- 1Biosciences and Biotechnology Division, Lawrence Livermore National Laboratory, Livermore, CA, United States
- 2Department of Plant and Microbial Biology, Rausser College of Natural Resources, University of California, Berkeley, Berkeley, CA, United States
- 3Department of Pathology and Laboratory Medicine, University of California, Irvine, Irvine, CA, United States
A worldwide estimate of over one million STIs are acquired daily and there is a desperate need for effective preventive as well as therapeutic measures to curtail this global health burden. Vaccines have been the most effective means for the control and potential eradication of infectious diseases; however, the development of vaccines against STIs has been a daunting task requiring extensive research for the development of safe and efficacious formulations. Nanoparticle-based vaccines represent a promising platform as they offer benefits such as targeted antigen presentation and delivery, co-localized antigen-adjuvant combinations for enhanced immunogenicity, and can be designed to be biologically inert. Here we discuss promising types of nanoparticles along with outcomes from nanoparticle-based vaccine preclinical studies against non-viral STIs including chlamydia, syphilis, gonorrhea, and recommendations for future nanoparticle-based vaccines against STIs.
1 Introduction
Sexually transmitted infections (STIs) are among the most common public health burdens worldwide (Thomas et al., 2019). They are the leading cause of severe reproductive health complications, congenital infections, and increased risk for acquiring other STIs such as human immunodeficiency virus (HIV) (Sexton et al., 2005; Barrow et al., 2020). STIs are caused by a range of bacteria, viruses, and parasites and are transmitted primarily via sexual contact (Wagenlehner et al., 2016; Dagnew et al., 2020). Many STI infections may present as asymptomatic diseases, which compounds the difficulty in overcoming associated disease burden (Wi et al., 2019). According to the World Health Organization (WHO), eight of these pathogens are responsible for the majority of STIs. Four of the eight infections are curable STIs, namely chlamydia, syphilis, gonorrhea, and trichomoniasis. The other four infections are incurable viral infections, including HIV, hepatitis B, human papillomavirus (HPV), and herpes simplex virus (HSV) (World Health Organization, 2018). Globally, over 1 million STIs are acquired daily (World Health Organization, 2018), and the high incidence of infected persons presenting with complications and other sequelae calls for extensive research on the development of new preventive measures.
Vaccines have been the most effective means for the control as well as potential eradication of infectious diseases (Reljic and González-Fernández, 2019). Over the years, global efforts focused on lowering vaccine-preventable diseases has led to tremendous advancements in vaccine development against infections including STIs. Traditional vaccines such as live-attenuated whole-pathogen, inactivated whole-pathogen, subunit, and nucleic acid vaccines have been studied for their potential to safely induce protective immune responses. While not every infectious agent can be effectively targeted with whole-pathogen or subunit vaccines, those that can be targeted have been met with undesired side effects. These effects include reversion to virulence of live-attenuated whole pathogens, development of more severe infection outcomes following vaccination with inactivated whole pathogens (Rose et al., 2018), as well as the need for routine booster shots to attain robust protection against diseases (Vartak and Sucheck, 2016). Vaccine development against STIs has faced additional challenges, ranging from socio-cultural vaccine acceptance to the selection of appropriate antigens, availability of adjuvants, and delivery systems suitable for humans (Zimet et al., 2000; Gottlieb and Johnston, 2017; Reljic and González-Fernández, 2019).
Subunit vaccines, in which only components of infectious agents are used instead of the entire pathogen, have shown significant promise for the development of safer vaccines against STIs. However, studies have shown that administering the subunit antigens alone is not sufficient to produce adequate long-term immunity needed to prevent disease. Major obstacles to vaccine development include difficulty in the identification and production of appropriate antigens for biological specificity (Flower et al., 2010; Vartak and Sucheck, 2016), identification of suitable adjuvants that will induce a robust protective immune response when formulated with antigens of interest, and the optimization of antigen-adjuvant interactions for maximum vaccine immunogenicity and stability (Fox et al., 2013). To address the need for suitable adjuvants, as well as effective and safe delivery platforms for promising antigens, researchers have adopted the use of nanoparticles as they provide facile platforms that can be designed to specifically improve immunogenicity with high efficacy (Pati et al., 2018).
In this article, we review the implications of nanoparticle-based vaccines and the outcomes of current delivery systems used in nanoparticle-based vaccines against non-viral pathogens, including the bacterial pathogens Chlamydia trachomatis (chlamydia), Treponema pallidum (syphilis), and Neisseria gonorrhoeae (gonorrhea). Given the success of nanocarriers used in the current COVID-19 vaccines and the early data from the CTH522 Chlamydia vaccine clinical trials (NCT02787109 and NCT03926728), we envisage that nanocarriers will continue to emerge as important components of pre-clinical vaccine studies especially for bacterial pathogens to dramatically change the current status of vaccines against STIs. In many ways, the preclinical vaccine studies discussed in this review are aspirational, however, we believe the timing is right to explore these concepts in the context of vaccines for bacterial STIs. We conclude with recommendations for future improvements in the efficacy and application of nanoparticle-based vaccines against STIs.
2 Implications of Nanotechnology in Vaccine Development for Sexually Transmitted Infections
For the past several decades, there has been increasing application of nanotechnology in fields including biology and the biomedical sciences, materials science, chemistry, and physics (Wang and Wang, 2014; Bayda et al., 2019). Nanoparticles are small particles that typically range between 1 and 100 nm in size and display disparate physical and chemical properties when compared to their larger bulk material equivalents (Jeevanandam et al., 2018), as their high surface area to volume ratio increases reactivity at the molecular level (Mourdikoudis et al., 2018). This intrinsic feature of nanoparticles allows them to exhibit distinct physical, chemical, and optical properties that enhance their versatility (Mourdikoudis et al., 2018) to be engineered for enhanced immune modulation and tailored antigen delivery.
Nanoparticle-based vaccines take advantage of a combination of nanoparticle size and composition. Their small size provides high surface area relative to bulk materials and mimics the size of particulates against which our immune systems are primed (e.g., viruses) (Pati et al., 2018). The disparate compositions of nanoparticles provide a diverse toolkit for vaccine formulation and enables fine-tuning of key physicochemical properties such as charge, functionalization, and stability (Chattopadhyay et al., 2017). These characteristics (Table 1), in turn, can be used to tune the biological effects of nanoparticle-based vaccines, including delivery, cellular uptake, biodistribution, stability, antigenicity, and efficacy (Al-Halifa et al., 2019). Nanoparticle-based vaccines are engineered from a variety of biodegradable materials including lipids, proteins, natural and synthetic polymers, as well as inorganic materials that exhibit important antigenic moieties (Pati et al., 2018) (Figure 1). Nanotechnology in vaccine development for STIs also accelerates the possibility for targeted antigen delivery, and regulated discharge of antigens to antigen presenting cells (APC)s (Peek et al., 2008). The conjugation or encapsulation of antigens to nanoparticles provides protection from proteolytic degradation while improving and prolonging antigen delivery to immune cells, a requirement that will facilitate the robust protection needed against STIs (Gao et al., 2018; Pati et al., 2018; Sahu et al., 2018). Various combinations of antigens and adjuvants can be prepared using nanoparticle platforms to generate both humoral immune responses and cellular immunity against infections (Mohan et al., 2013; Dykman, 2020), as well as generate longer lasting immunity, which is necessary for simplifying vaccination schedules (Lofano et al., 2020).
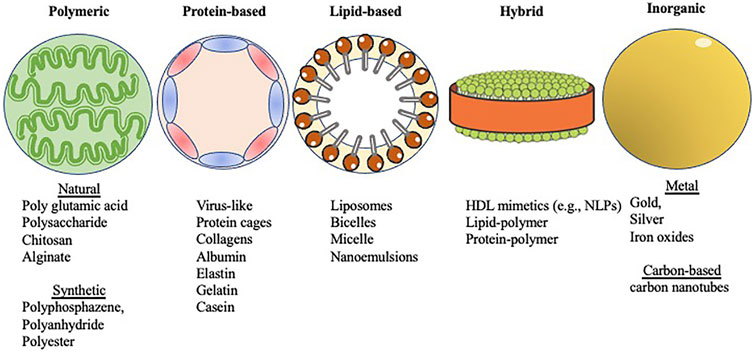
FIGURE 1. Shown in abstract form are five different types of nanoparticles covered within this review. The categories were selected based on the major constituents that form the different nanoparticles (see Section 3 text). Images were adapted from Servier Medical Art by Servier, which is licensed under a Creative Commons Attribution 3.0 Unported License. Different forms of nanoparticle scaffolds for vaccine development.
Modern vaccine design has benefited from the use of nanoparticles, as seen in recent times with the nanoparticle-based vaccines targeting SARS-CoV-2 (NCT04368728) (Shin et al., 2020; Walls et al., 2020). In their preclinical study, the authors described a very effective nanoparticle-based vaccine engineered with a self-assembling protein nanoparticle displaying 60 SARS-CoV-2 spike receptor-binding domains in a highly immunogenic array and was shown to induce a 10-fold higher neutralizing antibody titer even at a 5-fold lower dose than the prefusion-stabilized spike ectodomain S-2P trimer (Walls et al., 2020). The overall benefits of nanoparticle-based vaccines in general vaccine applications cannot be overemphasized (Peek et al., 2008; Nasir, 2009). Hence, the use of nanoparticle-based vaccines as a substitute to the traditional vaccines offer a very interesting and promising approach for vaccine delivery against STIs.
3 Nanoparticle-Based Vaccine Delivery Platforms
The different types of biodegradable materials from which nanoparticles are engineered offer a wide variety of delivery platforms for vaccines, such as protein-based nanoparticles, natural and synthetic polymeric nanoparticles, lipid-based nanoparticles, and hybrid nanoparticles (Al-Halifa et al., 2019). These biodegradable nanomaterials are usually preferred over inorganic nanoparticles due to the latter’s propensity for particle aggregation (Zhu et al., 2014; Al-Halifa et al., 2019) (Table 2).
3.1 Polymeric Nanoparticles
Many polymers are engineered as nanoparticle-based vaccine delivery platforms because of their biodegradability, biocompatibility, durability, and low toxicity. Polymers are extremely modifiable, hence their physiochemical properties can be readily modified to enhance their immunomodulatory effects (Bose et al., 2019). Polymeric nanoparticles enhance prolonged antigen delivery due to their increased stability in vivo (Thorp et al., 2020). This category can be further divided into two groups: natural and synthetic polymeric nanoparticles. Natural polymeric nanoparticles are further divided into poly glutamic acid-based nanoparticles and polysaccharide-based nanoparticles including chitosan, starch, alginate, and cellulose (Han et al., 2018). Chitosan-based nanoparticle vaccines, alginate-based nanoparticle vaccines, and their derivatives are the most studied polymeric materials as delivery platforms for vaccines against STIs, as well as other infectious diseases (Han et al., 2018; Bose et al., 2019). Many of these preclinical studies have shown the immunomodulatory effects of such nanoparticle-based vaccines against STIs. For example, several chitosan-based nanoparticle vaccines and therapies known for their intrinsic bio-adhesive properties have been shown to prolong interaction and contact duration with immune cells, which enhances their immunomodulatory effects (Prego et al., 2010; Zhao et al., 2013; Pradines et al., 2015; Rose et al., 2018). Synthetic polymeric nanoparticles include polyphosphazene, poly (glycolic acid) (PGA), poly (lactic acid) (PLA) and poly (DL-lactide-co-glycolic) acid (PLGA), poly (allymines)s, cell-penetrating poly (disulfide)s, and copolymer-based nanoformulations (Bose et al., 2019). Of all the synthetic polymeric nanoparticles, PLGA, a copolymer synthesized by the random polymerization of PLA and PGA, has been the extensively researched for vaccine delivery applications (Aikins et al., 2017).
