- 1Department of Biological Sciences Ålesund, Norwegian University of Science and Technology, Ålesund, Norway
- 2Department of Biotechnology and Food Science, Norwegian University of Science and Technology, Trondheim, Norway
- 3Food Chemistry and Technology Department, Teagasc Food Research Centre, Ashtown, Dublin, Ireland
Introduction: Fish rest raw material generated from the fish processing industry may be a useful resource for recovery of added value compounds. The application of non-thermal novel technologies can improve the extraction. High-pressure processing (HPP) has long been used for the preservation and extension of the shelf life of seafood. It also constitutes a promising technology for the increased recovery of valuable compounds, such as lipids and proteins. The objective of this study was to assess the yield and the chemical composition of the fractions obtained after enzymatic hydrolysis on a mixture of Atlantic salmon (Salmo salar) and rainbow trout (Oncorhynchus mykiss) rest raw material pretreated by high-pressure (HP).
Methods: Six different pretreatments were applied prior to enzymatic hydrolysis; 600 MPa x 8 min, 600 MPa x 4 min, 400 MPa x 8 min, 400 MPa x 4 min, 200 MPa x 8 min, 200 MPa x 4 min.
Results and discusssion: These applied pretreatments did not yield higher oil extraction compared to the control. However, the fish protein hydrolysates (FPH) contained higher amount of protein when compared to the FPH obtained from the control.
1 Introduction
Fishery and aquaculture global production came up to a record of 214 million tonnes in 2020, where 89% of it was directed to human consumption (FAO, 2022). Increased supplies, income growth, and improvement in applied technologies are among the factors contributing to the increased per capita consumption of aquatic food (20.2 kg) in 2020 compared to the previous years. Salmon and rainbow trout are species with high demand, especially in North America and Europe (FAO, 2022). However, as fisheries and aquaculture production expand, a proportional amount of fish rest raw material is generated. This consists of heads, bones, trimmings, viscera, skin, frames, belly flaps, and intestines, which may reach up to 70% of the processed fish (Rustad et al., 2011).
Several studies have shown that the enzymatic hydrolysis of fish rest raw material, utilizing exogenous enzymes, provides a method to increase the revenue through the recovery of valuable compounds, such as lipids and proteins. In addition to nutritional properties these can display a pharmaceutical potential (Liaset et al., 2000; Šližyte et al., 2005; Kim and Mendis, 2006; Araujo et al., 2021; Bartolomei et al., 2023).
Novel non-thermal technologies, such as UV-light (UV), ultrasound (US), irradiation (IR), cold plasma (CPL), high-pressure processing (HPP), and pulsed electric field (PEF), have been studied extensively for their ability to preserve and extend the shelf-life of seafood avoiding nutritional loss while keeping their sensory quality (Olatunde and Benjakul, 2018; Ekonomou and Boziaris, 2021). Furthermore, the recent application of these technologies in fish rest raw materials appeared promising and gained high commercial interest as they may facilitate an increased recovery of value-added products compared to traditional extraction methods (Al Khawli et al., 2019; Ali et al., 2021; De Aguiar Saldanha Pinheiro et al., 2021). More specifically, HPP may disrupt the non-covalent interactions in biological tissues, leading to cell membrane destruction, increased cell permeability, and moderate denaturation and unfolding of proteins (Tao et al., 2014). Thus, when HPP is implemented as pretreatment before enzymatic hydrolysis, an improved enzyme-substrate interaction can be observed, causing a significant generation of bioactive compounds (Thoresen et al., 2020; Zhang et al., 2021). However, due to limited knowledge of the influence of the application of high-pressure treatment on the extraction of valuable compounds from seafood rest raw material, more research in this field is needed (Hassoun et al., 2023; Siddiqui et al., 2023).
The current study aims to assess the impact of high-pressure processing at varying pressure levels and hold times on a mixture of salmon and rainbow trout side streams. This pretreatment occurs before enzymatic hydrolysis and focuses on extraction yield and the characteristics of the resulting fractions.
2 Materials and methods
2.1 Preparation of samples
Fresh Atlantic salmon (Salmo salar) and rainbow trout (Oncorhynchus mykiss) (4–6 h after slaughter) were purchased from Hofseth Aqua AS in Ålesund, Norway and transported on ice to the laboratory. Fish was immediately eviscerated by hand, and the rest raw material (heads, tails, skin, bones, and trimmings) was minced using a mincer (Hobart A200N) with a hole size of 9 mm. The minced raw material (1:1 w/w Atlantic salmon and rainbow trout) was placed in plastic bags of approximately 1.1 kg per unit before being transferred to −80°C. Further, the samples were sent frozen to Teagasc research center (Dublin, Ireland) for high-pressure (HP) treatment.
2.2 HP treatment
Frozen fish samples were thawed overnight (4°C) prior to HP treatment. The fish samples underwent a HP treatment using a commercial-scale Hyperbaric 420 High-Pressure Processor, with a pressure vessel of 200 L volume (HPP Tolling, St. Margaret’s Dublin, Ireland), where pressure conditions of 200, 400, and 600 MPa were applied for hold times of 4 and 8 min, respectively. Sample temperature prior to HP treatment was 3.4°C and post-treatment temperature of the product was 6.5°C. The inlet water temperature throughout HPP was 9.0 ± 0.5°C. The pressurization rate for each treatment was 100 MPa/min. A control sample was also included, which did not undergo HP treatment, providing a reference for comparison purposes. Following the HP treatment, all the samples, including the control, were stored at −80°C and then sent back frozen to NTNU Ålesund for further analysis.
2.3 Enzymatic hydrolysis
Frozen raw material, both pretreated with HP and control (without pretreatment), was left to thaw in the cold room overnight at 6 ± 2°C prior to enzymatic hydrolysis. A small amount of the minced raw material, corresponding to each pretreatment and the control, was freeze-dried (Freezone 12 L, −84°C, Labconco Corporation, Kansas City, USA) and stored at −80°C for further analysis, while the rest was subjected to enzymatic hydrolysis (Figure 1). The hydrolysis was carried out in duplicate in 4 L closed glass vessels, where an equal proportion of raw material and warm water (50°C) was used. To enhance the quality of the final product, the hydrolysis was performed away from direct sunlight and flushed by nitrogen (Yorulmaz et al., 2011), which was inserted into the vessels for 30 s. Then, the vessels were placed in a water bath with a temperature of 53°C under stirring (150 rpm). When the mixture’s temperature reached 50°C, natural antioxidants previously prepared at University of Zagreb (Dalsvåg et al., 2021) were added as a mix of chamomile and oregano extracts in a ratio of 1:1 which was added at an amount of 1.5 mL/kg raw material. After 10 min, the enzymes Papain FG and Bromelain 400 GDU/g (both from Enzybel) were introduced into the vessels in equal proportion, in a total amount of 0.1% (w/w) (1:1), and the hydrolysis was left to proceed for 1 h. Next, the mixture was filtered through a sieve with a hole size of 1.4 × 1.4 mm where solids/paste were removed, weighed, and stored at −80°C until analysis. Part of the solids/paste was freeze-dried prior to storage. The remaining hydrolysate mixture was equally divided into borosilicate glass beakers of 500 mL and transferred to a microwave oven where the inactivation of the enzymes took place at 90°C for 15 min. The hydrolysate mixture was left to chill before further centrifugation at 2250xg for 45 min. Centrifugation separated the hydrolysate mixture mass into four layers which were collected and analysed; the oil layer on the top, followed by the emulsion, the water-soluble fish protein hydrolysate (FPH), and the sludge at the bottom. After weighing, all the fractions were stored at −80°C for further analysis. Part of the emulsion, FPH, and sludge was freeze-dried and held at −80°C.
