- 1College of Basic Medical and Sciences, Heilongjiang University of Chinese Medicine, Harbin, China
- 2The Second Affiliated Hospital of Heilongjiang University of Chinese Medicine, Harbin, China
Objective: This study aims to elucidate the mitigating effects of the volatile oil of Acori tatarinowii rhizoma (ATR) on dementia, in order to provide a reference for future research and applications of the volatile oil of ATR in the field of dementia.
Materials and methods: A search strategy was developed using terms such as “Acori tatarinowii rhizoma,” “Acorus tatarinowii Schott,” “Asarone,” and “Dementia.” The literature search was conducted in PubMed, Web of Science, and Google Scholar, and studies not meeting the inclusion criteria were excluded. This study summarizes the main metabolites, active ingredients, toxicological properties, and pharmacokinetic characteristics of the volatile oil from ATR in mitigating dementia, with a particular focus on its potential mechanisms of action. Furthermore, the study highlights the limitations of existing research and offers insights into future research directions.
Results: The volatile oil of ATR mitigates dementia through multiple pathways, including reducing abnormal protein aggregation, promoting neurogenesis, inhibiting neuronal apoptosis, regulating neurotransmitters, improving synaptic function, modulating autophagy, countering cellular stress, reducing neuroinflammation, and alleviating vascular risk factors.
Conclusion: The multi-pathway pharmacological effects of the volatile oil of ATR are well-aligned with the complex mechanisms of dementia progression, highlighting its significant therapeutic potential for anti-dementia applications. This provides new perspectives for the development of more effective anti-dementia drugs. Nonetheless, further rigorous and high-quality preclinical and clinical investigations are required to address key issues, including the chemical characterization of the volatile oil of ATR, potential synergistic effects among active ingredients, toxicity profiles, and definitive clinical efficacy.
1 Introduction
Dementia is a clinical syndrome primarily characterized by progressive cognitive decline. As cognition deteriorates, most individuals with dementia develop psychological and behavioral abnormalities, and may even lose the ability to live independently, imposing substantial physical, mental, and economic burdens on both patients and caregivers (Wang C. et al., 2022). Data indicate that dementia caused a global economic loss of $1.3 trillion in 2019, with global dementia cases surpassing 55 million and increasing at a rate of 10 million new cases every year (WHO, 2023). By 2050, the worldwide number of dementia cases is projected to reach 150 million (Nichols et al., 2022), which will lead to an even greater burden of disease. Despite the already alarming statistics, the destructive impact of dementia may still be underestimated, as it is often misconceived in certain developing countries as an inevitable consequence of aging and, consequently, overlooked (Alladi and Hachinski, 2018). Undoubtedly, dementia has become one of the greatest public health challenges globally.
Based on pathological characteristics, dementia can be classified into several subtypes, including Alzheimer’s disease (AD), vascular dementia (VD), dementia with Lewy bodies (DLB), Frontotemporal dementia (FTD), Parkinson’s disease dementia (PD), and mixed dementias (MD). Numerous hypotheses have been proposed to explain the mechanisms underlying dementia onset and progression, such as the amyloid-beta (Aβ) hypothesis, the microtubule-associated protein tau (Tau) hyperphosphorylation hypothesis, mitochondrial dysfunction theory, neuroinflammation hypothesis, and the cholinergic hypothesis. Current drugs are often developed based on these theories, examples of which include cholinesterase inhibitors, memantine, and Aβ-targeting monoclonal antibodies. Unfortunately, none of these theories appear to independently provide a comprehensive explanation for the pathogenesis of dementia. For instance, the removal of insoluble Aβ plaques has not achieved the anticipated therapeutic effects in alleviating AD symptoms (Li and Selkoe, 2020). Consequently, the limited efficacy of drugs developed solely on the basis of a single hypothesis is unsurprising. Although each dementia subtype exhibits specific pathological characteristics, their boundaries are not always clear, and overlapping features may exist. With ongoing research, increasing evidence suggests that dementia may result from the interplay of multiple pathological mechanisms (Khan et al., 2016), highlighting the urgent global need for more comprehensive prevention and treatment strategies.
An increasing number of studies are exploring the use of medicinal plants or their extracts for mitigating dementia. These natural products may serve as a valuable resource for advancing the development of anti-dementia drugs. Acori tatarinowii rhizoma (ATR), the dried rhizome of Acorus tatarinowii Schott from the family Araceae, is a traditional medicine widely used in Asian countries such as China, Japan, Korea, and India for conditions such as stroke, dementia, epilepsy, insomnia, and gastralgia (Lam et al., 2016a). Research has shown that ATR and its extracts exhibit neuroprotective, anti-dementia, and antidepressant effects (Kim C. J. et al., 2022). Certain traditional medicine formulations containing ATR, such as Kaixinsan (Fu et al., 2019), Qisheng Wan formula (Xiong et al., 2022), and Sagacious Confucius’ Pillow Elixir (Hou et al., 2023), have demonstrated therapeutic effects on cognitive impairment, dementia, and depression. The plant metabolites of ATR include non-volatile metabolites such as alkaloids, flavonoids, and organic acids, as well as volatile metabolites, particularly phenylpropanoids and terpenoids. The volatile metabolites, primarily the volatile oil, are considered the main active ingredients of ATR (Wen et al., 2023). The volatile oil of ATR exhibits antidepressant, anticonvulsant, neuroprotective, and cognition-enhancing properties (Wang et al., 2023). Its main active ingredients can penetrate the blood-brain barrier (BBB) and are widely distributed within the brain (Liu and Fang, 2011; Lu et al., 2014; Balakrishnan et al., 2022; Chellian et al., 2017), forming the basis for its potential efficacy in mitigating dementia.
Given the complex mechanisms underlying dementia and the pharmacological properties of the volatile oil of ATR, this study highlights its potential as a candidate drug for mitigating dementia. Accordingly, the study aimed to elucidate the pharmacological mechanisms of the volatile oil of ATR in mitigating dementia. A comprehensive search strategy was developed using the following terms: “Acori tatarinowii rhizoma,” “Acorus tatarinowii Schott,” “Asarone,” “Dementia,” “Alzheimer’s Disease,” “Vascular Dementia,” “Dementia with Lewy Bodies,” “Frontotemporal Dementia,” “Parkinson’s Disease Dementia,” “cognitive function,” “central nervous system,” “neuroprotection,” “Neuron,” “neurodegeneration,” “pharmacology,” “pharmacokinetics,” and “toxicity.” Searches were conducted in PubMed, Web of Science, and Google Scholar for studies published from the inception of each database through 1 December, 2024. Duplicates, reviews, editorials, abstracts, comments, irrelevant studies, and those that did not meet the ConPhyMP—Guidelines (Heinrich et al., 2022) were excluded by screening titles, abstracts, or full-text reviews, while the remaining studies were included. These studies enhance our understanding of the potential of the volatile oil of ATR in mitigating dementia and provide valuable insights for the development of future anti-dementia drugs.
2 Main active ingredients of the volatile oil of ATR
The volatile oil are the main active ingredients of ATR, serving as crucial indices for its identification and evaluation (Lam et al., 2016b). A variety of metabolites have been identified in the volatile oil of ATR, which can be classified into four main categories: terpenoids, phenylpropanoids, aromatic compounds, and other aliphatic compounds (Yan et al., 2020a). Researchers used analytical testing techniques such as chromatography and GC-MS to analyze the plant metabolites in the volatile oil of ATR derived from different origins and batches (Table 1). While minor variations exist across sources, phenylpropanoids such as α-Asarone and β-Asarone (Figure 1) are consistently the main metabolites of the volatile oil of ATR. These metabolites are not only the characteristic components of the volatile oil of ATR but also the main active ingredients responsible for its dementia-mitigating effects (Wen et al., 2023; Lam et al., 2016b; Lam et al., 2019). Although α-Asarone and β-Asarone are not unique to A. tatarinowii Schott and are also present in other plants, such as Guatteria gaumeri Greenman (Annonaceae), Asarum europaeum Linné (Aristolochiaceae), as well as Acorus calamus Linn and Acorus gramineus Soland (Acoraceae) (Chellian et al., 2017; Uebel et al., 2021). Acorus tatarinowii Schott has emerged as a significant focus in research on dementia mitigation. This prominence is attributable to its high and stable levels of α-Asarone and β-Asarone, its long-standing use in traditional medicine for dementia treatment, and robust support from modern scientific studies (Wen et al., 2023; Wang et al., 2023; Lam et al., 2016b). In contrast, other plants containing these metabolites have limited applications in dementia research or treatment due to their lower metabolite levels, reduced stability, lack of traditional use in dementia therapy, and insufficient preclinical or clinical evidence (Ozarowski, 1956; Lam et al., 2017a; Leclercq et al., 1985; Maseehullah et al., 2022; Hernández et al., 1993; Chamorro et al., 1993). Also based on the aforementioned context, current research on the volatile oil of ATR primarily focuses on its two major active ingredients, while other metabolites are often overlooked due to their lower abundance. Existing studies have demonstrated that the volatile oil of ATR and its main active ingredients exhibit neuroprotective effects through mechanisms involving the reduction of abnormal protein aggregation (Deng et al., 2020), anti-inflammatory activity (Xiao et al., 2019), anti-apoptotic effects (Geng et al., 2010), autophagy-regulating effects (Han et al., 2020), antioxidant effects (Lam et al., 2017b), and regulation of neurotransmitters (Feng et al., 2024). These mechanisms underscore a broad spectrum of actions. This study reviews the pharmacological mechanisms of the volatile oil of ATR and its main active ingredients in the context of dementia mitigation.
3 Pharmacokinetics of the volatile oil of ATR
α-Asarone and β-Asarone, both classified as phenylpropanoids, have structural similarities and exhibit similar pharmacokinetic behavior. After oral administration of the volatile oil of ATR in Wistar rats, the peak plasma concentration (Tmax) of α-Asarone is 1.58 ± 0.19 h, with a half-life (T1/2) of 2.93 ± 0.67 h, while β-Asarone reaches its Tmax at 1.42 ± 0.18 h, with a T1/2 of 2.12 ± 0.22 h (Wang et al., 2014). Direct oral administration of α-Asarone and β-Asarone results in faster absorption and distribution. Studies in Sprague-Dawley (SD) rats showed that after administering an α-Asarone solution orally, Tmax reached 11.67 ± 4.08 min, and the terminal T1/2 was 66.99 ± 29.76 min (Qian et al., 2015). For β-Asarone, oral administration resulted in a Tmax of 12 min and a terminal T1/2 of 54 min (Wu and Fang, 2004). Both α-Asarone and β-Asarone can be quickly absorbed and distributed, but more importantly, they can penetrate the BBB and act on brain regions such as the hypothalamus, hippocampus, brainstem, cortex, and cerebellum (Liu and Fang, 2011; Lu et al., 2014; Balakrishnan et al., 2022; Chellian et al., 2017), forming the pharmacological basis for their dementia mitigation effects. It must be noted, however, that while many studies suggest that ATR extracts or their main active ingredients can cross the BBB and alleviate BBB dysfunction (Li et al., 2025; Gao X. et al., 2022; Luo et al., 2025), some studies also imply that the ability of ATR extracts or their main active ingredients to penetrate the BBB may be associated with an increase in BBB permeability (Wu et al., 2018; Hu et al., 2009). This could potentially raise the likelihood of toxic substances entering the brain, which may pose risks for dementia patients. Such discrepancies highlight the need for further in-depth research into the mechanisms by which the volatile oil of ATR or its main ingredients cross the BBB, in order to determine whether they have potential side effects.
The high lipophilicity of α-Asarone and β-Asarone facilitates their crossing of the BBB, however, it also leads to poor solubility in water, resulting in low oral bioavailability. The bioavailability of α-Asarone tablets in humans is reportedly less than 10% (Chellian et al., 2017; Deng et al., 2017). Research has suggested that intranasal delivery and intravenous injection can significantly enhance both absolute bioavailability and maximum plasma drug concentration. However, solubility promoters in α-Asarone injection solutions have been associated with severe allergic reactions (Deng et al., 2017). Intranasal delivery appears to be a better option, as part of the drug can directly target brain tissues via the nasal cavity, bypassing first-pass metabolism in the liver, thus improving bioavailability, maximum plasma concentration, and drug retention time in the body. Intranasal delivery also reduces high systemic concentrations, minimizing toxic side effects in blood and liver (Lu et al., 2014; Pan et al., 2018).
4 Toxicological studies of the volatile oil of ATR
While ATR is traditionally considered safe for medicinal use, toxicological studies on the volatile oil of ATR and its active ingredients remain necessary and warrant further investigation. Current toxicological research on the volatile oil and its main active ingredients is limited, and results are inconsistent.
In acute toxicity studies, oral administration of up to 5,000 mg/kg of ATR aqueous extract (containing volatile oil) in mice, a dosage far exceeding traditional uses, caused no toxicity or fatalities. In subacute experiments, daily doses of 1,250, 2,500, and 5,000 mg/kg over 28 days also resulted in no toxicity or fatalities, though some mild renal damage was observed in the high-dose group (5,000 mg/kg) (Liu et al., 2024). These findings suggest a high safety margin for clinical use, particularly for short-term, moderate doses, but long-term, high-dose use may carry potential nephrotoxic risks.
Toxicological studies on α-Asarone and β-Asarone are relatively more extensive. Acute toxicity experiments revealed that the median lethal dose (LD50) of oral α-Asarone in mice exceeds 1,000 mg/kg (Chen et al., 2013), while the LD50 for intraperitoneal injection is 245.2 mg/kg (Morales-Ramírez et al., 1992). For β-Asarone, the oral LD50 in rats is 1,010 mg/kg (Food, 2002), and the intravenous LD50 in mice is 1,560 mg/kg (Liu et al., 2013). In subacute toxicity experiments, mice receiving daily oral α-Asarone at doses of 50, 100, and 200 mg/kg for 28 days showed no significant adverse effects, although spontaneous activity decreased in the high-dose group (200 mg/kg) (Chen et al., 2013). Similarly, intraperitoneal injection of 100 mg/kg β-Asarone for five consecutive days in juvenile SD rats caused no toxicity or fatalities (Food, 2002). In chronic toxicity studies, intraperitoneal administration of α-Asarone (≤156 mg/kg) or β-Asarone (≤52 mg/kg) in juvenile mice increased the incidence of hepatocellular adenomas and carcinomas at 10–13 months compared to controls (KIM et al., 1999; Wiseman et al., 1987). However, a 30-day course of intraperitoneal injections of α-Asarone (9 mg/kg) in rats did not cause liver morphological changes (Manikandan and Devi, 2005). Oral administration of β-Asarone (200 μg/kg) in mice for 20 weeks caused no toxicity, while daily oral doses of 10, 20, or 50 mg/kg for 90 days caused abnormal blood parameters only at the highest dose (50 mg/kg) (Liu et al., 2013).
Certain bacterial reverse mutation assays showed that α-Asarone and β-Asarone exhibited no mutagenicity in Salmonella typhimurium strains (TA98, TA102, TA1535, TA1537, TA1538), regardless of metabolic activation. However, mutagenicity was observed in TA100 with metabolic activation (Marczewska et al., 2013; Berg et al., 2016; Cassani-Galindo et al., 2005; Göggelmann and Schimmer, 1983). Additionally, while 3′-hydroxylated metabolites of α-Asarone and β-Asarone showed no mutagenicity in human sulphotransferase-expressing Salmonella strains, β-Asarone’s side-chain epoxide metabolite demonstrated mutagenicity (Berg et al., 2016). Embryotoxicity studies revealed no teratogenic effects of α-Asarone in pregnant rats (Jimenez et al., 1988). However, in mice, α-Asarone showed embryotoxicity (15–60 mg/kg) and maternal toxicity (60 mg/kg), with potential teratogenic effects (Salazar et al., 1992). Short-term exposure to α-Asarone caused reproductive toxicity, such as reduced sperm concentration, motility, and seminal vesicle weight in CF1 mice at high doses (30 mg/kg intraperitoneally for 5 days) (Chamorro et al., 1998). However, long-term subacute toxicity studies (10 and 20 mg/kg, orally, 5 days per week for eight consecutive weeks) showed no significant effects on sperm count, testicular tissue morphology, testicular weight, or epididymal weight (Chamorro et al., 1999). Debates persist regarding the genotoxicity of α-Asarone and β-Asarone. Oral administration of α-Asarone at a dosage of 50 mg/kg does not influence the formation of micronuclei in the peripheral blood reticulocytes of normal mice (Sandeep and Nair, 2011), and injection of β-Asarone at a dosage of 182.4 mg/kg also shows no effect on the formation of micronuclei in polychromatic erythrocytes within the mouse bone marrow (Ashadevi et al., 2014). These findings suggest that the tested doses of α-Asarone and β-Asarone do not possess significant genotoxic effects. However, α-Asarone caused DNA damage in human lymphocytes (Morales-Ramírez et al., 1992), rat hepatocytes (Hasheminejad and Caldwell, 1994), and L929 fibroblast cells (Marczewska et al., 2013), indicating genotoxicity, while β-Asarone exhibited genotoxicity in Escherichia coli (Kevekordes et al., 1999), rat hepatocytes (Hasheminejad and Caldwell, 1994), hamster pulmonary fibroblasts (Haupenthal et al., 2015), HepG-2 cells, and human lymphocytes (Kevekordes et al., 2001).
In summary, while existing toxicity endpoints suggest a sufficient safety margin for regular therapeutic use of the volatile oil of ATR and its main active ingredients, more comprehensive and systematic toxicological studies are needed. These studies should conform to internationally recognized testing guidelines, such as OECD test guidelines, to better evaluate the safety profile of these plant metabolites.
5 Pharmacological effects of the volatile oil of ATR in mitigating dementia
Current evidence indicates that the volatile oil of ATR effectively mitigate dementia. Research primarily focuses on α-Asarone and β-Asarone, examining their intervention in the pathogenesis of dementia. Key mechanisms include reducing abnormal protein aggregation, promoting neurogenesis, inhibiting neuronal apoptosis, modulating neurotransmitters, improving synaptic function, regulating autophagy, mitigating cellular stress, inhibiting neuroinflammation, and mitigating vascular risk factors (Table 2; Figure 2).
