- 1State Key Laboratory of Soil and Sustainable Agriculture, Institute of Soil Science, Chinese Academy of Sciences, Nanjing, China
- 2University of the Chinese Academy of Sciences, Beijing, China
- 3School of Economic Geography, Hunan University of Finance and Economics, Changsha, China
The biocontrol efficiency of the antagonist yeast Meyerozyma guilliermondii is significantly reduced under oxidative stress in adverse environments. However, effective strategies to improve M. guilliermondii under such abiotic stress remain limited. As an effective protectant of yeasts, xylitol has significant potential to improve the performance of M. guilliermondii under abiotic stress. We investigated xylitol’s effects on the viability and efficiency of M. guilliermondii under oxidative stress. The results showed that 0.5 M and 1 M xylitol significantly enhanced yeast survival, antioxidant gene expression, and enzyme activity, including thioredoxin reductase (TrxR) and peroxidase (POD), while reducing intracellular reactive oxygen species levels as well as damage to mitochondrial membranes, and preserving the ATP content. Notably, xylitol-treated (XT) yeast exhibited higher intracellular xylitol levels and improved resistance to oxidative stress compared with the non-xylitol-treated cells. Additionally, XT yeast showed a greater biocontrol efficacy and lower postharvest fungal infection rate by gray mold and blue mold in apples. These results demonstrated that xylitol effectively boosts the resilience and biocontrol efficiency of M. guilliermondii, making it a promising candidate to improve postharvest disease management.
1 Introduction
Postharvest fungal control using biocontrol yeasts offers both practical and economic advantages (Droby et al., 2016; Wisniewski et al., 2016; Kim et al., 2018). Over recent decades, a number of biocontrol yeast strains have been identified as effective antagonists against fungal diseases, such as gray mold (Botrytis cinerea) and blue mold (Penicillium expansum) (Romanazzi et al., 2016; Vilanova et al., 2017). Among the effective biocontrol yeasts, Meyerozyma guilliermondii shows high efficacy against various postharvest fungal pathogens of multiple fruits, including apples, pears, and kiwifruit during storage (Sui and Liu, 2014; Sadeghi et al., 2021).
Despite the demonstrated efficacy of antagonistic yeasts, their efficacy as biocontrol agents can be constrained by environmental factors, such as temperature, oxidative stress, and salinity, thereby limiting their effectiveness (Macarisin et al., 2010; Sui et al., 2015; Spadaro and Droby, 2016). The stress tolerance of antagonistic yeasts is closely linked to their survival and proliferation within host tissues, as well as their biocontrol efficacy against pathogens (Liu et al., 2013). Consequently, identifying effective and cost-efficient protectants is a strategic approach to enhance the biocontrol capacity of these yeasts, offering both efficiency and rapid benefits (Liu et al., 2011; Ming et al., 2020).
Sugars or sugar alcohols have been shown to be effective protectants for biocontrol yeasts (Sui et al., 2012; Ming et al., 2020). Xylitol, a polyol derived from the hydrogenation of xylose, has demonstrated efficacy against ochratoxigenic fungus Aspergillus carbonarius and Listeria monocytogenes (Morón de Salim and Ramírez Mérida, 2013; Espinosa-Salgado et al., 2022). Xylitol’s low cost and widespread use as a food additive, along with its inherent antioxidant properties, make it particularly well-suited for use as a protectant for biocontrol yeasts in both pre-and post-harvest applications. However, there is limited research on the application of these compounds as protectants for antagonistic yeasts under adverse environmental conditions.
Taking into account the above research, the present study was conducted to determine the effects of xylitol on the antioxidant response, stress tolerance, and biocontrol efficacy of the yeast M. guilliermondii. We determined: (1) The cell survival rate of xylitol-treated (XT) cells following exposure to oxidative stress induced by H2O2; (2) the impact of xylitol treatment on the expression of antioxidant genes, including those encoding thioredoxin reductase (TrxR) and peroxidase (POD), as well as their corresponding enzyme activities; (3) the accumulation of intracellular reactive oxygen species (ROS), mitochondrial membrane potential analysis, changes in ATP production and intracellular xylitol levels; and (4) the biocontrol efficacy of XT M. guilliermondii yeast cells against B. cinerea and P. expansum infections in apples.
2 Materials and methods
2.1 Antagonistic yeast
The yeast M. guilliermondii, known for its antagonistic properties, was first isolated from apple surfaces and identified through its general morphology and DNA sequencing of the ribosomal internal transcribed spacer (ITS) region (Leaw et al., 2006). This yeast was cultured in a yeast extract-peptone-dextrose (YPD) medium, which contained 10 g of yeast extract, 20 g of peptone, and 20 g of glucose dissolved in 1 liter of water. To cultivate the yeast, 40 mL of YPD medium was added to a 100 mL Erlenmeyer flask, followed by inoculation with M. guilliermondii. The initial yeast cell concentration was measured at 105 cells/mL using a hemocytometer. The culture was then incubated at 25°C on a rotary shaker at 200 rpm for 16 h.
2.2 Fungal pathogens
The fungal pathogens B. cinerea and P. expansum were isolated from infected apples and pears and identified by DNA sequencing of the ITS region, and maintained on potato dextrose agar (PDA) at 4°C. To reactivate the culture and confirm its pathogenicity, each pathogen was inoculated into a wounded apple, and re-isolated onto PDA after infection was successfully confirmed. Spore suspensions of the fungal pathogens were prepared from 2-week PDA plates incubated at 25°C, with spore concentrations determined using a hemocytometer and adjusted to 104 spores/mL with sterile distilled water before use.
2.3 Test fruit
Apples (Malus × domestica Borkh) were harvested when commercially ripe. Only fruits that were uniform in shape and size, and free from physical damage, were selected for the study. The apples were thoroughly inspected to ensure they were intact and free from decay. To sterilize the fruit surfaces, a 2% (v/v) sodium hypochlorite solution was applied for 2 min, followed by rinsing with tap water and air-drying. The sterilized apples were then used in biocontrol experiments.
2.4 Xylitol treatment of Meyerozyma guilliermondii
In order to investigate the effect of xylitol treatment (XT) on M. guilliermondii, yeast cultures were centrifuged at 8,000 × g for 3 min. The yeast cells were washed three times with sterile distilled water to remove residual growth medium (Liu et al., 2012b). The cleaned cells were then resuspended in 20 mL of fresh YPD medium, and xylitol was added to achieve final concentrations of 0.5 or 1 M. The cultures were incubated on a rotary shaker at 25°C, with shaking at 200 rpm for 3 h. The xylitol concentrations were selected based on preliminary experiments. Briefly, given that xylitol concentrations typically range from 0.3 to 3 M in market food applications, we initially confirmed the efficacy of a lower concentration (1 M) in pilot experiments. To assess the potential effectiveness of even lower concentrations, we halved the treatment concentration to 0.5 M for further analysis. A control group, referred to as the Non-xylitol (NX) group, underwent the same procedure without xylitol addition. After centrifugation and washing, the yeast cells from both XT (0.5 and 1 M) and NX groups were resuspended in water at a concentration of 1 × 107 cells/mL for further analysis.
2.5 Effect of xylitol on the survival rate of Meyerozyma guilliermondii
Cell samples collected at 0, 10, 20, and 30 min after oxidative treatment were cultured on YPDA agar medium (YPD supplemented with 20 g of agar per liter). The medium was incubated at 25°C for 3 days, after which colony-forming units (CFUs) per medium were quantified. Cell viability was expressed as the percentage of CFUs following oxidative treatment, relative to the CFU count of total cells. Each treatment contained three replicates, and the experiment was repeated three times.
