- Department of Urology, the Third Xiangya Hospital, Central South University, Changsha, China
Immune checkpoint blockers (ICBs) have revolutionized cancer treatment by enabling durable responses. However, most patients showed resistance and limited efficacy. Elucidating mechanisms of resistance is imperative to extend the clinical utility of ICBs. Emerging evidence highlights cancer-associated fibroblasts (CAFs), particularly TGFβ-activated myofibroblastic CAFs, as key orchestrators of immunosuppressive TMEs and ICBs resistance. These CAFs drive T-cell exclusion preventing intratumoral T cells from engaging cancer cells. This review explores the role of TGFβ signaling in CAFs in driving immune evasion and therapy resistance. While targeting TGFβ or CAFs directly has shown limited inconsistent results, downstream molecules in TGFβ-activated CAFs, including induced TGFβ (βig-h3), endocytic receptor 180 (Endo180), leucine-rich repeat containing 15 (LRRC15), and NADPH oxidase 4 (NOX4), emerge as promising therapeutic targets to counteract T-cell exclusion and restore immunotherapy sensitivity. Advancing research on CAF heterogeneity and pro-tumorigenic subsets may uncover innovative strategies to expand immunotherapy benefits.
1 Introduction
Immune checkpoint blockers (ICBs), especially PD1/PDL1 inhibitors, have revolutionized cancer treatment due to their potential for durable responses and long-term disease control. However, only a subset of patients benefit from ICBs, with most being resistant (1). The efficacy of ICBs depends on pre-existing T-cell infiltration into tumors. However, high T-cell density alone does not guarantee a response. The spatial distribution of T cells is also critical, with physical interaction between T cells and cancer cells required for ICB efficacy (1).
Solid tumors are currently stratified into the following three distinct immune phenotypes based on T-cell spatial distribution (2): 1) inflamed/hot tumors, characterized by abundant intratumoral T-cell infiltration, yet frequently resistant to ICIs due to upregulation of alternative checkpoints (TIM3, LAG3, TIGIT) and non-PD-1/CTLA4-mediated inhibitory pathways (3, 4); 2) desert/cold tumors, defined by minimal T-cell presence, primarily attributed to defective antigen presentation or failed T-cell priming necessitating combinatorial strategies with immunogenic priming agents (e.g., neoantigen vaccines, oncolytic viruses) (5); and 3) immune-excluded tumors, distinguished by CD8+ T-cell sequestration within tumor stroma, forming a “peripheral immune shield”—host-derived antitumor T cells are physically barred from infiltrating cancer nests resulting in poor prognosis and primary resistance to ICIs (6).
Multiple factors likely contribute to T-cell exclusion, but cancer-associated fibroblasts (CAFs), specifically TGFβ-activated myofibroblastic CAFs, are increasingly recognized as major orchestrators. Via secretion of extracellular matrix proteins and other immunomodulators, these CAFs create fibrotic, immunosuppressive TMEs that restrict T-cell migration into tumor nests (7). The respective mechanisms underlying ICI resistance for the three immune subtypes and the corresponding methods for reversing are illustrated in Figure 1.
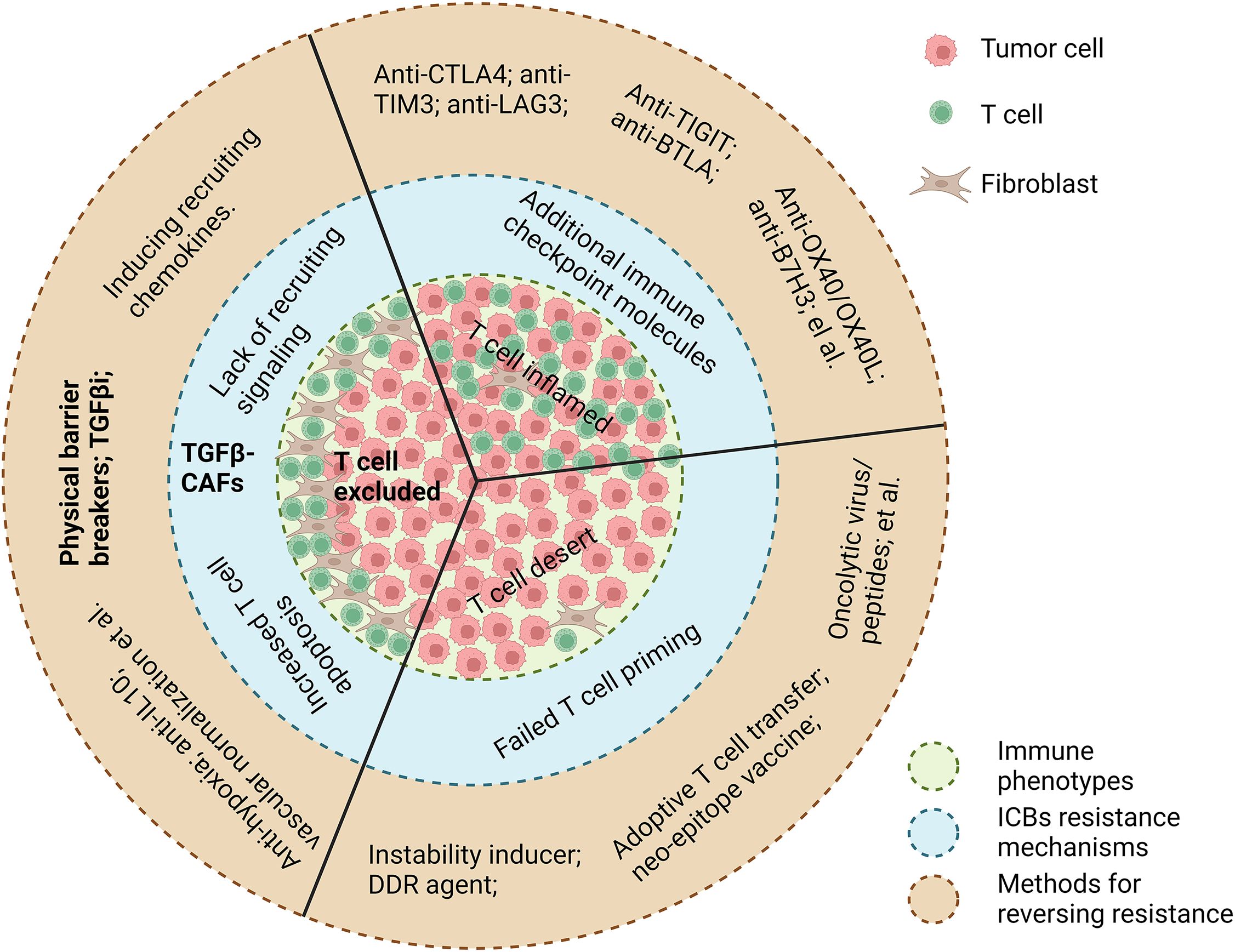
Figure 1. A summary of current knowledge on ICB resistance mechanisms underlying three immune phenotypes, respectively, and their corresponding methods for reversing resistance. Image created with Biorender.com.