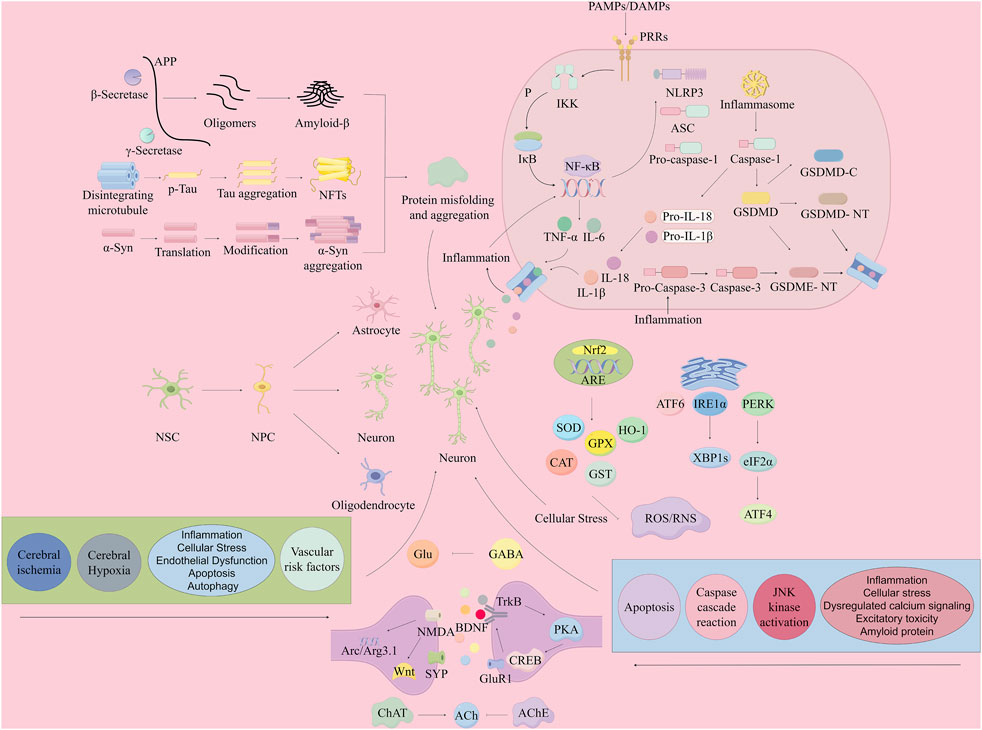
Figure 2. Mechanisms of neuronal damage in dementia (mitigation by the volatile oil of ATR). By Figdraw. Note: APP, Amyloid Precursor Protein; Aβ, Amyloid-β; NFTs, Neurofibrillary Tangles; NSC, Neural Stem Cells; NPC, Neural Progenitor Cells; PAMPs, Pathogen-Associated Molecular Patterns; DAMPs, Damage-Associated Molecular Patterns; PRRs, Pattern Recognition Receptors; IKK, Inhibitor of Kappa light polypeptide gene enhancer in B-cells Kinase; NF-κB, Nuclear Factor kappa-light-chain-enhancer of activated B cells; TNF-α, Tumor Necrosis Factor-alpha; IL-1β, Interleukin 1 beta; IL-18, Interleukin 18; IL-6, Interleukin 6; ASC, Apoptosis-associated speck-like protein containing a CARD; NLRP3, Nucleotide-binding oligomerization domain-like receptor family pyrin domain containing 3; GSDMD, Gasdermin D; GSDME, Gasdermin E; GSDMD-C, Gasdermin D C-terminal; GSDMD-NT, Gasdermin D N-terminal; GSDME-NT, Gasdermin E N-terminal; Caspase, Cysteinyl aspartate specific protease; Caspase-1, Cysteinyl aspartate specific protease 1; Caspase-3, Cysteinyl aspartate specific protease 3; PERK, Protein Kinase R-like endoplasmic reticulum kinase; ATF, Activating Transcription Factor; XBP1s, X-box binding protein 1 splicing; ROS/RNS, Reactive Oxygen Species/Reactive Nitrogen Species; SOD, Superoxide Dismutase; CAT, Catalase; CREB, cAMP Response Element-binding Protein; BDNF, Brain-Derived Neurotrophic Factor; ACh, Acetylcholine; NMDA, N-methyl-D-aspartate; PKA, Protein Kinase A; CaMKII, Calcium/calmodulin-dependent protein kinase II; ChAT, Choline Acetyltransferase.
5.1 Alleviating learning and memory impairments
Learning and memory impairments are pervasive throughout the progression of dementia, with mild cognitive impairment (MCI) serving as a precursor and risk state for dementia (Breton et al., 2019; Gauthier et al., 2006). MCI is characterized by declines in memory, attention, and cognitive abilities beyond what is expected for age and education level (Eshkoor et al., 2015). As a transitional phase, MCI offers a critical window for early intervention to reduce the risk of dementia onset. Learning and memory impairments are associated with pathological factors such as Aβ deposition, tau hyperphosphorylation, neurotransmitter imbalances, excitotoxicity, neuroinflammation, and aberrant neurotrophic signaling. Addressing these impairments is thus of significant importance for preventing and treating dementia.
Studies conducted in elderly rats with cognitive deficits have demonstrated the ability of α-Asarone to lower hippocampal Aβ1-42 levels, restore balance between the glutamatergic and GABAergic systems, alleviate excitotoxicity, and improve spatial learning and memory in the Morris water maze (Chen Y. et al., 2020). Similarly, in ethanol-induced learning and memory impairment models in mice, α-Asarone corrected the imbalance of glutamatergic and GABAergic systems and improved the object recognition index in a novel object recognition task (Li et al., 2019). In 3-month-old APP/PS1 transgenic mice, α-Asarone significantly ameliorated learning and memory deficits by reducing Aβ42, p-tau, and inflammatory factor levels (Zeng et al., 2021). β-Asarone has shown a potent neuroprotective effect in corticosterone-induced learning and memory impairment models by upregulating BDNF, CREB, and Bcl-2 expression while downregulating Bax, thereby improving cognitive deficits through neurotrophic support and inhibition of neuronal apoptosis (Lee et al., 2015). Synaptic function, a critical determinant of neuronal connectivity strength, plays an essential role in learning and memory (Khaspekov and Frumkina, 2023). β-Asarone promotes synaptic plasticity and synaptogenesis in lead-exposed rats, mitigating learning and memory impairments (Yang QQ. et al., 2016). Additionally, α-Asarone and β-Asarone have been shown to promote hippocampal neurogenesis in both healthy and impaired models, with neurogenesis closely linked to learning and memory enhancement (Mao et al., 2015). These findings highlight the potential of α-Asarone and β-Asarone to improve learning and memory during the MCI phase, thereby reducing the risk of dementia. Even in dementia stages, the volatile oil of ATR have been shown to alleviate such impairments. For instance, aqueous extracts of ATR decreased p-Tau aggregation and repaired myelin damage in the hippocampus of AD mice, significantly improving cognitive abilities (Fu et al., 2020), while β-Asarone improved synaptic plasticity and alleviated cognitive deficits in AD mice (Liu et al., 2016). These findings, across various animal models, collectively demonstrate that the volatile oil of ATR utilize multifaceted mechanisms to enhance learning and memory, underscoring their therapeutic potential for dementia.
5.2 Reducing abnormal protein aggregation
The Aβ deposition hypothesis is widely recognized as a central pathological mechanism in dementia. Aβ not only serves as a hallmark of AD but also plays a role in the pathogenesis of other dementia subtypes, such as VD, PD, and DLB (Sengupta and Kayed, 2022). Aβ is generated by sequential proteolysis of amyloid precursor protein (APP) by β-secretase and γ-secretase, resulting in 39–43 amino acid-long peptides, with Aβ1-40 and Aβ1-42 being the primary subtypes (Schwartz and Ziv, 2008; Ziv and Schwartz, 2008). The production of Aβ is related to APP, β-secretase and γ-secretase (Yang G. et al., 2021), and Aβ clearance occurs through enzymatic degradation, glial cell activity, or autophagy (Cai et al., 2023; Leissring, 2016). Dysfunction in the balance of Aβ production and clearance leads to its accumulation (CAI et al., 2023), transitioning from a soluble monomeric state to insoluble amyloid fibrils (Mattson, 2004; Orgogozo et al., 2003). Aβ deposition causes oxidative stress, damages neuronal cells (Koenig and Meyerhoff, 2003), activates glial cells to trigger inflammation (PHILLIPS et al., 2014; Franco-Bocanegra et al., 2019), and promotes tau hyperphosphorylation (Canudas et al., 2005). Tau is a microtubule-associated protein, mainly found in neurons of the central nervous system, and its main function is to bind to tubulin, promote the assembly and stability of microtubules, and thus maintain the structure and function of the cytoskeleton (Kosik, 1990). Once hyperphosphorylated, tau loses its ability to bind microtubules for cytoskeletal stability, forming intracellular neurofibrillary tangles (NFTs), which disrupt neuronal structure and function and contribute to the pathogenesis of AD, PD, VD, and other dementia subtypes (Sun et al., 2021; Li C. et al., 2024; Pan et al., 2022). α-Synuclein, a presynaptic neuronal protein, is highly enriched in presynaptic nerve endings and is associated with a variety of neurodegenerative diseases (Bennett, 2005). Abnormal aggregation of α-Synuclein is believed to lead to neuronal death, and is also a pathological marker of PD and DLB (Calabresi et al., 2023).
Aqueous extracts of ATR reduced p-Tau aggregation and repaired myelin damage in 3×Tg-AD mice, thereby improving cognitive function (Fu et al., 2020). Myelin sheath is a multilayer lipid and protein composite membrane structure wrapped outside the axon of neurons (Stassart et al., 2018), which can promote the efficiency of action potential conduction and the integration of neural networks, and is a key element in maintaining cognitive function (Chen X. et al., 2020). Recent studies have found that myelin damage may be a driving factor for Aβ deposition (Depp et al., 2023), so the extracts of ATR may alleviate Aβ deposition by reducing myelin damage. Neuronal hyperexcitability may promote the hydrolysis of APP (Canter et al., 2016). Gamma-Aminobutyric Acid (GABA) is the predominant inhibitory neurotransmitter in the central nervous system. α-Asarone can alleviate neuronal hyperexcitability by modulating GABA receptors, thereby mitigating APP hydrolysis and reducing Aβ formation (Chen Y. et al., 2020). Further studies have shown that α-Asarone can reduce neuroinflammation and restore autophagic flux, thereby decreasing Aβ1-42 and p-Tau aggregation in the hippocampus of APP/PS1 mice (Zeng et al., 2021). β-Asarone can reduce levels of Aβ40, Aβ42, and APP in the hippocampus of APP/PS1 mice, thereby mitigating Aβ deposition and inhibiting excessive autophagy. However, the relationship between the two phenomena requires further investigation (Deng et al., 2020). The receptor of advanced glycation end products (RAGE) is a cell surface receptor that can bind to Aβ. High RAGE expression enhances β-secretase activity, thereby increasing Aβ production. The signaling cascades triggered by their interaction are a critical mechanism by which Aβ contributes to AD (Chen et al., 2007). β-Asarone alleviates Aβ1-42 levels and Aβ plaque deposition in the cortex and hippocampus of APP/PS1 mice by reducing RAGE levels (Yang C. et al., 2016). β-Asarone reduces α-Synuclein expression levels in the brain tissue of PD mice, which has also been validated in vitro. This effect may be associated with the regulation of the long non-coding RNA MALAT1 (Zhang Q. S. et al., 2016). Studies have also indicated that abnormal calcium mobilization within cells may accelerate Aβ formation (Querfurth et al., 1997; Moreno-Ortega et al., 2015). Both α-Asarone and β-Asarone can inhibit Ca2+ uptake in PC-12 cells and reduce the cytotoxic effects of Aβ on these cells (Irie and Keung, 2003).
5.3 Promoting neurogenesis
Neurogenesis occurs throughout a human’s lifetime, with adult neurogenesis referring to the production of new neurons that are integrated into existing neural circuits of the adult brain. Decline in neurogenesis is closely associated with learning and memory impairments and is a critical factor in the onset and progression of dementia. Neurogenesis contributes to rejuvenating the nervous system and maintaining its functional integrity (Kim T. A. et al., 2022; Isaev et al., 2019). Neurogenesis relies on neural stem cells (NSCs), which are a class of stem cells capable of self-renewal and multipotent differentiation. NSCs can differentiate into three main cell types in the central nervous system: neurons, astrocytes, and oligodendrocytes. They play roles in neuronal nutrition, promoting neuroregeneration, and immune regulation. When neurons are damaged or degenerate, quiescent NSCs in the body are activated, proliferate, migrate to the damaged area, and differentiate, thereby repairing the injured nervous system (Chen et al., 2018). Slowly self-renewing NSCs produce neural progenitor cells (NPCs), which exhibit a faster cell division cycle and eventually differentiate into neurons or glial cells (Huang and Zhang, 2019; Vasic et al., 2019). Extensive neuronal loss is one of the main pathological features of dementia. Enhancing neurogenesis may help replenish deficient neurons in dementia patients, promote the secretion of neurotrophic factors, improve the microenvironment for neuronal survival, and increase neuronal survival rate, thus serving as a potential strategy for the mitigation of dementia (Roda et al., 2022; Zheng, 2022).
NPCs represent an intermediate stage in NSCs differentiation and possess both self-renewal capacities and multipotent differentiation potentials, making them critical for neurogenesis (Zhao et al., 2008). Reduction in NPCs proliferation and renewal may also be a significant contributor to cognitive impairment (Haughey et al., 2002; Seib et al., 2013). Ethanol extracts of ATR, along with its major active ingredients α-Asarone and β-Asarone, have been shown to enhance NPCs proliferation and neurogenesis in the hippocampi of healthy mice, aged mice, and APP/PS1 transgenic mice. In vitro studies further support these findings (Mao et al., 2015). NPCs proliferation is regulated by neurotrophic factors, which are a class of proteins that provide nutritional support to neurons. Adequate levels of neurotrophic factors are beneficial for neurogenesis and play key roles in maintaining nervous system function and facilitating injury repair. Numerous neurodegenerative diseases, including dementia, are closely linked to alterations in the expression of neurotrophic factors and their receptors. For instance, levels of brain-derived neurotrophic factor (BDNF), nerve growth factor (NGF), and glial cell line-derived neurotrophic factor (GDNF) are reduced in AD, FTD, PD, LBD, and VD (Mitra et al., 2021; Biane et al., 2014; Ventriglia et al., 2013). Therefore, increasing neurotrophic factor levels may be an effective strategy to restore neuronal function. α-Asarone and β-Asarone may stimulate the expression and secretion of BDNF, NGF, and GDNF by modulating the PKA/ERK/CREB pathway (Lam et al., 2019), thereby maintaining and repairing nervous system function. Another study indicates that β-Asarone might regulate BDNF levels by modulating ERK pathway activity through MKP-1 expression (Sun et al., 2015).
5.4 Inhibiting neuronal apoptosis
Apoptosis is a form of programmed cell death that occurs under physiological conditions. It is a process of active cellular death that plays a vital role in regulating development, cell differentiation, and the renewal of normal cells within the body. In the progression of dementia, apoptosis interacts with various pathogenic hypotheses, such as Aβ deposition-induced neuronal damage, oxidative stress, excitotoxicity, and inflammation (Erekat, 2022). A critical issue is that apoptosis leads to neuronal loss, which is a significant cause of dementia (Liang et al., 2024). Apoptosis is regulated through multiple pathways, and the volatile oil of ATR primarily exert their anti-apoptotic effects by modulating microRNA (miRNA), the c-Jun N-terminal kinase (JNK) pathway, and calcium homeostasis. miRNAs are a class of endogenous, non-coding single-stranded RNA molecules that regulate gene expression by binding to the 3′-untranslated region (3′-UTR) of target mRNAs. Evidence suggests that miRNAs contribute to the development and progression of dementia by regulating synaptic function, inflammatory factors, and oxidative stress (Song and Kim, 2017; Zhou et al., 2017; Zhang et al., 2018). JNK plays a crucial role in apoptosis-related gene activation, expression, and regulation. For instance, JNK signaling regulates the expression of members of the B-cell lymphoma 2 (Bcl-2) family, which are key players in apoptosis (Rajesh and Kanneganti, 2022). Activation of JNK can trigger a caspase cascade, wherein executioner caspases recognize and cleave specific substrates to execute apoptosis (Fan et al., 2005). Calcium homeostasis refers to the balance of Ca2+ concentration within and outside cells. Ca2+ is one of the most critical intracellular signaling molecules and regulates apoptosis by influencing endoplasmic reticulum and mitochondrial function as well as interactions with Bcl-2 family proteins (Pinton et al., 2008).
miR-873-5p is a microRNA located on human chromosome 9p21.1. Its target genes are closely associated with apoptosis (LIU et al., 2015). For example, miR-873-5p directly targets the 3′-UTR of heme oxygenase-1 (HMOX1) mRNA, thereby regulating HMOX1 expression. HMOX1 is a stress response enzyme closely linked to apoptosis and cognitive impairment (Cressatti et al., 2019). Aqueous extracts of ATR can promote miR-873-5p expression, suppress HMOX1 expression, and reduce apoptosis (Shi et al., 2018). Vascular injuries are major risk factors for dementia. Ischemic damage triggers excessive glutamate release, resulting in Ca2+ overload, which consequently leads to abnormal phosphorylation of calcium/calmodulin-dependent protein kinase II (CaMKII) and ultimately neuronal apoptosis and damage (Coultrap et al., 2011). α-Asarone restores the balance of excitatory and inhibitory signals, reduces calcium overload and CaMKII phosphorylation, and inhibits apoptosis, thereby preventing early brain injury following subarachnoid hemorrhage (Gao et al., 2024). CaMKII can phosphorylate critical sites of CREB (Yan et al., 2016), and CREB phosphorylation activates Bcl-2 (Lonze and Ginty, 2002). β-Asarone enhances the expression of CaMKII-α, p-CREB, and Bcl-2, indicating its ability to inhibit neuronal apoptosis via the activation of the CaMKII-α/p-CREB/Bcl-2 pathway (Wei et al., 2013). Apoptosis signal-regulating kinase 1 (ASK1) can be activated by various stress signals and plays a pivotal role in apoptosis. Activated ASK1 subsequently activates mitogen-activated protein kinase kinase 7 (MKK7), which regulates numerous fundamental physiological processes. Under specific stimuli, MKK7 primarily activates JNK (KANG et al., 2024), as previously discussed. Activation of the JNK pathway can promote apoptosis and is closely linked to the onset and progression of dementia. In vivo and in vitro studies demonstrate that β-Asarone can modulate the ASK1/MKK7/JNK pathway, inhibit the expression of BCL-2-associated X protein (Bax) and Bcl-2 antagonist/killer 1 (Bad), and block the caspase cascade, thereby suppressing apoptosis and protecting neurons (Geng et al., 2010; Zou et al., 2011; Liu et al., 2010; Li et al., 2010).
5.5 Modulating neurotransmitters
Neurotransmitters are synthesized and released by presynaptic neurons into the synaptic cleft, where they bind to specific receptors on postsynaptic neurons to modulate their electrical potentials, enabling the transmission of neural signals. Abnormalities in neurotransmission are closely associated with the onset and progression of dementia (Yang et al., 2023). Impairments in the cholinergic system are considered a key factor in learning and memory decline and cognitive dysfunction, forming the basis of the earliest hypothesis regarding the pathogenesis of AD. Severe cholinergic system degeneration in AD patients leads to reduced acetylcholine (ACh) levels, culminating in dementia symptoms (Chen Z. R. et al., 2022). The current first-line drugs for treating dementia, acetylcholinesterase (AChE) inhibitors, are designed based on the cholinergic hypothesis and work by increasing ACh levels in the synaptic cleft to improve cognitive function (Krall et al., 1999). Other neurotransmitters, such as dopamine (DA), glutamic acid (Glu), gamma-aminobutyric acid (GABA), and 5-hydroxytryptamine (5-HT), are also closely associated with learning and memory processes. Their dysfunction contributes to the onset and progression of various dementia subtypes (Duda, 2004; Huey et al., 2006). Research into the role of the volatile oil of ATR in neurotransmitter regulation has primarily focused on these aspects.
