into electrical power through a network of small-scale vertical-axis wind turbines (VAWTs) integrated into the tree-like structure. Unlike traditional horizontal-axis turbines, the vertical-axis design allows for omnidirectional wind capture, making wind trees suitable for urban and residential environments where wind direction is variable (Almadhhachi et al., 2022). As the wind flows through the tree, the vertical-axis turbines rotate around a central axis, typically arranged in a helical or similar configuration. The rotation of the turbines activates a generator, transforming the mechanical energy into electrical energy. This generated electricity is then channeled through the tree’s infrastructure to be utilized locally or fed into the electrical grid (Debadatta Behera et al., 2020). In wind power generation, the capacity factor and the tip speed ratio are two important metrics that help evaluate the performance and efficiency of wind turbines.
3.3.1 Capacity factor
The capacity factor of a wind turbine (or any power generation facility) is the ratio of the actual output over a period of time to the potential output if the turbine had operated at full capacity for the same period.
• CF is the capacity factor
• P is the average power output of wind turbine
• Prated is the rated power of wind turbine
• 8760 is the total hours in a year
Typical values:
• For wind turbines, the capacity factor typically ranges from 20% to 50%, depending on the location, wind conditions, and turbine technology.
Factors influencing capacity factor:
1. Wind Speed: More consistent and stronger winds lead to a higher capacity factor.
2. Turbine Technology: More advanced turbines with better efficiency and control systems can achieve higher capacity factors.
3. Maintenance and Downtime: Less downtime for maintenance and repairs results in a higher capacity factor.
4. Site Conditions: Geographic and climatic conditions at the site can significantly impact the capacity factor.
Tip speed ratio (TSR)
The tip speed ratio is the ratio of the speed of the tips of the turbine blades to the speed of the wind.
• λ is the tip speed ratio
• R is the radius of the blade (m)
•
•
Optimal TSR:
• Different types of wind turbines have different optimal TSRs.
• Horizontal Axis Wind Turbines (HAWTs) typically have an optimal TSR between 6 and 8.
• Vertical Axis Wind Turbines (VAWTs) have a lower optimal TSR, typically between 1 and 3.
Factors influencing TSR:
1. Blade Design: Longer, narrower blades (high aspect ratio) have higher optimal TSRs.
2. Turbine Type: HAWTs generally operate at higher TSRs compared to VAWTs.
3. Wind Speed: TSR needs to be adjusted based on varying wind speeds to maximize efficiency.
Relationship between capacity factor and tip speed ratio.
While the capacity factor and tip speed ratio are related to the overall performance of wind turbines, they are influenced by different aspects of turbine operation. The capacity factor is primarily a measure of how effectively a turbine converts available wind into electricity over time, whereas the tip speed ratio is a more immediate measure of how efficiently a turbine’s blades are converting wind energy into rotational energy.
Maintaining the optimal TSR is crucial for achieving high aerodynamic efficiency and, consequently, a higher capacity factor. If the TSR is too low, the blades are moving too slowly, leading to poor aerodynamic performance. If the TSR is too high, the blades move too fast, causing increased drag and reduced efficiency.
Practical considerations
1. Wind Resource Assessment: Before installing a wind turbine, a detailed wind resource assessment should be conducted to estimate the expected capacity factor.
2. Turbine Selection: Choose a turbine with a design that matches the wind conditions of the site to maintain an optimal TSR.
3. Monitoring and Control: Modern turbines are equipped with control systems to adjust the blade pitch and rotational speed to maintain an optimal TSR under varying wind conditions.
Understanding and optimizing both the capacity factor and tip speed ratio are crucial for maximizing the efficiency and output of wind power generation.
Wind power output:
where:
• Pwind is the power output in watts (W).
• ρ is the air density in kilograms per cubic meter (kg/m³), typically about 1.225 kg/m³ at sea level.
• A is the swept area of the wind turbine blades in square meters (m2), given by A=πR2 where R is the radius of the rotor.
• v is the wind speed in meters per second (m/s).
• Cp is the power coefficient (a fraction between 0 and 1), which represents the efficiency of the wind turbine in converting wind energy to electrical energy. The maximum theoretical value is the Betz limit, approximately 0.59.
3.4 Components of wind tree
The components of a wind tree are as follows:
Turbines: The heart of a wind tree lies in its turbines. Each turbine is made of alloy material and shaped based on vertical or horizontal axis design. These turbines capture wind energy and convert it into electricity.
Generator: The generator transforms the mechanical energy generated by the rotation of the turbines into electrical energy connected to the turbines. This electricity is then available for immediate use or can be stored in batteries for later use.
Tower or trunk structure: The tower or trunk is designed to mimic a tree’s trunk serving as the support structure for the turbines. It provides stability to the wind tree and serves as a conduit for electrical wiring, ensuring the efficient transmission of generated power.
Control system: An integral part of the wind tree, the control system monitors and manages the operation of the turbines. It adjusts the orientation of the turbines to optimize energy capture based on wind direction and speed. The control system may also include safety features to protect the wind tree during extreme weather conditions.
Base/Foundation: Anchored securely to the ground, the base or foundation provides stability and support to the entire wind tree structure. It is designed to withstand the forces exerted by the wind on the turbines and ensures overall structural integrity.
Electrical wiring and distribution system: This system facilitates the flow of electricity from the generator to the desired destination, whether for local consumption or integration into the electrical grid.
3.5 SWOT analysis of wind tree
This renewable energy source has been utilized for centuries and is crucial in our transition toward cleaner power generation. Wind energy originates in solar radiation, as temperature differences create air movements that we perceive as wind. Understanding wind physics and optimizing turbine design is essential for maximizing its potential and contributing to a greener future. Table 3 displays a detailed discussion of the SWOT analysis of the wind tree.
