- 1Department of Biology, University at Albany, State University of New York, Albany, NY, United States
- 2Department of Biology, Syracuse University, Syracuse, NY, United States
For more than 10 million years, large, herd forming ruminants have thrived as parts of sustainable grazing ecosystems. Conversely, since their domestication 8,000–11,000 years ago, cattle, sheep, and goats have often exhibited dysfunctional relationships with the ecosystems they inhabit. A considerable literature, developed over decades, documents the negative impacts of animal agriculture and associated activities (e.g., feed production) on grassland ecosystems. Coincident with the accumulating data documenting the impacts of “conventional” animal agriculture, has been a growing interest in restoring functionality to agricultural grazing ecosystems. These “regenerative” protocols often seek to mimic the structure and functions of wild grazing ecosystems. The objectives of this paper were two-fold: First to review the literature describing the structure and some key functional attributes of wild and agricultural grazing ecosystems; and second, to examine these attributes in conventionally and regeneratively managed grazing ecosystems and, assuming the wild condition to be the standard for sustainable grazer-environment relationships, to ascertain whether similar relationships exist in conventionally or regeneratively managed agricultural grazing ecosystems. Not unexpectedly our review revealed the complexity of both wild and agricultural grazing ecosystems and the interconnectedness of biological, chemical, and physical factors and processes within these systems. Grazers may increase or decrease system functionality, depending upon environmental conditions (e.g., moisture levels). Our review revealed that biodiversity, nitrogen cycling, and carbon storage in regenerative grazing systems more closely resemble wild grazing ecosystems than do conventional grazing systems. We also found multiple points of disagreement in the literature, particularly with respect to aboveground primary production (ANPP). Finally, we acknowledge that, while much has been accomplished in understanding grazing ecosystems, much remains to be done. In particular, some of the variability in the results of studies, especially of meta-analyses, might be reduced if datasets included greater detail on grazing protocols, and a common definition of the term, “grazing intensity.”
Introduction
Grasslands encompass some 40% of the earth's rock and snow-free landmass. They have historically supported migratory herds of abundant, large ungulates which, taken together, constitute productive “grazing ecosystems” (McNaughton, 1985; Frank et al., 1998) characterized by substantial energy transfers from primary producers to large mammalian herbivores, omnivores, and carnivores, and exceptional soil-carbon storage capacity (Kristensen et al., 2022). Given their spatiotemporal extent (39 million km2; 10 million years), wild grazing ecosystems can be considered highly sustainable. However, many of the earth's wild grazing ecosystems have been lost, and some of those remaining are threatened.
Grasses first appeared in northern South America, north Africa, and India during the late Cretaceous, ca. 107–129 Mya (Prasad et al., 2011), with a major emergence around 66 Mya (Piperno and Sues, 2005; Prasad et al., 2005). Today, ~12,000 grass species range over more than 40% of the earth's non-frozen surface. The cellulosic and silicious matrices that compose grass stems and leaves made their digestion by early herbivores difficult, facilitating their success and, coincidentally, the co-evolution of ruminant multi-chambered stomachs and abrasion-resistant hypsodont (high-crowned) dentition (McNaughton, 1991; Frank et al., 1998). Additional adaptations, such as keratin-encased digits suited for long distance travel over rough, uneven terrain emerged along a spectrum of climatological and geomorphic gradients at a multiplicity of spatial scales. The co-radiation of grasses, wild ungulates and large Australian macropods during the Miocene (Stebbins, 1981; Gould, 1986; Archibald, 2008), and the suites of reciprocally evolved, often interdependent morphological and physiological traits (Janis, 1993; Jacobs et al., 1999; Zachos et al., 2001; Hackmann and Spain, 2010; Saarinen, 2019) is thoroughly documented in the fossil record (e.g., Love, 1972; Morton, 1972; Stebbins, 1981; McNaughton, 1991; Jernvall and Fortelius, 2002; Bouchenak-Khelladi et al., 2009; Hodkinson, 2018). Though late Miocene declines in ungulate populations are associated with climate-forced transitioning from C3 to C4 grasses, hunting and land use practices of hominid and human populations for thousands of years resulted in body mass reductions and species extinctions among ungulates throughout Eurasia and Africa (Tsahar et al., 2009; Smith et al., 2018).
Domestication of animals and crops reached its zenith between 11,000 BC and 8,000 BC in the Middle East, Central, South and East Asia, and the Americas. While crop agriculture was confined to fertile soils, animal agriculture spread rapidly into the margins, where crop agriculture was impractical or impossible. Today, approximately two-thirds of the earth's landmass can only support animal agriculture (Kohler-Rollefson, 2021; World Bank, 2021). In arid and semi-arid regions, such as the African veld and Mongolian steppe, ruminant livestock for millennia has been moved by herders among circuits of oases and seasonally ephemeral grasslands and ponds (Turner and Hiernaux, 2002) or managed on grazing “circuits” across open plains, forest edges and wetlands (Gantuya et al., 2021). In boreal Europe, the movements of wild reindeer populations have been managed by Saami herders for centuries (Sommerseth, 2011). In cool temperate to subarctic regions, such as Scandinavia, Scotland and the English borderlands, small ruminants (sheep) were frequently released into high pastures in the spring and returned to their farms in the fall (McWhiney and McDonald, 1985). Similarly, rangeland in much of the western United States was freely grazed by cattle and sheep during the growing season, usually without concern for their impact on soil, vegetation, water, or ecosystem services. Throughout much of history, small-holder farmers integrated animal and crop agriculture when possible. Post-harvest crop remnants were grazed by livestock, and livestock dung provided fertilizer to crop fields (Howard, 1947).
With notable exceptions, agricultural ecosystems have been out of sync with wild grazing ecosystems, often disrupting wildlife distributions and changing plant communities and soil properties (Acocks, 1966a; Montgomery, 2017; Gordon, 2018; Kinka et al., 2021). As such, agriculture has contributed significantly to the degradation of native grasslands and the wild grazers they support (Daubenmire, 1940; Acocks, 1966a,b; Turner and Lawrence, 2012). The impacts of animal agriculture on wild ecosystems, particularly grazing ecosystems have been exacerbated by human-induced climate change (Hunninck et al., 2020). Agricultural disruption of native ecosystems increased during the mid-nineteenth century, and even more so since the early-twentieth century as a result of habitat disruption and land-use change (Jackson, 2002; Mligo, 2015; FAO, 2018; Gordon, 2018).
The emergence of industrial agriculture, with its focus on monoculture, the intensive use of inputs (e.g., synthetic fertilizers and pesticides), animal confinement, artificial growth stimulation, and increasing dominance by a few multinational meat production corporations, particularly since the end of World War II, has had significant impacts on farm and ranch profitability (Schlosser, 2001), food quality and safety (Daley et al., 2010), natural resources, ecosystem services and environmental health overall (Teague et al., 2011; Teague and Krueter, 2021). Although consideration of the socioeconomic impacts of industrial animal agriculture is beyond the scope of this paper, it must be stated that livestock management practices have implications not only to the functioning of grazing ecosystems, but to the functionality of human communities, economies, and public health systems. We confine ourselves here to the portion of the production system, and the portions of ruminant livestock (beef cattle, sheep, and goats) life cycles that occur on pasture or rangeland. In this paper we use the term conventional management (CM) to refer to the methods of livestock management often, but not always, associated with the industrial model of agriculture, that involves low density, long duration grazing. A more complete description of CM and its relationship to industrial agriculture is provided in Table 1.
An alternative to conventional management, which emerged from the organic and subsequent regenerative agricultural movements, is a suite of grazing management techniques that we refer to collectively as regenerative livestock management (RM). Because different authors and practitioners refer to RM by a variety of different terms, we have described some of the more commonly used terms in Table 1. Ultimately, RM seeks to mimic natural relationships between grazers, plant, and soil communities to restore and maintain soil health, protect natural resources, and optimize ecosystem services (Teague et al., 2011; Kleppel, 2014, 2021; Brown, 2018). Thus, RM has a dual focus: livestock production and environmental stewardship. As such, RM is necessarily pasture-based, i.e., livestock are on pasture during growing seasons for their entire lives. Ruminant diets consist solely of forages and hay and are not supplemented with high-energy feeds or growth stimulants.
There has been tension in the literature between proponents of RM and those who suggest that CM provides equal or superior results in terms of livestock production and certain ecosystem functions (Briske et al., 2008). We examine some of these issues below. However, as Flack (2016) points out, many farmers and ranchers integrate elements of several grazing strategies into their management plans, and most care little for what the exact strategy is called.
In this paper we ask: How do wild and agricultural grazing ecosystems compare? We describe and compare several key attributes and processes associated with the structure and functioning of wild, conventionally managed (CM) and regeneratively managed (RM) grazing ecosystems. Specifically, we focus on (i) the aggregation behaviors and movements of the ruminant grazers, (ii) the productivity of plants in grazed landscapes, (iii) the biodiversity and composition of plant communities in grazing ecosystems, and (iv) the dynamics of grazing ecosystem soils (nitrogen cycling, carbon storage, and microbial dynamics). Ultimately, we seek to ascertain the extent to which CM and RM agricultural grazing ecosystems are similar to or different from wild grazing ecosystems, which, given their persistence through time and space, could be considered a standard of sustainability.
Methods
We used the recently updated PRISMA guidelines as a framework for this review (Page et al., 2021), which sought to compile and, to the extent possible, integrate and compare data describing how wild, conventional and regenerative grazing ecosystems are structured and how they function. We were particularly interested in ascertaining how the structure and functioning of differently managed agricultural ecosystems are similar to and different from wild grazing ecosystems.
The literature searched consisted predominantly of the peer reviewed articles published in journals focused on wildlife management, ecology, agriculture, animal science, and soil science. We also accessed papers published in edited volumes, as well as books written by researchers, which summarize topical scientific research and theory with intended audiences of either professionals or the public-at-large. In addition, we obtained practical information on livestock management from Extension Service Fact Sheets and International (FAO, UNEP), US federal, and local governmental agency reports. Finally, because the value of practical, experiential knowledge is increasingly recognized within the research community, we have included information obtained from books and other publications prepared by and for ranchers and farmers as well as for popular consumption. When such literature, which was generally not peer reviewed, was included in the review we took care to ensure that the authors were knowledgeable and respected within their disciplines. In total, this review is based on 215 published sources.
The literature review was conducted with the use of conventional search engines, such as Google and Google Scholar. We also used Web of Science to search for publications using the following terms: Ungulates OR grazing OR herbivory AND grassland OR savanna. Finally, we depended heavily upon our personal libraries, which comprise more than a century of technical and popular ecological, evolutionary, biogeochemical, and agricultural literature.
Structure and functioning of wild and agricultural grazing ecosystems
Aggregation and movements
Wild grazers. Large herbivores are capable of migrating long distances to meet dietary requirements in landscapes where the availability of nutritious forage varies spatially across seasons (Fryxell et al., 1988; Fryxell and Sinclair, 1989; Sawyer and Kauffman, 2011; Merkle et al., 2016; Aikens et al., 2017; Kauffman et al., 2021). In tropical savanna habitats, animals migrate between wet and dry season ranges to optimize nutrition. For example, in the roughly 26,000 km2 Serengeti Ecosystem of East Africa, millions of wildebeest (Connechaetes spp.), zebra (Equus quagga), eland (Taurotragus oryx), and other ungulate species migrate from the tall grasslands and woodlands in the northwest, where animals feed on relatively low-quality forage during the dry season, to the fertile southeast plains that support phenologically young and nutritious forage during the wet season (McNaughton, 1983, 1985). Analyzing 16 nutrients required by grazing ungulates in grass samples collected from dry season (northwest corner), wet season (southeast plains) and transitional corridor ranges, McNaughton (1990) found that the mass movement of animals to the southeastern plains was associated with the mineral content of forages. The wet season grasses were enriched in key minerals compared to dry season grasses, with transitional range grasses displaying intermediate levels (McNaughton, 1990).
Although the environmental factors that create spatiotemporal patterns in forage supply and nutrition differ, the dietary drivers of‘ migrations in temperate grazing ecosystems are functionally the same as those in tropical grazing ecosystems. For instance, in temperate mountainous regions like the Greater Yellowstone Ecosystem of Wyoming, Montana, and Idaho, herds of elk (Cervus elaphus), bison (Bison bison), and pronghorn (Antilocarpa americana) feed on young, highly nutritious forage as it sweeps upslope, in a “green wave,” from low elevation winter range to high elevation summer range (Frank and McNaughton, 1992; Frank et al., 1998; Middleton et al., 2018). Animals graze grassland for 1–2 months of the growing season after which they move upslope (Frank and McNaughton, 1992). An analysis of many of the same elements examined in the Serengeti (McNaughton, 1990) revealed greater concentrations of nitrogen, phosphorus, and sodium in forages collected in the first month and potassium in the first two months of the growing season compared to elemental concentrations in forages collected later. Middleton et al. (2018) modeled the effect of the spring—summer migration on the body condition of Yellowstone elk and found that migrating upslope to feed on highly nutritious forage in the early spring and summer resulted in the animals having greater autumn body fat. Together these results indicate that Yellowstone ungulates migrate from low to high elevation range in the spring and early-summer for the same reason that Serengeti herbivores migrate between dry and wet season ranges—to increase the nutritional quality of their diets.
Aggregation is a common feature among free-roaming ungulates in tropical and temperate grassland ecosystems (McNaughton, 1984; Hempson et al., 2015; Ahrestani and Sankaran, 2016). Gregariousness is thought to have evolved as a behavioral solution to predation (Hamilton, 1971; Jarman, 1974; Sinclair, 1974; Fryxell, 1991). Individuals in dense aggregations have a lower probability of being attacked by predators compared to solitary animals (Estes, 1976; Kie, 1999; Fryxell et al., 2007). Members of a dense, aggregated group also benefit from reducing the time budgeted to anti-predator vigilance, which allows more time for feeding (Fryxell, 1991).
The size and trajectories of ungulate feeding groups can be fluid, waxing and waning over time (Owen-Smith et al., 2010). Animals often occur in dense herds that intensively graze vegetation at a single location for days, weeks, or sometimes months creating “grazing lawns” (McNaughton, 1984) characterized by prostrate growth forms, and a short-statured, dense layer of vegetation (McNaughton, 1984; Hempson et al., 2015). Free roaming herds of grazing white rhino (Ceratotherium simum), wildebeest (Connochaetes gnou), springbok (Antidorcas marsupialis), blesbok (Damaliscus pygargus), impala (Aepyceros melampus), Thomson's gazelle (Eudorcas thomsonii) in African tropical savanna; hog deer (Axis porcinus), chital (Axis axis), swamp deer (Rucervus duvaucelii), and sambar (Rusa unicolor) in South Asian savanna; bison (Bison bison) in temperate grassland; and reindeer (Rangifer tarandus) in alpine tundra create grazing lawns (e.g., McNaughton, 1988; Hempson et al., 2015; Geremia et al., 2019; Thapa et al., 2021). The grazing-induced shorter and denser vegetation of grazing lawns compared to nearby less intensively grazed grassland allows herbivores to remove more biomass per bite, thus increasing foraging efficiency (Frank et al., 1998; Hempson et al., 2015; Thapa et al., 2021). Moreover, forage on grazing lawns tends to be enriched in several minerals potentially limiting to large herbivores, including nitrogen, phosphorus, magnesium, and sodium (McNaughton, 1988; Anderson et al., 2010; Hempson et al., 2015; Mayengo et al., 2020). The greater concentration of leaf nitrogen and phosphorus is a function of higher leaf:stem ratios of forage (McNaughton, 1984; Frank et al., 1998; Verweij et al., 2006; Hempson et al., 2015; Thapa et al., 2021) and, often, greater soil N cycling rates (Coetsee et al., 2010; Frank et al., 2018) that occur on grazing lawns. Greater sodium concentrations in the forage of grazing lawns arises when grazers concentrate on sodic sites (McNaughton, 1988; Anderson et al., 2010; Hempson et al., 2015), or when plants adapted to grazing lawns have greater leaf sodium concentrations. The result is that the generation of grazing lawns elevates the grazing efficiency and diet quality of animals feeding on them, which can play an important role in increasing herbivore population size (Hempson et al., 2015).