ACh is a vital neurotransmitter in the central cholinergic system, and reduced ACh levels are closely linked to the pathogenesis of dementia (Chen Z. R. et al., 2022). AChE is a highly efficient hydrolytic enzyme that catalyzes ACh breakdown into choline and acetate, thereby regulating ACh concentration, whereas choline acetyltransferase (ChAT) is a key enzyme for ACh synthesis. Together, AChE and ChAT maintain the balance of ACh, which is essential for learning and memory (Yang X. X. et al., 2021). α-Asarone reduces AChE activity in the cortex, hippocampus, and striatum of rats, thereby inhibiting ACh hydrolysis, increasing ACh levels, and improving learning and memory function (Venkatesan, 2022). Glu is an excitatory neurotransmitter, while GABA is an inhibitory neurotransmitter. An imbalance between the two may lead to cognitive impairments (Zeydan et al., 2017; Cui et al., 2015). α-Asarone reduces Glu release, improves Glu transport, modulates the overexpression of Glu receptors, and restores the balance between Glu and GABA, thus enhancing cognitive abilities (LI et al., 2019). DA and 5-HT are critical neurotransmitters involved in learning and memory processes, and reductions in DA and 5-HT levels can lead to cognitive impairments (Speranza et al., 2021; Afshar et al., 2019). β-Asarone increases levels of DA metabolites, such as homovanillic acid (HVA) and 3,4-dihydroxyphenylacetic acid (DOPAC), and 5-HT metabolites, such as 5-hydroxyindoleacetic acid (5-HIAA), in the brains of Parkinsonian rats. It also elevates the levels of tyrosine hydroxylase (TH), a key enzyme in the dopamine synthesis pathway (Zhang S. et al., 2016).
5.6 Improving synaptic function
Synapses are key hubs for inter-neuronal communication, consisting of the presynaptic membrane, synaptic cleft, and postsynaptic membrane, through which neural information is transmitted sequentially. Synaptic dysfunction is closely associated with numerous neurological disorders. Synaptic impairments, such as synapse loss, dendritic spine damage, and reduced neurotransmission, are often observed in subtypes of dementia, including AD, PD, and VD (Che et al., 2023; Merino-Galán et al., 2022; Piscopo et al., 2022). The number of neurotransmitter receptors and their spatial organization at postsynaptic sites underlie synaptic functions and mediate forms of synaptic plasticity (Groc and Choquet, 2020). Furthermore, neurotrophic factors, especially BDNF, significantly influence synaptic function and structure, playing a crucial role in regulating changes in synaptic structure and functionality (Wang C. S. et al., 2022). Therefore, studies on the improvement of synaptic function by the volatile oil of ATR predominantly focus on these areas.
Ischemic brain injury activates NR2B, leading to intracellular Ca2+ influx and subsequent autophosphorylation of CaMKII (Meng et al., 2003). Phosphorylated CaMKII activates cAMP Response Element-Binding Protein (CREB), subsequently promoting BDNF expression. BDNF binds to TrkB receptors, triggering downstream signaling pathways essential for synaptic plasticity regulation (Zhang et al., 2023). α-Asarone enhances the interaction between NR2B and CaMKII, activating the CREB/BDNF/TrkB signaling pathway, thereby increasing hippocampal synapse density and plasticity (Gao et al., 2024). Synaptophysin (SYP), a membrane protein involved in synaptic vesicle fusion and a major component of the presynaptic membrane, correlates closely with cognitive function (Harrill et al., 2015; Xiao et al., 2014). SYP reflects synaptic density and distribution (Sarnat and Born, 1999), and it is thought to enhance neuronal plasticity by influencing synaptic structure and mediating neurotransmitter release through phosphorylation (Chan et al., 2002). Glutamate Receptors (GluRs) mediate excitatory synaptic transmission in the central nervous system, are associated with learning and memory, and play an essential role in synaptic plasticity (Sanderson et al., 2008). SYP primarily facilitates presynaptic neurotransmitter release, while GluR1 predominantly functions at the postsynaptic membrane, mediating signal reception and transmission. Together, they synergistically regulate synaptic strength and structure, impacting learning and memory processes. β-Asarone has been shown to upregulate the expression of SYP and GluR1, thereby modulating synaptic plasticity and mitigating Aβ-mediated neuronal damage (Liu et al., 2016). cAMP (cyclic adenosine monophosphate) is synthesized by Adenylate Cyclase (AC) from ATP. cAMP binds to the regulatory subunits of Protein Kinase A (PKA), releasing the catalytic subunits, which become activated. The activated PKA stimulates the phosphorylation of CREB, a critical transcription factor, which modulates the transcription of downstream proteins such as BDNF to improve synaptic function (Chandía-Cristi et al., 2023). β-Asarone inhibits excessive autophagy, reduces organelle degradation, and enhances mitochondrial energy production, activating the cAMP/PKA/CREB signaling pathway to ameliorate synaptic dysfunction in a mouse model of vascular dementia (Ning et al., 2024). Dendritic spines, primary sites for synaptic contacts, are crucial for receiving convergent synaptic input from other neurons. Abnormalities in dendritic spine morphology and density can contribute to synaptic dysfunction (Rochefort and Konnerth, 2012). Dendritic spine density decreases with aging, and dendritic spine loss is common among dementia patients (Mijalkov et al., 2021). β-Asarone has been observed to restore dendritic spine density in the hippocampal CA1 and DG regions of lead-exposure-induced rats. This is accompanied by increased expression of NR2B, Arc/Arg3.1, and Wnt7a proteins, leading to improved synaptic function (Yang Q. Q. et al., 2016). Among these, NR2B, a subunit of NMDA receptors, is critical for synaptic plasticity and learning and memory processes. Increased NR2B expression enhances synaptic function (Zhuo, 2024). Arc/Arg3.1 is an immediate early gene associated with long-term potentiation (LTP). NMDA receptor activation upregulates Arc/Arg3.1 levels (Messaoudi et al., 2007), while it also triggers Wnt activation within the hippocampus. Wnt promotes presynaptic assembly (Ahmad-Annuar et al., 2006), and Wnt7a, a crucial protein within the Wnt signaling pathway, facilitates synaptic formation and functionality through mechanisms like synapsin1 aggregation and increased miniature excitatory postsynaptic current (mEPSC) frequency (Cerpa et al., 2008).
5.7 Regulating autophagy
Autophagy is one of the principal mechanisms maintaining intracellular homeostasis, degrading and recycling damaged or unnecessary components to sustain cellular balance. Dysregulated autophagy has been implicated in the onset and progression of dementia. Impairment of autophagic function reduces the clearance of pathological proteins such as Aβ, p-Tau, and α-synuclein, leading to their aggregation, cytotoxicity, and eventual cell death (Salminen et al., 2013; Winslow and Rubinsztein, 2011). Excessive autophagy, however, is equally detrimental. As disease progresses and abnormal protein aggregates accumulate, sustained activation of autophagy can result in autophagic cell death, exacerbating neuronal loss (Han et al., 2015). The PI3K-Akt-mTOR signaling pathway is central to autophagy regulation. Phosphatidylinositol-3-Hydroxykinase (PI3K) catalyzes phosphatidylinositol phosphorylation to produce Phosphatidylinositol-3,4,5-Triphosphate (PIP3), which accumulates at the cell membrane to recruit and activate Protein Kinase B (Akt). Akt directly phosphorylates and activates mTORC1 (Hou et al., 2018), a key regulator of autophagy-related proteins (Wang and Zhang, 2019). The interaction between Bcl-2 and Beclin-1 is another critical regulatory mechanism. Under physiological conditions, Bcl-2 suppresses autophagy by inhibiting Beclin-1 (Decuypere et al., 2012). Beclin-1 initiates autophagosome formation and recruits other autophagy-related proteins to autophagic vesicles (Wang et al., 2017), making its expression a marker for autophagy activation or inhibition. LC3 and p62 are also considered specific autophagy markers. Microtubule-Associated Protein 1A/1 B-Light Chain 3 (LC3) is intimately involved in autophagosome formation. When catalyzed by Atg4, LC3-I is covalently linked to phosphatidylethanolamine (PE) to form LC3-II, which integrates into autophagosome membranes. Thus, LC3-II levels or the LC3-II/LC3-I ratio reflect autophagy activity and autophagosome quantity (Agrotis et al., 2019). Sequestosome 1(p62), a selective autophagic substrate, bridges ubiquitinated proteins targeted for degradation with LC3 and is similarly indicative of autophagy function (Zhang et al., 2019). Mitophagy—a selective form of autophagy targeting damaged mitochondria—is particularly relevant in AD, where mitochondrial dysfunction contributes to Aβ and Tau pathology, while Aβ and Tau in turn exacerbate mitochondrial dysfunction (Fang et al., 2019). Research on the volatile oil of ATR has predominantly focused on these mechanisms.
PINK1, a serine/threonine kinase localized to depolarized mitochondria, and Parkin, an E3 ubiquitin ligase, are key mediators of the PINK1-Parkin pathway, which facilitates mitophagy (Vincow et al., 2013). β-Asarone significantly increases PINK1 and Parkin expression in the hippocampus of AD rats, reducing Aβ deposition by enhancing mitophagy through the PINK1-Parkin pathway (Han et al., 2020). In vitro studies corroborate this, demonstrating that β-Asarone upregulates LC3, Beclin-1, PINK1, and Parkin, while downregulating p62 expression in PC12 cells within AD models, increasing autophagic flux and mitigating Aβ aggregation (Wang et al., 2019). Notably, while autophagosome quantity is traditionally used to assess autophagic activity, it reflects both formation and degradation processes. Impaired autophagosome clearance can lead to increased autophagosome counts, whereas enhanced autophagic flux can reduce their numbers. One in vivo study suggests that α-Asarone reduces autophagosome quantities in the hippocampi of APP/PS1 mice, restoring autophagic flux and enhancing autophagy activity, thereby reducing abnormal protein aggregates (Zeng et al., 2021). Another in vitro study demonstrated that Aβ inhibits autophagic flux by disrupting autophagosome-lysosome fusion or impairing lysosomal function. β-Asarone restores autophagic flux by reducing autophagosome numbers and increasing autolysosome counts, enhancing autophagosome-lysosome fusion or lysosomal functionality (Li et al., 2021).
Importantly, autophagy does not universally benefit dementia progression. Prolonged autophagy activation may induce neuronal death. α-Asarone lowers LC3-II/LC3-I ratios and upregulates p62 expression, attenuating ischemia-induced excessive autophagy in the rat cortex and protecting neurons (Zhang K. et al., 2021). β-Asarone reduces Beclin-1 and LC3 expression while upregulating p62 in the hippocampi of AD mice (Deng et al., 2020), exhibiting similar effects in vascular dementia models (Ning et al., 2024). Impairments in the PI3K-Akt-mTOR pathway, observed in dementia patients, exacerbate excessive autophagic activation (Rickle et al., 2004). β-Asarone activates the PI3K-Akt-mTOR pathway to inhibit α-synuclein overexpression-induced excessive autophagy in the striatum of Parkinson’s disease model rats (Wang et al., 2024). In vitro, β-Asarone similarly reduces Aβ-triggered autophagic overactivation via PI3K-Akt-mTOR pathway activation (Xue et al., 2014). Regulating the interaction between Bcl-2 and Beclin-1 is also pivotal. One in vitro study demonstrated that β-Asarone mitigates Aβ25-35-induced excessive autophagy via the Bcl-2/Beclin-1 pathway (Chang and Teng, 2015). Additionally, JNK activation downregulates Bcl-2, disrupting its interaction with Beclin-1, thereby promoting autophagy (Trenti et al., 2014). β-Asarone modulates the JNK/Bcl-2/Beclin-1 pathway, reducing JNK and p-JNK expression while increasing Bcl-2 and Beclin-1 levels in the striatum of 6-OHDA-induced Parkinson’s model rats, attenuating excessive autophagy (Zhang S. et al., 2016). Furthermore, misfolded protein accumulation induces endoplasmic reticulum stress (ER stress), activating the Unfolded Protein Response (UPR). While UPR attempts to restore normal ER function, it can also lead to excessive autophagy (Senft and Ronai, 2015), a process common in dementia pathology (Ajoolabady et al., 2022). β-Asarone inhibits the PERK/CHOP/Bcl-2/Beclin-1 pathway to alleviate ER stress and excessive autophagy in Parkinson’s disease rat models, reducing PERK phosphorylation, CHOP expression, and Beclin-1 release, while increasing Bcl-2 levels to inhibit autophagy (Ning et al., 2019).
5.8 Mitigating cellular stress
Oxidative stress, characterized by an imbalance between oxidant and antioxidant systems due to excessive accumulation of reactive oxygen species (ROS) and impaired antioxidant defenses, represents a deleterious consequence of free radical production often implicated in aging and disease (Chen and Zhong, 2014). Under physiological conditions, ROS are maintained at low levels and act as signaling molecules to stimulate cell growth. However, under pathological conditions, excessive ROS accumulation can overwhelm antioxidant defenses, resulting in oxidative stress and cellular damage (Halliwell, 2006). The brain, being a high metabolic organ with high oxygen consumption, generates substantial ROS during metabolism. Furthermore, its abundance of unsaturated fatty acids renders it prone to oxidative damage, as they easily undergo lipid peroxidation in the presence of ROS (Radak et al., 2011). Consequently, oxidative stress is closely associated with the onset and progression of dementia. For instance, abnormal proteins like Aβ can induce oxidative stress by affecting mitochondrial function and metal ion homeostasis (Pagani and Eckert, 2011; Sohrabi et al., 2023; Huang et al., 1999). Both acute and chronic hypoxia have been found to mediate oxidative stress (Snyder et al., 2017). In conclusion, oxidative stress represents an early pathological event in the brains of dementia patients, where increased levels of lipid peroxidation products and decreased antioxidant enzyme activities are often observed (Chen J. et al., 2022; Atiq et al., 2023). Endoplasmic reticulum (ER) stress is another pivotal factor in cellular stress that is closely associated with the pathogenesis of dementia. ER stress arises when protein folding or modification in the ER becomes dysregulated, triggering an unfolded protein response (UPR). The UPR, a primary adaptive mechanism against ER stress, is mediated by three major signaling pathways involving the ER stress sensors IRE1α, PERK, and ATF6. Under normal conditions, these sensors are found bound to the ER chaperone GRP78, maintaining them in an inactive state (Todd et al., 2008). Upon accumulation of misfolded or unfolded proteins, GRP78 preferentially binds to the abnormal proteins and dissociates from PERK, ATF6, and IRE1α, leading to the activation of these ER stress sensors (Hotamisligil, 2010). Mirroring oxidative stress, ER stress is a critical factor in the development of dementia (Jiang et al., 2010).
The primary defense mechanism against ROS involves the elimination of ROS by antioxidant enzymes such as superoxide dismutase (SOD), glutathione peroxidase (GPx), and catalase (CAT) (Jomova et al., 2024). A secondary line of defense involves uncoupling proteins (UCPs), which restrict excessive mitochondrial ROS production (Hass and Barnstable, 2021). The volatile oil of ATR has been shown to enhance CREB phosphorylation at Ser-133, thereby activating peroxisome proliferator-activated receptor gamma coactivator 1-alpha (PGC-1α) and upregulating SOD, GPx1, and UCP to counteract H2O2-induced oxidative stress in PC12 cells (Yan et al., 2020b). α-Asarone reduces ROS levels (Mikami et al., 2021), while β-Asarone elevates hippocampal SOD and GPx levels in AD rat models, reducing oxidative stress and neuronal injury (Saki et al., 2020). Further studies have demonstrated that the volatile oil of ATR, α-Asarone, and β-Asarone activate the Akt signaling pathway, inducing Antioxidant Response Element (ARE) transcriptional activation and upregulating antioxidant enzymes such as glutathione S-transferase (GST) and NAD(P)H quinone oxidoreductase 1 (NQO1), which facilitate ROS elimination (Lam et al., 2017b). β-Asarone promotes the phosphorylation of PI3K/Akt, enhancing the expression of NF-E2-related factor 2 (Nrf2) and heme oxygenase-1 (HO-1) (Meng et al., 2021). Activated Nrf2 dissociates from kelch-like ECH-associated protein 1 (Keap1), translocates to the nucleus, and binds to ARE, activating downstream genes coding for detoxifying enzymes and antioxidants like HO-1 (Raghunath et al., 2018). HO-1, which is positively regulated by Nrf2, increases GSH-Px, SOD, and CAT expression to improve antioxidant capacity (Ryter, 2021). Additionally, Aβ induces excessive nitric oxide (NO) production via nitric oxide synthase (NOS), and NO, as a free radical, participates in lipid peroxidation by forming peroxynitrite anions (Limón et al., 2009b). α-Asarone has been shown to reduce excessive NO levels in Aβ-induced hippocampal and temporal cortex injury in rats (Limón et al., 2009a). In vitro studies corroborate this, as α-Asarone and β-Asarone lower Aβ25–35-induced NO and inducible NOS (iNOS) levels in PC12 cells (Shi et al., 2021).
Regarding ER stress, PERK phosphorylates eukaryotic initiation factor 2 alpha (eIF2α) (Shi et al., 1998), with eIF2α enhancing Activating Transcription Factor 4 (ATF4) translation, subsequently promoting CHOP transcription (Zinszner et al., 1998). α-Asarone inhibits PERK phosphorylation and tunicamycin-induced ER stress (Mikami et al., 2021). β-Asarone exhibits similar effects by reducing GRP78, p-PERK, and CHOP expression at both mRNA and protein levels in the striatum of Parkinson’s disease rats, thereby mitigating ER stress and autophagy via the PERK/eIF2α pathway (Ning et al., 2019). Likewise, IRE1α, another key mediator of ER stress, undergoes autophosphorylation and oligomerization after dissociation from GRP78, resulting in the splicing of XBP1 mRNA to generate XBP1s, which degrades unfolded proteins (Li Y. et al., 2024). In Parkinson’s disease animal models, β-Asarone acts as an IRE1 inhibitor, modulating ER stress through the IRE1/XBP1 pathway (Ning et al., 2016).