4 Overview of solar wind hybrid tree
4.1 Theory of hybrid power
The theory of solar and wind hybrid power revolves around combining the strengths of solar photovoltaic (PV) systems and wind turbines to create a more reliable and efficient renewable energy solution. This hybrid approach aims to address the intermittent nature of individual solar and wind systems by capitalizing on their complementary characteristics (Vyas et al., 2022). Solar power is most abundant during daylight hours, while wind energy peaks at different times, often during the night or in periods of lower sunlight (Patil, 2018). By integrating both technologies, the hybrid system seeks to maximize energy production throughout the day and night, increasing overall system efficiency.
The hybrid system typically incorporates solar panels and wind turbines into a single energy generation infrastructure. The solar panels convert sunlight into electricity, and wind turbines harness the kinetic energy from the wind to generate power. Both energy sources are then integrated into a standard energy storage system, such as batteries, to store excess energy during peak production times and release it when demand is high or during low solar and wind activity (Gangwar et al., 2019b).
4.2 Classification of hybrid tree
Solar and wind hybrid trees have been classified based on their design and capacity. One classification is based on the capacity of the hybrid tree, such as a 3 kW hybrid tree consisting of 2 kW solar and 1 kW wind. Another classification is based on the capacity of the wind-solar tree, such as a compact and low-cost wind-solar tree of 500 W capacity. Hybrid trees can also be classified based on their structure, such as a metallic tree shape with micro-wind turbines and solar panels. Another classification is based on the support structure, where the hybrid tree is designed to resemble vegetation using camouflage elements. Furthermore, hybrid trees can also be classified based on the arrangement of the branches and elements, such as a tree with different orders of branch elements carrying wind-solar elements (Solomin et al., 2021).
4.3 Working principle and components of hybrid tree
A solar and wind hybrid tree’s working principle involves integrating solar photovoltaic (PV) panels and wind turbines or aero-leaves within a single structure, as shown in Figure 4. This hybrid system is designed to capitalize on the complementary nature of solar and wind energy, enhancing overall efficiency and providing a more consistent power supply. Here’s a breakdown of the working principle.
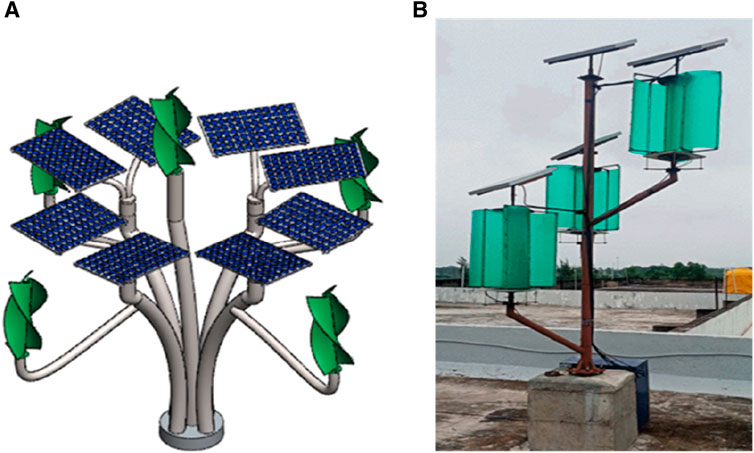
Figure 4. (A) Schematic of wind tree (Eltayeb et al., 2023). (B) real-time view of the hybrid solar-wind tree (Reddy et al., 2022).
During daylight hours, solar panels mounted on the tree’s structure capture sunlight and convert it into electrical energy through the photovoltaic effect. The solar energy the panels absorb is then transformed into direct current (DC) electricity (Kaur and Singh, 2021). Simultaneously, vertical-axis wind turbines, arranged on the tree, harness kinetic energy from the wind. The rotating blades of the turbines convert the wind’s mechanical energy into electricity through a generator. The electricity generated by solar panels and wind turbines is integrated into a standard electrical system within the tree. An energy storage system, often in the form of batteries, stores excess energy produced during peak solar or wind conditions. This stored energy can be tapped into during periods of low energy production, ensuring a continuous and reliable power supply. A sophisticated control system manages the operation of both the solar panels and wind turbines. It optimizes the orientation of the turbines based on wind direction and adjusts the solar panel angles for maximum sunlight exposure. The control system monitors energy production and distribution, making real-time adjustments to ensure efficiency and stability. The electricity generated by the hybrid tree can be used locally to power nearby structures or devices. Additionally, it can be integrated into the broader electrical grid to contribute renewable energy to the larger community (Karlekar et al., 2020).
The components of the hybrid tree are as follows:
Solar Panels (Photovoltaic Cells): Solar panels capture sunlight and convert it into electrical energy through photovoltaic cells. These panels generate electricity during sunny hours by harnessing the sun’s energy.
Wind Turbines (Aeroleaves): The “leaves” of the hybrid tree act as wind turbines. These Aeroleaves are designed based on the Savonius-type wind turbine. Unlike traditional wind turbines, they can operate regardless of wind direction and at low wind speeds (as low as 3 m/s).
DC Generators: Instead of using AC generators, wind trees utilize DC generators. This choice avoids the need for rectification circuits and simplifies the system design.
Power Converters: Power converters ensure a constant DC voltage even if the generated voltage varies due to wind speed fluctuations. These converters connect the terminals of all generators in series, adding up the generated voltage.
Battery Panel: Wiring runs through the branches of the wind tree and leads to a battery panel at the bottom. Excess energy generated by solar panels and wind turbines is stored in batteries. The stored energy can be used during low sunlight or calm wind conditions, ensuring a consistent power supply.
4.4 SWOT analysis of solar wind tree
SWOT analysis for solar and wind hybrid trees is essential for informed decision-making, risk management, and sustainable energy transition. Table 4 depicts the detailed SWOT analysis of the hybrid tree.