2.6 Intracellular ROS, mitochondrial membrane potential, and ATP determination
Intracellular ROS levels in yeast cells were determined using the oxidation-sensitive probe 2′,7′-dichlorodihydrofluorescein diacetate (H2DCFDA; Invitrogen, Eugene, OR, USA), as previously described (Liu et al., 2011). Samples of NX and XT yeast cells were exposed to 30 mM H2O2 for 10, 20, or 30 min, with pre-exposure samples designated as time 0. The yeast cells were washed with phosphate-buffered saline (PBS, pH 7.0) and resuspended in the same buffer with 25 μM H2DCFDA. The suspension was incubated in the dark at 30°C for 1 h, washed twice with PBS, and examined under an FV3000 confocal microscope (Olympus, Tokyo, Japan) using a 480 nm excitation and 520 nm emission filter. ROS-positive cells were quantified by randomly selecting 10 fields per slide (with a minimum of 200 cells per slide), and the percentage of fluorescent cells relative to the total count was determined. Each experiment included three biological replicates and was repeated three times.
Mitochondria damage and ATP levels analysis was according to previous studies (Liu et al., 2012a; Sui and Liu, 2014). Briefly, mitochondrial membrane potential was assessed using the Mitochondrial membrane potential assay kit with JC-1 (Beyotime, China), which contains the cationic dye JC-1 (5,5′,6,6′-tetrachloro-1,1′,3,3′-tetraethyl-imidacarbocyanine iodide). This dye emits red fluorescence in the mitochondria of healthy cells. Upon collapse of the mitochondrial membrane potential, the cationic dye accumulates in the cytoplasm, emitting green fluorescence. Thus, the ratio of red to green fluorescence is higher in healthy cells and lower in damaged cells. In this study, all M. guilliermondii samples were collected and resuspended in the JC-1 reagent at a final concentration of 1 × 106 cells/ml and incubated at 37°C for 15 min. Cells were then centrifuged and resuspended in 1 mL of assay buffer provided by the kit, and the ratio of red (excitation at 550 nm, emission at 600 nm) to green (excitation at 485 nm, emission at 535 nm) fluorescence was immediately measured using a Laser Scanning Confocal Microscopy (Zeiss, Germany). Each treatment contained three replicates, and the experiment was repeated three times.
ATP levels analysis was performed according to Li et al. (2010). Briefly, ATP of approximately 20 mg of fresh weight cultured M. guilliermondii cells that exposed to 30 mM H2O2 for 0, 10, 20, 30, 60, and 120 min were extracted with 50 μL of 2.5% trichloroacetic acid (TCA) and incubated at 4°C for 3 h. After centrifugation at 10,000 × g for 15 min, 10 μL supernatant was diluted with 115 μL ATP-free water and 125 μL ATP-free Tris-acetate buffer (40 mM, pH 8.0). ATP content was quantified using a luciferin/luciferase assay kit (Beyotime, China) according to the manufacturer’s instructions. Luminescence emission was measured using a Laser Scanning Confocal Microscopy (Zeiss, Germany). Each treatment contained three replicates, and the experiment was repeated three times.
2.7 Intracellular xylitol concentration measurement
To analyze the intracellular xylitol levels, yeast cells were disrupted by physical methods. After centrifugation at 8000 rpm at −4°C for 5 min, the yeast cell samples were collected and resuspended in HPLC-grade water. An HPLC system was employed to determine the xylitol concentrations (Agilent Series 1260, Agilent Technologies, Santa Clara, CA, United States). The mobile phase consisted of acetonitrile-water (80:20, v/v) with a flow rate of 1 mL/min. Xylitol was quantified using a standard (Sigma-Aldrich, Shanghai, China) with a linear response range of 0.05–10 mg/mL (Krallish et al., 1997). Xylitol concentrations of yeast cells were expressed as mg/g. Each treatment contained three replicates, and the experiment was repeated three times.
2.8 RNA isolation and reverse transcription–quantitative real-time PCR analysis of gene expression
Total RNA from NX and XT cells was extracted, treated with DNase, and purified using the EasyPure® Plant RNA Kit (TransGen Biotech, Beijing, China). RNA quality was assessed by gel electrophoresis and spectrophotometry (Nanodrop, Thermo Fisher Scientific, Waltham, MA, United States). First-strand cDNA was synthesized using 1 μg of total RNA, employing the TransScript® One-Step gDNA Removal and cDNA Synthesis SuperMix kit (TransGen Biotech). qPCR was performed on a Roche LightCycler® 480 (Roche, Basel, Switzerland) with the following program: 95°C for 5 min; 40 cycles of 95°C for 5 s and 60°C for 20 s; 95°C for 15 s; 60°C for 1 min, followed by a dissociation step at 95°C for 15 s. Gene expression of TrxR and POD was normalized to the reference gene 18S rRNA using the 2−ΔΔCT method (Livak and Schmittgen, 2001). Three independent biological replicates and three technical replicates were used, and the analysis was repeated three times.
2.9 Assay of enzyme activity
To measure antioxidant enzyme activity, XT and NX yeast cells were exposed to 30 mM H2O2 for 10, 20, or 30 min, with samples collected prior to oxidative stress exposure serving as time 0. Enzyme extracts were prepared according to the manufacturer’s instructions. Cells (1 × 108) were frozen in liquid nitrogen, resuspended in chilled potassium phosphate buffer (0.1 M, pH 7.4), and centrifuged at 10,000 × g for 20 min at 4°C. The supernatant was collected for enzyme activity analysis. Activities of TrxR and POD were measured using commercial assay kits (Nanjing Jiancheng Bioengineering Institute, Nanjing, China) and expressed as U/mg protein. Protein content was determined using the Bradford assay, with bovine serum albumin as the standard (Bradford, 1976). One unit of TrxR activity was defined as the amount of enzyme that reduces 1 nmol of 5,5′-dithiobis-(2-nitrobenzoic acid) (DTNB) per minute at 25°C. One unit of POD activity was defined as the amount of enzyme that causes a 0.01 absorbance change per minute at 470 nm due to guaiacol oxidation. Each assay was performed with three biological replicates and repeated three times.
2.10 Biocontrol assay
The biocontrol efficacy was evaluated following the method outlined by Wang et al. (2018), with some adjustments. In brief, three wounds (4 mm deep × 3 mm wide) were created on the equator of each apple using a sterile nail. A 10 μL suspension (1 × 107 cells/mL) of either XT or NX M. guilliermondii yeast cells was applied to each wound. One control group received sterile distilled water, while another control consisted of a 10 μL suspension (1 × 107 cells/mL) of fresh yeast cells, which had not been exposed to sugar or oxidative stresses. The treated apples were divided into two groups. After the fruits were air-dried for 2 h, 10 μL of a B. cinerea or P. expansum suspension (1 × 104 spores/mL) was introduced into each wound of the respective groups. The apples were then placed in covered plastic food trays, each sealed within a polyethylene bag, and stored at 25°C. After 4 days, the disease incidence and lesion diameter on each apple were measured. Incidence referred to the percentage of infected wounds, while lesion diameter was measured only for those wounds that showed infection. Each treatment was repeated three times with three replicates, each containing 10 apples. After 4 days treatments, uniformed apples of each treatment were photographed by a digital camera.
