- 1Instituto Nacional de Investigación Agropecuaria (INIA), Treinta y Tres, Uruguay
- 2Harper Adams University, Edgmond, Shropshire, United Kingdom
- 3Net Zero and Resilient Farming, Rothamsted Research, Okehampton, Devon, United Kingdom
The development of metrics to assess the sustainability of food production systems is vital for achieving sustainable global agri-food systems. Nitrogen use efficiency (NUE), defined as the ratio of nitrogen (N) in food outputs to total N inputs, is a key indicator of resource efficiency in crop and livestock systems. This study quantified and compared NUE, N surplus (NSURP, total N inputs minus N in food products), and N balance (N retained, calculated as inputs minus food outputs and losses) across four pasture-crop rotation systems with varying land-use intensity, evaluated at both component (crop and livestock) and system levels from 2019 to 2022. The systems included continuous cropping (CC), short rotation (SR; 2-year crops + 2-year pasture), long rotation (LR; 2-year crops + 4-year pasture), and forage rotation (FR; continuous pasture with tall fescue). Data were primarily collected at the field level. Major findings showed crop NUE ranging from 62.5% (CC) to 83.8% (SR), livestock NUE from 5.5% (FR) to 24.4% (CC), and system NUE from 5.5% (FR) to 43.4% (CC). Systems with pastures (SR, LR, FR) retained more soil N (up to 64 kg N ha−1 in FR) compared to CC (4.9 kg N ha−1), highlighting the role of pastures in nutrient retention. These component-specific differences underscore the need for tailored management strategies, such as optimizing organic N inputs from livestock and improving grazing practices, to enhance NUE and guide systems toward a sustainable “safe operating space.”
1 Introduction
Food production systems worldwide face considerable challenges in balancing nutrient provision with food security. Global food demand is projected to increase by 1.1–1.5% annually over the next decade, driven by population growth and economic development (OECD/FAO, 2022). However, this demand is accompanied by rising variability in international prices of agricultural inputs, exacerbated by global shocks such as the war in Ukraine (Rawtani et al., 2022). Concurrently, modern agriculture exerts increasing pressure on land and water resources (Spiertz, 2010), raising concerns about its environmental impact and the sustainability of production practices (Keeler et al., 2016; Wuepper et al., 2020).
Sustainable intensification offers a promising approach to meet food demands while achieving environmental and ecological goals (Haughey et al., 2023; Soria-Lopez et al., 2023). Sustainable intensification seeks to transform the entire food chain into a sustainable process by optimizing natural resource use and minimizing harm from agricultural activities (Cassman and Grassini, 2020). Mixed crop-livestock systems are a form of sustainable intensification, leveraging management strategies, such as enhancing agroecosystem diversity (Bowles et al., 2020) and integrating pastures into crop rotations (Carswell et al., 2022; Garcı́a-Préchac et al., 2004), to bolster resilience to weather extremes without compromising yield. In addition, tools such as high-yielding crop varieties, fertilization, irrigation, and pesticides enhance productivity (Franzluebbers and Martin, 2022; Paruelo and Sierra, 2023).
In Uruguay, mixed crop-livestock systems are increasingly vital, covering 17% of livestock-utilized land (DIEA–MGAP, 2022) due to crop rotation regulations (MGAP, 2020). In 2021, meat and grain production accounted for 23 and 22% of total exports, respectively (Uruguay, 2021), underscoring their economic significance. Nutrient recycling, particularly of nitrogen (N), enhances sustainability in these systems. For instance, Xia et al. (2017) demonstrated that substituting synthetic fertilizers with livestock manure boosts crop productivity, N use efficiency (NUE), and soil carbon stocks while reducing reactive N losses. In this study, nutrient recycling occurs within the farm: Livestock and crops share land across seasons, enabling manure to enrich soils without off-farm processing or transport, a practical form of nutrient circularity embedded in Uruguay’s production and landscape dynamics.
Despite their potential, mixed crop-livestock systems rely heavily on synthetic fertilizers, raising environmental concerns (Xue et al., 2010). Nutrient imbalances can occur (Fowler et al., 2013; Goulding et al., 2008), necessitating optimal N inputs to maximize NUE and minimize environmental N release (Löw et al., 2020; Powell and Rotz, 2015; Quemada et al., 2020). While Spiller et al. (2024) note that nutrient recycling alone is insufficient without tailored efficiency and sufficiency strategies, there is no uniform methodology to assess these risks across systems. Existing NUE studies vary widely in scope, covering different products, boundaries, scales, and regions (Ladha et al., 2005; Gerber et al., 2014; Powell et al., 2010; Ma et al., 2012), and literature on mixed systems remains limited compared to pure crop or livestock systems (e.g., Milroy et al., 2019; Bratti et al., 2022). The EU Nitrogen Expert Panel (2015) proposed a graphical NUE evaluation tool which includes several indicative thresholds to avoid N mining, low efficiency, high surplus (NSURP) potentially linked with environmental harm, and defining a system minimum N productivity, but its application to mixed systems is underexplored. This gap highlights the need to deepen understanding of N dynamics and efficiency in these systems, especially in regions such as Uruguay where they are economically and environmentally significant.
In this context, it becomes necessary to implement robust analytical frameworks to better understand nitrogen flows and their implications within mixed systems. System-level indicators such as NUE, nitrogen balance (NBAL), and nitrogen surplus (NSURP) provide useful insights into resource use efficiency and environmental performance, especially when applied to long-term, integrated production systems.
We hypothesize that mixed crop-livestock systems can circulate N between their crop and livestock components, enhancing N availability and reducing reliance on external inputs such as synthetic fertilizers. Therefore, this study aims to quantify and compare NUE, N surplus (NSURP), and N balance (N retained) across four pasture-crop rotations with varying land-use intensity, continuous cropping (CC), short rotation (SR), long rotation (LR), and forage rotation (FR), at both component (crop and livestock) and system levels, using data collected over 3 years (2019–2022). By developing and analyzing these indicators, we seek to identify management strategies that optimize resource use, reduce environmental impact, and guide these systems toward a “safe operating space” for sustainability.
2 Materials and methods
Measurements and metrics calculated in this study refer to the third phase of the Palo a Pique Long-Term Experiment (‘Land Expansion and Livestock Intensification’), which started in 2019, after a redesign, as described by Rovira et al. (2020). The main changes that occurred in this phase were (i) relocation of permanent pasture system, (ii) addition of a support grassland area to each system, and (iii) implementation of a bespoke livestock strategy for each system.
2.1 Experimental site
A long-term pasture-crop rotation experiment under no-tillage was established in 1995 at the ‘Palo a Pique’ Experimental Unit in Treinta y Tres (33°160 S, 54°290 W), Uruguay, at the National Institute of Agricultural Research (INIA) facilities. Uruguay is in the subtropical climate zone; the annual mean (± SD) accumulated rainfall in the experimental site for the last 28 years (1995–2022) was 1,249 ± 72.2 mm per year distributed uniformly throughout the year. The mean maximum and minimum air temperatures for the same period were 23 ± 0.1°C and 11 ± 0.6°C, respectively. The research site has a 3% average slope, and the loam soils are Oxyaquic Argiudolls according to USDA-NRCS (1996).
2.2 Description of the pasture-crop rotations
As described in a study by Pereyra-Goday et al. (2022), four systems were evaluated. Table 1 describes the crops included in each rotation (pasture-crop rotation or pasture rotation) and purpose of the crop phase (crop or grazing). Pasture-crop rotations represent alternative pasture-crop arrangements with different temporal and spatial combinations of land use.
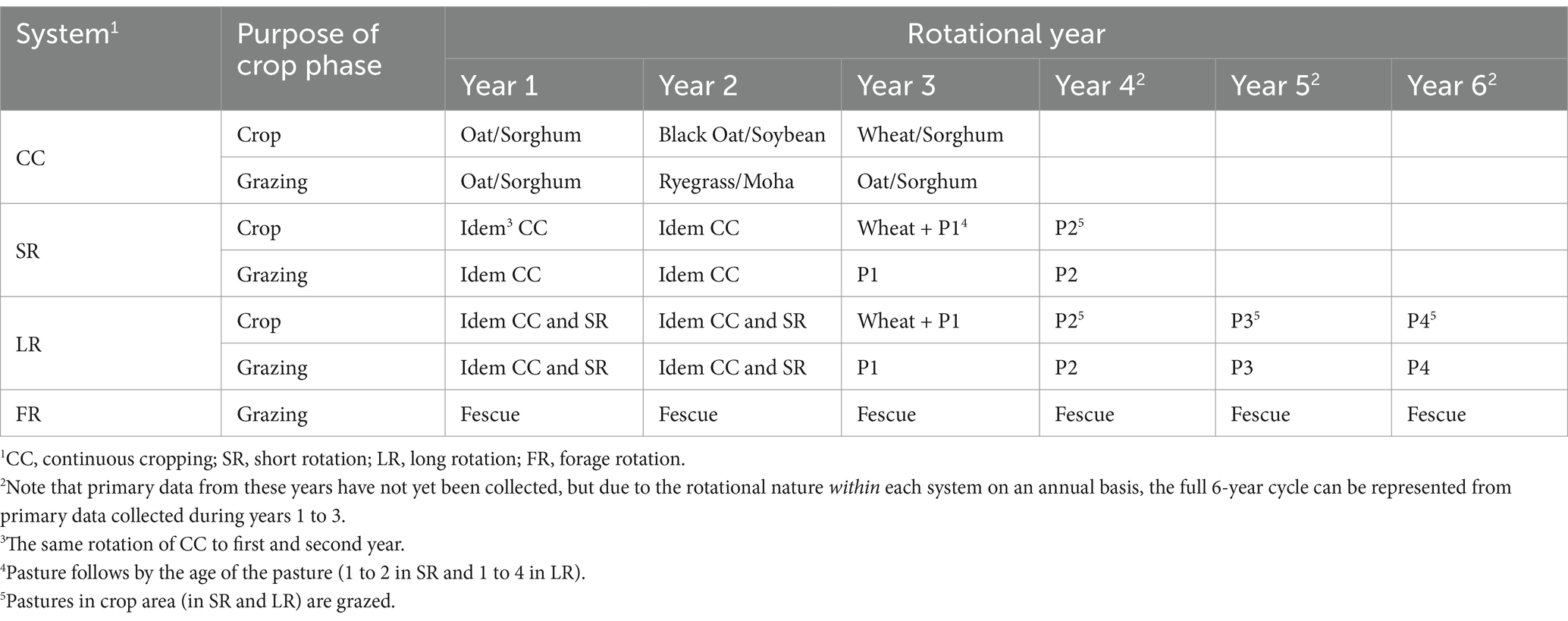
Table 1. Pasture and crop sequence for each system evaluated at Palo a Pique long-term experiment, Treinta y Tres, Uruguay (Pereyra-Goday et al., 2022).