5 Comparative analysis of solar, wind, and hybrid tree
5.1 Performance analysis
5.1.1 Performance of solar modules
A thorough performance assessment was undertaken on a solar module during a day characterized by clear, sunny weather conditions. Measurements were meticulously recorded at various intervals throughout the day to comprehensively analyze its power generation profile. The findings, delineated in Figure 5, elucidate a conspicuous pattern: power output exhibits a discernible ascent starting from 10:00 in the morning, peaking at a noteworthy 31 W precisely at 14:00, before gradually diminishing thereafter. This pattern signifies a pronounced diurnal variation in power generation, with the solar module exhibiting its highest efficiency during midday hours. The graphical representation accompanying the findings vividly illustrates the fluctuations in power production over the day, providing further insight into the module’s performance dynamics (Reddy et al., 2022). The significant factor influencing the performance of modules is incoming solar radiation. The rise in radiation leads to a rise in the power generated from the module.
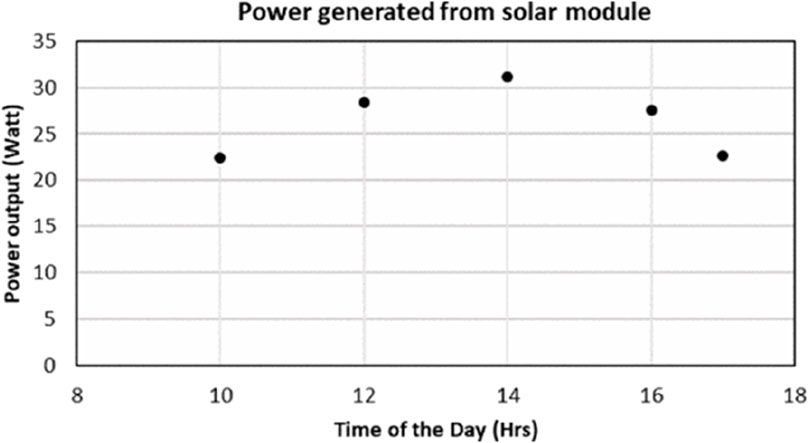
Figure 5. Power generated by solar module (Reddy et al., 2022).
5.1.2 Performance of wind turbine
In examining wind turbine performance, a pedestal fan simulates various wind velocities. An anemometer is employed to gauge wind velocity accurately. The wind turbine’s electrical output is linked to a 12 V battery circuit via a bridge rectifier. Both voltage and current are meticulously measured using a multimeter. The findings from these performance evaluations are tabulated in Table 5. Notably, it is observed that as wind velocity escalates, the power output of the turbine also rises. The turbine commences power generation at a wind velocity of 3.2 m/s. Subsequently, at 8.2 m/s wind velocity, it can supply 24.52 W of power to the connected battery bank (Reddy et al., 2022).
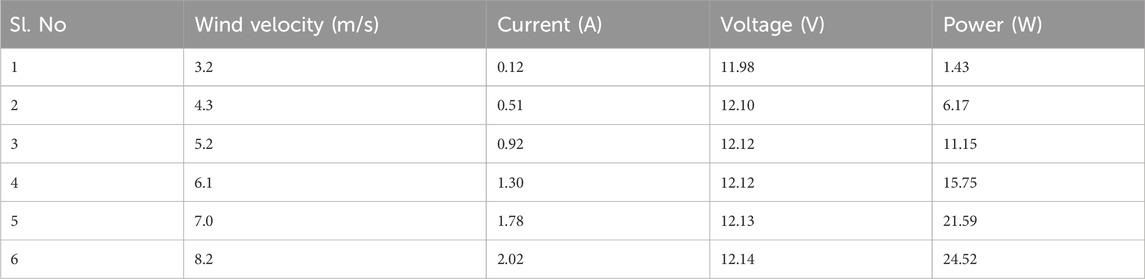
Table 5. Experimental results of a wind turbine (Reddy et al., 2022).
5.1.3 Performance of wind-solar tree
Key performance indicators are revealed by human monitoring of the wind-solar tree’s operational effectiveness. All wind turbines are seen to turn on when wind speeds approach 2.8 m per second (m/s). However, electricity is not delivered to the battery until winds exceed 3.2 m/s. When the wind speed is 5 m/s, power output peaks at 35 W (W), while wind turbines generate 75 W at 8.2 m/s. A steady and steady wind flow is necessary for the production of electricity. Solar modules supplement wind energy in terms of total power production. These modules have a 160 W yield, with a specific output of 126 W recorded at 14:00 h on clear, bright days. The most power output ever measured while solar panels and wind turbines run simultaneously is 210 W. A battery bank with a capacity of 1 kW-hour (kWh) holds the produced power. This stored energy is used for four to 5 hours a day to power a 240 W load, which includes tube lights and ceiling fans, through the use of an inverter (Reddy et al., 2022).
5.2 Design/technical factors
The key differences lie in the primary source of energy capture and the integration of solar panels, wind turbines, or both. Solar trees focus on solar energy, wind trees exclusively on wind energy, while hybrid trees combine both sources for enhanced sustainability and reliability. Design and technical considerations are tailored to optimize the specific energy capture mechanisms employed in each tree type, as discussed in Table 6.
5.3 Economical/cost factors
Economic analysis involves evaluating the costs and benefits of a project or initiative. In the context of renewable energy sources like solar, wind, and hybrid trees (assuming these are innovative technologies combining multiple forms of renewable energy generation), economic analysis would assess factors such as initial investment costs, operational expenses, potential savings in energy production, and overall return on investment (ROI). It also considers externalities such as environmental impacts and societal benefits. The economic factors are discussed below:
5.3.1 Solar tree
⁃ System Size: Larger solar trees with more panels will cost more.
⁃ Customization: Additional features like seating, tables, or branding customization can affect the cost.
⁃ Installation: Labor and installation costs contribute to the overall expense (Singh et al., 2019).
