- 1Laboratory of Functional Foods and Bioprocesses, Department of Food Industries Engineering, Universidad Nacional de Frontera, Sullana, Peru
- 2Laboratory of Emerging Technologies for Research and Innovation in Fruits and Vegetables, Department of Food Industries Engineering, Universidad Nacional de Frontera, Sullana, Peru
Iron deficiency in children and vulnerable people requires the intervention of effective emerging technologies to incorporate minerals into food, iron is an important micronutrient required by the human body to develop different functions. It’s oxidation and susceptibility when added directly to food hinders its absorption, impairs sensory aspects, causing rejection by consumers. Currently, efficient, low cost, high productivity, better bioaccessibility and bioavailability, microencapsulation techniques have been developed. This review focuses on the study of the different methods and techniques of iron microencapsulation and its behavior in food fortification. The type of coating material, the efficiency, yield, bioaccessibility and bioavailability evaluated for each technique. It has been shown that the most used coating materials were maltodextrin, sodium alginate, gum arabic and whey protein; while the morphological characteristics and the release profile studied from 1995 to the present, on average were in the following order: Percentage of microencapsulation (85%), yield (76%), bioavailability (60%), and bioaccessibility (52%).; However, the sensory evaluations of some foods fortified with iron microcapsules denoted a metallic taste, color and smell were also detected, decreasing their level of acceptance. These findings suggest the need for further research to establish new protocols to fortify foods while maintaining their nutritional and sensory quality.
1. Introduction
Iron (Fe) is a mineral and essential for human nutrition (Piskin et al., 2022). This item is present in the cells in the form of traces (Dasa and Abera, 2018), performing functions in the bone formation of the human body, production of energy Adenosine Triphosphate (ATP), synthesis of collagen and Red blood cells (Girelli et al., 2018); genetic differentiation and regulation synthesis of deoxyribonucleic acid (DNA); myelination of the mitochondrial and neuronal system (Gao et al., 2019). Approximately 66% of body iron is bound to hemoglobin, 8% to myoglobin, heme enzymes andiron-sulfur clusters. The rest (26%) is stored in the form of ferritin and hemosiderin in the liver (Figure 1; Ilich, 2020; Hall and Guyton, 2021; Salazar et al., 2021).
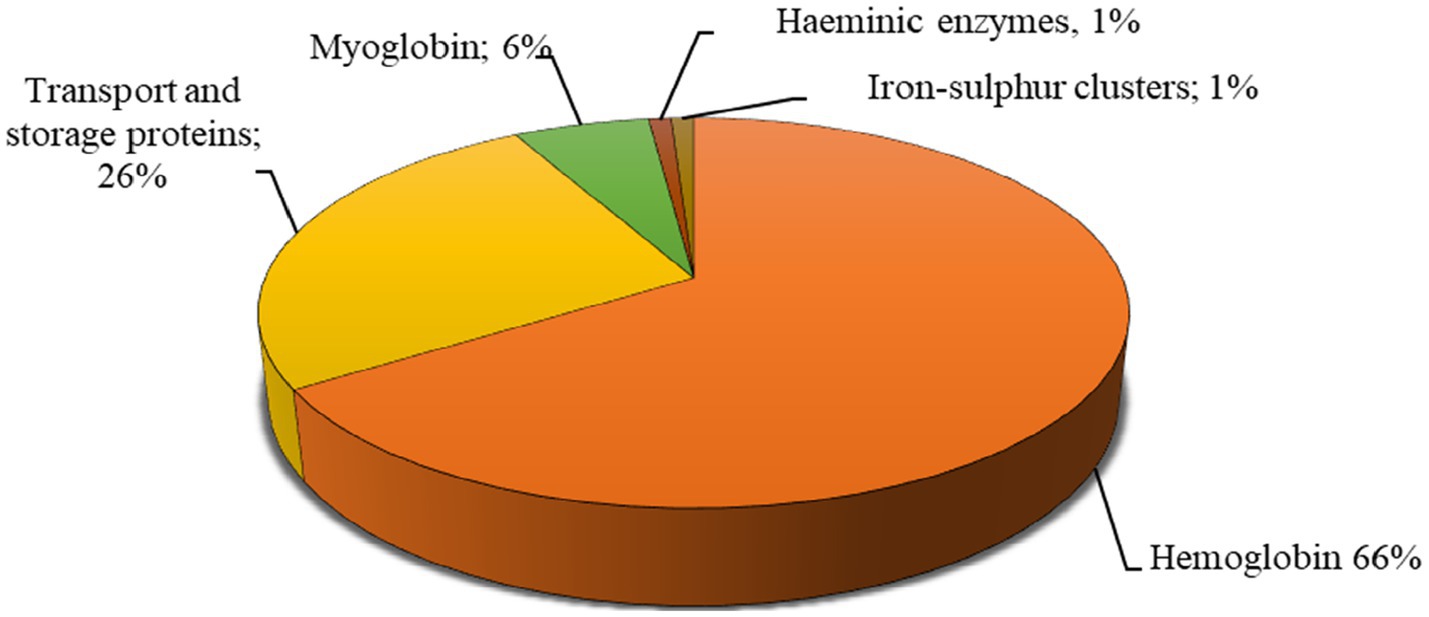
Figure 1. Iron proportions located in the human body. Adapted from Hall and Guyton (2021).
Food offers two sources of iron which are used by the human being; heme or heme iron, acquired by eating foods of animal origin with an absorption between 15 and 35% and non-heme iron, obtained from vegetables with an absorption of less than 10% (Skolmowska and Głąbska, 2019; Zhuo et al., 2019).
Iron deficiency in the body can contribute to the development of various diseases such as iron deficiency anemia (Cappellini et al., 2020), heart failure (Naito et al., 2021), kidney pathologies (Batchelor et al., 2020), intestinal inflammation (Nielsen et al., 2018), and development of neurobehavioral disorders (Pivina et al., 2019), 42% of children between 0 and 5 years of age, 33% of women of reproductive age and 40% of pregnant women suffer from iron deficiency anemia (El Bilbeisi et al., 2022), being Peru one of the countries whose population shows high rates of iron deficiency anemia (40% of children from 0 to 3 years of age) (Barrios Renteria et al., 2022), 28.3% of pregnant women between 15 and 18 years of age and late ages of 35 years and over, women who do not have health insurance (Espinola Sanchez et al., 2021).
The iron requirement in pregnant women is 27 mg/day (Cusick et al., 2018), while in children from 24 to 59 months it is 30 mg/day (Finkelstein et al., 2018). However, intestinal absorption of 1 to 2 mg/day is required for iron homeostasis to occur (Yiannikourides and Latunde-Dada, 2019). Worldwide there have been attempts to fortify commonly consumed foods with different concentrations of iron (Podder et al., 2018; Jahan et al., 2019; Pachón et al., 2021), nevertheless, the direct addition of the mineral in food negatively influences the organoleptic characteristics, mainly highlighting the metallic smell and taste, which causes limitations in its intake (Cheng et al., 2020). The use of microencapsulation to mask the sensory qualities of ferrous compounds has been extensively studied, generally using polymeric coating walls (Böger et al., 2021), which has allowed a correct bioavailability through the release and absorption in the gastrointestinal tract (Gaigher et al., 2022).
So far, several microencapsulation techniques, such as spray drying, spray cooling, ionic gelation, emulsification and freeze drying, have proven to be feasible for the microencapsulation of ferrous compounds (Bhosale et al., 2019). In the microencapsulation process, the encapsulation method, the wall material and the core constitute the factors that determine the product characteristics (particle size, shelf life, efficiency, performance, bioaccessibility, bioavailability and sensory aspects) (Gupta et al., 2014; Ballesteros et al., 2017; González Ortega et al., 2020). In this sense, this review aims to show the different methods and techniques of iron microencapsulation and its behavior in food fortification.
2. Microencapsulation
Microencapsulation is a coating method that confers the core (oxidizable substance, volatile additives, polyphenols, microorganisms), the necessary protection to prevent its loss or deterioration when exposed to various environmental factors (temperature, pH, oxygen), allowing it to keep the encapsulated material stable (Wang et al., 2017), reduces chemical interactions, prevents physicochemical changes and unpleasant sensory qualities (Pratap Singh et al., 2017); creating a barrier that allows controlled release and bioavailability of the active component at the time of ingestion, and facilitates transport and handling (Calderón Oliver and Ponce Alquicira, 2022; Figure 2) through a coating formed by proteins, carbohydrates and/or lipid compounds (Böger et al., 2021; Pratap-Singh and Leiva, 2021). Currently, there are different microencapsulation techniques that are low cost, easy to operate and highly efficient (Wardhani et al., 2021).
2.1. Spray drying
Among microencapsulation technologies, spray drying is considered one of the most important techniques to protect any excipient, including minerals, making use of a wide range of coating materials (Baldelli et al., 2023); the process is subjected to high temperatures (100–200°C) to shape a dry powder; the particle size ranging from 903.7 nm to 20 μm (Dueik and Diosady, 2017; Ligarda Samanez et al., 2022). Several studies have used spray drying to microencapsulate vitamins (Singh et al., 2018), probiotic microorganisms (Pratap Singh et al., 2017), animal blood cells (Ligarda Samanez et al., 2022), minerals (Cian et al., 2021), and other bioactive compounds (Ravichai and Muangrat, 2019). The advantages of this technique include ease of operation, low water activity of the final product, increased protection of some micronutrients such as iron against oxidation (Wardhani et al., 2021), particle distribution and loading capacity (Polekkad et al., 2021) and also improves iron release and absorption rate (Filiponi et al., 2019; Cian et al., 2021).
2.2. Ionic gelation
Ionic gelation is a favorable alternative to microencapsulate unstable compounds forming protein gel beads on their entire surface (Valenzuela et al., 2014). The composition of these pearls is due to the presence of sodium alginate and calcium chloride, which form a solid and stable structure with high encapsulation efficiency and soluble properties under neutral and alkaline pH conditions. These characteristics can be beneficial for the iron absorption because the duodenum has a similar pH (Yao et al., 2020). Perez-Moral et al. (2013) and Cengiz et al. (2019) performed simulations under gastrointestinal conditions under progressive release; in gastric conditions 45% was absorbed, a retention in the gastrointestinal tract of 43% and in duodenal conditions 12%; however, gels limit fortification in some food matrices (flours and powders) (Onsekizoglu Bagci and Gunasekaran, 2016) making it difficult to control the particle size, which usually varies between 3 and 5 nm (Katuwavila et al., 2016).