2.11 Data analysis
Statistical analyses were carried out using SPSS V26 (IBM Corp., Armonk, NY, United States). Comparisons between the NX and XT groups were made using Student’s t-test, with a significance level of p < 0.05. The results presented are based on pooled data from three independent experimental replicates, as a one-way analysis of variance (ANOVA) showed no significant effects of the treatments or interactions between variables and experiments.
3 Results
3.1 The effects of xylitol on the survival rate of Meyerozyma guilliermondii under oxidative stress
Exposure to 30 mM H2O2 resulted in a decrease in cell viability as the treatment duration increased from 10 to 30 min (Figure 1). After 10 min of H2O2 exposure, the survival rates across all treatments were comparable, averaging around 86%. For yeast cells suspended in the NX group, survival rates dropped to 69 and 51% after 20 and 30 min of H2O2 exposure, respectively. Interestingly, survival rate of XT cells showed an increasing trend. Yeast cells treated with 1 M xylitol exhibited survival rates of 75 and 78% after 20 and 30 min of H2O2 exposure, respectively. Similarly, cells treated with 0.5 M xylitol exhibited over 65% viability under oxidative stress at both 20 and 30 min.
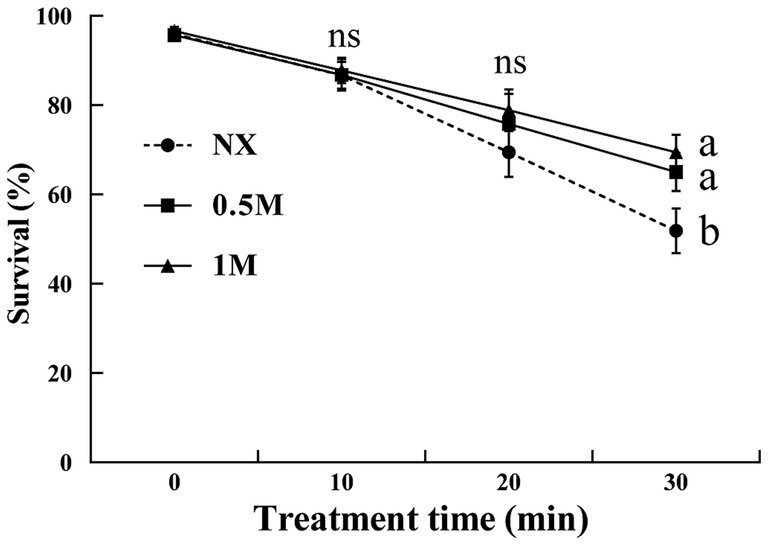
Figure 1. Percent viability of Meyerozyma guilliermondii cells under H2O or xylitol subjected to a subsequent oxidative (30 mM H2O2) stress for 10, 20 or 30 min. Prior to exposure to the subsequent oxidative stress served as Time 0. Data represent the mean ± standard deviation of three independent experiments, where each experiment consisted of three biological replicates (n = 9). Columns with different letters are significantly different according to a Duncan’s multiple range test at p < 0.05. ns, no significance.
3.2 Effect of xylitol on cell damage and ATP levels in Meyerozyma guilliermondii under oxidative stress
At the time 0 (immediately following a 30-min pre-treatment but before exposure to oxidative stress), about 5% of cells treated with 0.5 M and 1 M xylitol, as well as the NX cells, were ROS-positive (Figure 2A). This proportion increased after exposure to 30 mM H2O2. At each time point, the cells treated with 0.5 M and 1 M xylitol showed significantly lower ROS levels compared to the NX cells. To investigate if the increase in ROS under oxidative stress was linked to mitochondrial dysfunction, ΔΨm and ATP content were measured. Figure 2B showed that ΔΨm was significantly changed between NX and XT cells under oxidative stress. And a decreasing trend of ΔΨm was observed in the XT cells compared to the NX group. As higher damage was observed in NX cells, ATP levels, reached their lowest point at 120 min when exposed to 30 mM H2O2 stress. In contrast, the ATP decline in XT cells was notably slower, cells treated with 1 M xylitol maintaining ATP levels approximately 10 times higher than those in the NX group at the 120-min (Figure 2C).
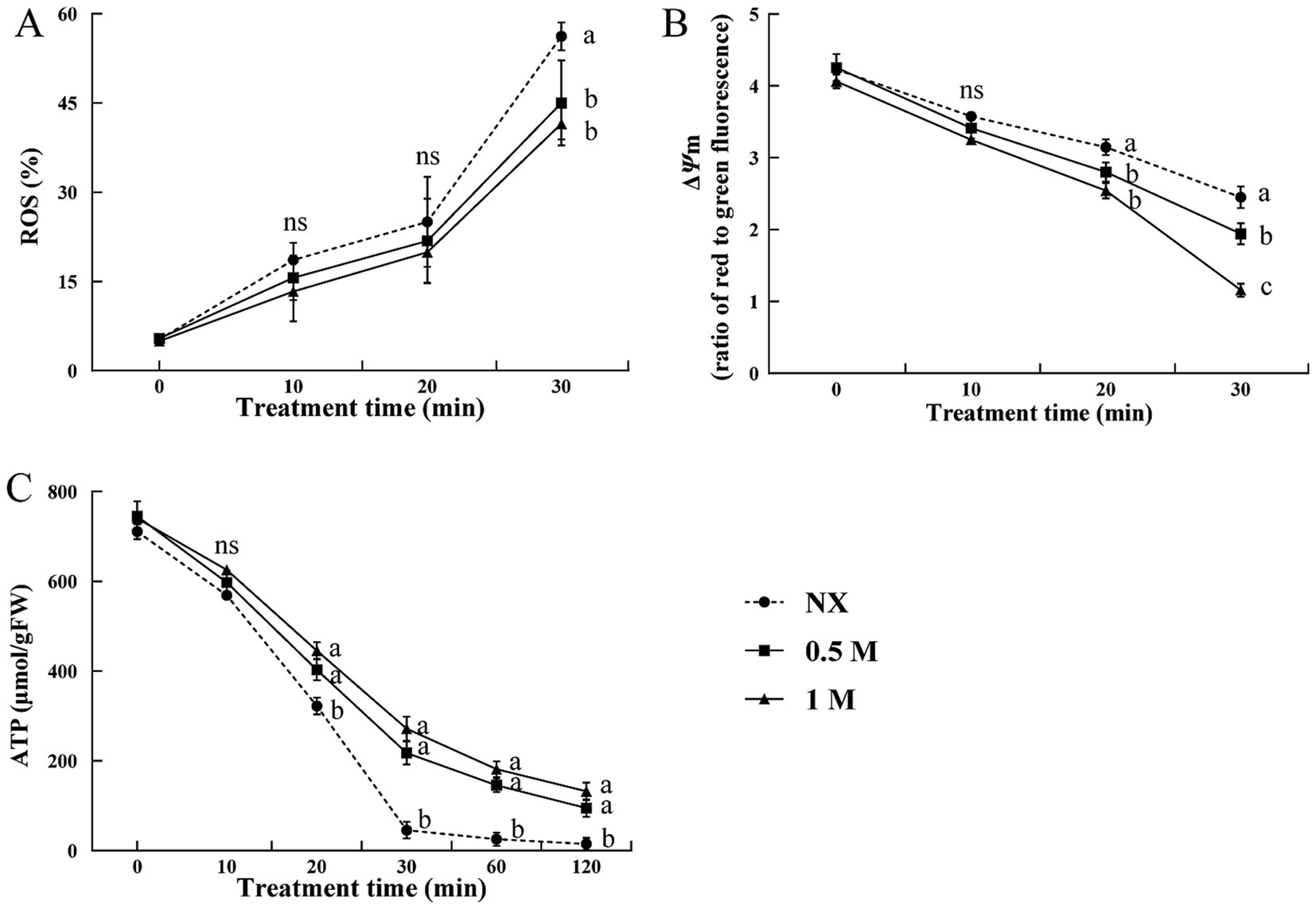
Figure 2. ROS accumulation (A), mitochondrial membrane potential analysis (B) and ATP levels (C) of Meyerozyma guilliermondii cells under H2O or xylitol subjected to a subsequent oxidative (30 mM H2O2) stress for 10, 20 or 30 min, (60 and 120 min for ATP analysis). Prior to exposure to the subsequent oxidative stress served as Time 0. Data represent the mean ± standard deviation of three independent experiments, where each experiment consisted of three biological replicates (n = 9). Columns with different letters are significantly different according to a Duncan’s multiple range test at p < 0.05. ns, no significance.