5.9 Inhibiting neuroinflammation
Neuroinflammation, a defensive response occurring within neural tissue, is closely linked to the onset and progression of dementia. Even at early stages of dementia, neuroinflammation—primarily mediated by microglial and astroglial cells—is detectable within the brain (Ahmad et al., 2022). Under normal conditions, these cells aid in clearing abnormal proteins and repairing neurons (Mcalpine et al., 2021). However, during advanced dementia, persistent glial cell activation results in the release of significant levels of inflammatory cytokines, exacerbating protein deposition and leading to neurotoxicity (Hoozemans et al., 2011). Pathogen-associated molecular patterns (PAMPs) or danger-associated molecular patterns (DAMPs) are recognized by pattern recognition receptors (PRRs), activating the IKK complex. This complex phosphorylates and degrades IκB, releasing nuclear factor kappa-B (NF-κB), which activates downstream production of inflammatory cytokines like TNF-α and IL-6 and upregulates NOD-like receptor thermal protein domain-associated protein 3 (NLRP3) expression (Kawasaki and Kawai, 2014). Activation of NLRP3 recruits ASC and pro-caspase-1, forming an inflammasome complex that activates caspase-1 (Zhan et al., 2022). Caspase-1 cleaves gasdermin-D (GSDMD) into GSDMD-N and GSDMD-C fragments, with GSDMD-N forming membrane pores (Meng et al., 2023), while simultaneously processing pro-IL-1β and pro-IL-18 into active IL-1β and IL-18, which are released to promote inflammation (Qin and Zhao, 2023). Pro-inflammatory cytokines like TNF-α and IL-1β further sustain NF-κB activation, creating a vicious cycle (Yu et al., 2020).
The volatile oil of ATR suppresses hippocampal NF-κB and IKKβ phosphorylation in 3×Tg-AD mice, reducing NLRP3 inflammasome activation and downregulating ASC, caspase-1, and the level of GSDMD-N, thereby exerting neuroprotective effects (Xu et al., 2023). Ionized calcium-binding adapter molecule 1 (Iba-1) and glial fibrillary acidic protein (GFAP) are markers of microglial and astroglial activation. α-Asarone decreases GFAP levels and suppresses astroglial activation in APP/PS1 mouse hippocampi, alongside reducing pro-inflammatory cytokines TNF-α, IL-1β, and IL-6 (Zeng et al., 2021). Similarly, β-Asarone decreases GFAP, IL-1β, and TNF-α levels in the brains of AD rats (Yang et al., 2017) and reduces Aβ25–35-induced mRNA levels of IL-6, IL-1β, and TNF-α in SH-SY5Y cells, mitigating both neuroinflammation and inflammation-induced autophagy (Chang and Teng, 2015). Both α-Asarone and β-Asarone inhibit microglial activation by reducing IκB-α phosphorylation and degradation, blocking NF-κB activation, and downregulating pro-inflammatory cytokines TNF-α, IL-1β, and IL-6. In vitro, α-Asarone decreases markers of microglial-mediated inflammation, including Mac-1, CD-68, Iba-1, iNOS, and COX-2 (Kim et al., 2015). The MAPK signaling pathway also contributes to microglial activation. α-Asarone and β-Asarone downregulate JNK and p38MAPK, reducing pro-inflammatory cytokine levels while increasing anti-inflammatory cytokine IL-10 expression via inhibition of the JNK/MAPK pathway (Shi et al., 2021).
5.10 Mitigating vascular risk factors
Cerebrovascular damage, characterized by anatomical disruptions via vascular pathology, is a major cause of vascular cognitive impairment and dementia (VCID). Increasing evidence also implicates vascular risk factors in the progression of various dementia subtypes. Pathological changes, including atherosclerosis, cerebral amyloid angiopathy, and venous collagenopathy, not only contribute to vascular lumen narrowing but also disrupt cerebral autoregulation, reducing cerebral blood flow (Yu et al., 2022). Ischemia and resulting hypoxia induce oxidative stress, endothelial dysfunction, neuroinflammation, neuronal apoptosis, autophagy, and BBB impairment, promoting dementia progression (Burtscher et al., 2021). For example, hypoxia disrupts mitochondrial mechanisms, reduces astrocytic glutamate reuptake, induces glutamate-mediated toxicity, and alters vascular-specific gene expression, resulting in endothelial dysfunction. Vascular endothelial damage in the substantia nigra, brain stem and cerebral cortex was detected in both MPTP-induced animal models and brain samples from PD patients (Sarkar et al., 2014). Furthermore, ischemia and hypoxia often result in impaired cerebrovascular reactivity (CVR), which is linked to dementia, including AD and PD, as well as MCI-to-dementia progression (Camargo et al., 2015; Buratti et al., 2015). In addition, damage to the BBB is one of the core mechanisms and pathological features of cerebrovascular disease (Gao Y. et al., 2022). When BBB integrity is damaged, harmful exudates and inflammatory factors enter the brain tissue, accelerating neuronal damage (Knox et al., 2022), and also leading to reduced Aβ drainage in the perivascular space, resulting in abnormal accumulation of Aβ in the brain (Thal, 2009). In summary, vascular damage and aging are vital contributors to dementia pathogenesis (Andersson and Stone, 2023), as evidenced across diverse dementia subtypes (Raz et al., 2016). Thus, reducing vascular risk factors is a promising strategy for dementia prevention and treatment.
Atherosclerosis is a major cause of reduced cerebral perfusion and hypoxia. α-Asarone has been shown to block endoplasmic reticulum (ER) stress-mediated macrophage apoptosis via interference with caspase-12, IRE1α, and PERK (Park et al., 2016), indicating its potential to antagonize arteriosclerosis and ameliorate the vascular risk factors associated with dementia. Ischemic injury triggers excessive glutamate release, leading to Ca2+ overload, which in turn induces abnormal phosphorylation of calcium/calmodulin-dependent protein kinase II (CaMKII), ultimately causing neuronal apoptosis and damage (Coultrap et al., 2011). α-Asarone has been found to restore excitatory-inhibitory balance, reduce calcium overload and CaMKII phosphorylation, and inhibit apoptosis, thereby preventing early neuronal injury following subarachnoid hemorrhage (Gao et al., 2024). Furthermore, α-Asarone mitigates cerebral infarct volume, improves neurological function, reduces GFAP and Iba-1 expression, inhibits glial activation, suppresses autophagy as evidenced by the reduced LC3II/LC3I ratio and increased p62 expression, and lowers the risk of ischemia-reperfusion-induced neuronal injury (Zhang K. et al., 2021). β-Asarone enhances the learning and memory abilities of AD model rats, improves relative cerebral blood flow (rCBF), reduces lactate and pyruvate levels in brain tissue, increases Na+-K+-ATPase activity, and downregulates hippocampal ET-1 mRNA expression, exhibiting vascular protective effects in AD rats (Li et al., 2012). An increasing body of evidence indicates that oxidative stress plays a central role in ischemia-reperfusion injury (Jakesevic et al., 2011). β-Asarone reduces the risk of localized cerebral ischemia-reperfusion injury and improves neurological function by enhancing the activity of enzymes involved in oxidative stress regulation, including lactate dehydrogenase (LDH), glutathione reductase (GR), catalase (CAT), glutathione S-transferase (GST), glutathione (GSH), glutathione peroxidase (GPx), and Na+-K+-ATPase (Yang et al., 2013). The Nrf2-ARE pathway not only regulates the transcription of numerous antioxidant genes but also modulates apoptosis, making the activation of Nrf2 and its downstream genes a promising strategy for preventing ischemia-reperfusion-induced neural injury (Shi et al., 2019). β-Asarone activates the Nrf2-ARE pathway, enhancing the expression of Nrf2, NQO1, GCLM, HO-1, and GCLC proteins, inhibiting cortical neuronal apoptosis, reducing cerebral infarct volume in MCAO rats, and improving neurological outcomes (Pan et al., 2021). Oxidative stress can also act as a signal for inducing autophagy (Redza-Dutordoir and Averill-Bates, 2021), forming a vicious cycle. Through modulation of the cAMP/PKA/CREB signaling pathway, β-Asarone reduces oxidative stress levels, suppresses excessive autophagy, restores mitochondrial function, and alleviates synaptic dysfunction and brain tissue damage in vascular dementia mice (Ning et al., 2024). Additionally, β-Asarone regulates the JNK/Bcl-2/Beclin-1 pathway, decreasing JNK and p-JNK expression while increasing Bcl-2 levels to modulate Beclin-1, thereby suppressing ischemia-reperfusion-induced excessive autophagy in brain tissue (Liu et al., 2012). As previously discussed, endothelial dysfunction plays a pivotal role in linking cerebrovascular disease and dementia. Endothelial dysfunction reduces NO bioavailability and inactivates endothelial nitric oxide synthase (eNOS) in cerebral microvascular endothelial cells, consequently upregulating amyloid precursor protein and its cleavage enzymes, ultimately leading to Aβ deposition (Koizumi et al., 2016). β-Asarone increases the number of CD31 and Ki-67-positive cells, promotes VEGFA expression, and upregulates eNOS levels in MCAO model rats (Sun et al., 2024), suggesting its potential to alleviate endothelial dysfunction and promote angiogenesis.
6 Discussion
Advancements in dementia research increasingly reveal that the underlying mechanisms of the disease’s pathogenesis are complex, involving the interplay of multiple factors rather than a singular cause. In various subtypes of dementia, interactions between processes such as abnormal protein aggregation, disrupted neurogenesis, autophagy dysregulation, neuronal apoptosis, cellular stress, synaptic dysfunction, neurotransmitter imbalances, neuroinflammation, and vascular damage create a vicious cycle that collectively drives disease progression. Furthermore, a specific pathological mechanism may contribute to multiple dementia subtypes, and the boundaries between these subtypes are not always distinct. For instance, while Aβ has long been associated with AD, it is also observed in PD and DLB (Sengupta and Kayed, 2022), as well as in the brains of VD patients, particularly in cerebral vessel walls, a condition termed cerebral amyloid angiopathy (CAA) (Kalaria, 2003). Numerous similar examples exist, and given the complexity of the disease’s mechanisms, single-mechanism therapies may be difficult to achieve substantial outcomes. Currently, the mitigation of dementia primarily depend on synthetic chemical drugs. However, the narrow therapeutic spectrum and singular mechanism of action are common limitations of these treatments. Conversely, the plant metabolites from medicinal plants are easily accessible, cost-effective, and have been employed for disease management throughout history. In recent years, a growing number of the plant metabolites have been found to exhibit analgesic, sedative, antidepressant, anticonvulsant, antiepileptic, and neuroprotective effects, demonstrating a distinct advantage (Zhang Y. et al., 2021).
Compared to conventional drugs, the volatile oil of ATR contains multiple metabolites targeting various pathways and mechanisms, which may potentially produce synergistic or additive effects. Currently, α-Asarone and β-Asarone are widely recognized as the main active ingredients of the volatile oil of ATR in mitigating dementia. Consequently, much of the current research focuses on testing drugs containing α-Asarone and β-Asarone, while studies exploring the combined effects of the volatile oil of ATR or other metabolites within it in mitigating dementia remain rare. This scarcity may be attributed to the challenges posed by studying medicinal plant extracts in pharmacological research. However, this also raises the question of whether there are synergistic responses among different metabolites in the process of mitigating dementia using the volatile oil of ATR. For instance, some researchers have proposed that α-Asarone and β-Asarone, in their natural ratio (1:4) as present in the volatile oil of ATR, exhibit synergistic effects in enhancing neurofilament promoter activity while reducing side effects (Lam et al., 2016b). Does a similar synergistic effect also exist among other metabolites in the volatile oil of ATR, even those present in relatively low abundance? This hypothesis may serve as one of the potential directions for further investigation in the future. Additionally, the high lipophilicity of the volatile oil of ATR facilitates its ability to cross the BBB, overcoming the challenges faced by many drugs in infiltrating brain tissue. However, as previously mentioned, while current studies generally agree that the volatile oil of ATR can traverse the BBB, there is ongoing debate regarding whether it repairs BBB damage or simply increases BBB permeability. Such discrepancies highlight the need for further in-depth research into the specific mechanisms by which the volatile oil of ATR or its main ingredients interacts with the BBB, in order to determine whether they have potential side effects. At the same time, it must be noted that while the volatile oil of ATR have demonstrated promising anti-dementia effects and are generally considered a safe traditional medicine, as previously mentioned, there may still be potential toxic side effects. To mitigate toxicity, appropriate dosage selection and treatment duration should be carefully considered. However, the volatile oil of ATR faces the challenge of low oral bioavailability, which presents a disadvantage for treatment regimens that require high doses or long-term administration. As noted earlier, advancements in delivery systems (Inamdar et al., 2024), such as nanoparticle delivery and intranasal delivery, can significantly enhance the bioavailability of the drug (Ahmad et al., 2018). Therefore, the issues of low bioavailability and potential toxic side effects associated with the volatile oil of ATR may be addressed or avoided through these improvements. Nonetheless, research on the administration routes of the volatile oil of ATR is currently very limited, and the extent to which different administration methods affect its safety requires further evaluation. Moreover, numerous recent studies on the volatile oil of ATR lack standardized experimental methodologies. For instance, significant variations exist in the extraction methods of the volatile oil of ATR, dosage selection, routes of administration (such as oral, inhalation, or injection), and experimental durations, making it challenging to compare or integrate the findings. While such inconsistencies may stem from the progressive nature of dementia as well as the inherent challenges of studying medicinal plant extracts in pharmacological research, the need for standardized and systematic studies remains paramount. Furthermore, although the volatile oil of ATR has a long history of medicinal use, the clinical efficacy and safety of botanical drugs and plant-derived metabolites should not be generalized. Current research primarily focuses on pharmacological studies, with evidence mostly derived from molecular mechanism studies in cell and animal models. Systematic preclinical toxicity studies remain lacking, and large-scale, long-term clinical trials using the volatile oil of ATR as a standalone therapy are scarce. Thus, systematic investigations into its efficacy and safety are urgently needed.
For future research, the following questions can be explored, but are not limited to these aspects: (1) Do other less abundant metabolites in the volatile oil of ATR play a role in dementia intervention? Are there synergistic or additive effects among these metabolites? (2) What is the mechanism by which the volatile oil of ATR penetrates the BBB? Does it increase BBB permeability and, if so, could this result in unintended side effects? (3) Does the efficacy of the volatile oil of ATR in dementia mitigation vary significantly depending on extraction methods, dosage, administration routes, and experiment duration? Is it possible to establish an optimized and standardized protocol for its extraction and application? (4) How can the safety of the volatile oil of ATR under long-term use or high dosages be determined? What internationally accepted standardized toxicological studies can be employed to comprehensively evaluate its safety? (5) Can optimizing drug delivery systems—such as nanoparticles or intranasal administration—significantly enhance the bioavailability of the volatile oil of ATR? Could these innovative delivery systems alter its efficacy, safety, and pharmacokinetic properties? (6) Are there significant differences in the mechanisms of action and efficacy of the volatile oil of ATR in preventing dementia during the cognitive impairment stage versus treating it during the dementia stage? (7) Can the extensive mechanistic studies conducted on cellular and animal models be effectively translated into systematic clinical research to verify its efficacy and safety?
Overall, the volatile oil of ATR demonstrates significant potential in alleviating dementia. While current research provides a relatively comprehensive understanding of its mechanisms of action in dementia intervention, further foundational studies and clinical research are required to work in tandem. Employing standardized and systematic approaches is essential to overcome critical challenges and ultimately achieve robust validation of its efficacy and safe application.
7 Conclusion
In conclusion, the multi-pathway, coordinated pharmacological effects of the volatile oil of ATR align well with the complex mechanisms underlying the development of dementia. This highlights its significant potential in mitigating dementia and presents a promising opportunity for the treatment of not only dementia but also other neurodegenerative diseases. This potential could pave new avenues for future research. Nonetheless, further rigorous and high-quality studies are required to overcome the associated challenges, including ensuring the accuracy and stability of the chemical characterization of the volatile oil of ATR, clarifying the synergistic interactions between its metabolites, identifying potential toxicities, and validating its exact clinical efficacy.
Author contributions
YB: Conceptualization, Data curation, Formal Analysis, Investigation, Methodology, Project administration, Software, Supervision, Validation, Visualization, Writing – original draft, Writing – review and editing. SL: Data curation, Formal Analysis, Methodology, Project administration, Software, Supervision, Validation, Visualization, Writing – review and editing. TY: Data curation, Formal Analysis, Methodology, Supervision, Visualization, Writing – review and editing. YW: Data curation, Methodology, Supervision, Writing – review and editing, Formal Analysis. MS: Data curation, Formal Analysis, Project administration, Supervision, Writing – review and editing. JC: Conceptualization, Formal Analysis, Funding acquisition, Methodology, Project administration, Resources, Software, Supervision, Validation, Writing – review and editing.
Funding
The author(s) declare that financial support was received for the research and/or publication of this article. This study was supported by the National Natural Science Foundation of China (Grant No. 82274395) and the Key Laboratory of Basic Theory of Traditional Chinese Medicine in Heilongjiang Province.
Acknowledgments
The authors express their gratitude for the financial support provided by the National Natural Science Foundation of China and for the resources and expertise offered by the Key Laboratory of Basic Theory Research in Traditional Chinese Medicine of Heilongjiang Province.
Conflict of interest
The authors declare that the research was conducted in the absence of any commercial or financial relationships that could be construed as a potential conflict of interest.
Generative AI statement
The author(s) declare that no Generative AI was used in the creation of this manuscript.
Publisher’s note
All claims expressed in this article are solely those of the authors and do not necessarily represent those of their affiliated organizations, or those of the publisher, the editors and the reviewers. Any product that may be evaluated in this article, or claim that may be made by its manufacturer, is not guaranteed or endorsed by the publisher.
References
Afshar, S., Shahidi, S., Rohani, A. H., Soleimani Asl, S., and Komaki, A. (2019). Protective effects of 5-HT(1A) receptor antagonist and 5-HT(2A) receptor agonist on the biochemical and histological features in a rat model of Alzheimer’s disease. J. Chem. Neuroanat. 96, 140–147. doi:10.1016/j.jchemneu.2019.01.008
Agrotis, A., Pengo, N., Burden, J. J., and Ketteler, R. (2019). Redundancy of human ATG4 protease isoforms in autophagy and LC3/GABARAP processing revealed in cells. Autophagy 15 (6), 976–997. doi:10.1080/15548627.2019.1569925
Ahmad, M. A., Kareem, O., Khushtar, M., Akbar, M., Haque, M. R., Iqubal, A., et al. (2022). Neuroinflammation: a potential risk for dementia. Int. J. Mol. Sci. 23 (2), 616. doi:10.3390/ijms23020616
Ahmad-Annuar, A., Ciani, L., Simeonidis, I., Herreros, J., Fredj, N. B., Rosso, S. B., et al. (2006). Signaling across the synapse: a role for Wnt and Dishevelled in presynaptic assembly and neurotransmitter release. J. Cell Biol. 174 (1), 127–139. doi:10.1083/jcb.200511054
Ahmad, N., Ahmad, R., Alam, M. A., and Ahmad, F. J. (2018). Quantification and brain targeting of eugenol-loaded surface modified nanoparticles through intranasal route in the treatment of cerebral ischemia. Drug Res. 68 (10), 584–595. doi:10.1055/a-0596-7288
Ajoolabady, A., Lindholm, D., Ren, J., and Pratico, D. (2022). ER stress and UPR in Alzheimer’s disease: mechanisms, pathogenesis, treatments. Cell death and Dis. 13 (8), 706. doi:10.1038/s41419-022-05153-5
Alladi, S., and Hachinski, V. (2018). World dementia: one approach does not fit all. Neurology 91 (6), 264–270. doi:10.1212/WNL.0000000000005941
Andersson, M. J., and Stone, J. (2023). Best medicine for dementia: the life-long defense of the brain. J. Alzheimer’s Dis. JAD 94 (1), 51–66. doi:10.3233/JAD-230429
Ashadevi, S., Babu, S., and Premkumar, K. (2014). In vivo genotoxic studies of β-asarone in mice bone marrow. Int. J. Drug Develop. Res. 6, 128–132.
