- 1Department of Urology, The Affiliated Hospital of Guizhou Medical University, Guiyang, China
- 2Department of Urology & Andrology, The First Affiliated Hospital of Guizhou University of Traditional Chinese Medicine, Guiyang, China
The gut microbiota helps to reveal the relationship between diseases, but the role of gut microbiota in prostate cancer (PCa) is still unclear. Recent studies have found that the composition and abundance of specific gut microbiota are significantly different between PCa and non-PCa, and the gut microbiota may have common and unique characteristics between different diseases. Intestinal microorganisms are affected by various factors and interact with the host in a variety of ways. In the complex interaction model, the regulation of intestinal microbial metabolites and the host immune system is particularly important, and they play a key role in maintaining the ecological balance of intestinal microorganisms and metabolites. However, specific changes in the composition of intestinal microflora may promote intestinal mucosal immune imbalance, leading to the formation of tumors. Therefore, this review analyzes the immune regulation of intestinal flora and the production of metabolites, as well as their effects and mechanisms on tumors, and briefly summarizes that specific intestinal flora can play an indirect role in PCa through their metabolites, genes, immunity, and pharmacology, and directly participate in the occurrence, development, and treatment of tumors through bacterial and toxin translocation. We also discussed markers of high risk PCa for intestinal microbiota screening and the possibility of probiotic ingestion and fecal microbiota transplantation, in order to provide better treatment options for clinic patients. Finally, after summarizing a number of studies, we found that changes in immunity, metabolites.
1 Introduction
1.1 Current Status of prostate cancer
Prostate cancer (PCa) is the second most common malignant tumor in men and the fifth largest cause of tumor-related death in the world (1). There are about 800,000 new cases of PCa and 300,000 deaths worldwide every year (2). The risk factors for PCa include genetic history, family history, diet, environment, African population, and elderly population (3–5). The incidence of PCa, according to global cancer statistics for 2020, varies by region and ranges from 6.3 to 83.4 per 100,000 people, with the highest rates found in Northern Europe, Western Europe, the Caribbean, Australia/New Zealand, North America, and Southern Africa, and the lowest in Asian nations (6). However, the pathogenesis and development of PCa are still unclear. One of the accepted explanations is the effect of androgen stimulation and the defect of prostate cell apoptosis (7). When PCa progresses to an advanced stage, it is usually treated with surgery or androgen deprivation therapy (ADT), but almost all patients develop resistance to ADT, which inevitably leads to castration-resistant prostate cancer (CRPC) with a worse prognosis (8). ADT is a significant PCa treatment, but there is no proof that it takes other PCa risk factors, like bacterial infection, environmental changes, or inflammatory effects, into account when planning a treatment plan (9). More and more evidence shows that microflora is involved in the microenvironment of tumors and may play a role in tumorigenesis (10). The gut microbiome can influence the growth and progression of PCa through its derived metabolites, suggesting the existence of a gut-prostate axis (11). For example, another source of androgen may be provided by intestinal microflora, which promotes the progress of PCa (12).
With the increasing incidence of PCa, in order to understand the potential contribution of intestinal microbiota and microbial metabolites to the emergence of PCa, as well as novel approaches for the prevention and treatment of PCa based on intestinal microbiota, this review examines studies on PCa and intestinal microbiota.
1.2 The gut microbiome and PCa
The human gut is home to trillions of microorganisms that form a complex community of bacteria, protozoa, fungi, and viruses, which together make up the intestinal microbiota (13). 99% of the total microorganisms in the human body are located in the intestinal tract. Intestinal microbiota not only plays an irreplaceable role locally, but also people pay more and more attention to the distal effect (14). According to their level of pathogenicity, the bacteria in the gastrointestinal tract can be categorized into three groups: pathogenic bacteria, possibly harmful bacteria, and helpful bacteria. When helpful bacteria are present, they can prevent the growth of harmful bacteria, promote the creation of vitamins, inhibit the breakdown and fermentation of food components, and stimulate the immune system and food tolerance. The pathogenic bacteria effects are the body’s food intolerance, infection, and inflammation. Potential pathogen species belong to the normal microbial community in the gut, such as Escherichia coli, Enterobacteria, Streptococci, Enterococci, and Bacteroides. When changes occur in the body’s gut that cause these microbes to be present in large numbers, they can cause disease (15). The imbalance of intestinal flora can lead to flora imbalance, and long-term flora imbalance may lead to obesity, type 2 diabetes, insulin resistance, hyperlipidemia, hypertension, metabolic dysfunction, inflammation, and cancer (16).
Intestinal microflora is affected by a variety of external factors, including environmental, dietary, and regional changes, and is involved in all stages of cancer, including initiation, progression, treatment outcomes, and adverse reactions (17–20). The presence of a tumor may lead to an increase in microbial diversity at the tumor site and a decrease in the diversity of gut microbiota (21), and this change may be a precursor to the occurrence and development of cancer, or even directly lead to the occurrence of cancer. Recent years have seen a rapid development of bacterial 16s ribosomal RNA genome sequencing and shotgun sequencing as human microbiome analytical tools. Future options for treating cancer, particularly PCa, are anticipated to include the microbiome because it plays a significant role in immune system control (22, 23). The set of genes of intestinal bacteria is defined as a functional estrogen genome, and its products can metabolize estrogen. The potential effect of individual intestinal microflora on estrogen group is also considered to be a possibility. Since there is an established correlation between estrogen levels and PCa risk, the metabolic effects of intestinal microbiota are associated with PCa risk (24). With the growing understanding of the role of microbes in the process of cancer development, several studies have been conducted to investigate the link between specific gut microbes and PCa. There were significant differences between PCa patients and healthy patients. Compared with healthy patients, the intestinal microbiota of PCa patients showed an increase in α-diversity and structural changes (25–27). Golombos et al. compared rectal swab samples from PCa patients with healthy patients and found a higher relative abundance of Bacteriodes massiliensis and a decrease in the number of bacteria that produce folic acid and biotin (26). Liss et al. found that Bacteroides and Streptococcal were significantly enriched in PCa patients compared with non-PCa patients (25). Matsushita et al. enrolled 152 Japanese men who underwent prostate biopsies and divided them into two groups: the high-risk group and the low-risk group. They found that the relative abundance of Rikenellaceae, Alistipes, and Lachnospira increased significantly in the high-risk group, and all of these bacteria produced short-chain fatty acids (28). Smith included the fecal microflora of 22 patients with overweight breast cancer or PCa (BMI > 25 kg/m2) and 22 controls. It was found that the β-diversity index of PCa was significantly different from that of the control group, and the abundance of Tissierellaceae, Lachnospira, and Ruminococcaceae was higher (29).
The decline in testosterone levels leads to harmful changes in the intestinal microbiota. This ecological imbalance may lead to increased weakness and an increased risk of adverse consequences in patients with PCa. Kure included 24 patients treated with ADT. It was found that the α-diversity and β-diversity of intestinal microbiota and the relative abundance of Gammaproteobacteria, Proteobacteria, Pseudomonas, and Pseudomonadale decreased significantly at 24 weeks after ADT (30). Joseph et al. performed 16SrRNA gene analysis on the fecal microflora of 86 PCa patients (56 ADT, 30 underwent prostatectomy) and found that the diversity was significantly decreased in the ADT group. There were significant differences in β diversity among groups. In the ADT group, the relative abundance of Ruminococcus and Bacteroides was higher, while Lachnospira and Roseburia decreased. In the ADT group, the ratio of Firmicutes to Bacteroidetes was also lower (31). Liu et al. analyzed the fecal microflora of 21 patients with hormone-sensitive prostate cancer (HSPC) and CRPC who received ADT by using the sequence of 16S rRNA gene amplifiers. It was found that with the increase in abundance of several bacteria, including Bacillus and Ruminococcus, the intestinal microflora in CRPC changed significantly (32).
Patients with colon cancer, pancreatic cancer, and melanoma patients can take oral broad-spectrum antibiotics to inhibit the growth and metastasis of their tumors (33). However, oral antibiotic therapy has the opposite effect in PCa, One of the external elements that contribute to ecological issues is antibiotic use, which is thought to be the primary cause of changes in the species of gut microbes that may be temporary or permanent. It has been demonstrated that the risk of numerous malignant tumors, including PCa, is somewhat increased by the use of antibiotics (34). Boursi et al. hypothesized that antibiotics can cause changes in intestinal bacterial diversity and lead to chronic infection. They found in 27212 PCa patients and 105940 volunteers that the use of penicillins, quinolones, sulfonamides, and tetracyclines moderately increased the risk of PCa (35). Tulstrup et al. hypothesized that alterations in intestinal permeability may be connected to antibiotic-induced changes in microbiota. For 10–11 days, they administered amoxicillin, cefotaxime, vancomycin, metronidazole, or water to 60 Wistar rats. It was discovered that the three antibiotics significantly altered bacterial α-diversity, composition, and cecal short-chain fatty acids. The general decrease of intestinal microbial diversity and the increase of Proteobacteria relative abundance were observed (36). Matsushita et al. gave PCa mice on a high-fat diet (HFD) an oral antibiotic mixture and found that the number of Rikenellaceae and Clostridiales increased and the composition of intestinal microflora was significantly different from that of the control group, which inhibited the proliferation of PCa cells and decreased the expression of Igf1 and the level of circulating insulin-like growth factor-1 (IGF1) in the prostate (37).
Diet is closely related to the distribution of intestinal microflora and the construction of intestinal microbial structures. Intestinal microflora also affect the absorption, digestion, and metabolism of food (38, 39). Studies have shown that HFD increases Anaerobion and Bacteroides (40), transforming a low-fat, plant polysaccharide-rich diet into a high-fat/high-sugar “western” diet, β-diversity changes significantly after a week and changes the representativeness of metabolic pathways in the microbiome (41). The number of Ochrobactrum overexpressed in prostatic fluid of PCa patients was also significantly increased in HFD mice (42).In a study of HFD mice, HFD promoted PCa growth through histamine signal transduction in mast cells, and changes in microflora in mouse feces promoted histamine biosynthesis and increased the growth of inflammatory cancer cells (43).
To sum up, compared with healthy patients, there are significant differences in intestinal microflora in patients with PCa, ADT treatment, antibiotic treatment, and HFD. Specific changes in gut microbes may play a role in different types of PCa, which can further explore the role of different intestinal microorganisms in the pathogenesis of PCa, Table 1 summarizes the studies of specific microbes and PCa risk.
2 Pathways of action of the gut microbiota on PCa
Manzoor et al. divided the relationship between microbiota and cancer into three levels: primary (interaction in the tumor proximal microenvironment), secondary (interaction between tumor and microbial community with general compartment tissue or organ system), and tertiary (interaction between tumor and remote microbial community). The main interaction is defined as the direct relationship between the tumor and the microflora in the local tumor microenvironment (44). Intestinal microbiota are not only directly related to local intestinal diseases such as inflammatory bowel disease and colorectal cancer (CRC) (45, 46), but also closely related to the pathogenesis of systemic diseases such as liver or nervous system diseases, which indicates the existence of an intestinal-liver axis and an intestinal-brain axis (47, 48). However, PCa does not have direct contact with the intestinal tract, and the role of intestinal microflora in it is still being explored. The possible factors of the intestinal microbiome in promoting the occurrence and development of PCa are related to the risk factors (obesity and inflammation) affected by the fecal microbiome and may directly cause distant effects in organs such as the prostate (25), Carcinogenesis can also be indirectly induced by metabolites of intestinal microorganisms (such as fatty acids and polyamines) in distal organs (49).
2.1 Indirect pathway of action
2.1.1 Metabolites
2.1.1.1 Short chain fatty acids/Growth factor-1
Short chain fatty acids (SCFA) are the main metabolites of the intestinal microbiota, including propionate, acetate, and butyrate, which play an important role in physiology (50). Intestinal microflora produces large amounts of SCFA from indigestible and fermentable carbohydrates, including dietary fiber (51). The change in intestinal structure changes the level of SCFA, which affects cell adhesion, cytokine production, chemotaxis, immune cell recruitment, and apoptosis (52).Intestinal microorganisms can regulate PCa through SCFA. It was found that Alistipes and Lachnospira producing SCFA were significantly increased in the intestinal microflora of patients with high-grade PCa, suggesting that SCFAs may be the promoter of PCa (28). Supplementation of SCFA increases systemic and local insulin-like IGF1 in the host prostate. IGF1 promotes tumor growth by activating local prostate MAPK and PI3K signal transduction, revealing the possible existence of the intestinal microbiome-IGF1- prostate axis (37). Antibiotics can reduce the SCFA in the feces of mice, and mice fed with a HFD can restore the level of serum IGF-1 and cancel the inhibition on the growth of PCa (53). IGF1 can also directly promote the proliferation of PCa cell lines DU145 and 22RV1 in vitro (37),The incidence of PCa and the risk of PCa-related mortality are also significantly increased in acromegaly patients with systemic high growth hormone and IGF-1 levels, indicating that IGF-1 plays a positive role in the development and progression of PCa (54).
2.1.1.2 Testosterone
Many disorders affecting male patients, including metabolic syndrome, PCa, and delayed hypogonadism syndrome, are heavily influenced by testosterone (55, 56). Intestinal microflora also plays a role in testosterone production. Makoto Matsushita et al. took rectal swab samples from 54 Japanese men with negative prostate biopsies and sequenced the 16S rRNA gene to analyze the intestinal microflora. It was discovered that the quantity of Firmicutes positively linked with the level of serum testosterone, and that the quantity of Firmicutes had no bearing on host variables (age, body mass index, triglycerides, and total cholesterol) (57).Another study found that there was a significant correlation between the number of Acinetobacter,Ruminococcus,Dorea, and Megamonas and serum testosterone levels in men, with high levels of estradiol having more Bacteroides and fewer Firmicutes than women with low levels of estradiol (58). Compared with HSPC patients, the number of Ruminococcus in the intestinal microflora of CRPC patients increased. In patients with CRPC, Ruminococcus is associated with a poor prognosis. Ruminococcus can convert pregnenolone and hydroxypregnenolone into downstream metabolites of this pathway, including dehydroepiandrosterone and testosterone (59). Ruminococcus can also promote the progression of PCa by up-regulating the expression of LPCAT1 and DNA repair proteins (60). Clostridium scindens ATCC 35704, Clonorchis sinensis, and Propionibacterium lymphoid are located in the intestinal microbiota. Their DesAB genes encode steroid 17,20-lyase, which can convert cortisol into C19 androgen by splitting side chains, which can be further metabolized by host tissue and the microbiome to form effective 11-oxygen-androgen, while DesAB-expressing microorganisms may be neglected sources of androgen in vivo and may lead to various disease states, such as PCa (12).
2.1.1.3 Estrogen
Estrogen may be involved in the occurrence and development of PCa (61), so intestinal microbes that cause high levels of estrogen in the body may make men vulnerable to PCa. Normal prostate stem cells express estrogen receptor-α (ER- α), estrogen receptor-β (ER- β) and G protein-coupled receptors. The activation of ER- α is considered to be the cause of PCa. ER- α participates in the transformation of epithelial cells into mesenchymal cells and is closely related to the occurrence and development of tumors. In the mouse model, the bone shape of osteoblasts is inhibited by the ER- α gene knockout model and the ER- α antagonist (62). Plottel and Blaser assume that the collection of genes from intestinal bacteria is a functional estrogenic genome whose products can metabolize estrogens. In particular, the intestinal microbiota contains β-glucuronidase and β-glucuronide. When the intestinal microbiota is maladjusted, it can promote the uncoupling and recycling of estrogen by secreting these two enzymes and bind to the estrogen receptor for the development of PCa. The activity of these two enzymes can help reduce the risk of PCa (24). By activating polycyclic aromatic hydrocarbons (PAHs), estrogen produces carcinogenic metabolites such as diol epoxides and free radical cations, which react with DNA to promote carcinogenesis. The functional estrogen genome of Plottel promotes this estrogenic mechanism to increase the rate of carcinogenesis (63). Therefore, we should pay attention to the increase of serum estrogen in patients with intestinal microbiota disorders and formulate a new treatment strategy.