This review synthesizes recent advances in our understanding on TGFβ-activated CAF-mediated T-cell exclusion, evaluates challenges in targeting TGFβ/CAFs, and highlights downstream effectors (e.g., LRRC15, NOX4) as precision therapeutic targets to restore ICB sensitivity.
2 T-cell exclusion: prognostic and predictive implications
As the main activators of antitumor immunity, tumor infiltrating T cells have been intensely investigated as prognostic and predictive biomarkers. However, until now, they have experienced limited clinical application because of modest efficacy and lack of applicable evaluation methods. The effect of spatial distribution of tumor infiltrating T cells on patients’ prognosis has been recognized for more than two decades (8, 9). The concept of T-cell exclusion, however, has emerged with the advent of the immunotherapy era, despite two decades of research on T-cell spatial distribution. A triple-negative breast cancer study revealed 10-year survival rates of 80% for inflamed tumors, 60% for excluded tumors, and 40% for desert tumors. Spatial phenotypes were prognostic independent of factors like nodal status, tumor size, and age (10). Artificial intelligence (AI) was recently leveraged to analyze non-small cell lung cancer tumors revealing 44% inflamed tumors, 37% excluded tumors, and 19% desert tumors. This could provide a supplementary biomarker to immunohistochemistry (11).
Besides its role in prognosis, T-cell exclusion is evolving as a potential biomarker for immunotherapy resistance. In two cohorts of patients with advanced NSCLC treated with monotherapy ICBs, the overall response rate to ICBs in patients with immune-excluded phenotype was 11.5%, compared with 26.8% and 11.2% in patients with inflamed and immune-desert phenotypes, respectively. Median progression-free survival and overall survival among patients with the immune-excluded phenotype were also significantly shorter than those with the inflamed phenotype (2.2 and 14.0 months vs. 4.1 and 24.8 months) (11). T-cell exclusion signatures could also predict resistance to ICBs (12), while a T cell-inflamed signature (13, 14) or a T-cell infiltration signature (15) corresponds highly with a clinical response to ICBs in multiple tumor types. T-cell exclusion might be responsible for both primary (16) as well as acquired ICB resistance (17).
3 MyoCAFs: architects of T-cell exclusion
CAFs are one of the most abundant and critical components of the TME, which not only provide physical support but also play a key role in metabolic and immune reprogramming of the TME (18). Evidence shows that CAFs dominate the complex cell–cell interactions in the TME (19). The role of CAFs in promoting the establishment of an immunosuppressive TME in various cancers is increasingly appreciated. Highly stromagenic cancers, such as pancreatic cancer, respond particularly poorly to ICB treatments. Lung fibrosis impairs T cell-mediated tumor control and limits the benefit of ICB treatment in NSCLC (20).
As the main drivers of stromagenesis and fibrosis, CAFs directly orchestrate CD8+ T-cell exclusion (21), thereby shaping an immunologically cold TME. This causal relationship is evidenced by dose-dependent correlations between CAF density and the excluded tumor phenotype (22). However, CAFs within tumors are not uniform, but have extensive heterogeneity, with at least two major CAFs subtypes, myoCAFs and inflammatory CAFs (iCAFs) (23–25). MyoCAFs have a matrix-producing myofibroblastic phenotype with high expression of α-smooth muscle actin (αSMA). By contrast, iCAFs have a secretory phenotype with the ability to generate immunomodulatory molecules such as interleukin 6 and C-X-C motif chemokine ligand 12 (26). Determining the heterogeneity within the CAFs is indispensable to better understand and treat cancers.
By using multicolor flow cytometry, a seminal breast cancer study identified four CAF subsets with distinct immunomodulatory capacities, with the αSMA-high CAF-S1 subset most deeply involved in establishing an immunosuppressive TME (27). Their subsequent work based on single-cell RNA sequencing (scRNA-seq) uncovered delineated further heterogeneity within the CAF-S1 subset revealing the role of myofibroblastic CAF subsets (ecm/TGFβ/wound-myoCAF) in orchestrating T-cell exclusion and ICB resistance (28). This role of the myoCAFs has been corroborated by another group (29).
MyoCAFs are increasingly recognized as key mediators in driving immune exclusion and resistance to ICB therapies through dual mechanisms as follows: (1) promoting fibrotic TMEs and (2) forming functional alliances with immunosuppressive immune cells, particularly tumor-associated macrophages (TAMs). Spatial analysis from a landmark Chinese study revealed that myoCAFs exhibit intensive cross-talk with TAMs to create physical (ECM and collagen organization) and biochemical (chemotaxis regulation) barriers that restrict cytotoxic T-lymphocyte penetration (30). The tumor-promoting role of myoCAFs has been validated through pan-cancer analysis encompassing 18 malignancies using a cell-type deconvolutional approach. The results showed that cancers with high numbers of myoCAFs have poorer prognosis and lower drug response sensitivity (31), while targeting myoCAFs increases infiltration of cytotoxic CD8+ T cells into the tumor parenchyma and improves ICB efficacy (32). These collective findings position myoCAFs as central architects of the immune-excluded phenotype and critical facilitators of tumor progression.
4 TGFβ-activated CAFs: fueling ICB resistance and T-cell exclusion
TGFβ is a well-established inducer of myoCAFs, in contrast to interleukin 1 and fibroblast growth factor, which drive the formation of iCAFs (33–35). Studies have highlighted the crucial role of TGFβ-activated CAFs in predicting resistance to ICBs across various cancers.
A Canadian study found that TGFβ-dysregulated CAF ECM genes predict PD1/PDL1 resistance better than established biomarkers like the tumor mutation burden, cytolytic activity, T cell-inflamed signature, TGFβ expression alone, and the CAF signature (36). Another study showed that a stroma/epithelial-to-mesenchymal transition (EMT)/TGFβ signature negatively associates with pembrolizumab response across cancers (37). TGFβ-responsive CAF signatures define poor prognosis subtypes and predict ICB resistance in colorectal cancer (38), including microsatellite instability high/mismatch repair-deficient cancers normally susceptible to ICBs (39). For urothelial carcinoma, TGFβ signaling in CAFs associated with ICB resistance in both metastatic (7) and neoadjuvant settings (40). TGFβ-activated CAFs are also responsible for T-cell exclusion in ovarian cancer (41).
