- 1Department of Gastrointestinal Surgery, the Affiliated Hospital of Qingdao University, Qingdao, Shandong, China
- 2Department of Integrated Chinese and Western Medicine, Yantai Yuhuangding Hospital, Yantai, Shandong, China
Transforming growth factor-beta (TGF-β) has long been known to be associated with early embryonic development and organogenesis, immune supervision, and tissue repair and homeostasis in adults. TGF-β has complex roles in fibrosis and cancer that may be opposing at different stages of these diseases. Under pathological conditions, overexpression of TGF-β causes epithelial–mesenchymal transition, deposition of extracellular matrix, and formation of cancer-associated fibroblasts, leading to fibrotic disease or cancer. Fibroblasts, epithelial cells, and immune cells are the most common targets of TGF-β, while fibrosis and cancer are the most common TGF-β-associated diseases. Given the critical role of TGF-β and its downstream molecules in fibrosis and progression of cancer, therapies targeting TGF-β signaling appear to be a promising strategy. Preclinical and clinical studies have investigated therapies targeting TGF-β, including antisense oligonucleotides, monoclonal antibodies, and ligand traps. However, development of targeted TGF-β therapy has been hindered by systemic cytotoxicity. This review discusses the molecular mechanisms of TGF-β signaling and highlights targeted TGF-β therapy for cancer and fibrosis as a therapeutic strategy for related diseases.
1 Background
Transforming growth factor-beta (TGF-β) is a multifunctional polypeptide cytokine that belongs to the TGF-β superfamily and plays an important role in multiple biological processes, including cell growth, differentiation, apoptosis, the immune response, and wound healing (1, 2). Accurate TGF-β signaling is essential for normal function and homeostasis in humans, and abnormalities of TGF-β can lead to a variety of diseases (2). However, TGF-β has dual functionality. In normal cells, TGF-β halts the cell cycle in G1 phase and can inhibit cell proliferation and promote apoptosis (3). In contrast, cancer cells become resistant to inhibition by TGF-β signaling through mutations or epigenetic modifications. In the tumor microenvironment (TME), TGF-β contributes to tumor growth, invasion, and spread, and advanced tumors produce an excessive amount of TGF-β that promotes proliferation of tumor cells, vascular cells, immune cells, and fibroblasts (4–7). These processes convert TGF-β into a tumor-promoting agonist as the disease progresses. TGF-β functions as a tumor promoter by stimulating tumor cells to undergo what is known as epithelial-mesenchymal transition (EMT). EMT can activate angiogenesis and cancer-related fibroblasts and enable tumors to evade inhibitory immune responses, further promoting growth and progression of cancer (8).
In recent years, researchers have found that inactivating the TGF-β signaling pathway in CD4+ cells leads to increased production of interleukin-4 by T helper 2 cells in mouse models of breast cancer and regression of the disease (9, 10). Given the important role of TGF-β in human fibrosis and cancer, anti-TGF-β approaches have been used to treat several diseases, including melanoma, colorectal cancer, and breast cancer (11–13). A growing body of preclinical and clinical data suggests that blocking TGF-β signaling is an effective treatment for cancer and fibrosis. This review focuses on the latest advances in use of TGF-β inhibitors for therapeutic management of these diseases. (see Figure 1).
2 TGF-β signaling in cancer and fibrosis
TGF-β is a multifunctional polypeptide cytokine and has three mammalian subtypes, namely, TGF-β1, TGF-β2, and TGF-β3, which are encoded by different genes but act through the same signaling system (14–16). During the synthesis of TGF-β in cell, TGF-β precursors are hydrolyzed by the endopeptidase furin in the Golgi apparatus and then transported to and bound to the extracellular matrix (ECM) together with the latency-associated peptide (LAP) in the amino terminal region (17–19). In the extracellular space, the LAP/TGF-β complex can be cleaved by a variety of proteases to release active TGF-β, including plasmin, matrix metalloproteinase (MMP) 2, and MMP9 (20, 21).TGF-β influences multiple processes, including cell growth and differentiation, apoptosis, cell motility, angiogenesis, and the immune response (22–25). These effects are heavily dependent on the TME, including tumor hypoxia. This pleiotropy is manifested in the following process: TGF-β is released from ECM. Subsequently, it binds to the transmembrane TGF-β receptor type II (TβRII) on the target cell and recruits the type I receptor (TβRI) to exert its function. The phosphorylation of type I receptor by type II receptor and subsequent phosphorylation of R-SMAD is a central feature of the TGF-β family of ligand signaling mechanisms (26, 27). TβRII is transphosphorylated, activated, and signals through phosphorylation of the SMAD 2/3 protein. In the process of signal transmission, auxiliary receptors facilitate or inhibit signal transmission through TGF-β receptors. Among them, endoglin can promote signal transmission to regulate angiogenesis, while BAMBI interferes with the signaling of receptor complexes, leading to the association of BAMBI expression with various human diseases, including cancer and tissue fibrosis (28–31). The key regulatory proteins in the TGF-β intracellular signaling axis mainly include the SMAD protein family, such as SMAD2 and SMAD3, which are receptor-regulated SMADs. Phosphorylation allows SMAD 2/3 to form heteromeric complexes with SMAD 4 proteins and translocate into the nucleus. By binding to transcription factors, SMAD complexes affect mRNA splicing, stability, and translation through RNA-binding proteins (RBPs) and non-coding RNAs (ncRNAs) (32, 33). SMAD can also control the maturation process of miRNAs. In addition, TGF-β/SMAD signaling can modulate the expression of several long non-coding RNAs (lncRNAs) that act as mediators of TGF-β reactions (34). R-Smads and Smad4 are not highly regulated by extracellular signaling in most cell types (35). Ubiquitin-proteasome-mediated degradation controls the levels of post-translational Smads, and E3 ubiquitin ligase, Smurf1, and Smurf2 antagonize TGF-β conduction by interacting with and targeting R-Smad (36). Following TGF-β signaling, phosphorylated Smad2 or Smad3 can form stable complexes with Smurf2. Thus, the majority of nucleus Smad2 or Smad3 is not a target for degradation, but is dephosphorylated and relocalized into the cytoplasm (37–39). In contrast to R-Smads, Smad4 is not normally subject to ubiquitin-mediated degradation, and some tumor-associated mutations allow ubiquitination or reduce the stability of Smad4 (40, 41). The initiation and propagation of TGF-β signaling are counteracted by the activities of SMAD6 and SMAD7, which are inhibitory SMADs (I-SMAD) (42). SMAD7 antagonizes the TGF-β pathway by recruiting the E3 ubiquitin ligases SMURF1 and SMURF2 to the type I receptor, promoting its ubiquitination and subsequent degradation, making it a more effective inhibitor of TGF-β signaling than SMAD6, which preferentially inhibits BMP signaling (43, 44). Although there is a typical SMAD-dependent pathway, TGF-β also initiates SMAD-independent signal transduction. Research indicates that ERK activation occurs in cholesterol-rich lipid rafts in human keratinocytes. The regulation of JNK and p38 MAP kinase pathways is crucial in inflammation, cell differentiation, and apoptosis, where TGF-β-activated kinase 1 (TAK1) also serves as a classic inhibitory regulator of TGF-β signaling (45, 46). The MAPK and phosphoinositide 3-kinase pathways also have potent agonists. Similarly, stress sensors regulate the activation of p38 MAPK and c-Jun amino-terminal kinase, while tumor necrosis factor, interleukin-1, and Toll-like receptors control activation of TAK1. Therefore, the role of TGF-β in the regulation of these pathways under normal physiological conditions and in disease remains difficult to determine.
Dysregulation of TGF-β signaling is associated with many pathological processes, including tumor progression and fibrosis (47). In advanced malignant disease, tumor cells undergo genetic and/or epigenetic changes that gradually render them insensitive to TGF-β by weakening the pathway via which TGF-β inhibits their growth and accumulate mutations in the TGF-β signaling cascade that allow them to evade the antitumor surveillance activity of TGF-β. Examples of such escape include SMAD4 mutations in gastric cancer and TβRI mutations in colon cancer, where loss of the functional mutant component of TGF-β is not sufficient to cause tumorigenesis but promotes transformation of precancerous cells to a more malignant phenotype (48–51). In the later stages of tumor development, TGF - β, through the secreted TGF - β protein, plays a multifaceted role. It stimulates cell proliferation, triggers the formation of new blood vessels, and strengthens the immunosuppressive state of the tumor (52). The carcinogenic events that lead to TGF-β switching are diverse. For example, co-activation of KRAS, inactivation of SMAD4, and changes in the CDKN2A genome lead to rapid transformation of non-invasive pancreatic intraepithelial neoplasms into invasive pancreatic ductal adenocarcinomas, a large number of which are related to tumor stage, with the SMAD4 inactivation rate in high-grade tumors reaching up to 31% (53–56). TGF-β increases the ability of cells to migrate and invade neighboring tissues via EMT, allowing epithelial cells to change from a cuboidal shape to an elongated spindle shape and an invasive phenotype (57). During EMT, epithelial cells lose E-cadherin and zonula occludens-1 proteins from the plasma membrane and upregulate their expression of vimentin, fibronectin, and N-cadherin, thereby increasing their mobility (58, 59). Studies in cultured cells and mice have shown that remodeling of the ECM can release the underlying TGF-β complex and interact with inflammatory mediators, resulting in a stiffer ECM, and also increased metastasis of hepatocellular carcinoma in rats (60). Furthermore, the TGF-β-regulated immunosuppressive microenvironment indirectly promotes tumor escape, inhibits natural killer cells, and regulates the proliferation of macrophages, antigen-presenting dendritic cells, and granulocytes to control the development and function of the innate immune system (61–63). Deletion or mutations of SMAD4 in NK cells leads to impairment of NK cell homeostasis, and NK cell immune surveillance against metastases (64).