Atiq, A., Lee, H. J., Khan, A., Kang, M. H., Rehman, I. U., Ahmad, R., et al. (2023). Vitamin E analog trolox attenuates MPTP-induced Parkinson’s disease in mice, mitigating oxidative stress, neuroinflammation, and motor impairment. Int. J. Mol. Sci. 24 (12), 9942. doi:10.3390/ijms24129942
Balakrishnan, R., Cho, D. Y., Kim, I. S., Seol, S. H., and Choi, D. K. (2022). Molecular mechanisms and therapeutic potential of α- and β-asarone in the treatment of neurological disorders. Antioxidants (Basel, Switz.) 11 (2), 281. doi:10.3390/antiox11020281
Bennett, M. C. (2005). The role of alpha-synuclein in neurodegenerative diseases. Pharmacol. and Ther. 105 (3), 311–331. doi:10.1016/j.pharmthera.2004.10.010
Berg, K., Bischoff, R., StegmüLLER, S., Cartus, A., and Schrenk, D. (2016). Comparative investigation of the mutagenicity of propenylic and allylic asarone isomers in the Ames fluctuation assay. Mutagenesis 31 (4), 443–451. doi:10.1093/mutage/gew007
Biane, J., Conner, J. M., and Tuszynski, M. H. (2014). Nerve growth factor is primarily produced by GABAergic neurons of the adult rat cortex. Front. Cell. Neurosci. 8, 220. doi:10.3389/fncel.2014.00220
Breton, A., Casey, D., and Arnaoutoglou, N. A. (2019). Cognitive tests for the detection of mild cognitive impairment (MCI), the prodromal stage of dementia: meta-analysis of diagnostic accuracy studies. Int. J. Geriat. Psych. 34 (2), 233–242. doi:10.1002/gps.5016
Buratti, L., Balestrini, S., Altamura, C., Viticchi, G., Falsetti, L., Luzzi, S., et al. (2015). Markers for the risk of progression from mild cognitive impairment to Alzheimer’s disease. J. Alzheimer’s Dis. JAD 45 (3), 883–890. doi:10.3233/JAD-143135
Burtscher, J., Mallet, R. T., Burtscher, M., and Millet, G. P. (2021). Hypoxia and brain aging: neurodegeneration or neuroprotection? Ageing Res. Rev. 68, 101343. doi:10.1016/j.arr.2021.101343
Cai, W., Wu, T., and Chen, N. (2023). The amyloid-beta clearance: from molecular targets to glial and neural cells. Biomolecules 13 (2), 313. doi:10.3390/biom13020313
Calabresi, P., Mechelli, A., Natale, G., Volpicelli-Daley, L., Di Lazzaro, G., and Ghiglieri, V. (2023). Alpha-synuclein in Parkinson’s disease and other synucleinopathies: from overt neurodegeneration back to early synaptic dysfunction. Cell death and Dis. 14 (3), 176. doi:10.1038/s41419-023-05672-9
Camargo, C. H., Martins, E. A., Lange, M. C., Hoffmann, H. A., Luciano, J. J., Young Blood, M. R., et al. (2015). Abnormal cerebrovascular reactivity in patients with Parkinson’s disease. Parkinson’s Dis. 2015, 523041. doi:10.1155/2015/523041
Canter, R. G., Penney, J., and Tsai, L. H. (2016). The road to restoring neural circuits for the treatment of Alzheimer’s disease. Nature 539 (7628), 187–196. doi:10.1038/nature20412
Canudas, A. M., Gutierrez-Cuesta, J., RodríGUEZ, M. I., Acuña-Castroviejo, D., Sureda, F. X., Camins, A., et al. (2005). Hyperphosphorylation of microtubule-associated protein tau in senescence-accelerated mouse (SAM). Mech. ageing Dev. 126 (12), 1300–1304. doi:10.1016/j.mad.2005.07.008
Cassani-Galindo, M., Madrigal-Bujaidar, E., Chamorro, G., Díaz, F., Tamariz, J., and Espinosa-Aguirre, J. J. (2005). In vitro genotoxic evaluation of three alpha-asarone analogues. Toxicol. vitro Int. J. Publ. Assoc. Bibra 19 (4), 547–552. doi:10.1016/j.tiv.2005.01.007
Cerpa, W., Godoy, J. A., Alfaro, I., Farías, G. G., Metcalfe, M. J., Fuentealba, R., et al. (2008). Wnt-7a modulates the synaptic vesicle cycle and synaptic transmission in hippocampal neurons. J. Biol. Chem. 283 (9), 5918–5927. doi:10.1074/jbc.M705943200
Chamorro, G., GarduñO, L., MartíNEZ, E., Madrigal, E., Tamariz, J., and Salazar, M. (1998). Dominant lethal study of alpha-asarone in male mice. Toxicol. Lett. 99 (2), 71–77. doi:10.1016/s0378-4274(98)00041-1
Chamorro, G., Salazar, M., Salazar, S., and Mendoza, T. (1993). Pharmacology and toxicology of Guatteria gaumeri and alpha-asarone. Rev. Investig. Clinica; Organo del Hosp. Enfermedades Nutr. 45 (6), 597–604.
Chamorro, G., Salazar, M., Tamariz, J., Díaz, F., and Labarrios, F. (1999). Dominant lethal study of alpha-asarone in male and female mice after sub-chronic treatment. Phytotherapy Res. PTR 13 (4), 308–311. doi:10.1002/(SICI)1099-1573(199906)13:4<308::AID-PTR449>3.0.CO;2-X
Chan, S. L., Furukawa, K., and Mattson, M. P. (2002). Presenilins and APP in neuritic and synaptic plasticity: implications for the pathogenesis of Alzheimer’s disease. Neuromolecular Med. 2 (2), 167–196. doi:10.1385/NMM:2:2:167
ChandíA-Cristi, A., Stuardo, N., Trejos, C., Leal, N., Urrutia, D., Bronfman, F. C., et al. (2023). c-Abl tyrosine kinase is required for BDNF-induced dendritic branching and growth. Int. J. Mol. Sci. 24 (3), 1944. doi:10.3390/ijms24031944
Chang, W., and Teng, J. (2015). β-asarone prevents Aβ25-35-induced inflammatory responses and autophagy in SH-SY5Y cells: down expression Beclin-1, LC3B and up expression Bcl-2. Int. J. Clin. Exp. Med. 8 (11), 20658–20663.
Chellian, R., Pandy, V., and Mohamed, Z. (2017). Pharmacology and toxicology of α- and β-Asarone: a review of preclinical evidence. Phytomedicine Int. J. Phytotherapy Phytopharm. 32, 41–58. doi:10.1016/j.phymed.2017.04.003
Chen, Q. X., Miao, J. K., Li, C., Li, X. W., Wu, X. M., and Zhang, X. p. (2013). Anticonvulsant activity of acute and chronic treatment with a-asarone from Acorus gramineus in seizure models. Biol. and Pharm. Bull. 36 (1), 23–30. doi:10.1248/bpb.b12-00376
Chen, Z. R., Huang, J. B., Yang, S. L., and Hong, F. F. (2022a). Role of cholinergic signaling in Alzheimer’s disease. Mol. (Basel, Switz.) 27 (6), 1816. doi:10.3390/molecules27061816
Chen, J., Zhang, M., Bai, H., Shi, P., Du, M., Zhang, S., et al. (2022b). Overexpression of C9orf72 exacerbates Aβ25-35-induced oxidative stress and apoptosis in PC12 cells. Acta Neurobiol. Exp. 82 (1), 77–87. doi:10.55782/ane-2022-007
Chen, X., Walker, D. G., Schmidt, A. M., Arancio, O., Lue, L. F., and Yan, S. D. (2007). RAGE: a potential target for Abeta-mediated cellular perturbation in Alzheimer’s disease. Curr. Mol. Med. 7 (8), 735–742. doi:10.2174/156652407783220741
Chen, X., Wang, F., Gan, J., Zhang, Z., Liang, X., Li, T., et al. (2020b). Myelin deficits caused by Olig2 deficiency lead to cognitive dysfunction and increase vulnerability to social withdrawal in adult mice. Neurosci. Bull. 36 (4), 419–426. doi:10.1007/s12264-019-00449-7
Chen, X., Zhou, B., Yan, T., Wu, H., Feng, J., Chen, H., et al. (2018). Peroxynitrite enhances self-renewal, proliferation and neuronal differentiation of neural stem/progenitor cells through activating HIF-1α and Wnt/β-catenin signaling pathway. Free Radic. Biol. and Med. 117, 158–167. doi:10.1016/j.freeradbiomed.2018.02.011
Chen, Y., Gao, X., Liu, Q., Zeng, L., Zhang, K., Mu, K., et al. (2020a). Alpha-asarone improves cognitive function of aged rats by alleviating neuronal excitotoxicity via GABA(A) receptors. Neuropharmacology 162, 107843. doi:10.1016/j.neuropharm.2019.107843
Chen, Z., and Zhong, C. (2014). Oxidative stress in Alzheimer’s disease. Neurosci. Bull. 30 (2), 271–281. doi:10.1007/s12264-013-1423-y
Che, P., Zhang, J., Yu, M., Tang, P., Wang, Y., Lin, A., et al. (2023). Dl-3-n-butylphthalide promotes synaptic plasticity by activating the Akt/ERK signaling pathway and reduces the blood-brain barrier leakage by inhibiting the HIF-1α/MMP signaling pathway in vascular dementia model mice. CNS Neurosci. and Ther. 29 (5), 1392–1404. doi:10.1111/cns.14112
Coultrap, S. J., Vest, R. S., Ashpole, N. M., Hudmon, A., and Bayer, K. U. (2011). CaMKII in cerebral ischemia. Acta Pharmacol. Sin. 32 (7), 861–872. doi:10.1038/aps.2011.68
Cressatti, M., Song, W., Turk, A. Z., Garabed, L. R., Benchaya, J. A., Galindez, C., et al. (2019). Glial HMOX1 expression promotes central and peripheral α-synuclein dysregulation and pathogenicity in parkinsonian mice. Glia 67 (9), 1730–1744. doi:10.1002/glia.23645
Cui, S. Q., Wang, Q., Zheng, Y., Xiao, B., Sun, H. W., Gu, X. L., et al. (2015). Puerarin protects against damage to spatial learning and memory ability in mice with chronic alcohol poisoning. Braz. J. Med. Biol. Res. = Revista Brasileira de Pesquisas Medicas e Biol. 48 (6), 515–522. doi:10.1590/1414-431X20144250
Decuypere, J. P., Parys, J. B., and Bultynck, G. (2012). Regulation of the autophagic bcl-2/beclin 1 interaction. Cells 1 (3), 284–312. doi:10.3390/cells1030284
Deng, L., Wang, Y., Gong, T., Sun, X., and Zhang, Z. R. (2017). Dissolution and bioavailability enhancement of alpha-asarone by solid dispersions via oral administration. Drug Dev. industrial Pharm. 43 (11), 1817–1826. doi:10.1080/03639045.2017.1349783
Deng, M., Huang, L., and Zhong, X. (2020). β-asarone modulates Beclin-1, LC3 and p62 expression to attenuate Aβ40 and Aβ42 levels in APP/PS1 transgenic mice with Alzheimer’s disease. Mol. Med. Rep. 21 (5), 2095–2102. doi:10.3892/mmr.2020.11026
Depp, C., Sun, T., Sasmita, A. O., Spieth, L., Berghoff, S. A., Nazarenko, T., et al. (2023). Myelin dysfunction drives amyloid-β deposition in models of Alzheimer’s disease. Nature 618 (7964), 349–357. doi:10.1038/s41586-023-06120-6
Duda, J. E. (2004). Pathology and neurotransmitter abnormalities of dementia with Lewy bodies. Dementia geriatric cognitive Disord. 17 (Suppl. 1), 3–14. doi:10.1159/000074677
Erekat, N. S. (2022). Apoptosis and its therapeutic implications in neurodegenerative diseases. Clin. Anat. 35 (1), 65–78. doi:10.1002/ca.23792
Eshkoor, S. A., Hamid, T. A., Mun, C. Y., and Ng, C. K. (2015). Mild cognitive impairment and its management in older people. Clin. Interventions Aging 10, 687–693. doi:10.2147/CIA.S73922
Fan, T. J., Han, L. H., Cong, R. S., and Liang, J. (2005). Caspase family proteases and apoptosis. Acta Biochimica Biophysica Sinica 37 (11), 719–727. doi:10.1111/j.1745-7270.2005.00108.x
Fang, E. F., Hou, Y., Palikaras, K., Adriaanse, B. A., Kerr, J. S., Yang, B., et al. (2019). Mitophagy inhibits amyloid-β and tau pathology and reverses cognitive deficits in models of Alzheimer’s disease. Nat. Neurosci. 22 (3), 401–412. doi:10.1038/s41593-018-0332-9
Feng, P., Chen, Y., Sun, K., Wei, X., Ding, Y., Shang, J., et al. (2024). Volatile oil from Acori graminei Rhizoma affected the synaptic plasticity of rats with tic disorders by modulating dopaminergic and glutamatergic systems. J. Ethnopharmacol. 335, 118676. doi:10.1016/j.jep.2024.118676
Food, S. C. O. (2002). Opinion of the scientific committee on food on the presence of β-asarone in flavourings and other food ingredients with flavouring properties, 1–15.
Franco-Bocanegra, D. K., George, B., Lau, L. C., Holmes, C., Nicoll, J. A. R., and Boche, D. (2019). Microglial motility in Alzheimer’s disease and after Aβ42 immunotherapy: a human post-mortem study. Acta neuropathol. Commun. 7 (1), 174. doi:10.1186/s40478-019-0828-x
Fu, H., Xu, Z., Zhang, X. L., and Zheng, G. Q. (2019). Kaixinsan, a well-known Chinese herbal prescription, for Alzheimer’s disease and depression: a preclinical systematic review. Front. Neurosci. 13, 1421. doi:10.3389/fnins.2019.01421
Fu, Y., Yang, Y., Shi, J., Bishayee, K., Lin, L., Lin, Y., et al. (2020). Acori tatarinowii rhizoma extract ameliorates Alzheimer’s pathological syndromes by repairing myelin injury and lowering Tau phosphorylation in mice. Die Pharm. 75 (8), 395–400. doi:10.1691/ph.2020.0492
Gao, X., Li, R., Luo, L., Liao, C., Yang, H., and Mao, S. (2024). Alpha-Asarone ameliorates neurological dysfunction of subarachnoid hemorrhagic rats in both acute and recovery phases via regulating the CaMKII-dependent pathways. Transl. stroke Res. 15 (2), 476–494. doi:10.1007/s12975-023-01139-3
Gao, X., Li, R., Luo, L., Zhang, D., Liu, Q., Zhang, J., et al. (2022a). Alpha-asarone ameliorates neurological deterioration of intracerebral hemorrhagic rats by alleviating secondary brain injury via anti-excitotoxicity pathways. Phytomedicine Int. J. phytotherapy Phytopharm. 105, 154363. doi:10.1016/j.phymed.2022.154363
Gao, Y., Li, D., Lin, J., Thomas, A. M., Miao, J., Chen, D., et al. (2022b). Cerebral small vessel disease: pathological mechanisms and potential therapeutic targets. Front. Aging Neurosci. 14, 961661. doi:10.3389/fnagi.2022.961661
Gauthier, S., Reisberg, B., Zaudig, M., Petersen, R. C., Ritchie, K., Broich, K., et al. (2006). Mild cognitive impairment. Lancet 367 (9518), 1262–1270. doi:10.1016/S0140-6736(06)68542-5
Geng, Y., Li, C., Liu, J., Xing, G., Zhou, L., Dong, M., et al. (2010). Beta-asarone improves cognitive function by suppressing neuronal apoptosis in the beta-amyloid hippocampus injection rats. Biol. and Pharm. Bull. 33 (5), 836–843. doi:10.1248/bpb.33.836
GöGGELMANN, W., and Schimmer, O. (1983). Mutagenicity testing of beta-asarone and commercial calamus drugs with Salmonella typhimurium. Mutat. Res. 121 (3-4), 191–194. doi:10.1016/0165-7992(83)90202-6
Groc, L., and Choquet, D. (2020). Linking glutamate receptor movements and synapse function. Sci. (New York, NY) 368 (6496), eaay4631. doi:10.1126/science.aay4631
Halliwell, B. (2006). Reactive species and antioxidants. Redox biology is a fundamental theme of aerobic life. Plant Physiol. 141 (2), 312–322. doi:10.1104/pp.106.077073
Han, K., Kim, J., and Choi, M. (2015). Autophagy mediates phase transitions from cell death to life. Heliyon 1 (1), e00027. doi:10.1016/j.heliyon.2015.e00027
Han, Y., Wang, N., Kang, J., and Fang, Y. (2020). β-Asarone improves learning and memory in Aβ(1-42)-induced Alzheimer’s disease rats by regulating PINK1-Parkin-mediated mitophagy. Metab. Brain Dis. 35 (7), 1109–1117. doi:10.1007/s11011-020-00587-2
Harrill, J. A., Chen, H., Streifel, K. M., Yang, D., Mundy, W. R., and Lein, P. J. (2015). Ontogeny of biochemical, morphological and functional parameters of synaptogenesis in primary cultures of rat hippocampal and cortical neurons. Mol. Brain 8, 10. doi:10.1186/s13041-015-0099-9
Hasheminejad, G., and Caldwell, J. (1994). Genotoxicity of the alkenylbenzenes alpha- and beta-asarone, myristicin and elimicin as determined by the UDS assay in cultured rat hepatocytes. Food Chem. Toxicol. Int. J. Publ. Br. Industrial Biol. Res. Assoc. 32 (3), 223–231. doi:10.1016/0278-6915(94)90194-5
Hass, D. T., and Barnstable, C. J. (2021). Uncoupling proteins in the mitochondrial defense against oxidative stress. Prog. Retin. Eye Res. 83, 100941. doi:10.1016/j.preteyeres.2021.100941
Haughey, N. J., Nath, A., Chan, S. L., Borchard, A. C., Rao, M. S., and Mattson, M. P. (2002). Disruption of neurogenesis by amyloid beta-peptide, and perturbed neural progenitor cell homeostasis, in models of Alzheimer’s disease. J. Neurochem. 83 (6), 1509–1524. doi:10.1046/j.1471-4159.2002.01267.x
Haupenthal, S., Vallicotti, S., Hemgesberg, M., and Melanie, E. (2015). The alkaline comet assay as a method to investigate the DNA strand breaking effect of phenylpropanoids in mammalian cells. Front. Genet. 6. doi:10.3389/conf.fgene.2015.01.00037
Heinrich, M., Jalil, B., Abdel-Tawab, M., Echeverria, J., Kulić, Ž., McGaw, L. J., et al. (2022). Best Practice in the chemical characterisation of extracts used in pharmacological and toxicological research-The ConPhyMP-Guidelines. Front. Pharmacol. 13, 953205. doi:10.3389/fphar.2022.953205
HernáNDEZ, A., LóPEZ, M. L., Chamorro, G., and Mendoza-Figueroa, T. (1993). Inhibition of lipid synthesis and secretion in long-term cultures of adult rat hepatocytes by alpha-asarone. Planta Medica 59 (2), 121–124. doi:10.1055/s-2006-959625
Hoozemans, J. J., Rozemuller, A. J., Van Haastert, E. S., Eikelenboom, P., and van Gool, W. A. (2011). Neuroinflammation in Alzheimer’s disease wanes with age. J. Neuroinflammation 8, 171. doi:10.1186/1742-2094-8-171
Hotamisligil, G. S. (2010). Endoplasmic reticulum stress and the inflammatory basis of metabolic disease. Cell 140 (6), 900–917. doi:10.1016/j.cell.2010.02.034
Hou, Y., Wang, K., Wan, W., Cheng, Y., Pu, X., and Ye, X. (2018). Resveratrol provides neuroprotection by regulating the JAK2/STAT3/PI3K/AKT/mTOR pathway after stroke in rats. Genes and Dis. 5 (3), 245–255. doi:10.1016/j.gendis.2018.06.001
Hou, Z., Yang, X., Jiang, L., Song, L., Li, Y., Li, D., et al. (2023). Active components and molecular mechanisms of Sagacious Confucius’ Pillow Elixir to treat cognitive impairment based on systems pharmacology. Aging 15 (14), 7278–7307. doi:10.18632/aging.204912
Huang, L., and Zhang, L. (2019). Neural stem cell therapies and hypoxic-ischemic brain injury. Prog. Neurobiol. 173, 1–17. doi:10.1016/j.pneurobio.2018.05.004
Huang, X., Cuajungco, M. P., Atwood, C. S., Hartshorn, M. A., Tyndall, J. D., Hanson, G. R., et al. (1999). Cu(II) potentiation of alzheimer abeta neurotoxicity. Correlation with cell-free hydrogen peroxide production and metal reduction. J. Biol. Chem. 274 (52), 37111–37116. doi:10.1074/jbc.274.52.37111
Huey, E. D., Putnam, K. T., and Grafman, J. (2006). A systematic review of neurotransmitter deficits and treatments in frontotemporal dementia. Neurology 66 (1), 17–22. doi:10.1212/01.wnl.0000191304.55196.4d
Hu, Y., Yuan, M., Liu, P., Mu, L., and Wang, H. (2009). Effect of Acorus tatarinowii schott on ultrastructure and permeability of blood-brain barrier. Zhongguo Zhong yao za zhi = Zhongguo zhongyao zazhi = China J. Chin. Materia Medica 34 (3), 349–351.