2.1.1.4 Folic acid
The intestinal microflora is considered one of the sources of folic acid. They cannot be synthesized by the human body and must be absorbed through the intestines from diet or intestinal flora. Bifidobacterium and Lactobacillus, which produce folic acid, may be the supplementary endogenous sources of folic acid and increase the level of folic acid in the human body (64). Folic acid is closely related to PCa, and the expression of the folate receptor is increased in PCa (65). Folic acid plays a key role in the synthesis of nucleotides needed for tumor cell proliferation and DNA methylation. The change in prostate methylation pattern is an important event in PCa (66). Folic acid is a transmembrane carboxypeptidase with hydrolase activity that can decompose prostate specific membrane antigen, which is overexpressed in almost all PCa, and the tissue level of this protein is positively correlated with a higher grade, a higher Gleason score, and disease recurrence (67, 68). Unmetabolized folic acid is associated with reduced cytotoxicity of natural killer cells, which may protect the clearance of malignant cells (65). When Liss et al. compared PCa patients with healthy people, they found that the number of bacteria related to carbohydrate production increased in prostate patients, while the number of bacteria related to folate, biotin, and riboflavin decreased (25). In a controlled randomized trial of 643 men, Figueiredo et al. found that the estimated probability of being diagnosed with PCa within 10 years was 9.7% in those with high folic acid levels and 3.3% in the placebo group. Men who randomly took folic acid supplements were 2.6 times more likely to be diagnosed with PCa than those in the placebo group (69).
2.1.1.5 Phenylacetylglutamine
Phenylacetylglutamine (PAGln) is a phenylalanine metabolite produced by the metabolism of intestinal microflora, which activates α-adrenergic and β-adrenergic receptors through adrenergic receptors (70). Reichard included baseline serum samples from 173 patients with lethal PCa and 519 patients with non-fatal PCa into a case-control design and found that adrenergic compounds produced by metabolism mediated by intestinal microflora were associated with an increased risk of fatal PCa, while β-adrenergic blockade may be another target for reduced risk of PCa (71).
These studies have shown that specific intestinal flora plays a crucial role in the progression of PCa indirectly through its metabolites (short-chain fatty acids, testosterone, estrogen, folic acid, and PAGln), and the metabolites of intestinal flora indirectly affect the occurrence and development of PCa. As for how to play a role in the prevention and treatment of PCa by influencing the production of metabolites, its carcinogenic mechanism and action pathway are worthy of further exploration.
2.1.2 Immunization
The host’s immune system can affect the microbial ecosystem and have an impact on the fecal metabolic content, even if the external environment has a significant role in developing the gut microbiome (72). The following are the effects of intestinal microbiota on tumor immune response: activation of regulatory T cell proliferation and differentiation; induction of IgA expression; and the influence of antimicrobial peptide expression, microbial metabolism, systemic inflammation regulation, and bacterial translocation (73). The intestinal mucosal immune system is made up of gut epithelial lymphocytes, lamina propria lymphocytes, collecting lymph nodes, and other components. The bulk of intestinal epithelial lymphocytes are CD3+ T cells, while B cells and NK cells are in the minority. T and B cells make up the vast majority of lymphocytes in the lamina propria. and regulatory T cells are characterized by the expression of CD4, CD25, and Foxp3. Additionally, the generation of the anti-inflammatory cytokines transforming growth factor-β (TGF-β) and IL-10, which reduce intestinal inflammation (74). There are four components to the defensive mechanism between the intestinal immune system and the mucosa: 1) The microbial barrier is the first element; the microbiome is found in the mucous layer’s upper layer. These symbiotic bacteria have the ability to inhibit the colonization of pathogens, create metabolites or immune signaling-regulating components, and support immunological homeostasis (75–77); 2) The mucus-based chemical firewall that covers the gut epithelium is the second firewall. Through the production of mucus by goblet cells in the epithelium, the release of antimicrobial peptides by the epithelium, and the production of mucosal IgA by dendritic cells (DCs) in the gut, mucus regulates contact between symbiotic bacteria and epithelial cells and protects the epithelium from symbiosis (76); 3) The single-celled epithelial cell layer, which serves as a physical barrier, makes up the third element. In addition to helping with food absorption, the intestinal epithelium serves as a physical barrier against disease invasion and the movement of symbiotic microbes outside the gut (78); 4)The final component is the immune barrier, which contains specialized immune cells (macrophages, DCs, and lymphocytes). Macrophages and DCs are DCs distributed in the lamina propria and mesenteric lymph nodes. Using the intestinal lumen as a source, DCs are efficient antigen-presenting cells that deliver microbial antigens to T lymphocytes in mucosal tissue. Leads to T cell subset development and activation (Th1, Th2, Th17, or Treg), which, along with macrophages, serves as a “bridge” between innate and acquired immune responses (79, 80).The intestinal mucosal immune system not only produces immune tolerance to symbiotic bacteria and food antigens but also produces a strong immune response to pathogenic bacteria and maintains a balanced response to infection (36). By controlling innate and adaptive immune responses, intestinal flora can keep the gut environment balanced. However, specific alterations in the intestinal microflora’s makeup may stimulate the mucosal immune system, cause chronic inflammation and mucosal damage, and encourage an intestinal mucosal immunological imbalance, which in turn promotes the growth of tumors.
2.1.2.1 Innate immunity
The cells of the intestinal flora that regulate innate immunity include monocyte/macrophage, DC, granulocytes, NK cells, and NKT cells. Pathogen-associated molecular patterns(PAMPs) is a conservative structure of pathogens, such as fat polysaccharide, peptidoglycan, lipoprotein, nucleic acid, and so on, with corresponding PAMPs pattern recognition receptors (PRRs), such as toll-like receptors (TLRs) and NoD-like receptors (NLRs), identify conserved structures of microorganisms to obtain innate immune cells and epithelial cells (81), and turn on particular signalling pathways that encourage inflammation, tumour growth, or combat cell death. The stimulation of NF-B signalling and the development of bacterial communities are both crucially dependent on NOD2. Mutations that cause NOD2 malfunction may result in an alteration of intestinal flora and a higher risk of CRC. And NoD1-mediated recognition of the MesoDAP peptidoglycan component in Gram-negative bacteria in the gut microflora has been shown to initiate neutrophils to kill bacterial pathogens (82). The control of intestinal homeostasis is greatly influenced by intestinal TLRs. Pro-inflammatory cytokines, including interleukin and tumour necrosis factor α, are produced when TLRs are activated. Through several growth factor receptor ligands (amphiregulin and hepatocyte growth factor), TLRs may potentially encourage the proliferation of cancer cells to have both local and distant impacts (83). In addition, the intestinal microbiome appears to act on intestinal DC directly or indirectly through epithelial cells, affecting the phenotype and activity of DC (34,102). This stable DC phenotype promotes non-inflammatory responses such as FoxP3+Treg induction and IgA secretion, allowing the host and intestinal mucosal microorganisms to maintain homeostasis (84, 85). IL-10 produced by enteric macrophages inhibits intestinal inflammation by maintaining FOXP3 expression in Treg cells and inhibits IL-12 and tumor necrosis factor-production in enteric myeloid cells by activating the transcription factor STAT3. Thus, mice with IL-10 deficiency and specific STAT3 mutations in myeloid cells (LysM-cre;Stat3flox/flox mice) spontaneously produce intestinal inflammation (86). In prostatitis, it is characterized by the infiltration of macrophages, neutrophils, and lymphocytes, which release reactive oxygen species, reactive nitrogen, and proinflammatory cytokines, leading to DNA damage, cell damage, and cell death. Persistent chronic inflammation leads to proliferative inflammatory atrophy. Prostatic intraepithelial neoplasia and PCa are the ultimate results of chronic inflammation (87).
2.1.2.2 Adaptive immunity
Both innate and adaptive immune responses are influenced by the symbiotic flora of the immune system. For example, mice without bacteria have an undeveloped adaptive immune system (88). Differentiation of Peyer’s patches(PPS), CD4+ T cells in the lamina propria, IgA-producing B cells, and intestinal epithelial lymphocytes have been associated with intestinal adaptive immunity induced by the intestinal microbiome. It is necessary to maintain the stability of the intestinal environment, the integrity of the intestinal barrier, and immune tolerance to symbiotic bacteria (89). Certain metabolites are essential for controlling the activity of adaptive immune cells, particularly CD4+ T and B lymphocytes. Additionally, in the gut and other distant organs, microbial imbalance makes the host more vulnerable to a variety of immunological, inflammatory, and allergy illnesses (90, 91). For instance, the microbiota promotes the development of cancer, which is directly influenced by gut Th1 and Th17 cells. Th1/Th17 balance has been shown to be associated with prognosis in patients with CRC, and one study found that Th17 cells produced a higher proportion of Th1 and Th17 cells as well as the cytokines IL-17A, IL-22, and IL-23A when fed stool from patients with CRC in regular mice (92, 93). In PCa tissues, the abundance of microbiome is highly correlated with the expression of Treg. These cells inhibit the activation and proliferation of effector T cells and ultimately inhibit the host immune response (94). Inflammation and carcinogenesis may trigger barrier failure, but barrier failure also promotes inflammation and cancer, creating a feedback loop (83), in which the intestinal barrier’s breakdown results in bacterial translocation and the emergence of a systemic inflammatory response (95). Several bacteria induce immunity during tumor development. Cytotoxic immune cells (cytotoxic T lymphocytes) are required for Bifidobacterium, Bacteroides thetaiotaomicron, and Bacteroides fragilis to enhance antitumor cytotoxic T cell immunity. Fusobacterium nucleatum promotes tumor growth by inhibiting T cell activity, which is associated with survival and antitumor therapeutic efficacy (96). Terrisse recently conducted a prospective study that included a mouse model of PCa and fecal and blood samples from 65 patients with HSPC and CRPC. It was found that ADT increased thymocyte count and output in normal mouse models. The response of PCa and ADT implantation in mice with T lymphocyte loss or thymus loss was lower than that in normal mice. Oral antibiotics destroyed the diversity of intestinal flora and decreased the efficacy of ADT. PCa also reduced the relative abundance of Akkermansia muciniphila (A.muciniphipla) in the intestine, which could be reversed by ADT. In addition, compared with the HSPC control group, the intestinal microflora of CRPC patients had a significant correlation with the abundance of thymic transitional cells, indicating a functional relationship between the intestinal ecosystem and the thymus (97).
2.1.2.3 Regulation of the immune response
In specific cases, cytokines regulate cell growth differentiation and effects by binding to corresponding receptors, regulate immune responses, and play a role in the development of many diseases, including inflammation. For example, TGF can be used by Staphylococcus aureus and Streptococcus Group A to promote the growth and spread of tumour cells (98–100). Intestinal microflora can establish a pro-inflammatory or anti-tumor environment by regulating the function of host physiological and immune cells (101), and its changes can affect the inflammation of distal organs (102, 103). Interleukin B, TGF-β, vascular endothelial growth factor, tumor necrosis factor, etc. are released in response to intestinal dysregulation. Both growth factors and cytokines cause inflammation, which impairs differentiation by preserving the presence and expansion of undesirable cells (104). If inflammation continues, cancer will develop as a result of the ongoing spread of inflammatory signals, inhibited apoptosis, and elevated amounts of growth factors (105). Verrucommicrobi, to which A.muciniphipla belongs, promoted the inflammation of intestinal microflora in mice, and its abundance increased with the increase of chemokines and cytokines in mucositis induced by 5-fluorouracil. Extracellular vesicles from A.muciniphipla can increase the number of M1-like macrophages and secrete more inflammatory cytokines (10). Poutahidis et al. found that Helicobacter hepaticus was found in the intestinal flora of ApcMin/+ mice, which significantly increased the incidence of prostatic intraepithelial neoplasia and PCa (106). Helicobacter hepaticus infection can cause systemic elevation of pro-inflammatory cytokines (eosinophil chemokine, IL-3, tumor necrosis factor-α and IL-1α) and enhance prostatic intraepithelial neoplasia and microinvasive carcinoma (10).
Gut bacteria have a direct influence on immune response, and changes in the composition and number of microbiota may influence local immune response. We now know that changes in the types of bacteria in the gut can also lead to immune changes in distant organs that can cause cancer.
2.1.3 Genetic phenotype
In the past, the interaction between intestinal flora and host function was not recognized. The number of microbial cells carried by the human body is 10 times that of the total number of cells in the human body, and the genetic information is 150 times that of the human genome. Therefore, there are many complex interactions between microbes and their hosts (107). The metabolic activity of intestinal microbiota is very important to maintain the homogeneity and health of the host. Although the existence of microbiota is very important, changes in its composition lead to metabolic changes, which may lead to changes in the host phenotype. Lynch et al. proposed the “common ground” hypothesis: genetically susceptible hosts disrupt intestinal microbiota due to environmental factors such as diet or chronic infection, resulting in polygenetic changes that result in host diseases such as cancer (108). It has been found that the gene toxin Colibactin can be secreted by Escherichia coli, which induces the appearance of senescent cells and promotes tumor growth by secreting growth factors (109). In addition, when FMT containing CRPC feces was injected into mice, it was found that the expression of DNA-PKcs, RAD51, and LPCAT1 in the prostate tissue of mice was positively correlated with the malignant degree of PCa (60). Sphingosine 1-phosphate receptor 2 (S1PR2) is a gene mainly regulated by a HFD, which is associated with abundantbody mass index, Lactobacillus, a low BMI and aggressive characteristics in patients with PCa (110). Additionally, S1PR2 expression is seen in host endothelial cells and tumor-infiltrating bone marrow cells, where it suppresses the expression of vascular endothelial growth factor and matrix metalloproteinase-9 activity to prevent tumor angiogenesis and tumor growth (111). HDF can promote the growth of Lactobacillus, reduce the number of Bacteroides, and change the composition of intestinal microorganisms, which is closely related to the progression of obesity (112). Obesity caused by HFD can lead to chronic systemic inflammation, activate signal pathways, and promote the progression of PCa through immune system-related mechanisms, including activating a series of chemical signaling pathways such as IL6/pSTAT3 or MCP-1/CCR2, inhibiting the tumor suppressor gene PTEN to induce the growth of PCa (113), and inducing local IL-6 upregulation in immune cells and MDSC, enhanced MYC transcriptional programming through metabolic changes increases histone hypomethylation in the promoter region of the MYC regulatory gene (114). HFD can also increase the number of Lgr5+ intestinal stem cells and promote tumor formation by activating and enhancing the peroxisome proliferator (PPAR-d) signal, making intestinal stem cells lose the tumor suppressor APC (115). Inflammation is a risk factor for tumorigenesis and progression. Inflammation may trigger cell repair, angiogenesis, and tissue repair cascades to a greater extent by affecting cell and genome damage, thus promoting tumor occurrence and progression (116). In addition, inflammation may promote immune cells to release reactive oxygen species and reactive nitrogen, which directly damage cells and DNA, resulting in proliferative inflammatory atrophy, which is considered to be the pathogenic factor of PCa and other cancers (63). Inflammation and HDF are closely related to the change of gene phenotype. The interaction between them and intestinal flora changes the composition of intestinal flora and thus changes the metabolic pathway and absorption, which may result in different responses to PCa.
2.2 Direct pathway of action
2.2.1 Bacterial translocation
The process of intestinal microorganisms and their metabolites transferring to mesenteric lymph nodes or portal veins through the intestinal mucosal barrier and entering other organs is called “bacterial translocation” (117). The risk factors include the disorder of intestinal flora (118), the increase in intestinal permeability (119, 120), and the deficiency of immune function (121, 122). Intestinal microbial translocation has been proven to play an important role in the pathogenesis of many diseases, such as pancreatitis (123), and liver cirrhosis (124).