Besides the significant link between TGFβ-activated CAFs and ICB resistance, in vivo and in vitro models recapitulating the role TGFβ-activated CAFs in driving T-cell exclusion have been developed. The EMT6 (epithelial cell line) and MC38 mouse mammary carcinoma models seem to naturally exhibit a T cell-excluded phenotype. Therapeutic blockade of PDL1 or TGFβ alone achieved little or no effect in these mice, while blocking both PD-L1 and TGFβ exhibited a significant reduction in tumor burden by promoting significant infiltration of T cells into the tumor nest (7).
A more elegant work was reported by a Spanish team, who generated mouse strains bearing combined mutations of Apc, Kras, Tgfbr2, and Trp53 in intestinal stem cells. These mice developed highly invasive colon cancer with 90% prevalence and reproduced key features of the T-cell exclusion phenotype induced by a prominent TGFβ-activated stroma. PD1/PDL1 inhibitors provoked a limited response in this model. In contrast, inhibition of TGFβ unleashed a potent response to PD1/PDL1 inhibitors (42). However, that study did not explain why these autochthonous tumors developed a TGFβ-activated stroma. Recently, another group performed unbiased analysis for regulators of the TME using spatial functional genomics and found that the Tgfbr2 knockout in cancer cells could increase TGFβ bioavailability, drive TGFβ-mediated CAF activation, and convert the TME to a fibro-mucinous state inducing T-cell exclusion and ICB resistance (43). This conclusion echoes the findings from the Spanish team and might provide a reproducible method to build mouse models of T cell-excluded tumors by knockout of the Tgfbr2 gene.
Another team described a preclinical model named the skin tumor array by microporation (STAMP), to characterize spatiotemporal immune response patterns, and showed enriched myofibroblasts and TGFβ activation in excluded tumors. Interestingly, immune phenotypes seem stochastic, not predetermined by genetics. Fibroblasts have a complex dual role—they drive exclusion but also orchestrate inflammation by recruiting T cells. Depleting fibroblasts increased desert tumors in STAMP revealing the multifaceted functions of fibroblasts in shaping immune phenotypes (16). These findings underscore the need to elucidate specific mechanisms and fibroblast heterogeneity.
Despite established links between TGFβ-activated CAFs and PD-1/PD-L1 resistance, therapeutic targeting remains fraught with paradoxes. Non-specific ablation of myoCAFs (e.g., αSMA+ depletion or Sonic hedgehog inhibition) not only fails to restore immune infiltration but accelerates tumor progression (44–47) likely due to inadvertent disruption of tumor-restraining CAF subsets within the population (Figure 2) (48, 49).
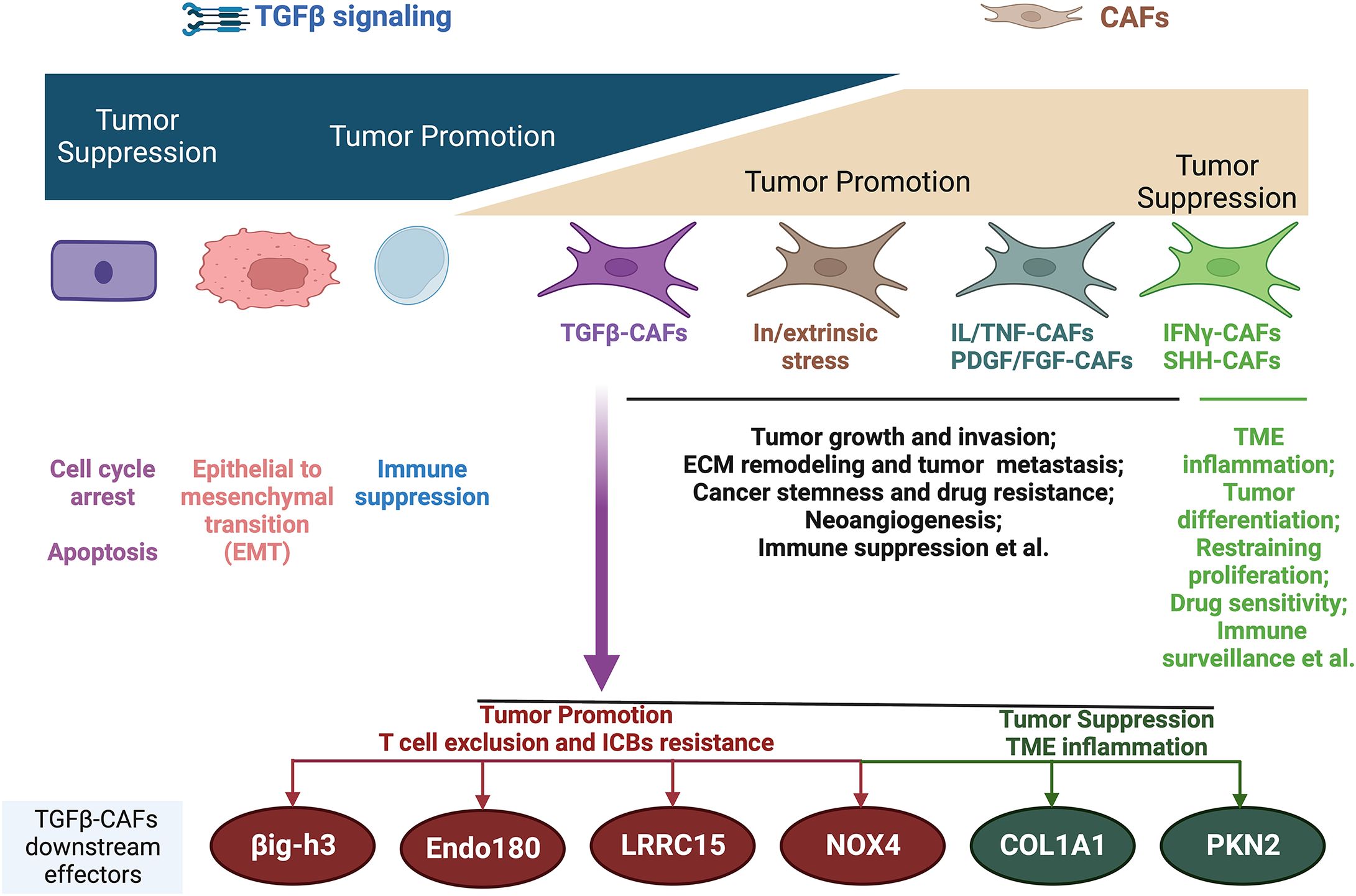
Figure 2. Biphasic functions of TGFβ and CAFs during cancer progression and the main mechanisms. TGFβ-CAFs emerge as the main contributors to T-cell exclusion immune phenotype and ICB resistance, with several downstream molecules being leveraged to reverse T-cell exclusion and ICB resistance. However, it should be noted that the downstream effectors of TGFβ-CAFs are functionally multifaceted, with some downstream molecules having tumor-suppressive roles. IL, interleukin; TNF, tumor necrosis factor; PDGF, platelet-derived growth factor; FGF, fibroblast growth factor; IFNγ, interferon-γ; SHH, Sonic Hedgehog; ECM, extracellular matrix; TME, tumor microenvironment. Image created with Biorender.com.