3.2 Protein-Based Nanoparticles
Protein‐based nanoparticles are highly biodegradable and possess other properties including biocompatibility, biofunctionality, the ability to interact with other molecules for recognition, and their adaptive potential for genetic engineering for specific cell targeting and targeted protein delivery. They are known to exit the vasculature, enter the lymphatic system and permeate tissues through passive diffusion, making them highly beneficial as antigen delivery platforms. Protein-based nanoparticles are made by self‐assembly and desolvation. Examples of proteins that can be used for the fabrication of nanoparticles include collagen, albumin, elastin, gelatin, casein, whey proteins, silk proteins, soy proteins, and lectins (Young Kim et al., 2015; Qin et al., 2019). Some structurally complex and defined protein‐based nanoparticles include virus-like nanoparticles (VLPs), protein cages, and protein-based complexes (Diaz et al., 2018; Nguyen and Tolia, 2021). VLPs are derived from plant, microbial, insect, and mammalian viruses, without the viral genome itself, and are easily internalized by cells (Zeltins, 2013). VLPs are formed from the autonomous oligomerization or self-assembly of monomeric protein building blocks. These protein building blocks assemble into larger, stable and highly ordered structures that can be engineered to have a diameter ranging from 20 to 100 nm and possess unique physical properties (López-Sagaseta et al., 2016). Protein cages on the other hand include viral capsids, ferritins, and heat shock proteins, while protein-based complexes include Cry3Aa fusion protein platform (well known for the delivery of reporter proteins such as GFP in mammalian cells), silk proteins, and bovine serum albumin (Nair et al., 2015; Qin et al., 2019).
In cancer vaccine studies, peptide and protein-based vaccines typically fail to induce efficient immune responses against tumors. However, delivery of the peptides and proteins encapsulated in protein nanoparticles has shown promising improvements in vaccine efficacy characterized by increased immunogenicity of the tumor microenvironment. The highly organized structures and symmetry of protein nanoparticles as well as their ability to have specific functions inside or outside or in between subunits of the protein cage allows for the co-delivery of adjuvants and antigens that promotes antigen-specific immune responses against tumors (Neek et al., 2019). A ferritin nanoparticle vaccine was investigated for its ability to present N. gonorrhoeae peptide antigens on its surface. The N. gonorrhoeae peptides were inserted at the N terminus or in a surface-exposed ferritin loop between helices αA and αB. While crystal structures of the chimeric proteins from this study showed that the proteins were assembled correctly into a 24-mer nanocage structure, the inserted N. gonorrhoeae peptides were disordered on the nanocage surface showing multiple conformations (Wang et al., 2017). Protein nanoparticles have been utilized in many preclinical vaccine studies for hepatitis B, HPV and HIV with promising outcomes including the induction of antigen specific antibodies and viral clearance in animal models (Kirnbauer et al., 1992; Oh et al., 2004; Slupetzky et al., 2007; Paz De la Rosa et al., 2009; Tyler et al., 2014; Sliepen et al., 2015; Motevalli et al., 2018; Tokatlian et al., 2019; Wang et al., 2020; Ximba et al., 2020). These studies confirm the need for more extensive research to harness the potential of protein nanoparticles for vaccines against bacterial STIs that will allow for effective delivery of antigenic peptides and adjuvants.
3.3 Lipid-Based Nanoparticles
Most lipid‐based nanoparticles are derived from dietary oils or fats and are sometimes referred to as solid lipid nanoparticles based on their inclusion of lipids that exist as a solid phase at physiological temperatures. They include nanoemulsions, micelles, bicelles (bilayered discoidal micelles) and liposomes made from natural, semi‐synthetic or synthetic lipids, such as fatty acids, fatty alcohols, phospholipids, and medium and long chain mono-, di- and triglycerides. Lipid‐based nanoparticles are regarded as promising delivery platforms due to their ability to bypass multiple biological barriers, low toxicity, biocompatibility, and biodegradability (Niu et al., 2016; Qin et al., 2019).
Lipid-based nanoparticles have been used for the delivery of antigens including nucleic acids and proteins of infectious agents as well as cancer therapeutic agents to induce humoral immune responses (Aldosari et al., 2021; Lee et al., 2021; Park et al., 2021). These lipid-based nanocarriers pass through the cell membrane for cellular uptake by macropinocytosis, a type of endocytosis where they must escape from the endosomal lumen to deliver their enclosed antigens into the cytosol (Guevara et al., 2020; Lee et al., 2021). While ionizable lipid pKa was thought to be the main factor involved in driving lipid nanoparticle potency and tolerability due to its effects on opsonization of the particles, cellular uptake and endosomal escape efficiency, it has been shown that other factors play a role in the immunogenicity of lipid nanoparticles (Hassett et al., 2019). Hence, a better understanding of mechanisms that regulate the biodistribution of antigen-loaded lipid nanoparticles will guide research outcomes for optimizing lipid nanoparticles as delivery systems.
3.4 Hybrid Nanoparticles
Hybrid nanoparticles incorporate key constituents from at least two different nanoparticle classes, leveraging the beneficial attributes of each to enhance their functionality. Hybrid nanoparticles include lipid-polymer hybrid nanoparticles, protein-dendrimer hybrid nanoparticles and high-density lipoprotein (HDL) mimetics (Qin et al., 2019). Lipid–polymer hybrid nanoparticles are core–shell nanoparticle structures made of polymer cores and lipid/lipid–PEG shells that show complementary properties in their biocompatibility and physical stability from both polymeric nanoparticles and liposomes (Hadinoto et al., 2013). Lipid–polymer hybrid nanoparticles exhibit higher in vivo cellular delivery when compared to either polymeric nanoparticles or liposomes. The formulation of lipid–polymer hybrid nanoparticles with chitosan has been shown to significantly increase mucosal immune responses against some STIs (Hadinoto et al., 2013; Rose et al., 2018).
In recent years newly engineered nanoparticles known as nanolipoproteins (NLPs), which are HDL mimetics consisting of discoidal lipid bilayer stabilized by apolipoproteins have been in development with desirable attributes for vaccine delivery. NLPs also known as nanodiscs are effective antigen carriers and supporting adjuvants that improve vaccine performance against infectious diseases. We have previously demonstrated that the use of NLPs enhances immune responses to both antigen and adjuvants in vivo (Fischer et al., 2013; Weilhammer et al., 2013; Weilhammer et al., 2017; Weilhammer et al., 2020). We have demonstrated that NLPs provide a membrane-like environment, which facilitate the embedding of functional membrane-bound proteins in their native conformations (He et al., 2017; Tifrea et al., 2021). The versatility of this type of nanoparticle underscores its potential for vaccines targeting bacterial and viral STIs.
3.5 Inorganic Nanoparticles
Inorganic nanoparticles include silica nanoparticles, metal nanoparticles (gold, silver, magnetic, metal organic framework-based nanoparticles), carbon-based nanomaterials (carbon nanotubes, nano-diamonds), and superparamagnetic nanoparticles (iron oxide nanoparticles). These nanoparticles are usually coated with organic molecules to make them biologically suitable as well as prevent particle aggregation. Commonly used inorganic nanoparticles for vaccine formulations include silver and gold nanoparticles. Gold nanoparticles are easily internalized by APCs and can facilitate their activation and elicit downstream immune responses, making them suitable platforms for antigen delivery (Bastús et al., 2009; Wang et al., 2018; Al-Halifa et al., 2019). A wide range of antigens and adjuvants can be conjugated on gold nanoparticles at high densities to prolong antigen delivery, improve immunogenicity as well as antigen presentation (Marques Neto et al., 2017). Although gold nanoparticles are a great platform for antigen conjugation, their ability to accumulate in organs such as the liver for long periods could ultimately result in toxicity (Arvizo et al., 2010). Coating with biocompatible materials has been shown to reduce their toxicity, although there is risk of alterations to their physicochemical and biological properties (Zhu et al., 2014).
4 Nanoparticle-Based Vaccines Against Sexually Transmitted Infections
Global estimates indicate that over one million STIs are acquired daily, suggesting a desperate need for the development of safe and efficacious vaccine formulations (Gottlieb and Johnston, 2017). We will highlight some of the preclinical studies dedicated towards nanoparticle-based vaccine research against chlamydia, syphilis, and gonorrhea in this section of the review and in Table 2.
4.1 Chlamydia
C. trachomatis is significant to human health worldwide (Paavonen and Eggert-Kruse, 1999; Dean, 2009; World Health Organization, 2018) as it remains the most diagnosed STI in developed countries despite the availability of effective and affordable therapy. Chlamydia exists either as an extracellular elementary body (EB), which is the non-replicating, infectious form or as a reticulate body (RB), the noninfectious form that replicates intracellularly (Nans et al., 2015; Elwell et al., 2016; Fischer and Rudel, 2018). The infection is mostly asymptomatic but can induce inflammatory responses at the site of infection, causing immunopathological sequelae such as pelvic inflammatory disease (PID), ectopic pregnancy, tubal infertility, miscarriage, and trachoma (an ocular disease) (Stamm, 1999; Vasilevsky et al., 2016; Lijek et al., 2018; Murthy et al., 2018). This has led to over 70 years of research dedicated to the development of vaccines that will provide adequate protection against C. trachomatis infection (Phillips et al., 2019). Chlamydial vaccines have utilized numerous approaches to improve vaccine immunogenicity, including the use of plasmid DNA, recombinant proteins, and subunit antigenic determinants, with or without adjuvants, yet the need for effective preventive measures remains unmet (Brunham and Rey-Ladino, 2005; Vasilevsky et al., 2014). Studies have indicated a critical role for chlamydia-specific CD4+ Th1 T cell responses in the clearing of infection and provision of protective immunity against chlamydia reinfection (Su and Caldwell, 1995; Gondek et al., 2012; Bakshi et al., 2018; Labuda and McSorley, 2018). The chlamydial major outer membrane protein (MOMP) has been shown to induce an immune response comparable to that seen with live bacteria in mice (Pal et al., 2005), while polymorphic membrane proteins (PMPs), have been explored for their ability to induce cross-species immunogenicity (Pal et al., 2017). MOMP and PMPs have been investigated as vaccine antigens in mice and nonhuman primates. Both antigens have shown suboptimal protection in many studies (Kari et al., 2009; Vasilevsky et al., 2016), hence the search for effective antigen-adjuvant combinations that can induce broad and long-lived protective immune responses continues (de la Maza et al., 2017).