The decrease in the diversity of intestinal microflora, the disorder of flora structure, and the quantitative changes in intestinal metabolites during PCa will eventually lead to intestinal microbial imbalance. Intestinal microbial disorders caused by various factors will damage the integrity of the intestinal wall, and increasing intestinal permeability will lead to intestinal metabolites or bacterial components that can cause disease in the systemic circulation (125, 126). We call this process “bacterial passive translocation.” A frequent indicator of intestinal health is intestinal permeability, bacteria may breach the epithelial barrier as a result of increased intestinal permeability, causing inflammation. Studies have shown that biological involvement in prostate cancer is complex, but it has been determined that epithelial barrier breakage is related to inflammatory changes in the prostate microenvironment and prostate infection, which promotes the development of prostate cancer (127). There is growing evidence that intestinal microflora may play a role in the progression of PCa by affecting intestinal permeability (128, 129). Existing studies have found that when intestinal permeability increases, the passive translocation of bacteria may occur in two main pathways: the paracellular pathway controlled by tight junctions or the specific intestinal epithelial cell pathway and the transcellular pathway controlled by the membrane pump (130). There are a variety of microorganisms that can produce cytotoxins that change the structure of intestinal epithelial cells and lead to passive translocation. Enterohemorrhagic E. coli, Salmonella typhimurium, Clostridium perfringens, Bacteroides fragilis, Vibrio cholerae, and rotaviruses can release virulence to attack intestinal epithelial cells, causing tight junction proteins to destroy and cross the membrane (131, 132). Enteropathogenic E. coli, Helicobacter pylori, Clostridium difficile, and Pseudomonas aeruginosa release toxins to increase the permeability of intestinal epithelial cells without changing the structure of tight junctions (133–136). Shigella flexneri, Listeria monocytogenes, and Clostridium botulinum can break the epithelial cell barrier by modifying the actin cytoskeleton (137, 138).
Studies have found that HFD leads to an increase in intestinal permeability, but subsequent antibiotic treatment will further reduce intestinal permeability (139). The destruction of intestinal microflora by metronidazole and other antibiotics led to an increase in intestinal inflammatory tension, an increase in bacterial stimulation of epithelial cells, a change in goblet cell function, and the thinning of the internal mucus layer, indicating the weakening of mucosal barrier function and the increase in intestinal permeability (140). In one mouse model, treatment with penicillin or metronidazole increased the number of Enterobacter in the cecum by an average of 1000 times, while treatment with clindamycin increased the number of Enterobacter by 100000 times. The average incidence of translocation to mesenteric lymph nodes was 100% after penicillin treatment, 97% after clindamycin treatment, and 62% after nail-file treatment (118). Steffen et al. found that the level of a specific cecal population may be the main factor promoting the translocation of bacteria from the intestine. In the mouse model, the number of E. coli, P. mirabilis, and Klebsiella pneumoniae in the cecum was significantly correlated with the number of these bacteria transferred to the mesenteric lymph node complex (141). In diseases such as trauma, shock, and heat injury, anoxic injury and ischemia or reperfusion of intestinal epithelial cells greatly disrupt the cytoskeleton, resulting in increased permeability of intestinal epithelial cells and bacterial translocation (142, 143). Immune deficiency is an important factor leading to intestinal microbial translocation. The incidence of intestinal bacterial translocation is 50% in non-thymic mice and only 7.8% in transplanted thymic mice (121). In a normal intestinal environment, intestinal mucus secretes immunoglobulin A (sIgA) to prevent bacteria from adhering to the surface of intestinal epithelial cells, cooperate with complement and lysozyme, and encapsulate invasive viruses, which is an important line of defense to maintain the intestinal immune barrier (144). When immune function deficiency occurs, it leads to a decrease in intestinal mucus sIgA, which greatly promotes the passive translocation of bacteria (145).
When bacteria invade extra-intestinal organs through the intestinal barrier without evidence of a pathological environment or trauma, “active bacterial translocation” occurs. The three basic steps of bacterial active translocation are adhesion, invasion, and actual movement through the intestinal barrier (146). When intestinal epithelial cells are intact, Cossart et al.’s mechanisms for internalizing bacteria into non-phagocytic cells such as intestinal epithelial cells are divided into three categories: 1) Some microbial pathogens usually express a surface protein that binds to eukaryotic receptors and participates in cell matrix or cell adhesion and expression, leading to vacuole formation, actin cytoskeleton rearrangement, and membrane extension receptor binding. Trigger the “zipper mechanism” to phagocytize the pathogen. 2) Other microbial pathogens can express a binding protein that acts as a bridge between bacteria and receptors, and thus transmembrane receptors mediate entry into the cell; 3) Other microbial pathogens do not play a role through the process of adhesion; their secretory systems inject special effectors into cells, directly regulate actin cytoskeleton rearrangement, and eventually form a large phagocytic bag inside the cell (147). In cells, microbial pathogens polymerize actin to provide energy, which makes bacteria move to the plasma membrane and form protuberances and finally form vacuoles through phagocytosis of adjacent cells (147).
After passing through the intestinal barrier, microbial pathogens may enter the systemic circulation mainly through two pathways: through the intestinal venous system into the portal vein or through intestinal lymphoid drainage (130). In pancreatitis, the pathway of bacterial translocation may be hematogenous spread, directly transferred to the peritoneal cavity or retroperitoneal and then to the pancreas, or secondary to the lymph near the pancreas (148, 149). A retrospective study found that bacterial translocation was involved in the biochemical recurrence of PCa. They found that plasma bacterial 16S rDNA levels increased in patients with PCa biochemical recurrence, and the grade of PCA patients was positively correlated with plasma 16S rDNA levels (150). Whether there is a bacterial translocation similar to the pancreatitis pathway in the prostate may be a focus of microbial research in the future.
2.2.2 Toxin translocation
Bacteria themselves do not need to cross the intestinal epithelial barrier to cause disease, but the infiltration and translocation of inflammatory or toxic substances produced by intestinal microorganisms may also lead to body damage, called “toxin translocation” (151). Outer membrane vesicles are regarded as “remote weapons” of bacteria, which can promote the interaction between bacteria and bacteria, bacteria and hosts, and transport bacterial toxins or other virulence factors to host cells to cause disease, making a great contribution to toxin translocation (152). Some studies have proposed the intestinal lymphoid hypothesis, which states that toxic mediators are released from the intestines after intestinal injury and are transported through mesenteric lymph nodes to cause disease, while another study supports this speculation. After blocking mesenteric lymph nodes, the distal organs of mice can be prevented from being damaged by toxin translocation (153, 154).
Toxin translocation most often leads to sepsis and multiple organ dysfunction syndrome (155, 156), but its role in tumors is still being explored. The intestinal tract is the largest pool of endotoxin in the human body. The level of endotoxin in the blood can reflect the damage to the intestinal mucosal barrier and the level of bacterial translocation. The intestine usually absorbs a small amount of endotoxin, but when endotoxin enters the liver through the hepatic portal vein, the liver produces Kupffer cells to clear the endotoxin. When the intestinal microflora is in disorder, it may induce the immune and inflammatory reactions of the intestinal mucosa and eventually lead to an increase in intestinal mucosal permeability and endotoxin translocation. With the progress of the disease, when the level of portal vein endotoxin is too high, it can stimulate liver Kupffer cells to release a series of cytokines, such as tumor necrosis factor, interleukin-1, interleukin-6, free radicals, and so on (157). Dietary factors such as fat, fructose, and alcohol can change intestinal flora and intestinal permeability and make enterogenic toxins pass through the intestinal barrier to activate hepatocytes and overproduce inflammatory cytokines. The pathogenesis is that NF-κB activates B cells, which leads to systemic inflammation and body injury (158, 159). When the intestinal microflora is destroyed or out of balance, the intestinal barrier function may be impaired, thus increasing permeability and thus increasing the chances of leakage of intestinal fluid, macromolecules, white blood cells, toxins, and compounds into the circulation, which may also lead to inflammation (160). The decrease in the diversity of intestinal microorganisms leads to the overgrowth of bacteria and leads to mild systemic inflammation, called endotoxemia, which leads to an overall inflammatory state in many organs and promotes tumor formation (25).
2.3 Pharmacological effects
2.3.1 Chemotherapy and immunotherapy
Through a number of processes, such as immunological interaction, diverse metabolism, and altered community structure, intestinal microflora can control the host’s reaction to chemotherapy. The pharmacological effects of some chemotherapeutic drugs and immunotherapy are closely related to intestinal bacteria, such as 5-fluorouracil, cyclophosphamide, irinotecan, oxaliplatin, gemcitabine, methotrexate, anti-PD-L1 therapy, and anti-CLTA-4 therapy (161). James Alexander et al. proposed a framework for how intestinal microflora affect the pharmacological effects of these drugs through important mechanisms: “TIMER,” which represents translocation, immunomodulation, metabolism, enzyme degradation, reduction of diversity, and ecological variation (162).
Immunotherapy is an essential part of the treatment of PCa. Emerging evidence suggests that the gut microbiome influences the response to anticancer treatments by modulating the host immune system. Martina Di Modica et al. studied the role of intestinal flora in the anti-tumor efficacy of immune-mediated trastuzumab and found that intestinal microflora was directly involved in the efficacy of trastuzumab. They concluded that controlling intestinal microflora was the best strategy to improve the efficacy of tumors in the future (163). Ayelet Sivan et al. believed that manipulating the microbiome might regulate cancer immunotherapy. They found that oral bifidobacterium controlled tumors better, and the PD-L1 blocking effect played a better role in mice with a higher abundance of bifidobacterium (164). Other studies have shown that Mycoplasma hyorhinis can metabolize gemcitabine into inactive metabolites, reducing its anti- PCa efficacy (165). Drug resistance is closely related to abnormal intestinal microflora and antibiotic therapy in patients with advanced PCa during immunotherapy (166). Liu et al. found that Docetaxel (DTX) reduced the diversity of the intestinal microbiota. In addition, intestinal diversity decreased and tumor growth accelerated in mice pretreated with the antibiotic mixture (167). Zhong et al. established a mouse model of intestinal flora imbalance and collected fecal samples from mice for 16S rRNA sequencing. It was found that intestinal microflora disorder after antibiotic exposure led to a significant increase in the number of Proteobacteria, intestinal permeability, and lipopolysaccharide in tumors, which promoted the progression of PCa and docetaxel resistance through NF- κ B-IL6-STAT3 axis (168). Marie Vétizou et al. revealed that intestinal flora played a key role in CTLA-4-blocked immunostimulation. They built aseptic mice and mice pretreated with antibiotics and found that they were less effective in anti-CTLA-4 therapy, after the fecal microorganism transplantation of patients containing Bacillus fragilis, the effect of anti-CTLA-4 treatment in mice was significantly improved (169).
The diversity and structure of the intestinal microbiota are affected by chemotherapeutic drugs, which in turn affect the efficacy and gastrointestinal toxicity of chemotherapy. Cyclophosphamide is an important anti- PCa drug, and recent studies have shown that its therapeutic efficacy also depends on intestinal microflora. Xu et al. found that cyclophosphamide decreased the proportion of Bacteroidetes and increased the proportion of Firmictutes in mice (170). Viaud et al. hypothesized that intestinal microflora played a part in shaping the anti-cancer immune response and found that part of the efficacy of cyclophosphamide was due to their ability to change the composition of intestinal microflora and then induce bacterial translocation to stimulate the anti-tumor immune response caused by lymphatic organ infiltration (171). By sequencing the 16S rRNA gene of 28 male stool samples, Montassier et al. found that, compared with the samples collected before chemotherapy, the abundance of fecal samples collected after chemotherapy decreased significantly, containing a small amount of Firmicutes and Actinobacteria, while the number of Proteobacteria increased significantly (172). By establishing a mouse model, Lida et al. found that intestinal microflora regulates the function of myelogenous cells and immune cell response to promote the efficacy of chemotherapeutic drugs, while the disorder of intestinal microflora caused by the introduction of antibiotics reduces the effect of chemotherapeutic drugs, indicating that complete intestinal microflora is needed to regulate immune cell response in the tumor microenvironment (161). Romain et al. established an aseptic cancer mouse model that showed reduced Th17 response and resistance to cyclophosphamide, while the anticancer effect of cyclophosphamide could be restored by oral administration of Gram-positive Enterobacter (173).
2.3.2 Anti androgenic therapy
Androgen deprivation therapy (ADT) is the basis for the treatment of advanced PCa. Intestinal microbes also affect the efficacy of ADT. A recent study found that symbiotic intestinal microflora play a role in endocrine resistance to CRPC by providing another source of androgen, Pernigoni et al. found that Rumencocci played a key role in promoting the progression of CRPC. They constructed a PCa model mouse that received castration treatment, and the abundance of Rumencocci was significantly increased. After the intestinal flora of mice was removed with antibiotics, the efficacy of ADT was increased, and the progress of CRPC in ovariectomized mice was inhibited. On the other hand, they transplanted intestinal microbes from CRPC patients into PCa mice, which also promoted their PCa progress (59). Liu et al. confirmed that, compared with the intestinal microflora of HSPC patients, the number of rumen cocci in CRPC patients increased significantly (32). Sfanos et al. hypothesized that oral androgen receptor axis-targeted therapy (ATT) may be related to differences in the composition of gastrointestinal microflora. They conducted a cross-sectional study of 30 healthy patients and PCa patients. It was found that the α-diversity of gastrointestinal microbiota was greater in healthy men, and there was a measurable difference in the bacterial composition of the gastrointestinal microbiota in men treated with ATT. The results showed that the abundance of A.muciniphipla and Ruminococcaceae spp. was higher, which may mean that the changes in gastrointestinal microbiota caused by ATT represent the mechanism of a potential alternative pathway of steroid metabolite production, which in turn affects the treatment and prognosis of tumors (27). Cimadamore et al. showed that both ruminococcus and A.muciniphipla are involved in steroid biosynthesis, and the relative abundance of A.muciniphipla and ruminococcus is higher in PCa patients taking ATT, which they believe is more beneficial to the efficacy of anti-programmed death-1 (PD-1) immunotherapy, while the use of antibiotics in patients with ruminococcus will increase the risk of progressive PCa (174). Androgen inhibitors are generally used in the treatment of advanced PCa; androgen inhibitors such as abirone acetate(AA), which is poorly absorbed and will stay in the intestine for a long time, and other drugs may produce heterometabolism and change the microflora, thus affecting the efficacy and activity of the drug (27). Daisley et al. found that the biotransformation of AA is affected by A.muciniphipla. Oral AA can repeatedly regulate the gastrointestinal microflora associated with patients by promoting the growth of A.muciniphipla, while the efficacy of AA is achieved by interacting with A.muciniphipla to increase the ability of intestinal microorganisms to synthesize vitamin K2 in PCa patients (175).
Therefore, the further research and development of intestinal microflora may become an important part of personalized and targeted anti-PCa therapy. (Figure 1) summarizes the pathway of the gut microbiome in prostate cancer development.