2.3. Single and double emulsion
Emulsion is a technique that does not require the intervention of high temperatures, has a controlled release and chemically stabilizes the encapsulation of nutrients and bioactive compounds (Choi et al., 2009; Ilyasoglu Buyukkestelli and El, 2019). There are two types of food emulsions, single emulsions with two delivery systems: oil-in-water (O/W) and water-in-oil (W/O) and double or hydrophilic emulsions composed of two inner (W1), outer (W2), and one oily (W1/O/W2) aqueous phases (Gupta et al., 2015). Single emulsion systems containing a core have a different sensory perception; one of the advantages of the simple O/W emulsion system is the core that is in direct contact with the palate, the oily phase acting as a filler causes the increase in taste perception by increasing the concentration of the core in the external aqueous phase, however, the same does not occur when working with the W/O system (Ilyasoglu Buyukkestelli and El, 2019) however, the most relevant drawback in this type of emulsions is the difficulty to turn into powder, but they do prove to be more stable than double emulsions during storage (Chang et al., 2016).
In double emulsions, the inner phase (W1) includes different solutes from the outer phase (W2), as well as the oily phase (O), which is composed of lipophilic substances, double emulsions have many applications in the food industry due to the multicompartmental system achieved by the single emulsion with suitable surfactants; some of their advantages are high efficiency during iron encapsulation, improvement of fatty acid profile and reduction of sugar/salt content in food (Ilyasoglu Buyukkestelli and Nehir El, 2019). Naktinienė et al. (2021) obtained an iron microencapsulation efficiency of 95% using polyglycerol polyricinoleate and whey protein dispersions; Ilyasoglu Buyukkestelli and Nehir El (2019) determined that the percentage bioaccessibility of ferric chloride in in vitro tests was 52.97% dispersing polyglycerol polyricinoleate in olive oil as the oily phase and whey protein isolate as the internal aqueous phase (W1) while sodium caseinate was used for the external aqueous phase (W2); this value is not constant as it increases while the dispersed phase decreases, causing the hydrolysis of sodium caseinate to act as an iron absorption enhancer; finally Katuwavila et al. (2016) found that the in vitro release rate of iron in alginate particles was 65% at 96 h under pH 7.4 compared to Churio and Valenzuela (2018) who observed the release of 31.8–37.4% under gastric conditions and around 100% of ferrous sulfate under intestinal conditions. Therefore, the double emulsion demonstrates better performance and can even microencapsulate chiral compounds.
2.4. Spray cooling
It is a technique characterized by its low cost and efficiency in the production of microparticles loaded with bioactive compounds, aromas and pigments (Figueiredo et al., 2022); its functions are based on the stabilization of ascorbic acid, vitamin D3, proanthoccocin and proanthocyanidins during storage (Carvalho et al., 2021). The technique consists of forming solid lipidic microcapsules in powder form of active sources of hydrophilic or hydrophobic origin, dispersed in dissolved lipid by spraying (Boesso et al., 2016; Sorita et al., 2021).
In one study, spray cooling has been used for the immobilization of ferrous sulfate heptahydrate using polyglycerol monostearate with a particle size of 2–5 μm, achieving an efficiency of 74% and a yield of 75% (Lee et al., 2003). However, to date there is limited research on the use of spray cooling to microencapsulate ferrous compounds, which may be related to the difficulty in finding lipid-based wall materials that meet the desired characteristics in terms of oxidative stability, food grade, melting point and storage stability (Figueiredo et al., 2022). For a better use of this technique, some additional operations could be considered and include the use of more sophisticated equipment to improve performance (Consoli et al., 2016).
2.5. Lyophilization
Freeze-drying is a technique that is performed at low temperatures, it manages to microencapsulate cells protecting them from bacteriophages and different factors during freezing and storage (Ribeiro Dias et al., 2017). It is a suitable technique for dehydration of heat-sensitive foods and microencapsulation (Desai and Park, 2005), its operations stabilize the material through four stages, firstly freezing of the core and wall material, sublimation of the lodged water avoiding friction with oxygen, desorption and finally storage (Ezhilarasi et al., 2013). Some of its advantages are related to low water activity, inhibiting the proliferation of microorganisms and improving the firmness of the microcapsules (Figueiredo et al., 2022); the technique is feasible to microencapsulate compounds intolerant to high temperatures, in addition to having a low oxidation rate (Kour et al., 2023); however, its disadvantages include the operation time (up to 20 h) and the production of porous spherical structures that cause rehydration of the microcapsules and, consequently, increased moisture (Karthik and Anandharamakrishnan, 2012). Wang et al. (2017) microencapsulated heme iron using maltodextrin, carboxymethyl cellulose and sodium caseinate achieving an efficiency of 98.64%.
Although all five techniques indicate different advantages over microcapsule formation, spray drying is considered the leading technique for protecting any excipient, including minerals, with its main advantage being the formation of microcapsules that meet physical properties (efficiency, moisture, bioavailability, and performance) that are stable in processing, packaging, distribution and storage; this is achieved by properly optimizing dry powders in formulations prior to producing microcapsules.
3. Coating or wall material
The wall material is one of the three essential factors involved in the microencapsulation process by acting as a protective agent of the core that covers the entire particle, which allows it to avoid reactions in food matrices (López Cruz et al., 2020), to form solutions with low viscosity at high concentrations, stable emulsions to form solid particles and with thermal stability (Đorđević et al., 2014; Mansour et al., 2020; Swami et al., 2021) withstand temperatures from 15 to 200°C to completely homogenize the samples (Jayalalitha et al., 2022), encapsulation is carried out continuously from a film on the particle (Karaaslan et al., 2021). Not all materials meet all the characteristics for encapsulation, for this reason, Gupta et al. (2015) and Polekkad et al. (2021) combined wall materials with similar origins and different properties to improve the efficiency and yield of microencapsulation.
The types of wall material used in microencapsulation are carbohydrates (Ribeiro Dias et al., 2017), proteins (Ying et al., 2016), and lipids (Zhu, 2017); they are characterized by being resistant to changes in temperature, oxygen and humidity, non-toxic, dissolve in acidic pH and are degraded by the endogenous microbiota of the small intestine (Pérez-Leonard et al., 2013), their stabilization depends on the time, speed of homogenization of the solutions and the ratio: core/wall material, if the amount of wall material is greater than that of the core, the microcapsules will be larger, spherical and with defined walls (Rocha-Selmi et al., 2013; Đorđević et al., 2014), the increase of solids in the formulation produces larger microparticles and lower bioavailability (Baldelli et al., 2023; Figure 2).
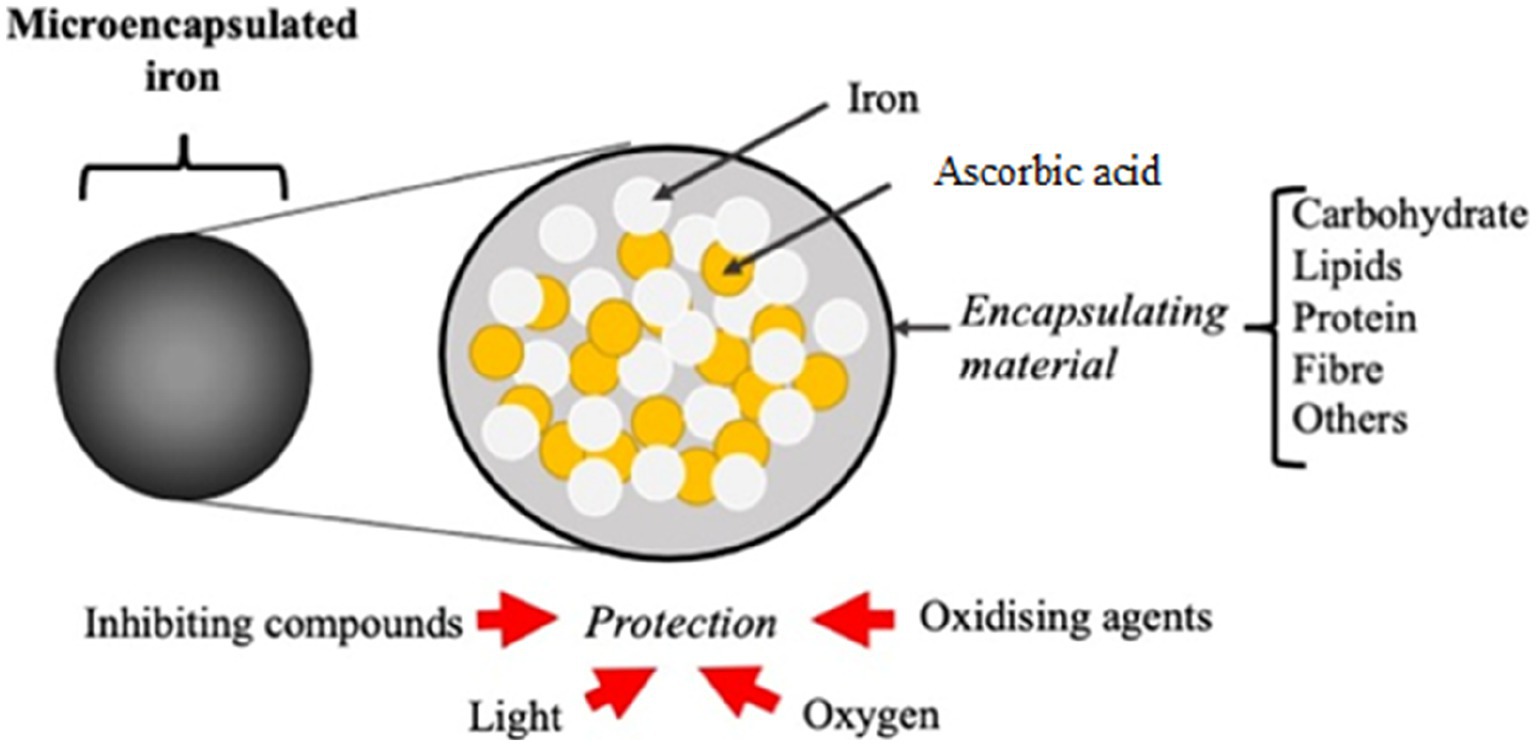
Figure 2. Model of a microcapsule of iron and ascorbic acid. Adapted from Calderón Oliver and Ponce Alquicira (2022).
The most commonly used wall materials in the microencapsulation process are: maltodextrin, gum arabic, sodium alginate, whey protein and modified starch (Krokida, 2017); maltodextrin is a white hydrolyzed starch that is prepared by heating it until soluble, it is suitable due to its low viscosity at high concentrations, good protection against oxidation and good solubility (Premi and Sharma, 2017). However, its emulsification capacity is relatively low, which implies the need to combine it with other components to improve its yield and prolong its shelf life (Kanwal et al., 2022). Some edible gums (arabic, guar, gellan, tara, and xanthan) are composed of polysaccharides and are considered a suitable material for microencapsulation due to their low viscosity at high solid concentrations (Kang et al., 2019; Karrar et al., 2021), neutral taste (Chew et al., 2018), and emulsifying properties (Karaaslan et al., 2021), gum arabic is one of the most widely used carbohydrates in microencapsulation by retaining volatile compounds during the drying process (Rojas, 2019); being suitable for the formation of films and capsules of different active compounds such as minerals and anthocyanins (Pieczykolan and Kurek, 2019).
