- 1Department of Food Systems and Nutrition, Montana State University, Bozeman, MT, United States
- 2Central Agricultural Research Center, Montana State University, Bozeman, MT, United States
- 3Western SARE, Montana State University, Bozeman, MT, United States
- 4Department of Land Resources and Environmental Sciences, Montana State University, Bozeman, MT, United States
Introduction: One-third of food in the United States is wasted, creating significant environmental and social challenges which anaerobic digestion (AD) can address by converting that waste into a nutrient-rich digestate suitable for use as a biofertilizer. While AD is commonly used in municipal and industrial-scale operations for obtaining biogas, household-scale AD to generate of biofertilizer is a promising on-site option without the infrastructure and process complexities associated with large-scale systems.
Methods: This community-based case study investigated the characteristics of food waste and digestate nutrient content from two household-scale AD designs. Digesters were deployed in 12 households (six households used commercial digesters and six used a smaller custom-built prototype) in Bozeman, Montana, USA. Food waste was collected from households, separated by categories, and nutrient content was determined.
Results and discussion: Over 12 weeks of digester operation, the nutrient content of the digestate was measured. It changed during digester operation with N, P, K, S, pH, and conductivity all increasing (p < 0.05) over time. While food waste C:N ratios varied from 12.1 to 25.7, the C:N ratio of digestate was significantly lower (p < 0.001), with a mean C:N ratio of 6.6. Digestate ammonia levels remained low (< 60 mg l−1) and below inhibitory thresholds during the collection period, but the ratio of NH3-N to total N increased from 0.09 in the food waste to 0.25 in the digestate. Digestate C and N content did not differ between digester models, while different pH (p < 0.05) was observed with a final pH of 6.1 in the commercial small-scale digester and a pH of 5.3 in the custom-built prototype. A survey of participating households revealed that 64% of respondents gained a new awareness of the volume of food waste they generated, and 87% expressed a willingness to recommend biodigesters despite challenges related to convenience and using this technology in a cold climate. Participants perceived the custom-built digester as more user-friendly than the commercial one. This case study highlights the potential of household-scale AD systems to generate biofertilizer, emphasizing the importance of user-friendliness and design tailored to household needs, generated feedstock, and environmental conditions.
1 Introduction
Every year, around 1.3 billion tons of human food go to waste (Kantor and Blazejczyk, 2020), and this amount is expected to increase within the next years (Tian et al., 2021; Wang et al., 2021). At a global scale, food loss across the supply chain is associated with ~8% of global greenhouse gas emissions (Szymkowiak et al., 2022) with high-income countries generating 40%−50% of food waste despite representing only 16% of the global population (Aschemann-Witzel, 2018). Most of this waste is caused by over-purchasing, improper storage, or premature discarding of food. The share of household of total food waste varies from 15% in the Netherlands (Aktas et al., 2018) to 40% in the US (Gunders and Bloom, 2017), where approximately one-third of food is wasted, corresponding to 600 g per person and day (Dalke et al., 2021; US Census Bureau, 2024).
Landfills and incineration are the most widely used methods of food waste disposal, despite adverse environmental effects such as the release of methane, contributing to climate change, and the emission of harmful pollutants (Siddiqua et al., 2022). In the US, where ~40 million households lack any recycling access aligned with their trash service (The Recycling Partnership, 2023), roughly 97% of the generated food waste is transferred to landfills, where it is the largest waste source (Mickan et al., 2022; Mou et al., 2023).
Effective food waste management involves coordinated efforts among governments, NGOs, and public and private institutions to build the necessary human and physical infrastructure. Solutions vary widely in scale and implementation, differing between rural and urban areas or municipal and household levels (Galanakis, 2015; Niles, 2020). Apart from efficiency, sustainability considerations, such as the extent to which byproducts can be generated, impact selecting the most convenient strategy to manage food waste (Bakan et al., 2022). For example, cities usually prioritize centralized food waste management such as large composting units (Bortolotti et al., 2018; Angouria-Tsorochidou et al., 2021). Community waste management is an emerging, less centralized approach, where sites are dispersed throughout a town, city, or neighborhood, including schools, farms, community gardens, parks, and other public and private lands (EPA, 2023). The most decentralized approach consists of individual, farm or household-scale waste management, which may or may not be embedded in a larger waste management network (Rajendran et al., 2012).