The continuous cropping system (CC, 12 ha) is represented by a rotation with two crops per year. CC does not rotate with pastures, but it is complemented with an external area (6 ha) of a permanent improved pasture composed of tall fescue (Festuca arundinacea Schreb.), birdsfoot trefoil (Lotus corniculatus L.), and white clover (Trifolium repens L.) re-seeded every 5 years with the same species to ensure sustained establishment. The short rotation system (SR, 24 ha) alternates in the same land for 2 years of crops identical to CC with another 2 years of grass–legume pastures based on Yorkshire fog (Holcus lanatus L.) and/or Italian ryegrass (Lolium multiflorum L.) interspersed with red clover (Trifolium pratense L.). The long rotation system (LR, 36 ha) alternates in the same land area with 2 years of crops identical to CC and SR followed by 4 years of grass–legume pastures composed of tall fescue, birdsfoot trefoil, and white clover. The forage rotation system (FR, 24 ha) is seeded with tall fescue and does not rotate with grain crops.
Each pasture-crop rotation (CC, SR and LR) was split into two halves: one half for grain production (defined as ‘crop area’), which were seeded with oats (Avena byzantina L.), black oat (Avena strigose Schreb.), and wheat (Triticum aestivum L.) in winter, and soybean (Glycine max L.) and sorghum (Sorghum bicolor L.) in summer. The remaining area was oriented to grazing animals (livestock component, defined as ‘grazing area’) which were seeded as follows: Italian ryegrass and oats in winter and sorghum and moha (Setaria italica) in summer. Winter crops and pastures were sown from March to June and were usually harvested in November. Summer crops were sown from October to November and harvested in April. Cover crops (black oat) were harvested for hay in October.
As part of the experimental platform redesign implemented in 2019 (Rovira et al., 2020), each system included a bespoke livestock strategy. In CC, SR, and LR, animals enter their respective experimental paddocks in April–May each year and remain for 1 year (rearing animals) or, in the case of finishing animals, until reaching target weights to the slaughterhouse. In FR, animals enter in November–December each year. British early-maturing beef cattle were used in the four systems (Hereford, Aberdeen Angus, and Hereford–Angus cross). Details of the livestock strategy for each system and the initial live weight are provided in Table 2.
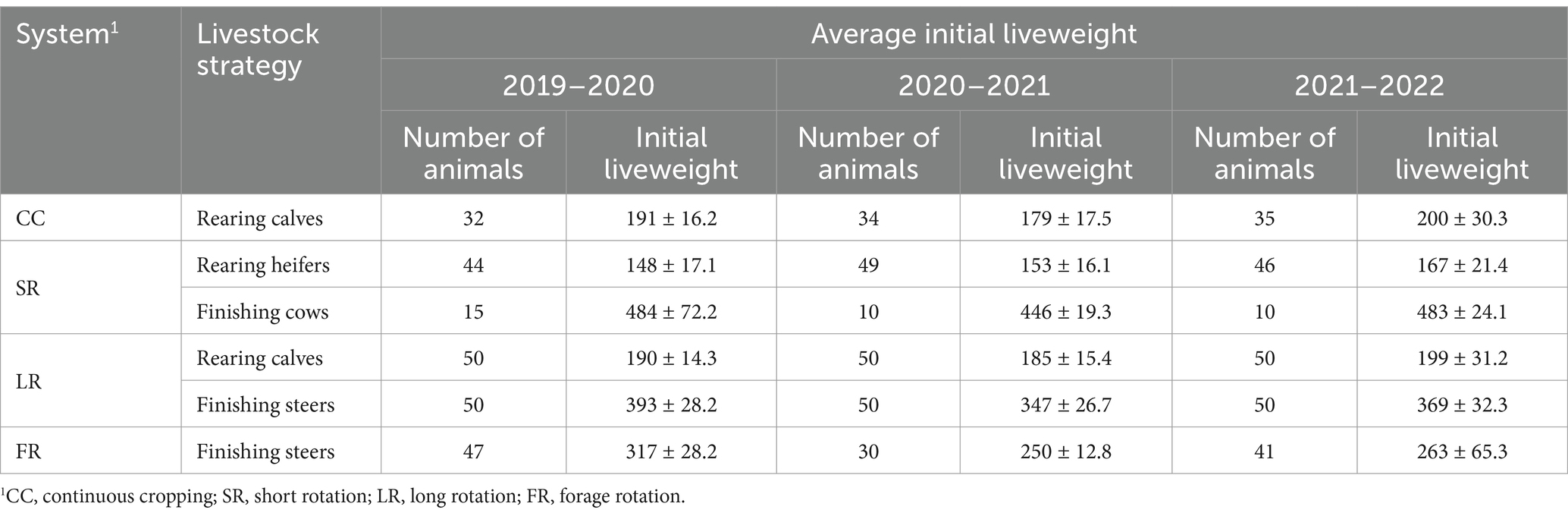
Table 2. Number of animals and average initial liveweight at ‘Palo a Pique’ long-term experiment, Treinta y Tres, Uruguay (Pereyra-Goday et al., 2022).
The experiment lacks synchronic replications, but all phases of the rotations are present each year, represented by paddocks of 3 ha in CC, SR, and LR. In FR, the 24 ha were divided into five paddocks of 4.8 ha each corresponding to fescue seeded in 2013 (9.6 ha), 2014 (9.6 ha), and 2020 (4.8 ha). Each system has a support area of natural grassland (NG) to handle the animals, when necessary (i.e., during periods with low forage availability in the seeded area), keeping the animals independently within each system. The proportion of NG surface is 33, 29, 26, and 33% of the total area for CC, SR, LR, and FR, respectively. Animals were handled to graze annual forage crops (ryegrass, oat, sorghum, and moha), permanent pastures (in grazing and crop area of SR and LR), permanent improved pasture (in CC), and natural grasslands. Detailed information about experimental design, management, and productive performance can be found in a study by Pereyra-Goday et al. (2022) and Rovira et al. (2020).
2.3 Data analysis and scope of the study
N balance (NBAL) was calculated as N inputs minus N outputs and NUE was calculated from food N outputs relative to all N inputs, according to Erisman et al. (2018). NSURP was calculated as all the N inputs minus N removed in food products (EU Nitrogen Expert Panel, 2015). We assessed NBAL, NUE, and NSURP at component level (crop and livestock) and system level. The study boundary was the farm gate. Crop component was defined as the area where exclusively grain was produced (6 ha in each system), and pasture phase was not included in the crop component. Livestock component included the total grazing area (permanent pastures, annual pastures, permanent improved, and NG) which was 18 ha in CC, 22 ha in SR, 50 ha in LR, and 36 ha in FR. For all systems, N inputs considered were synthetic fertilizers (diammonium phosphate and urea), biological N fixation (pasture legumes), atmospheric N deposition, estimated using annual precipitation data from the experimental site and rain N concentrations from Zunckel et al. (2003), and N in animal feed. Outputs included all N removed in food products and N losses (Figure 1). As atmospheric and leached N losses (N₂O, N₂, NH₃ gas, and leached NO₃−) were not directly measured, they were simulated using the DNDC model (Li et al., 2000). In this model, losses are estimated through mechanistic equations based on physical chemistry and microbial kinetics. Ammonia volatilization is modeled using a two-film approach, with emission rates governed by temperature-dependent chemical equilibria and concentration gradients across the soil–atmosphere interface, primarily driven by soil pH, temperature, and NH₄+ concentration. Denitrification is triggered when soil redox potential (Eh) falls below 500 mV, as calculated by the Nernst equation, and occurs under anaerobic conditions where nitrate and labile carbon are available, with N reduced sequentially from NO₃− to NO₂−, NO, N₂O, and finally N₂. Leaching of NO₃− is estimated based on soil water balance and vertical flow processes driven by precipitation, irrigation, and soil hydrological properties. Model calibration was performed by adjusting site-specific parameters (e.g., soil organic carbon, pH, texture, and management practices) to align simulated outputs with observed N fluxes and crop yield data (EOS, 2017). The DNDC is a widely applied, process-based simulation tool designed to represent soil carbon and N biogeochemical dynamics, particularly in agroecosystems. Initially developed to estimate greenhouse gas emissions from agricultural lands in the United States, the model has since been calibrated and implemented in diverse regions worldwide (Kesik et al., 2005; Abdalla et al., 2022). For this study, we utilized calibrated and validated coefficients from a previous study in the same region and climate, involving comparable crop-pasture-livestock rotations, which demonstrated good agreement between predicted and observed values for pasture and crop productivity as well as soil N dynamics (Castillo et al., 2023).
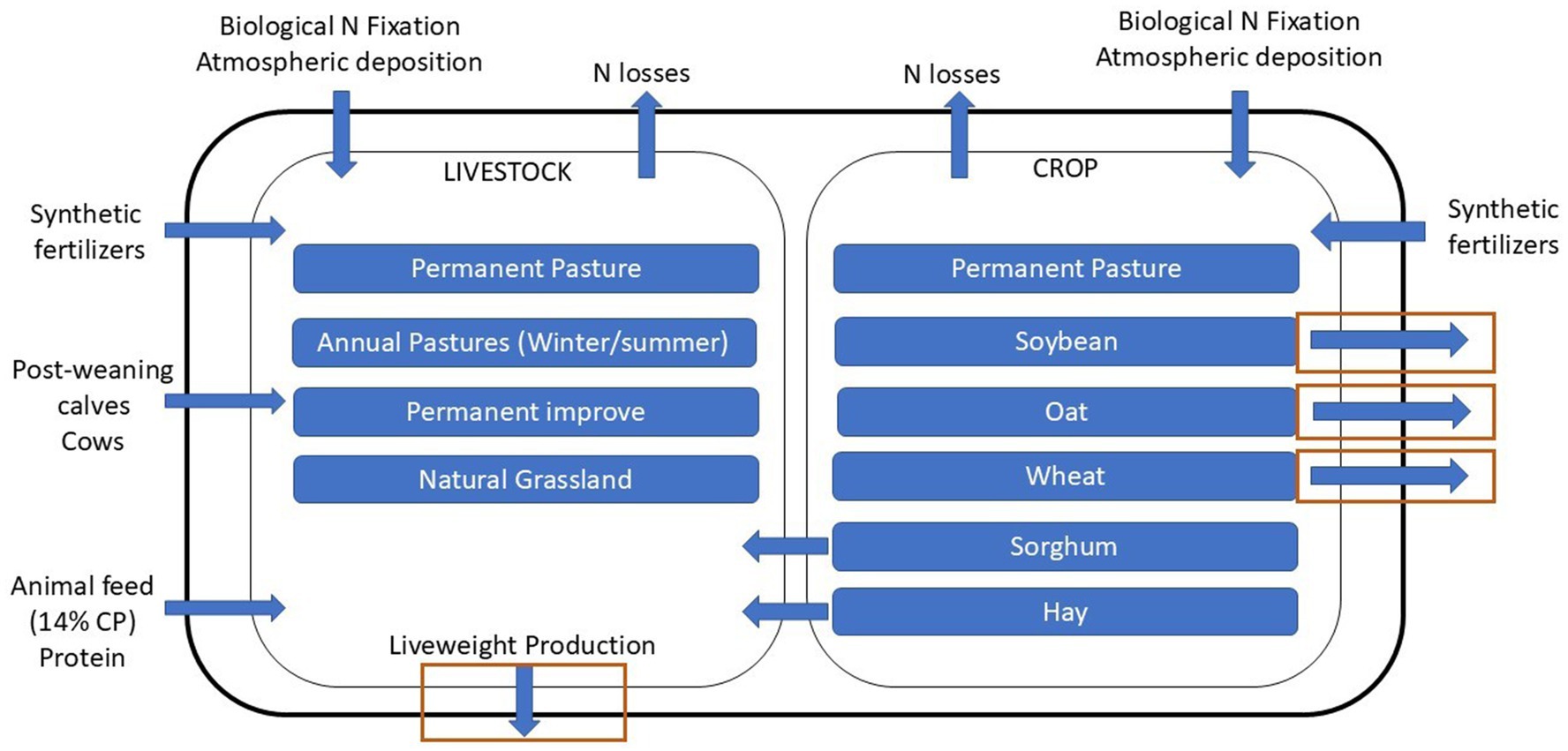
Figure 1. Scheme of N fluxes in pasture-crop rotation at ‘Palo a Pique’ long-term experiment in Treinta y Tres, Uruguay.