Sodium alginate is a biopolymer extracted from brown algae (Heckert Bastos et al., 2020), thermally stable (Singh et al., 2018), has the ability to form hydrogels and degrade at acidic pH (McKinney et al., 2022), presents thickening, stabilizing and film-forming properties (López Cruz et al., 2020). These characteristics allow it to be an important material in the microencapsulation of probiotics and minerals that allows its release in the digestive environment (Motalebi Moghanjougi et al., 2021). Whey proteins (β-lactoglobulin, α-lactalbumin) have the ability to interact and form aggregates, which determines their functional and technological properties; Can be used as a protective gel and form encapsulation coatings for bioactive compounds (Onsekizoglu Bagci and Gunasekaran, 2016; Pereira et al., 2017). Microencapsulation of iron in whey protein has shown high efficiency compared to other elements such as Zinc, due to the presence of a smaller atomic radius and the electronegativity of iron, which facilitates its binding with the carboxylic functional groups of the amino acids in the protein (Pratap-Singh and Leiva, 2021).
Modified starch can be obtained naturally or synthesized by chemical, physical or enzymatic processes, has adequate bioavailability, low viscosity, high solubility and low cost (Balakrishnan et al., 2021), and can be used in spray drying processes (Tatar Turan and Kahyaoglu, 2021). The use of modified starch in combination with maltodextrin and gum arabic was studied by Gupta et al. (2014) obtaining ferrous sulfate microcapsules with good stability and microencapsulation efficiency above 90%; however, for Ribeiro et al. (2020) the limitations of modified starch are related to its undesirable taste and poor aroma protection.
4. Iron microencapsulation efficiency and yield
The yield of the microencapsulation is determined by the proportion of the compound that makes up the microencapsulated nucleus in relation to the initial dispersion (Çam et al., 2014; Pratap Singh et al., 2017; Churio and Valenzuela, 2018; González Ortega et al., 2020). Yield is calculated using the following equation:
Efficiency is the difference in the percentage of microencapsulated sample compared to the initial sample (Gupta et al., 2014; Shahidi Noghabi and Molaveisi, 2020; Castejón et al., 2021), the expression is shown below:
Other authors use the division of the non-microencapsulated sample over the initial sample minus the unit (Zhang et al., 2007).
Microencapsulation percentages can vary due to three significant factors, the form of iron, the core/material ratio, and the technique used (Asghari Varzaneh et al., 2017; Calderón Oliver and Ponce Alquicira, 2022); it has been shown that the highest percentages of efficiency (>90%) were obtained using the methods of spray drying, double emulsion, ionic gelation and freeze-drying (Table 1).
Pratap Singh et al. (2017) observed that chitosan and Eudragit used as wall material in terms of efficiency (87–98%) and yield (68–75%), showed similar values; however, Eudragit demonstrated a yield (g microcapsules/L spray solution) 10 times higher than chitosan. Since the high viscosity of chitosan made it difficult to incorporate solids (1–2%) into the spray solution, unlike Eudragit (15–20% solids). Whey protein combined with Eudraguard has also been shown to be suitable for iron microencapsulation. The interaction of iron with the carboxylic groups of the amino acids of the whey proteins improves the protein-mineral affinity, this allows reaching efficiencies above 97% (Pratap-Singh and Leiva, 2021). Ligarda Samanez et al. (2022) used a wall material composed of potato starch and tara gum, allowing 94% efficiency in the microencapsulation of Cavia Porcellus erythrocytes.
The form of iron used in the microencapsulation process has implications for efficiency and yield. A study showed that when using iron gluconate and sulfate, 87 and 75% efficiency is obtained; however, when using iron citrate and chloride the efficiency is very low due to the formation of different particles and low solubility (Baldelli et al., 2023), it should be noted that ferrous sulfate is commonly used for food supplementation with iron, which generally leads to problems related to it is high reactivity, such as damage to the gastrointestinal mucosa due to free iron ions (Slivka et al., 1985) and also the development of undesirable color and flavor in the products in which it is applied. On the other hand, the operating conditions have a direct impact on the performance of microencapsulation. Increasing the inlet temperature in spray drying from 120 to 130°C improves the yield from 45 to 72% in iron microencapsulation using chitosan as the wall material, the iron loading density in the microcapsules was 25% for those conditions; however, increasing by 20°C would result in unacceptable iron oxidation (Dueik and Diosady, 2017). Furthermore, variations in spray drying performance may be subject to iron capsules sticking to the wall of the equipment or not passing through the filter, as well as very small particles being lost to the suction created by the vacuum pump (Dueik and Diosady, 2017; Pratap Singh et al., 2017).
Katuwavila et al. (2016) used the ionic gelation method to form nanoparticles with ferrous sulfate, ascorbic acid, calcium chloride, and sodium alginate; this system can function as a source of oral supply for the treatment of anemia, since it obtained 95% efficiency and 70% release at pH 6 (gastric conditions), the negative charge of sodium alginate is the driving force for the complexation of iron with the polymer, where chelation occurs between Fe2+ and carboxylate and hydroxyl molecules. Alginate beads loaded with ferrous sulfate of 0.76 mm in diameter were produced with 81% efficiency. It should be noted that the formation of beads can be stored for 45 days without altering the encapsulation efficiency (Cengiz et al., 2019). Wang et al. (2017) guaranteed 98.64% efficiency in the microencapsulation of blood with maltodextrin, sodium caseinate and carboxymethylcellulose (CMC) as emulsifier, because it preserves red blood cell proportions and guarantees the microbial quality of the blood. On the other hand, Mukhija et al. (2016) developed a microencapsulation by emulsions because it has a higher bioavailability in the release of iron, used sodium alginate as wall material and obtained 94% efficiency.
5. Bioaccessibility and bioavailability of iron microcapsules
The in vitro bioavailability and bioaccessibility as says of the microcapsules are models that simulate gastrointestinal conditions, which make it possible to determine if the microencapsulated iron is viable for the human body, given that the microencapsulated ferrous compounds must be adequately absorbed at the gastrointestinal level (Gaigher et al., 2022). Bioaccessibility represents the percentage of mineral that is soluble and ready to be absorbed in the digestive process. The main factors influencing this solubility are the chemical form of iron and the presence of inhibitors (Toledo Barbosa and Garcia Rojas, 2022). The in vitro bioaccessibility protocol is carried out by preparing salivary fluid, gastric and intestinal fluid and verifying enzymatic activity, this method is called “In vitro static simulation of gastrointestinal digestion of food” (INFOGEST 2.0) designed by Brodkorb et al. (2019). Ilyasoglu Buyukkestelli and Nehir El (2019) evaluated the bioaccessibility in the microencapsulation of iron in the technique (W/O:W) resulting in 52.97%; by increasing the dose of iron in the first phase and keeping the second phase constant, bioaccessibility can be improved. Cian et al. (2021) used brewery grain protein concentrate (BSG-PC), locust bean gum and maltodextrin to microencapsulate ferrous sulfate, determined the bioaccessibility percentage using the protocol of Miller et al. (1981) and Drago et al. (2005), preparing controlled pH media and adding the optimization process, obtaining as a result a high percentage of bioaccessibility. This result was favored by the presence of ascorbic acid (AA) in the microcapsules, by reducing the concentration of BSG-PC, the AA/Fe molar ratio increased, giving rise to the action as a chelating agent of AA on Fe. This effect, it was also provided by BSG-PC when its concentration increased. The reducing action of BSG-PC on Fe favored the increase in bioaccessibility values.
Toledo Barbosa and Garcia Rojas (2022) adapted the simulation of digestive intake of infants and adults, resulting in two acceptable bioaccessibility values, for each case they applied the INFOGEST method under biaccessibility conditions in adults and Ménard et al. (2018) in children, obtaining 49.54% while in adults it was 39.71%; the data obtained by the mentioned methods are replaced in the following formula to determine the percentage of bioaccessibility (BC).
Differences between adults and children can be explained by pH values and enzyme activity that affected digestion (Toledo Barbosa and Garcia Rojas, 2022).
Bioavailability is determined as the percentage of ingested metal free for the metabolic process (Blanco-Rojo and Vaquero, 2019), the most widely used method is transport by CaCo-2 cells (human colon adenocarcinoma cells), used by Filiponi et al. (2019) in the microencapsulation of ferrous sulfate with maltodextrin and polydextrose, obtaining as a result a bioavailability of 56.21%; Christides et al. (2018) evaluated the availability of iron in infants supplemented with prebiotics by adding a heat treatment to the mentioned method, the formulation with the best bioavailability had a 4:1 ratio. Hemalatha et al. (2007) evaluated the bioavailability of zinc and iron in food cereals, the result with zinc was not as expected, being decreased by the heat treatments used, while with iron the results increased significantly. The direct incorporation of iron in dairy foods stimulates a reaction with milk proteins and fats, lower bioavailability and organoleptic problems (Naktinienė et al., 2021). Iron oxidation is prevented by microencapsulation by forming an impermeable membrane as a barrier to oxygen expansion, covering unacceptable tastes and odors of iron salts, increasing their bioavailability (Gupta et al., 2014). The percentage of bioavailability in vitro in the investigation of Hashem Hosseini et al. (2019) it is found from 37.28 to 42.94% by double emulsion; 63.78% ± 0.23 was the result of microencapsulated iron fortification by Gupta et al. (2014) and Baldelli et al. (2023) obtained 80% bioavailability in microparticles of ferrous sulfate with hydroxypropylmethylcellulose in the experiment of Mukhija et al. (2016) they obtained 90.4% in an iron/alginate/oil emulsion; a balance must be considered between the percentage of solids, an increase of 80% solids increases the particle size by 30% and improves its spherical shape, however, it decreases the bioavailability to 25% because the large microcapsules need more time to dissolve. It should be noted that iron microcapsules are less affected by the presence of inhibitors, so the bioavailability of iron is high (Gupta et al., 2014). Both the AA and the peptide-metal complexes in the microparticles induced a higher iron absorption and, therefore, could be a good alternative to obtain more bioavailable iron (Filiponi et al., 2019). However, poor encapsulation has been correlated with a faster release rate and lower bioavailability. For example, in dairy products, free iron interacts with casein, leading to the formation of insoluble iron in the gastrointestinal tract, which reduces it’s bioavailability (Subash et al., 2015). For this reason, it is important that the coating materials have a high molecular weight, this is not only essential for its influence on the efficiency of microencapsulation, but also for its implication on the release rate of encapsulated iron (Baldelli et al., 2023).
CC = Quantity of Cells.