The use of nanotechnology in the development of C. trachomatis vaccines is a promising strategy to enhance the production of long-lived immune protection. Preclinical studies exploring nanoparticle-based vaccines have shown effective delivery of immunogens and the induction of chlamydia-specific immune responses that will be beneficial in translational medicine. Chitosan nanoparticles possess the required size necessary to facilitate uptake by APCs for the induction of immune responses. They are shown to have high encapsulation capacity for stability and antigen protection from enzymatic digestion, making them favorable delivery platforms for chlamydial nucleic acids such as MOMP DNA constructs (Cambridge et al., 2013). Recombinant C. trachomatis fusion antigen CTH522 adjuvanted with glycol-chitosan-coated lipid-polymeric hybrid nanoparticle was shown to induce antigen-specific mucosal immune responses in the lungs and genital tract of mice, characterized by CTH522-specific IgG/IgA antibodies and IFN-γ-producing Th1 cells after nasal immunization (Rose et al., 2018).
PLGA nanoparticles have been extensively explored as vaccine delivery platforms due to their self-adjuvanting properties whereby they possess both antigenic and an adjuvanting moieties, their ability to prolong the release of antigens to stimulate dendritic cells as well as enhance intracellular antigen delivery to activated T-cells via the CD40 co-stimulation mechanism (Makadia and Siegel, 2011; Sneh-Edri et al., 2011; Rosalia et al., 2015). The lactide/glycolide ratio of PLGA nanoparticles is critical for efficient encapsulation and prolonged delivery of both antigens or drugs (Sahu et al., 2020), and PLGA nanoparticles at an 85:15 ratio used for the encapsulation of recombinant MOMP (PLGA-rMOMP) enhanced the activation of dendritic cells, induced chlamydia-specific rMOMP CD4+ memory and effector T-cells and activated an antibody adaptive immune response in immunized mice. Mice immunized intranasally with PLGA-rMOMP generated enhanced numbers of CD4+ T-cells but not memory and effector T-cells, whereas subcutaneously immunized mice produced both CD4+ memory and effector T-cells (Sahu et al., 2020), demonstrating the importance of vaccination route to the resulting immune response. The extended release of rMOMP in this PLGA-rMOMP formulation provided protective immunity against C. muridarum genital challenge and rechallenge (Sahu et al., 2021). PLGA (85:15) nanoparticle encapsulating rMOMP-187 (a peptide derivative of MOMP) induced a significant production of interleukin (IL)-6, IL-12p40, Th1/Th17 cytokines and nitric oxide (NO) by mouse J774 macrophages in a dose dependent manner and with minimal toxicity (Taha et al., 2012). In another preclinical vaccine study, spleen cells from BALB/c mice immunized with PLGA-rMOMP exhibited higher numbers of CD4+ and CD8+ T-cells and an increased secretion of IFN-γ (Th1) and IL-12p40 versus IL-4 and IL-10 (Th2) cytokines in response to restimulation with purified rMOMP, as well as immunized mice producing higher serum IgG and IgG2a (Th1) than IgG1 (Th2) rMOMP-specific antibodies (Fairley et al., 2013). The ability of PLGA-rMOMP to induce Th1 immune responses in mice makes it an extremely suitable nanoparticle-based vaccine candidate against C. trachomatis to be explored for translational studies.
The encapsulation of chlamydial antigens in Poly (lactic acid)-poly (ethylene glycol) or PLA-PEG, another self-adjuvating, biodegradable co-polymeric nanoparticle, has been shown to induce potent anti-C. trachomatis immune responses. M278, a recombinant peptide derived from C. trachomatis MOMP encapsulated in PLA-PEG, showed an enhancement of long-lasting chlamydia-specific CD4+ T-cell effector responses facilitated by caveolin-mediated endosomal antigen processing and MHC class II-dependent antigen presentation (Dixit et al., 2018). More specifically, there was an enhanced expression of pathogen-sensing receptors, such as TLR2 and Nod1, surface activation markers, including, Cd1d2 and Fcgr1, effector cytokines and chemokines, MHC class I and II molecules, as well as co-stimulatory molecules including CD40, CD80 and CD86 (Dixit et al., 2018). Subcutaneous immunization of M278 encapsulated in PLA-PEG was shown to induce secretion of IFN-γ, resulting in the differentiation of chlamydia-specific CD4+ T-cells to memory and effector phenotypes. Significant protection of immunized mice characterized by reduced vaginal bacterial loads after a genital tract challenge was observed and associated to elevated mucosal IgG1 and MOMP-specific IgA in the mice. Additionally, immune sera with functional neutralizing antibodies collected from mice immunized with PLA-PEG-encapsulated M278 prevented the infection of McCoy cells by C. muridarum (Verma et al., 2018). In another study, mice immunized with PLA-PEG encapsulated M278 generated higher T-cell cytokines [Th1 (IFN-γ, IL-2), Th17 (IL-17)] and antibodies [Th1 (IgG2a), Th2 (IgG1, IgG2b)] when compared to mice immunized with unencapsulated M278 (Dixit et al., 2014).
We recently encapsulated MOMP from C. muridarum (mMOMP) in the membrane-like environment of NLPs and demonstrated that vaccination with MOMP-NLPs provided a balanced T cell response with partial protection in a respiratory challenge model (He et al., 2017; Tifrea et al., 2021). We used an Escherichia coli-based cell-free system to express a MOMP protein from C. muridarum (mMOMP) and supported it within a telodendrimer nanolipoprotein particle (mMOMP–tNLP) co-localized with CpG oligodeoxynucleotide 1826 (CpG), a single-stranded synthetic DNA adjuvant, functionalized with a cholesterol tag to enable conjugation to the lipid nanoparticle. This mMOMP-tNLP complex induced an enhanced antigen-specific IgG response in vaccinated mice (He et al., 2017; Tifrea et al., 2021). We first reported a C. muridarum-exosomes vaccine formulated with CpG plus Montanide as adjuvants that elicited robust Th1 humoral and cell-mediated immune responses against a respiratory challenge with C. muridarum EB in vaccinated mice as well as producing high levels of C. muridarum-specific neutralizing antibodies in their serum (Pal et al., 2020).
In another study, a peptide derived from Pmp-G, a chlamydial polymorphic membrane protein, which functions as an autotransporter adhesin and plays an essential role in the initial phase of chlamydial infection (Vasilevsky et al., 2016) was encapsulated in vault nanoparticles, made from a natural nanocapsule engineered from hollow barrel-shaped eukaryotic ribonucleoprotein complexes. This vaccine formulation was shown to significantly reduce the genital bacterial burden and histopathologic inflammation in immunized mice following a C. muridarum challenge. Protection correlated with the induction of a systemic antigen-specific cellular immune response, characterized by an increase in PmpG-specific splenic CD4+ central memory T-cells and IFN-γ+ natural killer (NK) cells, a decrease in the number of inflammatory cells, such as neutrophils and TNF-α+ CD8+ T-cells in the genital tract, and the secretion of IFN-γ, TNF-α, IL-17, and IL-2 by splenic CD4+ T-cells (Jiang et al., 2017). Lastly, the self-assembly of lipid phytantriol and the immunopotentiator monomycoloyl glycerol-1 into nanocarriers with an internal hexagonal phase encapsulating chlamydial MOMP induced stronger MOMP-specific IgG humoral responses and antigen-specific CD4+ T-cell responses than MOMP surface-adsorbed to CAF04 liposome [a cationic liposomal adjuvant formulation containing monomycolyl glycerol [MMG] and N,N-dimethyl-N,N-dioctadecylammonium (DDA)] or unadjuvanted MOMP in immunized mice. However, CAF04 liposomes elicited more robust effector T-cell responses than the MOMP encapsulated nanocarrier, suggesting that the nanostructural composition of the lipid-based delivery system exerts a significant impact on both the type and magnitude of the induced immune responses (Rodrigues et al., 2018). Reports from the phase I clinical trial of the first in-human vaccine against C. trachomatis infections has shown that the use of CTH522 adjuvanted with either CAF01, a cationic liposomal adjuvant or aluminum hydroxide was safe and well tolerated (Abraham et al., 2019) (NCT02787109). While further investigation is needed, positive results from this first clinical trial showed that CTH522 formulated with CAF01 induced better humoral immunogenicity than CTH522 formulated with aluminum hydroxide (Abraham et al., 2019). These results led to another phase I clinical trial for trachoma (NCT03926728) and indicates a bright future for nanoparticle-based vaccines against C. trachomatis.
4.2 Syphilis
As with other human STIs, there is an urgent need for the development of efficacious vaccines against the spirochete Treponema pallidum, etiological agent for the multistage STI syphilis. Syphilis is the cause of STI-related deaths, second to only acquired immune deficiency syndrome (AIDS) (Zhao et al., 2011; Zhao et al., 2013). Syphilis exhibits alternating asymptomatic and symptomatic disease stages with different clinical manifestations. It is associated with systemic disease ranging from multiple organ impairment in adults to adverse pregnancy outcomes including abortions, premature births, stillbirths, and congenital diseases (Walker et al., 2019). Although penicillin has been an effective treatment for syphilis, its effectiveness depends on such limitations as accurate diagnosis, patient compliance, drug availability, and disease stage (Cameron and Lukehart, 2014). Hence, the need for efficacious vaccine formulations that will prevent infection and provide robust protection against all T. pallidum strains remains.
Despite the critical need for effective vaccines against T. pallidum, only a limited number of preclinical vaccine studies were conducted for decades, partly due to the fragility of the T. pallidum outer membrane proteins (OMPs) and limited research on T. pallidum biology. Current vaccine studies are investigating the role of OMPs in the induction of opsonization and phagocytosis, as well as their role as potentiators of T. pallidum virulence. Some of these studies utilized conventional vaccine strategies such as whole cell attenuated, aged, and γ-irradiated T. pallidum, while other studies have utilized T. pallidum specific antigens for immunization. However, only rabbits immunized with γ-irradiated T. pallidum, Nichols strain, over a 37-week period were shown to be fully protected against challenge with homologous treponemes but with no protection against challenge with the T. pallidum subspecies pallidum strain (Lithgow and Cameron, 2017). Significantly reduced bacterial organ burden in immunized rabbits after a T. pallidum challenge was seen in a recent study that utilized Tp0751 (a vascular adhesin of T. pallidum subspecies pallidum) as a vaccine candidate against the T. pallidum subspecies pallidum strain. The transfer of popliteal lymph nodes from Tp0751-immunized, T. pallidum-challenged rabbits to naive rabbits also conferred sterile protection against a T. pallidum-challenge (Lithgow et al., 2017). While these studies were conducted in the absence of nanoparticle formulations, these promising findings suggest that nanoparticles vaccine carriers combining these protective T. pallidum antigens with adjuvants could provide efficacious vaccine formulations with robust protection against all T. pallidum strains.
To date, the only nanoparticle-based vaccine studies against T. pallidum have utilized plasmids expressing IL-2 and Tp92, a T. pallidum OMP, encapsulated in chitosan nanoparticles individually or in combination. Both the IL-2 and Tp92 plasmid-encapsulated chitosan nanoparticle-based vaccines increased anti-Tp92 antibody levels while the combination of both plasmids encapsulated in chitosan nanoparticles showed the greatest amplification of anti-Tp92 antibodies and T-cell proliferation, which resulted in significant protection against challenge with T. pallidum in immunized male New Zealand white rabbits (Zhao et al., 2011). Similar effects were seen with T. pallidum Gpd DNA vaccine adjuvanted with IL-2 and chitosan nanoparticles (Zhao et al., 2013). These findings indicate promising vaccine outcomes from DNA complexed with nanoparticles and the need for more research on developing T. pallidum vaccines.