In addition to its effects on tumor development, TGF-β-induced EMT leads to fibrosis in the tissues of many organs, including the lungs and liver (65). Fibrosis involves necrosis of parenchymal cells and excessive deposition of ECM, which results in connective tissue hyperplasia, fibrosis, and eventually organ failure. Fibrosis also entails conversion of fibroblasts into cancer-associated fibroblasts (CAFs) (66). All three isoforms of TGF-β have fibrotic effects, and TGF-β is considered a major factor in both classical and non-classical signaling pathways in fibrosis, affecting the liver, kidney, and lung (67–69). Dysregulation of TGF-β leads to excessive deposition of ECM, which promotes pathological fibrosis and tumorigenesis and is also associated with migration of tumor cells. Furthermore, studies in zebrafish have shown that breast and prostate cancers promote tissue fibrosis and migration of cancer cells in the unique microenvironment provided by TGF-β produced in CAFs (70–72). Other studies have demonstrated a strong association between pulmonary fibrosis and an increased risk of lung cancer. This pro-fibrotic state stimulates increased expression of TGF-β, which is followed by crosstalk between or activation of downstream pathways, such as SMAD3 or other atypical pathways, leading to resistance of cancer cells to apoptosis and progression of cancer via CAFs (68, 73, 74). Abnormal accumulation of ECM triggers fibrotic and immunosuppressive processes by linking SMAD4, BRAF, and TP53 mutations with MYC amplification and produces a CAF phenotype (66, 75–78). In breast cancer, TGF-β not only promotes tumor growth but also forms a stable breast cancer stem cell phenotype by changing the metabolic reprogramming of CAFs. EMT of breast cancer cells is regulated by a TGF-β/SMAD-dependent pathway and activated by tumor necrosis factor-alpha/nuclear factor-kappa B/Twist, promoting metastasis (79, 80). Calon A et al. found that all poor prognostic features in colorectal cancer patients had elevated TGF-β expression, and in colon cancer, CAF produced TGF-β, which in turn activated the gene expression program in CAF. On the other hand, TGF-β in CAFs improves the tumor-initiating capacity of colorectal cancer cells, even in patients classified as having a good prognosis, and if CAF levels are elevated, this property increases metastatic potential and the ability to regenerate disease after treatment (81–84).
3 Strategies for inhibition of TGF-β
Given that the mechanisms of tissue fibrosis and tumorigenesis are inextricably linked and TGF-β signaling plays an important role in both conditions, inhibition of TGF-β is an area of intense interest in cancer research. Inhibitors of TGF-β signal transduction have been investigated in preclinical studies and are divided into five types according to whether their mechanism of action entails blocking the synthesis of TGF-β, blocking the TGF-β ligand, blocking activation of latent TGF-β, blocking the TGF-β receptor, or blocking intracellular signal transduction (85).
3.1 Blocking synthesis of TGF-β
Transcription and translation, two crucial steps in gene expression, are key to converting genetic information into the protein - based material essential for biological functions. One strategy is to use antisense oligonucleotides, which are complementary to mRNA targets and generate single-stranded deoxyribonucleotides. One such agent is trabedersen (AP-12009), an 18-polymeric thiophosphate-modified antisense oligodeoxynucleotide that is complementary to TGF-β2 mRNA (86). Developed by Antisense Pharma GmbH (Regensburg, Germany), trabedersen is specifically designed for clinical use in patients with highly aggressive TGF-β2-overexpressing tumors, such as malignant melanoma and high-grade glioma. In a cell line established from a patient with high-grade glioma, trabedersen led to a significant reduction in synthesis of TGF-β2 protein and inhibited cell proliferation and migration. Trabedersen was also well tolerated in a safety study by Schlingensiepen et al. in animals (87, 88). In a Phase I/II study, seven patients achieved stable disease and two achieved complete remission (88, 89). These encouraging results led to a Phase IIb clinical trial (NCT00431561) in which the efficacy and safety of trabedersen were assessed in patients. In this study, trabedersen was well tolerated with no serious adverse events and performed better than conventional chemotherapy in terms of median survival. At present, large Phase III trials of trabedersen are underway in patients with high-grade glioma. Phase I trials of this agent have also been initiated in patients with pancreatic cancer and colon cancer after it was found to inhibit the proliferation and migration of pancreatic cancer cells in mice (90, 91). ISTH0036 is an antisense oligonucleotide developed by Isarna Therapeutics GmbH (Munich, Germany) that targets TGF-β2 mRNA. Pfeiffer et al. evaluated the ability of this agent to inhibit fibrosis after glaucoma filtration surgery in a Phase I clinical trial and confirmed its safety and potential antifibrotic activity (92). However, there are challenges in the development of antisense oligonucleotides as a therapeutic target, including off-target effects, delivery to target tissues, and RNA-binding affinity.
3.2 Blocking the TGF-β ligand
Therapies that block the TGF-β pathway have been successfully developed for a variety of cancers. Neutralizing antibodies are the preferred approach because they bind directly to the ligand and block into the receptor. Thus far, three drug candidates have been investigated in clinical trials by Cambridge Antibody Technology (Cambridge, UK), namely, lerdelimumab (CAT-152), metelimumab (CAT-192) and fresolimumab (CAT-193) (93, 94). Blocking TGF-β ligands from binding to the receptor by using ligand traps or neutralizing antibodies is a promising strategy in cancer therapy. Among the neutralizing antibodies, fresolimumab (GC1008) is a human monoclonal antibody designed to neutralize all three subtypes of TGF-β and is the most widely investigated agent in this class (95). Soluble TGF-β receptors are another effective strategy for blocking binding of TGF-β ligands to cell receptors. The Phase I clinical study of AVID200 is currently in progress (96). Chen et al. discovered that in a mouse model of pancreatic ductal adenocarcinoma (PDAC), AVID200 is capable of regulating the heterogeneity of cancer - associated fibroblasts (CAFs) and significantly decreasing tumor metastasis to the liver (97).
3.2.1 Studies in cancer
Fresolimumab has been investigated as a pan-TGF-β neutralizing antibody in patients with advanced melanoma and renal cell carcinoma, some of whom achieved stable disease or remission (98–100). A preclinical study in a mouse model of 4T1 breast cancer showed that use of 1D11 (Genzyme Corporation, Cambridge, MA, USA), another pan-TGF-β neutralizing antibody, slowed tumor growth, particularly when combined with radiotherapy. These findings indicated that the efficacy of TGF-β inhibitors depends on the combination of tumor parenchyma and microenvironment and drugs (101–103). Combination of fresolimumab with a programmed cell death protein 1 (PD-1) inhibitor has also shown promising results in a variety of cancers. However, although fresolimumab has a similar affinity for all TGF-β subtypes, these subtypes are expressed in varying amounts in different cancers, and thus antagonists need to be developed with specific targets. Accordingly, Sanofi Aventis has suspended clinical development of fresolimumab as an oncologic agent. Nevertheless, development of antibodies against fibrosis continues.
Similarly to neutralizing antibodies, soluble TGF-β receptors can interfere with or block the interaction between ligands and membrane binding receptors, acting as a “ligand trap.” Soluble TGF-β receptors have shown antitumor efficacy in preclinical mouse models of mesothelioma, liver cancer, and pancreatic cancer, with increased apoptosis of primary tumors and decreased metastasis (104, 105). TGF-β type III receptors bind to all subtypes of TGF-β. Furthermore, studies in the MDA-MB-23 breast cancer cell line showed that reduction of serum TGF-β levels decreased the movement and invasiveness of tumor cells and metastasis to the lungs (106–108).
3.2.2 Studies in fibrosis
Many studies have shown that TGF-β1 signaling plays an important role in the pathogenesis of fibrosis. TGF-β is an effective promoter of fibrosis in cardiac fibrosis and idiopathic or interstitial pulmonary fibrosis (109). In various clinical trials, direct neutralization of TGF-β has been shown to have an anti-fibrotic effect. AVID200 is an engineered TGF - β ligand trap. It exhibits higher sensitivity to TGF - β1 than to TGF - β2, where TGF - β2 is a positive factor for hematopoiesis and cardiac function. As a result, AVID200 is more precisely targeted in treating anemia, such as that associated with myelodysplastic syndrome. Moreover, its ability to increase platelet count represents an effect not previously observed in other therapeutic approaches (8, 110). Systemic sclerosis is characterized by excessive deposition of ECM components in tissues and organs resulting in fibrosis. Metelimumab has been evaluated as an early treatment for systemic sclerosis but has not shown a therapeutic effect (111). In a Phase I trial (NCT01284322), biomarkers of systemic sclerosis (thrombospondin 1 and cartilage oligomeric protein) declined rapidly after treatment with fresolimumab. These findings suggest that fresolimumab holds promise as a treatment for systemic sclerosis. The US Food and Drug Administration has approved disitertide (P144), a peptide derived from the human beta-glycan ligand-binding domain, for testing in cutaneous fibrosis (112).
3.3 Blocking activation of latent TGF-β
Blocking the conversion of latent TGF-β to activated TGF-β has become an attractive target. Integrin αvβ6 is usually detected on fibrotic and remodeled cells and can promote invasion of cancer cells in several solid tumors (113). Overexpression of integrin αvβ6 is associated with low survival rates in patients with colon cancer or lung cancer (114). Several studies have shown that inhibiting integrin or knocking out integrin genes can reduce or reverse drug resistance and the aggressiveness of breast cancer and stomach cancer. Therefore, integrins are considered to be therapeutic targets in a variety of cancers. Abituzumab is an antibody against integrins. In clinical trials, use of abituzumab was associated with increased progression-free survival and response rates in patients with metastatic colorectal cancer and high integrin expression (115). Cilengitide is a selective integrin inhibitor that has been studied in a series of Phase II/III studies in non-small cell lung cancer, pancreatic cancer, and prostate cancer (116–118). However, inhibition of activation of TGF-β has hampered development of abituzumab because of the effects on homeostasis and the risk of serious adverse events. However, Van Aarsen et al. found that TGF-β signaling did not need to be prevented completely, and only the phosphorylated portion of SMAD2/3 was suppressed, and its collagen expression was significantly reduced (119). For example, BG00011, an anti-αvβ6 monoclonal antibody, significantly reduced bronchoalveolar levels of phosphorylated SMAD2 in patients with idiopathic pulmonary fibrosis in a Phase IIA clinical trial, but the trial has been halted owing to safety concerns (120).
3.4 Blocking the TGF-β receptor
Blockade of the receptor inhibits the kinase activity of TGF-β, thereby preventing typical and atypical signaling downstream, where TGF-β1 receptors are associated with signal transduction by SMADs. Therefore, these receptors are attractive targets for inhibition. TGF-β receptor inhibitors include vactosertib and galunisertib. Vactosertib was found to inhibit metastasis of breast cancer and enhance anti-tumor T cell immunity and antigen diffusion in mouse models of breast cancer (121). To date, clinical trials of vactosertib have focused on the treatment of cancer, and the efficacy of this agent in human fibrotic diseases is yet to be investigated. Galunisertib is a TβRI kinase inhibitor that is showing promising anticancer activity in breast, colon, lung, and hepatocellular carcinoma xenografts. In a mouse model of colorectal cancer, clinically relevant doses of galunisertib were used to enhance the antitumor activity of anti-programmed death-1 ligand 1 (PD-L1) therapies (anti-mouse PD-L1 clones, which resulted in regression of tumors and enhanced activation of T cells) (122). The aforementioned studies further suggest that, in contrast to other receptor blockers, Galunisterib can remarkably decrease adverse events and toxicity, particularly cardiovascular toxicity.