Ruminant livestock. Unlike wild ungulate populations, the spatial disposition of livestock is largely determined by human managers. With notable exceptions, the stimuli that drive migratory behaviors in wild ungulates are rarely reflected in the movements of livestock at anything other than local spatial scales, i.e., reflective of localized foraging behavior. The amount of time livestock spends in a particular landscape, and the amount of time the landscape is “rested,” i.e., left un-grazed by domesticated ungulates once the stock has been moved, as well as the number of animals per unit space (stock density; stocking rate) and composition of the herd are generally management decisions. Such decisions are based on current or accepted grazing theory, cultural norms, available pasture or rangeland, and available financial and workforce resources (Flack, 2016).
There has been considerable, sometimes spirited, debate during the past half century about what constitutes the most practical approach to livestock management on pasture or rangeland (e.g., Savory, 1983; Briske et al., 2011; Teague et al., 2013; Augustine et al., 2020; Hawkins et al., 2022, and others). Key to much of the debate is whether the focus of the enterprise is on livestock production or on some combination of livestock production and environmental stewardship (Kleppel, 2021). Conventional agriculture tends to focus primarily on optimizing the rate of animal weight-gain (though there is interest in conservation; see, Smart et al., 2010). It has been argued that continuous grazing at low to moderate stocking rates, i.e., low to moderate grazing intensities or other pressure indices, results in the highest rates of weight gain, without damaging forage production (Derner and Hart, 2007; Venter et al., 2019; Augustine et al., 2020). Interestingly, Smart et al. (2010) observed that high grazing pressure increased grazing efficiency. Grazers select the most “desirable” plant species, optimizing nutrition. These behaviors parallel the selective behaviors of wild ungulates that follow the “green wave” of developing forage nutritional content, and the revisitation of preferred grazing sites, creating grazing lawns (McNaughton, 1990; Frank et al., 1998). As the length of the grazing period at a site increases, the impact of grazing may turn negative, sometimes creating bare ground and ultimately soil “capping” or cracking (Milchunas et al., 1988; Savory and Butterfield, 1999).
Furthermore, a substantial literature, dating back nearly a century, documents the damage that low density, continuous grazing may do to a landscape. Daubenmire (1940) described changes in biodiversity and community structure in southeastern Washington bunch grass prairie as a function of grazing intensity. Acocks (1966b) predicted the loss of the native South African veld by 2050, if low-density, continuous grazing of cattle were to continue, suggesting that selective feeding over long periods of time can degrade native vegetation to a point at which it is unlikely to recover. Alternatively, high stock densities, high rotation frequencies, and extended pasture rest have been proposed as being consistent with the way wild ungulates graze, move and aggregate (Goodloe, 1969; Savory, 1978, 1983; Teague et al., 2011, 2016). The question of how grazing either intermittent (i.e., rotationally) or continuously, impacts forage production thus remains unresolved.
As noted above, studies report that continuous grazing at low to moderate stocking rates encourages selective feeding on the most nutritious plants, which results in higher rates of weight gain than does intensive rotation of densely stocked animals, with minimal impact to forage quality (Derner and Hart, 2007; Venter et al., 2019; Augustine et al., 2020; Hawkins et al., 2022). High stock densities are thought to reduce selective feeding which, while obviating patch-scale overgrazing and the creation of bare ground, also provides sub-optimal nutrition, and hence lower rates of weight gain in the livestock, and potentially slower forage recovery (Hawkins et al., 2022). Proponents of RM argue that short bouts of high-density grazing create grazing lawns typical of wild grazing ecosystems, prevent excessive defoliation, and allow time for forage recovery (Gerrish, 2004). We are not aware of studies distinguishing mineral and other nutritional components in the forage as a function of CM vs. RM protocols. Venter et al. (2019) considered the responses of beef cattle in South African veld to three different grazing protocols: continuous, moderate intensity grazing; four-camp grazing, in which cattle stocked at moderate rates were moved periodically through a series of four large paddocks; and holistic planned grazing in which livestock at high densities were moved through a series of paddocks at high frequencies (<1–3 d) and paddocks, once grazed, were rested for extended periods of time. The authors reported no differences in the rate of weight gain as a function of grazing protocol though the financial cost of the holistic grazing was significantly higher than the others, due to higher labor costs. Rapiya et al. (2019) reported that livestock in four-camp grazing systems tended to have lower internal and external parasite burdens than continuously grazed cattle. Increasing the number of paddocks (camps), however, did not reduce parasite loads beyond that achieved with four camps.
Productivity in grazed plant communities
Wild grazers. Measuring production in grazed grasslands is challenging because the addition (i.e., growth) of plant tissue occurs simultaneously with its removal (i.e, grazing) (McNaughton et al., 1996). McNaughton (1985) overcame this problem by using a system of multiple short term exclosures to estimate aboveground net primary production (ANPP) in grazed grassland. Employing this method in the Serengeti, McNaughton (1985) discovered that the activities of ruminants increased plant production, challenging the then widely held view that herbivory reduced plant growth (Belsky, 1987). Over a gradient from dry to wet ranges, migratory herds of ruminants stimulated ANPP by 8–344%, (median, 104%) depending upon grazing intensity and soil moisture content (McNaughton, 1985; Frank et al., 1998).
The facilitating effect of native grazers on shoot production has been confirmed in other ecosystems. In Yellowstone National Park, for example, the amount that herds of bison, elk, and pronghorn increased grassland ANPP was contingent on the topo-edaphic-vegetation conditions of the grassland and, similar to the Serengeti Ecosystem, grazing intensity. Stimulation peaked at 34% at a grazing intensity of 40% at mesic and fertile sites (Frank et al., 2016). In Scandinavian tundra, reindeer-grazing increases ANPP under conditions that the grazing is heavy enough to transform moss-heath dominant tundra to productive graminoid-dominated tundra (Olafsson et al., 2004). Finally, in tall grasslands of the Konza Prairie grazed by bison (Collins and Uno, 1985) and Kruger National Park of South Africa grazed by a numerous species of ruminants (Koerner et al., 2014), herbivory had no effect on ANPP (but see, Buis et al., 2009) In both ecosystems, grazing stimulated the relative growth rates of grazed vegetation, but not enough for grazed vegetation to produce more shoot biomass than un-grazed vegetation (Oesterheld and McNaughton, 1998; Penner and Frank, 2021).
Ruminant livestock. The data on how grazing, and particularly management strategy (e.g., CM vs. RM), affects above ground net primary production in ruminant-grazed pastures and rangeland are mixed. ANPP in continuously grazed, semi-arid South African veld was either higher than, or not different from that in RM-grazed veld (Venter et al., 2019; Hawkins et al., 2022, also see above). Conversely, studies comparing ANPP in CM (continuously grazed) and RM in both semi-arid Texas rangelands and semi-humid New York pastures reported higher ANPP in RM-grazed sites (Teague et al., 2011; Girard Cartier and Kleppel, 2017; Kleppel, 2019).
Zeng et al. (2019) suggested that environmental conditions associated with climate change (e.g., increased aridity and temperature), rather than grazing alone, determine ANPP in high-altitude Tibetan grasslands. Girard Cartier (2017) comparing the environmental effects of CM and RM grazing in a study in upstate New York, USA, supported Zeng et al.'s observations on the importance of environmental conditions (particularly soil moisture) as drivers of ANPP. Furthermore, Derner and Hart (2007), reported on a 16-year study of the effects of pasture-duration and stocking rate on ANPP in northern, mixed grass prairie (Wyoming, USA). They observed that 54% of the variability in ANPP was explained by precipitation, which appeared to affect the outcomes of different grazing protocols differently. Thus, precipitation, and other climatic variables may play a dominant role in determining ANPP in agricultural grazing ecosystems. Unfortunately, comparisons between grazing protocols may be confounded by differences in how terms are defined in the literature (McSherry and Ritchie, 2013).
Subsurface plant (root) production is an often-overlooked component of grazing studies. Much of the carbon in grasslands grazed by large herbivores is stored below ground in root systems and as stable soil organic matter (Bardgett and Wardle, 2003; Sacks et al., 2014; Kristensen et al., 2022). As much as two-thirds of the total biomass of grasses may be below ground (Sacks et al., 2014). López-Marisco et al. (2015) reported that moderate intensity continuous cattle grazing stimulated net below-ground production and increased carbon turnover rates, relative to those in ungrazed, temperate Uruguayan grasslands. However, the influence of grazing management on belowground grassland production across environmental gradients remains poorly understood.
Biodiversity and community composition in grazing ecosystems
Wild grazers. The number of plant species in a grassland community (species richness, S) is the net effect of the rates of species colonization and extinction at the site (MacArthur and Wilson, 1967; Olff and Ritchie, 1998; Iravani et al., 2011). Herbivores can impact those processes by: (i) facilitating propagule dispersion on fur, hooves, and in dung (Collins and Uno, 1985), (ii) creating open sites that can be colonized, and (iii) reducing competitive exclusion by grazing dominant species and releasing sub-dominants. Milchunas et al. (1988) postulated that herbivory should increase both species richness, S, and diversity, Shannon H', in sub-humid, highly productive grasslands, and slightly reduce or have minimal effects on semi-arid, low-productive grasslands. They proposed that those patterns would be generated by grazing releasing species from strongly light-limiting conditions in tall, dense canopies of productive grasslands compared to less light-limiting conditions of more sparsely vegetated low productive grasslands. In addition, plant traits conferring tolerance to water stress in dry, low productive grasslands may also be traits that confer grazing tolerance (Coughenour, 1985; Milchunas et al., 1988).
The notion that wild ungulates promote biodiversity is supported by results from exclosure experiments comparing species diversity in grazed vs. fenced, un-grazed grassland (Table 2). To be included in Table 2, a grassland ecosystem had to support only wild, free roaming ungulates (no livestock). The number of ecosystems that met this criterion was limited (n = 7). In addition, the duration of exclusion, the diversity parameters measured, and the plot size within which species were counted varied across ecosystems. The pattern that emerged is that grazers increased grassland plant biodiversity, with the exception of the mesic savanna of Kruger National Park, South Africa (Koerner et al., 2014) (Table 1). Results of studies examining the effects of grazers on Shannon diversity (H') in two of the ecosystems (Serengeti and Royal Bardia National Parks) corroborated findings for their effect on S—grazing also increased H' (Table 2). In three ecosystems, temperate semi-arid grasslands of Yellowstone National Park, the sub-humid tallgrass prairie of Kansas (Konza Prairie Biological Station), and tropical savanna of Serengeti National Park, grazer-induced increases in S were associated with herbivores increasing the spatial heterogeneity of soil resources or species composition (McNaughton, 1983; Augustine and Frank, 2001; Bakker et al., 2003; Collins and Smith, 2003).
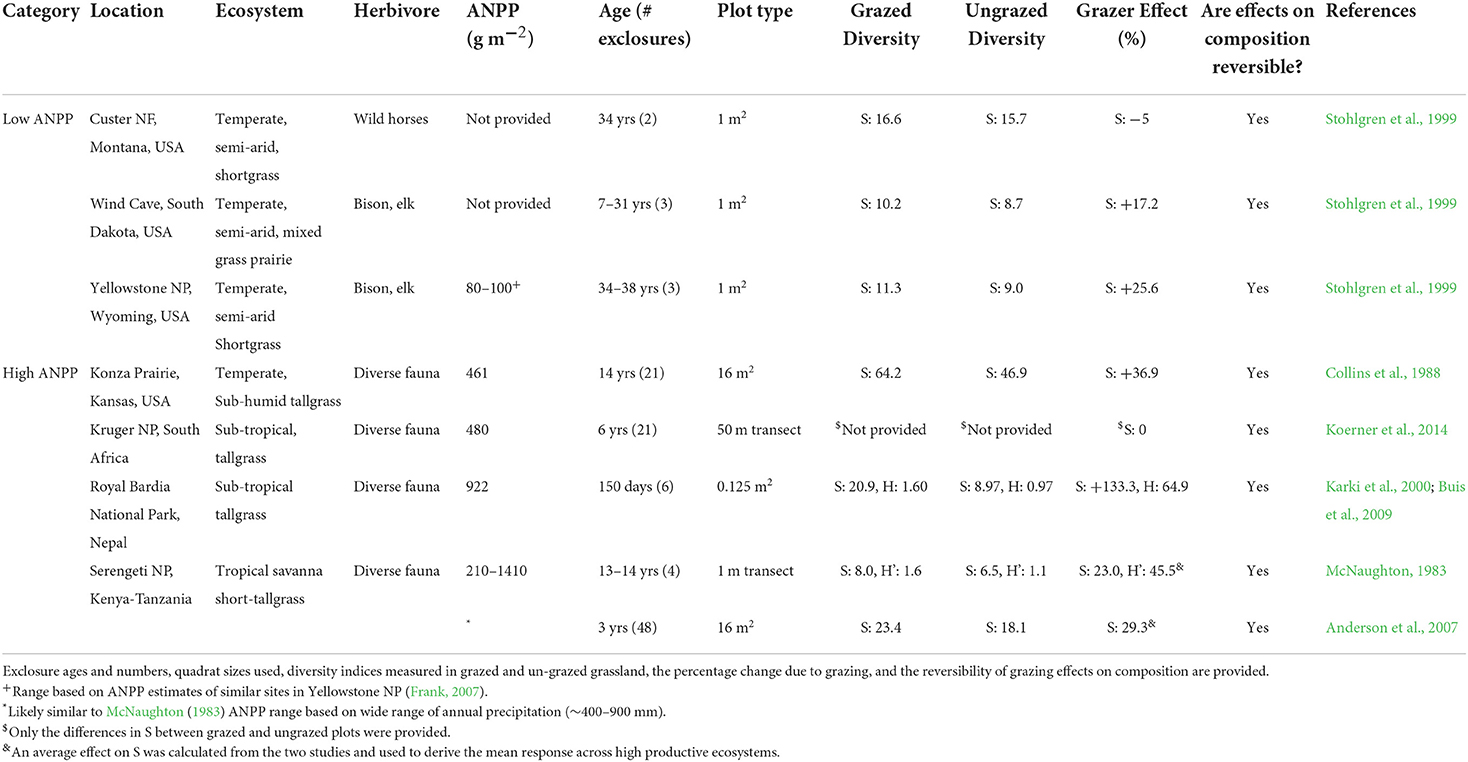
Table 2. The characteristics of grassland ecosystems that support abundant herds of migratory wild ungulates where exclosure studies measured the effects of herbivory on species diversity and the resiliency (i.e., reversibility) of grassland species composition to grazing.