4.3 Gonorrhea
Neisseria gonorrhoeae is the bacterial agent responsible for the STI gonorrhea that poses a critical public health problem worldwide due to its resistance to multiple antimicrobials (Rowley et al., 2019). Infection with N. gonorrhoeae is often asymptomatic but can lead to serious complications in both men and women including urethritis, cervicitis, proctitis, pelvic inflammatory diseases, chronic pelvic pain, infertility, ectopic pregnancy, and increased susceptibility to acquiring HIV (Workowski et al., 2008; Li et al., 2013; Rice et al., 2017). The development of effective vaccine candidates against gonococcal infection is critical for the prevention and eradication of gonorrhea; however, the extraordinary ability of N. gonorrhoeae to change its surface antigen composition, and a lack of knowledge regarding protective correlates of immunity in humans significantly complicates vaccine development (Rotman and Seifert, 2014; Edwards et al., 2016; Gulati et al., 2019; Russell et al., 2019). Some of the gonococcal surface antigens that have been considered as vaccine targets include the porin PorB, lipo-oligosaccharide glycan structures, type IV gonococcal surface pili, and gonococcal colony opacity-associated (Opa) proteins (Russell et al., 2019).
While the nanotechnology-based preclinical studies have focused on therapeutic approaches against N. gonorrheae (Li et al., 2013; Lucío et al., 2020), in this study (Gala et al., 2018), the microparticles were made containing spray dried, inactivated whole-cell gonococci strain CDC-F62 loaded in biodegradable crosslinked albumin-based particulate matrix for prolonged slow antigen release. This novel nanovaccine was administered transdermally to 6–8-week-old Swiss Webster female mice using biodegradable microneedle skin patches. The immune response of vaccinated mice was monitored for 10 weeks starting immediately after the prime vaccination, followed by two booster vaccination regimens, scheduled 1 week apart. When compared to either subcutaneous or microneedle transdermal administration of N. gonorrhoeae antigen in suspension, the novel nanovaccine induced a higher antigen-specific IgG antibody titer from week 2 with significantly higher titers at weeks 6 and 8. At week 10, all three vaccinated groups had significantly higher levels of antigen-specific CD4+ and CD8+ T lymphocytes when compared to empty microneedles and unvaccinated mice (Gala et al., 2018). Although this whole-cell-based nanovaccine did not address the current challenge of N. gonorrhoeae immune evasion due to surface antigen mutations, this preclinical study opens an avenue for the possibility of N. gonorrhoeae infection prevention with nanoparticle-based vaccines, where multiple N. gonorrhoeae strains with different immunogenic epitopes can be packaged and delivered in a nanocarrier. If successful, such a nanoparticle-based vaccine will limit immune evasion by providing protection against potential N. gonorrhoeae strains, eliminate the strenuous processes involved in the identification of a single immunogenic epitope, and ultimately reduce the rate of acquiring multi-drug resistant infections. Hence, further exploration of the immunopotentiating capacity of nanoparticle-based vaccines for novel strategies will be essential for the development of vaccines capable of overcoming the adaptive capability of N. gonorrhoeae.
5 Recommendations for Future Nanoparticle-Based Vaccines Against Sexually Transmitted Infections
To maximize the potential of engineered nanoparticle platforms, a better understanding of the mechanisms of action of all current orthodox and non-conventional vaccine formulations and therapies that provide protection against STIs including HPV and Hepatitis B is needed. Taking advantage of the suitable properties of nanoparticles as vaccine delivery platforms that will confer sustained protection against STIs is very promising. Some of these common physiochemical properties across different types of nanoparticles that make them advantageous for vaccine delivery include their ability to conjugate, encapsulate or adsorb vaccine molecules; their size and surface area, which determines the mode of cellular uptake and specificity; their hydrophobicity, which plays an integral role in interaction with immune cells and soluble proteins; their surface charge, which can be modified for targeted vaccine delivery; and their shape, which is critical for cellular interaction, intracellular trafficking and antigen release rate (Gatoo et al., 2014; Pati et al., 2018). In this review, we discussed the different nanoparticle-based vaccine platforms and their immunological benefits including improved access to lymphatic system, optimal packaging and presentation of antigens, and better induction of immune responses (Singh, 2021). In general, only a few nanoparticle-based vaccines have been used in advanced clinical trials for infectious diseases including influenza (NCT03293498, NCT03658629) and respiratory syncytial virus (Stephens and Varga, 2020) (NCT01960686, NCT02247726 and NCT02624947), and recently for Epstein-Barr virus (NCT04645147) and COVID-19 (NCT05007951). Although a handful of these platforms have been implemented for bacterial STI preclinical vaccine research, there is a critical need for more research and development that will translate these formulations to the clinics for all three STIs discussed in this review.
Author Contributions
AA-O and AR conceived the review. AA-O and AR wrote the manuscript. All authors contributed to the article and approved the submitted version.
Funding
This work was supported by Public Health Service grant U19 AI144184 from the National Institute of Allergy and Infectious Diseases.
Conflict of Interest
Patent applications have been submitted by LLNS based on NLP technology described in this review.
Publisher’s Note
All claims expressed in this article are solely those of the authors and do not necessarily represent those of their affiliated organizations, or those of the publisher, the editors and the reviewers. Any product that may be evaluated in this article, or claim that may be made by its manufacturer, is not guaranteed or endorsed by the publisher.
References
Abraham, S., Juel, H. B., Bang, P., Cheeseman, H. M., Dohn, R. B., Cole, T., et al. (2019). Safety and Immunogenicity of the chlamydia Vaccine Candidate CTH522 Adjuvanted with CAF01 Liposomes or Aluminium Hydroxide: a First-In-Human, Randomised, Double-Blind, Placebo-Controlled, Phase 1 Trial. Lancet Infect. Dis. 19, 1091–1100. doi:10.1016/S1473-3099(19)30279-8
Aikins, M. E., Bazzill, J., and Moon, J. J. (2017). Vaccine Nanoparticles for protection against HIV Infection. Nanomedicine (Lond) 12, 673–682. doi:10.2217/nnm-2016-0381
Akagi, T., Kaneko, T., Kida, T., and Akashi, M. (2005). Preparation and Characterization of Biodegradable Nanoparticles Based on Poly(gamma-Glutamic Acid) with L-Phenylalanine as a Protein Carrier. J. Control. Release 108, 226–236. doi:10.1016/j.jconrel.2005.08.003
Al-Halifa, S., Gauthier, L., Arpin, D., Bourgault, S., and Archambault, D. (2019). Nanoparticle-Based Vaccines against Respiratory Viruses. Front. Immunol. 10, 22. doi:10.3389/fimmu.2019.00022
Aldosari, B. N., Alfagih, I. M., and Almurshedi, A. S. (2021). Lipid Nanoparticles as Delivery Systems for RNA-Based Vaccines. Pharmaceutics 13, 206. doi:10.3390/pharmaceutics13020206
Arora, D., Sharma, N., Sharma, V., Abrol, V., Shankar, R., and Jaglan, S. (2016). An Update on Polysaccharide-Based Nanomaterials for Antimicrobial Applications. Appl. Microbiol. Biotechnol. 100, 2603–2615. doi:10.1007/s00253-016-7315-0
Arvizo, R., Bhattacharya, R., and Mukherjee, P. (2010). Gold Nanoparticles: Opportunities and Challenges in Nanomedicine. Expert Opin. Drug Deliv. 7, 753–763. doi:10.1517/17425241003777010
Aumiller, W. M., Uchida, M., and Douglas, T. (2018). Protein Cage Assembly across Multiple Length Scales. Chem. Soc. Rev. 47, 3433–3469. doi:10.1039/c7cs00818j
Azimi, B., Nourpanah, P., Rabiee, M., and Arbab, S. (2014). Producing Gelatin Nanoparticles as Delivery System for Bovine Serum Albumin. Iran Biomed. J. 18, 34–40. doi:10.6091/ibj.1242.2013
Bakshi, R. K., Gupta, K., Jordan, S. J., Chi, X., Lensing, S. Y., Press, C. G., et al. (2018). An Adaptive Chlamydia Trachomatis-specific IFN-γ-Producing CD4+ T Cell Response Is Associated with Protection against Chlamydia Reinfection in Women. Front. Immunol. 9, 1981. doi:10.3389/fimmu.2018.01981
Barrow, R. Y., Ahmed, F., Bolan, G. A., and Workowski, K. A. (2020). Recommendations for Providing Quality Sexually Transmitted Diseases Clinical Services. MMWR Recomm Rep. 68, 1–20. doi:10.15585/mmwr.rr6805a1
Bastús, N. G., Sánchez-Tilló, E., Pujals, S., Farrera, C., Kogan, M. J., Giralt, E., et al. (2009). Peptides Conjugated to Gold Nanoparticles Induce Macrophage Activation. Mol. Immunol. 46, 743–748. doi:10.1016/j.molimm.2008.08.277
Bayda, S., Adeel, M., Tuccinardi, T., Cordani, M., and Rizzolio, F. (2019). The History of Nanoscience and Nanotechnology: From Chemical-Physical Applications to Nanomedicine. Molecules 25, 112. doi:10.3390/molecules25010112
Bhaskar, S., and Lim, S. (2017). Engineering Protein Nanocages as Carriers for Biomedical Applications. NPG Asia Mater. 9, e371. doi:10.1038/am.2016.128
Bianco, A., Kostarelos, K., and Prato, M. (2005). Applications of Carbon Nanotubes in Drug Delivery. Curr. Opin. Chem. Biol. 9, 674–679. doi:10.1016/j.cbpa.2005.10.005
Bose, R. J., Kim, M., Chang, J. H., Paulmurugan, R., Moon, J. J., Koh, W. G., et al. (2019). Biodegradable Polymers for Modern Vaccine Development. J. Ind. Eng. Chem. 77, 12–24. doi:10.1016/j.jiec.2019.04.044
Bozzuto, G., and Molinari, A. (2015). Liposomes as Nanomedical Devices. Int. J. Nanomedicine 10, 975–999. doi:10.2147/IJN.S68861
Brunham, R. C., and Rey-Ladino, J. (2005). Immunology of Chlamydia Infection: Implications for a Chlamydia trachomatis Vaccine. Nat. Rev. Immunol. 5, 149–161. doi:10.1038/nri1551
Cambridge, C. D., Singh, S. R., Waffo, A. B., Fairley, S. J., and Dennis, V. A. (2013). Formulation, Characterization, and Expression of a Recombinant MOMP Chlamydia trachomatis DNA Vaccine Encapsulated in Chitosan Nanoparticles. Int. J. Nanomedicine 8, 1759–1771. doi:10.2147/IJN.S42723
Cameron, C. E., and Lukehart, S. A. (2014). Current Status of Syphilis Vaccine Development: Need, Challenges, Prospects. Vaccine 32, 1602–1609. doi:10.1016/j.vaccine.2013.09.053
Chattopadhyay, S., Chen, J. Y., Chen, H. W., and Hu, C. J. (2017). Nanoparticle Vaccines Adopting Virus-like Features for Enhanced Immune Potentiation. Nanotheranostics 1, 244–260. doi:10.7150/ntno.19796
Choi, B., Kim, H., Choi, H., and Kang, S. (2018). Protein Cage Nanoparticles as Delivery Nanoplatforms. Adv. Exp. Med. Biol. 1064, 27–43. doi:10.1007/978-981-13-0445-3_2
Dagnew, G. W., Asresie, M. B., and Fekadu, G. A. (2020). Factors Associated with Sexually Transmitted Infections Among Sexually Active Men in Ethiopia. Further Analysis of 2016 Ethiopian Demographic and Health Survey Data. PLoS One 15, e0232793. doi:10.1371/journal.pone.0232793
de la Maza, L. M., Zhong, G., and Brunham, R. C. (2017). Update on Chlamydia trachomatis Vaccinology. Clin. Vaccin. Immunol 24, e00543–16. doi:10.1128/CVI.00543-16
Dean, D. (2009). Chlamydia trachomatis Today: Treatment, Detection, Immunogenetics and the Need for a Greater Global Understanding of Chlamydial Disease Pathogenesis. Drugs Today (Barc) 45 (Suppl. B), 25–31.