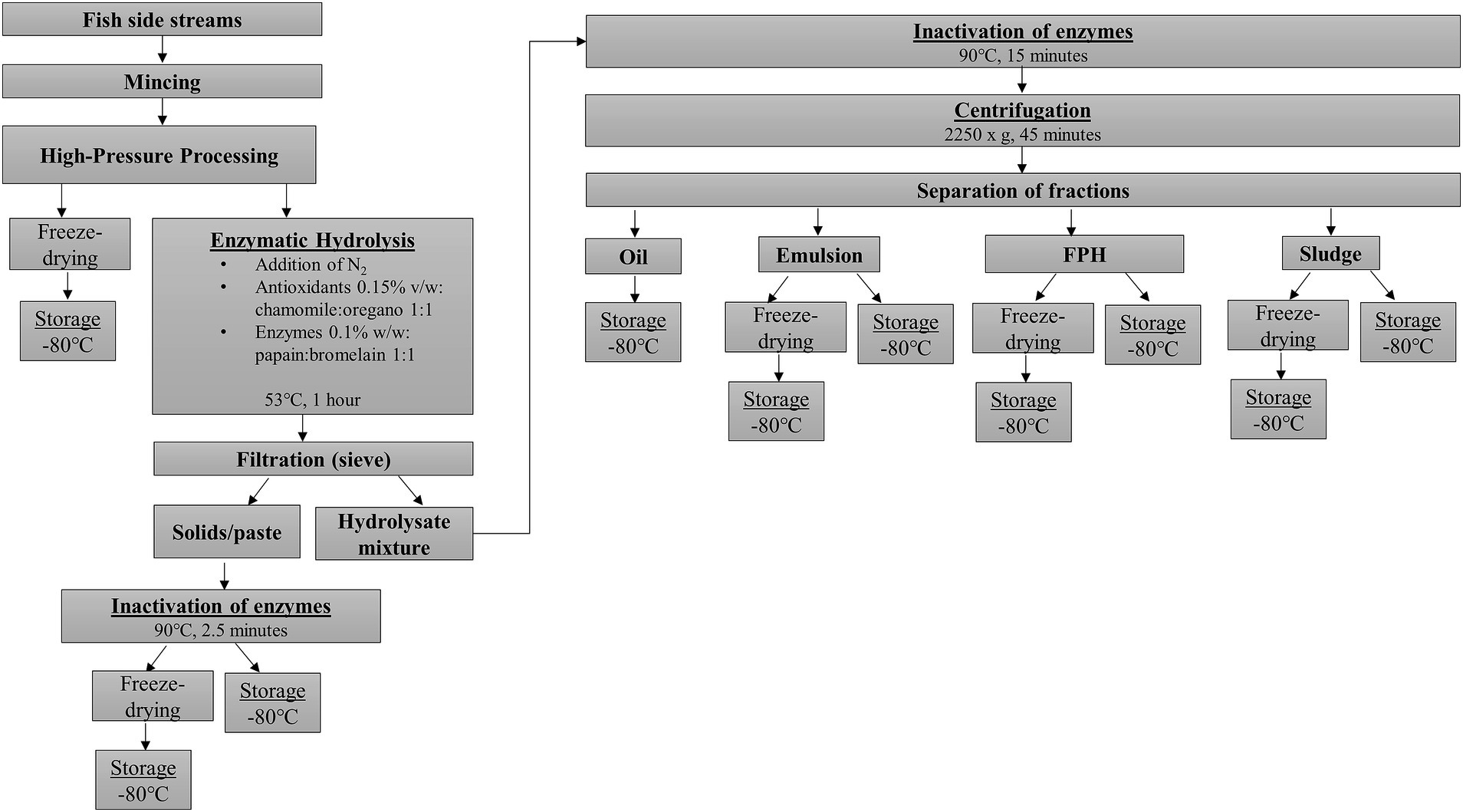
Figure 1. Schematic illustration of the procedure on applying HP pretreatment and enzymatic hydrolysis of a mixture of Atlantic salmon (Salmo salar) and rainbow trout (Oncorhynchus mykiss) rest raw materials and the final products.
2.4 Product yield and recovery of protein and oil
The product yield of each fraction was calculated as a percentage of the total initial weight of the raw material (w/w) before enzymatic hydrolysis. The protein and oil recovery in each fraction obtained after enzymatic hydrolysis was determined as a percentage of the total content of protein and oil (w/w), respectively, present in the raw material prior to enzymatic hydrolysis.
2.5 Chemical analysis
2.5.1 Moisture and ash content
The moisture content of raw material, solids/paste, protein, emulsion, and sludge, before and after the freeze-dryer, was determined by drying at 105°C until constant weight (24 h), according to (AOAC, 1990). The ash content of non-freeze-dried samples was determined by holding the samples at 550°C overnight (AOAC, 1990). Both moisture content and ash were determined in duplicate.
2.5.2 Crude protein content
The crude protein content was measured in triplicate in dried samples multiplying % total nitrogen by the nitrogen-to-protein conversion factor (NPCF) of 6.25, using the Dumas combustion principle, performed in an NDA 702 Dumas Nitrogen Analyser (Velp Scientifica, Italy). In cases where the measured crude protein content deviated from the expected value, a mathematical calculation was employed, taking into account the oil and ash content.
2.5.3 Total lipid content
Oil extraction was performed in duplicate using a mixture of water, methanol, and chloroform, as described by (Bligh and Dyer, 1959).
2.5.4 Determination of free amino acid (FAA) composition
The FAAs in the obtained protein hydrolysates was determined in triplicate, as described by Osnes and Mohr (1985) with modifications.
1 mL of water-soluble protein extract was transferred to Eppendorf tubes before adding 0.25 mL of 10% sulfosalicylic acid, followed by shaking. The solutions were stored in a cold room (4°C) for 30 min and then centrifuged for 10 min at 17400xg with an Eppendorf Microcentrifuge 5418R (Eppendorf AG, Germany). The obtained supernatant was suitably diluted and filtered before being inserted into vials and further analysed by high-pressure liquid chromatography (VHPLC Dionex Ultimate 3,000, Thermo Fisher Scientific Inc., USA) using a Nova Pak C18 column (Waters™, USA).
2.6 Degree of hydrolysis (DH)
The DH of fish protein hydrolysates was evaluated in duplicate by the formol titration method of Taylor (1957). Suitably diluted protein hydrolysate was titrated with NaOH after mixing with formaldehyde, using an automatic titrator (TitroLine® 7,800, Xylem Analytics, Mainz, Germany) coupled with a platinum electrode (Pt 62):
2.7 Statistical analysis
Effects of treatments on product yield and chemical composition was evaluated by ANOVA. A significance level of p < 0.05 was established to assess the statistical significance of observed differences between treatment groups and control. Post hoc analysis was performed using Tukey’s honestly significant difference (HSD) test and Student’s t-test to identify mean differences. The statistical analysis was conducted using SigmaPlot software, version 14 (Systat Software Inc., San Jose, California, USA).
3 Results and discussion
3.1 Product yield
The applied HPP conditions did not exhibit a significant influence on the yield of oil, emulsion, and FPH fractions obtained after enzymatic hydrolysis of samples consisting of a mixture of rainbow trout and Atlantic salmon rest raw material (Figure 2). However, between the quantity of oil and emulsion, and the applied pretreatment a correlation (r = 0.833 and r = 0.845, respectively) was observed. Specifically, higher applied pressure led to a decreased yield of oil and emulsion. The pretreatment conditions of 600 MPa x 8 min, 600 MPa x 4 min, 400 MPa x 8 min, and 400 MPa x 4 min resulted in significantly lower amounts of oil compared to the pretreatments 200 MPa x 8 min and 200 MPa x 4 min. The control demonstrated the highest oil yield. These findings are opposed to the results presented by Thoresen et al. (2020) in their study on HP pretreated residual materials from the chicken industry prior to enzymatic hydrolysis. Furthermore, the pretreatment 200 MPa x 4 min along with the control showed significantly higher emulsion yield compared to the other pretreatments. In contrast, the pretreatment 400 MPa x 4 min resulted in the lowest emulsion yield. However, it is worth highlighting that there was no significant difference in the emulsion yield among this pretreatment and the pretreatments 600 MPa x 4 min and 400 MPa x 8 min.