There is also support for the notion that wild ungulates increase species diversity more in highly productive, compared to less productive grasslands (Table 2); although the only low productive grasslands included in Table 2 were geographically constrained to the Rocky Mountains of North America (Stohlgren et al., 1999). Among the three Rocky Mountain grasslands, the mean percentage change in S by different combinations of wild ungulates was +12.6 (range: −5.0–+25.6%). Among relatively productive grasslands of the Serengeti and Kruger National Parks in Africa, the tallgrass of the Royal Bardia National Park in Asia, and the tallgrass prairie of Kansas in North America, the mean percent change in S due to grazing was +40.9% [range: 0 (no effect) to +133%]. Moreover, among 10 grasslands in Yellowstone National Park that ranged widely in aboveground net primary production (15–375 g m−2), the effect of grazing on S was positively associated with production (Frank, 2005).
The effect of herbivory on grassland species composition has been an important topic of grazing research Augustine and McNaughton, 1998; Cingolani et al., 2005; Díaz et al., 2007). Acknowledging the limitations of our small sample size (Table 2), it would appear that short-term grazing by migratory herds of ungulates can drive vegetation to alternative stable states that are reversible when the grazers are removed (Table 2) (Augustine and McNaughton, 1998). Plant species composition is also reversible in grazing lawns where intense herbivory can lead to nearly a complete turnover of species (McNaughton, 1983; Karki et al., 2000; Cingolani et al., 2005).
Ruminant livestock. Grazing by livestock may positively or negatively alter biodiversity in plant communities, and produce both reversible and irreversible state transitions, with compositional shifts as large as 95%, depending upon community successional status (Westoby et al., 1989; Laylock, 1991; Milchunas and Lauenroth, 1993). The relationships between livestock and plants in grazing ecosystems may require prolonged periods of time to develop (Loeser et al., 2001). In California coastal prairies, species richness and cover by native grasses and forbs in areas grazed by cattle over multiple years far exceeded those in un-grazed areas (Hayes and Holl, 2003). The authors observed a reduction in plant stature in grazed portions of the ecosystem with increases in species richness among annual forbs and decreases among perennial forbs. Similarly, Hart (2001) reported greater plant species richness in cattle-grazed Colorado grasslands than in grasslands from which cattle were excluded. Herrero-Jáuregui and Oestwald (2018) reported an average 25% decrease in S at both high and low stocking rates relative to that observed at moderate stocking rates, independent of environmental conditions. Whether the trend in biodiversity is positive or negative appears to depend largely on how the livestock and land are managed and, to a lesser extent, on interannual environmental variability. For instance, Teague et al. (2011) reported greater proportions of high seral grasses in regeneratively managed northeastern Texas pastures than in continuously (both low and high stocking rates) grazed pastures, where low grasses and forbs dominated.
An explanation for these observations may be found in disturbance theory. Grazing is among the predominant disturbances associated with grasslands. Grazing intensity appears to be key to determining whether the effect of grazing on biodiversity will be positive or negative (McNaughton et al., 1989; Milchunas and Lauenroth, 1993). Marty (2005) concluded that “moderate” grazing by livestock was critical to the maintenance of native plant biodiversity of California's vernal pool ecosystems. Similarly, in a global meta-analysis, Wang and Tang (2019) reported that species richness tended to increase under conditions of light-moderate grazing intensity, but to decrease under intense grazing intensity. Similarly, Allison and Bender (2017) reported that both overgrazing and under-grazing can be deleterious to biodiversity in semiarid rangelands. These observations are consistent with the general predictions of the intermediate disturbance hypothesis (Connell, 1978).
In an application of the theory, livestock has been used to manage invasive plants and restore functionality to biologically invaded landscapes. For example, increases in plant species richness of 10–90% have been documented when sheep were used around wetlands to control purple loosestrife (Lithrum salicaria) and Phragmites australis, and in upland ecosystems to control mile-a-minute (Persicaria perfoliata), and multiflora rose (Rosa multiflora) (Kleppel and LaBarge, 2011; Kleppel et al., 2011; Girard Cartier and Kleppel, 2015).
In summary, biodiversity responds in somewhat predictable ways to grazing, increasing or decreasing as a function of grazing intensity. As such, grazing has potential, not only for food production but for vegetation (e.g., invasive plant) management.
Soil dynamics—Nitrogen (N) cycling, carbon (C) storage, and microbial interactions
Photosynthetically acquired C and microbe-mineralized nutrients, including N, are exchanged between grassland plants and their rhizospheric soil microbial communities (Reynolds et al., 2003; van der Heijden et al., 2008). These plant-microbe interactions are key to maintaining energy and nutrient dynamics in grasslands and are significantly impacted by ungulates via grazing and their addition of dung and urine to the soil.
N dynamics in wild grazing ecosystems. The rate at which nitrogen is mineralized and becomes available for plant uptake limits or co-limits, grassland primary production (Fay et al., 2015). Consequently, considerable attention has been paid to the effects of large herbivores on soil N availability, and on ascertaining how grazing connects N-cycling to grassland production. The potential facilitating effects of dung and urine deposition on soil N-cycling has long been recognized (McNaughton et al., 1988). In addition, a prevailing conceptual framework predicts how herbivore regulation of N availability should differ in nutrient-poor vs. nutrient-rich habitats (Ritchie et al., 1998; Wardle et al., 2004; Bardgett and Wardle, 2010). In resource-poor habitat, animals feed selectively on the most nutritious plants encountered, creating a negative feedback loop that leaves N-poor species with high C:N ratios to increase in abundance and generate a low-quality litter pool that reduces decomposition rates and the availability of soil N. In contrast, in resource-rich habitats, grazing promotes the growth of acquisitive species with low C:N ratios that enhances soil N availability. In studies examining the effects of defoliation on rhizospheric processes, grazing has been shown to promote the rate that roots exude labile organic material, which stimulates microbial activity and N mineralization in the rhizosphere of grazed plants (Hamilton and Frank, 2001; Hamilton et al., 2008). Rates of root exudation are greater for acquisitive plants with high C assimilation and growth rates compared to plants with conservative growth strategies (Henneron et al., 2020). Consequently, by increasing the rate of root exudation and presumably soil N mineralization and plant N uptake by fast-growing plants, grazers may facilitate N availability in resource-rich environments by increasing the quality and decomposability of leaf litter.
Some results contradict this hypothesis, however (Stark and Grellmann, 2002; Bakker et al., 2009; Schrama et al., 2013; Millett and Edmondson, 2015), which may be a function of several other pathways that influence how herbivores affect soil N processes (Sitters and Olde Venteerink, 2015). For example, grazing, and the reduction of photosynthetic surface area, may reduce available C flowing to the soil, and thus lower the activity and mineralization rates of soil decomposers (Sankaran and Augustine, 2004). Trampling may increase soil compaction, which may lower soil oxygen at wet sites and soil water content at dry sites, both of which would reduce N mineralization rates (Schrama et al., 2013). Finally, herbivores transport nutrients between areas where they graze and areas where they deposit dung and urine. Thus, seasonal migrations of large herds result in net transport of nutrients from tropical wet season or temperate summer ranges, where animals gain biomass and store nutrients, to tropical dry season or temperate winter range, where herbivores lose biomass and add nutrients in urine and dung to the soil (Bakker et al., 2004; Frank, 2006). The loss and concentration of nutrients in different seasonal ranges can result in spatial variation in herbivore-induced impacts on nutrient cycling across ecosystems (De Mazancourt et al., 1998).
The results of studies of soil N cycling by large, wild herbivores are mixed. Nitrogen availability was increased by diverse herds of ruminants in tropical South African savanna (Coetsee et al., 2010) and the Serengeti Ecosystem (McNaughton et al., 1997), by elk, bison and pronghorn in the temperate grassland and shrub-grassland of Yellowstone National Park (Frank and Groffman, 1998; Frank et al., 2018), by bison in temperate tallgrass of Kansas (Veen et al., 2008), and by reindeer in Scandinavian arctic tundra (Sitters et al., 2017). However, soil N mineralization was unaffected by elk in grass-shrub habitat of Rocky Mountain National Park, Colorado, and inhibited by red deer (Cervus elaphus) and chamois (Rupicarpa rupicarpa) in subalpine meadows of the Swiss National Park (Risch et al., 2015). The mechanisms driving the effects of herbivores in the different ecosystems are unclear, except in the case of the arctic tundra where reindeer stimulated N mineralization by shifting the dominant vegetation from poor quality heath to relatively nutrient-rich graminoids. Such a directional shift toward greater forage quality in the nutrient-poor arctic heath seems contrary to the prediction that herbivores should reduce litter quality and rates of N cycling in nutrient-poor habitat, as observed in temperate systems (Wardle et al., 2004; Bardgett and Wardle, 2010).
N dynamics in agricultural grazing ecosystems. N dynamics in agricultural grazing ecosystems emerge from natural interactions involving plants, grazers, and soil microbes, as wells as the overlay of anthropogenic intervention, such as synthetic fertilizer and pesticide application to pastures, and tillage. Furthermore, the impacts of nutrients associated with animal agriculture may be proximate, i.e., on-farm, or remote, such as through the intensive use of inputs for feed production. While our principal interest here is on the former, the fact that livestock on pasture and rangeland may receive feed supplements and that during confinement stages of the life cycle in industrial systems, livestock diets consist almost completely of high-energy feeds, we must acknowledge the anthropogenic influence on nutrient dynamics in animal agriculture.
Synthetic-N inputs in fertilized pastures may alter plant-grazer-microbe interactions that are critical to the natural cycling of N in grassland ecosystems (Montgomery, 2017). To this point, Wei et al. (2013) suggest that pH changes associated with N addition impair the capacity of microbes to use plant-root organic exudates. Conversely, Bardgett et al. (1999) examined the response of four endemic plant species along a gradient in N amendments to soils from sheep-grazed grasslands in the hilly landscapes of the western United Kingdom. They observed that microbial biomass was correlated with plant growth and not with soil N amendment. However, N additions did result in an increase in fungal: bacterial biomass ratios. The amount of N added, was associated with different plant growth rates and community compositions, which appeared to determine whether plant-microbial interactions were positive, i.e., associated with higher plant growth and, hence, microbial biomass (intermediate N addition), had no effect, or were negative, i.e., resulted in lower plant growth, hence, microbial biomass (high N addition).
Defoliation by grazing influences C-allocation and root exudation which may enhance soil microbial biomass and activity (Bardgett et al., 1998), potentially leading to enhanced N mineralization and plant recovery rates. The capacity of pasture and rangeland soils to retain nutrients may be determined in part by the biomass and composition of the microbial community. This in turn, appears to be influenced by the grazing protocol applied to the landscape. For example, Park et al. (2017) reported a reduction in nutrient leaching and runoff on Texas ranches that employed adaptive multi-paddock (AMP, an RM protocol) grazing practices relative to ranches that employed continuous grazing practices.
The indirect effects of animal agriculture should not be ignored, and we consider some of these here. Critical excesses of N exist in most terrestrial and aquatic ecosystems in the developed world (Howarth and Marino, 2006; Groffman et al., 2009; Davidson et al., 2013), and agriculture contributes significantly to these imbalances (Bardgett and Leemans, 1995; US EPA, 2019). Anderson et al. (2014) performed an N mass balance at the Cornell University Animal Science Teaching and Research Center in central New York State. They observed that N inputs greatly exceeded outputs at the study site and were dominated by N used in feed production (63%). Turner et al. (2008) point out that runoff from the application of N fertilizer in the Mississippi River watershed, principally for grade 2 corn (for animal feed) production, has created an 18,000 km2 hypoxic zone (dissolved oxygen levels <2 ppm), in the Gulf of Mexico. Substantial atmospheric and aquatic ammonium and nitrous oxide loading result from manure management practices associated with livestock confinement (Webb et al., 2005; Parker et al., 2018). Failed manure management in confined swine operations has caused of algal blooms, riverine hypoxia, and massive fish kills in North Carolina estuaries (USA) (Paerl et al., 1998; Hounshell et al., 2022).
While animal agriculture bears considerable responsibility for the damaging effects of N and other nutrients in the biosphere, it also offers solutions to these impacts. Ultimately, an impact is a function of a management practice, and practices can be modified to mitigate impacts. For instance, so called “grass-fed” ruminant production obviates the use of feed grains. Continuous grazing in a single pasture can lead to concentration of dung around water sources and shade, and in locations where preferred forages occur. While the nutrients in dung on pasture appear to be re-cycled faster than when plants simply decay in the field, nutrient distributions on the pasture will be patchy (Liu et al., 2015). Short duration, RM grazing, however, results in more even dung distributions (Gerrish, 2004; Kleppel, 2014). Transition to RM reduces eutrophication risks (Park et al., 2017). Management practices that focus on strengthening the connections between plants, microbes and grazers are likely to support nutrient dynamics that sustain functionality in agricultural ecosystems, rather than stress them.
C dynamics in wild grazing ecosystems. The soil stores more than 200 trillion tons of C, making it one of the largest C-reservoirs in the biosphere (Kristensen et al., 2022). More than half of that C is stored in the vegetation and soils of grasslands and croplands, with some 79% of the organic carbon in grassland ecosystems being stored in the soil (Sacks et al., 2014). Given the dangers posed by climate change and the importance in limiting the amount of the global soil C pool transferred to the atmosphere, the role of grazing ecosystems in C cycling and storage are of great interest. Wild and agricultural grazing ecosystems have the potential to contribute to GHG draw-down via photosynthetic C-sequestration and microbially mediated C-stabilization in the soil (Derner and Schuman, 2007; Teague et al., 2016). The dominant, decomposition-resistant form of organic matter in sub-soils deeper than 30 cm occurs as stable micro-aggregates, i.e., mineral-adsorbed organic matter (MAOM)(Kristensen et al., 2022), as much as half of which is produced from microbial necromass (Liang et al., 2019; Wang et al., 2021). Soil organic carbon (SOC) storage results from the long-term difference between C inputs, via above- and belowground (i.e., root turnover) litter production, and soil C losses, primarily via soil decomposer respiration. With nearly all the earth's uncultivated grassland and savanna grazed by ruminants, herbivores alter plant aboveground and belowground litter production and directly contribute dung and urine inputs to the soil and therefore can have important effects on grassland source and sink dynamics (McSherry and Ritchie, 2013; Zhou et al., 2018; Kristensen et al., 2022).
Grazers impact decomposition rates by changing soil temperature and moisture conditions, decomposability of inputs and converted products, and, potentially, the rate at which organic material adsorbs to mineral surfaces as MAOM and as such, is protected from further decomposition (Kristensen et al., 2022). Grazing by large herbivores stimulates root exudation, increasing microbial biomass, which in turn may stimulate aboveground N-pools (Hamilton et al., 2008). The effects of herbivores on plant and soil processes may depend on grazing intensity, herbivore influence on fire intensity and frequency, and on such conditions as soil texture, temperature, and precipitation (Ritchie, 2014; Schmitz et al., 2014; Kristensen et al., 2022).