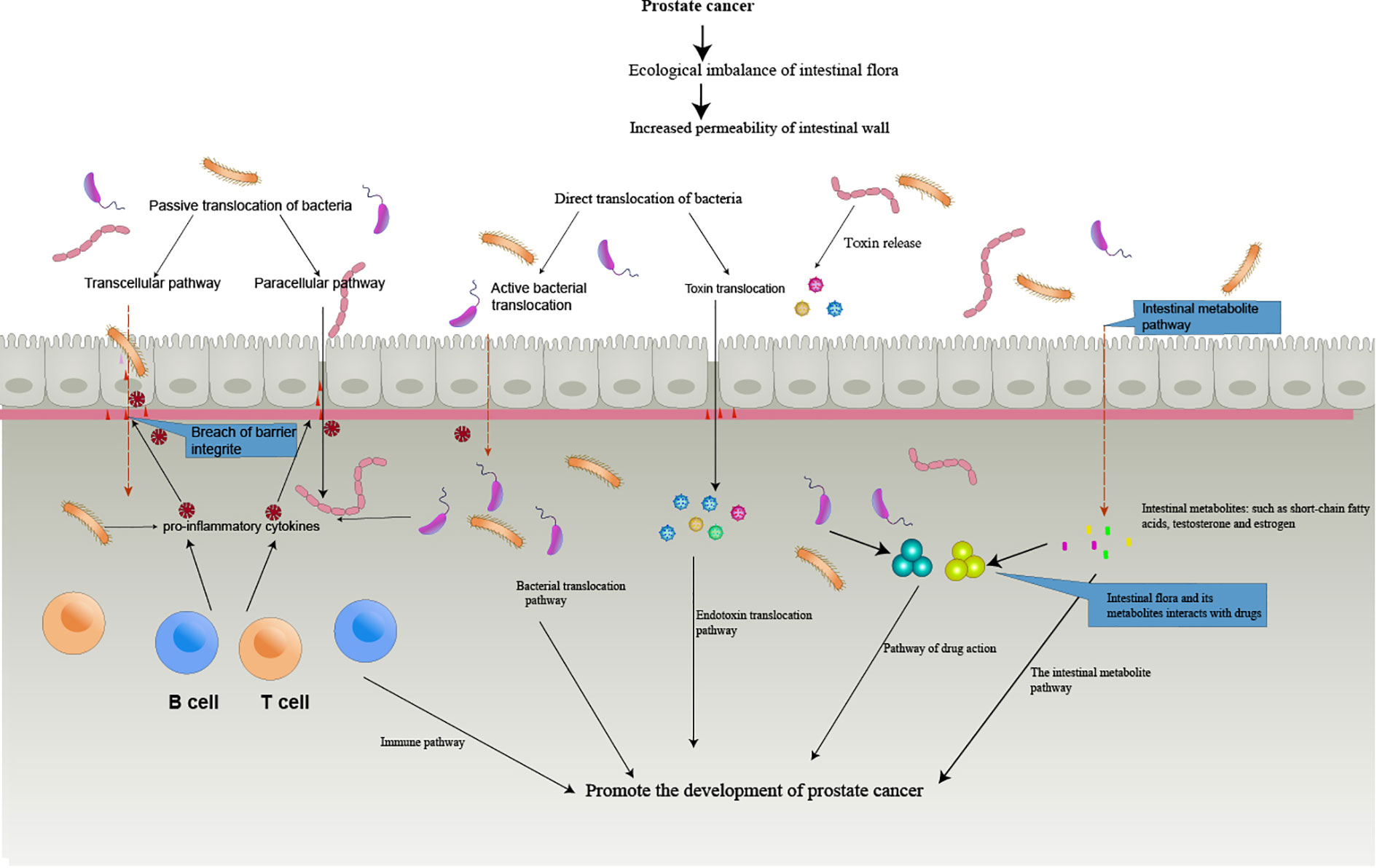
Figure 1 The Intestinal bacterial translocation and toxin translocation. The imbalance of intestinal flora leads to the destruction of the defense mechanism between the intestinal mucosa and the intestinal immune system, and the release of various inflammatory and cytokines destroys the epithelial cell barrier, leading to the active and passive translocation of bacteria, the translocation of toxins, the translocation of bacterial metabolites, and interactions with drugs that eventually enter the circulation, thus promoting the formation of tumors.
3 Treatment
3.1 Gut microbiota can be used as a diagnostic tool for PCa
There are more than 1000 species of bacteria in the human intestinal tract, but only 150 to 170 species of bacteria are common to individuals (176). Intestinal microbiota helps to reveal the relationship between complex human diseases, and different diseases may have common and unique characteristics of intestinal microflora (177). Intestinal microflora has been proven to be a tool for non-invasive diagnosis and screening of a variety of diseases, such as liver cancer (178), CRC (179), and gastric cancer (180). In the intestinal microbiota, Firmicutes and Bacteroidetes accounted for the vast majority, while Proteobacteria, Actinobacteria, Verrucomicrobia, and the candidate TM7 phylum were few (181). However, the increase of Proteobacteria in the intestinal tract may be a sign of ecological imbalance, and the long-term accumulation of Proteobacteria in the intestinal tract is another manifestation of the imbalance of microbial community homeostasis or that the host is in a state of disease (182). A recent study found that Proteobacteria may be used as a marker of intestinal microbes in metastatic PCa. The fecal samples of 35 patients with PCa were sequenced by 16S rRNA, and it was found that the abundance of Proteobacteria was significantly increased, which was positively correlated with many parameters such as distant metastasis. The relative abundance of Proteobacteria was better than the level of prostate-specific antigen in the evaluation of distant metastasis probability of PCa in ROC curve analysis (168). Fruge et al.’s study included 40 overweight men who underwent a radical prostatectomy. Through the microbiological analysis of the 16S rRNA gene in their fecal samples, it was found that the total score of Gleason was positively correlated with Deferribacteres, Proteobacteria, and Clostridium, and negatively correlated with Blautia (183). Matsushita et al. compared the intestinal microbiota of 152 patients with high-grade PCa with that of healthy Japanese men. It was found that 18 kinds of intestinal microorganisms can accurately detect men with high-risk PCa, and their accuracy is higher than the level of prostate-specific antigen, including Rikenellaceae, Alistipes, Lachnospira, Subdoligranulum, Lachnobacterium, and Christensenellaceae, which are significantly increased in high-risk PCa (28).Intestinal microflora, especially Proteobacteria, may be a new means of detecting and preventing high-level and metastatic PCa, which is essential for PCa, and more research is needed to explore this aspect.
3.2 Metabolites of gut microbiota contribute to the treatment of PCa
3.2.1 Polyphenols
The effects of dietary and nutritional factors on the occurrence and development of PCa have received more and more attention, such as fat and polyphenols (184). The most abundant ingredient in green tea is polyphenols. Intestinal microflora can biotransform dietary polyphenols and improve their bioavailability. Dietary polyphenols can regulate the composition and function of intestinal microorganisms by inhibiting the proliferation of pathogenic bacteria and stimulating beneficial bacteria (16). The proliferation and survival of PCa cells are coordinated by multiple signal pathways (185).Disruption of androgen receptor and PI3K/Akt signal pathways plays an important role in the development of PCa (186, 187), while intestinal microflora can metabolize the main polyphenol epigallocatechin-3-gallate in green tea, reducing the risk of PCa by reducing the influence of androgen receptor (188, 189) and PI3K/Akt signal pathways (190, 191). The relationship between PCa risk and green tea drinking was studied involving 49,920 Japanese men. People who consumed five or more cups of green tea each day had a decreased probability of developing advanced PCa compared to those who drank less than one cup each day (192). In a meta-analysis of nine case-control studies, the risk of PCa was significantly reduced by 57 percent compared with those with the highest green tea intake. Similar results were observed in a recent meta-analysis of 3 case-control and 4 cohort studies, in which the probability of the highest and lowest PCa intake of green tea was significantly reduced by 55% (193). The Ellagitannins (ETs) extracted from pomegranate juice are also a kind of bioactive polyphenol with a chemoprevention effect on PCa. ETs is not completely absorbed in the gastrointestinal tract but is hydrolyzed into different metabolites in the intestine, including ellagic acid, which can be converted into urea A by intestinal microorganisms (194, 195). Several studies have shown that ellagic acid and its microbial metabolite, urea A, can inhibit the growth of PCa cells (196, 197). Cytochrome P1B1 (CYP1B1) is an established target for the chemoprevention of PCa. Too many genotoxic compounds produced by CYP1B1 overexpression might damage normal cells’ DNA and hasten the onset of cancer. Although increased CYP1B1 expression does not result in tumor invasion or metastasis, it can cause anti-PCa medications like flutamide to lose their effectiveness (198). ETs and their microbial metabolites can inhibit CYP1B1 enzyme activity and expression inhibitors, reducing the occurrence and maintenance of PCa (199), causing cell cycle arrest in the G1 phase, and inhibiting whole cell growth (200). All in all, polyphenol-rich diets or compound polyphenol supplements can increase colon metabolites, which in turn contribute to the chemoprevention of PCa (7).
3.2.2 Intestinal lignans
Intestinal flora in the upper large intestine can convert most plant lignans in human food into enterolactone and intestinal diol, called intestinal lignans (EL). Increasing EL intake and enterolactone exposure can reduce the risk of PCa (201). In animal experiments, EL can activate estrogen signal transduction in mice. The ventral prostate is rich in estrogen receptor β. The activation of the estrogen receptor β reduces the epithelial dysplasia of the prostate in mice, which is negatively related to the risk of PCa (202).
3.2.3 Isoflavones
Soybean products usually contain isoflavones, including daidzein and genistein. A number of studies have proven that intestinal flora are involved in the metabolism and biological activity of isoflavones, including a Clostri-diumsp, Eubacterium ramulus, Escherichia coli, Bacteroides ovatus, Ruminococcus productus, and Streptococcus intermedius (203). Daidzein can induce PCa cell cycle arrest in the G0/G1 phase by affecting the gene expression of cyclin and cyclin-dependent protein kinase (204). Hisae Nakamura provides some experimental data to support the inhibitory effect of isoflavones on the metastasis of prostate tumor cells and suggests that these inhibitory effects may be mediated by ER-β signal transduction (205). A large-scale epidemiological survey of 82483 Hawaiian and Los Angeles men showed that men with the highest legume intake had an 11.1% lower risk of PCa and a 26% lower risk of non-localized or high PCa than those with the lowest legume intake (206). S-equol is a secondary metabolite of daidzein produced by intestinal microorganisms and has stronger anticancer activity than daidzein. Soybean isoflavones are similar to 17β-estradiol in structure. Thus, S-equol can bind to ER and act as a phytoestrogen. ER-β is expressed at a higher level in prostate tissue,the binding affinity of S-equol to ER-β is similar to that of 17β-estradiol, but stronger than that of ER-α, and soy isoflavones are more likely to induce ER-β transcriptional activity (207). Hirokazu Tsuji et al. recently discovered a new intestinal bacteria, Slackia sp. Strain NATTS, which belongs to the Slackia genus and can quickly degrade daidzein into S-equol (208). According to a study of 14203 Japanese men conducted by Kurahashi et al., the highest tertile of plasma S-equol was significantly associated with a reduction in total PCa risk, especially in local cancers (209). S-equol inhibits the growth of LNCaP, DU145, and PC3 cells of human PCa by up-regulating the expression of Forkhead box O3, a tumor-suppressing transcription factor in PCa (113). Among 28 healthy volunteers in Japan, 18 S-equol producers and 10 S-equol non-producers took soybean isoflavones for three months. Three months later, it was found that there was no significant change in serum estradiol and total testosterone levels, while serum sex hormone binding globulin levels significantly increased and serum free testosterone and dihydrotestosterone levels decreased significantly. Long-term soy isoflavone supplementation can stimulate the production of serum estradiol and reduce serum dihydrotestosterone levels in men, thereby reducing the risk of PCa (210). In a large population study, people who ate soy milk more than once a day were associated with a 70% lower risk of PCa, and serum phytoestrogens (genistein, daidzein, and S-equol) had dose-dependent protective effects on the development of PCa (211). Therefore, H. Akaza proposed that S-equol is a key factor in the difference in incidence between Asia and the West. PCA may be susceptible due to a lack of S-equol-transforming bacteria in the intestinal environment and an inability to convert daidzein into S-equol. S-equol-containing supplements can be used to improve the intestinal environment and prevent the occurrence of PCa (212).
3.2.4 Indole-3-methanol
Cauliflower, cabbage, and cauliflower all belong to the same family of cruciferous vegetables, which are significantly associated with the occurrence of PCa. Eating three or more servings of cruciferous vegetables per week can reduce the risk of PCa by 40% (213). Indole-3-methanol (I3C), a derivative of cruciferous vegetables, can derive a series of metabolites in an acidic gastrointestinal environment and prevent PCa. The addition of I3C significantly inhibited tumor growth (p < 0.0001) and changed the structure of the intestinal microbiome, in which the specific bacterial group (M.schaedleri) of Deferribacteres increased significantly. I3C also destroys the interaction between microorganisms, and its chemoprevention may be related to the changes in the composition of microflora and microbial interaction in the intestinal microbial community (214).
In conclusion, the supplementation of beneficial bacteria which produce polyphenols, EL, isoxanthol and indole-3-carbinol significantly reduced the risk of cancer. At present, dietary supplements are the simplest means to regulate the microbiota, and dietary pattern intervention may help to prevent PCa by changing the microbiota. Exploration of more beneficial microbiota is the key to understanding the influence of diet and nutrition on PCa.
3.3 Probiotics intake
Probiotics are crucial for maintaining the composition of intestinal microbiota and enhancing the equilibrium of intestinal microflora. By boosting mucosal barrier function and antibody production, boosting epithelial integrity, and preventing the entry of harmful microbes, they can enhance the host immune response (215, 216). The only probiotic strain that can compete with Escherichia coli, which causes chronic inflammation, is EcN (Escherichia coli Nissle 1917-EcN). Oral probiotics can alter the intestinal microbiota and have an impact on the prostatic inflammatory milieu, according to a new study by Manfredi et al. Two groups of patients with persistent bacterial prostatitis were randomly assigned. Levofloxacin was initially administered to all subjects, and on the basis of this, oral EcN was administered to the experimental group. The biological recurrence rates after 3 months and 6 months considerably lowered in the experimental group, and patient adverse responses were infrequent throughout the experiment. the combination of EcN with levofloxacin can better control the symptoms and biological recurrence in individuals with chronic bacterial prostatitis without reducing the safety of treatment (217).
In another study, mice fed foods rich in the probiotic strain L. reuteri could reduce systemic inflammation by reducing IL-17A and increasing serum testosterone levels and supplement L. reuteri or other probiotic supplements to prevent male hypogonadism, which may give individuals healthier reproductive hormones and gonadal characteristics (218). Anti-cancer immunotherapy will be more effective if specific intestinal resident flora are used. Lactobacillus rhamnosus GG (LGG), the most studied probiotic model of cancer, can be observed in animal models to have anti-inflammatory effects and promote tumor regression (219). In addition, cancer patients lack natural B vitamins, and folic acid and arginine have the greatest metabolic changes (25), Through the use of probiotics and the elimination of external supplements, natural folic acid production may increase in high-risk men, and external sources may increase cancer risk (25). Bifidobacterium can improve self-anti-tumor immunity by promoting the efficacy of antibodies on the axis between programmed cell death protein 1 and its ligand (164). Butyrate is an anti-inflammatory micronutrient produced by Faecalibacterium prausnitzii and Eubacterium rectalie in the intestinal tract and may be related to one of the ways to prevent PCa (7). Tumor necrosis factor-related TRAIL is an endogenous cytokine that can induce apoptosis in malignant tumor cells. Treatment with Lactobacillus can also antagonize PCa by producing TRAIL in peripheral blood mononuclear cells to promote NK activity (220). A recent study has found that intravenous injection of extracellular vesicles derived from A.muciniphipla in mice can establish a CD8+ cytotoxic T cell response to mouse PCa in immunoactive C57BL/6 mice and slow tumor progression in the absence of ADT (221). Probiotics do play a role in tumor prevention and treatment, and treatment with probiotics may be an economical and convenient anti-cancer strategy in the future.