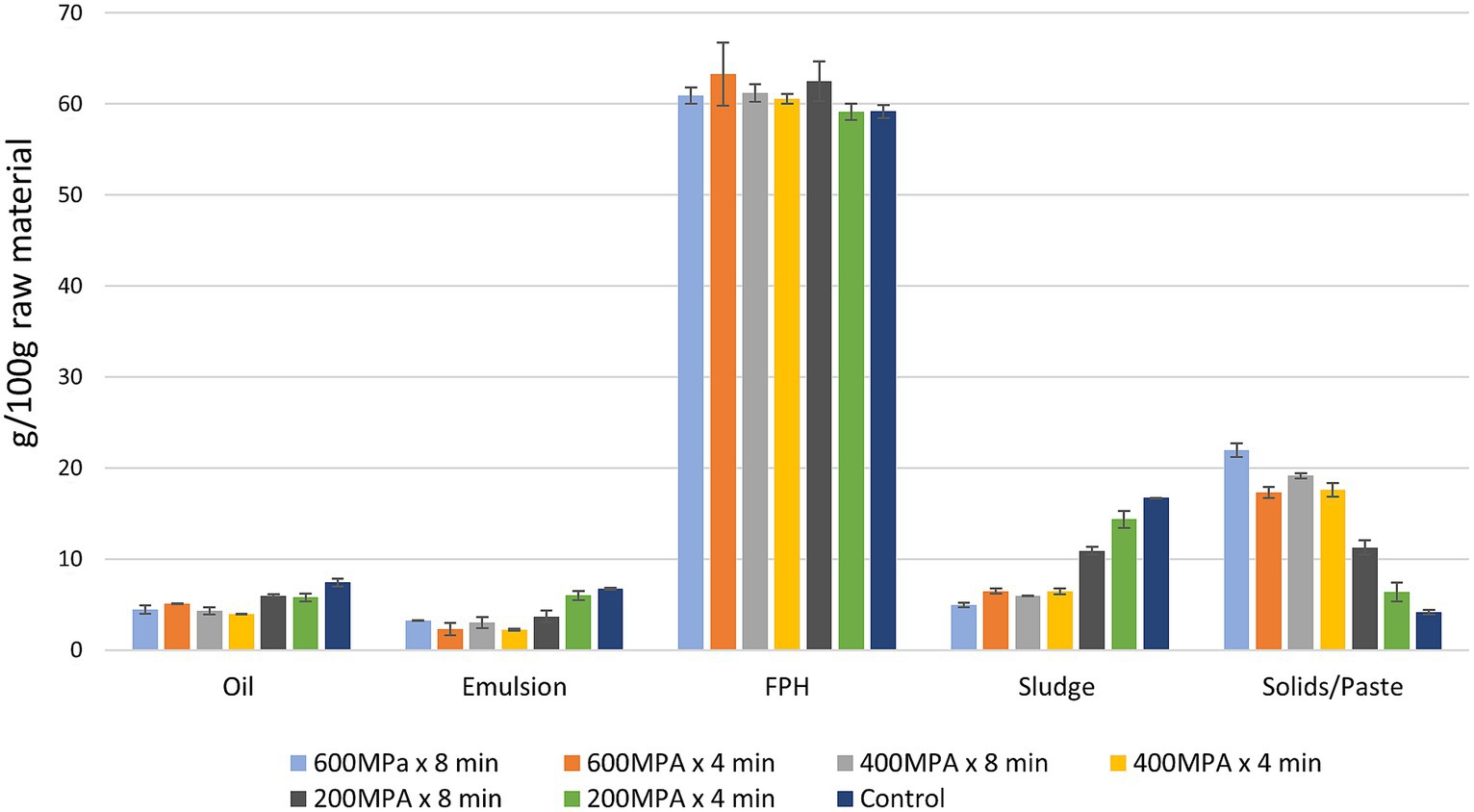
Figure 2. Quantification of the fractions obtained through enzymatic hydrolysis on samples consisting of a mixture of rainbow trout and Atlantic salmon rest raw material subjected to HPP and on the control after the addition of equal proportion of raw material and warm water in hydrolysis vessels expressed as g/100 g raw material (mean ± SD, n = 2).
As displayed in Figure 2, apart from the sample pretreated at 200 MPa x 4 min, the remaining samples subjected to various HPP conditions showed a slightly higher yield of FPH compared to the control. The highest FPH yield was obtained from the sample pretreated at 600 MPa x 4 min. However, this difference was not statistically significant. This increase could be attributed to protein unfolding due to HPP leading to enhanced FPH volume (Thoresen et al., 2020) during enzymatic hydrolysis and the subsequent reduction in hydrophobic free amino acids, as shown in section 3.4.
The yield of sludge and solids/paste obtained after enzymatic hydrolysis was significantly affected by the applied HPP conditions and particularly pressure (Figure 2). The quantity of sludge obtained from samples subjected to pretreatment at 600 MPa x 8 min, 600 MPa x 4 min, 400 MPa x 8 min, and 400 MPa x 4 min was found to be significantly lower compared to the sludge quantity obtained from samples pretreated at 200 MPa x 8 min, 200 MPa x 4 min, or the control. The latter resulted into a significantly higher amount of sludge among all tested conditions suggesting that no HP pretreatment or application of lower pressures can result in greater sludge formation after enzymatic hydrolysis compared to the samples pretreated at higher pressures. These findings could confirm the assumption that the conformational changes in proteins due to HPP may have improved the availability of proteins for hydrolysis contributing to reduced sludge generation.
Regarding the yield of solids/paste fractions, the highest quantity was observed in samples pretreated at 600 MPa x 8 min, 600 MPa x 4 min, 400 MPa x 8 min, and 400 MPa x 4 min with no statistically significant difference among them. However, the sample pretreated at 200 MPa x 8 min demonstrated a significantly lower yield of solids/paste. Further, the sample pretreated at 200 MPa x 4 min and the control resulted in the lowest amount of solids/paste. These findings, highlight the significant correlation (r = 0.938) between the HP pretreatment conditions and the subsequent yield of solids/paste after enzymatic hydrolysis (Figure 3).
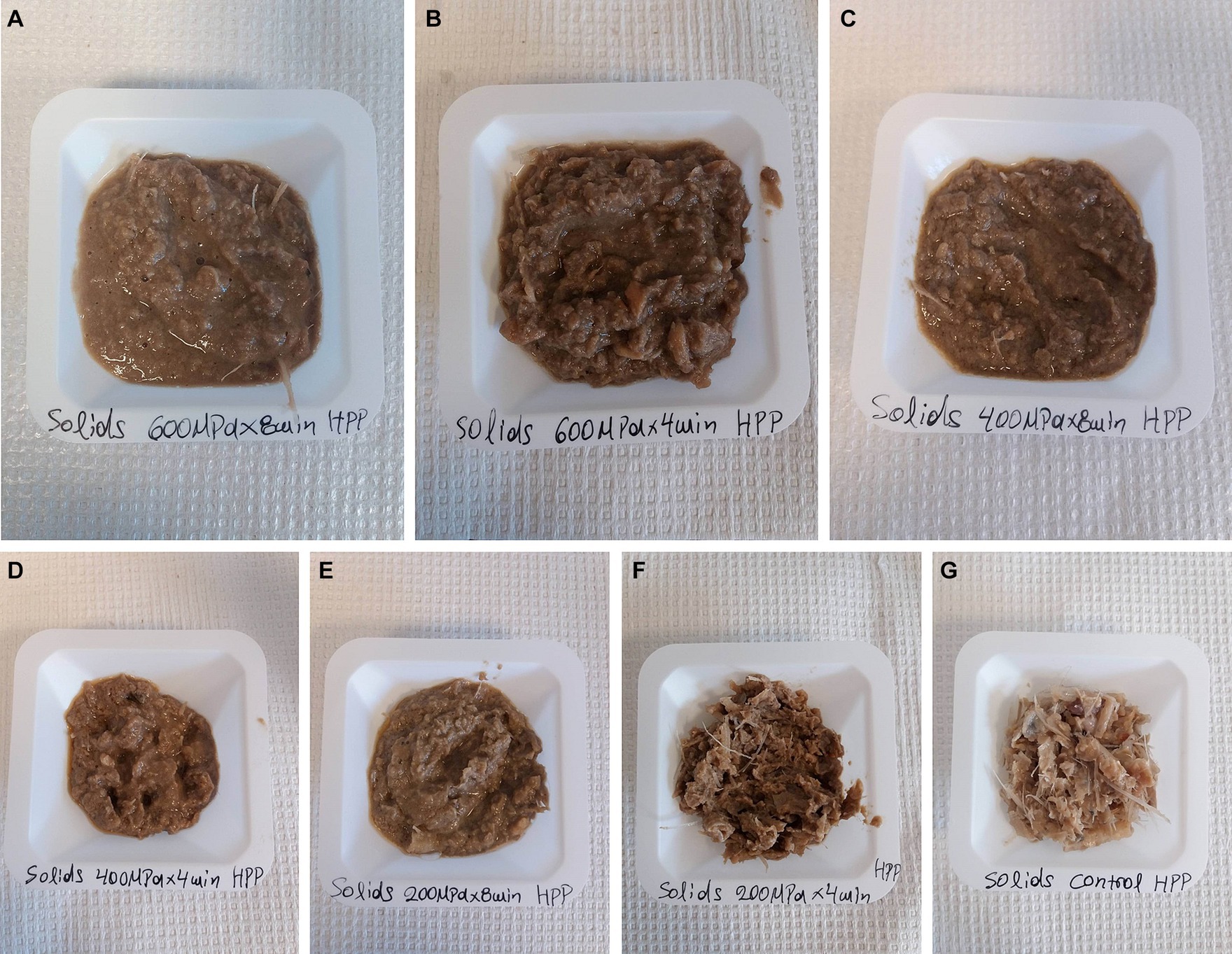
Figure 3. Illustration of the solids/paste fractions obtained after enzymatic hydrolysis on samples consisting of a mixture of rainbow trout and Atlantic salmon rest raw material subjected to HPP and on the control; (A) solids/paste corresponding to HPP 600 MPa x 8 min, (B) solids/paste corresponding to HPP 600 MPa x 4 min, (C) solids/paste corresponding to HPP 400 MPa x 8 min, (D) solids/paste corresponding to HPP 400 MPa x 4 min, (E) solids/paste corresponding to HPP 200 MPa x 8 min, (F) solids/paste corresponding to HPP 200 MPa x 4 min, (G) solids/paste corresponding to the control.