3.5 Blocking intracellular signal transduction
Targeted inhibition of intracellular Smad signaling molecules enables prevention of TGF - β activity triggered by other signaling pathways. This approach differs from blocking TGF - β at the ligand or receptor level. In the classical pathway, interfering with formation of SMAD 2/3 and SMAD 4 complexes reduces the expression of TGF-β response genes, which is a useful way of reducing the risk of negative outcomes (123). Using thioredoxin A as a scaffold, Cui et al. developed three peptide aptamers that can help disrupt subpopulations of TGF-β reactions (124). LY2157299 (Galunisertib) has been shown to inhibit lung cancer and breast cancer cell growth, inhibit the activity of TGF-β receptor I, reduce phosphorylation of SMAD2 and SMAD3, and indirectly affect the formation and function of SMAD2/3/4 complexes, and has been used to evaluate therapeutic effects in a variety of cancer clinical trials (125, 126). SB-431542 is a small-molecule selective inhibitor of ALK-5 that inhibits TGF-β-mediated transcription of renal cancer proteins. However, due to its unstable pharmacokinetics, it has only been studied in vitro (127, 128). Overall, targeting only one signaling pathway is impractical and inefficient in view of the complexity of carcinogenesis. Recent clinical studies have demonstrated the therapeutic potential of TGF-β inhibitors used in combination with adjuvant treatments. TGF-β has a pleiotropic effect on normal physiological function and in tumorigenesis, and thus long-term inhibition of TGF-β and related signaling pathways may produce adverse reactions (129). Therefore, fine-tuning the downstream signaling pathway of TGF-β rather than eliminating it completely at the ligand level would be a better therapeutic strategy. TGF-β signaling mediators, including SMAD2 and SMAD3, vary in their sensitivity to stimulation by TGF-β and bind to different transcription factors, thereby regulating expression of different genes.
4 Potential strategies and future prospects for TGF-β inhibitors
As of August 2023, 124 TGF-β blocking agents had been identified worldwide, two of which have received regulatory approval and 73 are still in the clinical research stage. Six agents are in clinical Phase III trials, 33 in clinical Phase II trials, 32 in clinical Phase I trials, and two in clinical application studies (18, 130). These clinical trials are summarized in Table 1. As can be seen in this table, inhibition of the TGF-β pathway remains an active area of investigation in cancer research. TGF-β-targeted neutralizing antibodies, vaccines, antisense oligonucleotides, and small molecule inhibitors have been studied in solid tumors in clinical trials (131). Belagenpneumatucel–L is an anti-cancer vaccine developed for non-small cell adenocarcinoma (NSCLC) that theoretically increases the immunogenicity of allogeneic lung cancer vaccine cells, resulting in an immune tumor response (24). However, owing to pleiotropic and safety issues, anti-TGF-β agents are difficult to develop. Fresolimumab appears to be relatively safe in clinical trials, and 10 μM has been identified as the optimal dose for clinical development of this agent. Vincenti et al. reported that inhibition of TGF-β delayed wound healing and caused mild gingival bleeding (132, 133). Adverse events are still higher compared to targeting TGF-β2 mRNA, but this finding could mean that future TGF-β-targeted therapies developed at the protein level will have a better safety profile than those developed at the gene level. Bintrafusp alfa is a bifunctional fusion protein that targets TGF-β and PD-L1 and is formed by fusing the extracellular domain of TGF-βRII with a human IgG1 monoclonal antibody that blocks PD-L1. This agent blocks both the TGF-β and PD-L1 immunosuppressive signaling pathways at the same time. It has been demonstrated that inhibition of both these pathways using a bi-functional approach has better antitumor activity relative to TGF-β “traps” and anti-PD-L1 antibodies. In mouse tumor models, bintrafusp alfa significantly reduced fibrosis, helped reduce local drug resistance, and was more effective in tumor regression on day 24 compared with anti-PD-L1 or anti-TGF-β alone (134). In mouse models of breast and colon cancer, Knudsom et al. demonstrated that bintrafusp alfa not only blocked activation of TGF-β signals in the TME but also significantly reduced the transduced TGF-β signal (135). Preclinical data indicate that bintrafusp alfa reduces the expression of vascular endothelial growth factor (VEGF) in cancer - associated fibrosis and the subsequent angiogenesis by sequestering TGF - β. It may also restore normal vascular homeostasis, thus facilitating drug delivery and the infiltration of T cells into the tumor microenvironment (TME) (134, 136, 137). Simultaneous targeting of two non-redundant immunosuppressive pathways may have superior antitumor activity. Liu et al. conducted a clinical expansion Phase I treatment for advanced solid tumors. They discovered that a dose of 30mg/kg demonstrated the optimal anti - tumor activity. Notably, compared to other doses, there was no significant increase in toxicity. Among the treated patients, 37% showed a reduction in the target - lesion tumor (138, 139).
Many TGF-β-targeted therapies are presently under investigation for their effects when used in combination with anti-PD-L1 therapies, particularly in cancers that do not respond well to PD-L1 monotherapy. Gemogenovatucel-T is being used alone or in combination with atezolizumab (NCT03073525) or duvaliumab (NCT02725489) in advanced gynecological cancers (140). Valeria et al. found that the neutralizing antibody 1D11 combined with anti-PD-1 helps T cells penetrate into the center of a tumor and enhances anti-tumor immunity (141, 142). Simultaneous administration of anti-TGF-β, anti-VEGF (Y332D), and anti-PD-1 inhibited tumor growth and metastasis in lung metastasis models and was superior to anti-VEGF and anti-TGF-β alone in reducing nodules in lung tissue (143, 144). Furthermore, anti-PD-1 and Y332D significantly increased survival time in mouse models of hepatocellular carcinoma (145). Y332D promotes transition of tumors from “cold” to “hot”, potentially increasing the sensitivity of PD-1 antibodies. Studies in pancreatic cancer cell lines and xenografts in mice have found that combination therapy consisting of galunisertib, a TGF-β receptor inhibitor, and lapatinib, an inhibitor of both the epidermal growth factor receptor and human epidermal growth factor receptor 2, reduces tumor growth and metastasis by inhibiting lymphangiogenesis and angiogenesis (147). Other studies in cultured cell lines and mouse xenografts have shown that LY2109761, an inhibitor of TGFBR1 and TGFBR2 kinase activity, has antitumor effects that are synergistic with those of gemcitabine and reduces metastasis (148, 149). Galunisertib in combination with gemcitabine extends survival of unresectable pancreatic cancer in humans (150). In conclusion, these results offer evidence suggesting that combination therapy involving TGF-β inhibitors may present a more favorable safety profile and be more easily managed compared to TGF-β monotherapy. Traditional Chinese Medicine is a multi-targeted treatment, in which each target can cooperate with others with a low risk of adverse effects. Therefore, there are many possibilities for integration of traditional Chinese and Western medicine. Dachaihu decoction reduced expression of TGF-β in a rodent model of non-alcoholic fatty liver disease, and psoralen decreased TGF-β levels in bleomycin-induced pulmonary fibrosis (146, 151). No biomarker of an inhibitory response to TGF-β has been identified to date. More studies of immunophenotypes and the characteristics of TGFβ-related gene expression, as well as genomic biomarkers, are warranted.
TGF-β is a ubiquitous, multifunctional cytokine that is believed to be a central pathway in the development and progression of cancer. TGF-β inhibition strategies have demonstrated beneficial effects in mouse models of cancer. Considering the pleiotropic nature of TGF-β and its role in biological homeostasis, the safety of TGF-β antagonists in human patients must be carefully evaluated. Considering that targeting TGF-β signaling by inhibition of its receptors or neutralizing all TGF-β subtypes may be associated with serious adverse events, such as keratoacanthoma, squamous cell carcinoma, and impaired immunity. Many of these approaches have shown promising anti-metastasis effects in preclinical models and potential for further clinical development.
5 Limitations of anti–TGF-β therapy
Preclinical and clinical data of anti-TGF-β therapies suggest promise for targeting this pathway as anti-cancer therapies. However, anti-TGF-β therapy has a number of limitations:
At present, most of the data for anti-TGF-β therapy are derived from animal experiments, which are difficult to fully simulate the complexity and heterogeneity of human tumors. However, some reports used in human experiments have pointed to adverse effects in some patients. For example, in a multicenter, phase II clinical trial, researchers used fresolimumab, an anti-TGF-β monoclonal antibody, in patients with advanced pancreatic cancer in an attempt to block the TGF-β signaling pathway to curb tumor progression, but the overall survival rate of patients was not significantly improved (152, 153). In addition, some early clinical studies in non-small cell lung cancer (NSCLC) have introduced anti-TGF-β therapies in combination with conventional chemotherapy, and some patients have not only not benefited from the combination therapy, but their disease has accelerated their deterioration (154–157). This has the opposite effect of TGF-β in the stage of tumorigenesis, so it is difficult for anti-TGF-β to accurately distinguish the stage of the tumor, which may counteract the original antitumor effect and accelerate the deterioration of the tumor.
For the treatment of tumors, a combination of drugs is often used, such as TGF-β is combined with radiotherapy, chemotherapy, immunotherapy and other drugs (90, 158). However, the TGF-β signaling pathway intersects and overlaps with many other anticancer drug pathways, and when combined, complex interactions between drugs may cancel out each other’s effects or cause unpredictable toxicity stacking. For example, the combination of anti-TGF-β drugs with platinum-based chemotherapy drugs may alter the uptake and metabolism of chemotherapy by tumor cells, making chemotherapy response unstable (159, 160). The sequence and time interval of combination therapy and the optimal timing of intervention in different tumor stages are different, and if anti-TGF-β therapy is intervened too early or too late, it will not be able to form a synergistic effect with other therapies.
In addition to the antitumor effects of TGF-β, this cytokine is also important for normal tissues homeostasis. Since TGF-β is involved in many normal physiological processes, systemic inhibition of TGF-β may have harmful side effects, and when anti-TGF-β drugs are applied systemically, healthy tissues will also be affected, such as interfering with the normal renewal of liver and kidney cells, resulting in abnormal liver and kidney function, and may also affect the repair of the gastrointestinal mucosa, causing digestive disorders (90, 161, 162).