Inamdar, A., Gurupadayya, B., Halagali, P., Tippavajhala, V. K., Khan, F., Pathak, R., et al. (2024). Unraveling neurological drug delivery: polymeric nanocarriers for enhanced blood-brain barrier penetration. Curr. Drug Targets 26, 243–266. doi:10.2174/0113894501339455241101065040
Irie, Y., and Keung, W. M. (2003). Rhizoma acori graminei and its active principles protect PC-12 cells from the toxic effect of amyloid-beta peptide. Brain Res. 963 (1-2), 282–289. doi:10.1016/s0006-8993(02)04050-7
Isaev, N. K., Stelmashook, E. V., and Genrikhs, E. E. (2019). Neurogenesis and brain aging. Rev. Neurosci. 30 (6), 573–580. doi:10.1515/revneuro-2018-0084
Jakesevic, M., Aaby, K., Borge, G. I., Jeppsson, B., Ahrné, S., and Molin, G. (2011). Antioxidative protection of dietary bilberry, chokeberry and Lactobacillus plantarum HEAL19 in mice subjected to intestinal oxidative stress by ischemia-reperfusion. BMC Complementary Altern. Med. 11, 8. doi:10.1186/1472-6882-11-8
Jiang, P., Gan, M., Ebrahim, A. S., Lin, W. L., Melrose, H. L., and Yen, S. H. C. (2010). ER stress response plays an important role in aggregation of α-synuclein. Mol. Neurodegener. 5, 56. doi:10.1186/1750-1326-5-56
Jimenez, L., Chamorro, G., Salazar, M., and Pages, N. (1988). Teratogenic evaluation of alpha-asarone in the rat. Ann. Pharm. Francaises 46 (3), 179–183.
Jomova, K., Alomar, S. Y., Alwasel, S. H., Nepovimova, E., Kuca, K., and Valko, M. (2024). Several lines of antioxidant defense against oxidative stress: antioxidant enzymes, nanomaterials with multiple enzyme-mimicking activities, and low-molecular-weight antioxidants. Archives Toxicol. 98 (5), 1323–1367. doi:10.1007/s00204-024-03696-4
Kalaria, R. N. (2003). Comparison between Alzheimer’s disease and vascular dementia: implications for treatment. Neurological Res. 25 (6), 661–664. doi:10.1179/016164103101201968
Kang, K., Chen, S. H., Wang, D. P., and Chen, F. (2024). Inhibition of endoplasmic reticulum stress improves chronic ischemic hippocampal damage associated with suppression of ire1α/TRAF2/ASK1/JNK-dependent apoptosis. Inflammation 47 (4), 1479–1490. doi:10.1007/s10753-024-01989-5
Kawasaki, T., and Kawai, T. (2014). Toll-like receptor signaling pathways. Front. Immunol. 5, 461. doi:10.3389/fimmu.2014.00461
Kevekordes, S., Mersch-Sundermann, V., Burghaus, C. M., Spielberger, J., Schmeiser, H. H., Arlt, V. M., et al. (1999). SOS induction of selected naturally occurring substances in Escherichia coli (SOS chromotest). Mutat. Res. 445 (1), 81–91. doi:10.1016/s1383-5718(99)00141-2
Kevekordes, S., Spielberger, J., Burghaus, C. M., Birkenkamp, P., Zietz, B., Paufler, P., et al. (2001). Micronucleus formation in human lymphocytes and in the metabolically competent human hepatoma cell line Hep-G2: results with 15 naturally occurring substances. Anticancer Res. 21 (1a), 461–469.
Khan, A., Kalaria, R. N., Corbett, A., and Ballard, C. (2016). Update on vascular dementia. J. Geriatric Psychiatry Neurology 29 (5), 281–301. doi:10.1177/0891988716654987
Khaspekov, L. G., and Frumkina, L. E. (2023). Molecular mechanisms of astrocyte involvement in synaptogenesis and brain synaptic plasticity. Biochem. Biokhimiia 88 (4), 502–514. doi:10.1134/S0006297923040065
Kim, B. W., Koppula, S., Kumar, H., Park, J. Y., Kim, I. W., More, S. V., et al. (2015). α-Asarone attenuates microglia-mediated neuroinflammation by inhibiting NF kappa B activation and mitigates MPTP-induced behavioral deficits in a mouse model of Parkinson’s disease. Neuropharmacology 97, 46–57. doi:10.1016/j.neuropharm.2015.04.037
Kim, C. J., Kwak, T. Y., Bae, M. H., Shin, H. K., and Choi, B. T. (2022a). Therapeutic potential of active components from Acorus gramineus and Acorus tatarinowii in neurological disorders and their application in Korean medicine. J. Pharmacopuncture 25 (4), 326–343. doi:10.3831/KPI.2022.25.4.326
Kim, S. G., Liem, A., Stewart, B. C., and Miller, J. A. (1999). New studies on trans-anethole oxide and trans-asarone oxide. Carcinogenesis 20 (7), 1303–1307. doi:10.1093/carcin/20.7.1303
Kim, T. A., Syty, M. D., Wu, K., and Ge, S. (2022b). Adult hippocampal neurogenesis and its impairment in Alzheimer’s disease. Zoological Res. 43 (3), 481–496. doi:10.24272/j.issn.2095-8137.2021.479
Knox, E. G., Aburto, M. R., Clarke, G., Cryan, J. F., and O’Driscoll, C. M. (2022). The blood-brain barrier in aging and neurodegeneration. Mol. Psychiatry 27 (6), 2659–2673. doi:10.1038/s41380-022-01511-z
Koenig, M. L., and Meyerhoff, J. L. (2003). In vitro neuroprotection against oxidative stress by pre-treatment with a combination of dihydrolipoic acid and phenyl-butyl nitrones. Neurotox. Res. 5 (4), 265–272. doi:10.1007/BF03033384
Koizumi, K., Wang, G., and Park, L. (2016). Endothelial dysfunction and amyloid-β-induced neurovascular alterations. Cell. Mol. Neurobiol. 36 (2), 155–165. doi:10.1007/s10571-015-0256-9
Kosik, K. S. (1990). Tau protein and neurodegeneration. Mol. Neurobiol. 4 (3-4), 171–179. doi:10.1007/BF02780339
Krall, W. J., Sramek, J. J., and Cutler, N. R. (1999). Cholinesterase inhibitors: a therapeutic strategy for Alzheimer disease. Ann. Pharmacother. 33 (4), 441–450. doi:10.1345/aph.18211
Lam, K. Y., Chen, J., Lam, C. T., Wu, Q., Yao, P., Dong, T. T. X., et al. (2016b). Asarone from acori tatarinowii rhizoma potentiates the nerve growth factor-induced neuronal differentiation in cultured PC12 cells: a signaling mediated by protein kinase A. PLoS One 11 (9), e0163337. doi:10.1371/journal.pone.0163337
Lam, K. Y., Ku, C. F., Wang, H. Y., Chan, G. K., Yao, P., Lin, H. Q., et al. (2016a). Authentication of Acori Tatarinowii Rhizoma (Shi Chang Pu) and its adulterants by morphological distinction, chemical composition and ITS sequencing. Chin. Med. 11, 41. doi:10.1186/s13020-016-0113-x
Lam, K. Y. C., Huang, Y., Yao, P., Wang, H., Dong, T. T. X., Zhou, Z., et al. (2017a). Comparative study of different Acorus species in potentiating neuronal differentiation in cultured PC12 cells. Phytotherapy Res. PTR 31 (11), 1757–1764. doi:10.1002/ptr.5904
Lam, K. Y. C., Wu, Q. Y., Hu, W. H., Yao, P., Wang, H. Y., Dong, T. T. X., et al. (2019). Asarones from Acori Tatarinowii Rhizoma stimulate expression and secretion of neurotrophic factors in cultured astrocytes. Neurosci. Lett. 707, 134308. doi:10.1016/j.neulet.2019.134308
Lam, K. Y. C., Yao, P., Wang, H., Duan, R., Dong, T. T. X., and Tsim, K. W. K. (2017b). Asarone from Acori Tatarinowii Rhizome prevents oxidative stress-induced cell injury in cultured astrocytes: a signaling triggered by Akt activation. PLoS One 12 (6), e0179077. doi:10.1371/journal.pone.0179077
Leclercq, J., Dehaussy, H., Goblet, M. C., Wauters, J. N., and Angenot, L. (1985). Description, identification and utilization of yumel (bark of Guatteria gaumeri Greenman). J. de Pharm. de Belg. 40 (4), 251–256.
Lee, B., Sur, B., Cho, S. G., Yeom, M., Shim, I., Lee, H., et al. (2015). Effect of beta-asarone on impairment of spatial working memory and apoptosis in the Hippocampus of rats exposed to chronic corticosterone administration. Biomol. and Ther. 23 (6), 571–581. doi:10.4062/biomolther.2015.027
Leissring, M. A. (2016). Aβ-degrading proteases: therapeutic potential in alzheimer disease. CNS drugs 30 (8), 667–675. doi:10.1007/s40263-016-0364-1
Liang, S., Zhou, J., Yu, X., Lu, S., and Liu, R. (2024). Neuronal conversion from glia to replenish the lost neurons. Neural Regen. Res. 19 (7), 1446–1453. doi:10.4103/1673-5374.386400
Li, C., Xing, G., Dong, M., Zhou, L., Li, J., Wang, G., et al. (2010). Beta-asarone protection against beta-amyloid-induced neurotoxicity in PC12 cells via JNK signaling and modulation of Bcl-2 family proteins. Eur. J. Pharmacol. 635 (1-3), 96–102. doi:10.1016/j.ejphar.2010.03.013
Li, C., Zhang, L., Li, X., Hu, Q., Mao, L., Shao, Y., et al. (2024a). Sulforaphane suppresses Aβ accumulation and tau hyperphosphorylation in vascular cognitive impairment(VCI). J. Nutr. Biochem. 136, 109803. doi:10.1016/j.jnutbio.2024.109803
Li, J., Shan, X., Gao, Y., Zhu, H., Cheng, H., Xing, C., et al. (2025). Acori Tatarinowii Rhizoma regulates OCT3/OATP2 and P-gp/MRP1 to “guide medicines upwards” in Kai-Xin-San to treat Alzheimer’s disease. J. Ethnopharmacol. 343, 119484. doi:10.1016/j.jep.2025.119484
LimóN, I. D., DíAZ, A., Mendieta, L., Chamorro, G., Espinosa, B., Zenteno, E., et al. (2009b). Amyloid-beta(25-35) impairs memory and increases NO in the temporal cortex of rats. Neurosci. Res. 63 (2), 129–137. doi:10.1016/j.neures.2008.11.006
Limón, I. D., Mendieta, L., Díaz, A., Chamorro, G., Espinosa, B., Zenteno, E., et al. (2009a). Neuroprotective effect of alpha-asarone on spatial memory and nitric oxide levels in rats injected with amyloid-beta((25-35)). Neurosci. Lett. 453 (2), 98–103. doi:10.1016/j.neulet.2009.02.011
Li, Q., Xu, F., Zhang, Q., Li, X., Guo, M., Zhang, Y., et al. (2019). Effect of α-asarone on ethanol-induced learning and memory impairment in mice and its underlying mechanism. Life Sci. 238, 116898. doi:10.1016/j.lfs.2019.116898
Li, S., and Selkoe, D. J. (2020). A mechanistic hypothesis for the impairment of synaptic plasticity by soluble Aβ oligomers from Alzheimer’s brain. J. Neurochem. 154 (6), 583–597. doi:10.1111/jnc.15007
Liu, S. J., Yang, C., Zhang, Y., Su, R. Y., Chen, J. L., Jiao, M. M., et al. (2016). Neuroprotective effect of β-asarone against Alzheimer’s disease: regulation of synaptic plasticity by increased expression of SYP and GluR1. Drug Des. Dev. Ther. 10, 1461–1469. doi:10.2147/DDDT.S93559
Liu, J., Li, C., Xing, G., Zhou, L., Dong, M., Geng, Y., et al. (2010). Beta-asarone attenuates neuronal apoptosis induced by Beta amyloid in rat hippocampus. Yakugaku zasshi J. Pharm. Soc. Jpn. 130 (5), 737–746. doi:10.1248/yakushi.130.737
Liu, J., Ping, X., Sun, S. J., Yang, J., Lu, Y., and Pei, L. (2024). Safety assessment of Acori Tatarinowii Rhizoma: acute and subacute oral toxicity. Front. Pharmacol. 15, 1377876. doi:10.3389/fphar.2024.1377876
Liu, L., Fang, Y. Q., Xue, Z. F., He, Y. P., Fang, R. M., and Li, L. (2012). Beta-asarone attenuates ischemia-reperfusion-induced autophagy in rat brains via modulating JNK, p-JNK, Bcl-2 and Beclin 1. Eur. J. Pharmacol. 680 (1-3), 34–40. doi:10.1016/j.ejphar.2012.01.016
Liu, L., and Fang, Y. Q. (2011). Analysis of the distribution of β-asarone in rat hippocampus, brainstem, cortex and cerebellum with gas chromatography–mass spectrometry. GC-MS 5 (9), 1728–1734.
Liu, L., Wang, J., Shi, L., Zhang, W., Du, X., Wang, Z., et al. (2013). β-Asarone induces senescence in colorectal cancer cells by inducing lamin B1 expression. Phytomedicine Int. J. phytotherapy Phytopharm. 20 (6), 512–520. doi:10.1016/j.phymed.2012.12.008
Liu, X., Wu, Y., Huang, Q., Zou, D., Qin, W., and Chen, Z. (2015). Grouping pentylenetetrazol-induced epileptic rats according to memory impairment and MicroRNA expression profiles in the Hippocampus. PLoS One 10 (5), e0126123. doi:10.1371/journal.pone.0126123
Li, Y., Liu, D., Wang, H., Zhang, X., Lu, B., and Li, S. (2024b). The IRE1/Xbp1 axis restores ER and tissue homeostasis perturbed by excess Notch in Drosophila. Dev. Biol. 507, 11–19. doi:10.1016/j.ydbio.2023.12.007
Li, Z., Ma, J., Kuang, Z., and Jiang, Y. (2021). β-Asarone attenuates aβ-induced neuronal damage in PC12 cells overexpressing APPswe by restoring autophagic flux. Front. Pharmacol. 12, 701635. doi:10.3389/fphar.2021.701635
Li, Z., Zhao, G., Qian, S., Yang, Z., Chen, X., Chen, J., et al. (2012). Cerebrovascular protection of β-asarone in Alzheimer’s disease rats: a behavioral, cerebral blood flow, biochemical and genic study. J. Ethnopharmacol. 144 (2), 305–312. doi:10.1016/j.jep.2012.09.013
Lonze, B. E., and Ginty, D. D. (2002). Function and regulation of CREB family transcription factors in the nervous system. Neuron 35 (4), 605–623. doi:10.1016/s0896-6273(02)00828-0
Lu, J., Fu, T., Qian, Y., Zhang, Q., Zhu, H., Pan, L., et al. (2014). Distribution of α-asarone in brain following three different routes of administration in rats. Eur. J. Pharm. Sci. Official J. Eur. Fed. Pharm. Sci. 63, 63–70. doi:10.1016/j.ejps.2014.06.006
Luo, L., Hu, Q., Yan, R., Gao, X., Zhang, D., Yan, Y., et al. (2025). Alpha-Asarone ameliorates neuronal injury after ischemic stroke and hemorrhagic transformation by attenuating blood-brain barrier destruction, promoting neurogenesis, and inhibiting neuroinflammation. Mol. Neurobiol. 62 (4), 5252–5272. doi:10.1007/s12035-024-04596-5
Manikandan, S., and Devi, R. S. (2005). Antioxidant property of alpha-asarone against noise-stress-induced changes in different regions of rat brain. Pharmacol. Res. 52 (6), 467–474. doi:10.1016/j.phrs.2005.07.007
Mao, J., Huang, S., Liu, S., Feng, X. L., Yu, M., Liu, J., et al. (2015). A herbal medicine for Alzheimer’s disease and its active constituents promote neural progenitor proliferation. Aging Cell 14 (5), 784–796. doi:10.1111/acel.12356
Marczewska, J., Drozd, E., Anuszewska, E., Chilmonczyk, Z., and Łozowicka, B. (2013). Assessment of the genotoxic activity of alpha-asarone and its derivatives in the comet assay. Acta Pol. Pharm. 70 (2), 349–354.