3.3 Yeast cells uptake xylitol under oxidative stresses
Compared to the NX group, yeast cells under oxidative stress exhibited a significant increase in intracellular xylitol levels following xylitol treatment. The intracellular xylitol concentration showed a positive relation to the increasing external xylitol concentrations. After 10 min treatment, the xylitol concentration in 0.5 and 1 M XT cells was significantly increased by 35.5% and 45%, respectively. When treated to 30 min, the highest intracellular xylitol levels were observed in 1 M XT cells (Figure 3).
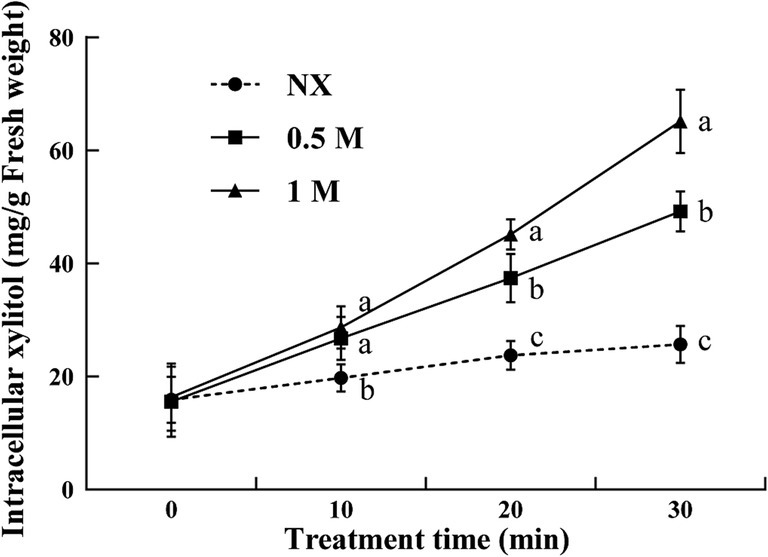
Figure 3. Intracellular xylitol concentrations of Meyerozyma guilliermondii cells under H2O or xylitol subjected to a subsequent oxidative (30 mM H2O2) stress for 10, 20 or 30 min. Prior to exposure to the subsequent oxidative stress served as Time 0. Xylitol concentrations represent as milligram per gram of yeast cell fresh weight (mg/g FW). Data represent the mean ± standard deviation of three independent experiments, where each experiment consisted of three biological replicates (n = 9). Columns with different letters are significantly different according to a Duncan’s multiple range test at p < 0.05. ns, no significance.
3.4 Effect of xylitol treatment on the antioxidant system of Meyerozyma guilliermondii
The activities of thioredoxin reductase (TrxR) and peroxidase (POD) were evaluated in pre-treated and NX samples in response to oxidative stress. The findings revealed that XT cells had significantly higher TrxR and POD activities compared to NX yeast cells (Figure 4). Under oxidative stress, TrxR activity in XT cells was significantly greater than in NX cells (Figure 4A). Similarly, POD activity in XT cells exposed to oxidative stress remained consistently higher than in NX cells across all time points (Figure 4B). In M. guilliermondii cells treated with xylitol, both the activities of these antioxidant enzymes and their corresponding gene expression levels (Figure 5) were significantly elevated at all-time points (from 0 to 30 min), both before and after exposure to oxidative stress, compared to untreated cells.
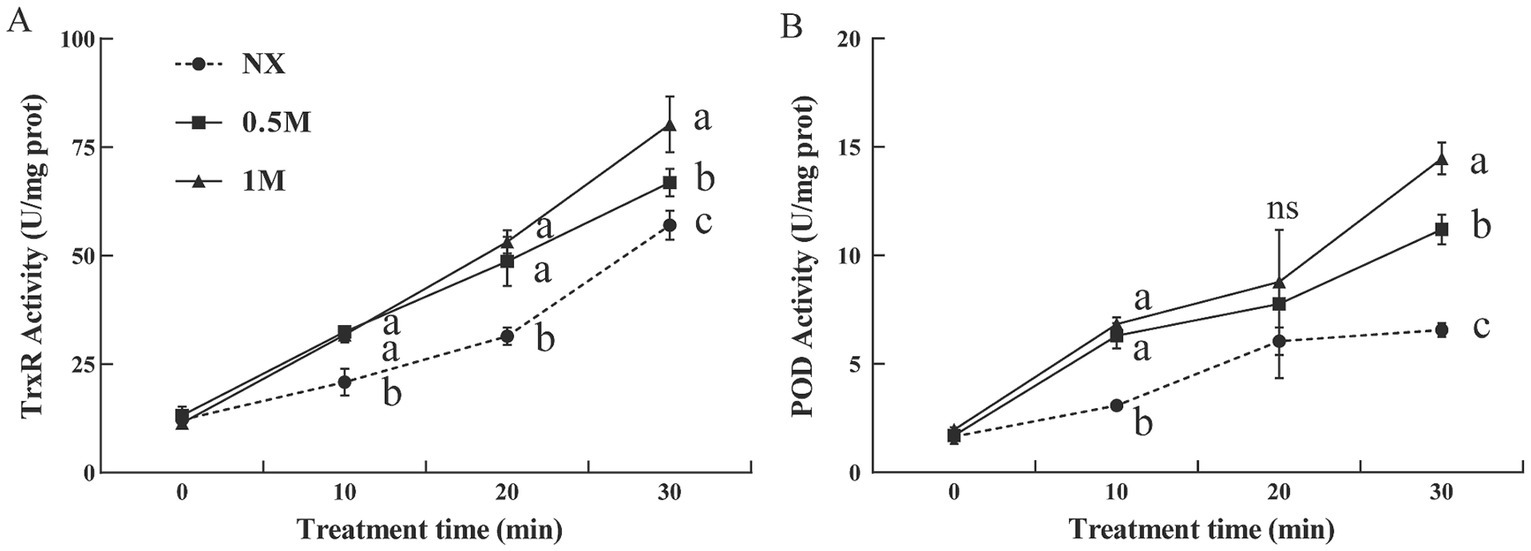
Figure 4. Thioredoxin reductase [(A), TrxR] and peroxidase [(B), POD] activity of Meyerozyma guilliermondii cells under H2O or xylitol subjected to a subsequent oxidative (30 mM H2O2) stress for 10, 20 or 30 min. Prior to exposure to the subsequent oxidative stress served as Time 0. Data represent the mean ± standard deviation of three independent experiments, where each experiment consisted of three biological replicates (n = 9). Columns with different letters are significantly different according to a Duncan’s multiple range test at p < 0.05. ns, no significance.