DeFrates, K., Markiewicz, T., Gallo, P., Rack, A., Weyhmiller, A., Jarmusik, B., et al. (2018). Protein Polymer-Based Nanoparticles: Fabrication and Medical Applications. Int. J. Mol. Sci. 19. doi:10.3390/ijms19061717
Diaz, D., Care, A., and Sunna, A. (2018). Bioengineering Strategies for Protein-Based Nanoparticles. Genes (Basel) 9, 370. doi:10.3390/genes9070370
Dixit, S., Sahu, R., Verma, R., Duncan, S., Giambartolomei, G. H., Singh, S. R., et al. (2018). Caveolin-mediated Endocytosis of the Chlamydia M278 Outer Membrane Peptide Encapsulated in Poly(lactic Acid)-Poly(ethylene Glycol) Nanoparticles by Mouse Primary Dendritic Cells Enhances Specific Immune Effectors Mediated by MHC Class II and CD4+ T Cells. Biomaterials 159, 130–145. doi:10.1016/j.biomaterials.2017.12.019
Dixit, S., Singh, S. R., Yilma, A. N., Agee, R. D., Taha, M., and Dennis, V. A. (2014). Poly(lactic Acid)-Poly(ethylene Glycol) Nanoparticles Provide Sustained Delivery of a Chlamydia trachomatis Recombinant MOMP Peptide and Potentiate Systemic Adaptive Immune Responses in Mice. Nanomedicine 10, 1311–1321. doi:10.1016/j.nano.2014.02.009
Donaldson, K., Aitken, R., Tran, L., Stone, V., Duffin, R., Forrest, G., et al. (2006). Carbon Nanotubes: a Review of Their Properties in Relation to Pulmonary Toxicology and Workplace Safety. Toxicol. Sci. 92, 5–22. doi:10.1093/toxsci/kfj130
Dürr, U. H., Gildenberg, M., and Ramamoorthy, A. (2012). The Magic of Bicelles Lights up Membrane Protein Structure. Chem. Rev. 112, 6054–6074. doi:10.1021/cr300061w
Dykman, L., and Khlebtsov, N. (2012). Gold Nanoparticles in Biomedical Applications: Recent Advances and Perspectives. Chem. Soc. Rev. 41, 2256–2282. doi:10.1039/c1cs15166e
Dykman, L. A. (2020). Gold Nanoparticles for Preparation of Antibodies and Vaccines against Infectious Diseases. Expert Rev. Vaccin. 19, 465–477. doi:10.1080/14760584.2020.1758070
Edwards, J. L., Jennings, M. P., Apicella, M. A., and Seib, K. L. (2016). Is Gonococcal Disease Preventable? the Importance of Understanding Immunity and Pathogenesis in Vaccine Development. Crit. Rev. Microbiol. 42, 928–941. doi:10.3109/1040841X.2015.1105782
Elwell, C., Mirrashidi, K., and Engel, J. (2016). Chlamydia Cell Biology and Pathogenesis. Nat. Rev. Microbiol. 14, 385–400. doi:10.1038/nrmicro.2016.30
Elzoghby, A. O., Samy, W. M., and Elgindy, N. A. (2012). Albumin-based Nanoparticles as Potential Controlled Release Drug Delivery Systems. J. Control. Release 157, 168–182. doi:10.1016/j.jconrel.2011.07.031
Fairley, S. J., Singh, S. R., Yilma, A. N., Waffo, A. B., Subbarayan, P., Dixit, S., et al. (2013). Chlamydia trachomatis Recombinant MOMP Encapsulated in PLGA Nanoparticles Triggers Primarily T Helper 1 Cellular and Antibody Immune Responses in Mice: a Desirable Candidate Nanovaccine. Int. J. Nanomedicine 8, 2085–2099. doi:10.2147/IJN.S44155
Fischer, A., and Rudel, T. (2018). Subversion of Cell-Autonomous Host Defense by Chlamydia Infection. Curr. Top. Microbiol. Immunol. 412, 81–106. doi:10.1007/82_2016_13
Fischer, N. O., Rasley, A., Corzett, M., Hwang, M. H., Hoeprich, P. D., and Blanchette, C. D. (2013). Colocalized Delivery of Adjuvant and Antigen Using Nanolipoprotein Particles Enhances the Immune Response to Recombinant Antigens. J. Am. Chem. Soc. 135, 2044–2047. doi:10.1021/ja3063293
Flower, D. R., Macdonald, I. K., Ramakrishnan, K., Davies, M. N., and Doytchinova, I. A. (2010). Computer Aided Selection of Candidate Vaccine Antigens. Immunome Res. 6 (Suppl. 2), S1. doi:10.1186/1745-7580-6-S2-S1
Fox, C. B., Kramer, R. M., Barnes V, L. L., Dowling, Q. M., and Vedvick, T. S. (2013). Working Together: Interactions between Vaccine Antigens and Adjuvants. Ther. Adv. Vaccin. 1, 7–20. doi:10.1177/2051013613480144
Gala, R. P., Zaman, R. U., D'Souza, M. J., and Zughaier, S. M. (2018). Novel Whole-Cell Inactivated Neisseria Gonorrhoeae Microparticles as Vaccine Formulation in Microneedle-Based Transdermal Immunization. Vaccines (Basel) 6. doi:10.3390/vaccines6030060
Gao, Y., Wijewardhana, C., and Mann, J. F. S. (2018). Virus-Like Particle, Liposome, and Polymeric Particle-Based Vaccines against HIV-1. Front. Immunol. 9, 345. doi:10.3389/fimmu.2018.00345
Gatoo, M. A., Naseem, S., Arfat, M. Y., Dar, A. M., Qasim, K., and Zubair, S. (2014). Physicochemical Properties of Nanomaterials: Implication in Associated Toxic Manifestations. Biomed. Res. Int. 2014, 498420. doi:10.1155/2014/498420
Geisler, R., Pedersen, M. C., Hannappel, Y., Schweins, R., Prévost, S., Dattani, R., et al. (2019). Aescin-Induced Conversion of Gel-phase Lipid Membranes into Bicelle-like Lipid Nanoparticles. Langmuir 35, 16244–16255. doi:10.1021/acs.langmuir.9b02077
Gericke, M., Schulze, P., and Heinze, T. (2020). Nanoparticles Based on Hydrophobic Polysaccharide Derivatives-Formation Principles, Characterization Techniques, and Biomedical Applications. Macromol Biosci. 20, e1900415. doi:10.1002/mabi.201900415
Gondek, D. C., Olive, A. J., Stary, G., and Starnbach, M. N. (2012). CD4+ T Cells Are Necessary and Sufficient to Confer protection against Chlamydia trachomatis Infection in the Murine Upper Genital Tract. J. Immunol. 189, 2441–2449. doi:10.4049/jimmunol.1103032
Gottlieb, S. L., and Johnston, C. (2017). Future Prospects for New Vaccines against Sexually Transmitted Infections. Curr. Opin. Infect. Dis. 30, 77–86. doi:10.1097/QCO.0000000000000343
Guevara, M. L., Persano, F., and Persano, S. (2020). Advances in Lipid Nanoparticles for mRNA-Based Cancer Immunotherapy. Front. Chem. 8, 589959. doi:10.3389/fchem.2020.589959
Gulati, S., Shaughnessy, J., Ram, S., and Rice, P. A. (2019). Targeting Lipooligosaccharide (LOS) for a Gonococcal Vaccine. Front. Immunol. 10, 321. doi:10.3389/fimmu.2019.00321
Hadinoto, K., Sundaresan, A., and Cheow, W. S. (2013). Lipid-polymer Hybrid Nanoparticles as a New Generation Therapeutic Delivery Platform: a Review. Eur. J. Pharm. Biopharm. 85, 427–443. doi:10.1016/j.ejpb.2013.07.002
Han, J., Zhao, D., Li, D., Wang, X., Jin, Z., and Zhao, K. (2018). Polymer-Based Nanomaterials and Applications for Vaccines and Drugs. Polymers (Basel) 10, 31. doi:10.3390/polym10010031
Hassett, K. J., Benenato, K. E., Jacquinet, E., Lee, A., Woods, A., Yuzhakov, O., et al. (2019). Optimization of Lipid Nanoparticles for Intramuscular Administration of mRNA Vaccines. Mol. Ther. Nucleic Acids 15, 1–11. doi:10.1016/j.omtn.2019.01.013
He, W., Felderman, M., Evans, A. C., Geng, J., Homan, D., Bourguet, F., et al. (2017). Cell-free Production of a Functional Oligomeric Form of a Chlamydia Major Outer-Membrane Protein (MOMP) for Vaccine Development. J. Biol. Chem. 292, 15121–15132. doi:10.1074/jbc.M117.784561
Husseini, G. A., and Pitt, W. G. (2008). Micelles and Nanoparticles for Ultrasonic Drug and Gene Delivery. Adv. Drug Deliv. Rev. 60, 1137–1152. doi:10.1016/j.addr.2008.03.008
Iravani, S., Korbekandi, H., Mirmohammadi, S. V., and Zolfaghari, B. (2014). Synthesis of Silver Nanoparticles: Chemical, Physical and Biological Methods. Res. Pharm. Sci. 9, 385–406.