3.4 Fecal microbiota Transplantation (FMT)
FMT can regulate intestinal microbiota to improve the response rate of immunotherapy-resistant patients (222). FMT is an effective method to transplant the intestinal microbiota of healthy donors into patients to restore intestinal microbiota diversity, which is generally treated through the upper or lower digestive tract (223). In the management of Clostridium difficile infection, FMT has demonstrated notable efficacy. FMT was approved as a clinical approach for the treatment of recurrent Clostridium difficile infection in the 2013 guidelines (223). Sivan et al. first revealed that intestinal microbiota may play a role in regulating anti-tumor immunity. They carried out FMT on melanoma mice with different intestinal microbiota contents and found that the tumor growth rate was surprisingly similar, and the tumor growth rate of the side with rapid tumor progress was significantly reduced after FMT (164). Baruch et al. designed a phase I clinical trial to perform FMT on 10 patients with malignant melanoma resistant to anti-PD-1 therapy, including 1 patient with complete remission and 2 patients with partial remission. Furthermore, both their intestinal lamina propria and tumor microenvironment show improved signals for gene spectrum and immune cell infiltration (224). Riquelme et al. used 16S rRNA gene sequencing to analyze the composition of tumor microbiota in pancreatic cancer patients. Long-term survivors of Pseudoxanthomonas, Saccharopolyspora, Streptomyces, and α-diversity outnumber short-term producers. They transplanted the fecal flora of patients with advanced pancreatic cancer with a survival period of > 5 years into mice, and the number of CD8+ T cells in the mice increased significantly, and their tumors shrank significantly. They speculated that FMT changed the diversity and structure of the tumor microenvironment flora to promote the immune response in the mouse model (225). Liu et al. found that the intestinal flora transplantation using CRPC feces accelerated the progress of PCa in mice and increased the abundance of ruminococcus related to poor prognosis, which proved that FMT did have an effect on PCa (60). Androgen deprivation is very important in the treatment of PCa, but almost all patients will be resistant. The FMT treatment is not complicated. FMT updates intestinal microbiota to reduce drug resistance in patients and carries out personalized bacterial transplantation, which may be a favorable weapon for PCa treatment in the future.
4 Conclusions
We summarized the different gut microbiota compositions of a large number of prostate cancer patients compared with non-prostate cancer patients and found that certain gut microbes were associated with an increased risk of prostate cancer, including (Bacteroides massiliensis, Bacteroides, Streptococcus Tissierellaceae, Lachnospiraceae, Pseudomonadales, Proteobacteria, Gammaproteobacteria, Ruminococcus, Bacillus, Rikenellaceae, and Clostridiales), and other organisms. Gut metabolites such as estrogen, androgen, folate, and short-chain fatty acids are involved in the pathogenesis of prostate cancer, while their other metabolites such as polyphenols, ETs, and isoflavones are helpful for the treatment of prostate cancer. It seems that intestinal microorganisms can directly affect PCa through bacterial translocation or toxins and may also be indirectly affected by intestinal microbiota, including metabolites, immunity, genes, and the effects of anti-tumor drugs. If PCa is confirmed to be affected by intestinal microbiota, changing intestinal microbiota through diet or FMT treatment will provide an effective treatment strategy for PCa prevention and treatment. Certain microorganisms may also be used as diagnostic markers of PCa. In a word, the intestinal microbiome may play an important role in the development, diagnosis, and treatment of PCa. More research is needed to explore the complex interrelationships and ways of action of the intestinal microbiome. More research is needed to understand the role of the intestinal microbiome in PCa patients, and personalized treatment is used to target the intestinal microbiome of different patients.
Author contributions
KT and QW conceptualized the review, analyzed the data, and helped to write the manuscript. CZ, KH, ZP, LZ, WL, BC, SX, YY, TH, and WZ helped to write the manuscript and prepared the figures. All authors contributed to the article and approved the submitted version.
Funding
This study was supported by the Science and Technology Fund Project of Guizhou Health Commission. (gzwkj2021-211).
Conflict of interest
The authors declare that the research was conducted in the absence of any commercial or financial relationships that could be construed as a potential conflict of interest.
Publisher’s note
All claims expressed in this article are solely those of the authors and do not necessarily represent those of their affiliated organizations, or those of the publisher, the editors and the reviewers. Any product that may be evaluated in this article, or claim that may be made by its manufacturer, is not guaranteed or endorsed by the publisher.
References
1. Wong MC, Goggins WB, Wang HH, Fung FD, Leung C, Wong SY, et al. Global incidence and mortality for prostate cancer: analysis of temporal patterns and trends in 36 countries. Eur Urol (2016) 70(5):862–74. doi: 10.1016/j.eururo.2016.05.043
2. Global Burden of Disease Cancer C, Fitzmaurice C, Dicker D, Pain A, Hamavid H, Moradi-Lakeh M, et al. The global burden of cancer 2013. JAMA Oncol (2015) 1(4):505–27. doi: 10.1001/jamaoncol.2015.0735
3. Rebbeck TR. Prostate cancer genetics: variation by race, ethnicity, and geography. Semin Radiat Oncol (2017) 27(1):3–10. doi: 10.1016/j.semradonc.2016.08.002
4. Di Zazzo E, Galasso G, Giovannelli P, Di Donato M, Di Santi A, Cernera G, et al. Prostate cancer stem cells: the role of androgen and estrogen receptors. Oncotarget (2016) 7(1):193–208. doi: 10.18632/oncotarget.6220
5. Di Zazzo E, Galasso G, Giovannelli P, Di Donato M, Castoria G. Estrogens and their receptors in prostate cancer: therapeutic implications. Front Oncol (2018) 8:2. doi: 10.3389/fonc.2018.00002
6. Sung H, Ferlay J, Siegel RL, Laversanne M, Soerjomataram I, Jemal A, et al. Global cancer statistics 2020: GLOBOCAN estimates of incidence and mortality worldwide for 36 cancers in 185 countries. CA Cancer J Clin (2021) 71(3):209–49. doi: 10.3322/caac.21660
7. Crocetto F, Boccellino M, Barone B, Di Zazzo E, Sciarra A, Galasso G, et al. The crosstalk between prostate cancer and microbiota inflammation: nutraceutical products are useful to balance this interplay? Nutrients (2020) 12(9):2648. doi: 10.3390/nu12092648
8. Cattrini C, Castro E, Lozano R, Zanardi E, Rubagotti A, Boccardo F, et al. Current treatment options for metastatic hormone-sensitive prostate cancer. Cancers (Basel) (2019) 11(9):1355. doi: 10.3390/cancers11091355
9. Wilson KM, Giovannucci EL, Mucci LA. Lifestyle and dietary factors in the prevention of lethal prostate cancer. Asian J Androl (2012) 14(3):365–74. doi: 10.1038/aja.2011.142
10. Huang PY, Yang YC, Wang CI, Hsiao PW, Chiang HI, Chen TW. Increase in akkermansiaceae in gut microbiota of prostate cancer-bearing mice. Int J Mol Sci (2021) 22(17):9626. doi: 10.3390/ijms22179626
11. Matsushita M, Fujita K, Hatano K, De Velasco MA, Uemura H, Nonomura N. Connecting the dots between the gut-IGF-1-Prostate axis: a role of IGF-1 in prostate carcinogenesis. Front Endocrinol (Lausanne) (2022) 13:852382. doi: 10.3389/fendo.2022.852382
12. Ly LK, Rowles JL 3rd, Paul HM, Alves JMP, Yemm C, Wolf PM, et al. Bacterial steroid-17,20-desmolase is a taxonomically rare enzymatic pathway that converts prednisone to 1,4-androstanediene-3,11,17-trione, a metabolite that causes proliferation of prostate cancer cells. J Steroid Biochem Mol Biol (2020) 199:105567. doi: 10.1016/j.jsbmb.2019.105567
13. Valdes AM, Walter J, Segal E, Spector TD. Role of the gut microbiota in nutrition and health. BMJ (2018) 361:k2179. doi: 10.1136/bmj.k2179
14. Human Microbiome Project, C. Structure, function and diversity of the healthy human microbiome. Nature (2012) 486(7402):207–14. doi: 10.1038/nature11234
15. Bouyahya A, Omari NE, El Hachlafi N, Jemly ME, Hakkour M, Balahbib A, et al. Chemical compounds of berry-derived polyphenols and their effects on gut microbiota, inflammation, and cancer. Molecules (2022) 27(10):3286. doi: 10.3390/molecules27103286
16. Jaye K, Li CG, Bhuyan DJ. The complex interplay of gut microbiota with the five most common cancer types: from carcinogenesis to therapeutics to prognoses. Crit Rev Oncol Hematol (2021) 165:103429. doi: 10.1016/j.critrevonc.2021.103429
17. Matsushita M, Fujita K, Nonomura N. Influence of diet and nutrition on prostate cancer. Int J Mol Sci (2020) 21(4):1447. doi: 10.3390/ijms21041447
18. Rothschild D, Weissbrod O, Barkan E, Kurilshikov A, Korem T, Zeevi D, et al. Environment dominates over host genetics in shaping human gut microbiota. Nature (2018) 555(7695):210–5. doi: 10.1038/nature25973
19. He Y, Wu W, Zheng HM, Li P, McDonald D, Sheng HF, et al. Regional variation limits applications of healthy gut microbiome reference ranges and disease models. Nat Med (2018) 24(10):1532–5. doi: 10.1038/s41591-018-0164-x
20. Ganesan K, Chung SK, Vanamala J, Xu B. Causal relationship between diet-induced gut microbiota changes and diabetes: a novel strategy to transplant faecalibacterium prausnitzii in preventing diabetes. Int J Mol Sci (2018) 19(12):3720. doi: 10.3390/ijms19123720
21. Katongole P, Sande OJ, Joloba M, Reynolds SJ, Niyonzima N. The human microbiome and its link in prostate cancer risk and pathogenesis. Infect Agent Cancer (2020) 15:53. doi: 10.1186/s13027-020-00319-2
22. Wang Y, Qian PY. Conservative fragments in bacterial 16S rRNA genes and primer design for 16S ribosomal DNA amplicons in metagenomic studies. PloS One (2009) 4(10):e7401. doi: 10.1371/journal.pone.0007401
23. Quince C, Walker AW, Simpson JT, Loman NJ, Segata N. Shotgun metagenomics, from sampling to analysis. Nat Biotechnol (2017) 35(9):833–44. doi: 10.1038/nbt.3935
24. Plottel CS, Blaser MJ. Microbiome and malignancy. Cell Host Microbe (2011) 10(4):324–35. doi: 10.1016/j.chom.2011.10.003
25. Liss MA, White JR, Goros M, Gelfond J, Leach R, Johnson-Pais T, et al. Metabolic biosynthesis pathways identified from fecal microbiome associated with prostate cancer. Eur Urol (2018) 74(5):575–82. doi: 10.1016/j.eururo.2018.06.033
26. Golombos DM, Ayangbesan A, O'Malley P, Lewicki P, Barlow L, Barbieri CE, et al. The role of gut microbiome in the pathogenesis of prostate cancer: a prospective, pilot study. Urology (2018) 111:122–8. doi: 10.1016/j.urology.2017.08.039
27. Sfanos KS, Markowski MC, Peiffer LB, Ernst SE, White JR, Pienta KJ, et al. Compositional differences in gastrointestinal microbiota in prostate cancer patients treated with androgen axis-targeted therapies. Prostate Cancer Prostatic Dis (2018) 21(4):539–48. doi: 10.1038/s41391-018-0061-x
28. Matsushita M, Fujita K, Motooka D, Hatano K, Fukae S, Kawamura N, et al. The gut microbiota associated with high-Gleason prostate cancer. Cancer Sci (2021) 112(8):3125–35. doi: 10.1111/cas.14998
29. Smith KS, Frugé AD, van der Pol W, Caston NE, Morrow CD, Demark-Wahnefried W, et al. Gut microbial differences in breast and prostate cancer cases from two randomised controlled trials compared to matched cancer-free controls. Benef Microbes (2021) 12(3):239–48. doi: 10.3920/BM2020.0098
30. Kure A, Tsukimi T, Ishii C, Aw W, Obana N, Nakato G, et al. Gut environment changes due to androgen deprivation therapy in patients with prostate cancer. Prostate Cancer Prostatic Dis (2022). doi: 10.1038/s41391-022-00536-3
31. Li JKM, Wang LL, Wong CYP, Chiu PKF, Teoh JYC, Kwok HSW, et al. A cross-sectional study on gut microbiota in prostate cancer patients with prostatectomy or androgen deprivation therapy. Prostate Cancer Prostatic Dis (2021) 24(4):1063–72. doi: 10.1038/s41391-021-00360-1
32. Liu Y, Jiang H. Compositional differences of gut microbiome in matched hormone-sensitive and castration-resistant prostate cancer. Transl Androl Urol (2020) 9(5):1937–44. doi: 10.21037/tau-20-566
33. Sethi V, Kurtom S, Tarique M, Lavania S, Malchiodi Z, Hellmund L, et al. Gut microbiota promotes tumor growth in mice by modulating immune response. Gastroenterology (2018) 155(1):33–37 e6. doi: 10.1053/j.gastro.2018.04.001
34. Petrelli F, Ghidini M, Ghidini A, Perego G, Cabiddu M, Khakoo S, et al. Use of antibiotics and risk of cancer: a systematic review and meta-analysis of observational studies. Cancers (Basel) (2019) 11(8):1174. doi: 10.3390/cancers11081174
35. Boursi B, Mamtani R, Haynes K, Yang YX. Recurrent antibiotic exposure may promote cancer formation–another step in understanding the role of the human microbiota? Eur J Cancer (2015) 51(17):2655–64. doi: 10.1016/j.ejca.2015.08.015
36. Tulstrup MV, Christensen EG, Carvalho V, Linninge C, Ahrné S, Højberg O, et al. Antibiotic treatment affects intestinal permeability and gut microbial composition in wistar rats dependent on antibiotic class. PloS One (2015) 10(12):e0144854. doi: 10.1371/journal.pone.0144854
37. Matsushita M, Fujita K, Hayashi T, Kayama H, Motooka D, Hase H, et al. Gut microbiota-derived short-chain fatty acids promote prostate cancer growth via IGF1 signaling. Cancer Res (2021) 81(15):4014–26. doi: 10.1158/0008-5472.CAN-20-4090
38. Liu Y, Wu X, Jiang H. Combined maternal and post-weaning high fat diet inhibits male offspring's prostate cancer tumorigenesis in transgenic adenocarcinoma of mouse prostate model. Prostate (2019) 79(5):544–53. doi: 10.1002/pros.23760
39. Carmody RN, Gerber GK, Luevano JM Jr, Gatti DM, Somes L, Svenson KL, et al. Diet dominates host genotype in shaping the murine gut microbiota. Cell Host Microbe (2015) 17(1):72–84. doi: 10.1016/j.chom.2014.11.010
40. Drasar BS, Crowther JS, Goddard P, Hawksworth G, Hill MJ, Peach S, et al. The relation between diet and the gut microflora in man. Proc Nutr Soc (1973) 32(2):49–52. doi: 10.1079/PNS19730014
41. Turnbaugh PJ, Ridaura VK, Faith JJ, Rey FE, Knight R, Gordon JI, et al. The effect of diet on the human gut microbiome: a metagenomic analysis in humanized gnotobiotic mice. Sci Transl Med (2009) 1(6):6ra14. doi: 10.1126/scitranslmed.3000322