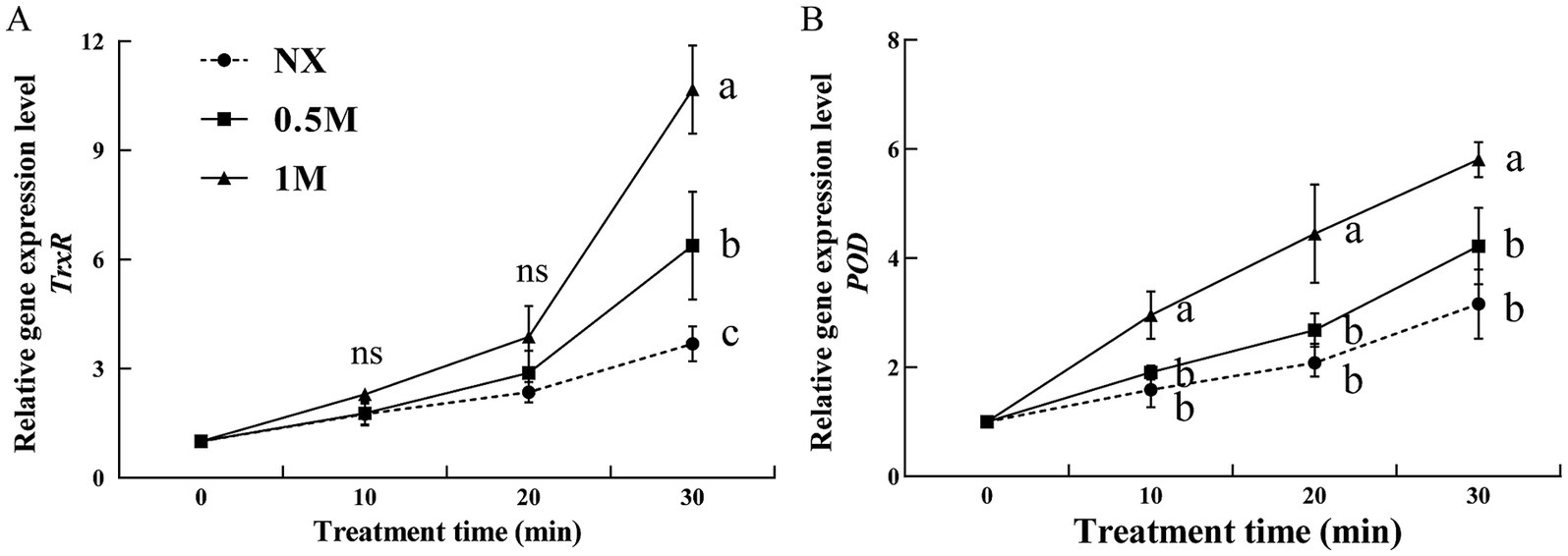
Figure 5. Two antioxidant genes [TrxR (A) and POD (B)] expression levels of Meyerozyma guilliermondii cells under H2O or xylitol subjected to a subsequent oxidative (30 mM H2O2) stress for 10, 20, or 30 min. Prior to exposure to the subsequent oxidative stress served as Time 0. Data represent the mean ± standard deviation of three independent experiments, where each experiment consisted of three biological replicates (n = 9). Columns with different letters are significantly different according to a Duncan’s multiple range test at p < 0.05. ns: no significance.
3.5 Biocontrol of postharvest diseases in apples using Meyerozyma guilliermondii
As shown, the antagonistic yeast M. guilliermondii significantly reduced the incidence and lesion diameter of blue mold and gray mold, caused by Botrytis cinerea and Penicillium expansum, respectively, in apples. Notably, the incidence of both molds in fruit treated with fresh yeast was approximately 50% lower than in the controls, where disease incidence reached 100% for both pathogens. In contrast, the incidence of apple rot was significantly reduced in XT cells compared to the controls and H2O2 treatments (Figures 6A,C,E). Additionally, the lesion diameter on apples infected with B. cinerea and P. expansum was significantly smaller in fruit treated with XT cells than in the control and H2O2 groups (Figures 6B,D,F). These results demonstrate that M. guilliermondii effectively reduced the incidence and severity of blue mold and gray mold pathogens, with XT yeast cells further enhancing the level of control over the control treatments.
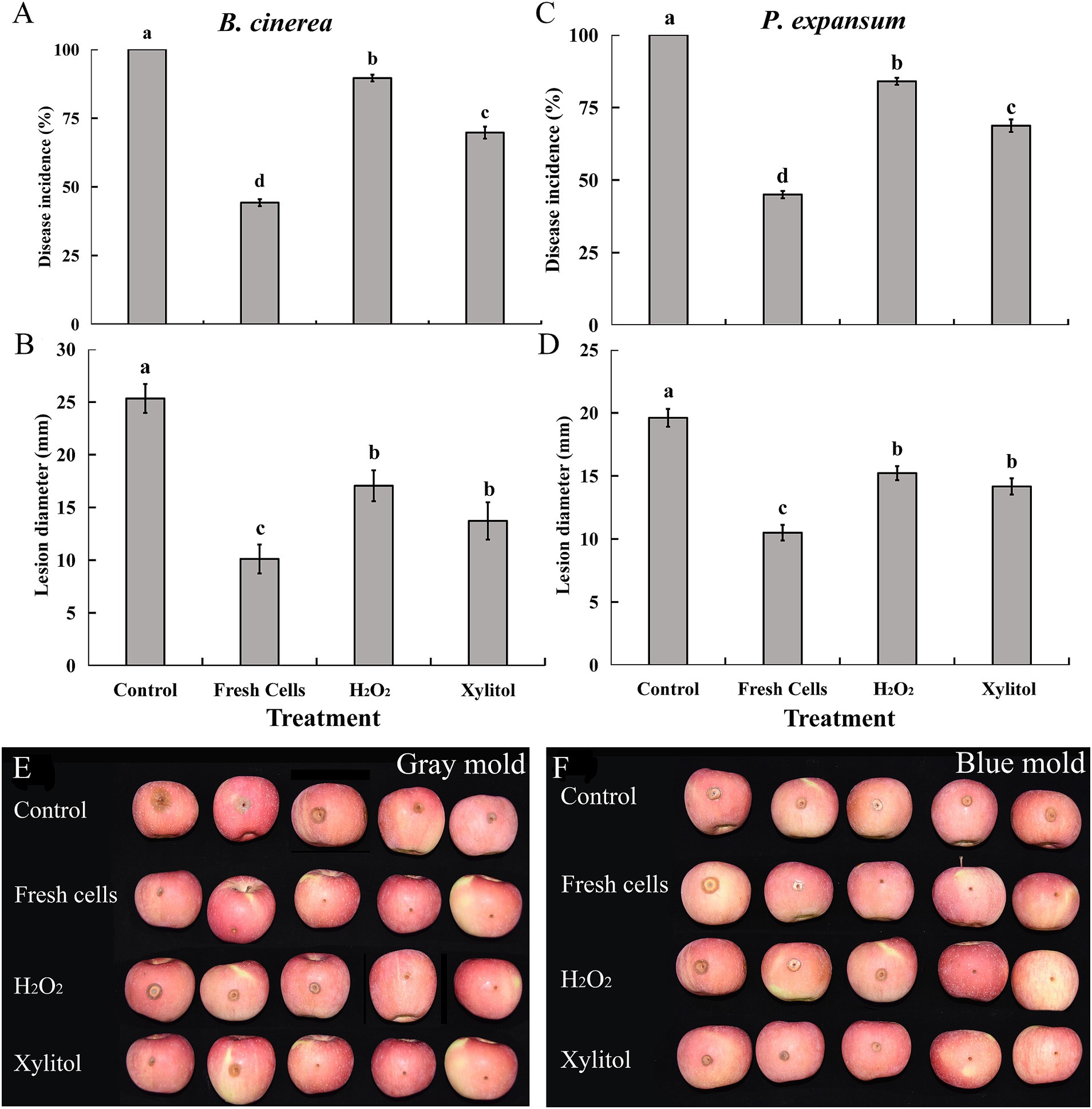
Figure 6. Biocontrol efficacy of Meyerozyma guilliermondii against gray mold caused by Botrytis cinerea (A,B), blue mold caused by Penicillium expansum (C,D) in apples. Panels (E,F) showing the efficacy of xylitol on M. guilliermondii against gray mold and blue mold. Data represent the mean ± standard deviation of three independent experiments, where each experiment consisted of three biological replicates (n = 9). Columns with different letters are significantly different according to a Duncan’s multiple range test at p < 0.05.