Empirical studies determining how wild herbivores influence SOC storage require comparison of soil C concentrations in paired grazed and fenced grassland that have remained un-grazed sufficiently long after fencing to avoid transient soil C dynamics. Very few studies of ecosystems grazed by wild ungulates meet these requirements (McSherry and Ritchie, 2013). The only data set we are aware of is from Yellowstone National Park where soil and plant properties were measured inside and outside 33- and 37-year-old exclosures established on grasslands ranging widely in topographic position, plant biomass, and soil C and moisture (Frank and Groffman, 1998). Deriving soil C concentration from soil percent C and bulk density measurements of Frank and Groffman (1998) reveals that YNP ungulates have no effect on soil C concentration across sites (un-grazed: 52.8 mg C/cm3; grazed: 51.1 mg C/cm3; P = 0.459), and their influence on soil C was unrelated to soil texture (percent clay, P = 0.61) or plant biomass (P = 0.20).
C dynamics in agricultural grazing ecosystems. Sun et al. (2017) suggested that root exudation, and soil C and N dynamics are affected by livestock grazing intensity. They reported that exudation, foliar C, and N-mineralization decreased under heavy grazing, while moderate grazing promoted root exudation, and increased foliar C levels, N-mineralization rates, and soil N concentrations. During a 21-day period of recovery from grazing, root exudation increased in heavily grazed forage, while foliar C and N-cycling returned to pre-grazing levels.
Brown (2018) described his personal experiences in regenerative ranching, coupling conservation crop production [zero-tillage, cover cropping and crop-animal rotation per Lal (2015; 2021)] with RM protocols for producing beef cattle and sheep. Soil organic matter analyses on the Brown Ranch documented an increase from 1.7 to 11.1% over 20 years.
Several meta-analyses have explored the effects of grazing on SOC. McSherry and Ritchie (2013) used a multi-factorial approach on data from 17 studies of agricultural grazing to evaluate the relationship between grazing and carbon sequestration as a function of environmental variability. Six variables—soil texture, precipitation, grass type, grazing intensity, study duration, and sampling depth—explained 85% of the variability in the effect that grazing had on soil C. Interactions among variables were crucial to understanding the effect of grazing on SOC. For instance, increasing precipitation decreased C in fine soils, but increased C in sandy soils. Increasing grazing intensity resulted in increased SOC in C4-dominated grasslands and mixed C3-C4 grasslands, but decreased SOC in C3-dominated grasslands. Phukubye et al. (2022), analyzed 235 grazing systems in 18 countries worldwide. They reported a 21% increase in C storage in soils with the implementation of RM protocols (all soils responded favorably), an average 15% increase in 60% of the soils from which grazers were excluded, and a 14% increase in C storage in soils of grasslands grazed continuously at low stock densities. It would appear that RM is a potent tool for C sequestration, even more so than continuous grazing or complete removal of grazers from the landscape.
To investigate the connections between grazing, microbial biomass (MB), and C storage (SOM), we used data from studies of CM (light and heavy continuous grazing) and RM (adaptive multi-paddock grazing) pastures on three ranches, and a grassland in a state park where livestock were excluded in north-central Texas (Teague et al., 2011) and from two CM (light continuous grazing) and two RM pastures (adaptive multi-paddock grazing) in New York's Helderberg Mountain region (Kleppel, 2019). Because SOM levels from New York were not published, SOM was estimated from the mean MB: SOM ratio for the Texas data, assuming a linear relationship between MB and SOM as had been demonstrated empirically by Batisda et al. (2021; their Figure 4; p.2087). Given the differences in soil types and precipitation, it is not surprising that the moist clayey New York soils were higher in organic matter than the dryer, sandy loams of north-central Texas (Magdoff and Van Es, 2021). Within regions (Texas, New York), microbial biomass and SOM were higher in grasslands grazed by RM compared to continuous grazing protocols. In Texas, the RM ranch and un-grazed parkland exhibited similar percentages of soil organic matter, but in the parkland, the MB: SOM ratio was higher than in the RM site. While it is difficult to extrapolate these observations to other managed grazing systems, they provide a rationale for further, more extensive investigation.
Several studies suggest that animal agriculture can play a significant role in the mitigation and sequestration of greenhouse gases. The modeling study of White and Hall (2017) predicts that termination of livestock agriculture and conversion to a completely plant-based food system would result in increased GHG loading to the atmosphere. Clark et al. (2020) and others point out that the current food system makes it nearly impossible constrain global temperature rise below target thresholds (1.5–2.0°C). Herrero et al. (2013) used a globally extensive data base on animal agricultural systems to identify feed (grain) production as a leading source of GHG emissions, the importance of grasslands for C-sequestration, and mixed crop-livestock agriculture (also see, Lal, 2021) as critical to the sustainable production of food in developed and developing countries. Kleppel (2021) suggested that the abandonment of energy intensive feed-grain production, along with implementation of improved manure management practices could reduce GHG emissions associated with beef production in the US by about 35%.
The results of an investigation of conventional and regenerative crop and animal agriculture led Teague et al. (2016) to conclude that the combination of conservation cropping (low till, high ground cover, extensive crop rotation; Lal, 2015) and adaptive multi-paddock livestock management could turn agriculture in North America C negative, removing 1.2 Gt y−1 more carbon than all agricultural activities on the continent produce. Life cycle assessments by Stanley et al. (2018), Rowntree et al. (2019), and others have supported these observations for the beef and dairy sectors, the principal methane sources in mammalian agriculture (US EPA (US Environmental Protection Agency), 2021).
In summary grazing by large herbivores contributes to the stabilization and storage of C in agricultural ecosystems. Certain management protocols appear to facilitate desirable outcomes with respect to C storage, but much remains to be learned in this area. What seems clear, however, is that livestock, when managed with an eye toward environmental stewardship, can become a vehicle for sustainable food production.
Ruminant-plant-soil microbial interactions. Soil microbes are the bridge between the plant community and grazers and, as such, are critical to the functioning of grazing ecosystems. The plant-grazer-microbe system involves multiple and multi-directional feedbacks, with each component affecting the others. Microbes are critical to C storage, nutrient cycling, soil structural stabilization and, indirectly, water storage in soil interstices (Bardgett et al., 1996; Bardgett and and McAllister, 1999; van der Heijden et al., 2008; Malik et al., 2016). Chemical communication (i.e., signaling) between microbes and plants, affects plant health and community diversity (Baker et al., 1997; Seneviratne et al., 2017). Bacterial and fungal processing of nitrogenous organic matter and phosphorus species forms the basis of plant nutrition in grassland and savannah ecosystems (van der Heidjen et al., 1998; van der Heijden et al., 2008). Organic exudates produced by plants and released at the root surface, provide nutritional resources that increase the capacity of the rhizosphere to support diversity within the microbial community (Wright and Upadhyaya, 1996). Bacteria and arbuscular mycorrhizae foster health within the vascular plant community by discouraging the growth of plant-pathogens and herbivory by insects, while making enzymes available that improve nutrient resource partitioning (Reynolds et al., 2003; van der Heijden et al., 2008; Harun-Or-Rashid and Chung, 2017; Wang et al., 2020).
Ruminants contribute to this feedback system in several ways. Processing and fermentation in the rumen and digestive tract decomposes plant material, resulting in dung mineralization rates ca. 20% greater than that of decomposing senescent plant material (Liu et al., 2015). Both soil characteristics and plant community composition may affect microbial biomass in grazed landscapes (Bardgett et al., 1997), and soil microbial community structure appears to vary in response to differences in land use and management (van der Heijden and Wagg, 2013). Kleppel (2019) described the soil microbial communities of 21 pastures (grazed by various ruminant species and breeds) and hayfields in upstate New York. The pastures were managed either by RM or CM protocols. Hayfields were cut once per season. Soil microbial biomass, Shannon H', and grazer impact on soil structural quality, as indicated by the fungal: bacterial ratio (Bailey et al., 2002; Malik et al., 2016) were higher in soils managed by RM than CM protocols. Microbial diversity in hayfields was highly variable and could not be distinguished from either grazing protocol. Soil microbial biomass and H' of CM pastures and hayfields were not different from one another, and always lower than the same attributes for the microbial communities of RM soils.
Bardgett et al. (2001) measured microbial community structure in soils along historical and grazing-intensity (by sheep) gradients in England. They reported that bacteria (B) dominated the microbial community in soils of intensively grazed landscapes, while fungi (F) were dominant in soil samples from low to moderately grazed landscapes and wild, un-grazed landscapes. Because higher F:B ratios are thought to be indicative of greater C storage in the soil (Bailey et al., 2002; Malik et al., 2016), Bardgett et al.'s results suggest that moderate grazing intensity is associated with greater C storage compared to intensive grazing.
Teague et al. (2011), working in tallgrass prairie ecosystems in north-central Texas, compared soil microbial communities from ranches managed by multi-paddock, light continuous with heavy continuous grazing. Also included in the study were soils from sites un-grazed by livestock. Microbial biomass and fungal:bacterial ratios were higher in soils from multi-paddock, and un-grazed sites, than from and soils where grazing was continuous. Girard Cartier and Kleppel (2017) compared the rates of development of soil microbial communities in experimental CM and RM enclosures on landscapes that were previously un-grazed, in moist bottomlands, and drier uplands in east-central New York State. Microbial biomass was higher in upland soils from RM sites compared to CM sites. In moist bottomlands, however, differences in microbial biomass were not significant among grazing treatments. These studies provide support for the idea that microbial community structure and function are driven by both environmental factors (e.g., soil moisture) and land management (grazing protocol) practices. While further study is clearly warranted, the preponderance of evidence supports the hypothesis that RM protocols, which seek to mimic the aggregation and movement patterns of wild ungulates, are associated with more robust soil microbial communities compared to CM protocols.
Discussion and conclusions
What emerges from this review is, first, an appreciation for the complexity of both wild and agricultural grazing ecosystems. Clearly, large herbivores are significant both as positive and negative engineers in grassland ecosystems. It is also apparent that the long co-evolution of ungulates and grasslands, has led to a suite of sustainable relationships that fosters fertility in the soil, productivity, and diversity in associated plant communities, and efficiency in the cycling of nutrients through soil, plants, and animals.
In many cases, animal agriculture evolved ignorant of and disinterested in the lessons provided by wild grazing ecosystems. Domestication and animal breeding concentrated on production of food and fiber, not environmental stewardship. Millennia passed before ecologists began thinking about farms and ranches as ecosystems. As such, agricultural research focused more on optimizing short term food and fiber production than on long term environmental stewardship. As the human population continues to increase, so will the demand for meat (Lal, 2021). Animal agriculture and associated agri-businesses have become significant contributors to environmental degradation (UNEP, 2009; Kleppel, 2021). For example, by 2005, nearly 276,000 km2 (17%) of the Amazon rainforest had been converted to agricultural use for cattle grazing and soya (for animal feed) production (UNEP, 2009). The demand for meat and the will to reduce the impacts of its production seem at odds.
Based on this review, however, we suggest that agricultural grazing ecosystems have the potential to both provide abundant food and sustain, and even improve upon natural ecosystem functions. For instance, while Frank and Groffman (1998) observed that wild ungulates in the Yellowstone Ecosystem had no discernible impact on carbon storage in the soil, studies of agricultural grazing ecosystems suggest that RM protocols may be capable of better than natural rates of C sequestration, even helping to make agriculture carbon negative (Teague et al., 2016; Kristensen et al., 2022; Phukubye et al., 2022; Figure 1).
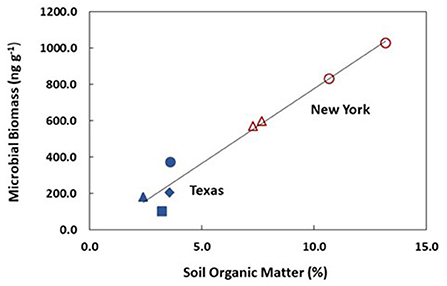
Figure 1. Least squares regression model of microbial biomass (MB) on soil organic matter (SOM, %) in soils from pastures in upstate New York (red; Kleppel, 2019) and ranches or un-grazed grassland in Texas (blue; Teague et al., 2011). Open symbols indicate that SOM was estimated from the assumed linear relationship between MB and SOM (Batisda et al., 2021). Circles indicate that an RM grazing protocol was used. Triangles indicate continuous grazing, and a square indicates heavy continuous grazing (both are CM protocols). The diamond represents un-grazed parkland.
Among the opportunities in agricultural grazing ecology that has much to offer but is underutilized, is to include livestock managers—farmers and ranchers—in the research environment (as have Augustine et al., 2020). Too few studies, in our opinion, take advantage of the experience and insights of farmers and ranchers, some of whom have achieved extraordinary results in C storage, biodiversity restoration, and production (Brown, 2018). Individual researchers, professional societies (e.g., Society for Conservation Biology) and government agencies (e.g., USDA) (Msoffe, 2013; Augustine et al., 2020; Bishopp, 2020; Skroblin et al., 2020) have called for greater inclusion of local and indigenous knowledge in grazing and agro-ecological research.
All components (e.g., production, nutrient cycling, carbon storage; precipitation; soil structure) of wild and agricultural grazing ecosystems are interdependent and much of the complexity in grazing ecosystems arises from the feedback interactions between grazers and the variable environments they inhabit. Many studies have recognized the multi-dimensionality of grazing ecosystems, and some have sought to identify the major forcing factors to which these systems respond (Milchunas et al., 1988; Milchunas and Lauenroth, 1993; McSherry and Ritchie, 2013; Conant et al., 2017; Kleppel, 2019 and others). While moisture (arid-to-humid; precipitation) and grazing intensity stand out as among the most commonly recognized forcing factors, many others have been identified, and multivariate tools are becoming key to progress toward understanding the structure and functioning of grazing ecosystems (McSherry and Ritchie, 2013; Conant et al., 2017). Similarly, key interactions among primary production, C storage, N cycling and microbial dynamics are not well-studied, and the roles of grazing in wild systems and different grazing protocols in agricultural systems, as well as a myriad of environmental factors that structure these dynamic interactions remains a fertile area for further investigation.
One of the most widely used measure of grazer activity and impact identified in this review, is grazing intensity. However, the definition of grazing intensity, in both wild and agricultural grazing ecosystems is elusive, leading McSherry and Ritchie (2013) to state:
“Due to a large discrepancy in both the grazing animals used (i.e., cattle, goats, sheep) and the units measured (dry sheep equivalent ha−1, ha steer−1, AUM ha−1, etc.) grazing intensity was based on the authors' qualitative classification as light, moderate or heavy.”
The authors speak to a problem that is not a unique to their work. Given its influence on numerous ecosystem functions, we suggest that a new method for describing grazing intensity should be standardized. There have been efforts to accomplish this, especially by the Society of Rangeland Scientists, and we do not undervalue that work (Scarnecchia, 1985). But the use of animal unit months per hectare, a standard originally developed to easily assess stocking parameters on US federal grazing lands (Jardine and Anderson, 1919), may not fully capture the seasonally variable interactions between grazer nutritional demand and forage production.