42. Yu H, Meng H, Zhou F, Ni X, Shen S, Das UN, et al. Urinary microbiota in patients with prostate cancer and benign prostatic hyperplasia. Arch Med Sci (2015) 11(2):385–94. doi: 10.5114/aoms.2015.50970
43. Matsushita M, Fujita K, Hatano K, Hayashi T, Kayama H, Motooka D, et al. High-fat diet promotes prostate cancer growth through histamine signaling. Int J Cancer (2022) 151(4):623–36. doi: 10.1002/ijc.34028
44. Manzoor SS, Doedens A, Burns MB. The promise and challenge of cancer microbiome research. Genome Biol (2020) 21(1):131. doi: 10.1186/s13059-020-02037-9
45. Rubinstein MR, Wang X, Liu W, Hao Y, Cai G, Han YW, et al. Fusobacterium nucleatum promotes colorectal carcinogenesis by modulating e-cadherin/beta-catenin signaling via its FadA adhesin. Cell Host Microbe (2013) 14(2):195–206. doi: 10.1016/j.chom.2013.07.012
46. Ishikawa D, Sasaki T, Osada T, Kuwahara-Arai K, Haga K, Shibuya T, et al. Changes in intestinal microbiota following combination therapy with fecal microbial transplantation and antibiotics for ulcerative colitis. Inflammation Bowel Dis (2017) 23(1):116–25. doi: 10.1097/MIB.0000000000000975
47. Loo TM, Kamachi F, Watanabe Y, Yoshimoto S, Kanda H, Arai Y, et al. Gut microbiota promotes obesity-associated liver cancer through PGE2-mediated suppression of antitumor immunity. Cancer Discovery (2017) 7(5):522–38. doi: 10.1158/2159-8290.CD-16-0932
48. Minter MR, Zhang C, Leone V, Ringus DL, Zhang X, Oyler-Castrillo P, et al. Antibiotic-induced perturbations in gut microbial diversity influences neuro-inflammation and amyloidosis in a murine model of alzheimer's disease. Sci Rep (2016) 6:30028. doi: 10.1038/srep30028
49. Picardo SL, Coburn B, Hansen AR. The microbiome and cancer for clinicians. Crit Rev Oncol Hematol (2019) 141:1–12. doi: 10.1016/j.critrevonc.2019.06.004
50. Ganapathy V, Thangaraju M, Prasad PD, Martin PM, Singh N. Transporters and receptors for short-chain fatty acids as the molecular link between colonic bacteria and the host. Curr Opin Pharmacol (2013) 13(6):869–74. doi: 10.1016/j.coph.2013.08.006
51. Miller TL, Wolin MJ. Pathways of acetate, propionate, and butyrate formation by the human fecal microbial flora. Appl Environ Microbiol (1996) 62(5):1589–92. doi: 10.1128/aem.62.5.1589-1592.1996
52. Mirzaei R, Afaghi A, Babakhani S, Sohrabi MR, Hosseini-Fard SR, Babolhavaeji K, et al. Role of microbiota-derived short-chain fatty acids in cancer development and prevention. BioMed Pharmacother (2021) 139:111619. doi: 10.1016/j.biopha.2021.111619
53. Ratajczak W, Mizerski A, Rył A, Słojewski M, Sipak O, Piasecka M, et al. Alterations in fecal short chain fatty acids (SCFAs) and branched short-chain fatty acids (BCFAs) in men with benign prostatic hyperplasia (BPH) and metabolic syndrome (MetS). Aging (Albany NY) (2021) 13(8):10934–54. doi: 10.18632/aging.202968
54. Watts EL, Goldacre R, Key TJ, Allen NE, Travis RC, Perez-Cornago A, et al. Hormone-related diseases and prostate cancer: an English national record linkage study. Int J Cancer (2020) 147(3):803–10. doi: 10.1002/ijc.32808
55. Ujike T, Uemura M, Kawashima A, Nagahara A, Fujita K, Miyagawa Y, et al. A novel model to predict positive prostate biopsy based on serum androgen level. Endocr Relat Cancer (2018) 25(1):59–67. doi: 10.1530/ERC-17-0134
56. Tsujimura A. The relationship between testosterone deficiency and men's health. World J Mens Health (2013) 31(2):126–35. doi: 10.5534/wjmh.2013.31.2.126
57. Matsushita M, Fujita K, Motooka D, Hatano K, Hata J, Nishimoto M, et al. Firmicutes in gut microbiota correlate with blood testosterone levels in elderly men. World J Mens Health (2022) 40(3):517–25. doi: 10.5534/wjmh.210190
58. Shin JH, Park YH, Sim M, Kim SA, Joung H, Shin DM, et al. Serum level of sex steroid hormone is associated with diversity and profiles of human gut microbiome. Res Microbiol (2019) 170(4-5):192–201. doi: 10.1016/j.resmic.2019.03.003
59. Pernigoni N, Zagato E, Calcinotto A, Troiani M, Mestre RP, Calì B, et al. Commensal bacteria promote endocrine resistance in prostate cancer through androgen biosynthesis. Science (2021) 374(6564):216–24. doi: 10.1126/science.abf8403
60. Liu Y, Yang C, Zhang Z, Jiang H. Gut microbiota dysbiosis accelerates prostate cancer progression through increased LPCAT1 expression and enhanced DNA repair pathways. Front Oncol (2021) 11:679712. doi: 10.3389/fonc.2021.679712
61. Nelles JL, Hu WY, Prins GS. Estrogen action and prostate cancer. Expert Rev Endocrinol Metab (2011) 6(3):437–51. doi: 10.1586/eem.11.20
62. Dobbs RW, Malhotra NR, Greenwald DT, Wang AY, Prins GS, Abern MR. Estrogens and prostate cancer. Prostate Cancer Prostatic Dis (2019) 22(2):185–94. doi: 10.1038/s41391-018-0081-6
63. Sha S, Ni L, Stefil M, Dixon M, Mouraviev V. The human gastrointestinal microbiota and prostate cancer development and treatment. Investig Clin Urol (2020) 61(Suppl 1):S43–50. doi: 10.4111/icu.2020.61.S1.S43
64. Rossi M, Amaretti A, Raimondi S. Folate production by probiotic bacteria. Nutrients (2011) 3(1):118–34. doi: 10.3390/nu3010118
65. Smyth MJ, Hayakawa Y, Takeda K, Yagita H. New aspects of natural-killer-cell surveillance and therapy of cancer. Nat Rev Cancer (2002) 2(11):850–61. doi: 10.1038/nrc928
66. Choi SW, Mason JB. Folate status: effects on pathways of colorectal carcinogenesis. J Nutr (2002) 132(8 Suppl):2413S–8S. doi: 10.1093/jn/132.8.2413S
67. Kawakami M, Nakayama J. Enhanced expression of prostate-specific membrane antigen gene in prostate cancer as revealed by in situ hybridization. Cancer Res (1997) 57(12):2321–4.
68. Ross JS, Sheehan CE, Fisher HA, Kaufman RP Jr, Kaur P, Gray K, et al. Correlation of primary tumor prostate-specific membrane antigen expression with disease recurrence in prostate cancer. Clin Cancer Res (2003) 9(17):6357–62.
69. Figueiredo JC, Grau MV, Haile RW, Sandler RS, Summers RW, Bresalier RS, et al. Folic acid and risk of prostate cancer: results from a randomized clinical trial. J Natl Cancer Inst (2009) 101(6):432–5. doi: 10.1093/jnci/djp019
70. Nemet I, Saha PP, Gupta N, Zhu W, Romano KA, Skye SM, et al. A cardiovascular disease-linked gut microbial metabolite acts via adrenergic receptors. Cell (2020) 180(5):862–877 e22. doi: 10.1016/j.cell.2020.02.016
71. Reichard CA, Naelitz BD, Wang Z, Jia X, Li J, Stampfer MJ, et al. Gut microbiome-dependent metabolic pathways and risk of lethal prostate cancer: prospective analysis of a PLCO cancer screening trial cohort. Cancer Epidemiol Biomarkers Prev (2022) 31(1):192–9. doi: 10.1158/1055-9965.EPI-21-0766
72. Visconti A, Le Roy CI, Rosa F, Rossi N, Martin TC, Mohney RP, et al. Interplay between the human gut microbiome and host metabolism. Nat Commun (2019) 10(1):4505. doi: 10.1038/s41467-019-12476-z
73. Pouncey AL, Scott AJ, Alexander JL, Marchesi J, Kinross J. Gut microbiota, chemotherapy and the host: the influence of the gut microbiota on cancer treatment. Ecancermedicalscience (2018) 12:868. doi: 10.3332/ecancer.2018.868
74. Ince MN, Blazar BR, Edmond MB, Tricot G, Wannemuehler MJ. Understanding luminal microorganisms and their potential effectiveness in treating intestinal inflammation. Inflammation Bowel Dis (2016) 22(1):194–201. doi: 10.1097/MIB.0000000000000599
75. Garrett WS, Gordon JI, Glimcher LH. Homeostasis and inflammation in the intestine. Cell (2010) 140(6):859–70. doi: 10.1016/j.cell.2010.01.023
76. Belkaid Y, Harrison OJ. Homeostatic immunity and the microbiota. Immunity (2017) 46(4):562–76. doi: 10.1016/j.immuni.2017.04.008
77. Belkaid Y, Hand TW. Role of the microbiota in immunity and inflammation. Cell (2014) 157(1):121–41. doi: 10.1016/j.cell.2014.03.011
78. Kogut MH, Lee A, Santin E. Microbiome and pathogen interaction with the immune system. Poult Sci (2020) 99(4):1906–13. doi: 10.1016/j.psj.2019.12.011
79. Kelsall B. Recent progress in understanding the phenotype and function of intestinal dendritic cells and macrophages. Mucosal Immunol (2008) 1(6):460–9. doi: 10.1038/mi.2008.61
80. Abraham C, Medzhitov R. Interactions between the host innate immune system and microbes in inflammatory bowel disease. Gastroenterology (2011) 140(6):1729–37. doi: 10.1053/j.gastro.2011.02.012
81. Maloy KJ, Powrie F. Intestinal homeostasis and its breakdown in inflammatory bowel disease. Nature (2011) 474(7351):298–306. doi: 10.1038/nature10208
82. Clarke TB, Davis KM, Lysenko ES, Zhou AY, Yu Y, Weiser JN. Recognition of peptidoglycan from the microbiota by Nod1 enhances systemic innate immunity. Nat Med (2010) 16(2):228–31. doi: 10.1038/nm.2087
83. Schwabe RF, Jobin C. The microbiome and cancer. Nat Rev Cancer (2013) 13(11):800–12. doi: 10.1038/nrc3610
84. Coombes JL, Powrie F. Dendritic cells in intestinal immune regulation. Nat Rev Immunol (2008) 8(6):435–46. doi: 10.1038/nri2335
85. Shida K, Nanno M. Probiotics and immunology: separating the wheat from the chaff. Trends Immunol (2008) 29(11):565–73. doi: 10.1016/j.it.2008.07.011
86. Takeda K, Clausen BE, Kaisho T, Tsujimura T, Terada N, Förster I, et al. Enhanced Th1 activity and development of chronic enterocolitis in mice devoid of Stat3 in macrophages and neutrophils. Immunity (1999) 10(1):39–49. doi: 10.1016/S1074-7613(00)80005-9
87. Sfanos KS, De Marzo AM. Prostate cancer and inflammation: the evidence. Histopathology (2012) 60(1):199–215. doi: 10.1111/j.1365-2559.2011.04033.x
88. Smith K, McCoy KD, Macpherson AJ. Use of axenic animals in studying the adaptation of mammals to their commensal intestinal microbiota. Semin Immunol (2007) 19(2):59–69. doi: 10.1016/j.smim.2006.10.002
89. Agace WW, McCoy KD. Regionalized development and maintenance of the intestinal adaptive immune landscape. Immunity (2017) 46(4):532–48. doi: 10.1016/j.immuni.2017.04.004
90. Meisel M, Mayassi T, Fehlner-Peach H, Koval JC, O'Brien SL, Hinterleitner R, et al. Interleukin-15 promotes intestinal dysbiosis with butyrate deficiency associated with increased susceptibility to colitis. ISME J (2017) 11(1):15–30. doi: 10.1038/ismej.2016.114
91. Chiu CY, Chan YL, Tsai MH, Wang CJ, Chiang MH, Chiu CC, et al. Gut microbial dysbiosis is associated with allergen-specific IgE responses in young children with airway allergies. World Allergy Organ J (2019) 12(3):100021. doi: 10.1016/j.waojou.2019.100021
92. Wong SH, Zhao L, Zhang X, Nakatsu G, Han J, Xu W, et al. Gavage of fecal samples from patients with colorectal cancer promotes intestinal carcinogenesis in germ-free and conventional mice. Gastroenterology (2017) 153(6):1621–1633 e6. doi: 10.1053/j.gastro.2017.08.022
93. Hurtado CG, Wan F, Housseau F, Sears CL. Roles for interleukin 17 and adaptive immunity in pathogenesis of colorectal cancer. Gastroenterology (2018) 155(6):1706–15. doi: 10.1053/j.gastro.2018.08.056
94. Ma J, Gnanasekar A, Lee A, Li WT, Haas M, Wang-Rodriguez J, et al. Influence of intratumor microbiome on clinical outcome and immune processes in prostate cancer. Cancers (Basel) (2020) 12(9):2524. doi: 10.3390/cancers12092524
95. Hackam DJ, Good M, Sodhi CP. Mechanisms of gut barrier failure in the pathogenesis of necrotizing enterocolitis: toll-like receptors throw the switch. Semin Pediatr Surg (2013) 22(2):76–82. doi: 10.1053/j.sempedsurg.2013.01.003
96. Fulbright LE, Ellermann M, Arthur JC. The microbiome and the hallmarks of cancer. PloS Pathog (2017) 13(9):e1006480. doi: 10.1371/journal.ppat.1006480
97. Terrisse S, Goubet AG, Ueda K, Thomas AM, Quiniou V, Thelemaque C, et al. Immune system and intestinal microbiota determine efficacy of androgen deprivation therapy against prostate cancer. J Immunother Cancer (2022) 10(3):e004191. doi: 10.1136/jitc-2021-004191
98. Zhang Q, Yu N, Lee C. Mysteries of TGF-beta paradox in benign and malignant cells. Front Oncol (2014) 4:94. doi: 10.3389/fonc.2014.00094
99. Menzies BE. The role of fibronectin binding proteins in the pathogenesis of staphylococcus aureus infections. Curr Opin Infect Dis (2003) 16(3):225–9. doi: 10.1097/00001432-200306000-00007
100. Li N, Ren A, Wang X, Fan X, Zhao Y, Gao GF, et al. Influenza viral neuraminidase primes bacterial coinfection through TGF-beta-mediated expression of host cell receptors. Proc Natl Acad Sci U.S.A. (2015) 112(1):238–43. doi: 10.1073/pnas.1414422112
101. Dzutsev A, Badger JH, Perez-Chanona E, Roy S, Salcedo R, Smith CK, et al. Microbes and cancer. Annu Rev Immunol (2017) 35:199–228. doi: 10.1146/annurev-immunol-051116-052133
102. Hooper LV, Littman DR, Macpherson AJ. Interactions between the microbiota and the immune system. Science (2012) 336(6086):1268–73. doi: 10.1126/science.1223490
103. Schroeder BO, Backhed F. Signals from the gut microbiota to distant organs in physiology and disease. Nat Med (2016) 22(10):1079–89. doi: 10.1038/nm.4185
104. Raskov H, Burcharth J, Pommergaard HC. Linking gut microbiota to colorectal cancer. J Cancer (2017) 8(17):3378–95. doi: 10.7150/jca.20497
105. Bultman SJ. Emerging roles of the microbiome in cancer. Carcinogenesis (2014) 35(2):249–55. doi: 10.1093/carcin/bgt392
106. Poutahidis T, Kleinewietfeld M, Erdman SE. Gut microbiota and the paradox of cancer immunotherapy. Front Immunol (2014) 5:157. doi: 10.3389/fimmu.2014.00157
107. Neuman H, Debelius JW, Knight R, Koren O. Microbial endocrinology: the interplay between the microbiota and the endocrine system. FEMS Microbiol Rev (2015) 39(4):509–21. doi: 10.1093/femsre/fuu010
108. Lynch SV, Pedersen O. The human intestinal microbiome in health and disease. N Engl J Med (2016) 375(24):2369–79. doi: 10.1056/NEJMra1600266
109. Cougnoux A, Dalmasso G, Martinez R, Buc E, Delmas J, Gibold L, et al. Bacterial genotoxin colibactin promotes colon tumour growth by inducing a senescence-associated secretory phenotype. Gut (2014) 63(12):1932–42. doi: 10.1136/gutjnl-2013-305257
110. Sato H, Narita S, Ishida M, Takahashi Y, Mingguo H, Kashima S, et al. Specific gut microbial environment in lard diet-induced prostate cancer development and progression. Int J Mol Sci (2022) 23(4):2214. doi: 10.3390/ijms23042214
111. Takuwa N, Du W, Kaneko E, Okamoto Y, Yoshioka K, Takuwa , et al. Tumor-suppressive sphingosine-1-phosphate receptor-2 counteracting tumor-promoting sphingosine-1-phosphate receptor-1 and sphingosine kinase 1 - Jekyll hidden behind Hyde. Am J Cancer Res (2011) 1(4):460–81.