4 Discussion
Biocontrol agents used in postharvest disease management face challenges posed by various abiotic stresses in packing houses and postharvest environments, such as oxidative stress, elevated temperatures, nutrient deficiencies, and unfavorable pH levels. The ability of yeast to survive and remain active under these stresses is critical for improving their biocontrol efficacy (Wang et al., 2010; An et al., 2012). Since biocontrol yeasts are particularly vulnerable to oxidative conditions, their survival is significantly compromised under oxidative stress (Wang et al., 2018).
Xylitol, a sugar alcohol commonly used as a food additive, acts as an antioxidant, presenting a potential strategy to enhance the stress tolerance of yeasts (Kulikova-Borovikova et al., 2018; Wei et al., 2022). This study assessed the survival of yeast cultures treated with xylitol for 1 h under oxidative stress. The results demonstrate that xylitol treatment showed a significant promoting effect on the survival rate of M. guilliermondii under oxidative stress, with higher xylitol concentrations leading to a more pronounced protective effect. The XT yeast cells exhibited higher survival rates, may be attributed to two potential mechanisms. Firstly, the xylitol treatment provides limited osmotic protection, which reduced oxidative stress-induced damage (Karlgren et al., 2005). Secondly, the uptake of xylitol by yeast cells (Figure 3) may improve intracellular water utilization, thereby contributing to the resistance against oxidative stress (Krallish et al., 1997). It should be noted that the viability in this study was quantified as CFU, which may result in an underestimation of the actual level of viable cells. This is due to the fact that, following treatment, some yeast cells may have reduced vitality, preventing them from growing on the culture medium. However, these cells still exhibited detectable changes in enzyme activity and lower levels of ATP.
Previous research has demonstrated that exposure to oxidative stress can severely impair cell viability by increasing ROS production (Liu et al., 2011; Sui and Liu, 2014). The application of modified minimal mineral media, sugars, and sugar alcohols has been shown to mitigate the impact of high-temperature and oxidative stress in biocontrol yeasts by reducing intracellular ROS levels and minimizing oxidative damage (Sui and Liu, 2014; Ming et al., 2020). In this study, yeast cells treated with xylitol (XT group) exhibited lower levels of ROS, mitochondria dysfunction and higher ATP levels compared to the control cells. The correlation between lower ROS production, mitochondrial membrane potential and improved cell viability under oxidative stress suggests that xylitol enhances the oxidative resistance of yeast used as biocontrol agents. Since xylitol is involved in the glycolysis and the pentose phosphate pathway (Bertels et al., 2021; Narisetty et al., 2022), the uptake of xylitol by the yeast may regulate sugar metabolism or act as energy source, thus maintaining ATP production (Figure 3).
Antioxidant gene expression in yeast is typically upregulated in response to various stressors, helping the cells cope with environmental challenges (Martínez-Pastor et al., 2010; Huang et al., 2021). It has been reported that POD and TrxR participate in the oxidative response and enzymatic detoxification of ROS (Wang et al., 2018; Sun et al., 2021). In this study, xylitol treatment resulted in increased expression of TrxR and POD in yeast cells exposed to oxidative stress, which may be effective in enhancing the antioxidative defense of yeasts. Moreover, the enzyme activities of TrxR and POD, both essential for ROS detoxification (Yan et al., 2018; Sun et al., 2021; Huang et al., 2022), were significantly higher in XT cells. TrxR catalyzes the reduction of thioredoxin, while POD protects cells from ROS damage, playing a key role in biocontrol yeast (Greetham and Grant, 2009; Gostimskaya and Grant, 2016; Sun et al., 2021). The enhanced activity of these enzymes, along with their increased gene expression, indicated improved ROS clearance and higher survival rates in the XT yeast cells. Oxidative stress has also been shown to cause mitochondrial damage (Sui and Liu, 2014; Ming et al., 2020). In this study, oxidative stress significantly decreased ATP levels in the control cells, whereas xylitol treatment effectively mitigated this decline. The increase in survival, enhanced antioxidant enzyme activity, and reduction in ROS levels with xylitol treatment suggest a potential improvement in the biocontrol efficiency of yeast.
Xylitol, a widely utilized food additive, has been confirmed safe and healthy for consumption (Chen et al., 2010; Rice et al., 2020). Previous studies have indicated that under dehydration stress, yeasts such as Saccharomyces cerevisiae and Pachysolen tannophilus preferentially synthesize intracellular xylitol, which enhances their resistance to dehydration, with survival rates increased to 68% and 57%, respectively (Krallish et al., 1997). These findings underscore the potential of xylitol in enhancing yeast stress tolerance. For instance, treatment with 1 M xylitol elevated the viability of dried S. cerevisiae cells to 70% under dehydration, while a 2% (approximately 0.13 M) xylitol treatment improved the survival rate of Zygosaccharomyces rouxii from 65% to 69% following a 20-min exposure to 40°C heat stress (Kulikova-Borovikova et al., 2018; Wei et al., 2022). In this study, we systematically examined the impact of xylitol treatment on biocontrol yeasts under oxidative stress. Given xylitol’s involvement in the xylose metabolic pathway (Kumar et al., 2022), its absorption by yeast may enhance metabolic activity, thereby improving cell survival. Under oxidative stress induced by 30 mM H2O2, XT cells exhibited at least a 6% higher viability compared to previous reports. Furthermore, we demonstrated for the first time that xylitol treatment significantly mitigated oxidative damage in M. guilliermondii, with TrxR and POD activities increasing by at least 18 and 40%, respectively, leading to a 25% increase in ATP production. Notably, XT cells showed enhanced efficacy in reducing apple infection by B. cinerea and P. expansum.
High survival and growth of biocontrol yeasts on wounds and fruit surfaces provide them with a competitive edge in acquiring nutrients and space (Liu et al., 2013). XT yeast cells exhibited improved survival and biocontrol efficiency compared to untreated cells. Previous studies have also shown that pretreatment with protectants such as glucose and sorbitol can enhance the biocontrol efficacy of yeast (Sui et al., 2012; Sui and Liu, 2014; Ming et al., 2020). Lesion diameters were measured at their largest point, providing an indicator of the maximum extent of infection. Additionally, XT cells were more effective in reducing the incidence of gray mold and blue mold than the control, consistent with previous research, which demonstrated that pretreatments can improve stress tolerance and activate antioxidant defenses, leading to enhanced biocontrol efficiency (Liu et al., 2011; Chi et al., 2015).