6 Conclusion
The TGF-β signaling pathway is essential under normal physiological conditions but is also involved in progression of cancer, and thus has attracted considerable research interest in recent years. TGF-β shapes the TME in a way that is conducive to tumor progression (Figure 2). Therefore, blocking TGF-β has the potential to reduce invasion and migration of tumor cells and holds promise for broad application in clinical practice. However, the potential adverse effects of TGF-β inhibitors have hindered their clinical application. The results of recent clinical trials have raised concerns regarding the toxicity of TGF-β inhibitors and suggest the possibility that inhibiting the TGF-β signaling pathway may worsen rather than improve the outlook for patients with cancer. At present, none of these agents has been approved for the treatment of cancer or fibrosis. Inflammation and bleeding are common adverse effects of TGF-β therapy, and TGF-βR inhibitors have been found to cause significant cardiotoxicity. Therefore, there is a need for more targeted clinical treatment. Targeted nanotechnology-based interventions have been used as an available measure to improve treatment. Considering the pleiotropic effect of TGF-β, targeting its downstream signal transduction may identify better targets for cancer therapy. Finally, patients need to be carefully selected for participation in clinical trials, and the indications and dosages of drugs should be carefully defined to limit both targeted and off-target adverse effects.
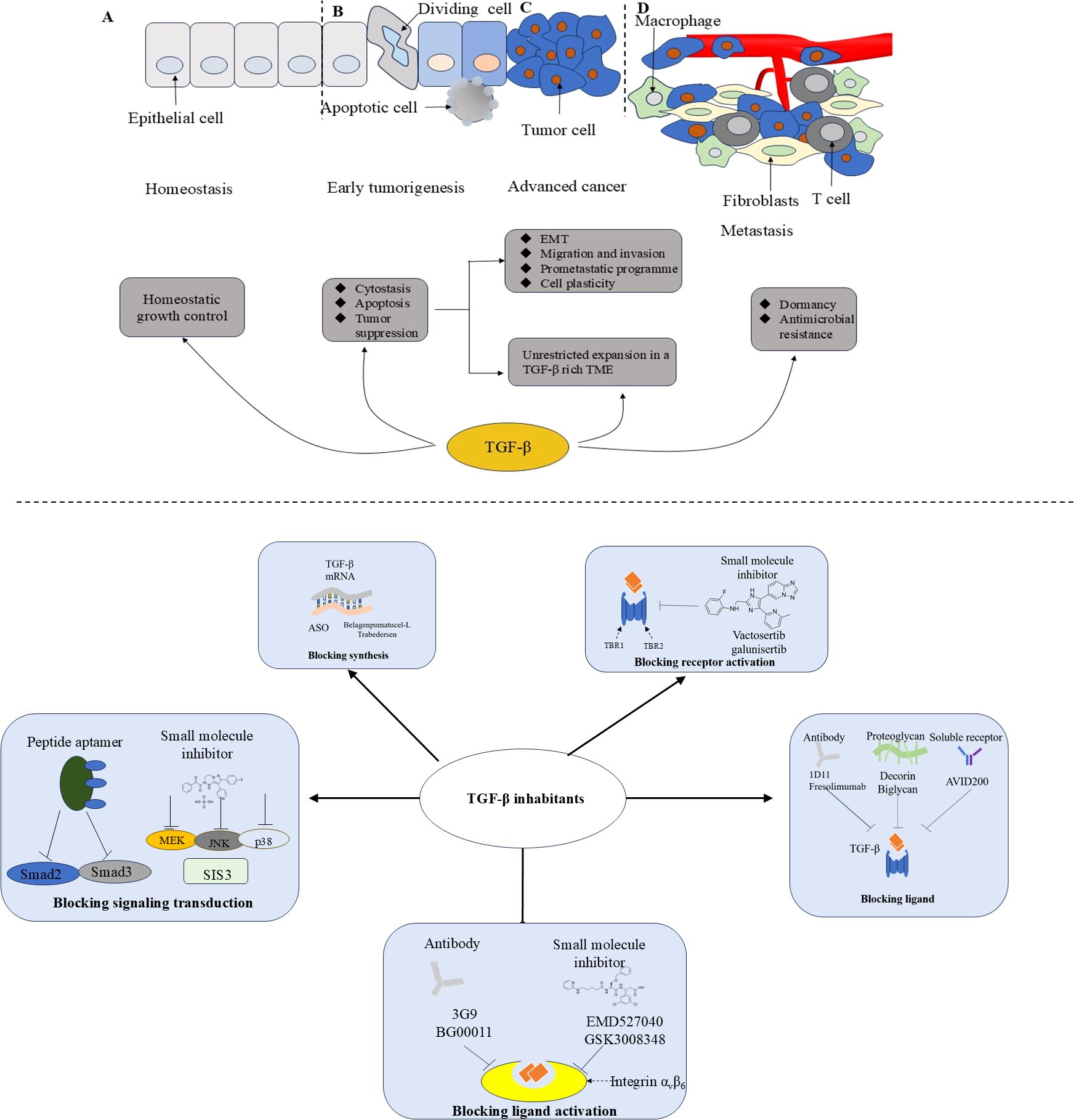
Figure 2. The function of TGF-β during tumor progression and therapeutic strategies of TGF-β inhibition. ASO, antisense oligonucleotides; EMT, Epithelial-mesenchymal transition; TME, Tumor micro-environment. During tumorigenesis, TGF - β transitions from acting as a tumor suppressor in the premalignant stages to promoting tumor growth in the later stages of the disease, a process that ultimately leads to metastasis. (A) Normal epithelium; (B) Early tumorigenesis; (C) Advanced cancer; (D) Invasive metastatic cancer.
Author contributions
HJ: Writing – original draft, Writing – review & editing. YG: Investigation, Writing – review & editing. LJ: Conceptualization, Writing – review & editing. HY: Conceptualization, Supervision, Writing – review & editing. SL: Resources, Supervision, Writing – original draft, Writing – review & editing.
Funding
The author(s) declare that financial support was received for the research and/or publication of this article. The study was supported by the National Natural Science Foundation of China (Grant No.81802888), the Key Technology Research and Development Program of Shandong (No.2018GSF118088), and the General Financial Grant from the China Postdoctoral Science Foundation (No. 2016M592143).
Acknowledgments
We thank Liwen Bianji (Edanz) (www.liwenbianji.cn) for editing the English text of a draft of this manuscript.
Conflict of interest
The authors declare that the research was conducted in the absence of any commercial or financial relationships that could be construed as a potential conflict of interest.
Publisher’s note
All claims expressed in this article are solely those of the authors and do not necessarily represent those of their affiliated organizations, or those of the publisher, the editors and the reviewers. Any product that may be evaluated in this article, or claim that may be made by its manufacturer, is not guaranteed or endorsed by the publisher.
References
1. Pardali E, Sanchez-Duffhues G, Gomez-Puerto MC, Ten Dijke P. TGF-β-induced endothelial-mesenchymal transition in fibrotic diseases. Int J Mol Sci. (2017) 18:2157. doi: 10.3390/ijms18102157
2. Xiang S, Reed DR, Alexandrow MG. The CMG helicase and cancer: A tumor ‘Engine’ and weakness with missing mutations. Oncogene. (2023) 42:473–90. doi: 10.1038/s41388-022-02572-8
3. Ceccherini E, Di Giorgi N, Michelucci E, Signore G, Tedeschi L, Vozzi F, et al. Biological effects of transforming growth factor beta in human cholangiocytes. Biol (Basel). (2022) 11:566. doi: 10.3390/biology11040566
4. Trinh-Minh T, Chen C-W, Tran Manh C, Li Y-N, Zhu H, Zhou X, et al. Noncanonical WNT5A controls the activation of latent TGF-β to drive fibroblast activation and tissue fibrosis. J Clin Invest. (2024) 134:e159884. doi: 10.1172/JCI159884
5. Shi Q, Huang F, Wang Y, Liu H, Deng H, Chen Y-G. HER2 phosphorylation induced by TGF-β promotes mammary morphogenesis and breast cancer progression. J Cell Biol. (2024) 223:e202307138. doi: 10.1083/jcb.202307138
6. Yuan S, Almagro J, Fuchs E. Beyond genetics: driving cancer with the tumour microenvironment behind the wheel. Nat Rev Cancer. (2024) 24:274–86. doi: 10.1038/s41568-023-00660-9
7. He J-Z, Chen Y, Zeng F-M, Huang Q-F, Zhang H-F, Wang S-H, et al. Spatial analysis of stromal signatures identifies invasive front carcinoma-associated fibroblasts as suppressors of anti-tumor immune response in esophageal cancer. J Exp Clin Cancer Res. (2023) 42:136. doi: 10.1186/s13046-023-02697-y
8. Kim B-G, Malek E, Choi SH, Ignatz-Hoover JJ, Driscoll JJ. Novel therapies emerging in oncology to target the TGF-β pathway. J Hematol Oncol. (2021) 14:55. doi: 10.1186/s13045-021-01053-x
9. Li S, Liu M, Do MH, Chou C, Stamatiades EG, Nixon BG, et al. Cancer immunotherapy via targeted TGF-β Signaling blockade in TH cells. Nature. (2020) 587:121–5. doi: 10.1038/s41586-020-2850-3
10. García de Durango CR, Escribese MM, Rosace D. The TGF-β-Th2 axis: A new target for cancer therapy? Allergy. (2021) 76:3563–5. doi: 10.1111/all.14965
11. Fan C, Wang Q, Kuipers TB, Cats D, Iyengar PV, Hagenaars SC, et al. LncRNA LITATS1 suppresses TGF-β-induced EMT and cancer cell plasticity by potentiating TβRI degradation. EMBO J. (2023) 42:e112806. doi: 10.15252/embj.2022112806
12. Huang Y, Chen Z, Lu T, Bi G, Li M, Liang J, et al. HIF-1α switches the functionality of TGF-β signaling via changing the partners of smads to drive glucose metabolic reprogramming in non-small cell lung cancer. J Exp Clin Cancer Res. (2021) 40:398. doi: 10.1186/s13046-021-02188-y
13. He Y, Goyette M-A, Chapelle J, Boufaied N, Al Rahbani J, Schonewolff M, et al. CdGAP is a talin-binding protein and a target of TGF-β signaling that promotes HER2-positive breast cancer growth and metastasis. Cell Rep. (2023) 42:112936. doi: 10.1016/j.celrep.2023.112936
14. Xu X, Zheng L, Yuan Q, Zhen G, Crane JL, Zhou X, et al. Transforming growth factor-β in stem cells and tissue homeostasis. Bone Res. (2018) 6:2. doi: 10.1038/s41413-017-0005-4
15. Gu Y-Y, Liu X-S, Lan H-Y. Therapeutic potential for renal fibrosis by targeting Smad3-dependent noncoding RNAs. Mol Ther. (2024) 32:313–24. doi: 10.1016/j.ymthe.2023.12.009
16. Padilla L, Barranco I, Martínez-Hernández J, Parra A, Parrilla I, Pastor LM, et al. Extracellular vesicles would be involved in the release and delivery of seminal TGF-β isoforms in pigs. Front Vet Sci. (2023) 10:1102049. doi: 10.3389/fvets.2023.1102049
17. Shi M, Zhu J, Wang R, Chen X, Mi L, Walz T, et al. Latent TGF-β structure and activation. Nature. (2011) 474:343–9. doi: 10.1038/nature10152
18. Deng Z, Fan T, Xiao C, Tian H, Zheng Y, Li C, et al. TGF-β signaling in health, disease, and therapeutics. Signal Transduct Target Ther. (2024) 9:61. doi: 10.1038/s41392-024-01764-w
19. Miranda P, Mirisis AA, Kukushkin NV, Carew TJ. Pattern detection in the TGFβ cascade controls the induction of long-term synaptic plasticity. Proc Natl Acad Sci. (2023) 120:e2300595120. doi: 10.1073/pnas.2300595120
20. Zhang Y, Liu J, Zou T, Qi Y, Yi B, Dissanayaka WL, et al. DPSCs treated by TGF-β1 regulate angiogenic sprouting of three-dimensionally co-cultured HUVECs and DPSCs through VEGF-Ang-Tie2 signaling. Stem Cell Res Ther. (2021) 12:281. doi: 10.1186/s13287-021-02349-y
21. Annes JP, Munger JS, Rifkin DB. Making sense of latent TGFbeta activation. J Cell Sci. (2003) 116:217–24. doi: 10.1242/jcs.00229
22. Batlle E, Massagué J. Transforming growth factor-β Signaling in immunity and cancer. Immunity. (2019) 50:924–40. doi: 10.1016/j.immuni.2019.03.024