Similarly, while preclinical models demonstrate synergy between TGFβ inhibitors and ICBs (7, 42), clinical trials reveal limited efficacy and unforeseen risks—TGFβ blockade may paradoxically promote metastasis and on-target toxicity (50–52). These contradictions stem from TGFβ’s context-dependent duality: it constrains early tumors via growth suppression yet drives advanced disease through stromal activation and immune evasion (52). TGFβ’s dual tumor-suppressive/promoting roles demand subset-specific targeting strategies.
5 Precision targeting TGFβ-activated CAFs
Studies suggested that nonspecific targeting of CAFs or TGFβ signaling in tumors might not suppress tumor progression; therefore, specific targeting of TGFβ-activated CAFs subtypes might be needed to improve the clinical outcome. Determining the molecular drivers of TGFβ-activated CAFs in T-cell exclusion might provide the understanding needed to design more effective immunotherapeutic approaches and address the unmet clinical need in ICB-resistant T cell-excluded cancers.
The well-established downstream effectors of TGFβ-activated CAFs are the ECM proteins, the dysregulation of which predicts a failure of PD-1 blockade across cancers (36, 53). One study identified ECM protein collagen type XIII alpha 1 chain as a biomarker for the TGFβ-responsive CAF subpopulation that produces chemokines to limit recruitment of dendritic cells and T cells into the tumor nest, thus leading to poor responsiveness to anti-PD1/PDL1 therapy in lung cancer (20). However, they did not provide ways to specifically target these distinct CAFs. βig-h3 (also known as induced TGFβ) is another ECM protein that is regulated by TGFβ in CAFs. βig-h3-secreting CAFs within the stroma can sequester T cells in the stroma leading to a T cell-excluded phenotype. Depletion of βig-h3 could drive the accumulation of CD8+ T cells and enhance the antitumoral response (54). Anti-βig-h3 antibody has exhibited promising anti-tumor efficacy by reshaping TMEs (55). Therefore, βig-h3 represents a novel and promising immunological target among ECM proteins in cancer.
A seminal work from Genentech reported an unbiased assessment of fibroblast heterogeneity in pancreatic ductal adenocarcinoma tissues by bulk and scRNA-seq of stromal cells. They identified LRRC15 as a marker of TGFβ-driven myoCAFs, which were the dominant fibroblasts in advanced tumors across multiple human cancer types (56). LRRC15 is a transmembrane protein that is physiologically involved in cell–cell and cell–ECM interactions (57). The LRRC15+ CAF signature has been found to not only predicts worse survival but also poor treatment response in patients receiving anti-PDL1 therapy (56, 58, 59). Recently, LRRC15 serves as a definitive marker for a terminally differentiated myoCAF subset that orchestrates immune-excluded TMEs (60). Further work revealed that LRRC15+ CAF depletion leads to persistent reduction in the tumor burden and significantly boosts anti-PD1/PDL1 treatment responses (61). However, targeting LRRC15 clinically seems difficult due to its poorly characterized functions and signaling pathways. Interestingly, recent advances in antibody–drug conjugates (ADCs) have produced a wide range of “magic bullets” to address previously unmet medical needs (62). LRRC15 shows highly restricted expression in some pro-tumorigenic and immunosuppressive CAFs; therefore, it has become a good cancer-specific antigen for therapeutic targeting. LRRC15-targeted ADCs, such as the ABBV-085 ADC conjugated with monomethyl auristatin E (MMAE) payloads, are under development, and promising results are emerging (57, 63).
Endocytic receptor 180 (also known as urokinase-type plasminogen activator receptor-associated protein, encoded by the MRC2 gene, is an endocytic receptor participating in collagenolysis and ECM turnover. The expression of Endo180 is physiologically restricted to normal tissue fibroblasts. Recent advances revealed that Endo180 is upregulated on a subset of myoCAFs and plays a critical role in mediating TGFβ-induced collagen accumulation and contractility. Genetic deletion of ENDO180 profoundly limited tumor growth and metastasis (64). The same groups’ subsequent work revealed the role of Endo180+ CAFs in driving the T-cell exclusion phenotype. High levels of Endo180 in tumors predict a poor response to PD1/PDL1 inhibitor therapy, and knockout of ENDO180 in CAFs promotes T-cell infiltration and enhanced sensitivity to PD1/PDL1 inhibitor therapy (22). The fact that Endo180 is specifically upregulated on a subset of tumor-promoting myoCAFs and is a constitutively recycled transmembrane receptor makes Endo180 an ideal target antigen to design an ADC. Currently, an Endo180-ADC with a payload of MMAE has been tested in a preclinical sarcoma tumor xenograft model resulting not only in tumor regression but also in a significant reduction of metastasis. Endo180-ADC could be rapidly internalized into target cells and cause specific cell death in Endo180-expressing CAFs (65).
NOX4 has been identified as another major downstream target of TGFβ-activated CAFs to promote T-cell exclusion and anti-PD1/PDL1 resistance (66). NOX4 catalyzes the reduction of O2 to produce reactive oxygen species (ROS), which are required for TGFβ-dependent myofibroblast transdifferentiation in various organ fibroses and malignancies (67–69). Pharmacological inhibition of NOX4 using GKT137831, an oral drug developed as an anti-fibrotic agent, abrogated TGFβ-dependent ROS production, myofibroblast transdifferentiation, and slow tumor growth (70). By using CAF-rich murine tumor models with a T-cell exclusion phenotype, the role of NOX4 inhibition in normalizing myoCAFs to a quiescent phenotype and overcoming T-cell exclusion by increasing intratumoral T-cell infiltration has been revealed. By contrast, TGFβ inhibition alone could prevent, but not reverse, CAF differentiation. This preclinical study showed that NOX4 inhibition could restore the response to immunotherapy by overcoming CAF-mediated T-cell exclusion (66).
Recent findings reveal tumor-restraining functions of some TGFβ-activated CAF effectors emphasizing the need for a nuanced understanding. Collagen 1A1, a TGFβ-induced effector, suppresses tumors when deleted in myoCAFs by recruiting immunosuppressive myeloid-derived suppressor cells (71, 72). Preserving collagen while targeting other CAF mediators is suggested. Similarly, the TGFβ mediator, Rho effector kinase protein kinase N2 (PKN2), restrains invasion, as its deletion switches myoCAFs to inflammatory CAFs (73). These results highlight the heterogeneity of CAFs, as well as myoCAFs, cautioning against blanket CAF targeting and warranting elucidating the multifaceted TGFβ–CAF roles.