Anaerobic digestion (AD), i.e., the process by which organic material is degraded under anaerobic conditions by a consortium of archaea and bacteria (Riviere et al., 2009), is an effective food waste management technology. AD reduces the amount of waste sent to landfills, mitigating greenhouse gas emissions, groundwater pollution, and air contamination, while simultaneously inactivating pathogens and generating two valuable byproducts, biogas and fertilizer (Dalke et al., 2021; Tian et al., 2021). The inputs for AD, called feedstock, can be animal manure, wastewater biosolids, or food waste. AD requires a sealed reactor, called digester, which varies in shapes and sizes specific to the site, goals, and feedstock type. Complex microbial communities, which are either introduced through inoculants or come with the feedstock, grow in digesters and are responsible for processing the waste. Biogas is the most used AD byproduct and contains 60–70% methane, 30–40% carbon dioxide, and traces of hydrogen and hydrogen sulfide (Ward et al., 2008). AD also delivers a digestate, the solid and liquid material end-product of the AD process, which can be used as biofertilizer (Holohan et al., 2022; EPA, 2024a).
Biofertilizers are often overlooked as a secondary byproduct of AD. Yet, the nutrient-rich digestate consists of diverse dissolved minerals, organic acids, small organic particles, dead and living bacteria, and water. When new feedstock or water is added to the digester, a portion of the liquid digestate (i.e., effluent) is displaced and can be collected for use. Together with indigestible fibers, solid particles sink to the bottom, where they need to be removed from time to time, resulting in the solid digestate fraction or sediment (Abel and Ebel, 2022). The effluent can be used as basal and foliar fertilizer or soil amendment (Lohri et al., 2017). Digestates from food waste are rich in mineralized N, P, K, and organic C (Ren et al., 2020; O'Connor et al., 2022). In addition, they can contain plant hormones and other phytochemicals that can modify plant growth (Ren et al., 2020). Application of digestates to perennial ryegrass (Lolium perenne L.), white clover (Trifolium repens L.), and vegetables resulted in crop yields comparable to the yields obtained when using commercial fertilizer (Coelho et al., 2019; Song et al., 2021). Digestate has also been used to fertilize ornamentals, pulses, and corn (Zea mays L.; Ebel, 2020; Sharma et al., 2023; Tiong et al., 2024a). An emerging application of digestate is in “digeponics,” a type of hydroponics in which digestate-based products are used as fertilizer (Stoknes et al., 2016).
Although AD is an emerging food waste management technology, its use in the US is still limited to large-scale operations and urban areas. Rural communities could benefit from AD to mitigate costs of food waste collection and processing (Xu et al., 2018; The Recycling Partnership, 2023). One way to increase the adoption of AD in rural areas is by reducing their operational costs through decentralized solutions (Ntostoglou et al., 2021; Almansa et al., 2023) that rely on small systems tailored to the unique waste streams, infrastructure, and resources of a specific community (Anyaoku and Baroutian, 2018; Xu et al., 2018). Decentralized AD solutions typically feature compact, modular digester designs with simple feedstock input mechanisms and outlets engineered for ease of use and minimal maintenance (Wang, 2014; Niles, 2020). Although decentralized food waste management has been assessed in rural areas of low-income countries (Schoeber et al., 2021), studies in high-income countries are rare (Niles, 2020).
Household-scale AD, the most decentral AD strategy, presents a viable alternative in areas lacking municipal organic waste management. Like larger AD models, household-scale biodigesters can generate both biogas and biofertilizer (Vaneeckhaute et al., 2018; Schoeber et al., 2021). However, diverse obstacles challenge their use for processing food waste. While AD systems are most effective with homogeneous feedstock (Capson-Tojo et al., 2016), household food waste is usually heterogeneous, and can vary by season, region, and dietary preferences (Strazzera et al., 2021; Xiao et al., 2022), making its composition difficult to predict (Malamis et al., 2015; Slopiecka et al., 2022). Categorization of food waste by food type, nutrient content, or other factors can help minimize the challenges associated with variable feedstocks by increasing planning certainty (Garcia-Garcia et al., 2017). Also, household-scale digesters can degrade food waste effectively but typically do not generate enough biogas to meet entire household needs (Lou et al., 2012; O'Connor et al., 2021), making them more suitable for fertilizer production than for generating energy. While household-scale systems are popular in warm climates throughout Africa and Asia (Surendra et al., 2013; Jegede et al., 2019), few studies have evaluated these systems in cooler temperate or continental climates, where short summers and cold winters may limit their operation. The present study attempts to bridge this knowledge gap, emphasizing a strategy feasible for towns and small cities of around 50,000 residents or fewer located in cool climates.