23. Bai X, Yi M, Jiao Y, Chu Q, Wu K. Blocking TGF-β Signaling to enhance the efficacy of immune checkpoint inhibitor. Onco Targets Ther. (2019) 12:9527–38. doi: 10.2147/OTT.S224013
24. Haque S, Morris JC. Transforming growth factor-β: A therapeutic target for cancer. Hum Vaccin Immunother. (2017) 13:1741–50. doi: 10.1080/21645515.2017.1327107
25. Barcellos-Hoff MH, Gulley JL. Molecular pathways and mechanisms of TGFβ in cancer therapy. Clin Cancer Res. (2023) 29:2025–33. doi: 10.1158/1078-0432.CCR-21-3750
26. Heldin C-H, Moustakas A. Signaling receptors for TGF-β Family members. Cold Spring Harb Perspect Biol. (2016) 8:a022053. doi: 10.1101/cshperspect.a022053
27. Groppe J, Hinck CS, Samavarchi-Tehrani P, Zubieta C, Schuermann JP, Taylor AB, et al. Cooperative assembly of TGF-β Superfamily signaling complexes is mediated by two disparate mechanisms and distinct modes of receptor binding. Mol Cell. (2008) 29:157–68. doi: 10.1016/j.molcel.2007.11.039
28. Lai H, Chen A, Cai H, Fu J, Salem F, Li Y, et al. Podocyte and endothelial-specific elimination of BAMBI identifies differential transforming growth factor-β pathways contributing to diabetic glomerulopathy. Kidney Int. (2020) 98:601–14. doi: 10.1016/j.kint.2020.03.036
29. David CJ, Massagué J. Publisher Correction: Contextual determinants of TGFβ action in development, immunity and cancer. Nat Rev Mol Cell Biol. (2018) 19:419–35. doi: 10.1038/s41580-018-0018-x
30. Velasco S, Alvarez-Muñoz P, Pericacho M, ten Dijke P, Bernabéu C, López-Novoa JM, et al. L- and S-endoglin differentially modulate TGFβ1 signaling mediated by ALK1 and ALK5 in L6E9 myoblasts. J Cell Sci. (2008) 121:913–9. doi: 10.1242/jcs.023283
31. Ollauri-Ibáñez C, Ayuso-Íñigo B, Pericacho M. Hot and cold tumors: is endoglin (CD105) a potential target for vessel normalization? Cancers. (2021) 13:1552. doi: 10.3390/cancers13071552
32. Tripathi V, Sixt KM, Gao S, Xu X, Huang J, Weigert R, et al. Direct regulation of alternative splicing by SMAD3 through PCBP1 is essential to the tumor-promoting role of TGF-β. Mol Cell. (2016) 64:549–64. doi: 10.1016/j.molcel.2016.09.013
33. Yuan J, Yang F, Wang F, Ma J, Guo Y, Tao Q, et al. A long noncoding RNA activated by TGF-β promotes the invasion-metastasis cascade in hepatocellular carcinoma. Cancer Cell. (2014) 25:666–81. doi: 10.1016/j.ccr.2014.03.010
34. Tzavlaki K, Moustakas A. TGF-β Signaling. Biomolecules. (2020) 10:487. doi: 10.3390/biom10030487
35. Chaikuad A, Bullock AN. Structural basis of intracellular TGF-β Signaling: receptors and smads. Cold Spring Harb Perspect Biol. (2016) 8:a022111. doi: 10.1101/cshperspect.a022111
36. Zhang Y, Chang C, Gehling DJ, Hemmati-Brivanlou A, Derynck R. Regulation of Smad degradation and activity by Smurf2, an E3 ubiquitin ligase. Proc Natl Acad Sci U.S.A. (2001) 98:974–9. doi: 10.1073/pnas.98.3.974
37. Wan M, Cao X, Wu Y, Bai S, Wu L, Shi X, et al. Jab1 antagonizes TGF-beta signaling by inducing Smad4 degradation. EMBO Rep. (2002) 3:171–6. doi: 10.1093/embo-reports/kvf024
38. Chen F, Zhong C-C, Song C-C, Chen S-W, He Y, Tan X-Y. Molecular characterization and functional analysis of two steroidogenic genes TSPO and SMAD4 in yellow catfish. Int J Mol Sci. (2021) 22:4505. doi: 10.3390/ijms22094505
39. Chen H, Moreno-Moral A, Pesce F, Devapragash N, Mancini M, Heng EL, et al. WWP2 regulates pathological cardiac fibrosis by modulating SMAD2 signaling. Nat Commun. (2019) 10:3616. doi: 10.1038/s41467-019-11551-9
40. Liang M, Liang Y-Y, Wrighton K, Ungermannova D, Wang X-P, Brunicardi FC, et al. Ubiquitination and proteolysis of cancer-derived Smad4 mutants by SCFSkp2. Mol Cell Biol. (2004) 24:7524–37. doi: 10.1128/MCB.24.17.7524-7537.2004
41. Yuan T, Chen Z, Yan F, Qian M, Luo H, Ye S, et al. Deubiquitinating enzyme USP10 promotes hepatocellular carcinoma metastasis through deubiquitinating and stabilizing Smad4 protein. Mol Oncol. (2020) 14:197–210. doi: 10.1002/1878-0261.12596
42. Derynck R, Budi EH. Specificity, versatility, and control of TGF-β family signaling. Sci Signal. (2019) 12:eaav5183. doi: 10.1126/scisignal.aav5183
43. Samba-Mondonga M, Calvé A, Mallette FA, Santos MM. MyD88 regulates the expression of SMAD4 and the iron regulatory hormone hepcidin. Front Cell Dev Biol. (2018) 6:105. doi: 10.3389/fcell.2018.00105
44. Hanyu A, Ishidou Y, Ebisawa T, Shimanuki T, Imamura T, Miyazono K. The N domain of Smad7 is essential for specific inhibition of transforming growth factor-beta signaling. J Cell Biol. (2001) 155:1017–27. doi: 10.1083/jcb.200106023
45. Zhao M, Mishra L, Deng C-X. The role of TGF-β/SMAD4 signaling in cancer. Int J Biol Sci. (2018) 14:111–23. doi: 10.7150/ijbs.23230
46. Eftychi C, Karagianni N, Alexiou M, Apostolaki M, Kollias G. Myeloid TAKI [corrected] acts as a negative regulator of the LPS response and mediates resistance to endotoxemia. PloS One. (2012) 7:e31550. doi: 10.1371/journal.pone.0031550
47. Caja L, Dituri F, Mancarella S, Caballero-Diaz D, Moustakas A, Giannelli G, et al. TGF-β and the tissue microenvironment: relevance in fibrosis and cancer. Int J Mol Sci. (2018) 19:1294. doi: 10.3390/ijms19051294
48. An H-W, Seok SH, Kwon J-W, Choudhury AD, Oh J-S, Voon DC, et al. The loss of epithelial Smad4 drives immune evasion via CXCL1 while displaying vulnerability to combinatorial immunotherapy in gastric cancer. Cell Rep. (2022) 41:111878. doi: 10.1016/j.celrep.2022.111878
49. Yatagai N, Saito T, Akazawa Y, Hayashi T, Yanai Y, Tsuyama S, et al. Frequent loss of heterozygosity of SMAD4 locus and prognostic impacts of SMAD4 immunohistochemistry in gastric adenocarcinoma with enteroblastic differentiation. Hum Pathol. (2019) 88:18–26. doi: 10.1016/j.humpath.2019.03.005
50. Li X, Lv X, Li Z, Li C, Li X, Xiao J, et al. Long noncoding RNA ASLNC07322 functions in VEGF-C expression regulated by smad4 during colon cancer metastasis. Mol Ther Nucleic Acids. (2019) 18:851–62. doi: 10.1016/j.omtn.2019.10.012
51. Mehrvarz Sarshekeh A, Advani S, Overman MJ, Manyam G, Kee BK, Fogelman DR, et al. Association of SMAD4 mutation with patient demographics, tumor characteristics, and clinical outcomes in colorectal cancer. PloS One. (2017) 12:e0173345. doi: 10.1371/journal.pone.0173345
52. Yu Y, Feng X-H. TGF-β signaling in cell fate control and cancer. Curr Opin Cell Biol. (2019) 61:56–63. doi: 10.1016/j.ceb.2019.07.007
53. Xu X, Ehdaie B, Ohara N, Yoshino T, Deng C-X. Synergistic action of Smad4 and Pten in suppressing pancreatic ductal adenocarcinoma formation in mice. Oncogene. (2010) 29:674–86. doi: 10.1038/onc.2009.375
54. Debernardi S, Liszka L, Ntala C, Steiger K, Esposito I, Carlotti E, et al. Molecular characteristics of early-onset pancreatic ductal adenocarcinoma. Mol Oncol. (2024) 18:677–90. doi: 10.1002/1878-0261.13576
55. Yan W, Menjivar RE, Bonilla ME, Steele NG, Kemp SB, Du W, et al. Notch signaling regulates immunosuppressive tumor-associated macrophage function in pancreatic cancer. Cancer Immunol Res. (2024) 12:91–106. doi: 10.1158/2326-6066.CIR-23-0037
56. Bertrand-Chapel A, Caligaris C, Fenouil T, Savary C, Aires S, Martel S, et al. SMAD2/3 mediate oncogenic effects of TGF-β in the absence of SMAD4. Commun Biol. (2022) 5:1068. doi: 10.1038/s42003-022-03994-6
57. Su J, Morgani SM, David CJ, Wang Q, Er EE, Huang Y-H, et al. TGF-β orchestrates fibrogenic and developmental EMTs via the RAS effector RREB1. Nature. (2020) 577:566–71. doi: 10.1038/s41586-019-1897-5
58. Wu N, Jiang M, Liu H, Chu Y, Wang D, Cao J, et al. LINC00941 promotes CRC metastasis through preventing SMAD4 protein degradation and activating the TGF-β/SMAD2/3 signaling pathway. Cell Death Differ. (2021) 28:219–32. doi: 10.1038/s41418-020-0596-y
59. Lu H, Cao LL, Ballout F, Belkhiri A, Peng D, Chen L, et al. Reflux conditions induce E-cadherin cleavage and EMT via APE1 redox function in oesophageal adenocarcinoma. Gut. (2023) 73:47–62. doi: 10.1136/gutjnl-2023-329455
60. Baglieri J, Brenner DA, Kisseleva T. The role of fibrosis and liver-associated fibroblasts in the pathogenesis of hepatocellular carcinoma. Int J Mol Sci. (2019) 20:1723. doi: 10.3390/ijms20071723