112. Brown K, DeCoffe D, Molcan E, Gibson DL. Diet-induced dysbiosis of the intestinal microbiota and the effects on immunity and disease. Nutrients (2012) 4(8):1095–119. doi: 10.3390/nu4081095
113. Liu Y, Wu X, Jiang H. High dietary fat intake lowers serum equol concentration and promotes prostate carcinogenesis in a transgenic mouse prostate model. Nutr Metab (Lond) (2019) 16:24. doi: 10.1186/s12986-019-0351-x
114. Fujita K, Matsushita M, Banno E, De Velasco MA, Hatano K, Nonomura N, et al. Gut microbiome and prostate cancer. Int J Urol (2022) 29(8):793–8. doi: 10.1111/iju.14894
115. Beyaz S, Mana MD, Roper J, Kedrin D, Saadatpour A, Hong SJ, et al. High-fat diet enhances stemness and tumorigenicity of intestinal progenitors. Nature (2016) 531(7592):53–8. doi: 10.1038/nature17173
116. Nakai Y, Nonomura N. Inflammation and prostate carcinogenesis. Int J Urol (2013) 20(2):150–60. doi: 10.1111/j.1442-2042.2012.03101.x
117. Wolochow H, Hildebrand GJ, Lamanna C. Translocation of microorganisms across the intestinal wall of the rat: effect of microbial size and concentration. J Infect Dis (1966) 116(4):523–8. doi: 10.1093/infdis/116.4.523
118. Berg RD. Promotion of the translocation of enteric bacteria from the gastrointestinal tracts of mice by oral treatment with penicillin, clindamycin, or metronidazole. Infect Immun (1981) 33(3):854–61. doi: 10.1128/iai.33.3.854-861.1981
119. Parks DA, Bulkley GB, Granger DN, Hamilton SR, McCord JM. Ischemic injury in the cat small intestine: role of superoxide radicals. Gastroenterology (1982) 82(1):9–15. doi: 10.1016/0016-5085(82)90115-9
120. Morehouse JL, Specian RD, Stewart JJ, Berg RD. Translocation of indigenous bacteria from the gastrointestinal tract of mice after oral ricinoleic acid treatment. Gastroenterology (1986) 91(3):673–82. doi: 10.1016/0016-5085(86)90638-4
121. Owens WE, Berg RD. Bacterial translocation from the gastrointestinal tract of athymic (nu/nu) mice. Infect Immun (1980) 27(2):461–7. doi: 10.1128/iai.27.2.461-467.1980
122. Gautreaux MD, Deitch EA, Berg RD. T Lymphocytes in host defense against bacterial translocation from the gastrointestinal tract. Infect Immun (1994) 62(7):2874–84. doi: 10.1128/iai.62.7.2874-2884.1994
123. Liu J, Huang L, Luo M, Xia X. Bacterial translocation in acute pancreatitis. Crit Rev Microbiol (2019) 45(5-6):539–47. doi: 10.1080/1040841X.2019.1621795
124. Cirera I, Bauer TM, Navasa M, Vila J, Grande L, Taurá P, et al. Bacterial translocation of enteric organisms in patients with cirrhosis. J Hepatol (2001) 34(1):32–7. doi: 10.1016/S0168-8278(00)00013-1
125. Tilg H, Zmora N, Adolph TE, Elinav E. The intestinal microbiota fuelling metabolic inflammation. Nat Rev Immunol (2020) 20(1):40–54. doi: 10.1038/s41577-019-0198-4
126. Hur KY, Lee MS. Gut microbiota and metabolic disorders. Diabetes Metab J (2015) 39(3):198–203. doi: 10.4093/dmj.2015.39.3.198
127. Sfanos KS, Yegnasubramanian S, Nelson WG, De Marzo AM. The inflammatory microenvironment and microbiome in prostate cancer development. Nat Rev Urol (2018) 15(1):11–24. doi: 10.1038/nrurol.2017.167
128. Karl JP, Margolis LM, Madslien EH, Murphy NE, Castellani JW, Gundersen Y, et al. Changes in intestinal microbiota composition and metabolism coincide with increased intestinal permeability in young adults under prolonged physiological stress. Am J Physiol Gastrointest Liver Physiol (2017) 312(6):G559–71. doi: 10.1152/ajpgi.00066.2017
129. Damms-Machado A, Louis S, Schnitzer A, Volynets V, Rings A, Basrai M, et al. Gut permeability is related to body weight, fatty liver disease, and insulin resistance in obese individuals undergoing weight reduction. Am J Clin Nutr (2017) 105(1):127–35. doi: 10.3945/ajcn.116.131110
130. Balzan S, de Almeida Quadros C, de Cleva R, Zilberstein B, Cecconello I. Bacterial translocation: overview of mechanisms and clinical impact. J Gastroenterol Hepatol (2007) 22(4):464–71. doi: 10.1111/j.1440-1746.2007.04933.x
131. Fasano A. Regulation of intercellular tight junctions by zonula occludens toxin and its eukaryotic analogue zonulin. Ann N Y Acad Sci (2000) 915:214–22. doi: 10.1111/j.1749-6632.2000.tb05244.x
132. Lencer WI. Microbes and microbial toxins: paradigms for microbial-mucosal toxins. v. cholera: invasion of the intestinal epithelial barrier by a stably folded protein toxin. Am J Physiol Gastrointest Liver Physiol (2001) 280(5):G781–6. doi: 10.1152/ajpgi.2001.280.5.G781
133. Hecht G. Microbes and microbial toxins: paradigms for microbial-mucosal interactions. VII. enteropathogenic escherichia coli: physiological alterations from an extracellular position. Am J Physiol Gastrointest Liver Physiol (2001) 281(1):G1–7. doi: 10.1152/ajpgi.2001.281.1.G1
134. Laughlin RS, Musch MW, Hollbrook CJ, Rocha FM, Chang EB, Alverdy JC. The key role of pseudomonas aeruginosa PA-I lectin on experimental gut-derived sepsis. Ann Surg (2000) 232(1):133–42. doi: 10.1097/00000658-200007000-00019
135. Pothoulakis C. Effects of clostridium difficile toxins on epithelial cell barrier. Ann N Y Acad Sci (2000) 915:347–56. doi: 10.1111/j.1749-6632.2000.tb05263.x
136. Feltis BA, Wiesner SM, Kim AS, Erlandsen SL, Lyerly DL, Wilkins TD, et al. Clostridium difficile toxins a and b can alter epithelial permeability and promote bacterial paracellular migration through HT-29 enterocytes. Shock (2000) 14(6):629–34. doi: 10.1097/00024382-200014060-00010
137. Francois M, Le Cabec V, Dupont MA, Sansonetti PJ, Maridonneau-Parini I. Induction of necrosis in human neutrophils by shigella flexneri requires type III secretion, IpaB and IpaC invasins, and actin polymerization. Infect Immun (2000) 68(3):1289–96. doi: 10.1128/IAI.68.3.1289-1296.2000
138. Sears CL. Molecular physiology and pathophysiology of tight junctions v. assault of the tight junction by enteric pathogens. Am J Physiol Gastrointest Liver Physiol (2000) 279(6):G1129–34. doi: 10.1152/ajpgi.2000.279.6.G1129
139. Cani PD, Bibiloni R, Knauf C, Waget A, Neyrinck AM, Delzenne NM, et al. Changes in gut microbiota control metabolic endotoxemia-induced inflammation in high-fat diet-induced obesity and diabetes in mice. Diabetes (2008) 57(6):1470–81. doi: 10.2337/db07-1403
140. Wlodarska M, Willing B, Keeney KM, Menendez A, Bergstrom KS, Gill N, et al. Antibiotic treatment alters the colonic mucus layer and predisposes the host to exacerbated citrobacter rodentium-induced colitis. Infect Immun (2011) 79(4):1536–45. doi: 10.1128/IAI.01104-10
141. Steffen EK, Berg RD. Relationship between cecal population levels of indigenous bacteria and translocation to the mesenteric lymph nodes. Infect Immun (1983) 39(3):1252–9. doi: 10.1128/iai.39.3.1252-1259.1983
142. Wiest R, Rath HC. Gastrointestinal disorders of the critically ill. bacterial translocation in the gut. Best Pract Res Clin Gastroenterol (2003) 17(3):397–425. doi: 10.1016/S1521-6918(03)00024-6
143. Baumgart DC, Dignass AU. Intestinal barrier function. Curr Opin Clin Nutr Metab Care (2002) 5(6):685–94. doi: 10.1097/00075197-200211000-00012
144. Wang YH. Current progress of research on intestinal bacterial translocation. Microb Pathog (2021) 152:104652. doi: 10.1016/j.micpath.2020.104652
145. Luyer MD, Buurman WA, Hadfoune M, Speelmans G, Knol J, Jacobs JA, et al. Strain-specific effects of probiotics on gut barrier integrity following hemorrhagic shock. Infect Immun (2005) 73(6):3686–92. doi: 10.1128/IAI.73.6.3686-3692.2005
146. Poole NM, Green SI, Rajan A, Vela LE, Zeng XL, Estes MK, et al. Role for FimH in extraintestinal pathogenic escherichia coli invasion and translocation through the intestinal epithelium. Infect Immun (2017) 85(11):e00581-17. doi: 10.1128/IAI.00581-17
147. Cossart, Sansonetti PJ. Bacterial invasion: the paradigms of enteroinvasive pathogens. Science (2004) 304(5668):242–8. doi: 10.1126/science.1090124
148. Penalva JC, Martínez J, Laveda R, Esteban A, Muñoz C, Sáez J, et al. A study of intestinal permeability in relation to the inflammatory response and plasma endocab IgM levels in patients with acute pancreatitis. J Clin Gastroenterol (2004) 38(6):512–7. doi: 10.1097/01.mcg.0000129060.46654.e0
149. Gianotti L, Munda R, Alexander JW, Tchervenkov JI, Babcock GF. Bacterial translocation: a potential source for infection in acute pancreatitis. Pancreas (1993) 8(5):551–8. doi: 10.1097/00006676-199309000-00004
150. Ou T, Zhou Z, Turner DP, Zhu B, Lilly M, Jiang W. Increased preoperative plasma level of microbial 16S rDNA translocation is associated with relapse after prostatectomy in prostate cancer patients. Front Oncol (2019) 9:1532. doi: 10.3389/fonc.2019.01532
151. Nagpal R, Yadav H. Bacterial translocation from the gut to the distant organs: an overview. Ann Nutr Metab (2017) 71 Suppl 1:11–6. doi: 10.1159/000479918
152. Rueter C, Bielaszewska M. Secretion and delivery of intestinal pathogenic escherichia coli virulence factors via outer membrane vesicles. Front Cell Infect Microbiol (2020) 10:91. doi: 10.3389/fcimb.2020.00091
153. Senthil M, Watkins A, Barlos D, Xu DZ, Lu Q, Abungu B, et al. Intravenous injection of trauma-hemorrhagic shock mesenteric lymph causes lung injury that is dependent upon activation of the inducible nitric oxide synthase pathway. Ann Surg (2007) 246(5):822–30. doi: 10.1097/SLA.0b013e3180caa3af
154. Deitch EA. Gut lymph and lymphatics: a source of factors leading to organ injury and dysfunction. Ann N Y Acad Sci (2010) 1207 Suppl 1:E103–11. doi: 10.1111/j.1749-6632.2010.05713.x
155. Carrico CJ, Meakins JL, Marshall JC, Fry D, Maier RV. Multiple-organ-failure syndrome. Arch Surg (1986) 121(2):196–208. doi: 10.1001/archsurg.1986.01400020082010
156. Ferraro FJ, Rush BF Jr, Simonian GT, Bruce CJ, Murphy TF, Hsieh JT, et al. A comparison of survival at different degrees of hemorrhagic shock in germ-free and germ-bearing rats. Shock (1995) 4(2):117–20. doi: 10.1097/00024382-199508000-00007
157. Dominguez JA, Coopersmith CM. Can we protect the gut in critical illness? the role of growth factors and other novel approaches. Crit Care Clin (2010) 26(3):549–65. doi: 10.1016/j.ccc.2010.04.005
158. Frazier TH, DiBaise JK, McClain CJ. Gut microbiota, intestinal permeability, obesity-induced inflammation, and liver injury. JPEN J Parenter Enteral Nutr (2011) 35(5 Suppl):14S–20S. doi: 10.1177/0148607111413772
159. Ding S, Chi MM, Scull BP, Rigby R, Schwerbrock NM, Magness S, et al. High-fat diet: bacteria interactions promote intestinal inflammation which precedes and correlates with obesity and insulin resistance in mouse. PloS One (2010) 5(8):e12191. doi: 10.1371/journal.pone.0012191
160. Lin PH, Howard L, Freedland SJ. Weight loss via a low-carbohydrate diet improved the intestinal permeability marker, zonulin, in prostate cancer patients. Ann Med (2022) 54(1):1221–5. doi: 10.1080/07853890.2022.2069853
161. Iida N, Dzutsev A, Stewart CA, Smith L, Bouladoux N, Weingarten RA, et al. Commensal bacteria control cancer response to therapy by modulating the tumor microenvironment. Science (2013) 342(6161):967–70. doi: 10.1126/science.1240527
162. Alexander JL, Wilson ID, Teare J, Marchesi JR, Nicholson JK, Kinross JM. Gut microbiota modulation of chemotherapy efficacy and toxicity. Nat Rev Gastroenterol Hepatol (2017) 14(6):356–65. doi: 10.1038/nrgastro.2017.20
163. Di Modica M, Gargari G, Regondi V, Bonizzi A, Arioli S, Belmonte B, et al. Gut microbiota condition the therapeutic efficacy of trastuzumab in HER2-positive breast cancer. Cancer Res (2021) 81(8):2195–206. doi: 10.1158/0008-5472.CAN-20-1659
164. Sivan A, Corrales L, Hubert N, Williams JB, Aquino-Michaels K, Earley ZM, et al. Commensal bifidobacterium promotes antitumor immunity and facilitates anti-PD-L1 efficacy. Science (2015) 350(6264):1084–9. doi: 10.1126/science.aac4255
165. Vande Voorde J, Vervaeke P, Liekens S, Balzarini J. Mycoplasma hyorhinis-encoded cytidine deaminase efficiently inactivates cytosine-based anticancer drugs. FEBS Open Bio (2015) 5:634–9. doi: 10.1016/j.fob.2015.07.007
166. Routy B, Le Chatelier E, Derosa L, Duong CPM, Alou MT, Daillère R, et al. Gut microbiome influences efficacy of PD-1-based immunotherapy against epithelial tumors. Science (2018) 359(6371):91–7. doi: 10.1126/science.aan3706
167. Liu Q, Lu Y, Xiao Y, Yuan L, Hu D, Hao Y, et al. Effects of docetaxel injection and docetaxel micelles on the intestinal barrier and intestinal microbiota. Adv Sci (Weinh) (2021) 8(24):e2102952. doi: 10.1002/advs.202102952
168. Zhong W, Wu K, Long Z, Zhou X, Zhong C, Wang S, et al. Gut dysbiosis promotes prostate cancer progression and docetaxel resistance via activating NF-kappaB-IL6-STAT3 axis. Microbiome (2022) 10(1):94. doi: 10.1186/s40168-022-01289-w
169. Chalabi M, Cardona A, Nagarkar DR, Dhawahir Scala A, Gandara DR, Rittmeyer A, et al. Efficacy of chemotherapy and atezolizumab in patients with non-small-cell lung cancer receiving antibiotics and proton pump inhibitors: pooled post hoc analyses of the OAK and POPLAR trials. Ann Oncol (2020) 31(4):525–31. doi: 10.1016/j.annonc.2020.01.006
170. Xu X, Zhang X. Effects of cyclophosphamide on immune system and gut microbiota in mice. Microbiol Res (2015) 171:97–106. doi: 10.1016/j.micres.2014.11.002
171. Viaud S, Saccheri F, Mignot G, Yamazaki T, Daillère R, Hannani D, et al. The intestinal microbiota modulates the anticancer immune effects of cyclophosphamide. Science (2013) 342(6161):971–6. doi: 10.1126/science.1240537