N exported from the crop component in grains (soybean, oat, and wheat) was estimated using the Dumas method (dry combustion) (Jung et al., 2003). The values were 2.1% N content in wheat, 5.8% N content in soybean, and 1.7% N content in oat. Crop straw was left in the field. N exported by cattle was estimated as 2.6% of empty body weight, which was assumed to be 90% of live weight (FAO, 2018). N fixation by pasture legumes was estimated based on Peoples et al. (1995), while N fixation by soybean was estimated according to Salvagiotti et al. (2015).
A low (≥50%) and high (≤90%) threshold was set up to evaluate NUE, according to EU Nitrogen Expert Panel (2015), and for the livestock component, the threshold was ≥10% and ≤25%, according to Gerber et al. (2014). For the crop component, NUE values greater than 90% could be associated with soil N mining, and values below 50% could be associated with low NUE. Animal systems, on the other hand, reach a lower NUE; therefore, both efficiency thresholds were set accordingly. For the whole system, we propose a low (<18%) and high (>45%) threshold to evaluate NUE, according to a prorated average taking into consideration the crop and the livestock area in the experiment. The maximum NSURP was defined at 80 kg N ha−1 year−1 for crop component and 110 kg N ha−1 year−1 for livestock component following EU Nitrogen Expert Panel (2015), whereas the system NSURP was defined at 104 kg N ha−1 year−1. For the livestock component, the minimum N desirable productivity was set at 10.4 kg N ha−1 year−1 according to an experimental hypothesis of 400 kg LW production ha−1 year−1 (Pereyra-Goday et al., 2022). For the crop component, the threshold was defined as 80 kg N ha−1 year−1, following EU Nitrogen Expert Panel (2015). The effect of components on NUE and NSURP was tested using the least significant difference (LSD) model in InfoStat statistical software (Di Rienzo et al., 2020), with year treated as a replicate.
3 Results
3.1 N inputs and N outputs
N inputs and outputs are presented in Tables 3, 4. The primary input in the crop component was biological N fixation from soybean, while the primary input in the livestock component was N fertilization (diammonium phosphate and urea). Biological N fixation in the livestock component was derived from legumes seeded in permanent pastures. Although ‘complementary outputs’ were not part of the NUE calculations, they were accounted as a transference from crop to the livestock component (e.g., hay and grain to feed animals). Differences in ‘feed production’ for animals and ‘feed to animals’ value in crop vs. livestock component are explained by differences in area considered and the inclusion of commercial feed. Animal deposition (feces and urine) was not considered for the calculation given that the grazing periods in the pastures of the crop phase were short and far from the beginning of cultivation period.
3.2 N losses
On average, N losses accounted for 38 ± 3.1, 22 ± 2.6, and 24 ± 1.1 kg N ha−1 year−1 for CC, SR, and LR, respectively, in the crop component. Approximately 71% of total N losses occurred during winter cash crops, and the remaining occurred during summer cash crops. The main N loss source in this component was volatilization (45%) followed by lixiviation (35%).
In the livestock component, N losses were 32 ± 4.0, 40 ± 4.3, 18 ± 0.9, and 52 ± 3.1 kg N ha−1 year−1 for CC, SR, LR, and FR, respectively. The highest values of N losses were observed in tall fescue in FR (81 kg N ha−1 year−1), which had inputs of 184 kg N ha−1 year−1 as fertilizer in tall fescue area. In this rotation, the main gas losses were volatilization (56%). In natural grassland (NG), N losses were 9.6 kg N ha−1 year−1 when grazing was included, whereas N losses dropped up to 3.6 kg N ha−1 year−1 when grazing was excluded. On average, N losses associated with the permanence of grazing animals in the experiment accounted for 29, 31, 29, and 26% of the total N losses to CC, SR, LR, and FR, respectively.
3.3 N balance
Nitrogen balance at crop component was 2.2 ± 9.21, −14.9 ± 20.43, and −3.5 ± 12.88 kg N ha−1 year−1 for CC, SR, and LR, respectively. Figure 2 shows N balance at crop component level. Considering all N inputs, fertilizers represented on average 38, 30.9, and 30.1%, whereas biological N fixation (BNF) from soybean accounted for 55.1, 61.8, and 63.3% for CC, SR, and LR, respectively. The remaining input was atmospheric deposition, being 6.9, 7.3, and 6.6% for CC, SR, and LR, respectively.
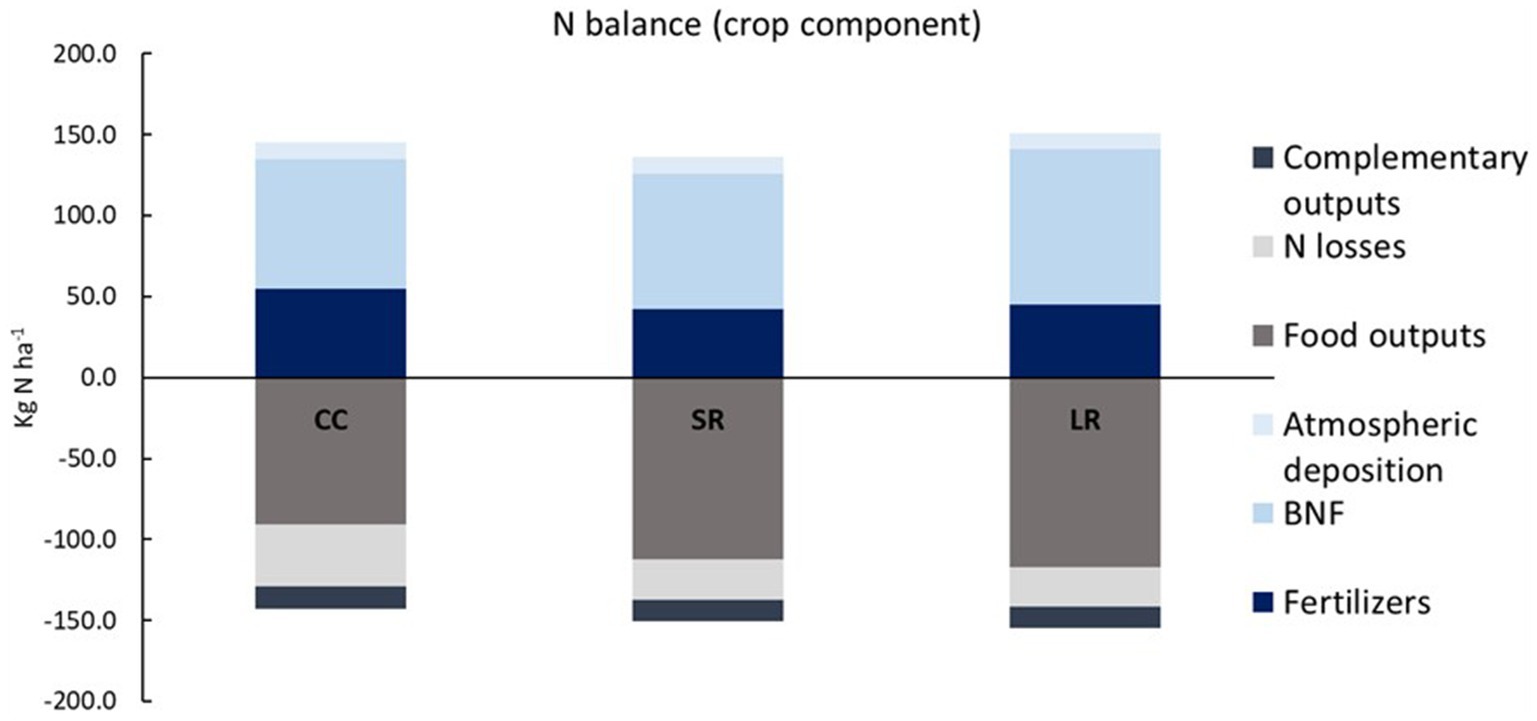
Figure 2. Components of N balance of crop component at ‘Palo a Pique’ long-term experiment. BNF: biological N fixation.
Figure 3 shows NBAL for livestock component, including rotation area and NG area. For the experimental period (2019–2022), NBAL was 6.1 ± 4.42, 59 ± 4.3, 29 ± 1.2, and 64 ± 3.5 kg N ha−1 year−1, for CC, SR, LR, and FR, respectively. In this component and for all N inputs, synthetic fertilizers accounted on average, for 64.3, 53.3, 44.3, and 90.5%, external feed represented 19.6, 18.9, 30.2, and 5.6%, and BNF was 5.8, 23.4, 17.3, and 0%, for CC, SR, LR, and FR, respectively. The remaining percentage was explained by atmospheric deposition, being 10.2, 4.5, 8.2, and 4% for CC, SR, LR, and FR, respectively.