61. Sanjabi S, Oh SA, Li MO. Regulation of the immune response by TGF-β: from conception to autoimmunity and infection. Cold Spring Harb Perspect Biol. (2017) 9:a022236. doi: 10.1101/cshperspect.a022236
62. Travis MA, Sheppard D. TGF-β activation and function in immunity. Annu Rev Immunol. (2014) 32:51–82. doi: 10.1146/annurev-immunol-032713-120257
63. Cortez VS, Ulland TK, Cervantes-Barragan L, Bando JK, Robinette ML, Wang Q, et al. SMAD4 impedes the conversion of NK cells into ILC1-like cells by curtailing non-canonical TGF-β signaling. Nat Immunol. (2017) 18:995–1003. doi: 10.1038/ni.3809
64. Wang Y, Chu J, Yi P, Dong W, Saultz J, Wang Y, et al. SMAD4 promotes TGF-β-independent NK cell homeostasis and maturation and antitumor immunity. J Clin Invest. (2018) 128:5123–36. doi: 10.1172/JCI121227
65. Lee JH, Massagué J. TGF-β in developmental and fibrogenic EMTs. Semin Cancer Biol. (2022) 86:136–45. doi: 10.1016/j.semcancer.2022.09.004
66. Peng D, Fu M, Wang M, Wei Y, Wei X. Targeting TGF-β signal transduction for fibrosis and cancer therapy. Mol Cancer. (2022) 21:104. doi: 10.1186/s12943-022-01569-x
67. Siapoush S, Rezaei R, Alavifard H, Hatami B, Zali MR, Vosough M, et al. Therapeutic implications of targeting autophagy and TGF-β crosstalk for the treatment of liver fibrosis. Life Sci. (2023) 329:121894. doi: 10.1016/j.lfs.2023.121894
68. Saito A, Horie M, Nagase T. TGF-β Signaling in lung health and disease. Int J Mol Sci. (2018) 19:2460. doi: 10.3390/ijms19082460
69. Yuan Q, Ren Q, Li L, Tan H, Lu M, Tian Y, et al. A Klotho-derived peptide protects against kidney fibrosis by targeting TGF-β signaling. Nat Commun. (2022) 13:438. doi: 10.1038/s41467-022-28096-z
70. Fior R, Póvoa V, Mendes RV, Carvalho T, Gomes A, Figueiredo N, et al. Single-cell functional and chemosensitive profiling of combinatorial colorectal therapy in zebrafish xenografts. Proc Natl Acad Sci United States America. (2017) 114:E8234–43. doi: 10.1073/pnas.1618389114
71. Veinotte CJ, Dellaire G, Berman JN. Hooking the big one: The potential of zebrafish xenotransplantation to reform cancer drug screening in the genomic era. DMM Dis Models Mech. (2014) 7:745–54. doi: 10.1242/dmm.015784
72. Liu S, Leach SD. Zebrafish models for cancer. Annu Rev Pathology: Mech Dis. (2011) 6:71–93. doi: 10.1146/annurev-pathol-011110-130330
73. Samarelli AV, Masciale V, Aramini B, Coló GP, Tonelli R, Marchioni A, et al. Molecular mechanisms and cellular contribution from lung fibrosis to lung cancer development. Int J Mol Sci. (2021) 22:12179. doi: 10.3390/ijms222212179
74. Zeitlmayr S, Zierler S, Staab-Weijnitz CA, Dietrich A, Geiger F, Horgen FD, et al. TRPM7 restrains plasmin activity and promotes transforming growth factor-β1 signaling in primary human lung fibroblasts. Arch Toxicol. (2022) 96:2767–83. doi: 10.1007/s00204-022-03342-x
75. Chakravarthy A, Khan L, Bensler NP, Bose P, De Carvalho DD. TGF-β-associated extracellular matrix genes link cancer-associated fibroblasts to immune evasion and immunotherapy failure. Nat Commun. (2018) 9:4692. doi: 10.1038/s41467-018-06654-8
76. Loevenich LP, Tschurtschenthaler M, Rokavec M, Silva MG, Jesinghaus M, Kirchner T, et al. SMAD4 loss induces c-MYC-mediated NLE1 upregulation to support protein biosynthesis, colorectal cancer growth, and metastasis. Cancer Res. (2022) 82:4604–23. doi: 10.1158/0008-5472.CAN-22-1247
77. Hartley R, Phoenix TN. MYC promotes aggressive growth and metastasis of a WNT-medulloblastoma mouse model. Dev Neurosci. (2024) 46:167–78. doi: 10.1159/000533270
78. Nucera C, Lawler J, Parangi S. BRAF(V600E) and microenvironment in thyroid cancer: a functional link to drive cancer progression. Cancer Res. (2011) 71:2417–22. doi: 10.1158/0008-5472.CAN-10-3844
79. Liu Z, Zhang Y, Zhang L, Zhou T, Li Y, Zhou G, et al. Duality of interactions between TGF-β and TNF-α During tumor formation. Front Immunol. (2022) 12:810286. doi: 10.3389/fimmu.2021.810286
80. Cieply B, Riley P, Pifer PM, Widmeyer J, Addison JB, Ivanov AV, et al. Suppression of the epithelial-mesenchymal transition by Grainyhead-like-2. Cancer Res. (2012) 72:2440–53. doi: 10.1158/0008-5472.CAN-11-4038
81. Calon A, Lonardo E, Berenguer-Llergo A, Espinet E, Hernando-Momblona X, Iglesias M, et al. Stromal gene expression defines poor-prognosis subtypes in colorectal cancer. Nat Genet. (2015) 47:320–9. doi: 10.1038/ng.3225
82. Isella C, Terrasi A, Bellomo SE, Petti C, Galatola G, Muratore A, et al. Stromal contribution to the colorectal cancer transcriptome. Nat Genet. (2015) 47:312–9. doi: 10.1038/ng.3224
83. Siemann DW, Horsman MR. Modulation of the tumor vasculature and oxygenation to improve therapy. Pharmacol Ther. (2015) 153:107–24. doi: 10.1016/j.pharmthera.2015.06.006
84. Rhim AD, Oberstein PE, Thomas DH, Mirek ET, Palermo CF, Sastra SA, et al. Stromal elements act to restrain, rather than support, pancreatic ductal adenocarcinoma. Cancer Cell. (2014) 25:735–47. doi: 10.1016/j.ccr.2014.04.021
85. Connolly EC, Freimuth J, Akhurst RJ. Complexities of TGF-β targeted cancer therapy. Int J Biol Sci. (2012) 8:964–78. doi: 10.7150/ijbs.4564
86. Wick W, Weller M. Trabedersen to target transforming growth factor-beta: when the journey is not the reward, in reference to Bogdahn et al. (Neuro-Oncology 2011;13:132-142). Neuro Oncol. (2011) 13:559–60. doi: 10.1093/neuonc/nor046
87. Li Y, Fan W, Link F, Wang S, Dooley S. Transforming growth factor β latency: A mechanism of cytokine storage and signalling regulation in liver homeostasis and disease. JHEP Rep. (2022) 4:100397. doi: 10.1016/j.jhepr.2021.100397
88. Fang H, Declerck YA. Targeting the tumor microenvironment: from understanding pathways to effective clinical trials. Cancer Res. (2013) 73:4965–77. doi: 10.1158/0008-5472.CAN-13-0661
89. Oh M-H, Sun I-H, Zhao L, Leone RD, Sun I-M, Xu W, et al. Targeting glutamine metabolism enhances tumor-specific immunity by modulating suppressive myeloid cells. J Clin Invest. (2020) 130:3865–84. doi: 10.1172/JCI131859
90. Smith AL, Robin TP, Ford HL. Molecular pathways: targeting the TGF-β Pathway for cancer therapy. Clin Cancer Res. (2012) 18:4514–21. doi: 10.1158/1078-0432.CCR-11-3224
91. Zhou Q, Chen D, Zhang J, Xiang J, Zhang T, Wang H, et al. Pancreatic ductal adenocarcinoma holds unique features to form an immunosuppressive microenvironment: A narrative review. J Pancreatol. (2022) 5:174–85. doi: 10.1097/JP9.0000000000000109
92. Pfeiffer N, Voykov B, Renieri G, Bell K, Richter P, Weigel M, et al. First-in-human phase I study of ISTH0036, an antisense oligonucleotide selectively targeting transforming growth factor beta 2 (TGF-β2), in subjects with open-angle glaucoma undergoing glaucoma filtration surgery. PloS One. (2017) 12:e0188899. doi: 10.1371/journal.pone.0188899
93. Zhou C, Huang Z, Lin H, Ma Z, Wang J, Wang Y, et al. Rhizoma curcumae Longae ameliorates high dietary carbohydrate-induced hepatic oxidative stress, inflammation in golden pompano Trachinotus ovatus. Fish Shellfish Immunol. (2022) 130:31–42. doi: 10.1016/j.fsi.2022.08.058
94. Lebrun J-J. The dual role of TGFβ in human cancer: from tumor suppression to cancer metastasis. ISRN Mol Biol. (2012) 2012:381428. doi: 10.5402/2012/381428
95. Song I-W, Nagamani SC, Nguyen D, Grafe I, Sutton VR, Gannon FH, et al. Targeting TGF-β for treatment of osteogenesis imperfecta. J Clin Invest. (2022) 132:e152571. doi: 10.1172/JCI152571
96. Ong CH, Tham CL, Harith HH, Firdaus N, Israf DA. TGF-β-induced fibrosis: A review on the underlying mechanism and potential therapeutic strategies. Eur J Pharmacol. (2021) 911:174510. doi: 10.1016/j.ejphar.2021.174510
97. Chen SY, Kung H-C, Espinoza B, Washington I, Chen K, Wang J, et al. Targeting heterogeneous tumor microenvironments in pancreatic cancer mouse models of metastasis by TGF-β depletion. JCI Insight. (2024) 9:e182766. doi: 10.1172/jci.insight.182766
98. Cardoso AL, Fernandes A, Aguilar-Pimentel JA, de Angelis MH, Guedes JR, Brito MA, et al. Towards frailty biomarkers: Candidates from genes and pathways regulated in aging and age-related diseases. Ageing Res Rev. (2018) 47:214–77. doi: 10.1016/j.arr.2018.07.004