⁃ Currently, a 1.8 kW solar tree can cost around $40,000. Larger systems (e.g., 5.4 kW) may reach up to $80,000, and extensive systems (e.g., 16.5 kW) can exceed $100,000 (Syafriyudin, 2018).
5.3.2 Wind tree
⁃ Wind trees are more expensive due to their unique design and limited efficiency.
⁃ A 5.4 kW wind tree can cost approximately $56,000 or roughly $10,370 per kilowatt capacity.
⁃ Compared to utility-scale wind turbines, wind trees are 6× more expensive per kilowatt of capacity (Kumar et al., 2020).
5.3.3 Hybrid tree
⁃ System Size: Larger hybrid trees will cost more.
⁃ Customization: Additional features, aesthetics, and branding options affect the price.
⁃ Installation: Labor and installation expenses contribute to the overall cost.
⁃ Hybrid trees aim to optimize solar and wind energy, but they may still be more expensive than standalone solar or wind systems (Madhavan and Subash, 2021).
5.4 Environmental and social impact
These tree-like structures contribute to sustainable energy, but their impact depends on factors like manufacturing, installation, and maintenance practices. The various environmental and social impacts of different trees are discussed in Table 7. As technology advances, minimizing adverse effects becomes crucial for a greener future.
5.5 Circular economic, sustainability, and recycling aspects
The circular economy aims to minimize waste and make the most of resources by closing the loop of product lifecycle through reuse, repair, remanufacturing, and recycling. Applied to renewable energy technologies like solar, wind, and hybrid trees, this concept would focus on designing systems that use materials efficiently, reduce waste generation during production and operation, and ensure that components are recycled or repurposed at the end of their lifecycle. Sustainability refers to meeting the needs of the present without compromising the ability of future generations to meet their own needs. In the context of energy generation from solar, wind, and hybrid trees, sustainability involves using renewable resources that are abundant, clean, and have minimal environmental impact compared to traditional fossil fuels. It also encompasses factors like energy efficiency, carbon footprint reduction, and long-term environmental stewardship. Recycling in the context of renewable energy technologies involves recovering valuable materials such as metals (e.g., aluminum, copper), silicon (from solar panels), and composite materials (from wind turbine blades or hybrid tree components) at the end of their operational life. Proper recycling ensures that these materials can be reused in new products or technologies, reducing the demand for virgin resources and minimizing waste. It also addresses environmental concerns associated with the disposal of complex electronic and mechanical components.
In all cases, sustainability considerations involve minimizing environmental impact, optimizing energy production efficiency, and adopting practices that support a circular economy (Hyder et al., 2018c). Recycling efforts play a crucial role in managing the end-of-life components of solar, wind, and hybrid trees, ensuring responsible waste management and the reuse of valuable materials in the production cycle. Balancing their environmental impact, circular practices, and recycling efforts will be crucial in achieving sustainable energy transitions (Kumar et al., 2022b).
5.5.1 Solar trees
Circular economy:
• Solar trees can contribute to the circular economy by extending the lifespan of solar panels. When these panels end their useful life, recycling them efficiently ensures that valuable materials (such as silicon, glass, and metals) are recovered and reused in new panels (Sim et al., 2020).
• Implementing circular design principles during solar tree manufacturing can minimize waste and promote resource efficiency.
Sustainability:
• Solar trees harness sunlight to generate clean energy, reducing dependence on fossil fuels.
• Properly designed solar trees can blend seamlessly into urban environments, promoting sustainable aesthetics (Li et al., 2021).
Recycling:
• Recycling solar panels involves separating components like glass, aluminum, and silicon. Efforts are ongoing to improve recycling technologies and recover valuable materials.
• Collaborations between manufacturers, recyclers, and policymakers are essential to establish efficient recycling systems for solar panels (Deep et al., 2020).
5.5.2 Wind trees
Circular economy:
• Wind trees can contribute to the circular economy by optimizing the use of materials. Their compact design minimizes the need for large quantities of steel and concrete, which is typical in traditional wind turbines (Dutta et al., 2020).
• Recycling wind tree components (such as blades and support structures) is crucial to reduce waste.
Sustainability:
• Wind trees offer decentralized energy production, reducing transmission losses and promoting local resilience.
• Their aesthetic appeal encourages public acceptance of renewable energy (Keshtegar et al., 2018).
Recycling:
• Wind turbine blades are challenging to recycle due to their composite materials (often fiberglass or carbon fiber). Research focuses on finding sustainable solutions for blade disposal.
• Innovations like repurposing blades for construction materials or converting them into biofuel are being explored (Fraisse et al., 2020).
5.5.3 Hybrid trees
Circular economy:
• Hybrid trees combine solar and wind energy, maximizing resource utilization. Circular design principles can enhance their efficiency (Janapati et al., 2020).
• Integrating recyclable materials in hybrid tree construction ensures a sustainable lifecycle.
Sustainability:
• Hybrid trees provide continuous energy output by leveraging solar and wind resources (Oluwafemi et al., 2019).
• Their versatility makes them suitable for various urban and rural settings.
Recycling:
• Recycling considerations for hybrid trees involve both solar panels and wind turbine components.
• Collaboration among stakeholders (manufacturers, researchers, and policymakers) is essential to develop effective recycling pathways for hybrid systems (Bantikatla et al., 2019).
6 Challenges and prospects
6.1 Solar technology
6.1.1 Challenges
Solar technology encounters several challenges that impact its widespread adoption. Firstly, its intermittency is a significant hurdle as solar energy generation is contingent upon sunlight availability, which varies throughout the day and is absent at night. Moreover, the extensive space requirements of large-scale solar farms pose limitations, particularly in densely populated areas where land availability is scarce. Additionally, solar energy production is vulnerable to weather conditions such as cloud cover and adverse weather, which can diminish output. Lastly, effective energy storage solutions are crucial to mitigate the variability of solar energy, requiring advancements in storage technologies to store surplus energy for use during periods of low sunlight.