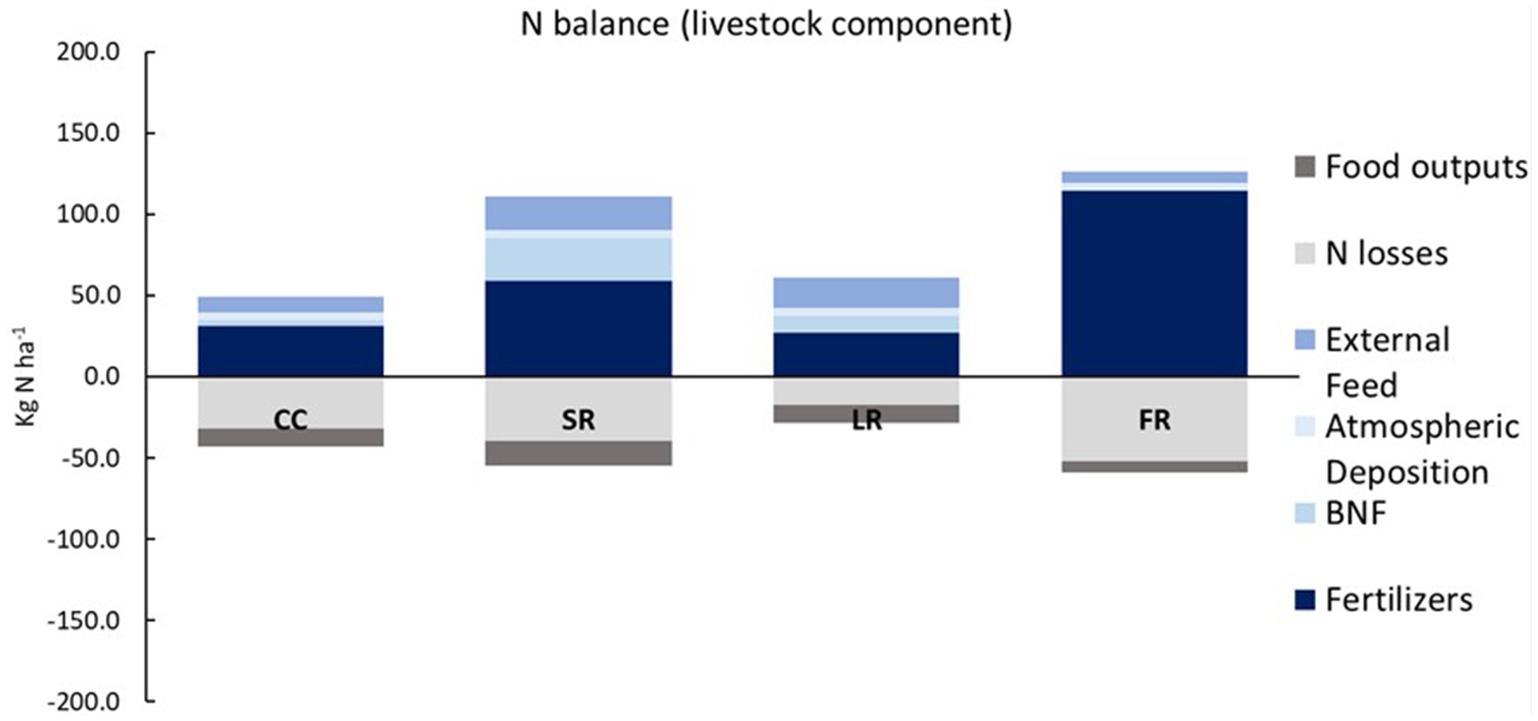
Figure 3. Components of N balance of livestock component at ‘Palo a Pique’ long-term experiment. BNF: biological N fixation.
Given that crop and livestock components are part of a single integrated system that combines agricultural and livestock production, a whole system balance was calculated (Figure 4). N balance was positive in all cases reaching 5 ± 1.5, 41 ± 3.1, 29 ± 2.2, and 64 ± 3.5 kg N ha−1 year−1 for CC, SR, LR, and FR, respectively.
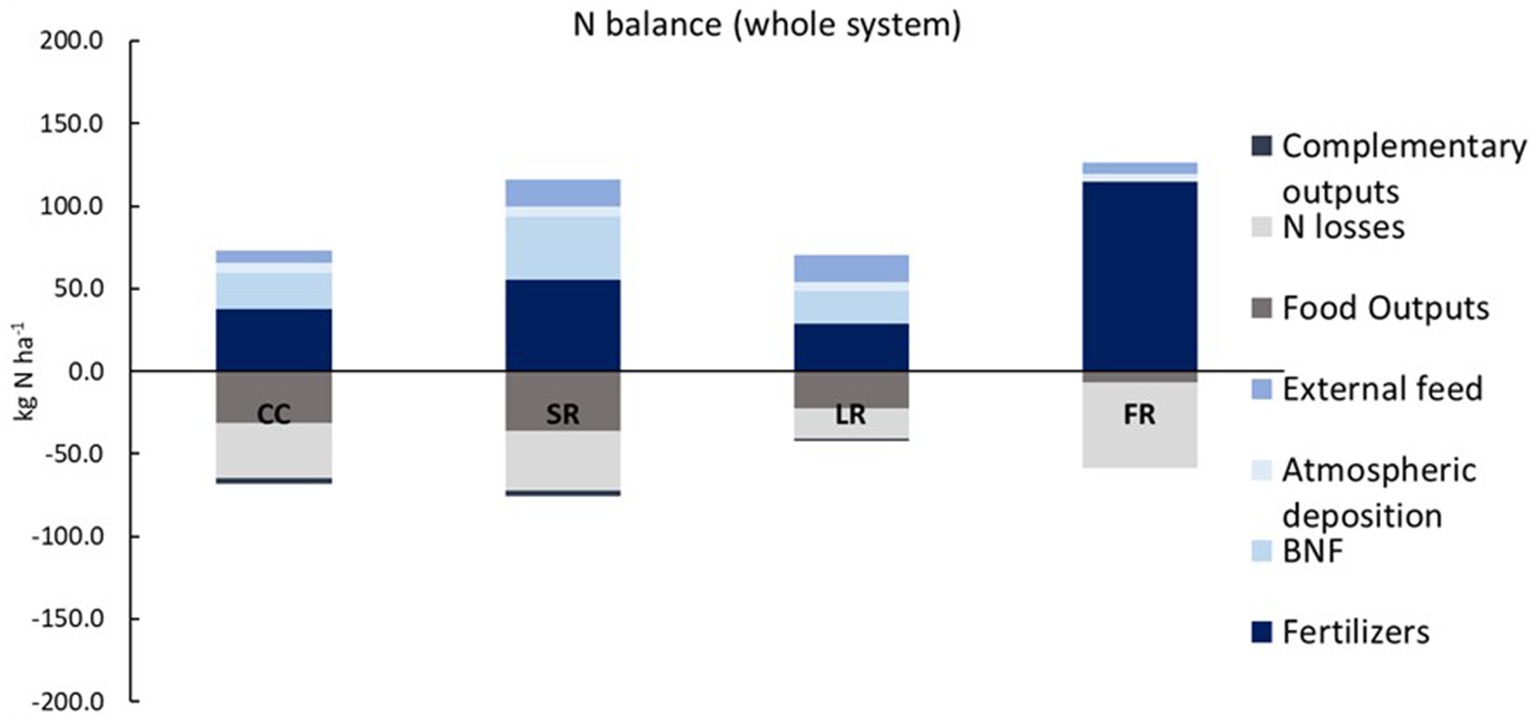
Figure 4. Components of N balance (whole system) at ‘Palo a Pique’ long-term experiment. BNF: biological N fixation.
3.4 Soil N
Soil N concentration (0–15 cm) for each pasture-crop rotation (CC, SR, LR, and FR) and component (crop from 2013 to 2021 and livestock from 2006 to 2021) ranged between 0.142 g kg−1 (CC) and 0.208 g kg−1 (LR) in the crop area, whereas in the grazing area (livestock component) values ranged between 0.132 g kg−1 (CC) and 0.211 g kg−1 (FR). For the entire historical data series, the increase of N content in crop component was on average 0.023 g kg−1 and differences were not significant among CC, SR, and LR. However, systems that included pastures in their rotation had higher values of N content in soils than the CC. For the livestock component, the increase in soil N content (2006–2021) was 0.024 g kg−1, and differences were also not significant among SR, LR, and FR.
An upward trend for all systems and components was observed when comparing 2019 vs. 2021. Soil N content increased by 4.3, 4.4, and 3.1% per year in the crop component in LR, SR, and CC, respectively, with differences among systems (p = 0.015). For the livestock component, soil N content increased by 6.8, 3.6, 6.9, and 8.7% on average in LR, SR, CC, and FR, respectively. Differences were not detected among systems.
3.5 Crop NUE and NSURP
On average, crop NUE (NUEC) values did not differ (p = 0.07) among systems, accounting for 67 ± 8.2, 84 ± 12.5, and 78 ± 5.8% for CC, SR, and LR, respectively, meaning that the achieved NUEC values were within the defined target NUE zone (Figure 5). The highest variability among years was observed for N inputs in SR, whereas the lowest variability was observed in N outputs in the same system. Highest NUE corresponded to SR, reaching 94%, and the lowest NUE was observed in CC (53.3%). The NSURP (kg N ha−1 year−1) was 54 ± 10.6, 23 ± 18.5, and 34 ± 6.2 kg N ha−1 year−1, for CC, SR, and LR, respectively, where all the calculated values were below the defined threshold (80 kg N ha−1 year−1).
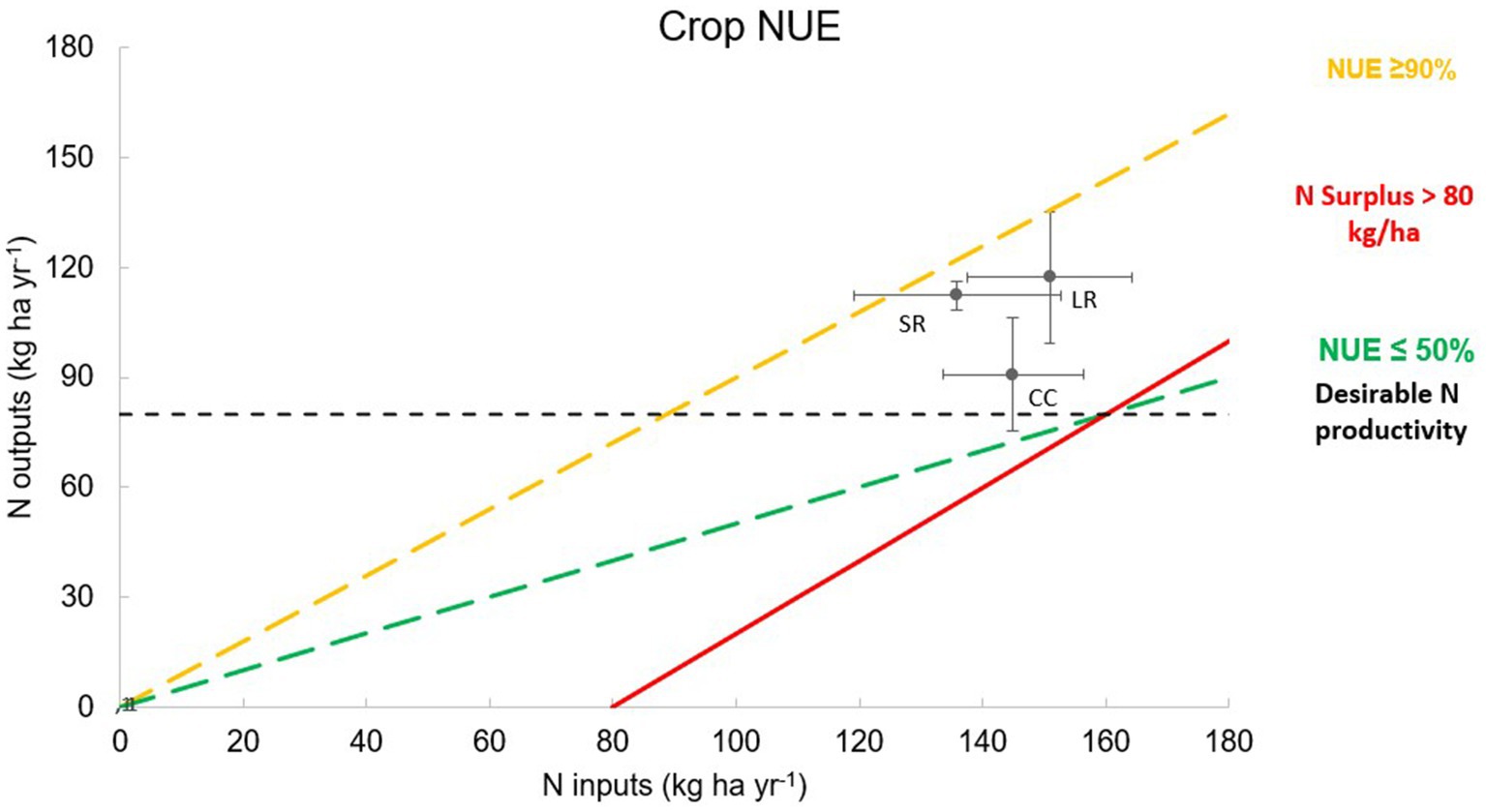
Figure 5. Average N inputs and outputs (2019–2022) for the crop component. Dashed yellow and green lines represent NUE (outputs/inputs × 100) of 90 and 50%, respectively, for crops. The dashed black line indicates the expected N output for a desirable production level. The red line represents an N surplus (80 kg N ha−1). Theoretical productivity was maintained due to the low crop yield potential in the study area, consistent with Terra et al. (2006).