99. Lan Y, Yeung T-L, Huang H, Wegener AA, Saha S, Toister-Achituv M, et al. Colocalized targeting of TGF-β and PD-L1 by bintrafusp alfa elicits distinct antitumor responses. J Immunother Cancer. (2022) 10:e004122. doi: 10.1136/jitc-2021-004122
100. Morris JC, Tan AR, Olencki TE, Shapiro GI, Dezube BJ, Reiss M, et al. Phase I study of GC1008 (fresolimumab): a human anti-transforming growth factor-beta (TGFβ) monoclonal antibody in patients with advanced Malignant melanoma or renal cell carcinoma. PloS One. (2014) 9:e90353. doi: 10.1371/journal.pone.0090353
101. Chen X, Yang Y, Zhou Q, Weiss JM, Howard OZ, McPherson JM, et al. Effective chemoimmunotherapy with anti-TGFβ antibody and cyclophosphamide in a mouse model of breast cancer. PloS One. (2014) 9:e85398. doi: 10.1371/journal.pone.0085398
102. Liu J, Liao S, Diop-Frimpong B, Chen W, Goel S, Naxerova K, et al. TGF-β blockade improves the distribution and efficacy of therapeutics in breast carcinoma by normalizing the tumor stroma. Proc Natl Acad Sci U.S.A. (2012) 109:16618–23. doi: 10.1073/pnas.1117610109
103. Gonzalez-Junca A, Reiners O, Borrero-Garcia LD, Beckford-Vera D, Lazar AA, Chou W, et al. Positron emission tomography imaging of functional transforming growth factor β (TGFβ) activity and benefit of TGFβ Inhibition in irradiated intracranial tumors. Int J Radiat Oncol Biol Phys. (2021) 109:527–39. doi: 10.1016/j.ijrobp.2020.09.043
104. Kelly RJ, Morris JC. Transforming growth factor-beta: a target for cancer therapy. J Immunotoxicol. (2010) 7:15–26. doi: 10.3109/15476910903389920
105. Zhang F, Dong W, Zeng W, Zhang L, Zhang C, Qiu Y, et al. Naringenin prevents TGF-β1 secretion from breast cancer and suppresses pulmonary metastasis by inhibiting PKC activation. Breast Cancer Res. (2016) 18:38. doi: 10.1186/s13058-016-0698-0
106. Yuan S, Zhu L, Chen X, Lin Q. Huanglian Jiedu Tang regulates the inflammatory microenvironment to alleviate the progression of breast cancer by inhibiting the RhoA/ROCK pathway. Tissue Cell. (2025) 95:102850. doi: 10.1016/j.tice.2025.102850
107. Dwyer AR, Perez Kerkvliet C, Truong TH, Hagen KM, Krutilina RI, Parke DN, et al. Glucocorticoid receptors drive breast cancer cell migration and metabolic reprogramming via PDK4. Endocrinology. (2023) 164:bqad083. doi: 10.1210/endocr/bqad083
108. Sanchez VC, Yang HH, Craig-Lucas A, Dubois W, Carofino BL, Lack J, et al. Host CLIC4 expression in the tumor microenvironment is essential for breast cancer metastatic competence. PloS Genet. (2022) 18:e1010271. doi: 10.1371/journal.pgen.1010271
109. Epstein Shochet G, Brook E, Bardenstein-Wald B, Shitrit D. TGF-β pathway activation by idiopathic pulmonary fibrosis (IPF) fibroblast derived soluble factors is mediated by IL-6 trans-signaling. Respir Res. (2020) 21:56. doi: 10.1186/s12931-020-1319-0
110. Varricchio L, Mascarenhas J, Migliaccio AR, O’Connor-McCourt M, Tremblay G, Denis J-F, et al. AVID200, a potent trap for TGF-β Ligands inhibits TGF-β1 signaling in human myelofibrosis. Blood. (2018) 132:1791. doi: 10.1182/blood-2018-99-116474
111. Gumkowska-Sroka O, Kotyla K, Mojs E, Palka K, Kotyla P. Novel therapeutic strategies in the treatment of systemic sclerosis. Pharm (Basel). (2023) 16:1066. doi: 10.3390/ph16081066
112. Cruz-Morande S, Dotor J, San-Julian M. P144 a transforming growth factor beta inhibitor peptide, generates antifibrogenic effects in a radiotherapy induced fibrosis model. Curr Oncol. (2022) 29:2650–61. doi: 10.3390/curroncol29040217
113. Bagati A, Kumar S, Jiang P, Pyrdol J, Zou AE, Godicelj A, et al. Integrin αvβ6-TGFβ-SOX4 pathway drives immune evasion in triple-negative breast cancer. Cancer Cell. (2021) 39:54–67.e9. doi: 10.1016/j.ccell.2020.12.001
114. Busenhart P, Montalban-Arques A, Katkeviciute E, Morsy Y, Van Passen C, Hering L, et al. Inhibition of integrin αvβ6 sparks T-cell antitumor response and enhances immune checkpoint blockade therapy in colorectal cancer. J Immunother Cancer. (2022) 10:e003465. doi: 10.1136/jitc-2021-003465
115. Jackstadt R, van Hooff SR, Leach JD, Cortes-Lavaud X, Lohuis JO, Ridgway RA, et al. Epithelial NOTCH signaling rewires the tumor microenvironment of colorectal cancer to drive poor-prognosis subtypes and metastasis. Cancer Cell. (2019) 36:319–336.e7. doi: 10.1016/j.ccell.2019.08.003
116. Jeong J, Kim J. Cyclic RGD pentapeptide cilengitide enhances efficacy of gefitinib on TGF-β1-induced epithelial-to-mesenchymal transition and invasion in human non-small cell lung cancer cells. Front Pharmacol. (2021) 12:639095. doi: 10.3389/fphar.2021.639095
117. Mas-Moruno C, Rechenmacher F, Kessler H. Cilengitide: the first anti-angiogenic small molecule drug candidate design, synthesis and clinical evaluation. Anticancer Agents Med Chem. (2010) 10:753–68. doi: 10.2174/187152010794728639
118. Wong P-P, Demircioglu F, Ghazaly E, Alrawashdeh W, Stratford MRL, Scudamore CL, et al. Dual-action combination therapy enhances angiogenesis while reducing tumor growth and spread. Cancer Cell. (2015) 27:123–37. doi: 10.1016/j.ccell.2014.10.015
119. Van Aarsen LAK, Leone DR, Ho S, Dolinski BM, McCoon PE, LePage DJ, et al. Antibody-mediated blockade of integrin alpha v beta 6 inhibits tumor progression in vivo by a transforming growth factor-beta-regulated mechanism. Cancer Res. (2008) 68:561–70. doi: 10.1158/0008-5472.CAN-07-2307
120. Raghu G, Mouded M, Chambers DC, Martinez FJ, Richeldi L, Lancaster LH, et al. A phase IIb randomized clinical study of an anti-αvβ6 monoclonal antibody in idiopathic pulmonary fibrosis. Am J Respir Crit Care Med. (2022) 206:1128–39. doi: 10.1164/rccm.202112-2824OC
121. Son JY, Park S-Y, Kim S-J, Lee SJ, Park S-A, Kim M-J, et al. EW-7197, a novel ALK-5 kinase inhibitor, potently inhibits breast to lung metastasis. Mol Cancer Ther. (2014) 13:1704–16. doi: 10.1158/1535-7163.MCT-13-0903
122. Melisi D, Oh D-Y, Hollebecque A, Calvo E, Varghese A, Borazanci E, et al. Safety and activity of the TGFβ receptor I kinase inhibitor galunisertib plus the anti-PD-L1 antibody durvalumab in metastatic pancreatic cancer. J Immunother Cancer. (2021) 9:e002068. doi: 10.1136/jitc-2020-002068
123. Xiang D, Zou J, Zhu X, Chen X, Luo J, Kong L, et al. Physalin D attenuates hepatic stellate cell activation and liver fibrosis by blocking TGF-β/Smad and YAP signaling. Phytomedicine. (2020) 78:153294. doi: 10.1016/j.phymed.2020.153294
124. Cui Q, Lim SK, Zhao B, Hoffmann FM. Selective inhibition of TGF-beta responsive genes by Smad-interacting peptide aptamers from FoxH1, Lef1 and CBP. Oncogene. (2005) 24:3864–74. doi: 10.1038/sj.onc.1208556
125. Radeke MJ, Radeke CM, Shih Y-H, Hu J, Bok D, Johnson LV, et al. Restoration of mesenchymal retinal pigmented epithelial cells by TGFβ pathway inhibitors: implications for age-related macular degeneration. Genome Med. (2015) 7:58. doi: 10.1186/s13073-015-0183-x
126. Laping NJ, Grygielko E, Mathur A, Butter S, Bomberger J, Tweed C, et al. Inhibition of transforming growth factor (TGF)-beta1-induced extracellular matrix with a novel inhibitor of the TGF-beta type I receptor kinase activity: SB-431542. Mol Pharmacol. (2002) 62:58–64. doi: 10.1124/mol.62.1.58
127. Colak S, Ten Dijke P. Targeting TGF-β Signaling in cancer. Trends Cancer. (2017) 3:56–71. doi: 10.1016/j.trecan.2016.11.008
128. Tanaka H, Shinto O, Yashiro M, Yamazoe S, Iwauchi T, Muguruma K, et al. Transforming growth factor β signaling inhibitor, SB-431542, induces maturation of dendritic cells and enhances anti-tumor activity. Oncol Rep. (2010) 24:1637–43. doi: 10.3892/or_00001028
129. Yang Y, Sun M, Li W, Liu C, Jiang Z, Gu P, et al. Rebalancing TGF-β/Smad7 signaling via Compound kushen injection in hepatic stellate cells protects against liver fibrosis and hepatocarcinogenesis. Clin Transl Med. (2021) 11:e410. doi: 10.1002/ctm2.410
130. Goenka A, Khan F, Verma B, Sinha P, Dmello CC, Jogalekar MP, et al. Tumor microenvironment signaling and therapeutics in cancer progression. Cancer Commun (Lond). (2023) 43:525–61. doi: 10.1002/cac2.12416
131. Zhao H, Wei J, Sun J. Roles of TGF-β signaling pathway in tumor microenvirionment and cancer therapy. Int Immunopharmacol. (2020) 89:107101. doi: 10.1016/j.intimp.2020.107101