C. = Cells.
6. Success rate in iron microencapsulation
Iron as a mineral has an undesirable sensory perception for its intake, despite the fact that it is vital for human health. Investigations focused on the use of technologies to modify the sensory aspects of the different forms of iron without impairing their nutritional value have been registered. Iron microencapsulation is a technology that presents the opportunity to prevent iron deficiency, increase bioavailability, avoid the perception of metallic taste, and protect from chemical reactions that occur when in contact with food matrices. The statistical data registered by the National Library of Medicine (PubMed) indicate that the iron microencapsulation method began in 1971, but it was deepened with the study of Boccio et al. (1995), when studying a new procedure to enrich liquid milk and its derivatives with iron; using ferrous sulfate as the core and soybean lecithin as the wall material, pointed out that iron absorption is influenced by some food additives, such as the presence of 10% (w/v) of cocoa, which manages to increase iron absorption, while that tea and coffee decrease these values. From this, 113 publications have been registered until the year 2023, regarding the microencapsulation of iron.
Microcapsules have numerous parameters that are studied through morphological tests and release profiles, which determine their stability and safety (Zárate-Hernández et al., 2021). The present study considers that the percentage of success in iron microencapsulation is reflected in the average value of efficiency, yield, bioavailability and bioaccessibility, parameters listed in Table 2.
Studies carried out in the years 1995–2001 used the vacuum drying method to microencapsulate ferrous sulfate in soy lecithin; in order to fortify milk and evaluate its bioavailability through the prophylactic-preventive method (Lysionek et al., 2001); the results varied from 7 to 12%, this was due to the iron-casein interaction. This is a phosphoprotein that oxidizes Fe2+ to Fe3+, producing insoluble compounds in milk, which produce an inhibitory effect on iron absorption; mechanism reflected in a low bioavailability around 12% (Boccio et al., 1998). However, in recent years new research has emerged that has allowed the microencapsulation process to be improved, obtaining better efficiency indicators.
7. Iron absorpion in the human body
The iron present in the human body fulfills three main functions, it forms myoglobin, hemoglobin, enzymes and hormones; receives and stores serum ferritin and hemosiderin when their input is decreased and transports oxygen to the tissues of the human body (Shubham et al., 2020).
The metabolism and absorption of iron is represented in Figure 3. The iron acquired by food intake is absorbed by the duodenum and proximal jejunum, giving off 3 phases of absorption: luminal, mucosal, and body (Dasa and Abera, 2018). The first phase is responsible for processing food with gastric acid, causing the release of iron from the microcapsules in its bivalent or ferrous form. The pH of gastric acid converts ferric or trivalent iron (Fe3+) to ferrous iron (Fe2+) through duodenal cytochrome B enzyme in the brush border of enterocytes (Lo et al., 2022).
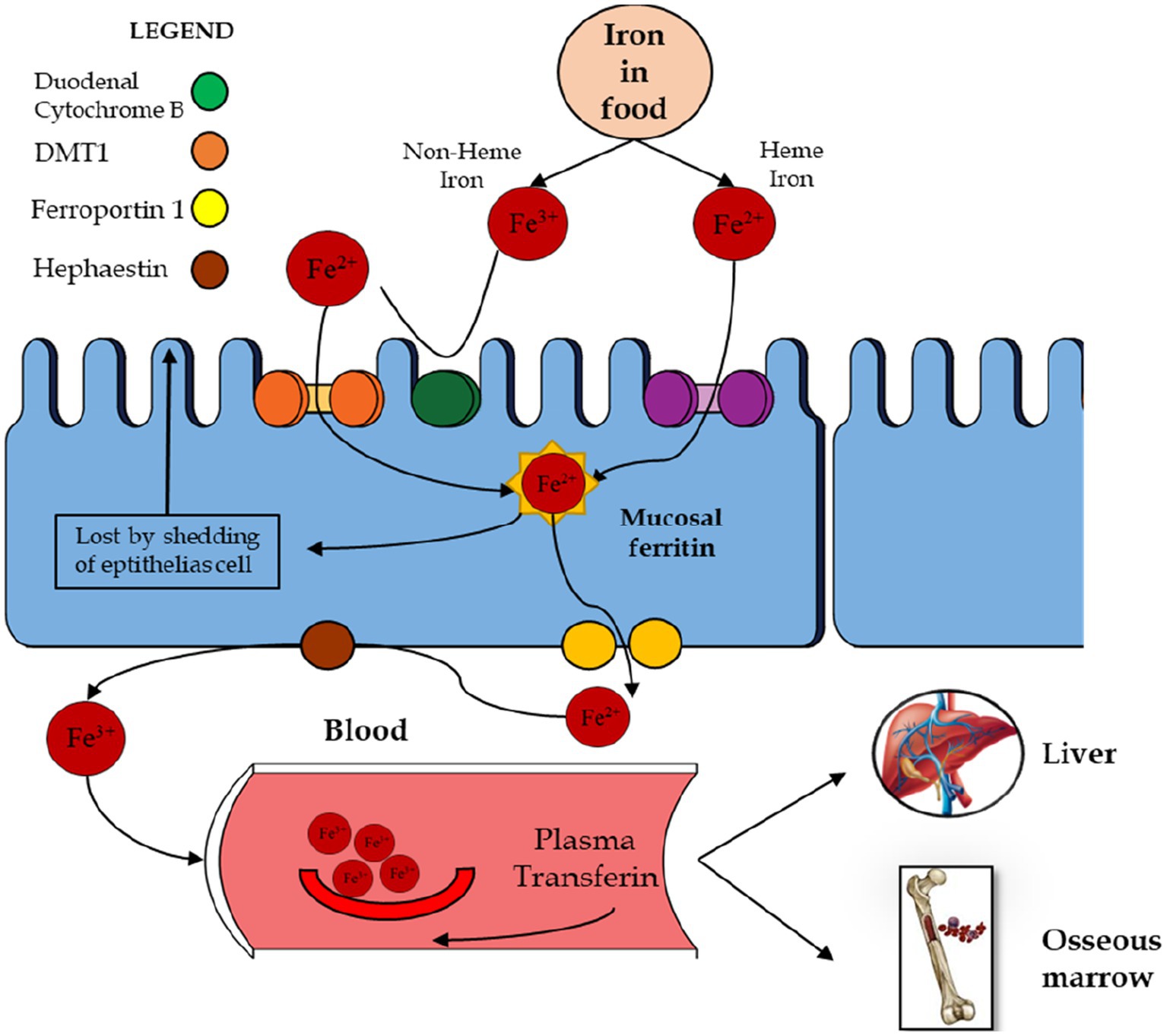
Figure 3. Iron absorption and metabolism. Adapted by Shubham et al. (2020).
The second phase occurs in the intestinal mucosa located in the upper part of the yuoyene, iron enters through the apical membrane through the divalent metal transporter I (DMTI); with in enterocytes Fe2+ is stored as ferritin and transported from the basolateral membrane via the transmembrane protein ferroportin 1 (Dasa and Abera, 2018). The body phase includes the process of transport and storage of the mineral, for the transport of iron at the body level, Fe2+ is converted to Fe3+ by means of the hephaestin protein found in the ferroportin transmembrane; iron circulates through transferrin which occupies 20–40% of the saturation percentage of transferrin; finally transported by bone marrow and storage organs (liver) (Hall and Guyton, 2021).
8. Shelf life and viability of iron microcapsules
The useful life and/or stability of microcapsules is evaluated according to the technique and wall material of the microencapsulation. Hae Soo (2014) describes that temperature is one of the factors that intervenes in the oxidation of lipids, influencing the acceptance of the product, therefore the microparticles of oil and fat (simple emulsions) deteriorate, reducing their useful life, the nutritional value and causing substances toxic. Toledo Barbosa and Garcia Rojas (2022) used the double emulsion technique made with whey protein and polyglycerin, demonstrating that after 7 days of storage at 25°C there is a significant increase in particle size and apparent viscosity. Naktinienė et al. (2021) confirmed that the reaction described above could be due to the interaction of the hydroxyls of polysorbate molecules with iron in the external phase and the formation of networks, causing the increase in viscosity, in addition, the diffusion of water from the external to the internal phase causes growth. Osmotic causing the particle size. Lee et al. (2011) verified that microencapsulated iron salts with whey protein and oil in water are easily modified to become dry powder through the intervention of a vacuum evaporator, prolonging their useful life and effective iron enrichment in milk. Baldelli et al. (2023) analyzed the stability of iron microcapsules obtained by spray drying, storing 20 mg at 21°C and 35% humidity in the environment. The estimated percentage in dry powders is 15%; however, at 90 days the values exceeded the moisture index. García et al. (2022) fortified chocolate milk with heat-treated non-microencapsulated heme iron, with a concentration of 9.3 mg/kg−1 of iron, was accepted by the Institute of Medicine to supplement iron levels in children 8–11 years of age (8 mg/day), the sensory level was acceptable but its useful life lasted only 5 days because the product exceeded the estimated percentage of aerobic mesophiles (50,000 CFU/mL−1) and evidenced organoleptic problems; Huaraca Aparco et al. (2021) confirmed the sensory acceptance and the stability of the physicochemical parameters when adding heme iron to yogurt; In comparison with Subash et al. (2015) who demonstrated its viability in compound yogurt with probiotic bacteria by adding 80 mg of ferrous sulfate microcapsules during 20 days of storage at 4°C, likewise Figueiredo et al. (2022) mentioned that there is no alteration in the intestinal microflora when ingesting yogurt fortified with iron microcapsules. Leiva Arrieta (2020) demonstrated that the absorption of iron by Caco-2 cells presents a cell viability greater than 75% in exposure at the intestinal level. Finally, Sadiq and Doosh (2019) they used sodium alginate and ferrous sulfate subjected to spray drying, their evaluation was carried out in yogurt for 21 days at 5°C, the initial stability values were 87.02 and 86.83%; while at 21 days they decreased to 86.62 and 86.62% respectively, decreasing 0.20%, there was no significant difference between the physicochemical properties, but low values of the degree of acidity and peroxide index.
9. Sensory analysis
Sensory characteristics condition the acceptance or rejection of a food by consumers (Mihafu et al., 2020; Böger et al., 2021). When the food is exposed to factors such as the presence of oxygen, light and changes in pH, chemical reactions of degradation and oxidation are generated between the components of the food; that negatively affect the sensory qualities and absorption rate of nutrients, including minerals (Prichapan et al., 2018); which conditions the direct addition of compounds that improve the nutritional and bioactive properties of food (Halahlah et al., 2022). Mainly, foods enriched with microencapsulated compounds must undergo a sensory evaluation in order to mask foreign aromas and flavors that may be found in the product (Dias et al., 2015).
In the case of the iodized salt fortified with micronutrients such as iron, folic acid, vitamin B 12 and zinc was organoleptically acceptable in terms of appearance, aroma, taste, texture and aftertaste, except for color, where significant differences were found (Puri et al., 2022).
In contrast, the use of a high-quality coating for iron, which, together with iodine in the fortification of a salt, can constitute a physical barrier between the two and could significantly improve their efficacy (retention level and sensory attributes) (Vatandoust et al., 2021).