3.6 Livestock NUE and NSURP
The livestock NUE (NUEL) showed differences among systems of which CC was 5-, 3-, and 1-fold greater than FR, SR, and LR, respectively. NUEL was 24.4 ± 4.14, 9.9 ± 1.23, 14.3 ± 1.92, and 5.5 ± 0.73 to CC, SR, LR, and FR, respectively. Differences were detected between FR and LR, FR and CC, SR and CC, and LR and CC (p = 0.0001). The pasture length did not influence the achieved NUEL when analyzing both SR and LR, being both closer to FR than CC.
Once N inputs were plotted against N outputs, differences in NUEL and the deviation values for this parameter among systems were observed. Except for FR and SR, the remaining systems predominately achieved NUE values between the defined thresholds, as well as reached the defined minimum N productivity (10.4 kg N ha−1 yr−1) (Figure 6). The NSURP was 37 ± 10.1, 50 ± 2.3, 100 ± 11.4, and 116 ± 5.4 kg N ha−1 year−1 for CC, LR, SR, and FR, respectively. Values for SR and FR approached or exceeded the defined threshold.
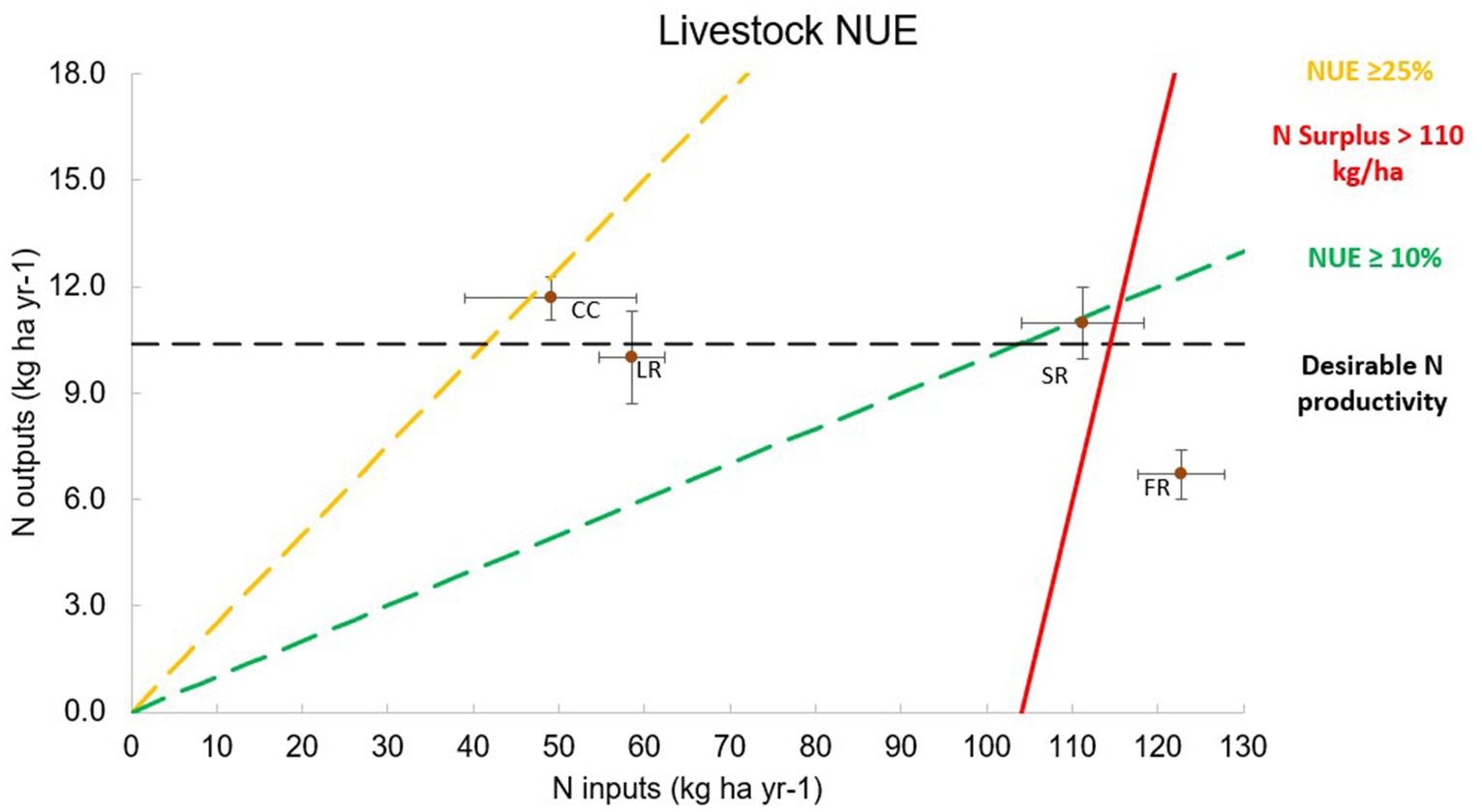
Figure 6. Average N inputs and outputs (2019–2022) for the livestock component. Dashed yellow and green lines represent NUE (outputs/inputs × 100) of 25 and 10%, respectively, for livestock. The dashed black line indicates the expected N output for a desirable production level (10.4 kg N ha−1 year−1 = 400 kg LWG ha−1 year−1). The red line represents an N surplus (110 kg N ha−1).
3.7 System NUE and NSURP
At system level (Figure 7), NUE was different between CC, SR, and LR (p < 0.0001). NUE values were 43.4 ± 5.93, 28.1 ± 2.03, 29.3 ± 3.83, and 5.5 ± 0.73 for CC, SR, LR, and FR, respectively. N surpluses at the system level were 42 ± 8.8, 84 ± 6.9, and 48 ± 2.2 kg N ha−1 year−1 for CC, SR, and LR, respectively.
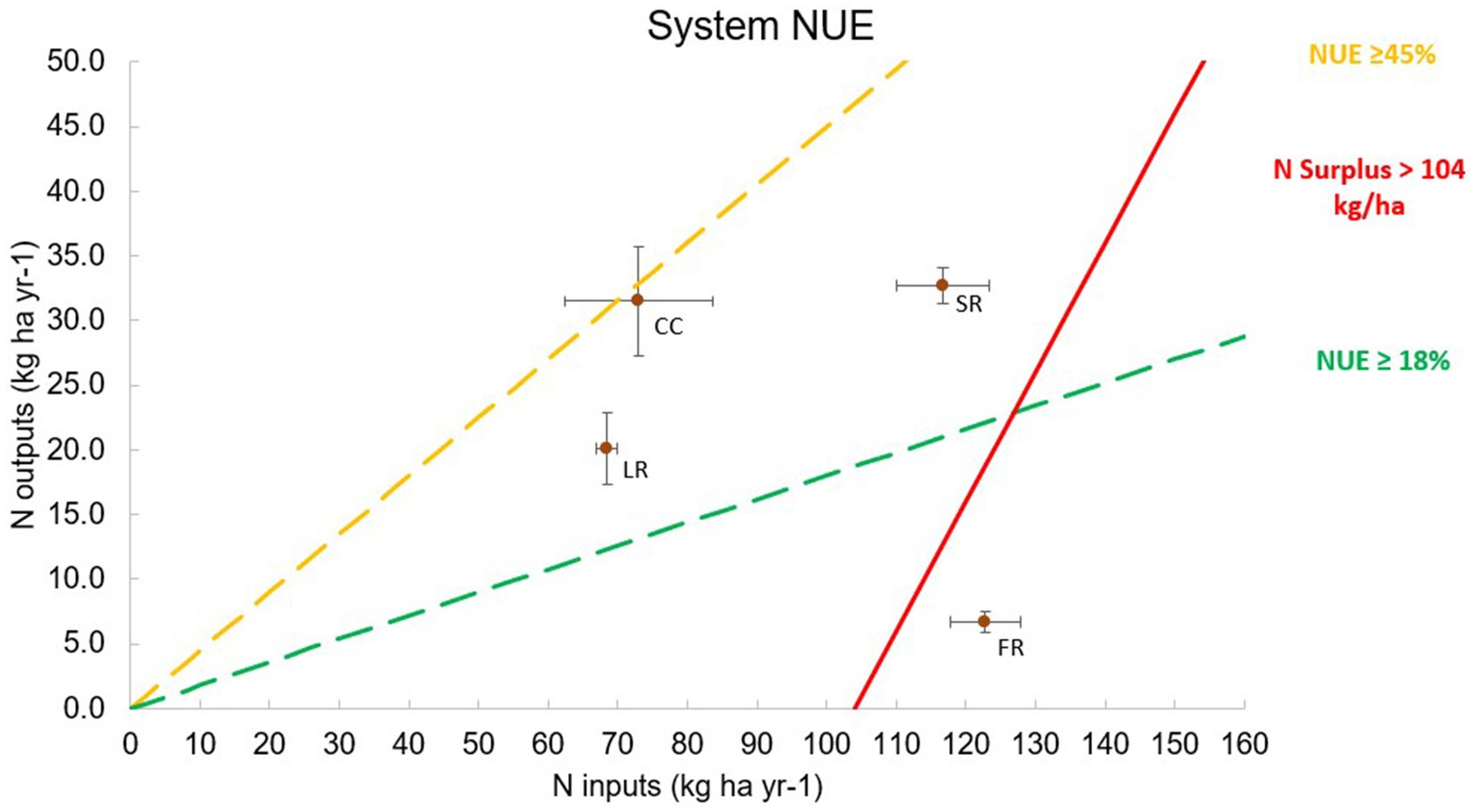
Figure 7. Average N inputs and outputs (2019–2022) for the crop-livestock system. Dashed yellow and green lines represent NUE (outputs/inputs × 100) of 45 and 18%, respectively, for the system. The red line represents an N surplus (104 kg N ha−1).