An objective of this review was to compare differently managed agricultural grazing ecosystems (i.e., CM and RM) with wild grazing ecosystems, to provide insights into how to manage livestock in ways that reflect the functioning of wild ecosystems, the assumed sustainability standard. Although we reviewed more than 200 studies, many being meta-analyses based on dozens to hundreds of studies, less than a third provided the management details required to address the objective. In several cases, the details of management were known to us, even though they were not specified in the paper. In our comparison of the structure and functioning of wild, CM and RM grazing ecosystems (Table 3) it became clear that significant knowledge gaps exist in several areas, limiting the ability to clearly distinguish the outcomes of different management approaches. In part, this seems due to ecosystem-level sensitivity to simultaneous variability in multiple interacting factors (McSherry and Ritchie, 2013; Conant et al., 2017; Kleppel, 2019).
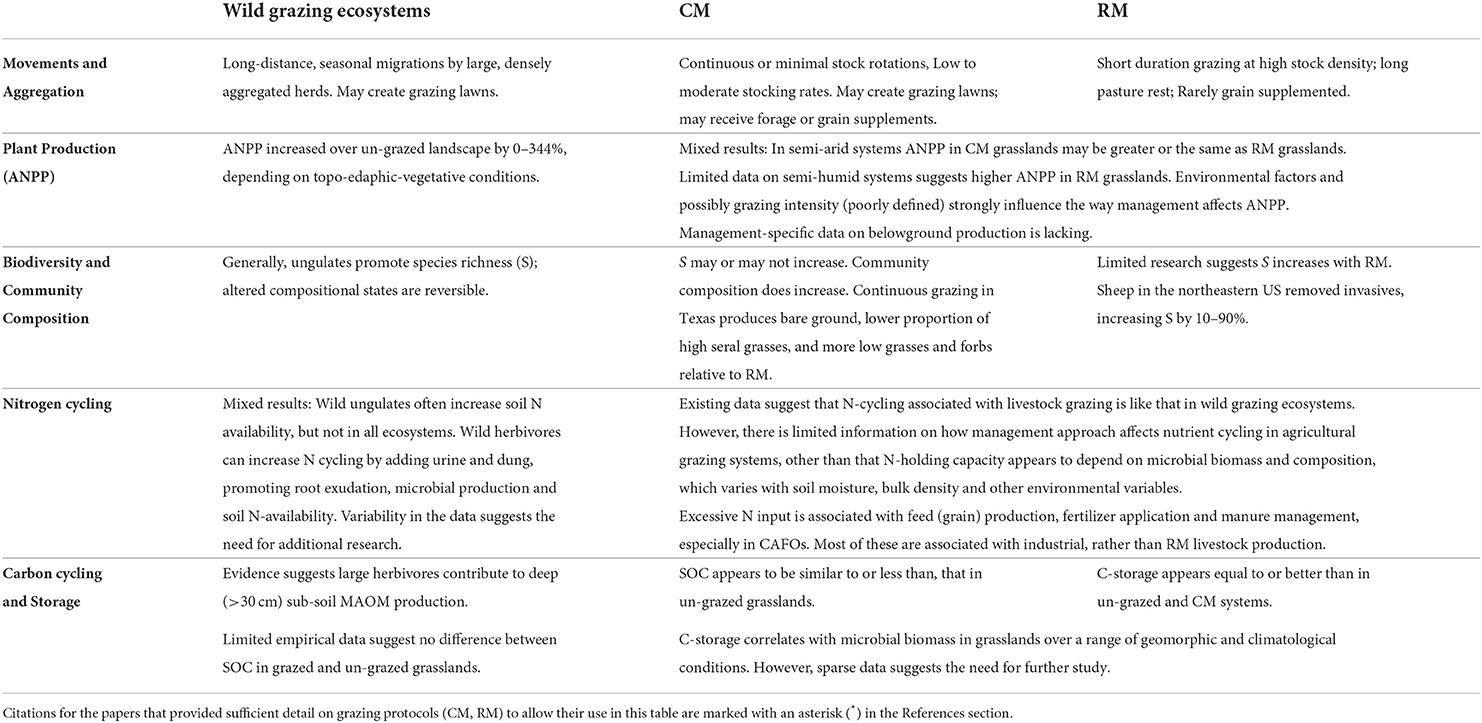
Table 3. Summary of key findings based on studies for which data allowed comparison of wild, conventionally managed (CM) and regeneratively managed (RM) grazing ecosystems.
Where comparisons are possible, RM seems to provide greater environmental stewardship values compared to CM. For instance, we would expect on- and off-farm N inputs (e.g., for grain production) to be generally lower in RM operations (where animals consume only forages) than in conventional livestock operations where animals may be grain-supplemented. Our review also leads us to suggest (with admittedly limited data) that microbial biomass and, hence C storage, will be higher in RM than CM operations under similar environmental conditions (Figure 1; Phukubye et al., 2022). Although we assumed that wild grazing ecosystems would be the standard against which livestock management is judged, at times, it appears that certain livestock management practices may be more environmentally benign than the wild condition. For instance, at times, wild ungulates will intensely graze a portion of a grassland, creating bare spots. If plants do not recruit rapidly into those areas, losses of CO2 and water may result (Savory, 2013; Lal, 2015). RM seeks to minimize bare ground on pasture specifically to reduce these losses. While the limited data available suggest no difference between grazed and un-grazed wild and agricultural grasslands in the semi-arid American west with respect to C storage, studies of RM ranch lands and pastures in semi-arid and semi-humid regions suggest that they store as much or more C than un-grazed grasslands. It was unclear from our analysis whether CM or RM ecosystems support greater ANPP. Observations from wild and agricultural grazing ecosystems suggest considerable variability in ANPP in response to grazing. Ultimately, environmental forcing may be as, or more, important than grazing in determining ANPP in grasslands (Zeng et al., 2019).
It is apparent from the breadth of research cited here that substantial progress has been made toward understanding how grazing ecosystems function at a time when that understanding is critical to our species and to many ecosystems on the planet. We have also highlighted some challenges in grazing ecology, which, if met, could lead to a more sustainable relationship between ecosystem functionality and the practicalities of providing food to a human population approaching the limits of its growth.
Author contributions
DF focused on reviewing the literature on wild grazing ecosystems. GK focused on reviewing the literature on agricultural grazing ecosystems. Both authors contributed substantively to the manuscript. Both authors contributed to the integration of the components of their individual reviews into the final manuscript.
Acknowledgments
The authors are grateful to the reviewers of the manuscript, whose comments and suggestions substantially improved the work.
Conflict of interest
The authors declare that the research was conducted in the absence of any commercial or financial relationships that could be construed as a potential conflict of interest.
Publisher's note
All claims expressed in this article are solely those of the authors and do not necessarily represent those of their affiliated organizations, or those of the publisher, the editors and the reviewers. Any product that may be evaluated in this article, or claim that may be made by its manufacturer, is not guaranteed or endorsed by the publisher.
References
Acocks, J. P. H. (1966a). Agriculture in relation to a changing vegetation. S. Afr. J. Sci. 52, 101–108.
Acocks, J. P. H. (1966b). Non-selective grazing as a means of veld reclamation. Proc. Grassld. Soc. South. Afr. 1, 33–39. doi: 10.1080/00725560.1966.9648517
Ahrestani, F. S., and Sankaran, M. (2016). The Ecology of Large Herbivores in South and Southeast Asia. Bade-Wuertemberg: Springer. doi: 10.1007/978-94-017-7570-0
Aikens, E. O., Kauffman, M. J., Merkle, J. A., Dwinnell, S. P. H., Fralick, G. L., and Monteith, K. L. (2017). The greenscape shapes surfing of resource waves in a large migratory herbivore. Ecol. Lett. 20, 741–750. doi: 10.1111/ele.12772
Allison C. D. and Bender L. C. (2017). Grazing and Biodiversity. CR-686. Cooperative Extension Service, New Mexico State University, Las Cruces.
Anderson, T. M., Hopcraft, J. G. C., Eby, S., Ritchie, M., Grace, J. B., and Olff, H. (2010). Landscape-scale analyses suggest both nutrient and antipredator advantages to Serengeti herbivore hotspots. Ecology 91, 1519–1529. doi: 10.1890/09-0739.1
Anderson, T. M., Ritchie, M. E., and McNaughton, S. J. (2007). Rainfall and soils modify plant community response to grazing in Serengeti National Park. Ecology 88, 1191–1201. doi: 10.1890/06-0399
Anderson, T. R., Goodale, C. L., Groffman, P. M., and Walter, M. T. (2014). Assessing denitrification from seasonally saturated soils in an agricultural landscape: a farm-scale mass balance approach. Agric. Ecosyst. Environ. 189, 60–69. doi: 10.1016/j.agee.2014.03.026
Archibald, S. (2008). African grazing lawns: how fire, rainfall, and grazer numbers interact to affect grass and community states. J. Wildl. Manage. 72, 492–501. doi: 10.2193/2007-045
*Augustine, D. J., Derner, J. D., Fernández-Giménez, M. E., Porensky, L. M., Wilmer, H., Briske, D. D., et al. (2020). Adaptive, multi-paddock rotational grazing management: a ranch-scale assessment of effects on vegetation and livestock performance in semi-arid rangeland. Rangeld. Ecol. Manage. 73, 796–810. doi: 10.1016/j.rama.2020.07.005
Augustine, D. J., and Frank, D. A. (2001). Effects of migratory grazers on spatial heterogeneity of soil nitrogen properties in a grassland ecosystem. Ecology 82, 3149–3162. doi: 10.1890/0012-9658(2001)082[3149:EOMGOS]2.0.CO;2
Augustine, D. J., and McNaughton, S. J. (1998). Ungulate effects on the functional species composition of plant communities: herbivore selectivity and plant tolerance. J. Wildl. Manage. 62, 1165–1183. doi: 10.2307/3801981
Bailey, V. L., Smith, J. L., and Bolton, H. Jr. (2002). Fungal-to-bacterial ratios in soils investigated for enhanced C sequestration. Soil Biol. Biochem. 34, 997–1007. doi: 10.1016/S0038-0717(02)00033-0
Baker, B., Zambryski, P., Staskawicz, B., and Dinesh-Kumar, S. P. (1997). Signaling in plant-microbe interactions. Science 276, 726–733. doi: 10.1126/science.276.5313.726
Bakker, C., Blair, J. M., and Knapp, A. K. (2003). Does resource availability, resource heterogeneity or species turnover mediate changes in plant species richness in grazed grasslands? Oecologia 137, 385–391. doi: 10.1007/s00442-003-1360-y
Bakker, E. S., Knops, J. M. H., Milchunas, D. G., Ritchie, M. E., and Olff, H. (2009). Cross-site comparison of herbivore impact on nitrogen availability in grasslands: the role of plant nitrogen concentration. Oikos 118, 1613–1622. doi: 10.1111/j.1600-0706.2009.17199.x
Bakker, E. S., Olff, H., Boekhoff, M., Gleichman, J. M., and Berendse, F. (2004). Impact of herbivores on nitrogen cycling: contrasting effects of small and large species. Oecologia 138, 91–101. doi: 10.1007/s00442-003-1402-5
Bardgett, R. D., Hobbs, P. J., and Frostegård, Å. (1996). Changes in soil fungal:bacterial biomass ratios following reductions in the intensity of management of an upland grassland. Biol. Fertil. Soils 22, 261–264. doi: 10.1007/BF00382522
Bardgett, R. D., Jones, A. C., Jones, D. L., Kemmitt, S. J., Cook, R., and Hobbs, P. J. (2001). Soil microbial community patterns related to the history and intensity of grazing in sub-montane ecosystems. Soil Biol. Biochem. 33, 1653–1664. doi: 10.1016/S0038-0717(01)00086-4
Bardgett, R. D., and Leemans, D. K. (1995). The short-term effects of cessation of fertilizer applications, liming, and grazing on microbial biomass and activity in a reseeded upland grassland soil. Biol. Fertil. Soils 19, 148–154. doi: 10.1007/BF00336151
Bardgett, R. D., Leemans, D. K., Cook, R., and Hobbs, P. J. (1997). Seasonality of soil biota of grazed and ungrazed hill grasslands. Soil Biol. Biochem. 29, 1285–1294. doi: 10.1016/S0038-0717(97)00019-9
Bardgett, R. D., Mawdsley, J. L., Edwards, S., Hobbs, P. J., Rodwell, J. S., and Davies, W. J. (1999). Plant species and nitrogen effects on soil biological properties of temperate upland grasslands. Funct. Ecol. 13, 650–660. doi: 10.1046/j.1365-2435.1999.00362.x
Bardgett, R. D., and Wardle, D. A. (2003). Herbivore-mediated linkages between aboveground and belowground communities. Ecology 84, 2258–2268. doi: 10.1890/02-0274
Bardgett, R. D., and Wardle, D. A. (2010). Aboveground–Belowground Linkages: Biotic Interactions, Ecosystem Processes, and Global Change. Oxford: Oxford University Press.
Bardgett, R. D., Wardle, D. A., and Yeates, G. W. (1998). Linking above-ground and below-ground interactions: how plant responses to foliar herbivory influence soil organisms. Soil Biol. Biochem. 30, 1867–1878. doi: 10.1016/S0038-0717(98)00069-8
Bardgett R. D. and McAllister E. (1999). The measurement of soil fungal:bacterial biomass ratios as an indicator of ecosystem self-regulation in temperate meadow grasslands. Biol. Fertil. Soils 29, 282–290. doi: 10.1007/s003740050554
Batisda, F., Eldridge, D. J., Garcia, C., Png, G. K., Bardgett, R. D., and Delgado-Baquerizo, M. (2021). Soil microbial diversity-biomass relationships are driven by soil carbon content across global biomes. ISME J. 15, 2081–2091. doi: 10.1038/s41396-021-00906-0
Belsky, A. J. (1987). The effects of grazing: confounding of ecosystem, community and organism scales. Am. Nat. 129, 777–783. doi: 10.1086/284674
*Bishopp, T. (2020). How a grazing plan prevented a wreck. On Pasture. Available online at: https://onpasture.com/2020/01/13/how-a-grazing-plan-prevented-a-wreck/ (accessed 27 April, 2022).
Bouchenak-Khelladi, Y., Verboom, G. A., Hodkinson, T. R., Salamin, N., Francois, O., Chonghaile, G. N., et al. (2009). The origins and diversification of C4 grasses and savanna-adapted ungulates. Glob. Chang. Biol. 15, 2397–2417. doi: 10.1111/j.1365-2486.2009.01860.x
*Briske, D. D., Derner, J. D., Brown, J. R., Fuhlendorf, S. D., Teague, W. R., Havstad, K. M., et al. (2008). Rotational grazing on rangelands: reconciliation of perception and experimental evidence. Rangeld. Ecol. Manage. 61, 3–17. doi: 10.2111/06-159R.1
*Briske, D. D. N. F, Sayre, N. F., Huntsinger, L., Fernandez-Gimenez, M., Budd, B., et al. (2011). Origin, persistence, and resolution of the rotational grazing debate: integrating human dimensions into rangeland research. Rangeld. Ecol. Manage. 64, 325–334. doi: 10.2111/REM-D-10-00084.1
Buis, G. M., Blair, J. M., Burkepile, D. E., Burns, C. E., Chamberlain, A. J., Chapman, P. L., et al. (2009). Controls of aboveground net primary production in mesic savanna grasslands: an inter-hemispheric comparison. Ecosystems 12, 982–995. doi: 10.1007/s10021-009-9273-1
Cassidy, L. R., and Kleppel, G. S. (2017). Effect of grazing regime on grassland breeding bird abundance. Northeast. Naturalist. 24, 86–98.