6.1.2 Prospects
Despite these challenges, solar technology exhibits promising prospects. The steady decline in solar panel costs enhances its competitiveness against conventional energy sources. Solar technology's scalability allows for deployment in diverse settings including rooftops, urban areas, and remote locations, thereby increasing its accessibility. Environmentally, solar energy is a clean and renewable resource, contributing significantly to reductions in greenhouse gas emissions and air pollution. Ongoing research and development efforts continue to improve the efficiency and durability of solar cells, bolstering the viability and attractiveness of solar power as a sustainable energy solution.
6.2. Wind technology
6.2.1 Challenges
Wind technology confronts several challenges that affect its integration and acceptance. Foremost among these challenges is the variability of wind speed and direction, which leads to fluctuations in power output and poses challenges to grid stability. Additionally, wind turbines can be visually intrusive and generate noise, which can provoke opposition from local communities. Finding suitable sites with consistent wind patterns and minimal environmental impact remains a significant challenge. Moreover, integrating wind power into existing electrical grids necessitates substantial investments in infrastructure and upgrades to ensure seamless grid compatibility.
6.2.2 Prospects
Despite these challenges, wind technology offers compelling prospects. Offshore wind farms, in particular, present substantial untapped potential for consistent and high-capacity electricity generation. Advances in turbine technology and economies of scale have driven down the cost of wind energy, making it increasingly competitive with traditional energy sources. Furthermore, wind energy projects create employment opportunities across manufacturing, installation, maintenance, and associated sectors, thereby stimulating economic growth. By reducing reliance on fossil fuels, wind energy enhances energy security and diversifies the energy mix, contributing to sustainable development goals.
6.3 Hybrid (wind and solar) technology
6.3.1 Challenges
Hybrid systems that combine wind and solar technologies face distinct challenges. Integrating these two renewable sources necessitates sophisticated control systems to manage their variability effectively. Aligning the complementary generation profiles of wind and solar to maximize overall output presents a technical challenge. Additionally, building hybrid systems may require additional investments in infrastructure and transmission lines to connect to the grid. Operational complexities, including maintenance and troubleshooting, further underscore the challenges of hybrid system deployment.
6.3.2 Prospects
Hybrid technology holds promising prospects for enhancing renewable energy reliability and efficiency. By integrating wind and solar resources, hybrid systems can offer greater stability and reliability by leveraging the intermittent generation profiles of both sources. They have the potential to maximize energy output across a broader range of environmental conditions, thereby enhancing overall efficiency. Moreover, hybrid systems contribute to grid flexibility by smoothing out fluctuations in energy production, which is critical for grid stability. Furthermore, co-locating wind and solar generation facilities optimizes land use efficiency and minimizes environmental impact, underscoring their potential as sustainable energy solutions.
In conclusion, while each renewable energy technology faces unique challenges, they also present significant prospects for advancing clean energy generation. Hybrid systems, in particular, represent an innovative approach to overcoming individual technology limitations and maximizing their combined benefits for a sustainable energy future.
7 Conclusion
In conclusion, the prospects of solar and wind tree technologies appear highly promising, marking a significant stride towards sustainable energy solutions. Propelled by continuous technological advancements and collaborative research endeavors, these innovations offer pathways to enhance energy efficiency and confront inherent challenges in energy generation. Further refinement and expansion through innovative technological approaches, coupled with a steadfast commitment to the principles of the circular economy, promise considerable potential.
The advent of hybrid solar and wind tree systems represents a pivotal advancement, effectively addressing the intermittency issues often associated with standalone systems and presenting a holistic solution for uninterrupted power supply. Positioned at the intersection of engineering ingenuity and environmental stewardship, solar and wind trees emerge as symbols of progress toward greener urban landscapes. As ongoing research refines these pioneering technologies, their integration into energy infrastructure holds promise for cultivating more sustainable and resilient cities, thus contributing significantly to the global transition to a low-carbon future.
A SWOT analysis underscores pertinent considerations surrounding solar and wind tree technologies. Strengths include their ability to provide tailored renewable energy solutions for urban settings, optimizing space utilization, and reducing dependence on conventional energy sources. Additionally, their aesthetically pleasing designs serve as catalysts for promoting environmental consciousness in urban environments.
Nonetheless, challenges persist, including performance variability, constraints related to land use, and substantial initial investment requirements. Concerns also linger regarding the reliability of renewable energy sources compared to traditional alternatives. Despite these challenges, opportunities abound, driven by ongoing advancements in energy storage capabilities and efficiency. Integration with smart grid systems and supportive regulatory frameworks can further bolster accessibility and feasibility.
However, the widespread adoption of solar and wind trees faces threats from entrenched interests in conventional energy sources, regulatory complexities, and economic considerations. It is essential to carefully navigate environmental impacts, land use dynamics, and aesthetic considerations to ensure successful implementation.
In summary, solar and wind tree technologies hold tremendous promise for advancing sustainability and resilience. Their trajectory towards integration into global energy frameworks underscores their transformative potential in shaping a more sustainable energy landscape. Through concerted efforts to address existing challenges and leverage inherent advantages, solar and wind trees can play a pivotal role in guiding the world toward a more sustainable energy future.
7.1 Recommendation and future scope
Integrating solar, wind, and solar and wind hybrid trees holds significant promise for advancing sustainable energy solutions in urban and residential environments. These innovative structures contribute to renewable energy generation and offer aesthetic and functional benefits. For widespread adoption, it is recommended to:
• Increase public awareness regarding the benefits of solar trees, wind trees, and hybrid trees through educational campaigns and community outreach.
• Showcase successful installations and emphasize the positive impact on energy sustainability.
• Encourage government and private sector incentives to promote the installation of these structures, fostering widespread adoption.
• Offer financial incentives, tax credits, or subsidies to make solar and wind technologies more accessible.