Jaiswal, M., Dudhe, R., and Sharma, P. K. (2015). Nanoemulsion: an Advanced Mode of Drug Delivery System. 3 Biotech. 5, 123–127. doi:10.1007/s13205-014-0214-0
Jeevanandam, J., Barhoum, A., Chan, Y. S., Dufresne, A., and Danquah, M. K. (2018). Review on Nanoparticles and Nanostructured Materials: History, Sources, Toxicity and Regulations. Beilstein J. Nanotechnol 9, 1050–1074. doi:10.3762/bjnano.9.98
Jiang, J., Liu, G., Kickhoefer, V. A., Rome, L. H., Li, L. X., McSorley, S. J., et al. (2017). A Protective Vaccine against Chlamydia Genital Infection Using Vault Nanoparticles without an Added Adjuvant. Vaccines (Basel) 5, 3. doi:10.3390/vaccines5010003
Kari, L., Whitmire, W. M., Crane, D. D., Reveneau, N., Carlson, J. H., Goheen, M. M., et al. (2009). Chlamydia trachomatis Native Major Outer Membrane Protein Induces Partial protection in Nonhuman Primates: Implication for a Trachoma Transmission-Blocking Vaccine. J. Immunol. 182, 8063–8070. doi:10.4049/jimmunol.0804375
Karimi, M., Bahrami, S., Ravari, S. B., Zangabad, P. S., Mirshekari, H., Bozorgomid, M., et al. (2016). Albumin Nanostructures as Advanced Drug Delivery Systems. Expert Opin. Drug Deliv. 13, 1609–1623. doi:10.1080/17425247.2016.1193149
Kelly, S. M., Mitra, A., Mathur, S., and Narasimhan, B. (2020). Synthesis and Characterization of Rapidly Degrading Polyanhydrides as Vaccine Adjuvants. ACS Biomater. Sci. Eng. 6, 265–276. doi:10.1021/acsbiomaterials.9b01427
Kim, H. S., Kwon, H. K., Lee, D. H., Le, T. N., Park, H. J., and Kim, M. I. (2019). Poly(γ-Glutamic Acid)/Chitosan Hydrogel Nanoparticles for Effective Preservation and Delivery of Fermented Herbal Extract for Enlarging Hair Bulb and Enhancing Hair Growth. Int. J. Nanomedicine 14, 8409–8419. doi:10.2147/IJN.S227514
Kirnbauer, R., Booy, F., Cheng, N., Lowy, D. R., and Schiller, J. T. (1992). Papillomavirus L1 Major Capsid Protein Self-Assembles into Virus-like Particles that Are Highly Immunogenic. Proc. Natl. Acad. Sci. U S A. 89, 12180–12184. doi:10.1073/pnas.89.24.12180
Koudelka, K. J., Pitek, A. S., Manchester, M., and Steinmetz, N. F. (2015). Virus-Based Nanoparticles as Versatile Nanomachines. Annu. Rev. Virol. 2, 379–401. doi:10.1146/annurev-virology-100114-055141
Labuda, J. C., and McSorley, S. J. (2018). Diversity in the T Cell Response to Chlamydia-Sum Are Better Than One. Immunol. Lett. 202, 59–64. doi:10.1016/j.imlet.2018.08.002
Lee, E. J., Khan, S. A., and Lim, K. H. (2011). Gelatin Nanoparticle Preparation by Nanoprecipitation. J. Biomater. Sci. Polym. Ed. 22, 753–771. doi:10.1163/092050610X492093
Lee, J., Sands, I., Zhang, W., Zhou, L., and Chen, Y. (2021). DNA-inspired Nanomaterials for Enhanced Endosomal Escape. Proc. Natl. Acad. Sci. USA 118, e2104511118. doi:10.1073/pnas.2104511118
Li, L. H., Yen, M. Y., Ho, C. C., Wu, P., Wang, C. C., Maurya, P. K., et al. (2013). Non-cytotoxic Nanomaterials Enhance Antimicrobial Activities of Cefmetazole against Multidrug-Resistant Neisseria Gonorrhoeae. PLoS One 8, e64794. doi:10.1371/journal.pone.0064794
Lijek, R. S., Helble, J. D., Olive, A. J., Seiger, K. W., and Starnbach, M. N. (2018). Pathology after Chlamydia trachomatis Infection Is Driven by Nonprotective Immune Cells that Are Distinct from Protective Populations. Proc. Natl. Acad. Sci. U S A. 115, 2216–2221. doi:10.1073/pnas.1711356115
Lithgow, K. V., and Cameron, C. E. (2017). Vaccine Development for Syphilis. Expert Rev. Vaccin. 16, 37–44. doi:10.1080/14760584.2016.1203262
Lithgow, K. V., Hof, R., Wetherell, C., Phillips, D., Houston, S., and Cameron, C. E. (2017). A Defined Syphilis Vaccine Candidate Inhibits Dissemination of Treponema pallidum Subspecies Pallidum. Nat. Commun. 8, 14273. doi:10.1038/ncomms14273
Lofano, G., Mallett, C. P., Bertholet, S., and O'Hagan, D. T. (2020). Technological Approaches to Streamline Vaccination Schedules, Progressing towards Single-Dose Vaccines. NPJ Vaccin. 5, 88. doi:10.1038/s41541-020-00238-8
Lohcharoenkal, W., Wang, L., Chen, Y. C., and Rojanasakul, Y. (2014). Protein Nanoparticles as Drug Delivery Carriers for Cancer Therapy. Biomed. Res. Int. 2014, 180549. doi:10.1155/2014/180549
López-Sagaseta, J., Malito, E., Rappuoli, R., and Bottomley, M. J. (2016). Self-assembling Protein Nanoparticles in the Design of Vaccines. Comput. Struct. Biotechnol. J. 14, 58–68. doi:10.1016/j.csbj.2015.11.001
Lucío, M. I., Kyriazi, M. E., Hamilton, J., Batista, D., Sheppard, A., Sams-Dodd, E., et al. (2020). Bactericidal Effect of 5-Mercapto-2-Nitrobenzoic Acid-Coated Silver Nanoclusters against Multidrug-Resistant Neisseria Gonorrhoeae. ACS Appl. Mater. Inter. 12, 27994–28003. doi:10.1021/acsami.0c06163
Lujan, H., Griffin, W. C., Taube, J. H., and Sayes, C. M. (2019). Synthesis and Characterization of Nanometer-Sized Liposomes for Encapsulation and microRNA Transfer to Breast Cancer Cells. Int. J. Nanomedicine 14, 5159–5173. doi:10.2147/IJN.S203330
Makadia, H. K., and Siegel, S. J. (2011). Poly Lactic-Co-Glycolic Acid (PLGA) as Biodegradable Controlled Drug Delivery Carrier. Polymers (Basel) 3, 1377–1397. doi:10.3390/polym3031377
Malekhosseini, P., Alami, M., Khomeiri, M., Esteghlal, S., Nekoei, A. R., and Hosseini, S. M. H. (2019). Development of Casein-Based Nanoencapsulation Systems for Delivery of Epigallocatechin Gallate and Folic Acid. Food Sci. Nutr. 7, 519–527. doi:10.1002/fsn3.827
Marques Neto, L. M., Kipnis, A., and Junqueira-Kipnis, A. P. (2017). Role of Metallic Nanoparticles in Vaccinology: Implications for Infectious Disease Vaccine Development. Front. Immunol. 8, 239. doi:10.3389/fimmu.2017.00239
Martinez, A. P., Qamar, B., Fuerst, T. R., Muro, S., and Andrianov, A. K. (2017). Biodegradable "Smart" Polyphosphazenes with Intrinsic Multifunctionality as Intracellular Protein Delivery Vehicles. Biomacromolecules 18, 2000–2011. doi:10.1021/acs.biomac.7b00537
Mathur, P., Jha, S., Ramteke, S., and Jain, N. K. (2018). Pharmaceutical Aspects of Silver Nanoparticles. Artif. Cell Nanomed Biotechnol 46, 115–126. doi:10.1080/21691401.2017.1414825
Mohan, T., Verma, P., and Rao, D. N. (2013). Novel Adjuvants & Delivery Vehicles for Vaccines Development: a Road Ahead. Indian J. Med. Res. 138, 779–795.
Monfort, D. A., and Koria, P. (2017). Recombinant Elastin-Based Nanoparticles for Targeted Gene Therapy. Gene Ther. 24, 610–620. doi:10.1038/gt.2017.54
Motevalli, F., Bolhassani, A., Hesami, S., and Shahbazi, S. (2018). Supercharged green Fluorescent Protein Delivers HPV16E7 DNA and Protein into Mammalian Cells In Vitro and In Vivo. Immunol. Lett. 194, 29–39. doi:10.1016/j.imlet.2017.12.005
Mourdikoudis, S., Pallares, R. M., and Thanh, N. T. K. (2018). Characterization Techniques for Nanoparticles: Comparison and Complementarity upon Studying Nanoparticle Properties. Nanoscale 10, 12871–12934. doi:10.1039/c8nr02278j
Murthy, A. K., Li, W., and Ramsey, K. H. (2018). Immunopathogenesis of Chlamydial Infections. Curr. Top. Microbiol. Immunol. 412, 183–215. doi:10.1007/82_2016_18
Nagarajan, U., Kawakami, K., Zhang, S., Chandrasekaran, B., and Unni Nair, B. (2014). Fabrication of Solid Collagen Nanoparticles Using Electrospray Deposition. Chem. Pharm. Bull. (Tokyo) 62, 422–428. doi:10.1248/cpb.c13-01004
Nair, M. S., Lee, M. M., Bonnegarde-Bernard, A., Wallace, J. A., Dean, D. H., Ostrowski, M. C., et al. (2015). Cry Protein Crystals: a Novel Platform for Protein Delivery. PLoS One 10, e0127669. doi:10.1371/journal.pone.0127669
Nans, A., Ford, C., and Hayward, R. D. (2015). Host-pathogen Reorganisation during Host Cell Entry by Chlamydia trachomatis. Microbes Infect. 17, 727–731. doi:10.1016/j.micinf.2015.08.004
Nasir, A. (2009). Nanotechnology in Vaccine Development: a Step Forward. J. Invest. Dermatol. 129, 1055–1059. doi:10.1038/jid.2009.63
Neek, M., Kim, T. I., and Wang, S. W. (2019). Protein-based Nanoparticles in Cancer Vaccine Development. Nanomedicine 15, 164–174. doi:10.1016/j.nano.2018.09.004
Nguyen, B., and Tolia, N. H. (2021). Protein-based Antigen Presentation Platforms for Nanoparticle Vaccines. NPJ Vaccin. 6, 70. doi:10.1038/s41541-021-00330-7
Niu, Z., Conejos-Sánchez, I., Griffin, B. T., O'Driscoll, C. M., and Alonso, M. J. (2016). Lipid-based Nanocarriers for Oral Peptide Delivery. Adv. Drug Deliv. Rev. 106, 337–354. doi:10.1016/j.addr.2016.04.001
Norris, E. J., Mullis, A. S., Phanse, Y., Narasimhan, B., Coats, J. R., and Bartholomay, L. C. (2020). Biodistribution of Degradable Polyanhydride Particles in Aedes aegypti Tissues. Plos Negl. Trop. Dis. 14, e0008365. doi:10.1371/journal.pntd.0008365
Oh, Y. K., Sohn, T., Park, J. S., Kang, M. J., Choi, H. G., Kim, J. A., et al. (2004). Enhanced Mucosal and Systemic Immunogenicity of Human Papillomavirus-like Particles Encapsidating Interleukin-2 Gene Adjuvant. Virology 328, 266–273. doi:10.1016/j.virol.2004.06.047
Paavonen, J., and Eggert-Kruse, W. (1999). Chlamydia trachomatis: Impact on Human Reproduction. Hum. Reprod. Update 5, 433–447. doi:10.1093/humupd/5.5.433