The increased quantity of solids/paste in samples subjected to higher pressures may be attributed to protein denaturation induced by HPP. It has been reported that higher pressures, specifically above 300 MPa, can lead to increased protein denaturation (Lullien-Pellerin and Balny, 2002), resulting in the formation of aggregates that are resistant to enzymatic degradation during hydrolysis (Wang et al., 2015; Joye, 2019). Consequently, the limited enzymatic breakdown of proteins, along with the possibility of denatured proteins encapsulating lipids within the protein matrix (Chapleau et al., 2004), may contribute to the elevated generation of solids/paste during enzymatic hydrolysis. This could also account for the lower oil and sludge yield obtained from samples pretreated by HPP compared to the control, as a correlation (r = 0.890) has been identified between the oil and solids/paste fractions. Furthermore, a significant correlation (r = 0.992) was observed between the sludge and solids/paste fractions. The solids/paste mass obtained from the control was dominated by fish bones.
3.2 Protein and oil recovery
The application of HPP had a significant impact on the protein recovery within the FPH, sludge, and solids/paste fractions. Moreover, HPP application significantly affected the recovery of oil within the sludge and solids/paste fractions. Concerning the FPH fractions, the protein recovery in the control (40.8 ± 0.1 g/100 g dry weight) was significantly higher than that recovered in samples subjected to HP pretreatment (Table 1). Among the pretreated samples, the FPH of the sample pretreated at 200 MPa x 4 min exhibited the highest protein recovery (39.5 ± 0.1 g/100 g dry weight), while the lowest was observed at 400 MPa x 8 min (32.5 ± 0.1 g/100 g dry weight). The oil recovery within FPH fractions was correlated (r = 0.837) with the applied pressure and time. The FPH of the control gave a significantly higher value (1.1 ± 0.1 g/100 g dry weight) compared to the FPH of samples pretreated by HP (Table 2). The values within the FPH fractions of the pretreated samples ranged between 0.3 ± 0.0 g/100 g dry weight and 0.8 ± 0.0 g/100 g dry weight, with the lowest oil recovery observed in FPH of the sample pretreated at 400 MPa x 8 min and the highest in FPH of the sample pretreated at 200 MPa x 8 min, respectively. The duration of the applied pretreatment influenced significantly the protein recovery within the FPH fractions, while it did not exhibit a notable effect on the oil recovery. Among samples subjected to the same pressure, the protein recovery within the FPH of samples pretreated for 4 min was significantly higher compared to those pretreated for 8 min. This observation could suggest that prolonged HP pretreatment could potentially result in decreased protein recovery in FPH.
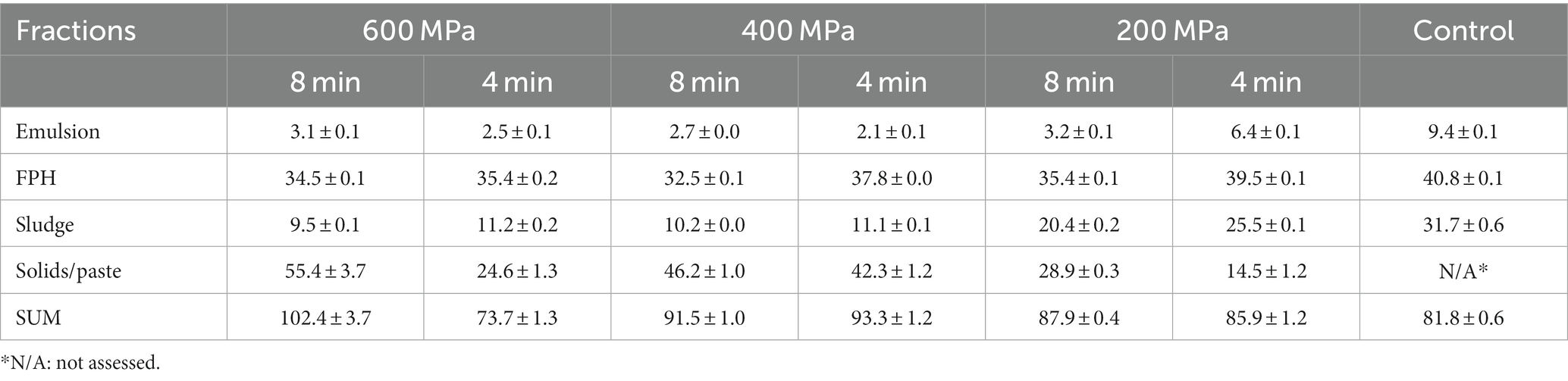
Table 1. Protein recovery in emulsion, FPH, sludge, and solids/paste fractions obtained after enzymatic hydrolysis on samples consisting of a mixture of rainbow trout and Atlantic salmon rest raw material subjected to HPP and on the control expressed as g/100 g dry weight (mean ± SD, n = 2).
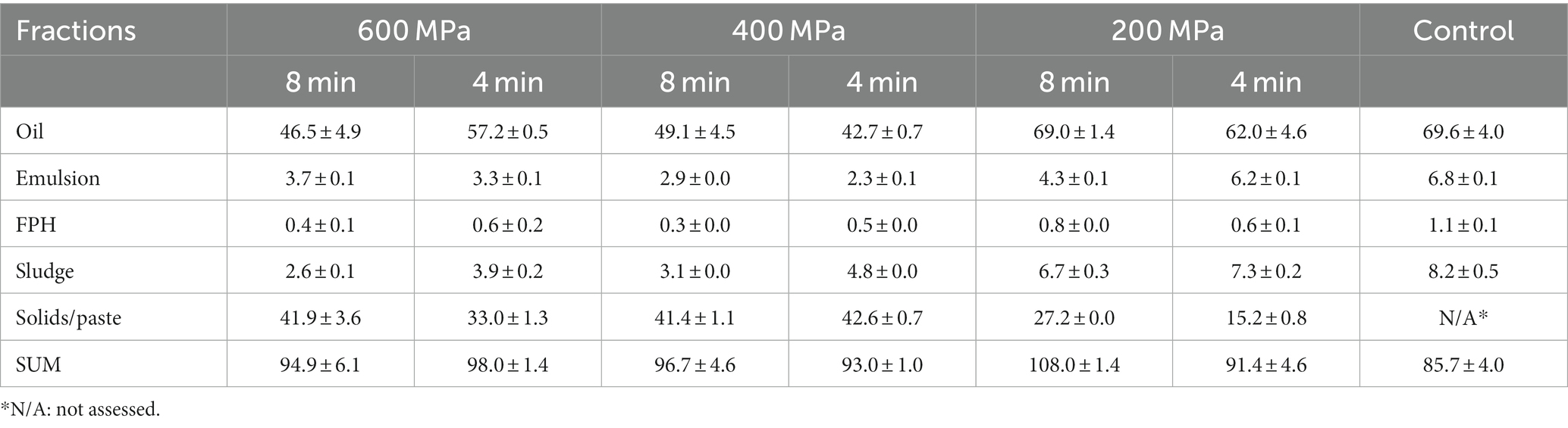
Table 2. Oil recovery in oil, emulsion, FPH, sludge, and solids/paste fractions obtained after enzymatic hydrolysis on samples consisting of a mixture of rainbow trout and Atlantic salmon rest raw material subjected to HPP and on the control expressed as g/100 g dry weight (mean ± SD, n = 2).