6 Discussion
TGFβ-activated CAFs have emerged as critical drivers of immunotherapy resistance and the T cell-excluded phenotype across various cancers. However, blanket targeting of CAFs or TGFβ has yielded mixed results clinically. Therefore, further research should focus on identifying and selectively targeting the specific downstream mediators induced by TGFβ in the subpopulations of tumor-promoting CAFs. Promising targets in early investigation include ECM proteins like βig-h3, membrane receptors like Endo180 and LRRC15, and cytosolic enzymes like NOX4. These effectors promote the immunosuppressive and T cell-excluding effects of TGFβ-activated CAFs and are absent or minimal in normal tissues. Drugs targeting them may provide more precise immunotherapies. Particularly promising are ADC drugs targeting specific membrane proteins induced by TGFβ in pro-tumorigenic CAFs exemplified by LRRC15 and Endo180-targeted ADCs.
Additionally, single-cell profiling has revealed further heterogeneity within CAF populations, so future work should examine how different CAF subtypes interact to shape distinct immune phenotypes. A nuanced understanding of CAF subsets may enable selective elimination of pro-tumorigenic CAFs while preserving anti-tumorigenic subtypes. Defining the spatiotemporal dynamics of CAF activation and crosstalk with other stromal and immune components is also warranted. Overall, research on the multi-faceted roles of CAFs and their subpopulations will uncover new strategies to overcome resistance and expand the benefits of cancer immunotherapy to more patients.
Author contributions
WH: Methodology, Visualization, Data curation, Project administration, Investigation, Conceptualization, Validation, Supervision, Resources, Funding acquisition, Writing – review & editing, Software, Formal Analysis, Writing – original draft.
Funding
The author(s) declare that financial support was received for the research and/or publication of this article. This study was supported in part by the National Natural Science Foundation of China (82002679), Natural Science Foundation of Hunan Province (2023JJ40890), and China Postdoctoral Science Foundation (2019M653196).
Conflict of interest
The author declares that the research was conducted in the absence of any commercial or financial relationships that could be construed as a potential conflict of interest.
Generative AI statement
The author(s) declare that no Generative AI was used in the creation of this manuscript.
Publisher’s note
All claims expressed in this article are solely those of the authors and do not necessarily represent those of their affiliated organizations, or those of the publisher, the editors and the reviewers. Any product that may be evaluated in this article, or claim that may be made by its manufacturer, is not guaranteed or endorsed by the publisher.
Glossary
ADCs: antibody–drug conjugates
AI: artificial intelligence
BTLA: molecule B and T-lymphocyte attenuator
CAFs: cancer-associated fibroblasts
ECM: extracellular matrix
EMT: epithelial-to-mesenchymal transition
Endo180: endocytic receptor 180
iCAFs: inflammatory CAFs
ICBs: immune checkpoint blockers
LRRC15: leucine-rich repeat containing 15
MMAE: monomethyl auristatin E
myoCAFs: myofibroblastic CAFs
NOX4: NADPH oxidase 4
NSCLC: non-small-cell lung cancer
OX40: TNF superfamily member 4
OX40L: ligand of OX40
PD1: programmed cell death 1
PDL1: programmed death ligand 1
PKN2: Rho effector kinase protein kinase N2
ROS: reactive oxygen species
scRNA-seq: single-cell RNA sequencing
STAMP: skin tumor array by microporation
TAMs: tumor-associated macrophages
TGFβ: transforming growth factor beta
TME: tumor microenvironment
αSMA: α-smooth muscle actin
βig-h3: induced TGFβ.
References
1. Joyce JA and Fearon DT. T cell exclusion, immune privilege, and the tumor microenvironment. Science. (2015) 348:74–80. doi: 10.1126/science.aaa6204
2. Mellman I, Chen DS, Powles T, and Turley SJ. The cancer-immunity cycle: Indication, genotype, and immunotype. Immunity. (2023) 56:2188–205. doi: 10.1016/j.immuni.2023.09.011
3. Wang Y, Zhang H, Liu C, Wang Z, Wu W, Zhang N, et al. Immune checkpoint modulators in cancer immunotherapy: recent advances and emerging concepts. J Hematol Oncol. (2022) 15:111. doi: 10.1186/s13045-022-01325-0
4. Marin-Acevedo JA, Dholaria B, Soyano AE, Knutson KL, Chumsri S, and Lou Y. Next generation of immune checkpoint therapy in cancer: new developments and challenges. J Hematol Oncol. (2018) 11:39. doi: 10.1186/s13045-018-0582-8
5. Galon J and Bruni D. Approaches to treat immune hot, altered and cold tumours with combination immunotherapies. Nat Rev Drug Discovery. (2019) 18:197–218. doi: 10.1038/s41573-018-0007-y
6. Clifton GT, Rothenberg M, Ascierto PA, Begley G, Cecchini M, Eder JP, et al. Developing a definition of immune exclusion in cancer: results of a modified Delphi workshop. J Immunother Cancer. (2023) 11:e006773. doi: 10.1136/jitc-2023-006773
7. Mariathasan S, Turley SJ, Nickles D, Castiglioni A, Yuen K, Wang Y, et al. TGFbeta attenuates tumour response to PD-L1 blockade by contributing to exclusion of T cells. Nature. (2018) 554:544–8. doi: 10.1038/nature25501
8. Naito Y, Saito K, Shiiba K, Ohuchi A, Saigenji K, Nagura H, et al. CD8+ T cells infiltrated within cancer cell nests as a prognostic factor in human colorectal cancer. Cancer Res. (1998) 58:3491–4.