• Invest in research and development to enhance the efficiency of solar panels, wind turbines, and hybrid systems.
• Explore advancements in energy storage technologies to address intermittent energy production.
• Establish clear and supportive regulatory frameworks that facilitate the integration of solar and wind technologies into urban planning and development.
• Streamline permitting processes to encourage the installation of these structures in both residential and commercial spaces.
The future scope of solar trees, wind trees, and solar and wind hybrid trees is promising, with potential developments in several key areas:
• Implement intelligent technologies for real-time monitoring and control of solar and wind systems, optimizing energy production and storage.
• Explore advanced energy storage solutions, such as high-capacity batteries or other emerging technologies, to address the intermittent nature of renewable energy sources.
• Collaborate with urban planners to seamlessly integrate these structures into the fabric of cities, creating energy-efficient and visually appealing urban landscapes.
• Optimize hybrid systems by refining the balance between solar and wind components to maximize energy production and system reliability.
• Enhance circular economy practices by focusing on the recyclability of materials used in these structures, promoting responsible end-of-life disposal and recycling processes.
• Foster community engagement in the design and implementation of solar trees, wind trees, and hybrid trees, ensuring that local needs and preferences are considered.
By implementing these recommendations and exploring the future scope, solar, wind, and hybrid trees can be pivotal in reshaping the urban energy landscape, fostering sustainability, and contributing to a cleaner, more resilient energy future.
Author contributions
KM: Data curation, Writing–original draft, Writing–review and editing. MS: Conceptualization, Funding acquisition, Supervision, Writing–review and editing. AP: Conceptualization, Data curation, Writing–review and editing. MN: Conceptualization, Methodology, Writing–review and editing. KK: Methodology, Validation, Writing–review and editing.
Funding
The author(s) declare that financial support was received for the research, authorship, and/or publication of this article. The authors would like to thank the Universiti Malaysia Pahang Al-Sultan Abdullah for the financial support under Internal Research Grant RDU233002 and the laboratory facilities provided.
Conflict of interest
The authors declare that the research was conducted in the absence of any commercial or financial relationships that could be construed as a potential conflict of interest.
The author(s) declared that they were an editorial board member of Frontiers, at the time of submission. This had no impact on the peer review process and the final decision.
Publisher’s note
All claims expressed in this article are solely those of the authors and do not necessarily represent those of their affiliated organizations, or those of the publisher, the editors and the reviewers. Any product that may be evaluated in this article, or claim that may be made by its manufacturer, is not guaranteed or endorsed by the publisher.
References
Ahmed, F. A. G., and Mohamed, E. L.-S. (2021). Shalaby in solar-tree model based on fibonacci-sequence for better awareness of solar technology in urban environment.
Almadhhachi, M., Seres, I., and Farkas, I. (2022). Significance of solar trees: configuration, operation, types and technology commercialization. Energy Rep. 8, 6729–6743. doi:10.1016/j.egyr.2022.05.015
Arunachalam, V. S., and Fleischer, E. L. (2008). The global energy landscape and materials innovation. MRS Bull. 33, 264–288. doi:10.1557/mrs2008.61
Awaze, S. S., Bhamburkar, K. N., Babare, A. P., Asode, A. R., and Bargat, S. P. (2018). In design and fabrication of solar tree.
Balaji, J., and Rao, P. N. (2020). Solar tree with different installation positions of photovoltaic module-Part 1. J. Green Eng. (JGE). 10 (6).
Bantikatla, H., Nulu, L. D. S. M. P., Bhogoju, R., Narlanka, P., Siddi, V. R., Royal, K. K., et al. (2019). In Design and fabrication of hybrid solar silicon PV system. Elsevier Ltd.
Batiles, JABR, and Culaba, A. B. (2019). “In Bamboo-based Solar PV Tree System under improved solar power economic conditions,” in 2019 IEEE 11th International Conference on Humanoid, Nanotechnology, Information Technology, Communication and Control, Environment, and Management (HNICEM), Laoag, Philippines, 29 November 2019 - 01 December 2019.
Bista, S., K, S. C., and Kasaudhan, D. (2018). Design and fabrication of solar tree. Int. J. Latest Eng. Res. Appl. (IJLERA) 22 (03).
Chaithanya, S., Reddy, V. N. B., and Kiranmayi, R. (2019). Modeling and analysis of grid-tied PMA based offshore wind energy system using PSCAD/EMTDC. Ain Shams Eng. J. 10, 411–417. doi:10.1016/j.asej.2019.01.007
Choifin, M., Fathoni Rodli, A., Kartika Sari, A., Tri Wahjoedi, S., Aziz, A., Fathoni, A., et al. (2021). Study of renewable energy and solar panel literature through bibliometric positioning during literature through bibliometric positioning during three decades three decades.
Cuce, P. M., Saxena, A., Cuce, E., and Riffat, S. (2022). Applications of solar PV tree systems with different design aspects and performance assessment. Int. J. Low-Carbon Technol. 17, 266–278. doi:10.1093/ijlct/ctac004
Debadatta Behera, D., Mohanty, A. M., Mohanty, R. C., Mohanty, A., and Mohanty, R. (2020). Modelling and simulation of solar tree for domestic lightning purpose A project done by a pg student view project A project done by a Ph.D research scholar view project modelling and simulation of solar tree for domestic lightning purpose. Indian J. Nat. Sci. 10.
Deep, R., Mishra, A., and Agarwal, A. (2020). Comparative analysis of solar panel output power: matrix vs tree form. MATEC Web Conf. 307, 01002. doi:10.1051/matecconf/202030701002
Dey, S., Lakshmanan, M. K., and Pesala, B. (2018). Optimal solar tree design for increased flexibility in seasonal energy extraction. Renew. Energy 125, 1038–1048. doi:10.1016/j.renene.2018.02.017
Dey, S., and Pesala, B. (2020). Solar tree design framework for maximized power generation with minimized structural cost. Renew. Energy 162, 1747–1762. doi:10.1016/j.renene.2020.07.035
Dutta, H., Sen, B., Bisharad, D., and Seban, L. (2020). Optimal selection of solar tracking system in India. A Rev.