4 Discussion
The upward worldwide trend in N consumption shows the dependence agricultural systems have for that nutrient (Tilman et al., 2002). Mixed crop-livestock systems play a crucial role in the production of high-quality food and in system sustainability, which is gaining interest in recent years (Ryschawy et al., 2012). Given the current dependence of these systems on N fertilizers, understanding the dynamics and processes associated with this nutrient, as well as management strategies aimed at improving the efficiency of use and reducing losses is critical. This is especially true for these systems where N inputs of synthetic fertilizer could be optimized through the strategic use of organic inputs from livestock via rotation. The inclusion of NUE calculations and N balance at farm level allows the interaction between crops and livestock to be investigated to help improve resource use and reduce environmental losses at a system level. As stated by Oliveira et al. (2022), NUE is an indicator of sustainability, and the evolution of this indicator is crucial to evaluate the intervention implemented in long-term experiments and, at the same time, provide information to farmers and policymakers with respect to sustainable food production systems and the use of input resources. In general, this type of NUE assessment is conducted at the system level and is not necessarily accompanied by direct validation of the results.
4.1 NUE of crop component
For all crop rotations, NUEC was within the defined thresholds. Differences observed in NUEC could be explained by differences in crop yield and fertilization strategies in each year (e.g., CC had lower crop yields as was stated in a previous study by Pereyra-Goday et al., 2022). NUEC values reported by other studies had high variability; Hutchings et al. (2020) reported NUEC values of 65–92% for arable crops in Northern and Southern Europe, Shen et al. (2023) found NUEC values of 49% for wheat production in China, while Gu et al. (2017) reported NUEC values of 39% for croplands in China. According to EU Nitrogen Expert Panel (2015), the reported values in this study for SR and LR fell in the ‘balanced N fertilization’ category, whereas CC seemed to indicate a ‘risk of N losses’, which is aligned with the higher value of N losses estimated in CC compared to SR and LR. On the other hand, N balance tended to be neutral with variability between years. For CC, we observed higher N losses compared to the literature which could be attributed to the source of N used and the way it was applied (urea without inhibitors, surface broadcast without incorporation into the soil), which is consistent with the N internal fluxes reported by Pravia et al. (2019).
4.2 NUE of livestock component
The livestock component of the four systems had greater productivity values than the average for rearing and fattening systems (200 kg LWG ha−1 year−1; Plan Agropecuario, 2021). However, the evaluated systems incorporate external sources of N (synthetic fertilizers and external feed), which determined medium-to-low NUE values. For the livestock component, NUEL values were lower than NUEC, due to biological differences at the trophic level (Godinot et al., 2015). Organic sources offer a promising alternative to synthetic fertilizers, enabling a reduction in their use within such systems, as discussed later.
CC presented the highest value of NUEL, close to the upper threshold, explained by the highest productivity in terms of kg LWG ha−1 year−1 (426 kg ha−1 year−1) due to the higher efficiency of rearing male calves (CSIRO, 2007). LR and SR had similar NUEL values, even though SR had higher values of productivity than LR (418 kg LWG ha−1 year−1 in SR vs. 369 kg LWG ha−1 year−1 in LR). This could be explained by the higher productivity of red clover in SR, which accounted for 31 ± 12.3% of total dry matter (DM) production of permanent pastures. Therefore, there was high values of biological N fixation, with high variability among years and N fertilization. The potential of red clover to increase performance in ruminant livestock systems has been previously reported in relation to an enzyme system (polyphenol oxidase) in the forage which reduces protein breakdown in the rumen and subsequently N retention by the animal (Lee, 2014).
The system with the lowest NUEL was FR, which was below the lower reference threshold. This was explained by high N inputs (184 kg N ha−1 year−1 as synthetic fertilizer in tall fescue area) and a lower productivity (310 kg LWG ha−1 year−1), since this system is focused on finishing animals to slaughterhouse, which is a low-efficiency process compared to the other livestock categories. Although DM production was high (6867 kg DM ha−1 year−1), conversion efficiency and forage utilization were low in this system (19.2 kg DM kg LWG−1 and 37% utilization, respectively). In addition, gas N losses were highest in this system associated with the greater amounts of N fertilizer applied. The potential impact of replacing synthetic fertilizer with organic manures and increasing forage utilization are discussed below (Section 4.3).
NUEL values were higher than those reported by Jin et al. (2021) for Chinese livestock systems (3–4%), while Castillo et al. (2021) reported similar values (13.2%) for extensive livestock systems in rotation with rice in Uruguay. Although the latter value was close to that of our study, the LWG differed considerably in favor of our study (+400%), mainly due to the livestock intensification level (extensive vs. intensive, respectively).
The positive N balance observed at the livestock component in the four systems agrees with Ryschawy et al. (2012). A positive balance could induce lower demand for N inputs in livestock compared with crop production (Oliveira et al., 2022; Powell and Rotz, 2015), given that grazing animals contribute positively with soil N content through manure and urine deposited in the field (Segura et al., 2023). Furthermore, livestock production plays a critical role in food security, by supplying high-quality, nutrient-dense food (Rivero et al., 2021).
Livestock in mixed crop-livestock systems play an important role in non-arable areas, where cropping is unsustainable (Wilkinson and Lee, 2018), using non-human edible crops/pastures or crop residues as feed (Sekaran et al., 2021). As was summarized by de Faccio Carvalho et al. (2021), livestock grazing provides several benefits such as system stability, resilience, profitability, soil health (more microbial activity), and biomass production. This broader perspective on sustainability contributes to the concept of circularity in livestock production (Lemaire et al., 2023; Ward et al., 2016), where livestock play a beneficial role in nutrient cycling and ecosystem services. Thus, maintaining soil fertility in agroecosystems could outweigh the adverse effects of a higher carbon footprint (driven by biogenic methane from enteric fermentation).
4.3 System NUE and prospects for improvement
Based on the findings of this study, we can categorize the assessed systems according to their results. Systems incorporating pasture in rotation with crops (SR and LR) consistently demonstrated, on average, lower N losses, higher soil N gains, and higher efficiency values within the optimal desirable range. Conversely, rotation excluding pastures (CC) led to greater N losses across both crop and livestock components, along with suboptimal efficiencies in the crop component but higher efficiency in the livestock component (attributable to the specific livestock management approach). On the other hand, rotation exclusively comprising pastures (FR) with substantial N synthetic fertilizer inputs showed the highest estimated N losses and notably low NUE, thereby raising significant concerns regarding potential environmental risks. Organic sources could replace synthetic fertilizers in such systems.
Although the four systems exhibited soil N gains during the experimental period, this observation must be put into perspective. It is important to note that a period of N accumulation in the soil is often followed by a subsequent decline. This pattern is frequently observed in annual or cash crops that are rotated with pastures (Grahmann et al., 2020). These increases in N stored in soil during the experimental period represented 26.3, 18.3, 30.1, and 41.4 kg N ha−1 year−1 for CC, SR, LR, and FR, respectively, which is close to the results obtained in the balance. Although the aim of this study was not to formally validate indicators such as NUE, NBAL, or NSURP, partial validation was observed through the consistency between soil N gains and the corresponding positive N balances and surpluses in systems with lower estimated losses. This coherence reinforced the utility of these indicators for evaluating nutrient dynamics at the system scale.
To improve all the assessed N parameters in this study, we believe it is possible to consider system-level improvements with a focus on the livestock component. This is because the livestock component offers greater opportunities for improvement, as stated by Castillo et al. (2023), through agronomy management to achieve a ‘safe operating space’ in terms of NUE. In general terms, greater utilization of pastures, i.e., improving grazing management (mainly in those systems that include perennial pastures), would allow an increase in N output from the system and therefore would have implications for NUE. In addition, the quantification of all N flows, including those derived from animal production (feces and urine), would optimize synthetic inputs to supplement naturally voided organic returns (manures and urine).
Following the EU Nitrogen Expert Panel (2015) approach, to improve NUE in CC system, we must ‘avoid soil degradation’ through improving biomass production, which could have implications for N and C cycles and reduce N losses (Bilotta et al., 2007; de Faccio Carvalho et al., 2010). Redesigning the rotation with the inclusion of high productivity legumes could improve the quality of the diet for animals (Soussana and Lemaire, 2014) and at the same time increase the organic N inputs. Furthermore, an increase in the use of feed supplements could help to improve in terms of growth rates, but it is important to consider the economic implication into the whole system as well as the balance between N and C efficiency.
On the other hand, the SR system should follow an ‘extensification’ strategy, given that it is close to the lower threshold (10%) for the livestock component. An alternative could be considering N fixed by legumes and reducing N fertilization accordingly (Carswell et al., 2022). Complementary, a redesign of the livestock strategy (i.e., exclusively rearing males) could lead to improved productivity. In this system, N inputs cannot be increased due to the risk of increased N losses; however, it is important to consider that these results could be influenced by weather conditions during the experimental period, and probably in the longer term this system would be more aligned with the LR, given the similarities it presents in the composition of the rotation and the combination of livestock strategies (rearing and fattening).
The LR system had on average lower productivity than the desirable level for livestock production. It could therefore be improved through ‘intensification’ of production to reach 400 kg LWG ha−1 year−1. For example, supplementing the reared calves (given their high feed conversion efficiency) could increase live weight gain by 0.010 kg calf−1 day−1, based on annual performance reported in a previous study (Pereyra-Goday et al., 2022). In addition, the grazing management could be altered to optimize higher quality forage intake. Another option could be increasing the proportion of rearing animals in the herd. However, this could have a negative effect on the economic results of the system, given the differences in sales prices between rearing and fattening animals.
Fertilization strategy defined for FR could be revised (e.g., N source, placement, and objective N fertilization method) to maximize tall fescue DM production and fertilization response, reduce N losses, and reduce nitrous oxide (N2O) emissions (Pereyra-Goday et al., 2024) thereby ‘increasing efficiency.’ According to the performance obtained, animals should increase approximately 35% LWG per animal to reach the target of 400 kg LWG ha−1 year−1. Hence, the inclusion of legumes could be beneficial (Soussana and Lemaire, 2014) by improving forage quality, and, at the same time, reducing the cost of production. In addition, grazing management could be adjusted, considering an optimal balance between animal production and soil–plant carbon balance to improve conversion efficiency and utilization of forage (Szymczak et al., 2023). On the other hand, the livestock strategy could be revised (i.e., exclusively finishing animals which would enter the system with higher LW).
Finally, adequately quantifying the N contribution from all components combining with modeling tools will reduce production costs and environmental risks, improve productivity, and move toward more efficient and sustainable crop-livestock systems through optimizing the potential of organic inputs (Castillo et al., 2023).
4.4 Limitations of the study
Given that our study draws information regarding the management and operation of a long-term semi-commercial scale experiment (Rovira et al., 2020), there are limitations in obtaining data for some N flows. For instance, N contributed to the soil by feces and urine (since the animals rotate grazing in different paddocks and natural grassland), and the N contribution from crop residues left in the field, which also help to increase N content in the soil. In addition, the management of N fertilization, which does not differ between years except for rare exceptions, does not allow for the observation of the potential effect of crop residues and the animal component on NUE. As previously explained, these limitations can be addressed with adequate quantification of these flows.
5 Conclusion
By evaluating NUE and N balance values, we gain insights into the underlying processes within mixed crop-livestock systems and can assess strategies for enhancing these indicators. Given the escalating concerns regarding the sustainability of food production processes, our findings contribute to advancing farm-level knowledge through high-resolution data and offer valuable insights for policymakers and farmers alike.