9. Galon J, Costes A, Sanchez-Cabo F, Kirilovsky A, Mlecnik B, Lagorce-Pages C, et al. Type, density, and location of immune cells within human colorectal tumors predict clinical outcome. Science. (2006) 313:1960–4. doi: 10.1126/science.1129139
10. Hammerl D, Martens JWM, Timmermans M, Smid M, Trapman-Jansen AM, Foekens R, et al. Spatial immunophenotypes predict response to anti-PD1 treatment and capture distinct paths of T cell evasion in triple negative breast cancer. Nat Commun. (2021) 12:5668. doi: 10.1038/s41467-021-25962-0
11. Park S, Ock CY, Kim H, Pereira S, Park S, Ma M, et al. Artificial intelligence-powered spatial analysis of tumor-infiltrating lymphocytes as complementary biomarker for immune checkpoint inhibition in non-small-cell lung cancer. J Clin Oncol. (2022) 40:1916–28. doi: 10.1200/JCO.21.02010
12. Jiang P, Gu S, Pan D, Fu J, Sahu A, Hu X, et al. Signatures of T cell dysfunction and exclusion predict cancer immunotherapy response. Nat Med. (2018) 24:1550–8. doi: 10.1038/s41591-018-0136-1
13. Cristescu R, Mogg R, Ayers M, Albright A, Murphy E, Yearley J, et al. Pan-tumor genomic biomarkers for PD-1 checkpoint blockade-based immunotherapy. Science. (2018) 362:eaar3593. doi: 10.1126/science.aar3593
14. Ott PA, Bang YJ, Piha-Paul SA, Razak ARA, Bennouna J, Soria JC, et al. T-cell-inflamed gene-expression profile, programmed death ligand 1 expression, and tumor mutational burden predict efficacy in patients treated with pembrolizumab across 20 cancers: KEYNOTE-028. J Clin Oncol. (2019) 37:318–27. doi: 10.1200/JCO.2018.78.2276
15. Grasso CS, Tsoi J, Onyshchenko M, Abril-Rodriguez G, Ross-Macdonald P, Wind-Rotolo M, et al. Conserved interferon-gamma signaling drives clinical response to immune checkpoint blockade therapy in melanoma. Cancer Cell. (2020) 38:500–15.e503. doi: 10.1016/j.ccell.2020.08.005
16. Ortiz-Munoz G, Brown M, Carbone CB, Pechuan-Jorge X, Rouilly V, Lindberg H, et al. In situ tumour arrays reveal early environmental control of cancer immunity. Nature. (2023) 618:827–33. doi: 10.1038/s41586-023-06132-2
17. Wang Y, Lu LC, Guan Y, Ho MC, Lu S, Spahn J, et al. Atezolizumab plus bevacizumab combination enables an unresectable hepatocellular carcinoma resectable and links immune exclusion and tumor dedifferentiation to acquired resistance. Exp Hematol Oncol. (2021) 10:45. doi: 10.1186/s40164-021-00237-y
18. Sahai E, Astsaturov I, Cukierman E, DeNardo DG, Egeblad M, Evans RM, et al. A framework for advancing our understanding of cancer-associated fibroblasts. Nat Rev Cancer. (2020) 20:174–86. doi: 10.1038/s41568-019-0238-1
19. Mayer S, Milo T, Isaacson A, Halperin C, Miyara S, Stein Y, et al. The tumor microenvironment shows a hierarchy of cell-cell interactions dominated by fibroblasts. Nat Commun. (2023) 14:5810. doi: 10.1038/s41467-023-41518-w
20. Herzog BH, Baer JM, Borcherding N, Kingston NL, Belle JI, Knolhoff BL, et al. Tumor-associated fibrosis impairs immune surveillance and response to immune checkpoint blockade in non-small cell lung cancer. Sci Transl Med. (2023) 15:eadh8005. doi: 10.1126/scitranslmed.adh8005
21. Song D, Wu Y, Li J, Liu J, Yi Z, Wang X, et al. Insulin-like growth factor 2 drives fibroblast-mediated tumor immunoevasion and confers resistance to immunotherapy. J Clin Invest. (2024) 134:e183366. doi: 10.1172/JCI183366
22. Jenkins L, Jungwirth U, Avgustinova A, Iravani M, Mills A, Haider S, et al. Cancer-associated fibroblasts suppress CD8+ T-cell infiltration and confer resistance to immune-checkpoint blockade. Cancer Res. (2022) 82:2904–17. doi: 10.1158/0008-5472.CAN-21-4141
23. Kieffer Y, Hocine HR, Gentric G, Pelon F, Bernard C, Bourachot B, et al. Single-cell analysis reveals fibroblast clusters linked to immunotherapy resistance in cancer. Cancer Discovery. (2020) 10:1330–51. doi: 10.1158/2159-8290.CD-19-1384
24. Elyada E, Bolisetty M, Laise P, Flynn WF, Courtois ET, Burkhart RA, et al. Cross-species single-cell analysis of pancreatic ductal adenocarcinoma reveals antigen-presenting cancer-associated fibroblasts. Cancer Discovery. (2019) 9:1102–23. doi: 10.1158/2159-8290.CD-19-0094
25. Ohlund D, Handly-Santana A, Biffi G, Elyada E, Almeida AS, Ponz-Sarvise M, et al. Distinct populations of inflammatory fibroblasts and myofibroblasts in pancreatic cancer. J Exp Med. (2017) 214:579–96. doi: 10.1084/jem.20162024
26. Chhabra Y and Weeraratna AT. Fibroblasts in cancer: Unity in heterogeneity. Cell. (2023) 186:1580–609. doi: 10.1016/j.cell.2023.03.016
27. Costa A, Kieffer Y, Scholer-Dahirel A, Pelon F, Bourachot B, Cardon M, et al. Fibroblast heterogeneity and immunosuppressive environment in human breast cancer. Cancer Cell. (2018) 33:463–79.e410. doi: 10.1016/j.ccell.2018.01.011
28. Grout JA, Sirven P, Leader AM, Maskey S, Hector E, Puisieux I, et al. Spatial positioning and matrix programs of cancer-associated fibroblasts promote T-cell exclusion in human lung tumors. Cancer Discovery. (2022) 12:2606–25. doi: 10.1158/2159-8290.CD-21-1714
29. Peyraud F, Guegan JP, Rey C, Lara O, Odin O, Del Castillo M, et al. Spatially resolved transcriptomics reveal the determinants of primary resistance to immunotherapy in NSCLC with mature tertiary lymphoid structures. Cell Rep Med. (2025) 6:101934. doi: 10.1016/j.xcrm.2025.101934
30. Xun Z, Ding X, Zhang Y, Zhang B, Lai S, Zou D, et al. Reconstruction of the tumor spatial microenvironment along the Malignant-boundary-nonmalignant axis. Nat Commun. (2023) 14:933. doi: 10.1038/s41467-023-36560-7
31. Li B, Pei G, Yao J, Ding Q, Jia P, and Zhao Z. Cell-type deconvolution analysis identifies cancer-associated myofibroblast component as a poor prognostic factor in multiple cancer types. Oncogene. (2021) 40:4686–94. doi: 10.1038/s41388-021-01870-x