Pal, S., Favaroni, A., Tifrea, D. F., Hanisch, P. T., Luczak, S. E. T., Hegemann, J. H., et al. (2017). Comparison of the Nine Polymorphic Membrane Proteins of Chlamydia trachomatis for Their Ability to Induce Protective Immune Responses in Mice against a C. Muridarum challenge. Vaccine 35, 2543–2549. doi:10.1016/j.vaccine.2017.03.070
Pal, S., Mirzakhanyan, Y., Gershon, P., Tifrea, D. F., and de la Maza, L. M. (2020). Induction of protection in Mice against a Respiratory challenge by a Vaccine Formulated with Exosomes Isolated from Chlamydia Muridarum Infected Cells. NPJ Vaccin. 5, 87. doi:10.1038/s41541-020-00235-x
Pal, S., Peterson, E. M., and de la Maza, L. M. (2005). Vaccination with the Chlamydia trachomatis Major Outer Membrane Protein Can Elicit an Immune Response as Protective as that Resulting from Inoculation with Live Bacteria. Infect. Immun. 73, 8153–8160. doi:10.1128/IAI.73.12.8153-8160.2005
Park, K. S., Bazzill, J. D., Son, S., Nam, J., Shin, S. W., Ochyl, L. J., et al. (2021). Lipid-based Vaccine Nanoparticles for Induction of Humoral Immune Responses against HIV-1 and SARS-CoV-2. J. Control. Release 330, 529–539. doi:10.1016/j.jconrel.2020.12.031
Pati, R., Shevtsov, M., and Sonawane, A. (2018). Nanoparticle Vaccines against Infectious Diseases. Front. Immunol. 9, 2224. doi:10.3389/fimmu.2018.02224
Paz De la Rosa, G., Monroy-García, A., Mora-García, Mde. L., Peña, C. G., Hernández-Montes, J., Weiss-Steider, B., et al. (2009). An HPV 16 L1-Based Chimeric Human Papilloma Virus-like Particles Containing a String of Epitopes Produced in Plants Is Able to Elicit Humoral and Cytotoxic T-Cell Activity in Mice. Virol. J. 6, 2. doi:10.1186/1743-422X-6-2
Peek, L. J., Middaugh, C. R., and Berkland, C. (2008). Nanotechnology in Vaccine Delivery. Adv. Drug Deliv. Rev. 60, 915–928. doi:10.1016/j.addr.2007.05.017
Phillips, S., Quigley, B. L., and Timms, P. (2019). Seventy Years of Chlamydia Vaccine Research - Limitations of the Past and Directions for the Future. Front. Microbiol. 10, 70. doi:10.3389/fmicb.2019.00070
Pradines, B., Bories, C., Vauthier, C., Ponchel, G., Loiseau, P. M., and Bouchemal, K. (2015). Drug-free Chitosan Coated Poly(isobutylcyanoacrylate) Nanoparticles Are Active against Trichomonas Vaginalis and Non-toxic towards Pig Vaginal Mucosa. Pharm. Res. 32, 1229–1236. doi:10.1007/s11095-014-1528-7
Prego, C., Paolicelli, P., Díaz, B., Vicente, S., Sánchez, A., González-Fernández, A., et al. (2010). Chitosan-based Nanoparticles for Improving Immunization against Hepatitis B Infection. Vaccine 28, 2607–2614. doi:10.1016/j.vaccine.2010.01.011
Qin, X., Yu, C., Wei, J., Li, L., Zhang, C., Wu, Q., et al. (2019). Rational Design of Nanocarriers for Intracellular Protein Delivery. Adv. Mater. 31, e1902791. doi:10.1002/adma.201902791
Rafique, M., Sadaf, I., Rafique, M. S., and Tahir, M. B. (2017). A Review on green Synthesis of Silver Nanoparticles and Their Applications. Artif. Cell Nanomed Biotechnol 45, 1272–1291. doi:10.1080/21691401.2016.1241792
Reljic, R., and González-Fernández, Á. (2019). Editorial: Nanoparticle Vaccines against Infectious Diseases. Front. Immunol. 10, 2615. doi:10.3389/fimmu.2019.02615
Rice, P. A., Shafer, W. M., Ram, S., and Jerse, A. E. (2017). Neisseria Gonorrhoeae: Drug Resistance, Mouse Models, and Vaccine Development. Annu. Rev. Microbiol. 71, 665–686. doi:10.1146/annurev-micro-090816-093530
Rodrigues, L., Raftopoulos, K. N., Tandrup Schmidt, S., Schneider, F., Dietz, H., Rades, T., et al. (2018). Immune Responses Induced by Nano-Self-Assembled Lipid Adjuvants Based on a Monomycoloyl Glycerol Analogue after Vaccination with the Chlamydia trachomatis Major Outer Membrane Protein. J. Control. Release 285, 12–22. doi:10.1016/j.jconrel.2018.06.028
Rosalia, R. A., Cruz, L. J., van Duikeren, S., Tromp, A. T., Silva, A. L., Jiskoot, W., et al. (2015). CD40-targeted Dendritic Cell Delivery of PLGA-Nanoparticle Vaccines Induce Potent Anti-tumor Responses. Biomaterials 40, 88–97. doi:10.1016/j.biomaterials.2014.10.053
Rose, F., Wern, J. E., Gavins, F., Andersen, P., Follmann, F., and Foged, C. (2018). A strong Adjuvant Based on Glycol-Chitosan-Coated Lipid-Polymer Hybrid Nanoparticles Potentiates Mucosal Immune Responses against the Recombinant Chlamydia trachomatis Fusion Antigen CTH522. J. Control. Release 271, 88–97. doi:10.1016/j.jconrel.2017.12.003
Rose, F., Wern, J. E., Ingvarsson, P. T., van de Weert, M., Andersen, P., Follmann, F., et al. (2015). Engineering of a Novel Adjuvant Based on Lipid-Polymer Hybrid Nanoparticles: A Quality-By-Design Approach. J. Control. Release 210, 48–57. doi:10.1016/j.jconrel.2015.05.004
Rotman, E., and Seifert, H. S. (2014). The Genetics of Neisseria Species. Annu. Rev. Genet. 48, 405–431. doi:10.1146/annurev-genet-120213-092007
Rowley, J., Vander Hoorn, S., Korenromp, E., Low, N., Unemo, M., Abu-Raddad, L. J., et al. (2019). Chlamydia, Gonorrhoea, Trichomoniasis and Syphilis: Global Prevalence and Incidence Estimates. Bull. World Health Organ. 97, 548–562P. doi:10.2471/BLT.18.228486
Russell, M. W., Jerse, A. E., and Gray-Owen, S. D. (2019). Progress toward a Gonococcal Vaccine: The Way Forward. Front. Immunol. 10, 2417. doi:10.3389/fimmu.2019.02417
Sahu, R., Dixit, S., Verma, R., Duncan, S. A., Coats, M. T., Giambartolomei, G. H., et al. (2020). A Nanovaccine Formulation of Chlamydia Recombinant MOMP Encapsulated in PLGA 85:15 Nanoparticles Augments CD4+ Effector (CD44high CD62Llow) and Memory (CD44high CD62Lhigh) T-Cells in Immunized Mice. Nanomedicine 29, 102257. doi:10.1016/j.nano.2020.102257
Sahu, R., Dixit, S., Verma, R., Duncan, S. A., Smith, L., Giambartolomei, G. H., et al. (2021). Encapsulation of Recombinant MOMP in Extended-Releasing PLGA 85:15 Nanoparticles Confer Protective Immunity against a Chlamydia Muridarum Genital Challenge and Re-Challenge. Front. Immunol. 12, 660932. doi:10.3389/fimmu.2021.660932
Sahu, R., Verma, R., Dixit, S., Igietseme, J. U., Black, C. M., Duncan, S., et al. (2018). Future of Human Chlamydia Vaccine: Potential of Self-Adjuvanting Biodegradable Nanoparticles as Safe Vaccine Delivery Vehicles. Expert Rev. Vaccin. 17, 217–227. doi:10.1080/14760584.2018.1435279
Sexton, J., Garnett, G., and Røttingen, J. A. (2005). Metaanalysis and Metaregression in Interpreting Study Variability in the Impact of Sexually Transmitted Diseases on Susceptibility to HIV Infection. Sex. Transm. Dis. 32, 351–357. doi:10.1097/01.olq.0000154504.54686.d1
Shen, Z., Ye, H., Kröger, M., Tang, S., and Li, Y. (2019). Interplay between Ligand Mobility and Nanoparticle Geometry during Cellular Uptake of PEGylated Liposomes and Bicelles. Nanoscale 11, 15971–15983. doi:10.1039/c9nr02408e
Shin, M. D., Shukla, S., Chung, Y. H., Beiss, V., Chan, S. K., Ortega-Rivera, O. A., et al. (2020). COVID-19 Vaccine Development and a Potential Nanomaterial Path Forward. Nat. Nanotechnol 15, 646–655. doi:10.1038/s41565-020-0737-y
Singh, A. (2021). Eliciting B Cell Immunity against Infectious Diseases Using Nanovaccines. Nat. Nanotechnol 16, 16–24. doi:10.1038/s41565-020-00790-3
Sliepen, K., Ozorowski, G., Burger, J. A., van Montfort, T., Stunnenberg, M., LaBranche, C., et al. (2015). Presenting Native-like HIV-1 Envelope Trimers on Ferritin Nanoparticles Improves Their Immunogenicity. Retrovirology 12, 82. doi:10.1186/s12977-015-0210-4
Slupetzky, K., Gambhira, R., Culp, T. D., Shafti-Keramat, S., Schellenbacher, C., Christensen, N. D., et al. (2007). A Papillomavirus-like Particle (VLP) Vaccine Displaying HPV16 L2 Epitopes Induces Cross-Neutralizing Antibodies to HPV11. Vaccine 25, 2001–2010. doi:10.1016/j.vaccine.2006.11.049
Sneh-Edri, H., Likhtenshtein, D., and Stepensky, D. (2011). Intracellular Targeting of PLGA Nanoparticles Encapsulating Antigenic Peptide to the Endoplasmic Reticulum of Dendritic Cells and its Effect on Antigen Cross-Presentation In Vitro. Mol. Pharm. 8, 1266–1275. doi:10.1021/mp200198c
Sosa-Acosta, J. R., Iriarte-Mesa, C., Ortega, G. A., and Díaz-García, A. M. (2020). DNA-iron Oxide Nanoparticles Conjugates: Functional Magnetic Nanoplatforms in Biomedical Applications. Top. Curr. Chem. (Cham) 378, 13. doi:10.1007/s41061-019-0277-9
Stamm, W. E. (1999). Chlamydia trachomatis Infections: Progress and Problems. J. Infect. Dis. 179 (Suppl. 2), S380–S383. doi:10.1086/513844
Stephens, L. M., and Varga, S. M. (2020). Nanoparticle Vaccines against Respiratory Syncytial Virus. Future Virol. 15, 763–778. doi:10.2217/fvl-2020-0174
Su, H., and Caldwell, H. D. (1995). CD4+ T Cells Play a Significant Role in Adoptive Immunity to Chlamydia trachomatis Infection of the Mouse Genital Tract. Infect. Immun. 63, 3302–3308. doi:10.1128/iai.63.9.3302-3308.1995
Su, X., Fricke, J., Kavanagh, D. G., and Irvine, D. J. (2011). In Vitro and In Vivo mRNA Delivery Using Lipid-Enveloped pH-Responsive Polymer Nanoparticles. Mol. Pharm. 8, 774–787. doi:10.1021/mp100390w