In the sludge fractions, the values varied between 9.5 ± 0.1 g/100 g dry weight and 31.7 ± 0.6 g/100 g dry weight for the protein and 2.6 ± 0.1 g/100 g dry weight and 8.2 ± 0.5 g/100 g dry weight for the oil recovery (Tables 1, 2). The sludge fraction obtained from the sample pretreated at 600 MPa x 8 min displayed the lowest protein and oil recovery values, in contrast to the control, which demonstrated the highest recovery levels. As the applied pressure decreased, there was a noticeable increase in protein and oil recovery within the sludge fractions. It is worth noting that the protein and oil recovery in sludge showed a significant correlation (r = 0.942 and r = 0.939, respectively) with the sludge yield (Figure 2), indicating that higher sludge yield was associated with an increased protein and oil recovery within the sludge fractions.
A significantly decreasing trend was evident in both the protein and oil recovery within the solids/paste fractions as the applied pressure decreased or no pressure was applied, as illustrated in Tables 1, 2. This could be attributed to the observed increase in solids/paste yield with higher applied pressure. The protein and oil recovery in solids/paste displayed a significant correlation (r = 0.928 and r = 0.952, respectively) with the quantity of this fraction (Figure 2). Thus, a significantly higher protein recovery was observed in the solids/paste fraction acquired from the sample pretreated at 600 MPa x 8 min (55.4 ± 3.7 g/100 g dry weight) compared to the other pretreatments. The pretreatment 400 MPa x 4 min gave the highest oil recovery (42.6 ± 0.7 g/100 g dry weight), although no statistically significant difference was exhibited between this pretreatment and the solids/paste fraction of the pretreatments 600 MPa x 8 min (41.9 ± 3.6 g/100 g dry weight) and 400 MPa x 8 min (41.4 ± 1.1 g/100 g dry weight). Additionally, a significant correlation (r = 0.945) was noticed between the oil and protein recovery within the solids/paste fractions corresponding to various pretreatments, supporting the assumption that lipids could be potentially entrapped by denatured proteins within the protein matrix (Chapleau et al., 2004). Thus, for the pretreatments 600 MPa x 8 min, 400 MPa x 8 min, 400 MPa x 4 min, the solids/paste fraction emerged as the fraction with the highest protein recovery.
The protein and oil recovery in the emulsion was correlated (r = 0.879 and r = 0.801, respectively) with the pretreatment conditions (Tables 1, 2) and the emulsion yield (Figure 2). The control exhibited significantly higher protein (9.4 ± 0.1 g/100 g dry weight) and oil (6.8 ± 0.1 g/100 g dry weight) recovery in the emulsion compared to the samples pretreated by HP. Among the various HP pretreatment conditions studied, the emulsion obtained from the sample pretreated at 200 MPa x 4 min exhibited the highest protein and oil recovery.
The oil fraction acquired from the control displayed the highest oil recovery (69.6 ± 4.0 g/100 g dry weight). However, no statistically significant difference was observed between the oil recovery in the control and that of samples subjected to pretreatment at 200 MPa x 8 min (69.0 ± 1.4 g/100 g dry weight) and 200 MPa x 4 min (62.0 ± 4.6 g/100 g dry weight). These findings align with the results reported by Zhang et al. (2021) in their study regarding oil extraction from fish heads, where ultra-high pressure was employed as a pretreatment before enzymatic hydrolysis. Both studies demonstrated that, among the various pretreatment conditions tested, the highest oil recovery was observed in samples that underwent pretreatment at lower pressure levels.
3.3 Proximate composition
The protein, lipid, and ash content within the FPH, sludge, and paste/solids fractions did not appear to be influenced by the applied HPP conditions (Figure 4). Nevertheless, significantly higher protein content was observed in the FPH obtained from the samples pretreated at 600 MPa x 8 min (96.43 ± 0.4 g/100 g dry weight), 400 MPa x 4 min (97.2 ± 0.1 g/100 g dry weight), and 200 MPa x 4 min (91.4 ± 0.1 g/100 g dry weight) compared to the control (90.6 ± 0.2 g/100 g dry weight) (Figure 4A). However, there was no significant difference in protein content between the FPH of the sample pretreated at 600 MPa x 8 min and 400 MPa x 4 min. Although the protein yield across the FPH fractions did not show significant differences (Figure 2), there was a tendency for a slightly higher protein composition in the FPH derived from these specific pretreatments. This could confirm the assumption that HPP may facilitate protein unfolding and, due to thermal fluctuations, enhance protein solubility in the FPH fractions (Thoresen et al., 2020), leading to improved protein concentration in these. Regarding the lipid content in the FPH, a correlation emerges with the applied pressure and duration of pretreatment (r = 0.836). The lipid content in the FPH from the control (2.83 ± 0.20 g/100 g dry weight) appeared significantly higher than that from HP pretreated samples. Among the various pretreated samples, the FPH derived from that subjected to 200 MPa x 4 min demonstrated the highest lipid content (1.8 ± 0.0 g/100 g dry weight). In contrast, the lowest lipid content was observed in the FPH obtained from the sample pretreated at 400 MPa x 8 min (0.7 ± 0.0 g/100 g dry weight). Regarding ash content, notable differences were observed, with significantly higher levels found in the FPH from the samples pretreated at 600 MPa x 4 min, 400 MPa x 8 min, and 200 MPa x 8 min compared to the other conditions.