Cingolani, A. M., Noy-Meir, I., and Díaz, S. (2005). Grazing effects on rangeland diversity: a synthesis of contemporary models. Ecol. Appl. 15, 757–773. doi: 10.1890/03-5272
Clark, M. A., Domingo, N. G. G., Kolgan, K, Thakrar, S. K., Tilman, D., Lynch, J., et al. (2020). Global food system emissions could preclude achieving the 1.5° and 2° C climate change targets. Science. 270, 705–708.
Coetsee, C., Stock, W. D., and Craine, J. M. (2010). Do grazers alter nitrogen dynamics on grazing lawns in a South African savannah? Afr. J. Ecol. 49, 62–69. doi: 10.1111/j.1365-2028.2010.01236.x
Collins, S. L., Knapp, A. K., Briggs, J. M., Blair, J. M., and Steinauer, E. M. (1988). Modulation of diversity by grazing and mowing in native tallgrass prairie. Science 280, 745–747. doi: 10.1126/science.280.5364.745
Collins, S. L., and Smith, M. D. (2003). Sale-dependent interaction of fire and grazing on community heterogeneity in tallgrass prairie. Ecology 87, 2058–2067. doi: 10.1890/0012-9658(2006)87[2058:SIOFAG]2.0.CO;2
Collins, S. L., and Uno, G. E. (1985). Seed predation, seed dispersal, and disturbance in grasslands: a comment. Am. Nat. 125, 866–872 doi: 10.1086/284384
Conant, R. T., Cerri, C. E. P., Osborne, B. B., and Paustian, K. (2017). Grassland management impacts on soil carbon stocks: a new synthesis. Ecol. Appl. 27, 662–668. doi: 10.1002/eap.1473
Connell, J. H. (1978). Diversity in tropical rainforests and coral reefs. Science 199, 1302–1310. doi: 10.1126/science.199.4335.1302
Coughenour, M. B. (1985). Graminoid responses to grazing by large herbivores: adaptations, exaptations, and interacting processes. Ann. Mo. Bot. Gard. 72, 852–863. doi: 10.2307/2399227
Daley, C. A., Abbott, A., Doyle, P. S., Nader, G. A., and Larson, S. (2010). A review of fatty acid profiles and antioxidant content in grass-fed and grain-fed beef. Nutr. J. 9:10. doi: 10.1186/1475-2891-9-10
Daubenmire, R. F. (1940). Plant succession due to overgrazing in the Agropyron bunchgrass prairie of Southeastern Washington. Ecology 21, 55–64. doi: 10.2307/1930618
Davidson, E. A., David, M. B., Galloway, J. N., Goodale, C. L., Haeuber, R., Harrison, J. A., et al. (2013). Excess nitrogen in the U.S. environment: Trends, risks and solutions. Issues in Ecology. Washington, D.C.: Ecological Society of America, 15.
De Mazancourt, C., Loreau, M., and Abbadie, L. (1998). Grazing optimization and nutrient cycling: when do herbivores enhance plant production? Ecology 79, 2242–2252. doi: 10.1890/0012-9658(1998)079[2242:GOANCW]2.0.CO;2
*Derner, J. D., and Hart (2007). Grazing-induced modifications to peak standing crop in northern mixed-grass prairie. Rangeld. Ecol. Manage. 60, 270–276. doi: 10.2111/1551-5028(2007)60[270:GMTPSC]2.0.CO;2
*Derner, J. D., and Schuman, G. E. (2007). Carbon sequestration and rangelands: a synthesis of land management and precipitation effects. J. Soil Water Conserv. 62, 77–85.
Díaz, S., Lavorel, S., McIntyre, S., Falczuk, V., Casanoves, F., Milchunas, D. G., et al. (2007). Plant trait responses to grazing—a global synthesis. Global Change Biol. 13, 313–341. doi: 10.1111/j.1365-2486.2006.01288.x
Doran, J. W. (2002). Soil health and global sustainability: Translating science into practice. Agricul. Ecosys. Environ. 88, 119–127.
Estes, R. (1976). The significance of breeding synchrony in the wildebeest. East Afr. Wildl. J. 14, 135–152. doi: 10.1111/j.1365-2028.1976.tb00158.x
FAO (Food and Agriculture Organization) (2018). Transforming the Livestock Sector Through the Sustainable Development Goals. Rome: The United Nations.
Fay, P. A., Prober, S. M., Harpole, W. S., Knops, J. M. H., et al. (2015). Grassland productivity limited by multiple nutrients. Nat. Plant. 1, 15080. doi: 10.1038/nplants2015.80
Frank, D. A. (2005). The interactive effects of grazing ungulates and aboveground production on grassland diversity. Oecologia 143, 629–634. doi: 10.1007/s00442-005-0019-2
Frank, D. A. (2006). “Large herbivores in heterogeneous grassland ecosystems,” in Large Mammalian Herbivores, Ecosystem Dynamics, and Conservation, eds K. Danell, R. Bergström, P. Duncan, and J. Pastor (Cambridge: Cambridge University Press). doi: 10.1017/CBO9780511617461.013
Frank, D. A. (2007). Drought effects on above and below ground production of a grazed temperate grassland ecosystem. Oecologia. 152, 131–139.
Frank, D. A., and Groffman, P. M. (1998). Denitrification in a semi-arid grazing system. Oecologia 117, 564–569. doi: 10.1007/s004420050693
Frank, D. A., and McNaughton, S. J. (1992). The ecology of plants, large mammalian herbivores and drought in Yellowstone National Park. Ecology 73, 2043–2058. doi: 10.2307/1941454
Frank, D. A., McNaughton, S. J., and Tracy, B. (1998). The ecology of the earth's grazing ecosystems. Bioscience 48, 513–521. doi: 10.2307/1313313
Frank, D. A., Wallen, R. L., Hamilton, E. W. III., White, P. J., and Fridley, J. D. (2018). Manipulating the system: how large herbivores control bottom-up regulation of grasslands. J.Ecol. 106, 434–443. doi: 10.1111/1365-2745.12884
Frank, D. A., Wallen, R. L., and White, P. J. (2016). Ungulate control of grassland production: grazing intensity and ungulate species composition in Yellowstone Park. Ecosphere 7, 1–10. doi: 10.1002/ecs2.1603
Fryxell, J. M. (1991). Forage quality and aggregation by large herbivores. Am. Nat. 138, 478–498 doi: 10.1086/285227
Fryxell, J. M., Greever, J., and Sinclair, A. R. E. (1988). Why are migratory ungulates so abundant? Am. Nat. 131, 781–798. doi: 10.1086/284822
Fryxell, J. M., Mosser, A., Sinclair, A. R. E., and Packer, C. (2007). Group formation stabilizes predator–prey dynamics. Nature 449, 1041–1044. doi: 10.1038/nature06177
Fryxell, J. M., and Sinclair, A. R. E. (1989). Causes and consequences of migration by large herbivores. Trends Ecol. Evol. 3, 237–241. doi: 10.1016/0169-5347(88)90166-8
Gantuya, B., Biro, M., Molnar, A., Avar, A., Buhraman, A. S., Babai, D., et al. (2021). How Mongolian herders perceive ecological change in a “stable” landscape. Ecol. Soc. 26:21. doi: 10.5751/ES-12454-260221
Geremia, C., Merkle, J. A., Eacker, D. R., Wallen, R. L., White, P. J., Hebblewhite, M., and Kauffmanet, M. J. (2019). Migrating bison engineer the green wave. Proc. Natl. Acad. Sci.U. S. A. 116, 25707–25713. doi: 10.1073/pnas.1913783116
*Girard Cartier, C. B. (2017). Grazing and the Coupling of Vascular Plant and Soil Microbial Diversity in Agricultural Landscapes of Eastern New York. Dissertation, State University of New York, Albany, NY.
*Girard Cartier, C. B., and Kleppel, G. S. (2015). Grazing as a control for the spread of Mile-a-Minute (Persicaria perfoliata) and the restoration of biodiversity in plant communities in a lower New York State parkland. Ecol. Restor. 33, 82–89. doi: 10.3368/er.33.1.82
*Girard Cartier, C. B., and Kleppel, G. S. (2017). Grazing and the coupling of biodiversity in the vascular plant and soil microbial communities. Special Issue on the Natural History of Agricultural Landscapes. Northeast. Nat. 24, 67–85. doi: 10.1656/045.024.0sp806
*Goodloe, S. (1969). Short duration grazing in Rhodesia. J. Range Manage. 22, 369–373. doi: 10.2307/3895844
Gordon, I. J. (2018). Review: livestock production increasingly replaces wildlife across the globe. Animal. 12, s372–s282. doi: 10.1017/S1751731118001349
Gould, S. J. (1986). Evolution and the triumph of homology, or why history matters. Am. Sci. 74, 60–69.
Groffman, P. M., Williams, C. O., Pouyant, R. V., Band, L. E., and Yesilinis, I. D. (2009). Nitrate leaching and nitrous oxide flux in urban forests and grasslands. J. Environ. Qual. 38, 1848–1860. doi: 10.2134/jeq2008.0521
Hackmann, T. J., and Spain, J. N. (2010). Invited review: ruminant ecology and evolution: perspectives useful to ruminant livestock research and production. J. Dairy Sci. 93, 1320–1334. doi: 10.3168/jds.2009-2071
Hamilton, E. A. I. I. I., Frank, D. A., Hinchey, P. M., and Murray, M. R. (2008s). Grazer-induced increases in root exudation trigger positive feedbacks in a temperate grassland. Soil Biol. Biochem. 40, 2865–2873. doi: 10.1016/j.soilbio.2008.08.007
Hamilton, E. W., and Frank, D. A. (2001). Can plants stimulate soil microbes and their own nutrient supply? Evidence from a grazing tolerant grass. Ecology 82, 2397–2402. doi: 10.1890/0012-9658(2001)082[2397:CPSSMA]2.0.CO;2
Hamilton, W. D. (1971). Geometry for the selfish herd. J. Theor. Biol. 31, 295–311. doi: 10.1016/0022-5193(71)90189-5
*Hart, R. H. (2001). Plant biodiversity on shortgrass steppe after 55 years of zero, light, moderate, or heavy cattle grazing. Plant Ecol. 155, 111–118. doi: 10.1023/A:1013273400543
Harun-Or-Rashid, M., and Chung, Y. R. (2017). Induction of systemic resistance against insect herbivores by beneficial microbes. Front. Plant Sci. 8:1816. doi: 10.3389/fpls.2017.01816
*Hawkins, H.-J., Venter, Z.-S., and Cramer, M. D. (2022). A holistic view of Holistic Management: what do farm-scale, carbon, and social studies tell us? Agric. Ecosyst. Environ. 323:107702. doi: 10.1016/j.agee.2021.107702
Hayes, G. F, and Holl, K. D. (2003). Cattle grazing impacts on annual forbs and vegetation composition of mesic grasslands in California. Conserv. Biol. 17, 1694–1702. doi: 10.1111/j.1523-1739.2003.00281.x
Hempson, G. P., Archibald, S., Bond, W. J., Ellis, R. P., Grant, C. C., Kruger, F. J., et al. (2015). Ecology of grazing lawns in Africa. Biol. Rev. 90, 979–994. doi: 10.1111/brv.12145
Henneron, L., Cros, C., Picon-Cochard, C., Rahimian, V., and Fontaine, S. (2020). Plant economic strategies of grassland species control soil carbon dynamics through rhizodeposition. J.Ecol. 108, 528–545. doi: 10.1111/1365-2745.13276
Herrero, M., Havlik, P., Valin, H., Notenbaert, A., Rofino, M. C., and Thornton, P. K. (2013). Biomass use, production, feed efficiencies, and greenhouse gas emissions from global livestock systems. Proc. Natl. Acad. Sci. U. S. A. 110, 20888–20893. doi: 10.1073/pnas.1308149110
Herrero-Jáuregui, C., and Oestwald, M. (2018). Effects of grazing intensity on plant richness and diversity: a meta-analysis. Oikos 127, 757–766. doi: 10.1111/oik.04893
Hodkinson, T. R. (2018). Evolution and taxonomy of the grasses (Poaceae): a model family for the study of species-rich groups. Annu. Plant Rev. 1, 1–39. doi: 10.1002/9781119312994.apr0622
Hounshell, A. G., Fegley, S. R., Hall, N. S., Osburn, C. L., and Paerl, H. W. (2022). Riverine discharge and phytoplankton biomass control dissolved and particulate organic matter dynamics over spatial and temporal scales in the Neuse River Estuary, North Carolina. Estuar. Coasts 45, 96–113. doi: 10.1007/s12237-021-00955-w
Howarth, R. W., and Marino, R. (2006). Nitrogen as the limiting nutrient for eutrophication in coastal marine ecosystems: evolving views over three decades. Limnol. Oceanogr. 51, 364–376. doi: 10.4319/lo.2006.51.1_part_2.0364
Hunninck, L., May, R., Jackson, C. R., Palme, R., Røskaft, E., and Sheriff, M. J. (2020). Consequences of climate-induced vegetation changes exceed those of human disturbance for wild impala in the Serengeti ecosystem. Conserv. Physiol. 8:coz117. doi: 10.1093/conphys/coz117
Iravani, M., Schütz, M., Edwards, P. J., Risch, A. C., Scheidegger, C., and Wagner, H. H. (2011). Seed dispersal in red deer (Cervus elaphus L.) dung and its potential importance for vegetation dynamics in subalpine grasslands. Basic Appl. Ecol. 12, 505–515. doi: 10.1016/j.baae.2011.07.004
*Jackson, W. (2002). Natural systems agriculture: a truly radical alternative. Agric. Ecosyst. Environ. 88, 111–117. doi: 10.1016/S0167-8809(01)00247-X
Jacobs B. F. Kingston J. D. and. Jacobs L. L. (1999). The origin of grass-dominated ecosystems. Ann. Mo. Bot. Gard. 86, 590–643. doi: 10.2307/2666186
Janis, C. M. (1993). Tertiary mammal evolution in the context of changing climates, vegetation and tectonic events. Annu. Rev. Ecol. Syst. 24, 467–500. doi: 10.1146/annurev.es.24.110193.002343
Jardine, J. T., and Anderson, M. (1919). Range management on the National Forests. USDA Bull. 790. doi: 10.5962/bhl.title.64562
Jarman, P. J. (1974). The social organization of antelope in relation to their ecology. Behaviour 48, 215–266. doi: 10.1163/156853974X00345
Jernvall, J., and Fortelius, M. (2002). Common mammals drive the evolutionary increase of hypsodonty in the Neogene. Nature 417, 538–540. doi: 10.1038/417538a
Karki, J. B., Jhala, Y. V., and Khanna, P. P. (2000). Grazing lawns in Terai grasslands, Royal Bardia National Park, Nepal. Biotropica 32, 423–429. doi: 10.1111/j.1744-7429.2000.tb00489.x
Kauffman, M. J., Aikens, E. O., Esmaeili, S., Kaczensky, P., Middleton, A., Monteith, K. L., et al. (2021). Causes, consequences, and conservation of ungulate migration. Annu. Rev. Ecol. Evol. Syst. 52, 453–478. doi: 10.1146/annurev-ecolsys-012021-011516
Kie, J. G. (1999). Optimal foraging and risk of predation: effects on behavior and social structure in ungulates. J. Mammal. 80, 1114–1129. doi: 10.2307/1383163
Kinka, D., Schultz, J. T., and Young, J. K. (2021). Wildlife responses to livestock guard dogs and domestic sheep on open range. Glob. Ecol. Conserv. 31:e01823. doi: 10.1016/j.gecco.2021.e01823
*Kleppel, G. S. (2019). Microbial community structure in pasture and hayfield soils of the Helderberg region of New York State: a comparison of management strategies. Agroecol. Sustain. Food Syst. 43, 1031–1053. doi: 10.1080/21683565.2019.1591564
*Kleppel, G. S. (2021). Do differences in livestock management practices influence environmental impacts? Front. Sustain. Food Syst. 4:690016. doi: 10.3389/fsufs.2021.690016
*Kleppel, G. S., Girard, C., LaBarge, E., and Caggiano, S. (2011). Invasive plant control by livestock: from targeted eradication to ecosystem restoration. Ecol. Restor. 29, 209–211. doi: 10.3368/er.29.3.209
*Kleppel, G. S., and LaBarge, E. (2011). Using sheep to control of purple loosestrife (Lythrum salicaria). Invas. Plant Sci. Manage. 4, 50–57. doi: 10.1614/IPSM-D-09-00061.1
Koerner, S. E., Burkepile, D. E., Fynn, R. W. S., Burns, C. E., Eby, S., Govender, N., et al. (2014). Plant community response to loss of large herbivores differs between North American and South African savanna grasslands. Ecology 95, 808–816. doi: 10.1890/13-1828.1
Kohler-Rollefson, I. (2021). Livestock for a Small Planet. Ober-Ramstadt: League for Pastoral Peoples and Endogenous Livestock Development.