172. Montassier E, Gastinne T, Vangay P, Al-Ghalith GA, Bruley des Varannes S, Massart S, et al. Chemotherapy-driven dysbiosis in the intestinal microbiome. Aliment Pharmacol Ther (2015) 42(5):515–28. doi: 10.1111/apt.13302
173. Daillere R, Vétizou M, Waldschmitt N, Yamazaki T, Isnard C, Poirier-Colame V, et al. Enterococcus hirae and barnesiella intestinihominis facilitate cyclophosphamide-induced therapeutic immunomodulatory effects. Immunity (2016) 45(4):931–43. doi: 10.1016/j.immuni.2016.09.009
174. Cimadamore A, Santoni M, Massari F, Gasparrini S, Cheng L, Lopez-Beltran A, et al. Microbiome and cancers, with focus on genitourinary tumors. Front Oncol (2019) 9:178. doi: 10.3389/fonc.2019.00178
175. Daisley BA, Chanyi RM, Abdur-Rashid K, Al KF, Gibbons S, Chmiel JA, et al. Abiraterone acetate preferentially enriches for the gut commensal akkermansia muciniphila in castrate-resistant prostate cancer patients. Nat Commun (2020) 11(1):4822. doi: 10.1038/s41467-020-18649-5
176. Patterson E, Ryan PM, Cryan JF, Dinan TG, Ross RP, Fitzgerald GF, et al. Gut microbiota, obesity and diabetes. Postgrad Med J (2016) 92(1087):286–300. doi: 10.1136/postgradmedj-2015-133285
177. Xu F, Fu Y, Sun TY, Jiang Z, Miao Z, Shuai M, et al. The interplay between host genetics and the gut microbiome reveals common and distinct microbiome features for complex human diseases. Microbiome (2020) 8(1):145. doi: 10.1186/s40168-020-00923-9
178. Schwabe RF, Greten TF. Gut microbiome in HCC - mechanisms, diagnosis and therapy. J Hepatol (2020) 72(2):230–8. doi: 10.1016/j.jhep.2019.08.016
179. Yu J, Feng Q, Wong SH, Zhang D, Liang QY, Qin Y, et al. Metagenomic analysis of faecal microbiome as a tool towards targeted non-invasive biomarkers for colorectal cancer. Gut (2017) 66(1):70–8. doi: 10.1136/gutjnl-2015-309800
180. Zhang Y, Shen J, Shi X, Du Y, Niu Y, Jin G, et al. Gut microbiome analysis as a predictive marker for the gastric cancer patients. Appl Microbiol Biotechnol (2021) 105(2):803–14. doi: 10.1007/s00253-020-11043-7
181. Backhed F, Ley RE, Sonnenburg JL, Peterson DA, Gordon JI. Host-bacterial mutualism in the human intestine. Science (2005) 307(5717):1915–20. doi: 10.1126/science.1104816
182. Shin NR, Whon TW, Bae JW. Proteobacteria: microbial signature of dysbiosis in gut microbiota. Trends Biotechnol (2015) 33(9):496–503. doi: 10.1016/j.tibtech.2015.06.011
183. Fruge AD, Ptacek T, Tsuruta Y, Morrow CD, Azrad M, Desmond RA, et al. Dietary changes impact the gut microbe composition in overweight and obese men with prostate cancer undergoing radical prostatectomy. J Acad Nutr Diet (2018) 118(4):714–723 e1. doi: 10.1016/j.jand.2016.10.017
184. Perez-Burillo S, avajas-Porras B, López-Maldonado A, Hinojosa-Nogueira D, Pastoriza S, Rufián-Henares JÁ, et al. Green tea and its relation to human gut microbiome. Molecules (2021) 26(13):3907. doi: 10.3390/molecules26133907
185. da Silva HB, Amaral EP, Nolasco EL, de Victo NC, Atique R, Jank CC, et al. Dissecting major signaling pathways throughout the development of prostate cancer. Prostate Cancer (2013) 2013:920612. doi: 10.1155/2013/920612
186. Toren, Zoubeidi A. Targeting the PI3K/Akt pathway in prostate cancer: challenges and opportunities (review). Int J Oncol (2014) 45(5):1793–801. doi: 10.3892/ijo.2014.2601
187. Culig Z, Santer FR. Androgen receptor signaling in prostate cancer. Cancer Metastasis Rev (2014) 33(2-3):413–27. doi: 10.1007/s10555-013-9474-0
188. Harper CE, Patel BB, Wang J, Eltoum IA, Lamartiniere CA. Epigallocatechin-3-Gallate suppresses early stage, but not late stage prostate cancer in TRAMP mice: mechanisms of action. Prostate (2007) 67(14):1576–89. doi: 10.1002/pros.20643
189. Siddiqui IA, Asim M, Hafeez BB, Adhami VM, Tarapore RS, Mukhtar H. Green tea polyphenol EGCG blunts androgen receptor function in prostate cancer. FASEB J (2011) 25(4):1198–207. doi: 10.1096/fj.10-167924
190. Adhami VM, Siddiqui IA, Ahmad N, Gupta S, Mukhtar H. Oral consumption of green tea polyphenols inhibits insulin-like growth factor-i-induced signaling in an autochthonous mouse model of prostate cancer. Cancer Res (2004) 64(23):8715–22. doi: 10.1158/0008-5472.CAN-04-2840
191. Adhami VM, Siddiqui IA, Syed DN, Lall RK, Mukhtar H. Oral infusion of pomegranate fruit extract inhibits prostate carcinogenesis in the TRAMP model. Carcinogenesis (2012) 33(3):644–51. doi: 10.1093/carcin/bgr308
192. Kurahashi N, Sasazuki S, Iwasaki M, Inoue M, Tsugane S, JPHC Study Group. Green tea consumption and prostate cancer risk in Japanese men: a prospective study. Am J Epidemiol (2008) 167(1):71–7. doi: 10.1093/aje/kwm249
193. Kumar NB, Hogue S, Pow-Sang J, Poch M, Manley BJ, Li R, et al. Effects of green tea catechins on prostate cancer chemoprevention: the role of the gut microbiome. Cancers (Basel) (2022) 14(16):3988. doi: 10.3390/cancers14163988
194. Seeram NP, Henning SM, Zhang Y, Suchard M, Li Z, Heber D. Pomegranate juice ellagitannin metabolites are present in human plasma and some persist in urine for up to 48 hours. J Nutr (2006) 136(10):2481–5. doi: 10.1093/jn/136.10.2481
195. Mertens-Talcott SU, Jilma-Stohlawetz P, Rios J, Hingorani L, Derendorf H. Absorption, metabolism, and antioxidant effects of pomegranate (Punica granatum l.) polyphenols after ingestion of a standardized extract in healthy human volunteers. J Agric Food Chem (2006) 54(23):8956–61. doi: 10.1021/jf061674h
196. Seeram NP, Aronson WJ, Zhang Y, Henning SM, Moro A, Lee RP, et al. Pomegranate ellagitannin-derived metabolites inhibit prostate cancer growth and localize to the mouse prostate gland. J Agric Food Chem (2007) 55(19):7732–7. doi: 10.1021/jf071303g
197. Malik A, Afaq S, Shahid M, Akhtar K, Assiri A. Influence of ellagic acid on prostate cancer cell proliferation: a caspase-dependent pathway. Asian Pac J Trop Med (2011) 4(7):550–5. doi: 10.1016/S1995-7645(11)60144-2
198. Martinez VG, O'Connor R, Liang Y, Clynes M. CYP1B1 expression is induced by docetaxel: effect on cell viability and drug resistance. Br J Cancer (2008) 98(3):564–70. doi: 10.1038/sj.bjc.6604195
199. Kasimsetty SG, Bialonska D, Reddy MK, Thornton C, Willett KL, Ferreira D. Effects of pomegranate chemical constituents/intestinal microbial metabolites on CYP1B1 in 22Rv1 prostate cancer cells. J Agric Food Chem (2009) 57(22):10636–44. doi: 10.1021/jf902716r
200. Narayanan BA, Geoffroy O, Willingham MC, Re GG, Nixon DW. p53/p21(WAF1/CIP1) expression and its possible role in G1 arrest and apoptosis in ellagic acid treated cancer cells. Cancer Lett (1999) 136(2):215–21. doi: 10.1016/S0304-3835(98)00323-1
201. Adlercreutz H. Lignans and human health. Crit Rev Clin Lab Sci (2007) 44(5-6):483–525. doi: 10.1080/10408360701612942
202. Damdimopoulou P, Nurmi T, Salminen A, Damdimopoulos AE, Kotka M, van der Saag P, et al. A single dose of enterolactone activates estrogen signaling and regulates expression of circadian clock genes in mice. J Nutr (2011) 141(9):1583–9. doi: 10.3945/jn.111.140277
203. Atkinson C, Frankenfeld CL, Lampe JW. Gut bacterial metabolism of the soy isoflavone daidzein: exploring the relevance to human health. Exp Biol Med (Maywood) (2005) 230(3):155–70. doi: 10.1177/153537020523000302
204. Rabiau N, Kossaï M, Braud M, Chalabi N, Satih S, Bignon YJ, et al. Genistein and daidzein act on a panel of genes implicated in cell cycle and angiogenesis by polymerase chain reaction arrays in human prostate cancer cell lines. Cancer Epidemiol (2010) 34(2):200–6. doi: 10.1016/j.canep.2009.12.018
205. Nakamura H, Wang Y, Xue H, Romanish MT, Mager DL, Helgason CD, et al. Genistein versus ICI 182, 780: an ally or enemy in metastatic progression of prostate cancer. Prostate (2013) 73(16):1747–60. doi: 10.1002/pros.22712
206. Park SY, Murphy SP, Wilkens LR, Henderson BE, Kolonel LN;, Multiethnic Cohort Study. Legume and isoflavone intake and prostate cancer risk: the multiethnic cohort study. Int J Cancer (2008) 123(4):927–32. doi: 10.1002/ijc.23594
207. Mahmoud AM, Yang W, Bosland MC. Soy isoflavones and prostate cancer: a review of molecular mechanisms. J Steroid Biochem Mol Biol (2014) 140:116–32. doi: 10.1016/j.jsbmb.2013.12.010
208. Tsuji H, Moriyama K, Nomoto K, Miyanaga N, Akaza H. Isolation and characterization of the equol-producing bacterium slackia sp. strain NATTS. Arch Microbiol (2010) 192(4):279–87. doi: 10.1007/s00203-010-0546-z
209. Kurahashi N, Iwasaki M, Inoue M, Sasazuki S, Tsugane S. Plasma isoflavones and subsequent risk of prostate cancer in a nested case-control study: the Japan public health center. J Clin Oncol (2008) 26(36):5923–9. doi: 10.1200/JCO.2008.16.8807
210. Tanaka M, Fujimoto K, Chihara Y, Torimoto K, Yoneda T, Tanaka N, et al. Isoflavone supplements stimulated the production of serum equol and decreased the serum dihydrotestosterone levels in healthy male volunteers. Prostate Cancer Prostatic Dis (2009) 12(3):247–52. doi: 10.1038/pcan.2009.10
211. Jacobsen BK, Knutsen SF, Fraser GE. Does high soy milk intake reduce prostate cancer incidence? the adventist health study (United states). Cancer Causes Control (1998) 9(6):553–7. doi: 10.1023/A:1008819500080
212. Akaza H. Prostate cancer chemoprevention by soy isoflavones: role of intestinal bacteria as the "second human genome". Cancer Sci (2012) 103(6):969–75. doi: 10.1111/j.1349-7006.2012.02257.x
213. Cohen JH, Kristal AR, Stanford JL. Fruit and vegetable intakes and prostate cancer risk. J Natl Cancer Inst (2000) 92(1):61–8. doi: 10.1093/jnci/92.1.61
214. Wu Y, Li RW, Huang H, Fletcher A, Yu L, Pham Q, et al. Inhibition of tumor growth by dietary indole-3-Carbinol in a prostate cancer xenograft model may be associated with disrupted gut microbial interactions. Nutrients (2019) 11(2):467. doi: 10.3390/nu11020467
215. Seidel DV, Azcárate-Peril MA, Chapkin RS, Turner ND. Shaping functional gut microbiota using dietary bioactives to reduce colon cancer risk. Semin Cancer Biol (2017) 46:191–204. doi: 10.1016/j.semcancer.2017.06.009
216. Conlon MA, Bird AR. The impact of diet and lifestyle on gut microbiota and human health. Nutrients (2014) 7(1):17–44. doi: 10.3390/nu7010017
217. Manfredi C, Calace FP, Fusco F, Quattrone C, Giordano D, Crocetto F, et al. Escherichia coli nissle 1917 as adjuvant therapy in patients with chronic bacterial prostatitis: a non-blinded, randomized, controlled trial. World J Urol (2021) 39(12):4373–9. doi: 10.1007/s00345-021-03773-8
218. Poutahidis T, Springer A, Levkovich T, Qi P, Varian BJ, Lakritz JR, et al. Probiotic microbes sustain youthful serum testosterone levels and testicular size in aging mice. PloS One (2014) 9(1):e84877. doi: 10.1371/journal.pone.0084877
219. Vivarelli S, Salemi R, Candido S, Falzone L, Santagati M, Stefani S, et al. Gut microbiota and cancer: from pathogenesis to therapy. Cancers (Basel) (2019) 11(1):38. doi: 10.3390/cancers11010038
220. Horinaka M, Yoshida T, Kishi A, Akatani K, Yasuda T, Kouhara J, et al. Lactobacillus strains induce TRAIL production and facilitate natural killer activity against cancer cells. FEBS Lett (2010) 584(3):577–82. doi: 10.1016/j.febslet.2009.12.004
221. Wu Q, Tian AL, Li B, Leduc M, Forveille S, Hamley P, et al. IGF1 receptor inhibition amplifies the effects of cancer drugs by autophagy and immune-dependent mechanisms. J Immunother Cancer (2021) 9(6):e002722. doi: 10.1136/jitc-2021-002722
222. Woelk CH, Snyder A. Modulating gut microbiota to treat cancer. Science (2021) 371(6529):573–4. doi: 10.1126/science.abg2904
223. Bakken JS, Borody T, Brandt LJ, Brill JV, Demarco DC, Franzos MA, et al. Treating clostridium difficile infection with fecal microbiota transplantation. Clin Gastroenterol Hepatol (2011) 9(12):1044–9. doi: 10.1016/j.cgh.2011.08.014
224. Baruch EN, Youngster I, Ben-Betzalel G, Ortenberg R, Lahat A, Katz L, et al. Fecal microbiota transplant promotes response in immunotherapy-refractory melanoma patients. Science (2021) 371(6529):602–9. doi: 10.1126/science.abb5920
Keywords: prostate cancer, intestinal microorganisms, the gut microbiome, microbial metabolites, immune, tumor marker
Citation: Zha C, Peng Z, Huang K, Tang K, Wang Q, Zhu L, Che B, Li W, Xu S, Huang T, Yu Y and Zhang W (2023) Potential role of gut microbiota in prostate cancer: immunity, metabolites, pathways of action? Front. Oncol. 13:1196217. doi: 10.3389/fonc.2023.1196217
Received: 29 March 2023; Accepted: 04 May 2023;
Published: 17 May 2023.
Edited by:
Alfonso Urbanucci, Oslo University Hospital, NorwayReviewed by:
Natasha Kyprianou, Icahn School of Medicine at Mount Sinai, United StatesLi Zhang, University of Minnesota Twin Cities, United States
Copyright © 2023 Zha, Peng, Huang, Tang, Wang, Zhu, Che, Li, Xu, Huang, Yu and Zhang. This is an open-access article distributed under the terms of the Creative Commons Attribution License (CC BY). The use, distribution or reproduction in other forums is permitted, provided the original author(s) and the copyright owner(s) are credited and that the original publication in this journal is cited, in accordance with accepted academic practice. No use, distribution or reproduction is permitted which does not comply with these terms.
*Correspondence: Kaifa Tang, doc.tangkf@hotmail.com; Qiang Wang, gymnwq@163.com
† These authors have contributed equally to this work