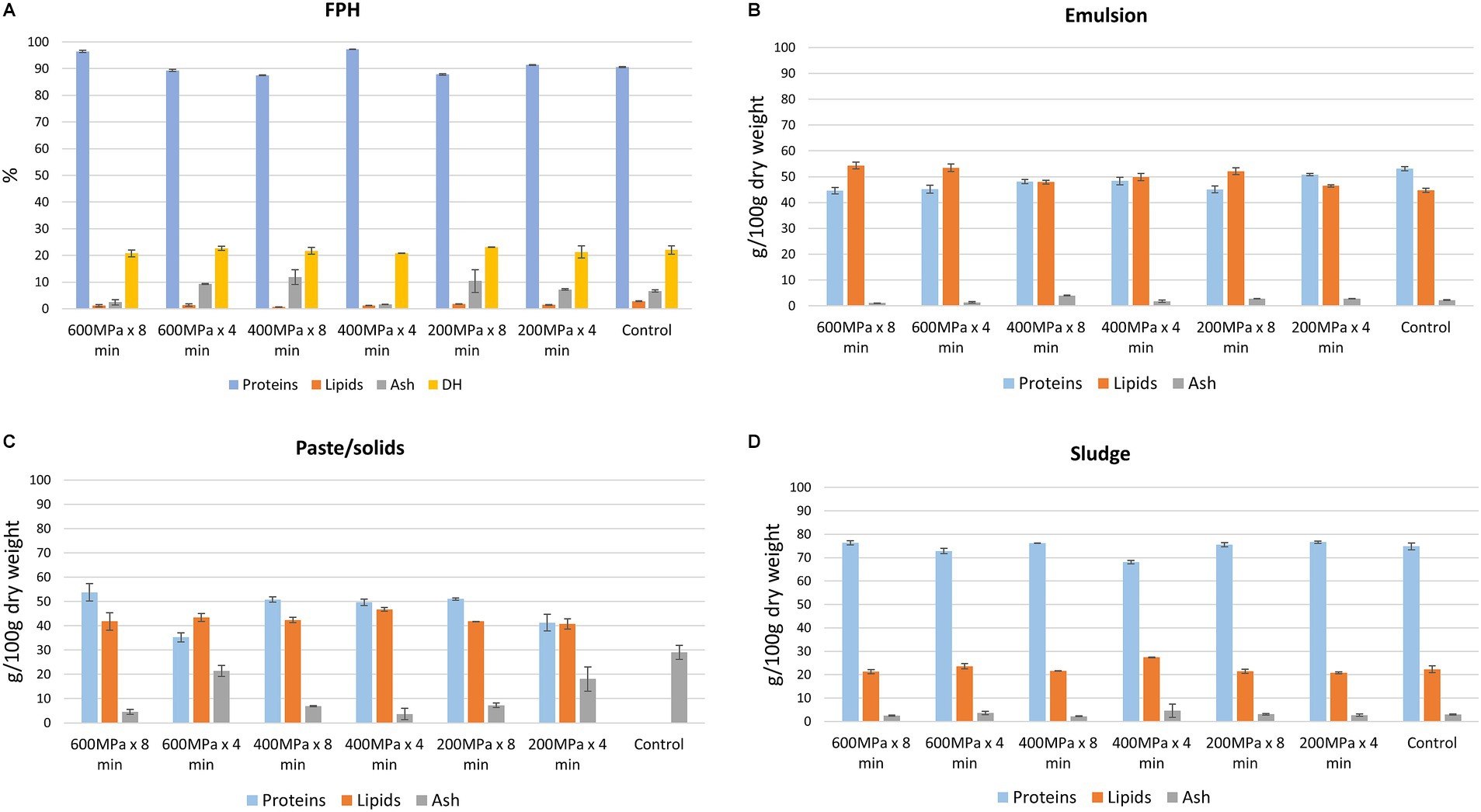
Figure 4. Chemical composition of different fractions obtained after enzymatic hydrolysis on samples pretreated by HPP and the control; (A) proximate composition (g/100 g dry weight, mean ± SD, n = 2), DH (%, mean ± SD, n = 2) of FPH (B) proximate composition of emulsion (g/100 g dry weight, mean ± SD, n = 2), (C) proximate composition of paste/solids (g/100 g dry weight, mean ± SD, n = 2), (D) proximate composition of sludge (g/100 g dry weight, mean ± SD, n = 2).
DH in the FPH fractions did not appear to be influenced by the pretreatment conditions. As depicted in Figure 4A, the samples pretreated at 600 MPa x 4 min (22.6% ± 0.8), and 200 MPa x 8 min (23.1% ± 0.0) showed slightly higher DH in the obtained FPH compared to the FPH from the control (22.0% ± 1.6). However, this difference was not statistically significant.
The protein content within the paste/solids fractions did not exhibit significant difference among the pretreatments 600 MPa x 8 min (53.7 ± 3.6 g/100 g dry weight), 400 MPa x 8 min (50.8 ± 1.1 g/100 g dry weight), 400 MPa x 4 min (49.6 ± 1.4 g/100 g dry weight), and 200 MPa x 8 min (51.0 ± 0.5 g/100 g dry weight) (Figure 4C). On the other hand, the highest lipid content was observed in the paste/solids fractions obtained from the pretreatments 600 MPa x 4 min (43.4 ± 1.6 g/100 g dry weight) and 400 MPa x 4 min (46.7 ± 0.8 g/00 g dry weight), with no significant difference between these two conditions. As for the ash content, there was a correlation between the pretreatment conditions (applied pressure and time) and the ash content in the fractions (r = 0.816). The solids/paste fraction derived from the control, which primarily consisted of bones, exhibited the highest ash value (29.0 ± 2.90 g/100 g dry weight). In contrast, the fraction corresponding to the 400 MPa x 4 min pretreatment condition displayed the lowest (3.7 ± 2.3 g/100 g dry weight). One of the possible utilization of the solids/paste fraction could be its application as a nutrient-rich substrate for microalgae cultivation (Pleissner et al., 2023). This approach could represent a sustainable utilization of the fractions generated in this study.
The sludge fractions displayed a significant correlation between the protein and lipid content (r = 0.995). Sludge obtained from the pretreatments 600 MPa x 8 min (76.3 ± 0.9 g/100 g dry weight), 400 MPa x 8 min (76.2 ± 0.1 g/100 g dry weight), 200 MPa x 8 min (75.6 ± 0.9 g/100 g dry weight), 200 MPa x 4 min (76.6 ± 0.4 g/100 g dry weight), and the control (74.8 ± 1.5 g/100 g dry weight) exhibited higher protein content (Figure 4D). These pretreatments were associated with lower lipid content. Nevertheless, no significant difference was detected among these fractions concerning both protein and lipid content. Furthermore, there were no significant differences in the ash content among sludge fractions derived from the pretreated samples and the control.
In the emulsion fractions, a correlation was found between the protein and lipid content and the applied pretreatment conditions (r = 0.882 and r = 0.833, respectively). As the applied pressure increased, protein content decreased while lipid content increased (Figure 4B). Thus, a significant correlation was observed between the protein and lipid content within the emulsion fractions (r = 0.967). The emulsion obtained from the control displayed a significantly higher protein content (53.1 ± 0.8 g/100 g dry weight) than the emulsion from samples exposed to HP pretreatment. Conversely, lower protein content was detected in emulsion fractions from the samples pretreated at 600 MPa x 8 min (44.6 ± 1.3 g/100 g dry weight), 600 MPa x 4 min (45.2 ± 1.5 g/100 g dry weight), and 200 MPa x 8 min (45.1 ± 1.3 g/100 g dry weight). The same pretreatment conditions corresponded to emulsion fractions with the highest observed lipid content, while the emulsion corresponding to the control demonstrated the lowest. The ash content values exhibited a range between 1.1 ± 0.0 g/100 g dry weight and 4.0 ± 0.1 g/100 g dry weight. The emulsion from the sample treated at 400 MPa x 8 min yielded the lowest ash content, while the highest ash content was observed in the emulsion from the sample subjected to pretreatment at 600 MPa x 8 min.
3.4 Free amino acid composition
The FAA composition of the FPH obtained from different HP pretreatments in a mixture of rainbow trout and Atlantic salmon rest raw material is presented in Table 3. Seventeen FAAs were identified and analyzed in this study. The results indicated that there was a significant reduction in the levels of all individual FAAs between the FPH derived from HP pretreated samples and the control. A significant correlation (r = 0.921) was observed between the applied HP pretreatment and the total amount of FAAs. Higher pressure levels resulted in lower total FAA levels, with the FPH pretreated at 600 MPa x 4 min exhibiting the lowest content (37.58 ± 0.64 mg/g dry weight), while the FPH from the control showed the highest content (72.06 ± 1.31 mg/g dry weight). However, significant differences were neither found between the samples pretreated at 600 and 400 MPa nor between the FPH from the control and the samples subjected to pretreatment at 200 MPa x 4 min. A similar trend was observed in the quantities of essential FAAs and non-essential FAAs. In contrast to our findings, the study conducted by Yue et al. (2016) did not observe a comparable decrease in the levels of essential FAAs on day 0 of storage during their investigation into the effects of HPP on squid muscles. In the current study, leucine and lysine emerged as the predominant essential FAAs, while glycine/arginine and alanine dominated the non-essential FAAs. A significant observation was made regarding the content of essential FAAs, as well as glutamine and alanine (non-essential FAA), in the FPH obtained from samples subjected to different levels of HPP. The data revealed a significant decrease in the concentration of these amino acids in FPH derived from samples pretreated at 600 and 400 MPa, with the content being approximately half of that observed in samples pretreated at 200 MPa or the control. These results are consistent with the findings reported by Ahmed et al. (2021) in their study on HP treated hammour (Epinephelus coioides) fillets. The observed decrease in the concentration of the amino acids may be explained by the solubilization from muscle proteins which occurred at a slower rate compared to their conversion into biogenic amines through the process of decarboxylation (Ciampa et al., 2012). A slight increase in the concentration of serine, glycine/arginine, methionine, phenylalanine, leucine, and lysine was observed in FPH samples subjected to pretreatment at 200 MPa x 4 min compared to control, although the difference was not statistically significant. This observation can be attributed to the release of these amino acids (AAs) from degraded proteins as a result of improved proteolysis induced by HPP (Yue et al., 2016).