Kim et al. (2003) they fortified pasteurized milk with microencapsulated ferrous sulfate, after 24 h of storage, the color and flavor presented a slight change, while on the fifth day the perception of the metallic flavor was intense; Gupta et al. (2014) found changes in color, taste, smell and appearance in general on the fifth day, while the sensation in the mouth was perceived on the third day. On the other hand, Gupta et al. (2015) fortified milk by adding microencapsulated ferrous sulfate in a concentration of 10 mg/L, the result was feasible as no significant differences were found between control milk (without fortification) and fortified milk. In the same line, Chang et al. (2016) fortified milk with iron adding 0.1–0.2% demonstrating that under these conditions no reactions of thiobarbituric acid (responsible for fat oxidation) were observed. Besides, García et al. (2022) incorporated 6.76 mg of powdered porcine blood into 1 L of chocolate milk, which until the fourth day of storage, the sensory characteristics remained acceptable; however, when this concentration was exceeded, the unpleasant metallic taste in the product was predominant. In the fortification of Cheddar cheese with microencapsulated ferrous sulfate, Arce and Ustunol (2018) and Siddique and Park (2019) they failed to mask the taste, color and smell of iron; however, in the study of Jalili (2016), the feta cheese did not present unpleasant metallic flavors.
For Onsekizoglu Bagci and Gunasekaran (2016), adding concentrations <60 mg of microencapsulated iron for each liter of yogurt, do not affect the sensory qualities of the product for 14 days; in the same way Jasim and Al-Saadi (2020) evaluated that there are no alterations when adding 2.5 and 5 mg of ferrous sulfate directly. Huaraca Aparco et al. (2021) They found that the addition of 10% pig blood meal in yogurt favors acceptance at the sensory level; It should be noted that the fermentation produced by lactic bacteria increases the levels of iron bioavailability by 20 to 30%, which makes yogurt a favorable vehicle for fortification with iron microcapsules.
10. Limitations found in the reviewed studies
The main limitations found in the different studies was the need to take measurements in the iron suspension formulation, data necessary to identify the estimated values in the particle formation process (Baldelli et al., 2023). Some coating materials used have limited solubility in hot water, when it manages to disperse it swells and forms a highly viscous solution, unpleasant odor, non-Newtonian behavior and rapid biodegradability, such is the case of tamarind gum (Halahlah et al., 2022). Some methods to determine bioavailability have not yet been fully studied, such as the Caco-2 cell model, since other organs (liver) are involved in its regulation in its absorption process and are not considered in the studies (Gaigher et al., 2022). So far, the perception of sensory attributes in foods fortified with microencapsulated iron limit their use, allowing low concentrations, with a perceived metallic taste, in addition to factors such as oxygen, temperature that limit the addition of iron in free form, causing the alterations (Dias et al., 2015).
11. Conclusion
The different methods and techniques of iron microencapsulation and its behavior in food fortification have been studied, being the three determining factors for an optimal microencapsulation: the technique used, a correct formulation between the wall material and the core, elements that whose control have managed to have a favorable success rate greater than 73% taking into account the efficiency, yield and bioavailability of the microencapsulates. It has been shown that the wall material has a significant effect on the iron microcapsules, by providing greater stability, better encapsulation structure and useful life, however, at a higher concentration of solids in the material ratio of wall and core, the bioavailability decreases.
Author contributions
JN-J, HM-M, JV-M, ME-D, ZS-J, LR-F, and LE-E made a concrete, intellectual and scientific contribution to the conception, design and revision of the article, read the final manuscript, analyzed, and approved for publication. All authors contributed to the article and approved the submitted version.
Funding
This research received financial support from the Universidad Nacional de Frontera, Peru (Resolution No. 126-2023-UNF-VPAC/FIIAB).
Acknowledgments
Thanks to the Universidad Nacional de la Frontera for access to its virtual library.
Conflict of interest
The authors declare that the research was conducted in the absence of any commercial or financial relationships that could be construed as a potential conflict of interest.
Publisher’s note
All claims expressed in this article are solely those of the authors and do not necessarily represent those of their affiliated organizations, or those of the publisher, the editors and the reviewers. Any product that may be evaluated in this article, or claim that may be made by its manufacturer, is not guaranteed or endorsed by the publisher.
References
Al Gawhari, F. J. (2016). Preparation of ferrous sulfate microcapsules as a sustained release dosage forms. Int. J. Appl. Pharm. 8, 16–19. doi: 10.22159/ijap.2016v8i3.11251
Arce, A., and Ustunol, Z. (2018). Effect of microencapsulated ferrous sulfate particle size on Cheddar cheese composition and quality. J. Dairy Sci. 101, 6814–6822. doi: 10.3168/jds.2017-13952
Asghari Varzaneh, E., Shahedi, M., and Shekarchizadeh, H. (2017). Iron microencapsulation in gum tragacanth using solvent evaporation method. Int. J. Biol. Macromol. 103, 640–647. doi: 10.1016/j.ijbiomac.2017.05.047
Balakrishnan, M., Gayathiri, S., Preetha, P., Pandiselvam, R., and Jeevarathinam, G. (2021). Microencapsulation of bixin pigment by spray drying: evaluation of characteristics. LWT 145:111343. doi: 10.1016/j.lwt.2021.111343
Baldelli, A., Liang, D. Y., Guo, Y., and Pratap-singh, A. (2023). Effect of the formulation on mucoadhesive spray-dried microparticles containing iron for food fortification. Food Hydrocoll. 134:107906. doi: 10.1016/j.foodhyd.2022.107906
Ballesteros, L. F., Ramirez, M. J., Orrego, C. E., Teixeira, J. A., and Mussatto, S. I. (2017). Encapsulation of antioxidant phenolic compounds extracted from spent coffee grounds by freeze-drying and spray-drying using different coating materials. Food Chem. 237, 623–631. doi: 10.1016/j.foodchem.2017.05.142
Barrios Renteria, J. C., Espinoza-Espinoza, L. A., Valdiviezo-Marcelo, J., and Moreno-Quispe, L. A. (2022). Sensorially accepted Mangifera indica and Myrciaria dubia yogurts with high ascorbic acid content. Front. Sustain. Food Syst. 6, 1–12. doi: 10.3389/fsufs.2022.999400
Batchelor, E. K., Kapitsinou, P., Pergola, P. E., Kovesdy, C. P., and Jalal, D. I. (2020). Iron deficiency in chronic kidney disease: updates on pathophysiology, diagnosis, and treatment. J. Am. Soc. Nephrol. 31, 456–468. doi: 10.1681/ASN.2019020213
Bhosale, S., Desale, R. J., and Fulpagare, Y. G. (2019). Microencapsulation: applications in the different dairy products. Int. J. Pharm. Biomed. Eng. 6, 7–11. doi: 10.14445/23942576/ijpbe-v6i3p102
Blanco-Rojo, R., and Vaquero, M. P. (2019). Iron bioavailability from food fortification to precision nutrition. A review. Innov. Food Sci. Emerg. Technol. 51, 126–138. doi: 10.1016/j.ifset.2018.04.015
Boccio, J., Zubillaga, M. B., Card, A., Lysionek, A. E., Salgueiro, M. J., Calmanovici, P., et al. (1998). Microencapsulated ferrous sulfate to fortify cow milk: absorption and distribution in mice. J. Nutr. Sci. Vitaminol. 44, 381–389. doi: 10.3177/jnsv.44.381
Boccio, J., Zubillaga, M., Caro, R., Gotelli, C., Gotelli, M., and Weill, R. (1995). New procedure to fortify fluid milk and derivatives with iron: a comparative study in mice. J. Nutr. Sci. Vitaminol., 619–626. doi: 10.3177/jnsv.41.619
Boesso, V., Dutra, I., Consoli, L., Molina, G., Maria, G., and Dupas, M. (2016). Solid lipid microparticles produced by spray chilling technique to deliver ginger oleoresin: structure and compound retention. Food Res. Int. 80, 41–49. doi: 10.1016/j.foodres.2015.12.015
Böger, B. R., Acre, L. B., Viegas, M. C., Kurozawa, L. E., and Benassi, M. T. (2021). Roasted coffee oil microencapsulation by spray drying and complex coacervation techniques: characteristics of the particles and sensory effect. Innov. Food Sci. Emerg. Technol. 72:102739. doi: 10.1016/j.ifset.2021.102739
Brodkorb, A., Egger, L., Alminger, M., Alvito, P., Assunção, R., Ballance, S., et al. (2019). INFOGEST static in vitro simulation of gastrointestinal food digestion. Nat. Protoc. 14, 991–1014. doi: 10.1038/s41596-018-0119-1
Calderón Oliver, M., and Ponce Alquicira, E. (2022). The role of microencapsulation in food application. Molecules 27, 1–16. doi: 10.3390/molecules27051499
Çam, M., Içyer, N. C., and Erdoagˇn, F. (2014). Pomegranate peel phenolics: microencapsulation, storage stability and potential ingredient for functional food development. Lwt 55, 117–123. doi: 10.1016/j.lwt.2013.09.011