132. Bogdahn U, Hau P, Stockhammer G, Venkataramana NK, Mahapatra AK, Suri A, et al. Targeted therapy for high-grade glioma with the TGF-β2 inhibitor trabedersen: results of a randomized and controlled phase IIb study. Neuro-Oncology. (2011) 13:132–42. doi: 10.1093/neuonc/noq142
133. Vincenti F, Fervenza FC, Campbell KN, Diaz M, Gesualdo L, Nelson P, et al. A phase 2, double-blind, placebo-controlled, randomized study of fresolimumab in patients with steroid-resistant primary focal segmental glomerulosclerosis. Kidney Int Rep. (2017) 2:800–10. doi: 10.1016/j.ekir.2017.03.011
134. Lan Y, Zhang D, Xu C, Hance KW, Marelli B, Qi J, et al. Enhanced preclinical antitumor activity of M7824, a bifunctional fusion protein simultaneously targeting PD-L1 and TGF-β. Sci Transl Med. (2018) 10:eaan5488. doi: 10.1126/scitranslmed.aan5488
135. Knudson KM, Hicks KC, Luo X, Chen J-Q, Schlom J, Gameiro SR. M7824, a novel bifunctional anti-PD-L1/TGFβ Trap fusion protein, promotes anti-tumor efficacy as monotherapy and in combination with vaccine. Oncoimmunology. (2018) 7:e1426519. doi: 10.1080/2162402X.2018.1426519
136. Eser PÖ, Jänne PA. TGFβ pathway inhibition in the treatment of non-small cell lung cancer. Pharmacol Ther. (2018) 184:112–30. doi: 10.1016/j.pharmthera.2017.11.004
137. Courau T, Nehar-Belaid D, Florez L, Levacher B, Vazquez T, Brimaud F, et al. TGF-β and VEGF cooperatively control the immunotolerant tumor environment and the efficacy of cancer immunotherapies. JCI Insight. (2016) 1:e85974. doi: 10.1172/jci.insight.85974
138. Liu D, Zhou J, Wang Y, Li M, Jiang H, Liu Y, et al. Bifunctional anti-PD-L1/TGF-βRII agent SHR-1701 in advanced solid tumors: a dose-escalation, dose-expansion, and clinical-expansion phase 1 trial. BMC Med. (2022) 20:408. doi: 10.1186/s12916-022-02605-9
139. Li T, Wang X, Niu M, Wang M, Zhou J, Wu K, et al. Bispecific antibody targeting TGF-β and PD-L1 for synergistic cancer immunotherapy. Front Immunol. (2023) 14:1196970. doi: 10.3389/fimmu.2023.1196970
140. Barve M, Aaron P, Manning L, Bognar E, Wallraven G, Horvath S, et al. Pilot study of combination gemogenovatucel-T (Vigil) and durvalumab in women with relapsed BRCA-wt triple-negative breast or ovarian cancer. Clin Med Insights Oncol. (2022) 16:11795549221110501. doi: 10.1177/11795549221110501
141. Scuruchi M, Mannino F, Imbesi C, Pallio G, Vermiglio G, Bagnato G, et al. Biglycan involvement in heart fibrosis: modulation of adenosine 2A receptor improves damage in immortalized cardiac fibroblasts. Int J Mol Sci. (2023) 24:1784. doi: 10.3390/ijms24021784
142. He C, Wang J, Mao Y, Lao X, Li S. An evaluation of safety and survival for patients with locally advanced pancreatic cancer treated with irreversible electroporation combined with chemotherapy: a retrospectively observational study. J Pancreatol. (2022) 5:27–35. doi: 10.1097/JP9.0000000000000088
143. Wu Y, Yan Y, Guo Y, Niu M, Zhou B, Zhang J, et al. Anti-TGF-β/PD-L1 bispecific antibody synergizes with radiotherapy to enhance antitumor immunity and mitigate radiation-induced pulmonary fibrosis. J Hematol Oncol. (2025) 18:24. doi: 10.1186/s13045-025-01678-2
144. Zhang G, Li M, Zhou D, Yang X, Zhang W, Gao R. Loss of endothelial EMCN drives tumor lung metastasis through the premetastatic niche. J Transl Med. (2022) 20:446. doi: 10.1186/s12967-022-03649-4
145. Niu M, Yi M, Wu Y, Lyu L, He Q, Yang R, et al. Synergistic efficacy of simultaneous anti-TGF-β/VEGF bispecific antibody and PD-1 blockade in cancer therapy. J Hematol Oncol. (2023) 16:94. doi: 10.1186/s13045-023-01487-5
146. Wang C, Al-ani MK, Sha Y, Chi Q, Dong N, Yang L, et al. Psoralen protects chondrocytes, exhibits anti-inflammatory effects on synoviocytes, and attenuates monosodium iodoacetate-induced osteoarthritis. Int J Biol Sci. (2019) 15:229–38. doi: 10.7150/ijbs.28830
147. Gough NR, Xiang X, Mishra L. TGF-β Signaling in liver, pancreas, and gastrointestinal diseases and cancer. Gastroenterology. (2021) 161:434–452.e15. doi: 10.1053/j.gastro.2021.04.064
148. Gao Y, Shan N, Zhao C, Wang Y, Xu F, Li J, et al. LY2109761 enhances cisplatin antitumor activity in ovarian cancer cells. Int J Clin Exp Pathol. (2015) 8:4923–32.
149. Tao S, Liu M, Shen D, Zhang W, Wang T, Bai Y. TGF-β/smads signaling affects radiation response and prolongs survival by regulating DNA repair genes in Malignant glioma. DNA Cell Biol. (2018) 37:909–16. doi: 10.1089/dna.2018.4310
150. Pietrobono S, Sabbadini F, Bertolini M, Mangiameli D, De Vita V, Fazzini F, et al. Autotaxin secretion is a stromal mechanism of adaptive resistance to TGFβ Inhibition in pancreatic ductal adenocarcinoma. Cancer Res. (2024) 84:118–32. doi: 10.1158/0008-5472.CAN-23-0104
151. Cui J, Wu D, Zhou H, Chen H, Dong Z, Yang J. Natural Chinese herbs for the prevention and treatment of acute pancreatitis: A narrative review. J Pancreatol. (2022) 5:186–97. doi: 10.1097/JP9.0000000000000111
152. Mucciolo G, Araos Henríquez J, Jihad M, Pinto Teles S, Manansala JS, Li W, et al. EGFR-activated myofibroblasts promote metastasis of pancreatic cancer. Cancer Cell. (2024) 42:101–118.e11. doi: 10.1016/j.ccell.2023.12.002
153. Zhou J, Lyu N, Wang Q, Yang M, Kimchi ET, Cheng K, et al. A novel role of TGFBI in macrophage polarization and macrophage-induced pancreatic cancer growth and therapeutic resistance. Cancer Lett. (2023) 578:216457. doi: 10.1016/j.canlet.2023.216457
154. Lai X-N, Li J, Tang L-B, Chen W-T, Zhang L, Xiong L-X. MiRNAs and lncRNAs: dual roles in TGF-β Signaling-regulated metastasis in lung cancer. Int J Mol Sci. (2020) 21:1193. doi: 10.3390/ijms21041193
155. Huang H, Zhu X, Yu Y, Li Z, Yang Y, Xia L, et al. EGFR mutations induce the suppression of CD8+ T cell and anti-PD-1 resistance via ERK1/2-p90RSK-TGF-β axis in non-small cell lung cancer. J Transl Med. (2024) 22:653. doi: 10.1186/s12967-024-05456-5
156. Zhang S, Wang Y, Luo D, Cheng Z, Zeng Q, Wang G, et al. Pirfenidone inhibits TGF-β1-induced metabolic reprogramming during epithelial-mesenchymal transition in non-small cell lung cancer. J Cell Mol Med. (2024) 28:e18059. doi: 10.1111/jcmm.18059
157. Jeon H-S, Jen J. TGF-beta signaling and the role of inhibitory Smads in non-small cell lung cancer. J Thorac Oncol. (2010) 5:417–9. doi: 10.1097/JTO.0b013e3181ce3afd
158. Gulley JL, Schlom J, Barcellos-Hoff MH, Wang X, Seoane J, Audhuy F, et al. Dual inhibition of TGF-β and PD-L1: a novel approach to cancer treatment. Mol Oncol. (2022) 16:2117–34. doi: 10.1002/1878-0261.13146
159. Li Y, Wang H, Chen M, Ma X. The immune subtype contributes to distinct overall survival for ovarian cancer patients with platinum-based adjuvant therapy. Front Immunol. (2022) 13:872991. doi: 10.3389/fimmu.2022.872991
160. Li Z, Zhou W, Zhang Y, Sun W, Yung MMH, Sun J, et al. ERK regulates HIF1α-mediated platinum resistance by directly targeting PHD2 in ovarian cancer. Clin Cancer Res. (2019) 25:5947–60. doi: 10.1158/1078-0432.CCR-18-4145
161. Zhang M, Zhang YY, Chen Y, Wang J, Wang Q, Lu H. TGF-β Signaling and resistance to cancer therapy. Front Cell Dev Biol. (2021) 9:786728. doi: 10.3389/fcell.2021.786728
Keywords: transforming growth factor-beta, cancer-associated fibrosis, targeted therapies, cancer biology, epithelial-mesenchymal transition
Citation: Jing H, Gao Y, Jing L, Yang H and Liu S (2025) Recent advances in therapeutic use of transforming growth factor-beta inhibitors in cancer and fibrosis. Front. Oncol. 15:1489701. doi: 10.3389/fonc.2025.1489701
Received: 01 September 2024; Accepted: 03 April 2025;
Published: 25 April 2025.
Edited by:
Matiullah Khan, AIMST University, MalaysiaCopyright © 2025 Jing, Gao, Jing, Yang and Liu. This is an open-access article distributed under the terms of the Creative Commons Attribution License (CC BY). The use, distribution or reproduction in other forums is permitted, provided the original author(s) and the copyright owner(s) are credited and that the original publication in this journal is cited, in accordance with accepted academic practice. No use, distribution or reproduction is permitted which does not comply with these terms.
*Correspondence: Shanglong Liu, bGl1c2hhbmdsb25nQHFkdS5lZHUuY24=