5 Conclusion
This study demonstrated that xylitol treatment significantly enhances the tolerance and efficacy of M. guilliermondii under oxidative stress conditions. Xylitol treatment notably improved yeast survival rates, antioxidant enzyme activities and intracellular xylitol levels, thereby reducing the intracellular accumulation of ROS, mitochondrial dysfunction and preserving ATP levels during oxidative stress. The enhanced oxidative stress tolerance conferred by xylitol also contributed to improved biocontrol performance, as evidenced by reduced disease incidence and lesion diameter in apples subjected to pathogenic fungal infections. These findings underscore the potential of xylitol as an effective treatment to enhance the efficiency of biocontrol yeasts.
Data availability statement
The original contributions presented in the study are included in the article/supplementary material, further inquiries can be directed to the corresponding author.
Author contributions
WZ: Data curation, Formal analysis, Investigation, Software, Visualization, Writing – original draft. SZ: Conceptualization, Funding acquisition, Resources, Supervision, Validation, Writing – review & editing. JG: Data curation, Investigation, Software, Visualization, Writing – review & editing.
Funding
The author(s) declare that financial support was received for the research and/or publication of this article. This work was supported by the National Natural Science Foundation of China (32471979) and Education Department of Hunan Province of China (24C0638).
Conflict of interest
The authors declare that the research was conducted in the absence of any commercial or financial relationships that could be construed as a potential conflict of interest.
Generative AI statement
The authors declare that no Gen AI was used in the creation of this manuscript.
Publisher’s note
All claims expressed in this article are solely those of the authors and do not necessarily represent those of their affiliated organizations, or those of the publisher, the editors and the reviewers. Any product that may be evaluated in this article, or claim that may be made by its manufacturer, is not guaranteed or endorsed by the publisher.
References
An, B., Li, B., Qin, G., and Tian, S. (2012). Exogenous calcium improves viability of biocontrol yeasts under heat stress by reducing ROS accumulation and oxidative damage of cellular protein. Curr. Microbiol. 65, 122–127. doi: 10.1007/s00284-012-0133-4
Bertels, L.-K., Fernández Murillo, L., and Heinisch, J. J. (2021). The pentose phosphate pathway in yeasts-more than a poor cousin of glycolysis. Biomol. Ther. 11:725. doi: 10.3390/biom11050725
Bradford, M. M. (1976). A rapid and sensitive method for the quantitation of microgram quantities of protein utilizing the principle of protein-dye binding. Anal. Biochem. 72, 248–254. doi: 10.1016/0003-2697(76)90527-3
Chen, X., Jiang, Z.-H., Chen, S., and Qin, W. (2010). Microbial and bioconversion production of D-xylitol and its detection and application. Int. J. Biol. Sci. 6, 834–844. doi: 10.7150/ijbs.6.834
Chi, M., Li, G., Liu, Y., Liu, G., Li, M., Zhang, X., et al. (2015). Increase in antioxidant enzyme activity, stress tolerance and biocontrol efficacy of Pichia kudriavzevii with the transition from a yeast-like to biofilm morphology. Biol. Control 90, 113–119. doi: 10.1016/j.biocontrol.2015.06.006
Droby, S., Wisniewski, M., Teixidó, N., Spadaro, D., and Jijakli, M. H. (2016). The science, development, and commercialization of postharvest biocontrol products. Postharvest Biol. Technol. 122, 22–29. doi: 10.1016/j.postharvbio.2016.04.006
Espinosa-Salgado, R., Tamayo-Galván, V., Perraud-Gaime, I., Rodríguez-Serrano, G. M., González-Robles, R. O., Durand, N., et al. (2022). Polyols induce the production of antifungal compounds by Lactobacillus plantarum. Curr. Microbiol. 79:99. doi: 10.1007/s00284-022-02761-4
Gostimskaya, I., and Grant, C. M. (2016). Yeast mitochondrial glutathione is an essential antioxidant with mitochondrial thioredoxin providing a back-up system. Free Radic. Biol. Med. 94, 55–65. doi: 10.1016/j.freeradbiomed.2016.02.015
Greetham, D., and Grant, C. M. (2009). Antioxidant activity of the yeast mitochondrial one-cys peroxiredoxin is dependent on thioredoxin reductase and glutathione in vivo. Mol. Cell. Biol. 29, 3229–3240. doi: 10.1128/MCB.01918-08
Huang, Y., Cai, Y., and Yu, T. (2022). Sodium glutamate as a booster: inducing Rhodosporidium paludigenum to enhance the inhibition of Penicillium expansum on pears. J. Appl. Microbiol. 132, 1239–1249. doi: 10.1111/jam.15212
Huang, K., Sui, Y., Miao, C., Chang, C., Wang, L., Cao, S., et al. (2021). Melatonin enhances the resistance of ginger rhizomes to postharvest fungal decay. Postharvest Biol. Technol. 182:111706. doi: 10.1016/j.postharvbio.2021.111706
Karlgren, S., Pettersson, N., Nordlander, B., Mathai, J. C., Brodsky, J. L., Zeidel, M. L., et al. (2005). Conditional osmotic stress in yeast: A system to study transport through aquaglyceroporins and osmostress signaling. J. Biol. Chem. 280, 7186–7193. doi: 10.1074/jbc.M413210200
Kim, S. M., Lee, S. M., Seo, J.-A., and Kim, Y.-S. (2018). Changes in volatile compounds emitted by fungal pathogen spoilage of apples during decay. Postharvest Biol. Technol. 146, 51–59. doi: 10.1016/j.postharvbio.2018.08.003
Krallish, I., Jeppsson, H., Rapoport, A., and Hahn-Hägerdal, B. (1997). Effect of xylitol and trehalose on dry resistance of yeasts. Appl. Microbiol. Biotechnol. 47, 447–451. doi: 10.1007/s002530050954
Kulikova-Borovikova, D., Lisi, S., Dauss, E., Alamae, T., Buzzini, P., Hallsworth, J. E., et al. (2018). Activity of the α-glucoside transporter Agt1 in Saccharomyces cerevisiae cells during dehydration-rehydration events. Fungal Biol. 122, 613–620. doi: 10.1016/j.funbio.2018.03.006
Kumar, K., Singh, E., and Shrivastava, S. (2022). Microbial xylitol production. Appl. Microbiol. Biotechnol. 106, 971–979. doi: 10.1007/s00253-022-11793-6
Leaw, S. N., Chang, H. C., Sun, H. F., Barton, R., Bouchara, J.-P., and Chang, T. C. (2006). Identification of medically important yeast species by sequence analysis of the internal transcribed spacer regions. J. Clin. Microbiol. 44, 693–699. doi: 10.1128/jcm.44.3.693-699.2006
Li, B., Lai, T., Qin, G., and Tian, S. (2010). Ambient ph stress inhibits spore germination of Penicillium expansum by impairing protein synthesis and folding: A proteomic-based study. J. Proteome Res. 9, 298–307. doi: 10.1021/pr900622j
Liu, J., Sui, Y., Wisniewski, M., Droby, S., and Liu, Y. (2013). Review: utilization of antagonistic yeasts to manage postharvest fungal diseases of fruit. Int. J. Food Microbiol. 167, 153–160. doi: 10.1016/j.ijfoodmicro.2013.09.004