Kristensen, J. A., Svenning, J.-C., Georgiou, K., and Malhi, Y. (2022). Can large herbivores enhance ecosystem carbon persistence? Trends Ecol. Evol. 37, 117–128. doi: 10.1016/j.tree.2021.09.006
Lal, R. (2015). Sequestering carbon and increasing productivity by conservation agriculture. J. Soil Water Conserv. 70, 55A−62A. doi: 10.2489/jswc.70.3.55A
Lal, R. (2021). Integrating animal husbandry with crops and trees. Front. Sustain. Food Syst. 4:113. doi: 10.3389/fsufs.2020.00113
Laylock, W. A. (1991). Stable states and thresholds of range condition on North American rangelands: a viewpoint. J. Rangeld. Manage. 44, 427–433. doi: 10.2307/4002738
Liang, C., Amelung, W., Lehmann, J., and Kästner, M. (2019). Quantitative assessment of microbial necromass contribution to soil organic matter. Glob. Chang. Biol. 25, 3578–3590. doi: 10.1111/gcb.14781
Liu, H., Li, J., Li, X, Zheng, Y., Feng, S., and Jiang, G. (2015). Mitigating greenhouse gas emissions through replacement of chemical fertilizer with organic manure in a temperate farmland. Science Bull. 60, 598–606. doi: 10.1007/s11434-014-0679-6
Loeser, M. R., Sisk, T. D., and Crews, T. E. (2001). Plant community responses to livestock grazing: an assessment of alternative management practices in a semiarid grassland. USDA For. Serv. Proc. RMRS-P-22. Ogden, UT: U.S. Department of Agriculture, Forest Service, Rocky Mountain Research Station, 80–87.
López-Marisco, L., Altesor, A., Oyarzabal, M., Baldassini, P., and Paruclo, J. M. (2015). Grazing increases below-ground biomass and net primary production in a temperate grassland. Plant Soil 392, 155–165. doi: 10.1007/s11104-015-2452-2
Love, R. M. (1972). “Selection and breeding of grasses for forage and other uses,” in Chapter 5: The Biology and Utilization of Grasses, eds V. B. Youngner, and C. M. McKell (New York, NY: Academic Press). doi: 10.1016/B978-0-12-774750-7.50011-7
MacArthur, R. H., and Wilson, E. O. (1967). The Theory of Island Biogeography. Princeton, NJ: Princeton University Press.
Magdoff, F., and Van Es, H. (2021). Building Soils for Better Crops, 4th Edn. Washington, D.C.: U.S. Department of Agriculture, Sustainable Agriculture and Education, National Institute of Food and Agriculture.
Malik, A. A., Chowdbury, S., Schlager, V., Oliver, A., Puissaant, J., Vazguez, P. G. M., et al. (2016). Soil fungal:bacterial ratios are linked to altered carbon cycling. Front. Microbiol. 7:1247. doi: 10.3389/fmicb.2016.01247
Marty, J. T. (2005). Effects of cattle grazing on diversity in ephemeral wetlands. Conserv. Biol. 19, 1626–1632. doi: 10.1111/j.1523-1739.2005.00198.x
Mayengo, G., Piel, A. K., and Treydte, A. C. (2020). The importance of nutrient hotspots for grazing ungulates in a Miombo ecosystem, Tanzania. PLoS ONE 15:e0230192. doi: 10.1371/journal.pone.0230192
McNaughton, S. J. (1983). Compensatory plant growth as a response to herbivory. Oikos 40, 329–336. doi: 10.2307/3544305
McNaughton, S. J. (1984). Grazing lawns: animals in herds, plant form, and coevolution. Am. Nat. 124, 863–886. doi: 10.1086/284321
McNaughton, S. J. (1985). Ecology of a grazing ecosystem: The Serengeti. Ecol. Monogr. 55, 259–294. doi: 10.2307/1942578
McNaughton, S. J. (1988). Mineral nutrition and spatial concentrations of African ungulates. Nature 334, 343–345 doi: 10.1038/334343a0
McNaughton, S. J. (1990). Mineral nutrition and seasonal movements of African migratory ungulates. Nature 345, 613–615 doi: 10.1038/345613a0
McNaughton, S. J. (1991). “Evolutionary ecology of large tropical herbivores,” in Plant-Animal Interactions: Evolutionary Ecology in Tropical and Temperate Regions, eds P. W. Price, T. M. Lewinsohn, G. Wilson Fernandes, and W. W. Benson (New York, NY: John Wiley and Sons), 509–522.
McNaughton, S. J., Banyikwa, F. F., and McNaughton, M. M. (1997). Promotion of the cycling of diet-enhancing nutrients by African grazers. Science 278, 1798–1800. doi: 10.1126/science.278.5344.1798
McNaughton, S. J., Milchunas, D., and Frank, D. A. (1996). How can primary productivity be measured in grazing ecosystems? Ecology 77, 974–977. doi: 10.2307/2265518
McNaughton, S. J., Oesterheld, M., Frank, D. A., and Williams, K. J. (1989). Ecosystem-level patterns of primary productivity and herbivory in terrestrial ecosystems. Nature 341, 142–144. doi: 10.1038/341142a0
McNaughton, S. J., Ruess, R. W., and Seagle, S. W. (1988). Large mammals and process dynamics in African ecosystems. BioSci. 18, 794–800.
McSherry, M. E., and Ritchie, M. E. (2013). Effects of grazing on grassland soil carbon: a global review. Glob. Chang. Biol. 19, 1347–1357. doi: 10.1111/gcb.12144
McWhiney, G., and McDonald, F. (1985). Celtic origins of Southern herding practices. J. South. Hist. 51, 165–182. doi: 10.2307/2208823
Merkle, J. A., Monteith, K. L., Aikens, E. O., Hayes, M. M., Hersey, K. R. Middleton, A. D., et al. (2016). Large herbivores surf waves of green-up during spring. Proc. R. Soc. B 283:20160456. doi: 10.1098/rspb.2016.0456
Middleton, A. D., Merkle, J. A., McWhirter, D. E., Cook, J. G., Cook, R. C., White, P. J., et al. (2018). Green-wave surfing increases fat gain in migratory ungulates. Oikos 127, 1060–1068. doi: 10.1111/oik.05227
Milchunas, D. G., and Lauenroth, W. K. (1993). Quantitative effects of grazing on vegetation and soils over a global range of environments. Ecol. Monogr. 63, 327–366. doi: 10.2307/2937150
Milchunas, D. G., Sala, O. E., and Lauenroth, W. K. (1988). A generalized model of the effects of grazing by large herbivores on grassland community structure. Am. Nat. 132, 87–106. doi: 10.1086/284839
Millett, J., and Edmondson, S. (2015). The impact of 36 years of grazing management on soil nitrogen (N) supply rate and Salix repens N status and internal cycling in dune slacks. Plant Soil. 396, 411–420. doi: 10.1007/s11104-015-2628-9
Mligo, C. (2015). The impact of livestock grazing on soil characteristics in Mount Kilimanjaro, Tanzania. J. Geosci. Environ. Protect. 3, 24–37. doi: 10.4236/gep.2015.39004
Morton, J. K. (1972). “Phytogeography of the West African mountains,” in Taxonomy, Phytogeography and Evolution, ed D. H. Valentine (New York, NY: Academic Press), 221–236.
Msoffe, G. E. P. (2013). Indigenous knowledge in environmental conservation. Climate Change Impacts, Adaption and Mitigation Programme in Tanzania. Available online at: http://www.suanet.ac.tz/cciam/ (accessed 28 April, 2022).
Newton, P., Civita, N., Frankel-Goldwater, L., Bartel, K., and Johns, C. (2020). What is regenerative agriculture? A review of scholar and practitioner definitions based on processes and outcomes. Front. Sustain. Food Sys. 4, 577723. doi: 10.3389/fsufs.2020.577723
Oesterheld, M., and McNaughton, S. J. (1998). Effect of stress and time for recovery on the amount of compensatory growth after grazing. Oecologia 85, 303–313. doi: 10.1007/BF00320604
Olafsson, J., Stark, S., and Oksanen, L. (2004). Reindeer influences on ecosystem processes in the tundra. Oikos 105, 386–396. doi: 10.1111/j.0030-1299.2004.13048.x
Olff, H., and Ritchie, M. E. (1998). Effects of herbivores on grassland plant diversity. Trends Ecol. Evol. 13, 261–265. doi: 10.1016/S0169-5347(98)01364-0
Owen-Smith, N., Fryxell, J. M., and Merrill, E. H. (2010). Foraging theory upscaled: behavioral ecology of herbivore movement. Philos. Trans. R. Soc. B 365, 2267–2278. doi: 10.1098/rstb.2010.0095
Paerl, H. W., Pinckney, J. L., Fear, J. M., and Peierls, B. (1998). Ecosystem responses to internal and watershed organic matter loading: consequences for hypoxia in the eutrophying Neuse River Estuary, North Carolina, U.S.A. Mar. Ecol. Prog. Ser. 166, 17–25. doi: 10.3354/meps166017
Page, M. J., McKenzie, J. E., Bossuyt, P. M., and Boutron, I. 23 others. (2021). The PRISMA 2020 statement: an updated guideline for reporting systematic reviews. PLoS Med. 18:e1003583. doi: 10.1371/journal.pmed.1003583
*Park, J.-Y., Ale, S., Teague, W. R., and Jeong, J. (2017). Evaluating the ranch and watershed scale impacts of using traditional and adaptive multi-paddock grazing on runoff, sediment and nutrient losses in North Texas, USA. Agric. Ecosyst. Environ. 240, 32–44. doi: 10.1016/j.agee.2017.02.004
Parker, D. B., Meyer, B. E., Jennings, T., Jennings, J., Dougherty, H., Cole, A. N., et al. (2018). Enteric nitrous oxide emissions from beef cattle. Prof. Anim. Sci. 34, 594–607. doi: 10.15232/pas.2018-01769
Penner, J. F., and Frank, D. A. (2021). Density-dependent plant growth drives grazer stimulation of aboveground net primary production in Yellowstone grasslands. Oecologia 196, 851–861. doi: 10.1007/s00442-021-04960-5
*Phukubye, K., Mutema, M., Buthelezi, N., Muchaonyerwa, P., Cerri, C., and Chaplot, V. (2022). On the impact of soil management on soil carbon stocks: a worldwide meta-analysis. Geoder. Reg. 28:e00479. doi: 10.1016/j.geodrs.2021.e00479
Piperno, D. R., and Sues, H.-D. (2005). Dinosaurs dined on grass. Science 310, 1126–1128. doi: 10.1126/science.1121020
Prasad, V., Strömberg, C. A., Leaché, A. D., Samant, B., Patnaik, R., Tang, L., et al. (2011). Late Cretaceous origin of the rice tribe provides evidence for early diversification in Poaceae. Nat. Commun. 2:480. doi: 10.1038/ncomms1482
Prasad, V, Strömberg, C. A. E., Alimohammadian, H., and Sahni, A. (2005). Dinosaur coprolites and the early evolution of grasses and grazers. Science 310, 1177–1180. doi: 10.1126/science.1118806
*Rapiya, M., Hawkins, V.-J., Muchanje, V., Mupangua, J. F., Marufu, M. C., Dzama, K., et al. (2019). Rotational grazing approaches lowers external and internal parasite loads in cattle. Afr. J. Range Forage Sci. 36, 151–159. doi: 10.2989/1026019.2019.1628104
Reynolds, H. L., Packer, A., Bever, J. D., and Clay, K. (2003). Grassroots ecology: plant-microbe-soil interactions as drivers of plant community structure and dynamics. Ecology 84, 2281–2291. doi: 10.1890/02-0298
Risch, A. C., Sch?tz, M., Vandegehuchte, M. L., van der Putten, W. H., Duyts, H., Raschein, U., et al. (2015). Aboveground vertebrate and invertebrate herbivore impact on net N mineralization in subalpine grasslands. Ecology 96, 3312–3322. doi: 10.1890/15-0300.1
Ritchie, M. E. (2014). Plant compensation to grazing and soil carbon dynamics in a tropical grassland. Peer J. 2:e233; doi: 10.7717/peerj.233
Ritchie, M. E., Tilman, D., and Knops, J. M. H. (1998). Herbivore effects on plant and nitrogen dynamics in oak savanna. Ecology 79, 165–177. doi: 10.1890/0012-9658(1998)079[0165:HEOPAN]2.0.CO;2
Rodale Institute. (2022). Our story. Available online at: https://rodaleinstitute.org/about/our-story. (accessed October 3, 2022).
*Rowntree, J. E., Ryals, R., DeLonge, M. S., Teague, W. R., Chiavegato, M. B., Byck, P., et al. (2019). Potential mitigation of midwest grass-finished beef production emissions with soil carbon sequestration in the United States of America. Future Food J. Food Agric. Soc. 4, 31–38.