Elnozahy, A., Rahman, A. K. A., Ali, A. H. H., Abdel-Salam, M., and Ookawara, S. (2015). Performance of a PV module integrated with standalone building in hot arid areas as enhanced by surface cooling and cleaning. Energy Build. 88, 100–109. doi:10.1016/j.enbuild.2014.12.012
Fraisse, G., Merlin, G., Pailha, M., Cloet, D., and Bernard, L. (2020). New concept of 3D bio-inspired solar thermal collector. Sol. Energy 195, 329–339. doi:10.1016/j.solener.2019.11.055
Gangwar, P., Kumar, N. M., Singh, A. K., Jayakumar, A., and Mathew, M. (2019a). Solar photovoltaic tree and its end-of-life management using thermal and chemical treatments for material recovery. Case Stud. Therm. Eng. 14, 100474. doi:10.1016/j.csite.2019.100474
Gangwar, P., Singh, R., Tripathi, R. P., and Singh, A. K. (2019b). Effective solar power harnessing using a few novel solar tree designs and their performance assessment. Energy Sources, Part A Recovery, Util. Environ. Eff. 41, 1828–1837. doi:10.1080/15567036.2018.1549162
Gürel, A. E., Ağbulut, Ü., Bakır, H., Ergün, A., and Yıldız, G. (2023). A state of art review on estimation of solar radiation with various models. Heliyon 9, e13167. doi:10.1016/j.heliyon.2023.e13167
Hyder, F., Baredar, P., and Sudhakar, K. (2018c). In A novel Sun tracking technique through a Solar PV Tree and a smart controller. Chennai, India: Institute of Electrical and Electronics Engineers Inc.
Hyder, F., Baredar, P., Sudhakar, K., and Mamat, R. (2018b). Performance and land footprint analysis of a solar photovoltaic tree. J. Clean. Prod. 187, 432–448. doi:10.1016/j.jclepro.2018.03.249
Hyder, F., Sudhakar, K., and Mamat, R. (2018a). Solar PV tree design: a review. Renew. Sustain. Energy Rev. 82, 1079–1096. doi:10.1016/j.rser.2017.09.025
Janamala, V. (2021). Solar pv tree: shade-free design and cost analysis considering Indian scenario. Walailak J. Sci. Technol. 18. doi:10.48048/wjst.2021.8995
Janapati, B., Pulivarthi, N. R., Balaji, J., Rao, P. N., and Rao, S. S. (2020). Solar tree with different installation positions of photovoltaic module-Part 2. J. Green Eng. 10 (9).
Karlekar, G. S., Professor, A., Sheikh, A., Wasekar, A., and Student, S. R. (2020). A review paper on solar power tree. Int. J. Eng. Appl. Sci. Technol. 4, 106–108. doi:10.33564/ijeast.2020.v04i10.020
Kaur, G., and Singh, M. (2021). Analysis of various optimization techniques in machine learning. Turkish J. Comput. Math. Educ. 12 (01), 472–482. doi:10.17762/turcomat.v12i2.855
Keshtegar, B., Mert, C., and Kisi, O. (2018). Comparison of four heuristic regression techniques in solar radiation modeling: kriging method vs RSM, MARS and M5 model tree. Renew. Sustain. Energy Rev. 81, 330–341. doi:10.1016/j.rser.2017.07.054
Kumar, N. M., Chopra, S. S., Malvoni, M., Elavarasan, R. M., and Das, N. (2020). Solar cell technology selection for a pv leaf based on energy and sustainability indicators-a case of a multilayered solar photovoltaic tree. Energies 13, 6439. doi:10.3390/en13236439
Kumar, R., Bansal, K., Kumar, A., Yadav, J., Gupta, M. K., and Singh, V. K. (2022a). Renewable energy adoption: design, development, and assessment of solar tree for the mountainous region. Int. J. Energy Res. 46, 743–759. doi:10.1002/er.7197
Kumar, R., Kumar, A., Gupta, M. K., Yadav, J., and Jain, A. (2022b). Solar tree-based water pumping for assured irrigation in sustainable Indian agriculture environment. Sustain. Prod. Consum. 33, 15–27. doi:10.1016/j.spc.2022.06.013
Li, Y., Fan, J., Wang, R., Shou, W., Wang, L., and Liu, Y. (2021). 3D tree-shaped hierarchical flax fabric for highly efficient solar steam generation. J. Mater. Chem. A 9, 2248–2258. doi:10.1039/d0ta09570b
Liu, J., Chen, X., Cao, S., and Yang, H. (2019). Overview on hybrid solar photovoltaic-electrical energy storage technologies for power supply to buildings. Energy Convers. Manag. 187, 103–121. doi:10.1016/j.enconman.2019.02.080
Madhavan, N., and Subash, T. D. (2021). Advanced solar tree with sun tracking and cleaning technolgy. Mater. Today Proc.
Mi, B., Finnerty, C., and Conway, K. (2019). Prospects of artificial tree for solar desalination. Curr. Opin. Chem. Eng. 25, 18–25. doi:10.1016/j.coche.2019.06.004
Mishra, S. P., Giri, N. C., Behera, D. D., and Nayak, S. R. (2020). Solar trees: shift from grey to green sky for future fuel pumps under clean/green energy: India. Int. J. Environ. Clim. Change, 68–86. doi:10.9734/ijecc/2020/v10i1130267
Oluwafemi, I., Laseinde, T., and Salau, A. O. (2019). Design and construction of a 0.5 kW solar tree for powering farm settlements. Int. J. Mech. Eng. Technol. (IJMET) 10, 19–33.