Cappellini, M. D., Musallam, K. M., and Taher, A. T. (2020). Iron deficiency anaemia revisited. J. Intern. Med. 287, 153–170. doi: 10.1111/joim.13004
Carvalho, G. R., Massarioli, A. P., Alvim, I. D., and Augusto, P. E. D. (2021). Iron-fortified pineapple chips produced using microencapsulation, ethanol, ultrasound and convective drying. Food Eng. Rev. 13, 726–739. doi: 10.1007/s12393-020-09259-4
Castejón, N., Luna, P., and Señoráns, F. J. (2021). Microencapsulation by spray drying of omega-3 lipids extracted from oilseeds and microalgae: effect on polyunsaturated fatty acid composition. Lwt 148:111789. doi: 10.1016/j.lwt.2021.111789
Cengiz, A., Schroën, K., and Berton-Carabin, C. (2019). Lipid oxidation in emulsions fortified with iron-loaded alginate beads. Foods 8:361. doi: 10.3390/foods8090361
Chang, Y. H., Lee, S. Y., and Kwak, H. S. (2016). Physicochemical and sensory properties of milk fortified with iron microcapsules prepared with water-in-oil-in-water emulsion during storage. Int. J. Dairy Technol. 69, 452–459. doi: 10.1111/1471-0307.12282
Cheng, J., Kenaan, A., Zhao, D., Qi, D., and Song, J. (2020). Photo-polymerizable ferrous sulfate liposomes as vehicles for iron fortification of food. Nanomed. Nanotechnol. Biol. Med. 30:102286. doi: 10.1016/j.nano.2020.102286
Chew, S. C., Tan, C. P., and Nyam, K. L. (2018). Microencapsulation of refined kenaf (Hibiscus cannabinus L.) seed oil by spray drying using β-cyclodextrin/gum arabic/sodium caseinate. J. Food Eng. 237, 78–85. doi: 10.1016/j.jfoodeng.2018.05.016
Choi, S. J., Decker, E. A., and McClements, D. J. (2009). Impact of iron encapsulation within the interior aqueous phase of water-in-oil-in-water emulsions on lipid oxidation. Food Chem. 116, 271–276. doi: 10.1016/j.foodchem.2009.02.045
Christides, T., Ganis, J. C., and Sharp, P. A. (2018). In vitro assessment of iron availability from commercial young child formulae supplemented with prebiotics. Eur. J. Nutr. 57, 669–678. doi: 10.1007/s00394-016-1353-3
Churio, O., Pizarro, F., and Valenzuela, C. (2018). Preparation and characterization of iron-alginate beads with some types of iron used in supplementation and fortification strategies. Food Hydrocoll. 74, 1–10. doi: 10.1016/j.foodhyd.2017.07.020
Churio, O., and Valenzuela, C. (2018). Development and characterization of maltodextrin microparticles to encapsulate heme and non-heme iron. Lwt 96, 568–575. doi: 10.1016/j.lwt.2018.05.072
Cian, R. E., Proaño, J. L., Salgado, P. R., Mauri, A. N., and Drago, S. R. (2021). High iron biaccessibility from co-microencapsulated iron/ascorbic acid using chelating polypeptides from brewers’ spent grain protein as wall material. LWT 139:110579. doi: 10.1016/j.lwt.2020.110579
Consoli, L., Grimaldi, R., Sartori, T., and Menegalli, F. C. (2016). Gallic acid microparticles produced by spray chilling technique: production and characterization. LWT Food Sci. Technol. 65, 79–87. doi: 10.1016/j.lwt.2015.07.052
Cusick, S. E., Georgieff, M. K., and Rao, R. (2018). Approaches for reducing the risk of early-life iron deficiency-induced brain dysfunction in children. Nutrients 10, 1–14. doi: 10.3390/nu10020227
Dasa, F., and Abera, T. (2018). Factors affecting iron absorption and mitigation mechanisms: a review. Int. J. Agric. 4, 24–30. doi: 10.17352/2455-815X.000033
Desai, K. G. H., and Park, H. J. (2005). Recent developments in microencapsulation of food ingredients. Dry. Technol. 23, 1361–1394. doi: 10.1081/DRT-200063478
Dias, M. I., Ferreira, I. C. F. R., and Barreiro, M. F. (2015). Microencapsulation of bioactives for food applications. Food Funct. 6, 1035–1052. doi: 10.1039/c4fo01175a
Đorđević, V., Balanč, B., Belščak-Cvitanović, A., Lević, S., Trifković, K., Kalušević, A., et al. (2014). Trends in encapsulation technologies for delivery of food bioactive compounds. Food Eng. Rev. 7, 452–490. doi: 10.1007/s12393-014-9106-7
Drago, S. R., Binaghi, M. J., and Valencia, M. E. (2005). Effrect of gastric digestion pH on Iron, zinc, and calcium Dialyzability from preterm and term starting infant formulas. J. Food Sci. 70, S107–S112. doi: 10.1111/j.1365-2621.2005.tb07113.x
Dueik, V., and Diosady, L. L. (2017). Microencapsulation of iron in a reversed enteric coating using spray drying technology for double fortification of salt with iodine and iron. J. Food Process Eng. 40, 1–9. doi: 10.1111/jfpe.12376
El Bilbeisi, A. H., Al-Jawaldeh, A., Albelbeisi, A., Abuzerr, S., Elmadfa, I., and Nasreddine, L. (2022). Association of household food insecurity with dietary intakes and nutrition-related knowledge, attitudes, and practices among parents, aged ≥ 18 years in Gaza strip, Palestine: a descriptive study. Heliyon 8:e09582. doi: 10.1016/j.heliyon.2022.e09582
Espinola Sanchez, M., Sanca Valeriano, S., and Ormeño Julca, A. (2021). Factores sociales y demográficos asociados a la anemia en mujeres embarazada en Perú. Rev. Chil. Obstet. Ginecol. 86, 192–201. doi: 10.4067/S0717-75262021000200192
Ezhilarasi, P. N., Indrani, D., Jena, B. S., and Anandharamakrishnan, C. (2013). Freeze drying technique for microencapsulation of Garcinia fruit extract and its effect on bread quality. J. Food Eng. 117, 513–520. doi: 10.1016/j.jfoodeng.2013.01.009
Figueiredo, J. D. A., Silva, C. R. D. P., Souza Oliveira, M. F., Norcino, L. B., Campelo, P. H., Botrel, D. A., et al. (2022). Microencapsulation by spray chilling in the food industry: opportunities, challenges, and innovations. Trends Food Sci. Technol. 120, 274–287. doi: 10.1016/j.tifs.2021.12.026
Filiponi, M. P., Gaigher, B., Caetano-Silva, M. E., Dutra Alvim, I., and Bertoldo Pacheco, M. T. (2019). Microencapsulation performance of Fe-peptide complexes and stability monitoring. Food Res. Int. 125:108505. doi: 10.1016/j.foodres.2019.108505
Finkelstein, J. L., Herman, H. S., Guetterman, H. M., Peña-Rosas, J. P., and Mehta, S. (2018). Daily iron supplementation for prevention or treatment of iron deficiency anaemia in infants, children, and adolescents. Cochrane Database Syst. Rev. 12:CD013227. doi: 10.1002/14651858.CD013227
Gaigher, B., Do Nascimento Da Silva, E., Lacerda Sanches, V., Fernanda Milani, R., Galland, F., Cadore, S., et al. (2022). Formulations with microencapsulated Fe–peptides improve in vitro bioaccessibility and bioavailability. Curr. Res. Food Sci. 5, 687–697. doi: 10.1016/j.crfs.2022.03.007
Gao, G., Li, J., Zhang, Y., and Chang, Y. (2019). “Cellular iron metabolism and regulation” in Brain iron metabolism and CNS diseases, ed. Yan-Zhong Chang (Singapore: Springer), 21–32.
García, D., Changanaqui, K., Vásquez, R. E., Neira, E., Espinoza, J. B., Moran, J. R. V., et al. (2022). Heme iron fortified flavored milk: quality and sensory analysis. Brazilian J. Food Technol. 25, 1–10. doi: 10.1590/1981-6723.17621
Girelli, D., Ugolini, S., Busti, F., Marchi, G., and Castagna, A. (2018). Modern iron replacement therapy: clinical and pathophysiological insights. Int. J. Hematol. 107, 16–30. doi: 10.1007/s12185-017-2373-3
González Ortega, R., Faieta, M., Di Mattia, C. D., Valbonetti, L., and Pittia, P. (2020). Microencapsulation of olive leaf extract by freeze-drying: effect of carrier composition on process efficiency and technological properties of the powders. J. Food Eng. 285:110089. doi: 10.1016/j.jfoodeng.2020.110089
Gupta, C., Chawla, P., and Arora, S. (2015). Development and evaluation of iron microencapsules for milk fortification. CyTA J. Food 13, 116–123. doi: 10.1080/19476337.2014.918179
Gupta, C., Chawla, P., Arora, S., Tomar, S. K., and Singh, A. K. (2014). Iron microencapsulation with blend of gum arabic, maltodextrin and modified starch using modified solvent evaporation method - milk fortification. Food Hydrocoll. 43, 622–628. doi: 10.1016/j.foodhyd.2014.07.021
Hae Soo, K. (2014). Nano-and microencapsulation for foods John Wiley & Sons Ltd Available at: https://sci-hub.st/10.1002/9781118292327.
Halahlah, A., Piironen, V., Mikkonen, K. S., and Ho, T. M. (2022). Polysaccharides as wall materials in spray-dried microencapsulation of bioactive compounds: physicochemical properties and characterization. Crit. Rev. Food Sci. Nutr, 1–33. doi: 10.1080/10408398.2022.2038080
Hall, J. E., and Guyton, A. C. (2021). Tratado de fisiología médica 14 Ed., eds. J. Hall E and M. Hall E.
Hashem Hosseini, S. M., Hashemi Gahruie, H., Razmjooie, M., Sepeidnameh, M., Rastehmanfard, M., Tatar, M., et al. (2019). Effects of novel and conventional thermal treatments on the physicochemical properties of iron-loaded double emulsions. Food Chem. 270, 70–77. doi: 10.1016/j.foodchem.2018.07.044
Heckert Bastos, L. P., De Sá Costa, B., Pinto Siqueira, R., and Garcia Rojas, E. E. (2020). Complex coacervates of β-lactoglobulin/sodium alginate for the microencapsulation of black pepper (Piper nigrum L.) essential oil: Simulated gastrointestinal conditions and modeling release kinetics, 1–10.