The sustainability of mixed crop-livestock systems hinges on the complementarity and interconnectivity between their components and the appropriate combination of crops and pastures. While both components—crop and livestock—have room for improvement in terms of management and outcomes (as discussed), the greatest potential for enhancement lies within the livestock component, especially when it has the potential to further improve crop production via rotation (soil health). The utilization of software tools for modeling processes within mixed crop-livestock systems warrants adjustments to better serve as a tool for enhancing our understanding of these systems.
The long-term nature of these studies presents an opportunity to explore further into the dynamics, outcomes, and processes involved. Therefore, extending this study over additional years is imperative for gaining comprehensive insights.
Data availability statement
The original contributions presented in the study are included in the article/supplementary material, further inquiries can be directed to the corresponding author.
Author contributions
FP-G: Conceptualization, Formal analysis, Methodology, Writing – original draft, Writing – review & editing. JC: Conceptualization, Formal analysis, Methodology, Writing – original draft, Writing – review & editing. PR: Writing – review & editing. WA: Writing – review & editing. ML: Writing – review & editing. MR: Writing – original draft, Writing – review & editing.
Funding
The author(s) declare that financial support was received for the research and/or publication of this article. This research was funded by Long Term Experimental Platforms project (INIA), FP-G was funded by postgraduate fellowship: National Institute of Agricultural Research doctoral fellowship and National Agency of Research and Innovation (ANII) code MOV_CA_2021_1_171482 (mobility fellowship). Support in writing up the work was greatly received by the Biotechnology and Biological Sciences Research Council (BBSRC) through the institute strategic program Growing Health (BB/X010953/1) at Rothamsted Research. The contributions by MR were also funded by the Natural Environment Research Council (NERC) under research Programme NE/W005050/1 AgZero+: Towards sustainable, climate-neutral farming. AgZero+ is an initiative jointly supported by NERC and BBSRC.
Acknowledgments
We thank INIA’s field and laboratory staff and students who participated in the collection and processing of data. INIA, Rothamsted Research, and Harper Adams University are all members of the Global Farm Platform initiative (www.globalfarmplatform.org) collaboratively working toward sustainable ruminant livestock production systems.
Conflict of interest
The authors declare that the research was conducted in the absence of any commercial or financial relationships that could be construed as a potential conflict of interest.
Generative AI statement
The authors declare that no Gen AI was used in the creation of this manuscript.
Publisher’s note
All claims expressed in this article are solely those of the authors and do not necessarily represent those of their affiliated organizations, or those of the publisher, the editors and the reviewers. Any product that may be evaluated in this article, or claim that may be made by its manufacturer, is not guaranteed or endorsed by the publisher.
References
Abdalla, M., Song, X., Ju, X., and Smith, P. (2022). Evaluation of the DNDC model to estimate soil parameters, crop yield and nitrous oxide emissions for alternative long-term multi-cropping Systems in the North China Plain. Agronomy 12:109. doi: 10.3390/agronomy12010109
Bilotta, G. S., Brazier, R. E., and Haygarth, P. M. (2007). The impacts of grazing animals on the quality of soils. Vegetation Surface Waters Intensively Managed Grasslands 94, 237–280. doi: 10.1016/S0065-2113(06)94006-1
Bowles, T. M., Mooshammer, M., Socolar, Y., Calderón, F., Cavigelli, M. A., Culman, S. W., et al. (2020). Long-term evidence shows that crop-rotation diversification increases agricultural resilience to adverse growing conditions in North America. One Earth 2, 284–293. doi: 10.1016/j.oneear.2020.02.007
Bratti, F., Luiz Locatelli, J., Henrique Ribeiro, R., Renan Besen, M., Dieckow, J., Bayer, C., et al. (2022). Nitrous oxide and methane emissions affected by grazing and nitrogen fertilization in an integrated crop-livestock system. Geoderma 425:116027. doi: 10.1016/j.geoderma.2022.116027
Carswell, A., Sánchez-Rodríguez, A. R., Saunders, K., le Cocq, K., Shaw, R., Cotton, J., et al. (2022). Combining targeted grass traits with red clover improves grassland performance and reduces need for nitrogen fertilisation. Eur. J. Agron. 133:126433. doi: 10.1016/j.eja.2021.126433
Cassman, K. G., and Grassini, P. (2020). A global perspective on sustainable intensification research. Nat. Sustain. 3, 262–268. doi: 10.1038/s41893-020-0507-8
Castillo, J., Kirk, G. J. D., Rivero, M. J., Dobermann, A., and Haefele, S. M. (2021). The nitrogen economy of rice-livestock systems in Uruguay. Glob. Food Sec. 30:100566. doi: 10.1016/j.gfs.2021.100566
Castillo, J., Kirk, G. J. D., Rivero, M. J., and Haefele, S. M. (2023). Regional differences in nitrogen balance and nitrogen use efficiency in the rice–livestock system of Uruguay. Front. Sustain. Food Syst. 7:1104229. doi: 10.3389/fsufs.2023.1104229
de Faccio Carvalho, P. C., Anghinoni, I., de Moraes, A., de Souza, E. D., Sulc, R. M., Lang, C. R., et al. (2010). Managing grazing animals to achieve nutrient cycling and soil improvement in no-till integrated systems. Nutr. Cycl. Agroecosyst. 88, 259–273. doi: 10.1007/s10705-010-9360-x
de Faccio Carvalho, P. C., de Albuquerque Nunes, P. A., Pontes-Prates, A., Szymczak, L. S., de Souza Filho, W., Moojen, F. G., et al. (2021). Reconnecting grazing livestock to crop landscapes: reversing specialization trends to restore landscape multifunctionality. Front. Sustain. Food Syst. 5. doi: 10.3389/fsufs.2021.750765
Di Rienzo, J.A., Casanoves, F., Balzarini, M.G., Gonzalez, L., Tablada, M., and Robledo, C.W. (2020). InfoStat Versión 2020. Centro de Transferencia InfoStat, FCA, Universidad Nacional de Córdoba, Argentina. Available online at: http://www.infostat.com.ar (Accessed July 18, 2022).
DIEA–MGAP (2022). Available at: https://www.gub.uy/ministerio-ganaderia-agricultura-pesca/comunicacion/publicaciones/anuario-estadistico-agropecuario-2022
EOS (2017). DNDC. Scientific basis and processes, vol. 9.5: Institute for the Study of Earth, Oceans, and Space (EOS), New Hampshire. 1–28.
Erisman, J., Leach, A., Bleeker, A., Atwell, B., Cattaneo, L., and Galloway, J. (2018). An integrated approach to a nitrogen use efficiency (NUE) Indicator for the food production–consumption chain. Sustain. For. 10:925. doi: 10.3390/su10040925
EU Nitrogen Expert Panel (2015). Nitrogen use efficiency (NUE) - an indicator for the utilization of nitrogen in agriculture and food systems. Netherlands: Wageningen.
FAO (2018). Nutrient flows and associated environmental impacts in livestock supply chains: Guidelines for assessment (version 1). Rome: FAO.
Fowler, D., Coyle, M., Skiba, U., Sutton, M. A., Cape, J. N., Reis, S., et al. (2013). The global nitrogen cycle in the twenty-first century. Philos. Trans. Royal Soc. B Biol. Sci. 368:20130164. doi: 10.1098/rstb.2013.0164
Franzluebbers, A. J., and Martin, G. (2022). Farming with forages can reconnect crop and livestock operations to enhance circularity and foster ecosystem services. Grass Forage Sci. 77, 270–281. doi: 10.1111/gfs.12592
Garcı́a-Préchac, F., Ernst, O., Siri-Prieto, G., and Terra, J. A. (2004). Integrating no-till into crop–pasture rotations in Uruguay. Soil Tillage Res. 77, 1–13. doi: 10.1016/j.still.2003.12.002
Gerber, P., Uwizeye, A., Schulte, R., Opio, C., and de Boer, I. (2014). Nutrient use efficiency: a valuable approach to benchmark the sustainability of nutrient use in global livestock production? Curr. Opin. Environ. Sustain. 9-10, 122–130. doi: 10.1016/j.cosust.2014.09.007
Godinot, O., Leterme, P., Vertès, F., Faverdin, P., and Carof, M. (2015). Relative nitrogen efficiency, a new indicator to assess crop livestock farming systems. Agron. Sustain. Dev. 35, 857–868. doi: 10.1007/s13593-015-0281-6
Goulding, K., Jarvis, S., and Whitmore, A. (2008). Optimizing nutrient management for farm systems. Philos. Trans. Royal Soc. B Biol. Sci. 363, 667–680. doi: 10.1098/rstb.2007.2177
Grahmann, K., Rubio Dellepiane, V., Terra, J. A., and Quincke, J. A. (2020). Long-term observations in contrasting crop-pasture rotations over half a century: statistical analysis of chemical soil properties and implications for soil sampling frequency. Agric. Ecosyst. Environ. 287:106710. doi: 10.1016/j.agee.2019.106710
Gu, B., Ju, X., Chang, S. X., Ge, Y., and Chang, J. (2017). Nitrogen use efficiencies in Chinese agricultural systems and implications for food security and environmental protection. Reg. Environ. Chang. 17, 1217–1227. doi: 10.1007/s10113-016-1101-5
Haughey, E., Neogi, S., Portugal-Pereira, J., van Diemen, R., and Slade, R. B. (2023). Sustainable intensification and carbon sequestration research in agricultural systems: a systematic review. Environ. Sci. Pol. 143, 14–23. doi: 10.1016/j.envsci.2023.02.018
Hutchings, N. J., Sørensen, P., Cordovil, C. M. d. S., Leip, A., and Amon, B. (2020). Measures to increase the nitrogen use efficiency of European agricultural production. Glob. Food Sec. 26:100381. doi: 10.1016/j.gfs.2020.100381
Jin, X., Zhang, N., Zhao, Z., Bai, Z., and Ma, L. (2021). Nitrogen budgets of contrasting crop-livestock systems in China. Environ. Pollut. 288:117633. doi: 10.1016/j.envpol.2021.117633
Jung, S., Rickert, D. A., Deak, N. A., Aldin, E. D., Recknor, J., Johnson, L. A., et al. (2003). Comparison of kjeldahl and dumas methods for determining protein contents of soybean products. J. Am. Oil Chem. Soc. 80, 1169–1173. doi: 10.1007/S11746-003-0837-3
Keeler, B. L., Gourevitch, J. D., Polasky, S., Isbell, F., Tessum, C. W., Hill, J. D., et al. (2016). The social costs of nitrogen. Sci. Adv. 2:e1600219. doi: 10.1126/sciadv.1600219
Kesik, M., Ambus, P., Baritz, R., Bru ggemann, N., Butterbach-Bahl, K., Damm, M., et al. (2005). Inventory of N2O and NO emissions from European forest soils. Biogeosciences 2, 353–375. doi: 10.5194/bg-2-353-2005
Ladha, J. K., Pathak, H. J., Krupnik, T., Six, J., and van Kessel, C. (2005). Efficiency of fertilizer nitrogen in cereal production: retrospects and prospects. Advan. Agronomy. 87, 85–156. doi: 10.1016/S0065-2113(05)87003-8
Lee, M. R. F. (2014). Forage polyphenol oxidase and ruminant livestock nutrition. Front. Plant Sci. 5:694. doi: 10.3389/fpls.2014.00694
Lemaire, G., Garnier, J., da Silveira Pontes, L., de Faccio Carvalho, P. C., Billen, G., and Simioni Assmann, T. (2023). Domestic herbivores, the crucial trophic level for sustainable agriculture: avenues for reconnecting livestock to cropping systems. Agronomy 13:982. doi: 10.3390/agronomy13040982
Li, C., Aber, J., Stange, F., Butterbach-Bahl, K., and Papen, H. (2000). A process-oriented model of N2O and NO emissions from forest soils: 1. Model development. J. Geophys. Res. 105, 4369–4384. doi: 10.1029/1999JD900949
Löw, P., Nadi Karatay, Y., and Osterburg, B. (2020). Erratum: nitrogen use efficiency on dairy farms with different grazing systems in northwestern Germany (2020 environ. Res. Commun. 2 105002). Environ. Res. Commun. 2:119601. doi: 10.1088/2515-7620/abccbc
Ma, L., Velthof, G. L., Wang, F. H., Qin, W., Zhang, W. F., Liu, Z., et al. (2012). Nitrogen and phosphorus use efficiencies and losses in the food chain in China at regional scales in 1980 and 2005. Sci. Total Environ. 434, 51–61. doi: 10.1016/j.scitotenv.2012.03.028
MGAP (2020). Normativa de Suelos y Aguas. Available online at: https://www.gub.uy/ministerio-ganaderia-agricultura-pesca/politicas-y-gestion/normativa-suelos-aguas.