Patil, D. M., and Madiwal, S. R. (2016). Design and development of solar tree for domestic applications. Int. J. Eng. Sci. and Res. Technol. 5. doi:10.5281/zenodo.59963
Patil, R. K. (2018). Solar tree-working principle, arrangements-A study. Int. J. Creative Res. Thoughts 6, 291.
Pérez-Lombard, L., Ortiz, J., and Pout, C. (2008). A review on buildings energy consumption information. Energy Build. 40, 394–398. doi:10.1016/j.enbuild.2007.03.007
Pfaffel, S., Faulstich, S., and Rohrig, K. (2017). Performance and reliability of wind turbines: a review. Energies 10 (11), 1904. doi:10.3390/en10111904
Rajamony, R. K., Kalidasan, B., Lagari, I. A., Paw, J. K. S., Sofiah, A. G. N., Suraparaju, S. K., et al. (2024). Progress in research and technological developments of phase change materials integrated photovoltaic thermal systems: the allied problems and their mitigation strategies. Sustain. Mater. Technol. 40, e00921. doi:10.1016/j.susmat.2024.e00921
Reddy, A. S., Prasad, M., Bagalkot, H., Naik, M., and Bhat, V. (2022). In design and development of wind-solar tree: a hybrid renewable energy system for domestic applications. Vijayapur, India: Institute of Electrical and Electronics Engineers Inc.
Rekioua, D., Rekioua, T., Elsanabary, A., and Mekhilef, S. (2023). Power management control of an autonomous photovoltaic/wind turbine/battery system. Energies 16, 2286. doi:10.3390/en16052286
Sachin, M., Kadam, S., Patil, M. B., Pandurang, D., and Kirdat, A. (2023). Solar power tree-A effective energy source. Int. J. Res. Publ. Rev. 4, 253–257.
Shaikh, M. R. S. (2017). A review paper on electricity generation from solar energy. Int. J. Res. Appl. Sci. Eng. Technol. V, 1884–1889. doi:10.22214/ijraset.2017.9272
Sikka, R. (2019). Design and implementation of solar tree. Int. J. Innovative Technol. Explor. Eng. 8, 180–182.
Sim, Y. H., Yun, M. J., Cha, S. I., and Lee, D. Y. (2020). Fractal solar cell array for enhanced energy production: applying rules underlying tree shape to photovoltaics. Proc. R. Soc. A Math. Phys. Eng. Sci. 476, 20200094. doi:10.1098/rspa.2020.0094
Singh, B. P., Goyal, S. K., and Kumar, P. (2021). In Solar pv cell materials and technologies: analyzing the recent developments. Elsevier Ltd.
Singh, R., Rawat, N., and Srivastava, R. (2019). Performance evaluation of a solar tree design and a fixed solar panel for effective solar power harnessing. Int. J. Appl. Eng. Res. 14 (11).
Solomin, E., Sirotkin, E., Cuce, E., Selvanathan, S. P., and Kumarasamy, S. (2021). Hybrid floating solar plant designs: a review. Energies 14 (10), 2751. doi:10.3390/en14102751
Suliński, J., Sypka, P., and Starzak, R. (2021). Methodology based on volumetric biomass density to estimate solar radiation transmission through tree stands. For. Ecol. Manag. 501, 119701. doi:10.1016/j.foreco.2021.119701
Thakur, A. K., Singh, R., Gehlot, A., Kaviti, A. K., Aseer, R., Suraparaju, S. K., et al. (2022). Advancements in solar technologies for sustainable development of agricultural sector in India: a comprehensive review on challenges and opportunities. Environ. Sci. Pollut. Res. 29, 43607–43634. doi:10.1007/s11356-022-20133-0
Tomczak, S. K., Skowronska-Szmer, A., and Szczygielski, J. J. (2020). Is investing in companies manufacturing solar components a lucrative business? A decision tree based analysis. Energies 13, 499. doi:10.3390/en13020499
Torres-Barrán, A., Alonso, Á., and Dorronsoro, J. R. (2019). Regression tree ensembles for wind energy and solar radiation prediction. Neurocomputing 326–327, 151–160. doi:10.1016/j.neucom.2017.05.104
Vyas, M., Chowdhury, S., Verma, A., and Jain, V. K. (2022). Solar Photovoltaic Tree: urban PV power plants to increase power to land occupancy ratio. Renew. Energy 190, 283–293. doi:10.1016/j.renene.2022.03.129
Keywords: SWOT analyses, renewable energy, solar tree, wind tree, sustainability
Citation: Mohanaravi K, Samykano M, Pandey AK, Noor MM and Kadirgama K (2024) A Succinct review of strengths, weaknesses, opportunities, and threats (SWOT) analyses, challenges and prospects of solar and wind tree technologies for hybrid power generation. Front. Energy Res. 12:1417511. doi: 10.3389/fenrg.2024.1417511
Received: 15 April 2024; Accepted: 24 June 2024;
Published: 23 August 2024.
Edited by:
Nallapaneni Manoj Kumar, HICCER – Hariterde International Council of Circular Economy Research, IndiaReviewed by:
Mobi Mathew, Rajiv Gandhi Institute of Petroleum Technology, IndiaAfaf Zaza, Green Energy Park, Morocco
Arjun Singh Kopalakrishnaswami, Vellore Institute Of Technology, India
Copyright © 2024 Mohanaravi, Samykano, Pandey, Noor and Kadirgama. This is an open-access article distributed under the terms of the Creative Commons Attribution License (CC BY). The use, distribution or reproduction in other forums is permitted, provided the original author(s) and the copyright owner(s) are credited and that the original publication in this journal is cited, in accordance with accepted academic practice. No use, distribution or reproduction is permitted which does not comply with these terms.
*Correspondence: Mahendran Samykano, bWFoZW5kcmFuQHVtcHNhLmVkdS5teQ==