32. Broz MT, Ko EY, Ishaya K, Xiao J, De Simone M, Hoi XP, et al. Metabolic targeting of cancer associated fibroblasts overcomes T-cell exclusion and chemoresistance in soft-tissue sarcomas. Nat Commun. (2024) 15:2498. doi: 10.1038/s41467-024-46504-4
33. Biffi G, Oni TE, Spielman B, Hao Y, Elyada E, Park Y, et al. IL1-induced JAK/STAT signaling is antagonized by TGFbeta to shape CAF heterogeneity in pancreatic ductal adenocarcinoma. Cancer Discovery. (2019) 9:282–301. doi: 10.1158/2159-8290.CD-18-0710
34. Bordignon P, Bottoni G, Xu X, Popescu AS, Truan Z, Guenova E, et al. Dualism of FGF and TGF-beta signaling in heterogeneous cancer-associated fibroblast activation with ETV1 as a critical determinant. Cell Rep. (2019) 28:2358–72.e2356. doi: 10.1016/j.celrep.2019.07.092
35. Nicolas AM, Pesic M, Engel E, Ziegler PK, Diefenhardt M, Kennel KB, et al. Inflammatory fibroblasts mediate resistance to neoadjuvant therapy in rectal cancer. Cancer Cell. (2022) 40:168–84.e113. doi: 10.1016/j.ccell.2022.01.004
36. Chakravarthy A, Khan L, Bensler NP, Bose P, and De Carvalho DD. TGF-beta-associated extracellular matrix genes link cancer-associated fibroblasts to immune evasion and immunotherapy failure. Nat Commun. (2018) 9:4692. doi: 10.1038/s41467-018-06654-8
37. Cristescu R, Nebozhyn M, Zhang C, Albright A, Kobie J, Huang L, et al. Transcriptomic determinants of response to pembrolizumab monotherapy across solid tumor types. Clin Cancer Res. (2022) 28:1680–9. doi: 10.1158/1078-0432.CCR-21-3329
38. Guinney J, Dienstmann R, Wang X, de Reynies A, Schlicker A, Soneson C, et al. The consensus molecular subtypes of colorectal cancer. Nat Med. (2015) 21:1350–6. doi: 10.1038/nm.3967
39. Endo E, Okayama H, Saito K, Nakajima S, Yamada L, Ujiie D, et al. A TGFbeta-dependent stromal subset underlies immune checkpoint inhibitor efficacy in DNA mismatch repair-deficient/microsatellite instability-high colorectal cancer. Mol Cancer Res. (2020) 18:1402–13. doi: 10.1158/1541-7786.MCR-20-0308
40. Powles T, Kockx M, Rodriguez-Vida A, Duran I, Crabb SJ, van der Heijden MS, et al. Clinical efficacy and biomarker analysis of neoadjuvant atezolizumab in operable urothelial carcinoma in the ABACUS trial. Nat Med. (2019) 25:1706–14. doi: 10.1038/s41591-019-0628-7
41. Desbois M, Udyavar AR, Ryner L, Kozlowski C, Guan Y, Durrbaum M, et al. Integrated digital pathology and transcriptome analysis identifies molecular mediators of T-cell exclusion in ovarian cancer. Nat Commun. (2020) 11:5583. doi: 10.1038/s41467-020-19408-2
42. Tauriello DVF, Palomo-Ponce S, Stork D, Berenguer-Llergo A, Badia-Ramentol J, Iglesias M, et al. TGFbeta drives immune evasion in genetically reconstituted colon cancer metastasis. Nature. (2018) 554:538–43. doi: 10.1038/nature25492
43. Dhainaut M, Rose SA, Akturk G, Wroblewska A, Nielsen SR, Park ES, et al. Spatial CRISPR genomics identifies regulators of the tumor microenvironment. Cell. (2022) 185:1223–39.e1220. doi: 10.1016/j.cell.2022.02.015
44. Ozdemir BC, Pentcheva-Hoang T, Carstens JL, Zheng X, Wu CC, Simpson TR, et al. Depletion of carcinoma-associated fibroblasts and fibrosis induces immunosuppression and accelerates pancreas cancer with reduced survival. Cancer Cell. (2014) 25:719–34. doi: 10.1016/j.ccr.2014.04.005
45. Rhim AD, Oberstein PE, Thomas DH, Mirek ET, Palermo CF, Sastra SA, et al. Stromal elements act to restrain, rather than support, pancreatic ductal adenocarcinoma. Cancer Cell. (2014) 25:735–47. doi: 10.1016/j.ccr.2014.04.021
46. Lee JJ, Perera RM, Wang H, Wu DC, Liu XS, Han S, et al. Stromal response to Hedgehog signaling restrains pancreatic cancer progression. Proc Natl Acad Sci U S A. (2014) 111:E3091–3100. doi: 10.1073/pnas.1411679111
47. Shin K, Lim A, Zhao C, Sahoo D, Pan Y, Spiekerkoetter E, et al. Hedgehog signaling restrains bladder cancer progression by eliciting stromal production of urothelial differentiation factors. Cancer Cell. (2014) 26:521–33. doi: 10.1016/j.ccell.2014.09.001
48. Benson AB 3rd, Wainberg ZA, Hecht JR, Vyushkov D, Dong H, Bendell J, et al. A phase II randomized, double-blind, placebo-controlled study of simtuzumab or placebo in combination with gemcitabine for the first-line treatment of pancreatic adenocarcinoma. Oncologist. (2017) 22:241–e215. doi: 10.1634/theoncologist.2017-0024
49. Narra K, Mullins SR, Lee HO, Strzemkowski-Brun B, Magalong K, Christiansen VJ, et al. Phase II trial of single agent Val-boroPro (Talabostat) inhibiting Fibroblast Activation Protein in patients with metastatic colorectal cancer. Cancer Biol Ther. (2007) 6:1691–9. doi: 10.4161/cbt.6.11.4874
50. Metropulos AE, Munshi HG, and Principe DR. The difficulty in translating the preclinical success of combined TGFbeta and immune checkpoint inhibition to clinical trial. EBioMedicine. (2022) 86:104380. doi: 10.1016/j.ebiom.2022.104380
51. Biswas T, Gu X, Yang J, Ellies LG, and Sun LZ. Attenuation of TGF-beta signaling supports tumor progression of a mesenchymal-like mammary tumor cell line in a syngeneic murine model. Cancer Lett. (2014) 346:129–38. doi: 10.1016/j.canlet.2013.12.018
52. Yang Y, Yang HH, Tang B, Wu AML, Flanders KC, Moshkovich N, et al. The outcome of TGFbeta antagonism in metastatic breast cancer models in vivo reflects a complex balance between tumor-suppressive and proprogression activities of TGFbeta. Clin Cancer Res. (2020) 26:643–56. doi: 10.1158/1078-0432.CCR-19-2370
53. Lu X, Gou Z, Chen H, Li L, Chen F, Bao C, et al. Extracellular matrix cancer-associated fibroblasts promote stromal fibrosis and immune exclusion in triple-negative breast cancer. J Pathol. (2025) 265:385–99. doi: 10.1002/path.v265.3