Maseehullah, M. D., Zakir, M., Anas, M., and Kazmi, M. H. (2022). Ethno-pharmacology of asaroon (Asarum europaeum L.) with special reference to unani system of medicine. J. Complementary and Integr. Med. 19 (2), 181–192. doi:10.1515/jcim-2021-0021
Mattson, M. P. (2004). Pathways towards and away from Alzheimer’s disease. Nature 430 (7000), 631–639. doi:10.1038/nature02621
Mcalpine, C. S., Park, J., Griciuc, A., Kim, E., Choi, S. H., Iwamoto, Y., et al. (2021). Astrocytic interleukin-3 programs microglia and limits Alzheimer’s disease. Nature 595 (7869), 701–706. doi:10.1038/s41586-021-03734-6
Meng, F., Guo, J., Zhang, Q., Song, B., and Zhang, G. (2003). Autophosphorylated calcium/calmodulin-dependent protein kinase II alpha (CaMKII alpha) reversibly targets to and phosphorylates N-methyl-D-aspartate receptor subunit 2B (NR2B) in cerebral ischemia and reperfusion in hippocampus of rats. Brain Res. 967 (1-2), 161–169. doi:10.1016/s0006-8993(02)04267-1
Meng, H., Zhou, J., Wang, M., Zheng, M., Xing, Y., and Wang, Y. (2023). SARS-CoV-2 papain-like protease negatively regulates the NLRP3 inflammasome pathway and pyroptosis by reducing the oligomerization and ubiquitination of ASC. Microorganisms 11 (11), 2799. doi:10.3390/microorganisms11112799
Meng, M., Zhang, L., Ai, D., Wu, H., and Peng, W. (2021). β-Asarone ameliorates β-amyloid-induced neurotoxicity in PC12 cells by activating P13K/Akt/Nrf2 signaling pathway. Front. Pharmacol. 12, 659955. doi:10.3389/fphar.2021.659955
Merino-GaláN, L., Jimenez-Urbieta, H., Zamarbide, M., Rodríguez-Chinchilla, T., Belloso-Iguerategui, A., Santamaria, E., et al. (2022). Striatal synaptic bioenergetic and autophagic decline in premotor experimental parkinsonism. Brain A J. Neurology 145 (6), 2092–2107. doi:10.1093/brain/awac087
Messaoudi, E., Kanhema, T., Soulé, J., Tiron, A., Dagyte, G., da Silva, B., et al. (2007). Sustained Arc/Arg3.1 synthesis controls long-term potentiation consolidation through regulation of local actin polymerization in the dentate gyrus in vivo. J. Neurosci. Official J. Soc. Neurosci. 27 (39), 10445–10455. doi:10.1523/JNEUROSCI.2883-07.2007
Mijalkov, M., Volpe, G., Fernaud-Espinosa, I., DeFelipe, J., Pereira, J. B., and Merino-Serrais, P. (2021). Dendritic spines are lost in clusters in Alzheimer’s disease. Sci. Rep. 11 (1), 12350. doi:10.1038/s41598-021-91726-x
Mikami, M., Takuya, O., Yoshino, Y., Nakamura, S., Ito, K., Kojima, H., et al. (2021). Acorus calamus extract and its component α-asarone attenuate murine hippocampal neuronal cell death induced by l-glutamate and tunicamycin. Biosci. Biotechnol. Biochem. 85 (3), 493–501. doi:10.1093/bbb/zbaa071
Mitra, S., Turconi, G., Darreh-Shori, T., Mätlik, K., Aquilino, M., Eriksdotter, M., et al. (2021). Increased endogenous GDNF in mice protects against age-related decline in neuronal cholinergic markers. Front. Aging Neurosci. 13, 714186. doi:10.3389/fnagi.2021.714186
Morales-RamíREZ, P., Madrigal-Bujaidar, E., Mercader-MartíNEZ, J., Cassini, M., González, G., Chamorro-Cevallos, G., et al. (1992). Sister-chromatid exchange induction produced by in vivo and in vitro exposure to alpha-asarone. Mutat. Res. 279 (4), 269–273. doi:10.1016/0165-1218(92)90243-s
Moreno-Ortega, A. J., Buendia, I., Mouhid, L., Egea, J., Lucea, S., Ruiz-Nuño, A., et al. (2015). CALHM1 and its polymorphism P86L differentially control Ca2+homeostasis, mitogen-activated protein kinase signaling, and cell vulnerability upon exposure to amyloid β. Aging Cell 14 (6), 1094–1102. doi:10.1111/acel.12403
Nichols, E., Steinmetz, J. D., Vollset, S. E., Fukutaki, K., Chalek, J., Abd-Allah, F., et al. (2022). Estimation of the global prevalence of dementia in 2019 and forecasted prevalence in 2050: an analysis for the Global Burden of Disease Study 2019. Lancet Public health 7 (2), e105–e125. doi:10.1016/S2468-2667(21)00249-8
Ning, B., Deng, M., Zhang, Q., Wang, N., and Fang, Y. (2016). β-Asarone inhibits IRE1/XBP1 endoplasmic reticulum stress pathway in 6-OHDA-induced parkinsonian rats. Neurochem. Res. 41 (8), 2097–2101. doi:10.1007/s11064-016-1922-0
Ning, B., Zhang, Q., Wang, N., Deng, M., and Fang, Y. (2019). β-Asarone regulates ER stress and autophagy via inhibition of the PERK/CHOP/Bcl-2/Beclin-1 pathway in 6-OHDA-induced parkinsonian rats. Neurochem. Res. 44 (5), 1159–1166. doi:10.1007/s11064-019-02757-w
Ning, Z., Zhong, X., Wu, Y., Wang, Y., Hu, D., Wang, K., et al. (2024). β-asarone improves cognitive impairment and alleviates autophagy in mice with vascular dementia via the cAMP/PKA/CREB pathway. Phytomedicine Int. J. Phytotherapy Phytopharm. 123, 155215. doi:10.1016/j.phymed.2023.155215
Orgogozo, J. M., Gilman, S., Dartigues, J. F., Laurent, B., Puel, M., Kirby, L. C., et al. (2003). Subacute meningoencephalitis in a subset of patients with AD after Abeta42 immunization. Neurology 61 (1), 46–54. doi:10.1212/01.wnl.0000073623.84147.a8
Ozarowski, A. (1956). Asarum europaeum L. in the light of phytochemical, toxicological, pharmacodynamic and clinical investigations. Die Pharm. 11 (1), 63–69.
Pagani, L., and Eckert, A. (2011). Amyloid-Beta interaction with mitochondria. Int. J. Alzheimer’s Dis. 2011, 925050. doi:10.4061/2011/925050
Pan, H., Xu, Y., Cai, Q., Wu, M., and Ding, M. (2021). Effects of β-asarone on ischemic stroke in middle cerebral artery occlusion rats by an nrf2-antioxidant response elements (ARE) pathway-dependent mechanism. Med. Sci. Monit. Int. Med. J. Exp. Clin. Res. 27, e931884. doi:10.12659/MSM.931884
Pan, L., Li, C., Meng, L., Tian, Y., He, M., Yuan, X., et al. (2022). Tau accelerates α-synuclein aggregation and spreading in Parkinson’s disease. Brain A J. neurology 145 (10), 3454–3471. doi:10.1093/brain/awac171
Pan, L., Zhou, J., Ju, F., and Zhu, H. (2018). Intranasal delivery of α-asarone to the brain with lactoferrin-modified mPEG-PLA nanoparticles prepared by premix membrane emulsification. Drug Deliv. Transl. Res. 8 (1), 83–96. doi:10.1007/s13346-017-0438-8
Park, S. H., Kang, M. K., Choi, Y. J., Kim, Y. H., Antika, L. D., Lim, S. S., et al. (2016). Dietary compound α-asarone alleviates ER stress-mediated apoptosis in 7β-hydroxycholesterol-challenged macrophages. Mol. Nutr. and Food Res. 60 (5), 1033–1047. doi:10.1002/mnfr.201500750
Phillips, E. C., Croft, C. L., Kurbatskaya, K., O’Neill, M. J., Hutton, M. L., Hanger, D. P., et al. (2014). Astrocytes and neuroinflammation in Alzheimer’s disease. Biochem. Soc. Trans. 42 (5), 1321–1325. doi:10.1042/BST20140155
Pinton, P., Giorgi, C., Siviero, R., Zecchini, E., and Rizzuto, R. (2008). Calcium and apoptosis: ER-mitochondria Ca2+ transfer in the control of apoptosis. Oncogene 27 (50), 6407–6418. doi:10.1038/onc.2008.308
Piscopo, P., Crestini, A., Carbone, E., Rivabene, R., Ancidoni, A., Lo Giudice, M., et al. (2022). A systematic review on drugs for synaptic plasticity in the treatment of dementia. Ageing Res. Rev. 81, 101726. doi:10.1016/j.arr.2022.101726
Qian, Y. Y., Lu, J., Zhang, L. H., Shi, F. y., Fu, T. m., and Guo, L. w. (2015). Pharmacokinetic study on dry powder inhalation administration of α-asarone in rats. Zhongguo Zhong yao za zhi = Zhongguo zhongyao zazhi = China J. Chin. Materia Medica 40 (4), 739–743. doi:10.4268/cjcmm20150430
Qin, Y., and Zhao, W. (2023). Posttranslational modifications of NLRP3 and their regulatory roles in inflammasome activation. Eur. J. Immunol. 53 (10), e2350382. doi:10.1002/eji.202350382
Querfurth, H. W., Jiang, J., Geiger, J. D., and Selkoe, D. J. (1997). Caffeine stimulates amyloid beta-peptide release from beta-amyloid precursor protein-transfected HEK293 cells. J. Neurochem. 69 (4), 1580–1591. doi:10.1046/j.1471-4159.1997.69041580.x
Radak, Z., Zhao, Z., Goto, S., and Koltai, E. (2011). Age-associated neurodegeneration and oxidative damage to lipids, proteins and DNA. Mol. Aspects Med. 32 (4-6), 305–315. doi:10.1016/j.mam.2011.10.010
Raghunath, A., Sundarraj, K., Nagarajan, R., Arfuso, F., Bian, J., Kumar, A. P., et al. (2018). Antioxidant response elements: discovery, classes, regulation and potential applications. Redox Biol. 17, 297–314. doi:10.1016/j.redox.2018.05.002
Rajesh, Y., and Kanneganti, T. D. (2022). Innate immune cell death in neuroinflammation and Alzheimer’s disease. Cells 11 (12), 1885. doi:10.3390/cells11121885
Raz, L., Knoefel, J., and Bhaskar, K. (2016). The neuropathology and cerebrovascular mechanisms of dementia. J. Cereb. Blood Flow Metabolism Official J. Int. Soc. Cereb. Blood Flow Metabolism 36 (1), 172–186. doi:10.1038/jcbfm.2015.164
Redza-Dutordoir, M., and Averill-Bates, D. A. (2021). Interactions between reactive oxygen species and autophagy: special issue: death mechanisms in cellular homeostasis. Biochimica Biophysica Acta Mol. Cell Res. 1868 (8), 119041. doi:10.1016/j.bbamcr.2021.119041
Rickle, A., Bogdanovic, N., Volkman, I., Winblad, B., Ravid, R., and Cowburn, R. F. (2004). Akt activity in Alzheimer’s disease and other neurodegenerative disorders. Neuroreport 15 (6), 955–959. doi:10.1097/00001756-200404290-00005
Rochefort, N. L., and Konnerth, A. (2012). Dendritic spines: from structure to in vivo function. EMBO Rep. 13 (8), 699–708. doi:10.1038/embor.2012.102
Roda, A. R., Serra-Mir, G., Montoliu-Gaya, L., Tiessler, L., and Villegas, S. (2022). Amyloid-beta peptide and tau protein crosstalk in Alzheimer’s disease. Neural Regen. Res. 17 (8), 1666–1674. doi:10.4103/1673-5374.332127
Ryter, S. W. (2021). Heme oxgenase-1, a cardinal modulator of regulated cell death and inflammation. Cells 10 (3), 515. doi:10.3390/cells10030515
Saki, G., Eidi, A., Mortazavi, P., Panahi, N., and Vahdati, A. (2020). Effect of β-asarone in normal and β-amyloid-induced Alzheimeric rats. Archives Med. Sci. AMS 16 (3), 699–706. doi:10.5114/aoms.2020.94659
Salazar, M., Salazar, S., Ulloa, V., Mendoza, T., Pages, N., and Chamoro, G. (1992). Teratogenic action of alpha-asarone in the mouse. J. de Toxicol. Clinique Exp. 12 (3), 149–154.