Saarinen, J. (2019). “The palaeontology of browsing and grazing,” in The Ecology of Browsing and Grazing II. Ecological Studies eds I. J. Gordon and H. H. T. Prins (Bade-Wuertemberg: Springer), 5–60. doi: 10.1007/978-3-030-25865-8_2
*Sacks, A. D., Teague, R., Provenza, F., Itzkan, S., and Laurie, J. (2014). “Restoring atmospheric carbon dioxide to pre-industrial levels: re-establishing the evolutionary grassland-grazer relationship,” in Geotherapy, eds. T. Goreau, R. W. Larson, J. Campe (Boca Raton, FL: CRC Press), 155–194.
Sankaran, M., and Augustine, D. J. (2004). Large herbivores suppress herbivore abundance in a semiarid grazing ecosystem. Ecology 85, 1052–1061. doi: 10.1890/03-0354
*Savory, A. (1978). “A holistic approach to ranch management using short duration grazing,” in Proceedings of the 1st International Rangeland Congress, ed D. N. Hyder (Denver, CO: Society for Range Management), 555–557.
*Savory, A. (1983). The Savory grazing method or holistic resource management. Rangelands 5, 155–159.
*Savory, A. (2013). How to green the world's deserts and reverse climate change. TEDxTalk, Long Beach, CA, 4 March, 2013. Available online at: https://www.bing.com/videos/search?q=alan±savory±tedx±talkanddocid=608046036138919958andmid=A979A6423A680ED5400CA979A6423A680ED5400Candview=detailandFORM=VIRE (accessed 8 July, 2020).
Savory, A., and Butterfield, J. (2016). Holistic Management, 3rd edition. Washington, DC: Island Press.
Sawyer, H., and Kauffman, M. J. (2011). Stopover ecology of a migratory ungulate. J. Anim. Ecol. 80, 1078–1087. doi: 10.1111/j.1365-2656.2011.01845.x
Scarnecchia, D. L. (1985). The animal-unit and animal-unit-equivalent concepts in range science. J. Range Sci. Manage. 38, 346–349. doi: 10.2307/3899419
Schmitz, O. J., Raymond, P. A., Estes, J. A., Kurz, W. A., Holtgrieve, G. W., Ritchie, M. E., et al. (2014). Animating the carbon cycle. Ecosystems 17, 344–359. doi: 10.1007/s10021-013-9715-7
Schrama, M., Veen, G. F., Bakker, E. S., Ruifrok, J. L., Bakker, J. P., and Olff, H. (2013). An integrated perspective to explain nitrogen mineralization in grazed ecosystems. Perspect. Plant Ecol. 15, 32–44. doi: 10.1016/j.ppees.2012.12.001
Seneviratne, G., Weerasekara, M. L. M. A.W., Kumaresan, D., and Zavahir, J. S. (2017). “Microbial signaling in plant—microbe interactions and its role on sustainability of agroecosystems,” in Agro-Environmental Sustainability, eds J. Singh and G. Seneviratne (Cham: Springer), 1–17. doi: 10.1007/978-3-319-49724-2_1
Sinclair, A. R. E. (1974). “The social organization of the East African buffalo (Syncerus caffer Sparr-man),” in The Behaviour of Ungulates and its Relation to Management, eds V. Geist and F. Walther (Morges: IUCN), 676–689.
Sitters, J., Beest, M., Cherif, M., Giesler, R., and Olofsson, J. (2017). Interactive effects between reindeer and habitat fertility drive soil nutrient availabilities in arctic tundra. Ecosystems 20, 1266–1277. doi: 10.1007/s10021-017-0108-1
Sitters, J., and Olde Venteerink, H. (2015). The need for a novel integrative theory on feedbacks between herbivores, plants and nutrient cycling. Plant Soil 396, 421–426. doi: 10.1007/s11104-015-2679-y
Skroblin, A., Carboon, T., Bidu, G., Chapman, N., Miller, M., Taylor, W., et al. (2020). Including indigenous knowledge in species distribution modeling for increased ecological insights. Conserv. Biol. 35, 587–597. doi: 10.1111/cobi.13373
*Smart, A. J., Derner, J. D., Hendrickson, J. R., Gillen, R. L., Dunn, B. H., Mousel, E. M., et al. (2010). Effects of grazing pressure on efficiency of grazing on Norther American Great Plains rangelands. Rangeld. Ecol. Manage. 60, 397–406. doi: 10.2111/REM-D-09-00046.1
Smith, F., Smith, R. E. E., Lyons, S. K., and Payne, J. L. (2018). Body size downgrading of mammals during the late Quaternary. Science 360, 310–313. doi: 10.1126/science.aao5987
Sommerseth, I. (2011). Archaeology and the debate on the transition from reindeer hunting to pastoralism. Rangifer 31, 111–127. doi: 10.7557/2.31.1.2033
*Stanley, P. L., Rowntree, J. E., Beede, D. K., DeLonge, M. S., and Hamm, M.W. (2018). Impacts of soil carbon sequestration on life cycle greenhouse gas emissions in Midwestern USA beef finishing systems. Agric. Syst. 162, 249–258. doi: 10.1016/j.agsy.2018.02.003
Stark, S., and Grellmann, D. (2002). Soil microbial responses to herbivory in an arctic tundra heath at two levels of nutrient availability. Ecology 83, 2736–2744. doi: 10.1890/0012-9658(2002)083[2736:SMRTHI]2.0.CO;2
Stebbins, G. L. (1981). Coevolution of grasses and herbivores. Ann. Mo. Bot. Gard. 68, 75–86. doi: 10.2307/2398811
Stohlgren, T. J., Schell, L. D., and Heuvel, B. V. (1999). How grazing and soil quality affect native and exotic plant diversity in Rocky Mountain grasslands. Ecol. Appl. 9, 45–64. doi: 10.1890/1051-0761(1999)009[0045:HGASQA]2.0.CO;2
Sun, G., Zhu-Barker, X., Chen, D., Liu, L., Zhang, N., Shi, C., et al. (2017). Responses of root exudation and nutrient cycling to grazing intensities and recovery practices in an alpine meadow: an implication for pasture management. Plant Soil 416, 515–525. doi: 10.1007/s11104-017-3236-7
*Teague, R., and Krueter, U. (2021). Managing grazing to restore soil health, ecosystem function and ecosystem services. Front. Sustain. Food Syst. 4:534187. doi: 10.3389/fsufs.2020.534187
*Teague, R., Provenza, F., Kreuter, U., Steffens, T., and Barnes, M. (2013). Multi-paddock grazing on rangelands: why the perceptual dichotomy between research results and rancher experience? J. Environ. Manage. 128, 699–717. doi: 10.1016/j.jenvman.2013.05.064
*Teague, W. R., Apfelbaum, S., Lal, R., Kreuter, U. P., Rowntree, J., Davies, C. A., et al. (2016). The role of ruminants in reducing agriculture's carbon footprint in North America. J. Soil Water Conserv. 71, 156–164. doi: 10.2489/jswc.71.2.156
*Teague, W. R., Dowhower, S. L., Baker, S. A., Haile, N., DeLaune, P. B., and Conover, D. M. (2011). Grazing management impacts on vegetation, soil biota, soil chemical, physical and hydrological properties in tall grass prairie. Agric. Ecosyst. Environ. 141, 310–322. doi: 10.1016/j.agee.2011.03.009
Teague, W. R., Provenza, F. D., Norton, B. E., Steffens, T., Barnes, M. K., Kothmann, M. M., et al. (2009). “Benefits of multi-paddock grazing on rangelands: Limitations of experimental grazing research and knowledge gaps,” in Grasslands: Ecology, Management and Restoration, ed. H.G. Schroder (New York, NY: Nova Science Publishers) 41–80.
Thapa, S. K., de Jong, J. F., Subedi, N., Hof, A. R., Corradini, G., Basnet, S., et al. (2021). Forage quality in grazing lawns and tall grasslands in the subtropical region of Nepal and implications for wild herbivores. Glob. Ecol. Conserv. 30:e01747. doi: 10.1016/j.gecco.2021.e01747
Tsahar, E., Izahaki, I., Lev-Yardun, S., and Bar-Oz, G. (2009). Distribution and extinction of ungulates during the Holocene of the Southern Levant. PLoS ONE 4:e5316. doi: 10.1371/journal.pone.0005316
Turner, B. L. II., and Lawrence, D. (2012). “Land architecture in the Maya lowlands: implications for sustainability,” in Biodiversity in Agriculture, eds P. Gepts, T. R. Famula, R. L. Bettinger, S. B. Brush, A. B. Damania, P. E. McGuire, and C. O. Qualset (Cambridge: Cambridge Press), 445–463.
Turner, M. D., and Hiernaux, P. (2002). The use of herders' accounts to map livestock activities across agropastoral landscapes in Semi-Arid Africa. Landsc. Ecol. 17, 367–385. doi: 10.1023/A:1021238208019
Turner, R. E., Rabalais, N. N., and Dubravko, J. (2008). Gulf of Mexico hypoxia: alternate states and a legacy. Environ. Sci. Technol. 42, 2323–2327. doi: 10.1021/es071617k
UNEP (United Nations Environmental Program) (2009). Climate Change Science Compendium, eds C. P. Mullen and J. Jabbour (Nairobi: United Nations).
US EPA (US Environmental Protection Agency) (2019). Inventory of US Greenhouse Gas Emissions and Sinks, 1990–2017. Washington, D.C.
US EPA (US Environmental Protection Agency) (2021). Inventory of Greenhouse Gases Sources and Sinks, 1990–2019. Chapter 5, Agriculture. Washington, D.C.
van der Heidjen, M. G. A., Klironomos, J. N., Ursic, M., Moutoglis, P., Streitwolf-Engel, R., Boller, T., et al. (1998). Mycorrhizal fungal diversity determines plant biodiversity, ecosystem variability and productivity. Nature 396, 69–72. doi: 10.1038/23932
van der Heijden, M. G. A., Bardgett, R. D., and van Straalen, N. M. (2008). The unseen majority: soil microbes as drivers of plant diversity and productivity in terrestrial ecosystems. Ecol. Lett. 11, 296–310. doi: 10.1111/j.1461-0248.2007.01139.x
van der Heijden, M. G. A., and Wagg, C. (2013). Soil microbial diversity and agro-ecosystem functioning. Soil Plant 363, 1–5. doi: 10.1007/s11104-012-1545-4
Veen, G. F., Blair, J. M., Smith, M. D., and Collins, S. L. (2008). Influence of grazing and fire frequency on small-scale plant community structure and resource variability in native tallgrass prairie. Oikos 117, 859–866. doi: 10.1111/j.0030-1299.2008.16515.x
*Venter, Z. S., Hawkins, H.-J., and Cramer, M. D. (2019). Cattle don't care: animal behaviour is similar regardless of grazing management in grasslands. Agric. Ecosyst. Environ. 272, 175–187. doi: 10.1016/j.agee.2018.11.023
Verweij, R. J. T., Verrelst, J., Loth, P. E., Heitkonig, I. M. A., and Brunsting, A. M. H. (2006). Grazing lawns contribute to the subsistence of mesoherbivores on dystrophic savannas. Oikos 114, 108–116. doi: 10.1111/j.2006.0030-1299.14209.x
Wang, B., An, S., Liang, C., Liu, Y., and Kuzyakov, Y. (2021). Microbial necromass as a source of soil organic matter in global ecosystems. Soil Biol. Biochem. 162:108422. doi: 10.1016/j.soilbio.2021.108422
Wang, C., and Tang, Y. (2019). A global meta-analysis of the response of multi-taxa diversity to grazing intensity in grasslands. Environ. Res. Lett. 14:114003. doi: 10.1088/1748-9326/ab4932
Wang, K.-D., Borrego, E. J., Kenerley, C. M., and Kolomiets, M. V. (2020). Oxylipins other than jasmonic acid are xylem-resident signals regulating systemic resistance induced by Trichoderma virens in maize. Plant Cell 32, 166–185. doi: 10.1105/tpc.19.00487
Wardle, D. A., Bardgett, R. D., Klironomos, J. N., Setälä, H., van der Putten, W. H., and Wall, D. H. (2004). Ecological linkages between aboveground and belowground biota. Science 304, 16. doi: 10.1126/science.1094875
Webb, J., Menzi, H., Pain, B. F., Misselbrook, T. H., Dämmgen, U., Hendricks, H., et al. (2005). Managing ammonia emissions from livestock in Europe. Environ. Pollut. 135, 399–406. doi: 10.1016/j.envpol.2004.11.013
Wei, C., Yu, Q., Bai, E., Lu, X., Xia, J., Kardol, P., et al. (2013). Nitrogen deposition weakens plant-microbe interaction in grassland ecosystems. Glob. Chang. Biol. 19, 3688–3697. doi: 10.1111/gcb.12348
Westoby, M., Walker, B., and Noy-Meir, I. (1989). Opportunistic management for rangelands not at equilibrium. J. Range Manage. 42, 266–274. doi: 10.2307/3899492
White, R. R., and Hall, M. B. (2017). Nutritional and greenhouse gas impacts of removing animals from US agriculture. Proc. Natl. Acad. Sci. U. S. A. 114, E10301–E10308. doi: 10.1073/pnas.1707322114
World Bank (2021). Arable Land (% of Land Area). Available online at: https://data.worldbank.org/indicator/AG.LND.ARBL.ZS (accessed 29 April, 2022).
Wright, S. F., and Upadhyaya, A. (1996). Extraction of an abundant and unusual protein from soils and comparison with hyphal protein of arbuscular mycorrhizal fungi. Soil Sci. 161, 575–586. doi: 10.1097/00010694-199609000-00003
Zachos, J., Pagani, M., Sloan, L., Thomas, E., and Billups, K. (2001). Trends, rhythms and aberrations in global climate 65 Ma to present. Science 292, 686–693. doi: 10.1126/science.1059412
Zeng, C., Jianshuang, W., and Zhang, X. (2019). Effects of grazing on above- vs. below-ground biomass allocation of alpine grasslands in the northern Tibetan Plateau. PLoS ONE 10:e0135173. doi: 10.1371/journal.pone.0135173
Keywords: wild grazing ecosystems, agricultural grazing ecosystems, regenerative livestock management, conventional livestock management, plant-grazer-soil microbe interactions
Citation: Kleppel GS and Frank DA (2022) Structure and functioning of wild and agricultural grazing ecosystems: A comparative review. Front. Sustain. Food Syst. 6:945514. doi: 10.3389/fsufs.2022.945514
Received: 16 May 2022; Accepted: 26 September 2022;
Published: 31 October 2022.
Edited by:
Rebecca Chaplin-Kramer, Stanford University, United StatesReviewed by:
Yan Yan, Institute of Mountain Hazards and Environment (CAS), ChinaBlaz Stres, University of Ljubljana, Slovenia
Copyright © 2022 Kleppel and Frank. This is an open-access article distributed under the terms of the Creative Commons Attribution License (CC BY). The use, distribution or reproduction in other forums is permitted, provided the original author(s) and the copyright owner(s) are credited and that the original publication in this journal is cited, in accordance with accepted academic practice. No use, distribution or reproduction is permitted which does not comply with these terms.
*Correspondence: Gary S. Kleppel, Z2tsZXBwZWxAYWxiYW55LmVkdQ==