Hemalatha, S., Platel, K., and Srinivasan, K. (2007). Influence of heat processing on the bioaccessibility of zinc and iron from cereals and pulses consumed in India. J. Trace Elem. Med. Biol. 21, 1–7. doi: 10.1016/j.jtemb.2006.10.002
Huaraca Aparco, R., Taípe Pardo, F., and Delgado Laime, M. C. (2021). Fortification of yogurt with hemic iron and its acceptance in children of the rural sector of the Apurímac region. Manglar 18, 117–122. doi: 10.17268/manglar.2021.015
Ilich, J. Z. (2020). Nutritional and behavioral approaches to body composition and low-grade chronic inflammation management for older adults in the ordinary and COVID-19 times. Nutrients 12:3898. doi: 10.3390/nu12123898
Ilyasoglu Buyukkestelli, H., and El, S. N. (2019). Preparation and characterization of double emulsions for saltiness enhancement by inhomogeneous spatial distribution of sodium chloride. LWT-Food Sci. Technol. 101, 229–235. doi: 10.1016/j.lwt.2018.10.086
Ilyasoglu Buyukkestelli, H., and Nehir El, S. (2019). Development and characterization of double emulsion to encapsulate iron. J. Food Eng. 263, 446–453. doi: 10.1016/j.jfoodeng.2019.07.026
Jahan, T. A., Vandenberg, A., Glahn, R. P., Tyler, R. T., Reaney, M. J. T., and Tar’an, B. (2019). Iron fortification and bioavailability of chickpea (Cicer arietinum L.) seeds and flour. Nutrients 11, 1–17. doi: 10.3390/nu11092240
Jalili, M. (2016). Chemical composition and sensory characteristics of feta cheese fortified with iron and ascorbic acid. Dairy Sci. Technol. 96, 579–589. doi: 10.1007/s13594-016-0280-7
Jasim, A. A., and Al-Saadi, J. M. S. (2020). Study the effect of adding iron salts on the physiochemical and sensory properties of Yoghurt. Indian J. Forensic Med. Toxicol. 14, 2771–2778. doi: 10.37506/ijfmt.v14i4.12010
Jayalalitha, V., Elango, A., and Pugazhenthi, T. R. (2022). Iron fortification in Yoghurt: methods and processes, as well as their influence on Physico-chemical and sensory qualities. Int. J. Agric. Environ. Biotechnol. 15, 135–140. doi: 10.30954/0974-1712.01.2022.17
Kang, Y. R., Lee, Y. K., Kim, Y. J., and Chang, Y. H. (2019). Characterization and storage stability of chlorophylls microencapsulated in different combination of gum Arabic and maltodextrin. Food Chem. 272, 337–346. doi: 10.1016/j.foodchem.2018.08.063
Kanwal, S., Hafeez-Ur-rehman, M., Hussain, A., Nadeem, M., Abbas, F., Akram, M., et al. (2022). Development of chitosan based microencapsulated spray dried powder of tuna fish oil: oil load impact and oxidative stability. Brazilian J. Biol. 84, 1–8. doi: 10.1590/1519-6984.254010
Karaaslan, M., Şengün, F., Cansu, Ü., Başyiğit, B., Sağlam, H., and Karaaslan, A. (2021). Gum arabic/maltodextrin microencapsulation confers peroxidation stability and antimicrobial ability to pepper seed oil. Food Chem. 337:127748. doi: 10.1016/j.foodchem.2020.127748
Karrar, E., Mahdi, A. A., Sheth, S., Mohamed Ahmed, I. A., Manzoor, M. F., Wei, W., et al. (2021). Effect of maltodextrin combination with gum arabic and whey protein isolate on the microencapsulation of gurum seed oil using a spray-drying method. Int. J. Biol. Macromol. 171, 208–216. doi: 10.1016/j.ijbiomac.2020.12.045
Karthik, P., and Anandharamakrishnan, C. (2012). Microencapsulation of docosahexaenoic acid by spray-freeze-drying method and comparison of its stability with spray-drying and freeze-drying methods. Food Bioprocess Technol. 6, 2780–2790. doi: 10.1007/s11947-012-1024-1
Katuwavila, N. P., Perera, A. D. L. C., Dahanayake, D., Karunaratne, V., Amaratunga, G. A. J., and Karunaratne, D. N. (2016). Alginate nanoparticles protect ferrous from oxidation: potential iron delivery system. Int. J. Pharm. 513, 404–409. doi: 10.1016/j.ijpharm.2016.09.053
Kim, S. J., Ahn, J., Seok, J. S., and Kwak, H. S. (2003). Microencapsulated iron for drink yogurt fortification. Asian-Australasian J. Anim. Sci. 16, 581–587. doi: 10.5713/ajas.2003.581
Kour, J., Ui Haq, R., Ahmad Wani, S., and Hyoti, B. (2023). Handbook of nanoencapsulation, preparation, characterization, delivery, and Safety of nutraceutical nanocomposites 1st Ed. eds. J. Kour, R. UI Haq, S. Ahmad Wani, and B. Jyoti Taylor & Francis.
Krokida, M. (2017). Thermal and nonthermal encapsulation methods. Available at: https://www.taylorfrancis.com/books/edit/10.1201/9781315267883/thermal-nonthermal-encapsulation-methods-magdalini-krokida.
Kumar, D., Chandra Rai, D., and Kumar, S. (2017). Encapsulation process optimization of Iron, L-ascorbic acid and L. acidophilus with sodium alginate using CCRD-RSM. Int. J. Curr. Microbiol. Appl. Sci. 6, 1803–1813. doi: 10.20546/ijcmas.2017.603.206
Lee, S. J., Choi, S. J., Li, Y., Decker, E. A., and McClementes, D. J. (2011). Protein-stabilized Nanoemulsions and emulsions: comparison of physicochemical stability, lipid oxidation, and lipase digestibility. Agric. Food Chem. 59, 415–427. doi: 10.1021/jf103511v
Lee, J. B., Ahn, J., and Kwak, H. S. (2003). Microencapsulated ascorbic acid for milk fortification. Arch. Pharm. Res. 26, 575–580. doi: 10.1007/BF02976884
Leiva Arrieta, A. (2020). Double (iron and zinc) fortified black tea: assesing the biaccessibility and bioavailability using spray dryind microencapsulation technology. doi: 10.14288/1.0394846
Ligarda Samanez, C. A., Moscoso Moscoso, E., Choque Quispe, D., Palomino Rincncon, H., Martrtines Huaman, E. L., Huaman Carrion, M., et al. (2022). Microencapsulation of erythrocytes extracted from Cavia porcellus blood in matrices of tara gum and native potato starch. Food 11:2107. doi: 10.3390/foods11142107
Lo, J. O., Benson, A. E., Hedges, M. A., Mcmurry, H. S., Shatzel, J. J., Deloughery, T., et al. (2022). The role of oral iron in the treatment of adults with iron deficiency. Eur. J. Haematol. 110, 123–130. doi: 10.1111/ejh.13892
López Cruz, R., Ragazzo Sánchez, J. A., and Calderón Santoyo, M. (2020). Microencapsulation of Meyerozyma guilliermondii by spray drying using sodium alginate and soy protein isolate as wall materials: a biocontrol formulation for anthracnose disease of mango. Biocontrol Sci. Tech. 30, 1116–1132. doi: 10.1080/09583157.2020.1793910
Lysionek, A. E., Zubillaga, M. B., Caro, R. A., Weil, R., and Boccio, R. (2001). Bioavailability study of dried microencapsulated ferrous sulfate-SFE 171- by means of the prophylactic-preventive method. J. Trace Elem. Med. Biol. 15, 255–259. doi: 10.1016/S0946-672X(01)80041-9
Mansour, M., Salah, M., and Xu, X. (2020). Effect of microencapsulation using soy protein isolate and gum arabic as wall material on red raspberry anthocyanin stability, characterization, and simulated gastrointestinal conditions. Ultrason. Sonochem. 63:104927. doi: 10.1016/j.ultsonch.2019.104927
McKinney, J. M., Pucha, K. A., Doan, T. N., Wang, L., Weinstock, L. D., Tignor, B. T., et al. (2022). Sodium alginate microencapsulation of human mesenchymal stromal cells modulates paracrine signaling response and enhances efficacy for treatment of established osteoarthritis. Acta Biomater. 141, 315–332. doi: 10.1016/j.actbio.2021.12.034
Ménard, O., Bourlieu, C., De Oliveira, S. C., Dellarosa, N., Laghi, L., Carrière, F., et al. (2018). A first step towards a consensus static in vitro model for simulating full-term infant digestion. Food Chem. 240, 338–345. doi: 10.1016/j.foodchem.2017.07.145
Mihafu, F. D., Issa, J. Y., and Kamiyango, M. W. (2020). Implication of sensory evaluation and quality assessment in food product development: a review. Curr. Res. Nutr. Food Sci. 8, 690–702. doi: 10.12944/CRNFSJ.8.3.03
Miller, D. D., Schricker, B. R., Rasmussen, R. R., and Van Campen, D. (1981). An in vitro method for estimation of iron availability from meals. Am. J. Clin. Nutr. 34, 2248–2256. doi: 10.1093/ajcn/34.10.2248
Motalebi Moghanjougi, Z., Rezazadeh Bari, M., Alizadeh Khaledabad, M., Amiri, S., and Almasi, H. (2021). Microencapsulation of Lactobacillus acidophilus LA-5 and Bifidobacterium animalis BB-12 in pectin and sodium alginate: a comparative study on viability, stability, and structure. Food Sci. Nutr. 9, 5103–5111. doi: 10.1002/fsn3.2470
Mukhija, K., Singhal, K., Angmo, S., Yadav, K., Yadav, H., Sandhir, R., et al. (2016). Potential of alginate encapsulated ferric Saccharate microemulsions to ameliorate Iron deficiency in mice. Biol. Trace Elem. Res. 172, 179–192. doi: 10.1007/s12011-015-0564-4
Naito, Y., Masuyama, T., and Ishihara, M. (2021). Iron and cardiovascular diseases. J. Cardiol. 77, 160–165. doi: 10.1016/j.jjcc.2020.07.009
Naktinienė, M., Eisinaitė, V., Keršienė, M., Jasutienė, I., and Leskauskaitė, D. (2021). Emulsification and gelation as a tool for iron encapsulation in food-grade systems. Lwt 149:111895. doi: 10.1016/j.lwt.2021.111895
Nielsen, O. H., Soendergaard, C., Vikner, M. E., and Weiss, G. (2018). Rational management of iron-deficiency anaemia in inflammatory bowel disease. Nutrients 10, 1–25. doi: 10.3390/nu10010082
Onsekizoglu Bagci, P., and Gunasekaran, S. (2016). Iron-encapsulated cold-set whey protein isolate gel powder - part 2: effect of iron fortification on sensory and storage qualities of Yoghurt. Int. J. Dairy Technol. 69, 601–608. doi: 10.1111/1471-0307.12316
Pachón, H., Reynolds, B., Duong, M., Tsang, B. L., Childs, L., Luthringer, C. L., et al. (2021). The potential contribution of fortified maize flour, oil, rice, salt, and wheat flour to estimated average requirements and tolerable upper intake levels for 15 nutrients in 153 countries. Nutrients 13, 1–14. doi: 10.3390/nu13020579
Pereira, R., Rodrigues, R., Altinok, E., Ramos, O., Malcata, X., Maresca, P., et al. (2017). Development of iron-rich whey protein hydrogels following application of ohmic heating – effects of moderate electric fields. Food Res. Int. 99, 435–443. doi: 10.1016/j.foodres.2017.05.023
Pérez-Leonard, H., Bueno-García, G., and Brizuela-Herrada, M. A. (2013). Microencapsulation: a protection pathway for probiotic microorganisms. ICIDCA. Sobre los Deriv. la Caña Azúcar, 47, 14–25.
Perez-Moral, N., Chueca Gonzalez, M., and Parker, R. (2013). Food hydrocolloids preparation of iron-loaded alginate gel beads and their release characteristics under simulated gastrointestinal conditions. Food Hydrocoll. 31, 114–120. doi: 10.1016/j.foodhyd.2012.09.015
Pieczykolan, E., and Kurek, M. A. (2019). Use of guar gum, gum arabic, pectin, beta-glucan and inulin for microencapsulation of anthocyanins from chokeberry. Int. J. Biol. Macromol. 129, 665–671. doi: 10.1016/j.ijbiomac.2019.02.073
Piskin, E., Cianciosi, D., Gulec, S., Tomas, M., and Capanoglu, E. (2022). Iron absorption: factors, limitations, and improvement methods. ACS Omega 7, 20441–20456. doi: 10.1021/acsomega.2c01833
Pivina, L., Semenova, Y., Doşa, M. D., Dauletyarova, M., and Bjørklund, G. (2019). Iron deficiency, cognitive functions, and neurobehavioral disorders in children. J. Mol. Neurosci. 68, 1–10. doi: 10.1007/s12031-019-01276-1
Podder, R., Dellavalle, D. M., Tyler, R. T., Glahn, R. P., Tako, E., and Vandenberg, A. (2018). Relative bioavailability of iron in Bangladeshi traditional meals prepared with iron-fortified lentil dal. Nutrients 10:354. doi: 10.3390/nu10030354
Polekkad, A., Franklin, M. E. E., Pushpadass, H. A., Battula, S. N., Rao, S. B. N., and Pal, D. T. (2021). Microencapsulation of zinc by spray-drying: Characterisation and fortification. Elsevier B.V.