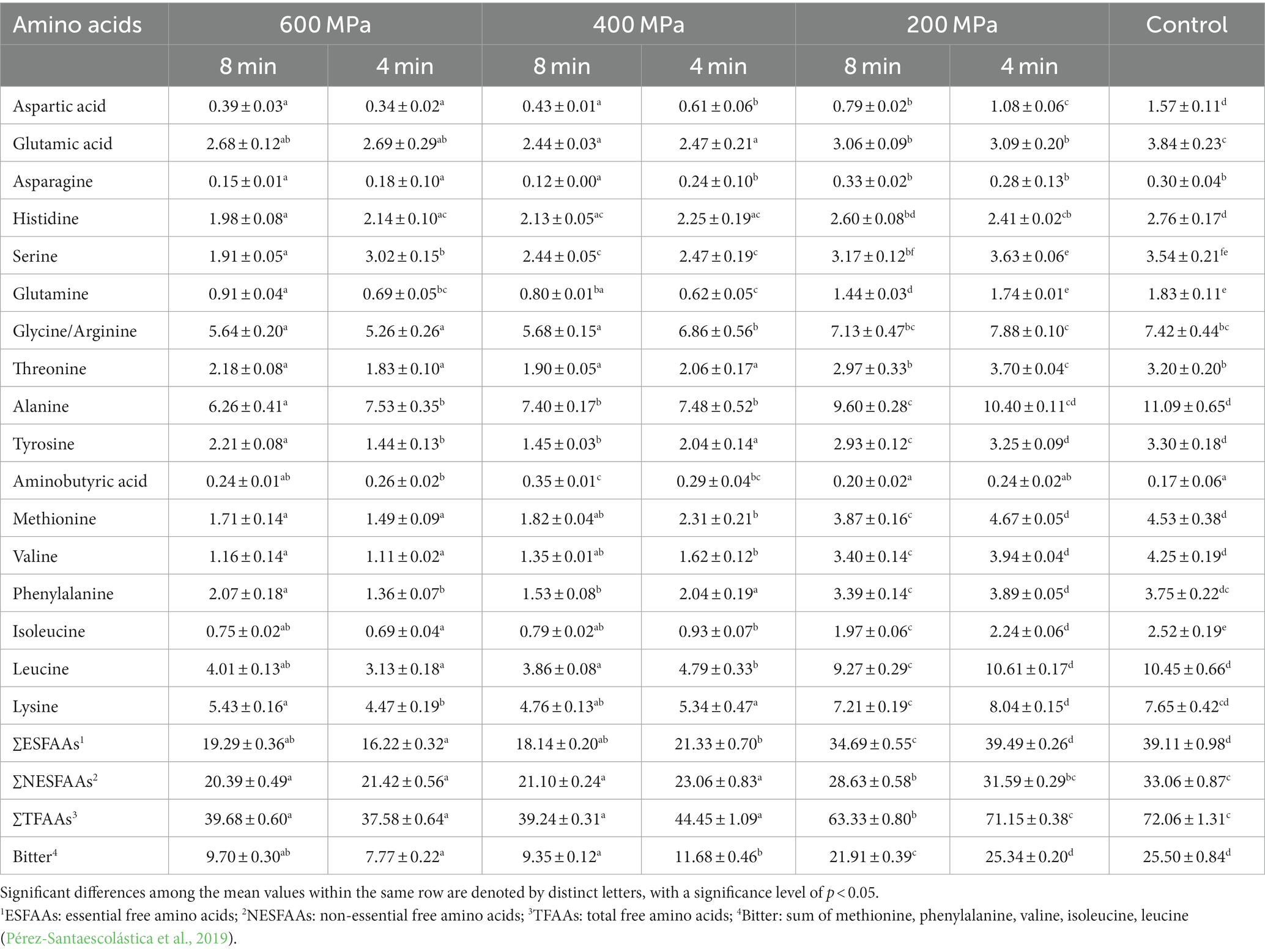
Table 3. Effect of HPP treatments on the FAA composition within a mixture of rainbow trout and Atlantic salmon rest raw material, mg/g dry weight, in FPH (mean ± SD, n = 3).
Alterations in the concentration of individual FAAs can significantly impact the taste properties of fish protein hydrolysates. Specifically, the presence of hydrophobic AAs, such as phenylalanine, valine, leucine, isoleucine, methionine, and proline, is closely associated with the perception of bitterness (Pérez-Santaescolástica et al., 2019). The findings of our study could indicate that the bitter taste of FPH could be influenced by HPP. Higher pressure levels applied during HPP could be associated with reduced bitterness in FPH (Table 3). Specifically, the FPH obtained from samples pretreated at 600 MPa x 4 min displayed the lowest content of hydrophobic FAAs, and therefore should probably have the lowest bitter taste, while the FPH from the control exhibited the highest amount of hydrophobic FAAs.
4 Conclusion
The study has shown that the applied HPP has a significant effect on the protein recovery, but not on the protein, lipid, and ash content within the FPH, sludge, and solids/paste fractions. Among the samples, the control exhibited the highest protein and oil recovery in the FPH and sludge, while the sample pretreated at 600 MPa x 8 min yielded sludge with the lowest protein and oil recovery. The samples pretreated at 600 MPa x 8 min and 400 MPa x 4 min produced FPH with the highest protein content. Degree of hydrolysis was found to be unaffected by the HPP. In addition, the application of HPP did not significantly impact the yield of oil, emulsion, and FPH fractions. However, the yield of sludge and solids/paste was notably influenced by the applied HPP conditions, especially the applied pressure levels. The control sample exhibited the highest oil, emulsion, and sludge yield, while the pretreatments at 400 MPa x 4 min and 600 MPa x 8 min resulted in the lowest emulsion yield. Furthermore, as the applied pressure increased, a decrease in the total FAAs of FPH fractions was observed, with an equal decrease in the amount of both essential and non-essential FAAs.
Data availability statement
The raw data supporting the conclusions of this article will be made available by the authors, without undue reservation.
Author contributions
EK: Conceptualization, Data curation, Formal analysis, Investigation, Methodology, Resources, Software, Validation, Writing – original draft. ED: Formal analysis, Investigation, Resources, Supervision, Validation, Writing – review & editing. GA: Supervision, Writing – review & editing. TR: Investigation, Resources, Supervision¸ Writing – review & editing. BT: Investigation, Methodology, Resources, Writing – review & editing. JC: Conceptualization, Formal analysis, Investigation, Methodology, Resources, Supervision, Writing – review & editing.
Funding
The author(s) declare that no financial support was received for the research, authorship, and/or publication of this article.
Acknowledgments
The authors wish to express their gratitude to Siri Stavrum for her contribution to the determination of free amino acid composition.
Conflict of interest
The authors declare that the research was conducted in the absence of any commercial or financial relationships that could be construed as a potential conflict of interest.
Publisher’s note
All claims expressed in this article are solely those of the authors and do not necessarily represent those of their affiliated organizations, or those of the publisher, the editors and the reviewers. Any product that may be evaluated in this article, or claim that may be made by its manufacturer, is not guaranteed or endorsed by the publisher.
References
Ahmed, J., Habeebullah, S. F. K., Thomas, L., Mulla, M. Z., Jacob, H., and Alagarsamy, S. (2021). Effect of high-pressure treatment and refrigerated storage on the amino acid profile, color, and texture of hammour (Epinephelus coioides) fillets. Journal of Food Processing and Preservation 45:e15977. doi: 10.1111/jfpp.15977
Al Khawli, F., Pateiro, M., Domínguez, R., Lorenzo, J. M., Gullón, P., Kousoulaki, K., et al. (2019). Innovative green technologies of intensification for valorization of seafood and their by-products. Mar. Drugs 17:689. doi: 10.3390/md17120689
Ali, A., Wei, S., Liu, Z., Fan, X., Sun, Q., Xia, Q., et al. (2021). Non-thermal processing technologies for the recovery of bioactive compounds from marine by-products. Lwt 147:111549. doi: 10.1016/j.lwt.2021.111549
A.O.A.C. (1990). Official Methods of Analysis. 15th Edition. Washington DC: Association of Official Analytical Chemist.