54. Goehrig D, Nigri J, Samain R, Wu Z, Cappello P, Gabiane G, et al. Stromal protein betaig-h3 reprogrammes tumour microenvironment in pancreatic cancer. Gut. (2019) 68:693–707. doi: 10.1136/gutjnl-2018-317570
55. Lecker LSM, Berlato C, Maniati E, Delaine-Smith R, Pearce OMT, Heath O, et al. TGFBI production by macrophages contributes to an immunosuppressive microenvironment in ovarian cancer. Cancer Res. (2021) 81:5706–19. doi: 10.1158/0008-5472.CAN-21-0536
56. Dominguez CX, Muller S, Keerthivasan S, Koeppen H, Hung J, Gierke S, et al. Single-cell RNA sequencing reveals stromal evolution into LRRC15(+) myofibroblasts as a determinant of patient response to cancer immunotherapy. Cancer Discovery. (2020) 10:232–53. doi: 10.1158/2159-8290.CD-19-0644
57. Ray U, Pathoulas CL, Thirusangu P, Purcell JW, Kannan N, and Shridhar V. Exploiting LRRC15 as a novel therapeutic target in cancer. Cancer Res. (2022) 82:1675–81. doi: 10.1158/0008-5472.CAN-21-3734
58. Storey CM, Cheng M, Altai M, Park JE, Tran J, Lueong SS, et al. Key regulatory elements of the TGFbeta-LRRC15 axis predict disease progression and immunotherapy resistance across cancer types. bioRxiv. (2024). doi: 10.1101/2024.11.22.624939
59. Yang Z, Tian H, Chen X, Li B, Bai G, Cai Q, et al. Single-cell sequencing reveals immune features of treatment response to neoadjuvant immunochemotherapy in esophageal squamous cell carcinoma. Nat Commun. (2024) 15:9097. doi: 10.1038/s41467-024-52977-0
60. Gao Y, Li J, Cheng W, Diao T, Liu H, Bo Y, et al. Cross-tissue human fibroblast atlas reveals myofibroblast subtypes with distinct roles in immune modulation. Cancer Cell. (2024) 42:1764–83.e1710. doi: 10.1016/j.ccell.2024.08.020
61. Krishnamurty AT, Shyer JA, Thai M, Gandham V, Buechler MB, Yang YA, et al. LRRC15(+) myofibroblasts dictate the stromal setpoint to suppress tumour immunity. Nature. (2022) 611:148–54. doi: 10.1038/s41586-022-05272-1
62. Zinn S, Vazquez-Lombardi R, Zimmermann C, Sapra P, Jermutus L, and Christ D. Advances in antibody-based therapy in oncology. Nat Cancer. (2023) 4:165–80. doi: 10.1038/s43018-023-00516-z
63. Ray U, Jung DB, Jin L, Xiao Y, Dasari S, Sarkar Bhattacharya S, et al. Targeting LRRC15 inhibits metastatic dissemination of ovarian cancer. Cancer Res. (2022) 82:1038–54. doi: 10.1158/0008-5472.CAN-21-0622
64. Jungwirth U, van Weverwijk A, Evans RJ, Jenkins L, Vicente D, Alexander J, et al. Impairment of a distinct cancer-associated fibroblast population limits tumour growth and metastasis. Nat Commun. (2021) 12:3516. doi: 10.1038/s41467-021-23583-1
65. Evans RJ, Perkins DW, Selfe J, Kelsey A, Birch GP, Shipley JM, et al. Endo180 (MRC2) antibody-drug conjugate for the treatment of sarcoma. Mol Cancer Ther. (2023) 22:240–53. doi: 10.1158/1535-7163.MCT-22-0312
66. Ford K, Hanley CJ, Mellone M, Szyndralewiez C, Heitz F, Wiesel P, et al. NOX4 inhibition potentiates immunotherapy by overcoming cancer-associated fibroblast-mediated CD8 T-cell exclusion from tumors. Cancer Res. (2020) 80:1846–60. doi: 10.1158/0008-5472.CAN-19-3158
67. Hecker L, Vittal R, Jones T, Jagirdar R, Luckhardt TR, Horowitz JC, et al. NADPH oxidase-4 mediates myofibroblast activation and fibrogenic responses to lung injury. Nat Med. (2009) 15:1077–81. doi: 10.1038/nm.2005
68. Hotta Y, Uchiyama K, Takagi T, Kashiwagi S, Nakano T, Mukai R, et al. Transforming growth factor beta1-induced collagen production in myofibroblasts is mediated by reactive oxygen species derived from NADPH oxidase 4. Biochem Biophys Res Commun. (2018) 506:557–62. doi: 10.1016/j.bbrc.2018.10.116
69. Hecker L, Logsdon NJ, Kurundkar D, Kurundkar A, Bernard K, Hock T, et al. Reversal of persistent fibrosis in aging by targeting Nox4-Nrf2 redox imbalance. Sci Transl Med. (2014) 6:231ra247. doi: 10.1126/scitranslmed.3008182
70. Hanley CJ, Mellone M, Ford K, Thirdborough SM, Mellows T, Frampton SJ, et al. Targeting the myofibroblastic cancer-associated fibroblast phenotype through inhibition of NOX4. J Natl Cancer Inst. (2018) 110:109–20. doi: 10.1093/jnci/djx121
71. Chen Y, Kim J, Yang S, Wang H, Wu CJ, Sugimoto H, et al. Type I collagen deletion in alphaSMA(+) myofibroblasts augments immune suppression and accelerates progression of pancreatic cancer. Cancer Cell. (2021) 39:548–65.e546. doi: 10.1016/j.ccell.2021.02.007
72. Bhattacharjee S, Hamberger F, Ravichandra A, Miller M, Nair A, Affo S, et al. Tumor restriction by type I collagen opposes tumor-promoting effects of cancer-associated fibroblasts. J Clin Invest. (2021) 131:e146987. doi: 10.1172/JCI146987
Keywords: cancer associated fibroblasts (CAFs), immune checkpoint blockers (ICBs), transforming growth factor beta (TGFβ), immunotherapy resistance, T cell exclusion, myofibroblast
Citation: Hou W (2025) Role of TGFβ-activated cancer-associated fibroblasts in the resistance to checkpoint blockade immunotherapy. Front. Oncol. 15:1602452. doi: 10.3389/fonc.2025.1602452
Received: 29 March 2025; Accepted: 02 May 2025;
Published: 21 May 2025.
Edited by:
Elio Gregory Pizzutilo, Grande Ospedale Metropolitano Niguarrda, ItalyReviewed by:
Giulia Grisendi, University of Modena and Reggio Emilia, ItalyCopyright © 2025 Hou. This is an open-access article distributed under the terms of the Creative Commons Attribution License (CC BY). The use, distribution or reproduction in other forums is permitted, provided the original author(s) and the copyright owner(s) are credited and that the original publication in this journal is cited, in accordance with accepted academic practice. No use, distribution or reproduction is permitted which does not comply with these terms.
*Correspondence: Weibin Hou, aG91d2JAeW1haWwuY29t