Pratap Singh, A., Siddiqui, J., and Diosady, L. L. (2017). Characterizing the pH-dependent release kinetics of food-grade spray drying encapsulated Iron microcapsules for food fortification. Food Bioprocess Technol. 11, 435–446. doi: 10.1007/s11947-017-2022-0
Pratap-Singh, A., and Leiva, A. (2021). Double fortified (iron and zinc) spray-dried microencapsulated premix for food fortification. Lwt 151:112189. doi: 10.1016/j.lwt.2021.112189
Premi, M., and Sharma, H. K. (2017). Effect of different combinations of maltodextrin, gum arabic and whey protein concentrate on the encapsulation behavior and oxidative stability of spray dried drumstick (Moringa oleifera) oil. Int. J. Biol. Macromol. 105, 1232–1240. doi: 10.1016/j.ijbiomac.2017.07.160
Prichapan, N., McClements, D. J., and Klinkesorn, U. (2018). Iron encapsulation in water-in-oil emulsions: effect of ferrous sulfate concentration and fat crystal formation on oxidative stability. J. Food Sci. 83, 309–317. doi: 10.1111/1750-3841.14034
Puri, S., Rekhi, T. K., Thomas, T., Jadhav, M. H., Mannar, V., and Diosady, L. L. (2022). Sensory Trial of Quintuple Fortified Salt-Salt Fortified With Iodine, Iron, Folic Acid, Vitamin B12, and Zinc-Among Consumers in New Delhi, India. Food Nutr. Bull, 43, 340–350. doi: 10.1177/03795721221078361
Ravichai, K., and Muangrat, R. (2019). Effect of different coating materials on freeze-drying encapsulation of bioactive compounds from fermented tea leaf wastewater. J. Food Process. Preserv. 43, 1–15. doi: 10.1111/jfpp.14145
Ribeiro Dias, D., Alvarenga Botrel, D., De Barros Fernandes, R. V., and Vilela Borges, S. (2017). Encapsulation as a tool for bioprocessing of functional foods. Curr. Opin. Food Sci. 13, 31–37. doi: 10.1016/j.cofs.2017.02.001
Ribeiro, A. M., Shahgol, M., Estevinho, B. N., and Rocha, F. (2020). Microencapsulation of vitamin a by spray-drying, using binary and ternary blends of gum arabic, starch and maltodextrin. Food Hydrocoll. 108:106029. doi: 10.1016/j.foodhyd.2020.106029
Rocha-Selmi, G. A., Favaro-Trindade, C. S., and Grosso, C. R. F. (2013). Morphology, stability, and application of lycopene microcapsules produced by complex coacervation. J. Chem. 2013:982603. doi: 10.1155/2013/982603
Rojas, J. (2019). Microencapsulación de extractos de chile habanero (capsicum chinense) empleando secado por aspersión y CO2 supercritico. Available at: https://rinacional.tecnm.mx/bitstream/TecNM/1603/1/2019OlguinRojasJoseArturo.pdf
Sadiq, I. H., and Doosh, K. S. (2019). Study of the physochemical, rheological and sensory properties of Yoghurt fortified with microencapsulation Iron. Iraqi J. Agric. Sci. 50, 1345–1355. doi: 10.36103/ijas.v50i5.799
Salazar, G. J. T., Dias, F. J., Ribeiro, P. R. V., de Brito, E. S., Canuto, K. M., Coutinho, H. D. M., et al. (2021). Antioxidant activity of Stryphnodendron rotundifolium Mart. stem bark fraction in an iron overload model. Foods 10:2683. doi: 10.3390/foods10112683
Shahidi Noghabi, M., and Molaveisi, M. (2020). Microencapsulation optimization of cinnamon essential oil in the matrices of gum Arabic, maltodextrin, and inulin by spray-drying using mixture design. J. Food Process Eng. 43, 1–13. doi: 10.1111/jfpe.13341
Shubham, K., Anukiruthika, T., Dutta, S., Kashyap, A. V., Moses, J. A., and Anandharamakrishnan, C. (2020). Iron deficiency anemia: a comprehensive review on iron absorption, bioavailability and emerging food fortification approaches. Trends Food Sci. Technol. 99, 58–75. doi: 10.1016/j.tifs.2020.02.021
Siddique, A., and Park, Y. W. (2019). Effect of iron fortification on microstructural, textural, and sensory characteristics of caprine milk Cheddar cheeses under different storage treatments. J. Dairy Sci. 102, 2890–2902. doi: 10.3168/jds.2018-15427
Singh, J., Kaur, K., and Kumar, P. (2018). Optimizing microencapsulation of α-tocopherol with pectin and sodium alginate. J. Food Sci. Technol. 55, 3625–3631. doi: 10.1007/s13197-018-3288-6
Skolmowska, D., and Głąbska, D. (2019). Analysis of heme and non-heme iron intake and iron dietary sources in adolescent menstruating females in a national polish sample. Nutrients 11:1049. doi: 10.3390/nu11051049
Slivka, A., Kang, J., and Cohen, G. (1985). Hydroxyl radicals and the toxicity of oral iron. Gastroenterology 72, 193–556. doi: 10.1016/S0016-5085(77)80340-5
Sorita, G. D., Santamaria-Echart, A., Gozzo, A. M., Gonçalves, O. H., Leimann, F. V., Bona, E., et al. (2021). Lipid composition optimization in spray congealing technique and testing with curcumin-loaded microparticles. Adv. Powder Technol. 32, 1710–1722. doi: 10.1016/j.apt.2021.03.028
Subash, R., Elango, A., Pandiyan, C., Karthikeyan, N., Kumaresan, G., and Raghunath, B. (2015). The viability of Yoghurt probiotic culture in microencapsulated iron fortified Yoghurt. J. Cell Tissue Res. 15, 5053–5057.
Swami, S. B., Sawant, A. A., and Khandetod, Y. P. (2021). Microencapsulation techniques used for underutilized fruits of western Ghat: an overview. Pharma Innov. J. 10, 826–833.
Tatar Turan, F., and Kahyaoglu, T. (2021). The effect of an ultrasonic spray nozzle on carbohydrate and protein-based coating materials for blueberry extract microencapsulation. J. Sci. Food Agric. 101, 120–130. doi: 10.1002/jsfa.10622
Toledo Barbosa, B. S., and Garcia Rojas, E. E. (2022). Double emulsions as delivery systems for iron: stability kinetics and improved bioaccessibility in infants and adults. Curr. Res. Food Sci. 5, 718–725. doi: 10.1016/j.crfs.2022.04.003
Valenzuela, C., Hernández, V., Morales, M. S., Neira-Carrillo, A., and Pizarro, F. (2014). Preparation and characterization of heme iron-alginate beads. Lwt 59, 1283–1289. doi: 10.1016/j.lwt.2014.04.030
Vatandoust, A., Venkatesh Mannar, M. G., and Diosady, L. L. (2021). Organoleptic effects of salt fortification with Iron and iodine: a review. J. Nutr. 151, 1690–1702. doi: 10.1093/jn/nxab078
Wang, B., Cheng, F., Gao, S., Ge, W., and Zhang, M. (2017). Double enzymatic hydrolysis preparation of heme from goose blood and microencapsulation to promote its stability and absorption. Food Chem. 217, 699–704. doi: 10.1016/j.foodchem.2016.09.007
Wardhani, D. H., Ulya, H. N., Rahmawati, A., Sugiarto, T. V. K., Kumoro, A. C., and Aryanti, N. (2021). Preparation of degraded alginate as a pH-dependent release matrix for spray-dried iron and its encapsulation performances. Food Biosci. 41:101002. doi: 10.1016/j.fbio.2021.101002
Yao, X., Yao, X., Xu, K., Wu, K., Jiang, F., Nishinari, K., et al. (2020). Iron encapsulated microstructured gel beads using an emulsification-gelation technique for an alginate-caseinate matrix. Food Funct. 11, 3811–3822. doi: 10.1039/c9fo02184a
Yiannikourides, A., and Latunde-Dada, G. (2019). A short review of Iron metabolism and pathophysiology of Iron disorders. Medicines 6:85. doi: 10.3390/medicines6030085
Ying, D. Y., Sanguansri, L., Weerakkody, R., Bull, M., Singh, T. K., and Augustin, M. A. (2016). Effect of encapsulant matrix on stability of microencapsulated probiotics. J. Funct. Foods 25, 447–458. doi: 10.1016/j.jff.2016.06.020
Zárate-Hernández, E., Hernández-Esquivel, R. A., and Pérez-Urizar, J. T. (2021). Microcápsulas y microesferas: una visión a la caracterización integral y aplicación para la liberación de medicamentos biotecnológicos. CienciaUAT 15, 21–36. doi: 10.29059/cienciauat.v15i2.1472
Zhang, L., Mou, D., and Du, Y. (2007). Procyanidins: extraction and micro-encapsulation. Rev. Fitoter. 13, 125–135. doi: 10.1002/jsfa
Zhu, F. (2017). Encapsulation and delivery of food ingredients using starch based systems. Food Chem. 229, 542–552. doi: 10.1016/j.foodchem.2017.02.101
Keywords: functional foods, ferrous compounds, anemia, fortification, iron homeostasis
Citation: Muñoz-More HD, Nole-Jaramillo JM, Valdiviezo-Marcelo J, Espinoza-Delgado MdP, Socola-Juarez ZM, Ruiz-Flores LA and Espinoza-Espinoza LA (2023) Microencapsulated iron in food, techniques, coating material, efficiency, and sensory analysis: a review. Front. Sustain. Food Syst. 7:1146873. doi: 10.3389/fsufs.2023.1146873
Edited by:
Alexandru Rusu, Biozoon Food Innovations GmbH, GermanyReviewed by:
Lochan Singh, National Institute of Food Technology Entrepreneurship and Management, IndiaLevente Diosady, University of Toronto, Canada
Copyright © 2023 Muñoz-More, Nole-Jaramillo, Valdiviezo-Marcelo, Espinoza-Delgado, Socola-Juarez, Ruiz-Flores and Espinoza-Espinoza. This is an open-access article distributed under the terms of the Creative Commons Attribution License (CC BY). The use, distribution or reproduction in other forums is permitted, provided the original author(s) and the copyright owner(s) are credited and that the original publication in this journal is cited, in accordance with accepted academic practice. No use, distribution or reproduction is permitted which does not comply with these terms.
*Correspondence: Luis Alfredo Espinoza-Espinoza, bGVzcGlub3phQHVuZi5lZHUucGU=
†These authors have contributed equally to this work and share first authorship