Taha, M. A., Singh, S. R., and Dennis, V. A. (2012). Biodegradable PLGA85/15 Nanoparticles as a Delivery Vehicle for Chlamydia trachomatis Recombinant MOMP-187 Peptide. Nanotechnology 23, 325101. doi:10.1088/0957-4484/23/32/325101
Thomas, P. P. M., Yadav, J., Kant, R., Ambrosino, E., Srivastava, S., Batra, G., et al. (2019). Sexually Transmitted Infections and Behavioral Determinants of Sexual and Reproductive Health in the Allahabad District (India) Based on Data from the ChlamIndia Study. Microorganisms 7, 557. doi:10.3390/microorganisms7110557
Thorp, E. B., Boada, C., Jarbath, C., and Luo, X. (2020). Nanoparticle Platforms for Antigen-specific Immune Tolerance. Front. Immunol. 11, 945. doi:10.3389/fimmu.2020.00945
Thukral, A., Ross, K., Hansen, C., Phanse, Y., Narasimhan, B., Steinberg, H., et al. (2020). A Single Dose Polyanhydride-Based Nanovaccine against Paratuberculosis Infection. NPJ Vaccin. 5, 15. doi:10.1038/s41541-020-0164-y
Tifrea, D. F., He, W., Pal, S., Evans, A. C., Gilmore, S. F., Fischer, N. O., et al. (2021). Induction of Protection in Mice against a Chlamydia Muridarum Respiratory Challenge by a Vaccine Formulated with the Major Outer Membrane Protein in Nanolipoprotein Particles. Vaccines (Basel) 9. doi:10.3390/vaccines9070755
Tokatlian, T., Read, B. J., Jones, C. A., Kulp, D. W., Menis, S., Chang, J. Y. H., et al. (2019). Innate Immune Recognition of Glycans Targets HIV Nanoparticle Immunogens to Germinal Centers. Science 363, 649–654. doi:10.1126/science.aat9120
Tyler, M., Tumban, E., Peabody, D. S., and Chackerian, B. (2014). The Use of Hybrid Virus-like Particles to Enhance the Immunogenicity of a Broadly Protective HPV Vaccine. Biotechnol. Bioeng. 111, 2398–2406. doi:10.1002/bit.25311
Tyrrell, Z. L., Shen, Y., and Radosz, M. (2010). Fabrication of Micellar Nanoparticles for Drug Delivery through the Self-Assembly of Block Copolymers. Prog. Polym. Sci. 35, 1128–1143. doi:10.1016/j.progpolymsci.2010.06.003
Vartak, A., and Sucheck, S. (2016). Recent Advances in Subunit Vaccine Carriers. Vaccines 4, 12. doi:10.3390/vaccines4020012
Vasilevsky, S., Greub, G., Nardelli-Haefliger, D., and Baud, D. (2014). Genital Chlamydia trachomatis: Understanding the Roles of Innate and Adaptive Immunity in Vaccine Research. Clin. Microbiol. Rev. 27, 346–370. doi:10.1128/CMR.00105-13
Vasilevsky, S., Stojanov, M., Greub, G., and Baud, D. (2016). Chlamydial Polymorphic Membrane Proteins: Regulation, Function and Potential Vaccine Candidates. Virulence 7, 11–22. doi:10.1080/21505594.2015.1111509
Verma, R., Sahu, R., Dixit, S., Duncan, S. A., Giambartolomei, G. H., Singh, S. R., et al. (2018). The Chlamydia M278 Major Outer Membrane Peptide Encapsulated in the Poly(lactic Acid)-Poly(ethylene Glycol) Nanoparticulate Self-Adjuvanting Delivery System Protects Mice against a Chlamydia Muridarum Genital Tract Challenge by Stimulating Robust Systemic and Local Mucosal Immune Responses. Front. Immunol. 9, 2369. doi:10.3389/fimmu.2018.02369
Wagenlehner, F. M., Brockmeyer, N. H., Discher, T., Friese, K., and Wichelhaus, T. A. (2016). The Presentation, Diagnosis, and Treatment of Sexually Transmitted Infections. Dtsch Arztebl Int. 113, 11–22. doi:10.3238/arztebl.2016.0011
Wahajuddin , , and Arora, S. (2012). Superparamagnetic Iron Oxide Nanoparticles: Magnetic Nanoplatforms as Drug Carriers. Int. J. Nanomedicine 7, 3445–3471. doi:10.2147/IJN.S30320
Walker, G. J., Walker, D., Molano Franco, D., and Grillo-Ardila, C. F. (2019). Antibiotic Treatment for Newborns with Congenital Syphilis. Cochrane Database Syst. Rev. 2, CD012071. doi:10.1002/14651858.CD012071.pub2
Walls, A. C., Fiala, B., Schäfer, A., Wrenn, S., Pham, M. N., Murphy, M., et al. (2020). Elicitation of Potent Neutralizing Antibody Responses by Designed Protein Nanoparticle Vaccines for SARS-CoV-2. Cell 183, 1367–e17.e1317. doi:10.1016/j.cell.2020.10.043
Wang, C., Zhu, W., Luo, Y., and Wang, B. Z. (2018). Gold Nanoparticles Conjugating Recombinant Influenza Hemagglutinin Trimers and Flagellin Enhanced Mucosal Cellular Immunity. Nanomedicine 14, 1349–1360. doi:10.1016/j.nano.2018.03.007
Wang, D., Ye, J., Hudson, S. D., Scott, K. C., and Lin-Gibson, S. (2014). Effects of Nanoparticle Size and Charge on Interactions with Self-Assembled Collagen. J. Colloid Interf. Sci 417, 244–249. doi:10.1016/j.jcis.2013.11.019
Wang, E. C., and Wang, A. Z. (2014). Nanoparticles and Their Applications in Cell and Molecular Biology. Integr. Biol. (Camb) 6, 9–26. doi:10.1039/c3ib40165k
Wang, L., Xing, D., Le Van, A., Jerse, A. E., and Wang, S. (2017). Structure-based Design of Ferritin Nanoparticle Immunogens Displaying Antigenic Loops of Neisseria Gonorrhoeae. FEBS Open Bio 7, 1196–1207. doi:10.1002/2211-5463.12267
Wang, W., Zhou, X., Bian, Y., Wang, S., Chai, Q., Guo, Z., et al. (2020). Dual-targeting Nanoparticle Vaccine Elicits a Therapeutic Antibody Response against Chronic Hepatitis B. Nat. Nanotechnol 15, 406–416. doi:10.1038/s41565-020-0648-y
Weilhammer, D., Dunkle, A. D., Blanchette, C. D., Fischer, N. O., Corzett, M., Lehmann, D., et al. (2017). Enhancement of Antigen-specific CD4+ and CD8+ T Cell Responses Using a Self-Assembled Biologic Nanolipoprotein Particle Vaccine. Vaccine 35, 1475–1481. doi:10.1016/j.vaccine.2017.02.004
Weilhammer, D. R., Blanchette, C. D., Fischer, N. O., Alam, S., Loots, G. G., Corzett, M., et al. (2013). The Use of Nanolipoprotein Particles to Enhance the Immunostimulatory Properties of Innate Immune Agonists against Lethal Influenza challenge. Biomaterials 34, 10305–10318. doi:10.1016/j.biomaterials.2013.09.038
Weilhammer, D. R., Dunkle, A. D., Boone, T., Gilmore, S. F., Khemmani, M., Peters, S. K. G., et al. (2020). Characterization of Bacillus Anthracis Spore Proteins Using a Nanoscaffold Vaccine Platform. Front. Immunol. 11, 1264. doi:10.3389/fimmu.2020.01264
Wi, T. E., Ndowa, F. J., Ferreyra, C., Kelly-Cirino, C., Taylor, M. M., Toskin, I., et al. (2019). Diagnosing Sexually Transmitted Infections in Resource-Constrained Settings: Challenges and Ways Forward. J. Int. AIDS Soc. 22 (Suppl. 6), e25343. doi:10.1002/jia2.25343
Workowski, K. A., Berman, S. M., and Douglas, J. M. (2008). Emerging Antimicrobial Resistance in Neisseria Gonorrhoeae: Urgent Need to Strengthen Prevention Strategies. Ann. Intern. Med. 148, 606–613. doi:10.7326/0003-4819-148-8-200804150-00005
Ximba, P., Chapman, R., Meyers, A. E., Margolin, E., van Diepen, M. T., Williamson, A. L., et al. (2020). Characterization and Immunogenicity of HIV Envelope Gp140 Zera® Tagged Antigens. Front. Bioeng. Biotechnol. 8, 321. doi:10.3389/fbioe.2020.00321
Young Kim, H., Young Yum, S., Jang, G., and Ahn, D. R. (2015). Discovery of a Non-cationic Cell Penetrating Peptide Derived from Membrane-Interacting Human Proteins and its Potential as a Protein Delivery Carrier. Sci. Rep. 5, 11719. doi:10.1038/srep11719
Zeltins, A. (2013). Construction and Characterization of Virus-like Particles: a Review. Mol. Biotechnol. 53, 92–107. doi:10.1007/s12033-012-9598-4
Zhao, F., Wu, Y., Zhang, X., Yu, J., Gu, W., Liu, S., et al. (2011). Enhanced Immune Response and Protective Efficacy of a Treponema pallidum Tp92 DNA Vaccine Vectored by Chitosan Nanoparticles and Adjuvanted with IL-2. Hum. Vaccin. 7, 1083–1089. doi:10.4161/hv.7.10.16541
Zhao, F., Zhang, X., Liu, S., Zeng, T., Yu, J., Gu, W., et al. (2013). Assessment of the Immune Responses to Treponema pallidum Gpd DNA Vaccine Adjuvanted with IL-2 and Chitosan Nanoparticles before and after Treponema pallidum challenge in Rabbits. Sci. China Life Sci. 56, 174–180. doi:10.1007/s11427-012-4434-4
Zhu, M., Wang, R., and Nie, G. (2014). Applications of Nanomaterials as Vaccine Adjuvants. Hum. Vaccin. Immunother. 10, 2761–2774. doi:10.4161/hv.29589
Keywords: STIs, vaccines, nanoparticles, delivery platforms, immunogenicity, chlamydia, syphilis, gonorrhea
Citation: Abisoye-Ogunniyan A, Carrano IM, Weilhammer DR, Gilmore SF, Fischer NO, Pal S, de la Maza LM, Coleman MA and Rasley A (2021) A Survey of Preclinical Studies Evaluating Nanoparticle-Based Vaccines Against Non-Viral Sexually Transmitted Infections. Front. Pharmacol. 12:768461. doi: 10.3389/fphar.2021.768461
Received: 31 August 2021; Accepted: 01 November 2021;
Published: 24 November 2021.
Edited by:
Mariusz Skwarczynski, The University of Queensland, AustraliaReviewed by:
Shaomin Tian, University of North Carolina at Chapel Hill, United StatesTimothy Chang, Harvard Medical School, United States
Copyright © 2021 Abisoye-Ogunniyan, Carrano, Weilhammer, Gilmore, Fischer, Pal, de la Maza, Coleman and Rasley. This is an open-access article distributed under the terms of the Creative Commons Attribution License (CC BY). The use, distribution or reproduction in other forums is permitted, provided the original author(s) and the copyright owner(s) are credited and that the original publication in this journal is cited, in accordance with accepted academic practice. No use, distribution or reproduction is permitted which does not comply with these terms.
*Correspondence: Amy Rasley, cmFzbGV5MkBsbG5sLmdvdg==