Araujo, J., Sica, P., Costa, C., and Márquez, M. (2021). Enzymatic hydrolysis of fish waste as an alternative to produce high value-added products. Waste and Biomass Valorization 12, 847–855. doi: 10.1007/s12649-020-01029-x
Bartolomei, M., Cropotova, J., Bollati, C., Kvangarsnes, K., d’Adduzio, L., Li, J., et al. (2023). Rainbow trout (Oncorhynchus mykiss) as source of multifunctional peptides with antioxidant. ACE and DPP-IV Inhibitory Activities. Nutrients 15:829. doi: 10.3390/nu15040829
Bligh, E. G., and Dyer, W. J. (1959). A rapid method of total lipid extraction and purification. Can. J. Biochem. Physiol. 37, 911–917. doi: 10.1139/o59-099
Chapleau, N., Mangavel, C., Compoint, J. P., and de Lamballerie-Anton, M. (2004). Effect of high-pressure processing on myofibrillar protein structure. J. Sci. Food Agric. 84, 66–74. doi: 10.1002/jsfa.1613
Ciampa, A., Picone, G., Laghi, L., Nikzad, H., and Capozzi, F. (2012). Changes in the amino acid composition of Bogue (Boops boops) fish during storage at different temperatures by 1H-NMR spectroscopy. Nutrients 4, 542–553. doi: 10.3390/nu4060542
Dalsvåg, H., Cropotova, J., Jambrak, A. R. E., Janči, T., Španěl, P., Dryahina, K., et al. (2021). Mass spectrometric quantification of volatile compounds released by fresh Atlantic salmon stored at 4° C under modified atmosphere packaging and vacuum packaging for up to 16 days. ACS Food Science & Technology 2, 400–414. doi: 10.1021/acsfoodscitech.1c00259
De Aguiar Saldanha Pinheiro, A. C., Martí-Quijal, F. J., Barba, F. J., Tappi, S., and Rocculi, P. (2021). Innovative non-thermal technologies for recovery and valorization of value-added products from crustacean processing by-products—an opportunity for a circular economy approach. Foods 10:2030. doi: 10.3390/foods10092030
Ekonomou, S. I., and Boziaris, I. S. (2021). Non-thermal methods for ensuring the microbiological quality and safety of seafood. Appl. Sci. 11:833. doi: 10.3390/app11020833
FAO (2022). The state of world fisheries and aquaculture 2022. Towards blue transformation. Rome, Italy: FAO Rome.
Hassoun, A., Cropotova, J., Trollman, H., Jagtap, S., Garcia-Garcia, G., Parra-López, C., et al. (2023). Use of industry 4.0 technologies to reduce and valorize seafood waste and by-products: a narrative review on current knowledge. Current research in food science 6:100505. doi: 10.1016/j.crfs.2023.100505
Kim, S.-K., and Mendis, E. (2006). Bioactive compounds from marine processing byproducts–a review. Food Res. Int. 39, 383–393. doi: 10.1016/j.foodres.2005.10.010
Liaset, B., Lied, E., and Espe, M. (2000). Enzymatic hydrolysis of by-products from the fish-filleting industry; chemical characterisation and nutritional evaluation. J. Sci. Food Agric. 80, 581–589. doi: 10.1002/(SICI)1097-0010(200004)80:5%3C581::AID-JSFA578%3E3.0.CO;2-I
Lullien-Pellerin, V., and Balny, C. (2002). High-pressure as a tool to study some proteins’ properties: conformational modification, activity and oligomeric dissociation. Innovative Food Sci. Emerg. Technol. 3, 209–221. doi: 10.1016/S1466-8564(02)00045-0
Olatunde, O. O., and Benjakul, S. (2018). Nonthermal processes for shelf-life extension of seafoods: a revisit. Compr. Rev. Food Sci. Food Saf. 17, 892–904. doi: 10.1111/1541-4337.12354
Osnes, K. K., and Mohr, V. (1985). Peptide hydrolases of Antartic krill, Euphausia superba. Comparative Biochemistry and Physiology Part B: Comparative Biochemistry 82, 599–606. doi: 10.1016/0305-0491(85)90496-1
Pérez-Santaescolástica, C., Carballo, J., Fulladosa, E., Munekata, P., Campagnol, P. B., Gómez, B., et al. (2019). Influence of high-pressure processing at different temperatures on free amino acid and volatile compound profiles of dry-cured ham. Food Res. Int. 116, 49–56. doi: 10.1016/j.foodres.2018.12.039
Pleissner, D., Schönfelder, S., Händel, N., Dalichow, J., Ettinger, J., Kvangarsnes, K., et al. (2023). Heterotrophic growth of Galdieria sulphuraria on residues from aquaculture and fish processing industries. Bioresour. Technol. 384:129281. doi: 10.1016/j.biortech.2023.129281
Rustad, T., Storrø, I., and Slizyte, R. (2011). Possibilities for the utilisation of marine by-products. Int. J. Food Sci. Technol. 46, 2001–2014. doi: 10.1111/j.1365-2621.2011.02736.x
Siddiqui, S. A., Schulte, H., Pleissner, D., Schönfelder, S., Kvangarsnes, K., Dauksas, E., et al. (2023). Transformation of seafood side-streams and residuals into valuable products. Foods 12:422. doi: 10.3390/foods12020422
Šližyte, R., Daukšas, E., Falch, E., Storrø, I., and Rustad, T. (2005). Yield and composition of different fractions obtained after enzymatic hydrolysis of cod (Gadus morhua) by-products. Process Biochem. 40, 1415–1424. doi: 10.1016/j.procbio.2004.06.033
Tao, Y., Sun, D.-W., Hogan, E., and Kelly, A. L. (2014). High-pressure processing of foods: an overview. Emerging technologies for food processing, 3–24. doi: 10.1016/B978-0-12-411479-1.00001-2
Taylor, W. (1957). Formol titration: an evaluation of its various modifications. Analyst 82, 488–498. doi: 10.1039/AN9578200488
Thoresen, P. P., Álvarez, R. G., Vaka, M. R., Rustad, T., Sone, I., and Fernández, E. N. (2020). Potential of innovative pre-treatment technologies for the revalorisation of residual materials from the chicken industry through enzymatic hydrolysis. Innovative Food Sci. Emerg. Technol. 64:102377. doi: 10.1016/j.ifset.2020.102377
Wang, Z., Ju, X., He, R., Yuan, J., and Aluko, R. E. (2015). Effect of high pressure treatment on rapeseed protein microparticle properties and gastrointestinal release behavior of the encapsulated peptides. Food Res. Int. 77, 549–555. doi: 10.1016/j.foodres.2015.09.023
Yorulmaz, A., Tekin, A., and Turan, S. (2011). Improving olive oil quality with double protection: destoning and malaxation in nitrogen atmosphere. Eur. J. Lipid Sci. Technol. 113, 637–643. doi: 10.1002/ejlt.201000481
Yue, J., Zhang, Y., Jin, Y., Deng, Y., and Zhao, Y. (2016). Impact of high hydrostatic pressure on non-volatile and volatile compounds of squid muscles. Food Chem. 194, 12–19. doi: 10.1016/j.foodchem.2015.07.134
Keywords: fish protein hydrolysates, fish oil, high-pressure processing, fish rest raw material, salmon, trout
Citation: Kotsoni E, Daukšas E, Aas GH, Rustad T, Tiwari B and Cropotova J (2024) Effect of high-pressure pretreatment on enzymatic hydrolysis of a mixture of rainbow trout (Oncorhynchus mykiss) and Atlantic salmon (Salmo salar) rest raw material. Front. Sustain. Food Syst. 8:1313975. doi: 10.3389/fsufs.2024.1313975
Edited by:
Dandan Ren, Dalian Ocean University, ChinaReviewed by:
Saiful Irwan Zubairi, National University of Malaysia, MalaysiaShi Wenzheng, Shanghai Ocean University, China
Copyright © 2024 Kotsoni, Daukšas, Aas, Rustad, Tiwari and Cropotova. This is an open-access article distributed under the terms of the Creative Commons Attribution License (CC BY). The use, distribution or reproduction in other forums is permitted, provided the original author(s) and the copyright owner(s) are credited and that the original publication in this journal is cited, in accordance with accepted academic practice. No use, distribution or reproduction is permitted which does not comply with these terms.
*Correspondence: Elissavet Kotsoni, ZWxpc3NhdmV0LmtvdHNvbmlAbnRudS5ubw==