Milroy, S. P., Wang, P., and Sadras, V. O. (2019). Defining upper limits of nitrogen uptake and nitrogen use efficiency of potato in response to crop N supply. Field Crop Res. 239, 38–46. doi: 10.1016/j.fcr.2019.05.011
Oliveira, J. G., Luiz Santana Júnior, M., Jaqueline Costa Maia, N., Batista Dubeux Junior, J. C., Hauber Gameiro, A., Kunrath, T. R., et al. (2022). Nitrogen balance and efficiency as indicators for monitoring the proper use of fertilizers in agricultural and livestock systems. Sci. Rep. 12:12021. doi: 10.1038/s41598-022-15615-7
Paruelo, J. M., and Sierra, M. (2023). Sustainable intensification and ecosystem services: how to connect them in agricultural systems of southern South America. J. Environ. Stud. Sci. 13, 198–206. doi: 10.1007/s13412-022-00791-9
Peoples, M. B., Herridge, D. F., and Ladha, J. K. (1995). Biological nitrogen fixation: an efficient source of nitrogen for sustainable agricultural production? Plant Soil 174, 3–28. doi: 10.1007/BF00032239
Pereyra-Goday, F., Jebari, A., Takahashi, T., Rovira, P., Ayala, W., Lee, M. R. F., et al. (2024). Carbon footprint of mixed farming crop-livestock rotational-based grazing beef systems using long term experimental data. Agron. Sustain. Dev. 44:41. doi: 10.1007/s13593-024-00977-1
Pereyra-Goday, F., Rovira, P., Ayala, W., and Rivero, M. J. (2022). Management and productivity of key integrated crop–livestock systems in Uruguay: the Palo a pique long-term experiment’s third phase. Agronomy 12:3023. doi: 10.3390/agronomy12123023
Plan Agropecuario (2021). La invernada en sistemas pastoriles. Montevideo, Uruguay: Una mirada conceptual desde los productores.
Powell, J. M., Gourley, C. J. P., Rotz, C. A., and Weaver, D. M. (2010). Nitrogen use efficiency: a potential performance indicator and policy tool for dairy farms. Environ. Sci. Pol. 13, 217–228. doi: 10.1016/j.envsci.2010.03.007
Powell, J. M., and Rotz, C. A. (2015). Measures of nitrogen use efficiency and nitrogen loss from dairy production systems. J. Environ. Qual. 44, 336–344. doi: 10.2134/jeq2014.07.0299
Pravia, M. V., Kemanian, A. R., Terra, J. A., Shi, Y., Macedo, I., and Goslee, S. (2019). Soil carbon saturation, productivity, and carbon and nitrogen cycling in crop-pasture rotations. Agric. Syst. 171, 13–22. doi: 10.1016/J.AGSY.2018.11.001
Quemada, M., Lassaletta, L., Jensen, L. S., Godinot, O., Brentrup, F., Buckley, C., et al. (2020). Exploring nitrogen indicators of farm performance among farm types across several European case studies. Agric. Syst. 177:102689. doi: 10.1016/j.agsy.2019.102689
Rawtani, D., Gupta, G., Khatri, N., Rao, P. K., and Hussain, C. M. (2022). Environmental damages due to war in Ukraine: a perspective. Sci. Total Environ. 850:157932. doi: 10.1016/j.scitotenv.2022.157932
Rivero, M. J., Lopez-Villalobos, N., Evans, A., Berndt, A., Cartmill, A., Neal, A. L., et al. (2021). Key traits for ruminant livestock across diverse production systems in the context of climate change: perspectives from a global platform of research farms. Reprod. Fertil. Dev. 33, 1–19. doi: 10.1071/RD20205
Rovira, P., Ayala, W., Terra, J., García-Préchac, F., Harris, P., Lee, M. R. F., et al. (2020). The ‘Palo a pique’ long-term research platform: first 25 years of a crop–livestock experiment in Uruguay. Agronomy 10. doi: 10.3390/agronomy10030441
Ryschawy, J., Choisis, N., Choisis, J. P., Joannon, A., and Gibon, A. (2012). Mixed crop-livestock systems: an economic and environmental-friendly way of farming? Animal 6, 1722–1730. doi: 10.1017/S1751731112000675
Salvagiotti, F., Collino, D. J., Perticari, A., Piccinetti, C., Ovando, G., Urquiaga, S., et al. (2015). El aporte de la fijación biológica de nitrógeno en el cultivo de soja en Argentina : IAH.
Segura, C., Horrocks, C., Lopez-Aizpun, M., Blackwell, M. S. A., Darch, T., Hood, J., et al. (2023). Response of soil health indicators to dung, urine and mineral fertilizer application in temperate pastures. J. Environ. Manag. 330:117096. doi: 10.1016/j.jenvman.2022.117096
Sekaran, U., Lai, L., Ussiri, D. A. N., Kumar, S., and Clay, S. (2021). Role of integrated crop-livestock systems in improving agriculture production and addressing food security – a review. J Agric Food Res. 5:100190. doi: 10.1016/j.jafr.2021.100190
Shen, H., Gao, Y., Sun, K., Gu, Y., and Ma, X. (2023). Effects of differential irrigation and nitrogen reduction replacement on winter wheat yield and water productivity and nitrogen-use efficiency. Agric. Water Manag. 282:108289. doi: 10.1016/j.agwat.2023.108289
Soria-Lopez, A., Garcia-Perez, P., Carpena, M., Garcia-Oliveira, P., Otero, P., Fraga-Corral, M., et al. (2023). Challenges for future food systems: from the green revolution to food supply chains with a special focus on sustainability. Food Front 4, 9–20. doi: 10.1002/fft2.173
Soussana, J.-F., and Lemaire, G. (2014). Coupling carbon and nitrogen cycles for environmentally sustainable intensification of grasslands and crop-livestock systems. Agricul. Ecosys. Environ 190, 9–17. doi: 10.1016/j.agee.2013.10.012
Spiertz, J. H. J. (2010). Nitrogen, sustainable agriculture and food security. A review. Agron Sustain. Dev. 30, 43–55. doi: 10.1051/agro:2008064
Spiller, M., Vingerhoets, R., Vlaeminck, S. E., Wichern, F., and Papangelou, A. (2024). Beyond circularity! Integration of circularity, efficiency, and sufficiency for nutrient management in agri-food systems. Nutr. Cycl. Agroecosyst. 129, 287–297. doi: 10.1007/s10705-024-10339-8
Szymczak, L. S., de Moraes, A., Sulc, R. M., Barker, D., Monteiro, A. L. G., Lang, C. R., et al. (2023). Convergence points of optimal herbage accumulation and intake rate by sheep grazing tall fescue. Grass Forage Sci. 78, 578–589. doi: 10.1111/gfs.12630
Terra, J., García-Préchac, F., Salvo, L., and Hernández, J. (2006). Soil use intensity impact on total and particulate soil organic matter under no till crop pasture rotations under direct grazing. Adv. GeoEcology 38, 233–241.
Tilman, D., Cassman, K. G., Matson, P. A., Naylor, R., and Polasky, S. (2002). Agricultural sustainability and intensive production practices. Nature 418, 671–677. doi: 10.1038/nature01014
Uruguay (2021). Informe Anual de Comercio Exterior. Available at: https://www.uruguayxxi.gub.uy/es/centro-informacion/articulo/informe-anual-de-comercio-exterior-de-uruguay-2021/
Ward, S.M., Holden, N.M., White, E.P., and Oldfield, T.L. (2016). The “circular economy” applied to the agriculture (livestock production) sector-discussion paper. Brussels, Belgium: In proceedings of the workshop on the sustainability of the eu’s livestock production systems. 14–15.
Wilkinson, J. M., and Lee, M. R. F. (2018). Review: use of human-edible animal feeds by ruminant livestock. Animal 12, 1735–1743. doi: 10.1017/S175173111700218X
Wuepper, D., Le Clech, S., Zilberman, D., Mueller, N., and Finger, R. (2020). Countries influence the trade-off between crop yields and nitrogen pollution. Nat. Food 1, 713–719. doi: 10.1038/s43016-020-00185-6
Xia, L., Lam, S. K., Yan, X., and Chen, D. (2017). How does recycling of livestock manure in agroecosystems affect crop productivity, reactive nitrogen losses and soil carbon balance? Environ Sci Technol. 51, 7450–7457. doi: 10.1021/acs.est.6b06470
Xue, H., Mainville, D., You, W., and Nayga, R. M. (2010). Consumer preferences and willingness to pay for grass-fed beef: empirical evidence from in-store experiments. Food Qual. Prefer. 21, 857–866. doi: 10.1016/j.foodqual.2010.05.004
Keywords: sustainability, food security, crop-pasture systems, grazing, nitrogen use efficiency
Citation: Pereyra-Goday F, Castillo J, Rovira P, Ayala W, Lee MRF and Rivero MJ (2025) Nitrogen use efficiency in mixed crop-livestock systems: insights for sustainable intensification. Front. Sustain. Food Syst. 9:1522557. doi: 10.3389/fsufs.2025.1522557
Edited by:
Anindya Chanda, Mycologics LLC, United StatesReviewed by:
Modupe Olufemi Doyeni, Lithuanian Research Centre for Agriculture and Forestry, LithuaniaVenkatesh Paramesh, Indian Council of Agricultural Research (ICAR), India
Copyright © 2025 Pereyra-Goday, Castillo, Rovira, Ayala, Lee and Rivero. This is an open-access article distributed under the terms of the Creative Commons Attribution License (CC BY). The use, distribution or reproduction in other forums is permitted, provided the original author(s) and the copyright owner(s) are credited and that the original publication in this journal is cited, in accordance with accepted academic practice. No use, distribution or reproduction is permitted which does not comply with these terms.
*Correspondence: Fabiana Pereyra-Goday, ZnBlcmV5cmFnb2RheUBnbWFpbC5jb20=