Salminen, A., Kaarniranta, K., Kauppinen, A., Ojala, J., Haapasalo, A., Soininen, H., et al. (2013). Impaired autophagy and APP processing in Alzheimer’s disease: the potential role of Beclin 1 interactome. Prog. Neurobiol. 106-107, 33–54. doi:10.1016/j.pneurobio.2013.06.002
Sandeep, D., and Nair, C. K. (2011). Radioprotection by α-asarone: prevention of genotoxicity and hematopoietic injury in mammalian organism. Mutat. Res. 722 (1), 62–68. doi:10.1016/j.mrgentox.2011.03.005
Sanderson, D. J., Good, M. A., Seeburg, P. H., Sprengel, R., Rawlins, J. N. P., and Bannerman, D. M. (2008). The role of the GluR-A (GluR1) AMPA receptor subunit in learning and memory. Prog. Brain Res. 169, 159–178. doi:10.1016/S0079-6123(07)00009-X
Sarkar, S., Raymick, J., Mann, D., Bowyer, J. F., Hanig, J. P., Schmued, L. C., et al. (2014). Neurovascular changes in acute, sub-acute and chronic mouse models of Parkinson’s disease. Curr. Neurovascular Res. 11 (1), 48–61. doi:10.2174/1567202610666131124234506
Sarnat, H. B., and Born, D. E. (1999). Synaptophysin immunocytochemistry with thermal intensification: a marker of terminal axonal maturation in the human fetal nervous system. Brain and Dev. 21 (1), 41–50. doi:10.1016/s0387-7604(98)00068-0
Schwartz, M., and Ziv, Y. (2008). Immunity to self and self-maintenance: a unified theory of brain pathologies. Trends Immunol. 29 (5), 211–219. doi:10.1016/j.it.2008.01.003
Seib, D. R., Corsini, N. S., Ellwanger, K., Plaas, C., Mateos, A., Pitzer, C., et al. (2013). Loss of Dickkopf-1 restores neurogenesis in old age and counteracts cognitive decline. Cell Stem Cell 12 (2), 204–214. doi:10.1016/j.stem.2012.11.010
Senft, D., and Ronai, Z. A. (2015). UPR, autophagy, and mitochondria crosstalk underlies the ER stress response. Trends Biochem. Sci. 40 (3), 141–148. doi:10.1016/j.tibs.2015.01.002
Sengupta, U., and Kayed, R. (2022). Amyloid β, Tau, and α-Synuclein aggregates in the pathogenesis, prognosis, and therapeutics for neurodegenerative diseases. Prog. Neurobiol. 214, 102270. doi:10.1016/j.pneurobio.2022.102270
Shi, Y. S., Zhang, Y., Liu, B., Li, C. B., and Wu, J. (2019). Nomilin protects against cerebral ischemia-reperfusion induced neurological deficits and blood-brain barrier disruption via the Nrf2 pathway. Food and Funct. 10 (9), 5323–5332. doi:10.1039/c9fo01481k
Shi, J., Li, R., Yang, Y., Ji, L., and Li, C. (2021). Protective effect of α-asarone and β-asarone on Aβ -induced inflammatory response in PC12 cells and its. Zhejiang da xue xue bao Yi xue ban = J. Zhejiang Univ. Med. Sci. 50 (5), 591–600. doi:10.3724/zdxbyxb-2021-0162
Shi, R., Zhang, S., Cheng, G., Yang, X., Zhao, N., and Chen, C. (2018). Ginsenoside Rg1 and acori graminei rhizoma attenuates neuron cell apoptosis by promoting the expression of miR-873-5p in alzheimer’s disease. Neurochem. Res. 43 (8), 1529–1538. doi:10.1007/s11064-018-2567-y
Shi, Y., Vattem, K. M., Sood, R., An, J., Liang, J., Stramm, L., et al. (1998). Identification and characterization of pancreatic eukaryotic initiation factor 2 alpha-subunit kinase, PEK, involved in translational control. Mol. Cell. Biol. 18 (12), 7499–7509. doi:10.1128/mcb.18.12.7499
Snyder, B., Shell, B., Cunningham, J. T., and Cunningham, R. L. (2017). Chronic intermittent hypoxia induces oxidative stress and inflammation in brain regions associated with early-stage neurodegeneration. Physiol. Rep. 5 (9), e13258. doi:10.14814/phy2.13258
Sohrabi, T., Mirzaei-Behbahani, B., Zadali, R., Pirhaghi, M., Morozova-Roche, L. A., and Meratan, A. A. (2023). Common mechanisms underlying α-synuclein-induced mitochondrial dysfunction in Parkinson’s disease. J. Mol. Biol. 435 (12), 167992. doi:10.1016/j.jmb.2023.167992
Song, J., and Kim, Y. K. (2017). Identification of the role of miR-142-5p in alzheimer’s disease by comparative bioinformatics and cellular analysis. Front. Mol. Neurosci. 10, 227. doi:10.3389/fnmol.2017.00227
Speranza, L., Di Porzio, U., Viggiano, D., de Donato, A., and Volpicelli, F. (2021). Dopamine: the neuromodulator of long-term synaptic plasticity, reward and movement control. Cells 10 (4), 735. doi:10.3390/cells10040735
Stassart, R. M., MöBIUS, W., Nave, K. A., and Edgar, J. M. (2018). The axon-myelin unit in development and degenerative disease. Front. Neurosci. 12, 467. doi:10.3389/fnins.2018.00467
Sun, X. Y., Li, L. J., Dong, Q. X., Zhu, J., Huang, Y. R., Hou, S. J., et al. (2021). Rutin prevents tau pathology and neuroinflammation in a mouse model of Alzheimer’s disease. J. Neuroinflammation 18 (1), 131. doi:10.1186/s12974-021-02182-3
Sun, Y. R., Wang, X. Y., Li, S. S., Dong, H. Y., and Zhang, X. J. (2015). β-asarone from Acorus gramineus alleviates depression by modulating MKP-1. Genet. Mol. Res. GMR 14 (2), 4495–4504. doi:10.4238/2015.May.4.7
Sun, D., Wu, L., Lan, S., Chi, X., and Wu, Z. (2024). β-asarone induces viability and angiogenesis and suppresses apoptosis of human vascular endothelial cells after ischemic stroke by upregulating vascular endothelial growth factor A. PeerJ 12, e17534. doi:10.7717/peerj.17534
Thal, D. R. (2009). The pre-capillary segment of the blood-brain barrier and its relation to perivascular drainage in Alzheimer’s disease and small vessel disease. The Scientific World J. 9, 557–563. doi:10.1100/tsw.2009.72
Todd, D. J., Lee, A. H., and Glimcher, L. H. (2008). The endoplasmic reticulum stress response in immunity and autoimmunity. Nat. Rev. Immunol. 8 (9), 663–674. doi:10.1038/nri2359
Trenti, A., Grumati, P., Cusinato, F., Orso, G., Bonaldo, P., and Trevisi, L. (2014). Cardiac glycoside ouabain induces autophagic cell death in non-small cell lung cancer cells via a JNK-dependent decrease of Bcl-2. Biochem. Pharmacol. 89 (2), 197–209. doi:10.1016/j.bcp.2014.02.021
Uebel, T., Hermes, L., Haupenthal, S., Müller, L., and Esselen, M. (2021). α-Asarone, β-asarone, and γ-asarone: current status of toxicological evaluation. J. Appl. Toxicol. JAT 41 (8), 1166–1179. doi:10.1002/jat.4112
Vasic, V., Barth, K., and Schmidt, M. H. H. (2019). Neurodegeneration and neuro-regeneration-alzheimer’s disease and stem cell therapy. Int. J. Mol. Sci. 20 (17), 4272. doi:10.3390/ijms20174272
Venkatesan, K. (2022). Anti-amnesic and anti-cholinesterase activities of α-asarone against scopolamine-induced memory impairments in rats. Eur. Rev. Med. Pharmacol. Sci. 26 (17), 6344–6350. doi:10.26355/eurrev_202209_29660
Ventriglia, M., Zanardini, R., Bonomini, C., Zanetti, O., Volpe, D., Pasqualetti, P., et al. (2013). Serum brain-derived neurotrophic factor levels in different neurological diseases. BioMed Res. Int. 2013, 901082. doi:10.1155/2013/901082
Vincow, E. S., Merrihew, G., Thomas, R. E., Shulman, N. J., Beyer, R. P., MacCoss, M. J., et al. (2013). The PINK1-Parkin pathway promotes both mitophagy and selective respiratory chain turnover in vivo. Proc. Natl. Acad. Sci. U. S. A. 110 (16), 6400–6405. doi:10.1073/pnas.1221132110
Wang, C. S., Kavalali, E. T., and Monteggia, L. M. (2022b). BDNF signaling in context: from synaptic regulation to psychiatric disorders. Cell 185 (1), 62–76. doi:10.1016/j.cell.2021.12.003
Wang, C., Song, P., and Niu, Y. (2022a). The management of dementia worldwide: a review on policy practices, clinical guidelines, end-of-life care, and challenge along with aging population. Biosci. Trends 16 (2), 119–129. doi:10.5582/bst.2022.01042
Wang, G., Li, Y., Liu, J., Yuan, Y., Shen, Z., and Mei, X. (2017). Ultrasensitive multiplexed immunoassay of autophagic biomarkers based on Au/rGO and Au nanocages amplifying electrochemcial signal. Sci. Rep. 7 (1), 2442. doi:10.1038/s41598-017-02766-1
Wang, M., Tang, H. P., Wang, S., Hu, W. J., Li, J. Y., Yu, A. Q., et al. (2023). Acorus tatarinowii schott: a review of its botany, traditional uses, phytochemistry, and pharmacology. Mol. (Basel, Switz.) 28 (11), 4525. doi:10.3390/molecules28114525
Wang, N., Wang, H., Li, L., Li, Y., and Zhang, R. (2019). β-Asarone inhibits amyloid-β by promoting autophagy in a cell model of Alzheimer’s disease. Front. Pharmacol. 10, 1529. doi:10.3389/fphar.2019.01529
Wang, Y., and Zhang, H. (2019). Regulation of autophagy by mTOR signaling pathway. Adv. Exp. Med. Biol. 1206, 67–83. doi:10.1007/978-981-15-0602-4_3
Wang, Z., Huang, P. E., Wang, N., Zhang, Q., Kang, J., Fang, Y., et al. (2024). β-asarone inhibits autophagy by activating the PI3K/Akt/mTOR pathway in a rat model of depression in Parkinson’s disease. Behav. Brain Res. 465, 114966. doi:10.1016/j.bbr.2024.114966
Wang, Z., Wang, Q., Yang, B., Li, J., Yang, C., Meng, Y., et al. (2014). GC-MS method for determination and pharmacokinetic study of four phenylpropanoids in rat plasma after oral administration of the essential oil of Acorus tatarinowii Schott rhizomes. J. Ethnopharmacol. 155 (2), 1134–1140. doi:10.1016/j.jep.2014.06.035
Wei, G., Chen, Y. B., Chen, D. F., Lai, X. P., Liu, D. H., Deng, R. D., et al. (2013). β-Asarone inhibits neuronal apoptosis via the CaMKII/CREB/Bcl-2 signaling pathway in an in vitro model and AβPP/PS1 mice. J. Alzheimer’s Dis. JAD 33 (3), 863–880. doi:10.3233/JAD-2012-120865
Wen, J., Yang, Y., and Hao, J. (2023). Acori Tatarinowii Rhizoma: a comprehensive review of its chemical composition, pharmacology, pharmacokinetics and toxicity. Front. Pharmacol. 14, 1090526. doi:10.3389/fphar.2023.1090526
Winslow, A. R., and Rubinsztein, D. C. (2011). The Parkinson disease protein α-synuclein inhibits autophagy. Autophagy 7 (4), 429–431. doi:10.4161/auto.7.4.14393
Wiseman, R. W., Miller, E. C., Miller, J. A., and Liem, A. (1987). Structure-activity studies of the hepatocarcinogenicities of alkenylbenzene derivatives related to estragole and safrole on administration to preweanling male C57BL/6J x C3H/HeJ F1 mice. Cancer Res. 47 (9), 2275–2283. doi:10.1016/0378-8741(88)90255-3
Wu, H. B., and Fang, Y. Q. (2004). Pharmacokinetics of beta-asarone in rats. Yao xue xue bao = Acta Pharm. Sin. 39 (10), 836–838.
Wu, J. Y., Li, Y. J., Yang, L., Hu, Y. Y., Hu, X. B., Tang, T. T., et al. (2018). Borneol and Α-asarone as adjuvant agents for improving blood-brain barrier permeability of puerarin and tetramethylpyrazine by activating adenosine receptors. Drug Deliv. 25 (1), 1858–1864. doi:10.1080/10717544.2018.1516005
Xiao, X., Xu, X., Li, F., Xie, G., and Zhang, T. (2019). Anti-inflammatory treatment with β-asarone improves impairments in social interaction and cognition in MK-801 treated mice. Brain Res. Bull. 150, 150–159. doi:10.1016/j.brainresbull.2019.05.017
Xiao, Y., Fu, H., Han, X., Hu, X., Gu, H., Chen, Y., et al. (2014). Role of synaptic structural plasticity in impairments of spatial learning and memory induced by developmental lead exposure in Wistar rats. PLoS One 9 (12), e115556. doi:10.1371/journal.pone.0115556
Xiong, W., Zhao, X., Xu, Q., Wei, G., Zhang, L., Fan, Y., et al. (2022). Qisheng Wan formula ameliorates cognitive impairment of Alzheimer’s disease rat via inflammation inhibition and intestinal microbiota regulation. J. Ethnopharmacol. 282, 114598. doi:10.1016/j.jep.2021.114598
Xue, Z., Guo, Y., Zhang, S., Huang, L., He, Y., Fang, R., et al. (2014). Beta-asarone attenuates amyloid beta-induced autophagy via Akt/mTOR pathway in PC12 cells. Eur. J. Pharmacol. 741, 195–204. doi:10.1016/j.ejphar.2014.08.006
Xu, Z., Zhou, X., Hong, X., Wang, S., Wei, J., Huang, J., et al. (2023). Essential oil of Acorus tatarinowii Schott inhibits neuroinflammation by suppressing NLRP3 inflammasome activation in 3 × Tg-AD transgenic mice. Phytomedicine Int. J. Phytotherapy Phytopharm. 112, 154695. doi:10.1016/j.phymed.2023.154695
Yang, Q. Q., Xue, W. Z., Zou, R. X., Xu, Y., Du, Y., Wang, S., et al. (2016a). β-Asarone rescues Pb-induced impairments of spatial memory and synaptogenesis in rats. PLoS One 11 (12), e0167401. doi:10.1371/journal.pone.0167401
Yang, X. X., Wu, Z. Y., Yang, Y., Zhang, C., Lin, X. F., Zhou, L., et al. (2021b). Sevoflurane inhalation has a cognitive impairing effect of aging rats involving the regulation of AChE and ChAT. Ibrain 7 (3), 192–199. doi:10.1002/j.2769-2795.2021.tb00083.x
Yang, Y. X., Chen, Y. T., Zhou, X. J., Hong, C. L., Li, C. Y., and Guo, J. Y. (2013). Beta-asarone, a major component of Acorus tatarinowii Schott, attenuates focal cerebral ischemia induced by middle cerebral artery occlusion in rats. BMC Complementary Altern. Med. 13, 236. doi:10.1186/1472-6882-13-236
Yang, C., Li, X., Mo, Y., Liu, S., Zhao, L., Ma, X., et al. (2016b). β-Asarone mitigates amyloidosis and downregulates RAGE in a transgenic mouse model of Alzheimer’s disease. Cell. Mol. Neurobiol. 36 (1), 121–130. doi:10.1007/s10571-015-0226-2
Yang, G., Zhou, R., Guo, X., Yan, C., Lei, J., and Shi, Y. (2021a). Structural basis of γ-secretase inhibition and modulation by small molecule drugs. Cell 184 (2), 521–533.e14. doi:10.1016/j.cell.2020.11.049
Yang, Y., Xuan, L., Chen, H., Dai, S., Ji, L., Bao, Y., et al. (2017). Neuroprotective effects and mechanism of β-asarone against aβ1-42-induced injury in astrocytes. Evidence-based complementary Altern. Med. eCAM 2017, 8516518. doi:10.1155/2017/8516518
Yang, Z., Zou, Y., Wang, L., Zeng, J., Dai, H., and Ding, X. (2023). Comprehensive analysis of NAC transcription factors reveals their evolution in malvales and functional characterization of AsNAC019 and AsNAC098 in Aquilaria sinensis. Int. J. Mol. Sci. 24 (4), 17384. doi:10.3390/ijms242417384
Yan, L., Liu, Z., Xu, L., Qian, Y., Song, P., and Wei, M. (2020a). Identification of volatile active components in Acori tatarinowii Rhizome essential oil from different regions in China by C6 glioma cells. BMC Complementary Med. Ther. 20 (1), 255. doi:10.1186/s12906-020-03020-4
Yan, L., Mahady, G., Qian, Y., Song, P., Jian, T., Ding, X., et al. (2020b). The essential oil from acori tatarinowii rhizome (the dried rhizome of Acorus tatarinowii schott) prevents hydrogen peroxide-induced cell injury in PC12 cells: a signaling triggered by CREB/PGC-1α activation. Evidence-Based Complementary Altern. Med. eCAM 2020, 4845028. doi:10.1155/2020/4845028
Yan, X., Liu, J., Ye, Z., Huang, J., He, F., Xiao, W., et al. (2016). CaMKII-mediated CREB phosphorylation is involved in Ca2+-induced BDNF mRNA transcription and neurite outgrowth promoted by electrical stimulation. PLoS One 11 (9), e0162784. doi:10.1371/journal.pone.0162784
Yu, H., Lin, L., Zhang, Z., Zhang, H., and Hu, H. (2020). Targeting NF-κB pathway for the therapy of diseases: mechanism and clinical study. Signal Transduct. Target. Ther. 5 (1), 209. doi:10.1038/s41392-020-00312-6
Yu, W., Li, Y., Hu, J., Li, H. M., and Kuo, H. C. (2022). A study on the pathogenesis of vascular cognitive impairment and dementia: the chronic cerebral hypoperfusion hypothesis. J. Clin. Med. 11 (16), 6221. doi:10.3390/jcm11206221
Zeng, L., Zhang, D., Liu, Q., Zhang, J., Mu, K., Gao, X., et al. (2021). Alpha-asarone improves cognitive function of APP/PS1 mice and reducing aβ(42,) P-tau and neuroinflammation, and promoting neuron survival in the Hippocampus. Neuroscience 458, 141–152. doi:10.1016/j.neuroscience.2020.12.026
Zeydan, B., Deelchand, D. K., Tosakulwong, N., Lesnick, T. G., Kantarci, O. H., Machulda, M. M., et al. (2017). Decreased glutamate levels in patients with amnestic mild cognitive impairment: an sLASER proton MR spectroscopy and PiB-PET study. J. Neuroimaging Official J. Am. Soc. Neuroimaging 27 (6), 630–636. doi:10.1111/jon.12454
Zhang, Q. S., Wang, Z. H., Zhang, J. L., Duan, Y. L., Li, G. F., and Zheng, D. L. (2016a). Beta-asarone protects against MPTP-induced Parkinson’s disease via regulating long non-coding RNA MALAT1 and inhibiting α-synuclein protein expression. Biomed. and Pharmacother. = Biomedecine and Pharmacother. 83, 153–159. doi:10.1016/j.biopha.2016.06.017
Zhang, H., Gao, Z., Zhang, Y., Wang, H., and Li, Y. (2018). MiR-873-5p regulated LPS-induced oxidative stress via targeting heme oxygenase-1 (HO-1) in KGN cells. RSC Adv. 8 (68), 39098–39105. doi:10.1039/c8ra06697c
Zhang, K., Liu, Q., Luo, L., Feng, X., Hu, Q., Fan, X., et al. (2021a). Neuroprotective effect of alpha-asarone on the rats model of cerebral ischemia-reperfusion stroke via ameliorating glial activation and autophagy. Neuroscience 473, 130–141. doi:10.1016/j.neuroscience.2021.08.006
Zhang, S., Gui, X. H., Huang, L. P., Deng, M. Z., Fang, R. M., Ke, X. H., et al. (2016b). Neuroprotective effects of β-asarone against 6-hydroxy dopamine-induced parkinsonism via JNK/Bcl-2/Beclin-1 pathway. Mol. Neurobiol. 53 (1), 83–94. doi:10.1007/s12035-014-8950-z
Zhang, Y., Long, Y., Yu, S., Li, D., Yang, M., Guan, Y., et al. (2021b). Natural volatile oils derived from herbal medicines: a promising therapy way for treating depressive disorder. Pharmacol. Res. 164, 105376. doi:10.1016/j.phrs.2020.105376
Zhang, Y., Zhang, Y., Jin, X. F., Zhou, X. H., Dong, X. H., Yu, W. T., et al. (2019). The role of astragaloside IV against cerebral ischemia/reperfusion injury: suppression of apoptosis via promotion of P62-LC3-autophagy. Mol. (Basel, Switz.) 24 (9), 1838. doi:10.3390/molecules24091838
Zhang, Z., Yu, Z., Yuan, Y., Yang, J., Wang, S., Ma, H., et al. (2023). Cholecystokinin signaling can rescue cognition and synaptic plasticity in the APP/PS1 mouse model of Alzheimer’s disease. Mol. Neurobiol. 60 (9), 5067–5089. doi:10.1007/s12035-023-03388-7
Zhan, X., Li, Q., Xu, G., Xiao, X., and Bai, Z. (2022). The mechanism of NLRP3 inflammasome activation and its pharmacological inhibitors. Front. Immunol. 13, 1109938. doi:10.3389/fimmu.2022.1109938
Zhao, C., Deng, W., and Gage, F. H. (2008). Mechanisms and functional implications of adult neurogenesis. Cell 132 (4), 645–660. doi:10.1016/j.cell.2008.01.033
Zheng, J. (2022). Hippocampal neurogenesis and pro-neurogenic therapies for Alzheimer’s disease. Animal models Exp. Med. 5 (1), 3–14. doi:10.1002/ame2.12212
Zhou, C., Zhao, L., Zheng, J., Wang, K., Deng, H., Liu, P., et al. (2017). MicroRNA-144 modulates oxidative stress tolerance in SH-SY5Y cells by regulating nuclear factor erythroid 2-related factor 2-glutathione axis. Neurosci. Lett. 655, 21–27. doi:10.1016/j.neulet.2017.06.045
Zhuo, M. (2024). Long-term plasticity of NMDA GluN2B (NR2B) receptor in anterior cingulate cortical synapses. Mol. Pain 20, 17448069241230258. doi:10.1177/17448069241230258
Zinszner, H., Kuroda, M., Wang, X., Batchvarova, N., Lightfoot, R. T., Remotti, H., et al. (1998). CHOP is implicated in programmed cell death in response to impaired function of the endoplasmic reticulum. Genes and Dev. 12 (7), 982–995. doi:10.1101/gad.12.7.982
Ziv, Y., and Schwartz, M. (2008). Orchestrating brain-cell renewal: the role of immune cells in adult neurogenesis in health and disease. Trends Mol. Med. 14 (11), 471–478. doi:10.1016/j.molmed.2008.09.004
Keywords: Acori tatarinowii rhizoma, α-asarone, β-asarone, dementia, cognitive functions
Citation: Bu Y, Li S, Ye T, Wang Y, Song M and Chen J (2025) Volatile oil of Acori tatarinowii rhizoma: potential candidate drugs for mitigating dementia. Front. Pharmacol. 16:1552801. doi: 10.3389/fphar.2025.1552801
Received: 29 December 2024; Accepted: 08 April 2025;
Published: 23 April 2025.
Edited by:
Michael Heinrich, University College London, United KingdomReviewed by:
Enzo Spisni, University of Bologna, ItalyHemanga Hazarika, Girijananda Chowdhury University, India
Ming-Jie Li, Shanghai Institute for Biomedical and Pharmaceutical Technologies, China
Copyright © 2025 Bu, Li, Ye, Wang, Song and Chen. This is an open-access article distributed under the terms of the Creative Commons Attribution License (CC BY). The use, distribution or reproduction in other forums is permitted, provided the original author(s) and the copyright owner(s) are credited and that the original publication in this journal is cited, in accordance with accepted academic practice. No use, distribution or reproduction is permitted which does not comply with these terms.
*Correspondence: Jing Chen, Y2hlbmppbmc2Mzg1QDE2My5jb20=