Liu, J., Sui, Y., Wisniewski, M., Droby, S., Tian, S., Norelli, J., et al. (2012a). Effect of heat treatment on inhibition of Monilinia fructicola and induction of disease resistance in peach fruit. Postharvest Biol. Technol. 65, 61–68. doi: 10.1016/j.postharvbio.2011.11.002
Liu, J., Wisniewski, M., Droby, S., Norelli, J., Hershkovitz, V., Tian, S., et al. (2012b). Increase in antioxidant gene transcripts, stress tolerance and biocontrol efficacy of Candida oleophila following sublethal oxidative stress exposure. FEMS Microbiol. Ecol. 80, 578–590. doi: 10.1111/j.1574-6941.2012.01324.x
Liu, J., Wisniewski, M., Droby, S., Vero, S., Tian, S., and Hershkovitz, V. (2011). Glycine betaine improves oxidative stress tolerance and biocontrol efficacy of the antagonistic yeast Cystofilobasidium infirmominiatum. Int. J. Food Microbiol. 146, 76–83. doi: 10.1016/j.ijfoodmicro.2011.02.007
Livak, K. J., and Schmittgen, T. D. (2001). Analysis of relative gene expression datausing realtime quantitative PCR and the 2–ΔΔCT method. Methods 25, 402–408. doi: 10.1006/meth.2001.1262
Macarisin, D., Droby, S., Bauchan, G., and Wisniewski, M. (2010). Superoxide anion and hydrogen peroxide in the yeast antagonist–fruit interaction: A new role for reactive oxygen species in postharvest biocontrol? Postharvest Biol Technol. 58, 194–202. doi: 10.1016/j.postharvbio.2010.07.008
Martínez-Pastor, M., Proft, M., and Pascual-Ahuir, A. (2010). Adaptive changes of the yeast mitochondrial proteome in response to salt stress. OMICS 14, 541–552. doi: 10.1089/omi.2010.0020
Ming, X., Wang, Y., and Sui, Y. (2020). Pretreatment of the antagonistic yeast, Debaryomyces hansenii, with mannitol and sorbitol improves stress tolerance and biocontrol efficacy. Front. Microbiol. 11:601. doi: 10.3389/fmicb.2020.00601
Morón de Salim, A. R., and Ramírez Mérida, L. G. (2013). Bacteriostatic effect and/or xylitol bactericide of crops on Listeria monocytogenes. Arch. Latinoam. Nutr. 63, 173–179
Narisetty, V., Cox, R., Bommareddy, R., Agrawal, D., Ahmad, E., Pant, K. K., et al. (2022). Valorisation of xylose to renewable fuels and chemicals, an essential step in augmenting the commercial viability of lignocellulosic biorefineries. Sustain. Energy Fuels 6, 29–65. doi: 10.1039/D1SE00927C
Rice, T., Zannini, E., Arendt, K., and Coffey, A. (2020). A review of polyols—biotechnological production, food applications, regulation, labeling and health effects. Crit. Rev. Food Sci. Nutr. 60, 2034–2051. doi: 10.1080/10408398.2019.1625859
Romanazzi, G., Smilanick, J. L., Feliziani, E., and Droby, S. (2016). Integrated management of postharvest gray mold on fruit crops. Postharvest Biol. Technol. 113, 69–76. doi: 10.1016/j.postharvbio.2015.11.003
Sadeghi, R., Aminian, H., Remize, F., Sheikh, M., and Ebrahimi, L. (2021). Integrated control of blue and gray molds of apples with antagonistic yeasts combined with carbon dioxide or ozone. J. Plant Pathol. 103, 943–953. doi: 10.1007/s42161-021-00891-5
Spadaro, D., and Droby, S. (2016). Development of biocontrol products for postharvest diseases of fruit: the importance of elucidating the mechanisms of action of yeast antagonists. Trends Food Sci. Tech. 47, 39–49. doi: 10.1016/j.tifs.2015.11.003
Sui, Y., and Liu, J. (2014). Effect of glucose on thermotolerance and biocontrol efficacy of the antagonistic yeast Pichia guilliermondii. Biol. Control 74, 59–64. doi: 10.1016/j.biocontrol.2014.04.003
Sui, Y., Liu, J., Wisniewski, M., Droby, S., Norelli, J., and Hershkovitz, V. (2012). Pretreatment of the yeast antagonist, Candida oleophila, with glycine betaine increases oxidative stress tolerance in the microenvironment of apple wounds. Int. J. Food Microbiol. 157, 45–51. doi: 10.1016/j.ijfoodmicro.2012.04.010
Sui, Y., Wisniewski, M., Droby, S., and Liu, J. (2015). Responses of yeast biocontrol agents to environmental stress. Appl. Environ. Microbiol. 81, 2968–2975. doi: 10.1128/AEM.04203-14
Sun, K., Wang, Z., Zhang, X., Wei, Z., Zhang, X., Li, L., et al. (2021). Enhancement of biocontrol efficacy of Pichia kudriavzevii induced by ca ascorbate against Botrytis cinerea in cherry tomato fruit and the possible mechanisms of action. Microbiol. Spectr 9, e01507–e01521. doi: 10.1128/spectrum.01507-21
Vilanova, L., Vall-llaura, N., Torres, R., Usall, J., Teixidó, N., Larrigaudière, C., et al. (2017). Penicillium expansum (compatible) and Penicillium digitatum (non-host) pathogen infection differentially alter ethylene biosynthesis in apple fruit. Plant Physiol. Biochem. 120, 132–143. doi: 10.1016/j.plaphy.2017.09.024
Wang, Y., Luo, Y., Sui, Y., Xie, Z., Liu, Y., Jiang, M., et al. (2018). Exposure of Candida oleophila to sublethal salt stress induces an antioxidant response and improves biocontrol efficacy. Biol. Control 127, 109–115. doi: 10.1016/j.biocontrol.2018.09.002
Wang, Y., Wang, P., Xia, J., Yu, T., Lou, B., Wang, J., et al. (2010). Effect of water activity on stress tolerance and biocontrol activity in antagonistic yeast Rhodosporidium paludigenum. Int. J. Food Microbiol. 143, 103–108. doi: 10.1016/j.ijfoodmicro.2010.07.035
Wei, Y., Yan, Z., Liu, M., Chen, D., Chen, X., and Li, X. (2022). Metabolic characteristics of intracellular trehalose enrichment in salt-tolerant Zygosaccharomyces rouxii. Front. Microbiol. 13:935756. doi: 10.3389/fmicb.2022.935756
Wisniewski, M., Droby, S., Norelli, J., Liu, J., and Schena, L. (2016). Alternative management technologies for postharvest disease control: the journey from simplicity to complexity. Postharvest Biol. Technol. 122, 3–10. doi: 10.1016/j.postharvbio.2016.05.012
Keywords: Meyerozyma guilliermondii, biocontrol efficiency, xylitol, antagonist, gray mold
Citation: Zhang W, Zhuang S and Gao J (2025) Xylitol promotes the antioxidant and biocontrol efficiency of the antagonistic yeast, Meyerozyma guilliermondii. Front. Microbiol. 16:1545248. doi: 10.3389/fmicb.2025.1545248
Edited by:
Clemencia Chaves, University of Teramo, ItalyReviewed by:
Johannes Delgado-Ospina, University of San Buenaventura Cali, ColombiaChunyu Zhu, Liaoning University, China
Copyright © 2025 Zhang, Zhuang and Gao. This is an open-access article distributed under the terms of the Creative Commons Attribution License (CC BY). The use, distribution or reproduction in other forums is permitted, provided the original author(s) and the copyright owner(s) are credited and that the original publication in this journal is cited, in accordance with accepted academic practice. No use, distribution or reproduction is permitted which does not comply with these terms.
*Correspondence: Shunyao Zhuang, c3l6aHVhbmdAaXNzYXMuYWMuY24=