To better understand household-scale AD performance and feasibility, we conducted a community-based case study in a cool climate in the northern USA to characterize domestic food waste and digestate that can be used as fertilizer from household-scale AD systems. The objectives of our work were to (1) analyze the nutrient content of different household organic waste sources, (2) evaluate small-scale anaerobic digester performance and nutrient quality of digestates from household food waste, and (3) obtain direct feedback from households on the practicality of using this technology.
2 Background
2.1 Food waste management in the US
In the US, food waste from households, institutions, and commercial settings constitutes the largest fraction of all generated solid waste (Ntostoglou et al., 2021). Despite widely inconsistent data on food waste (Dalke et al., 2021), food waste recovery in the US, though increasing, lags other countries (Platt and Goldstein, 2014). In 2017, 31% of US food waste volume consisted of vegetables, followed by meat, eggs, and nuts (21%), fruits (18%), grains (10%), dairy (9%), sugars and other sweeteners (7%), and fats (4%). For most of these categories, the largest portion was generated through household food waste (Dalke et al., 2021). The US Environmental Protection Agency (EPA) prioritizes waste prevention, donation, and upcycling (i.e., transforming food waste into high-value products such as turning orange peels into flavoring, AD, or composting) while landfilling, incineration, and disposing of food through a drain are not recommended due to damaging environmental impacts (EPA, 2024c).
Four main technologies can treat food and other organic waste: direct use (e.g., combustion); biochemical treatment (e.g., composting or AD); physicochemical treatment (e.g., transesterification of cooking oil into biodiesel); and thermochemical treatments such as pyrolysis (Lohri et al., 2017). Composting is by far the most common approach to processing organic waste in the US, where 70% of compost sites are dedicated to processing yard trimmings and lawn (Platt and Goldstein, 2014). Composting requires low initial investment compared to other technologies. Its disadvantages include relatively high operating costs as constant rotation of the feedstock is necessary. If rotation is limited, parts of the compost pile go anaerobic, emitting uncaptured methane into the atmosphere (Bond and Templeton, 2011).
2.2 Anaerobic digestion
AD has a high potential not only for nutrient but also energy recovery. In countries with appropriate food waste collection infrastructure, including Germany, Sweden, Denmark, and the UK, biopower generation through AD is an essential part of the national energy supply. In the EU, biogas resulting from AD had an operational capacity of 30 GW in 2015, compared to 16.7 GW in the US (Scarlat et al., 2018; Dalke et al., 2021). The AD of food waste emerged in the US in the early 2000s and primarily uses single feedstock sources from the food industry (Dalke et al., 2021).
The AD of organic materials involves four steps: (1) hydrolysis, (2) acidogenesis, (3) acetogenesis, and (4) methanogenesis. Hydrolysis breaks down complex organic compounds which are then fermented into volatile fatty acids, hydrogen, and carbon dioxide during acidogenesis. Through acetogenesis, hydrogen and carbon dioxide are reduced to acetic acid. The ultimate step is methanogenesis, wherein anaerobic bacteria utilize hydrogen and acetate from previous stages to produce methane (Dalke et al., 2021).
Although AD is a well-established technology, numerous factors have limited its adoption for food waste management (Xu et al., 2018). The major challenge consists in food waste heterogeneity, requiring co-digestion, breaking down multiple organic materials (EPA, 2024a). Co-digestion is slower than the digestion of homogenous feedstock because the microbial community in the digester must adapt to processing substrates with varying chemical compositions. However, the risk of rapid hydrolysis of the soluble organic matter that can cause corrosion of the digester due to acidification, is less likely to occur under co-digestion (Hagos et al., 2017; Meng et al., 2022).
Inconsistency in the volume and content of the supplied feedstock can negatively impact AD, particularly in smaller facilities (Nghiem et al., 2017). Feedstocks rich in cellulose, hemicellulose, starch, and pectin, polysaccharides commonly found in fruits, grains, vegetables, and root crops, comprise roughly 57% of food waste in the US and are difficult to degrade due to an elevated carbon-nitrogen ratio (C:N; Capson-Tojo et al., 2016; Dalke et al., 2021). An optimal C:N for AD is between 20 and 30. High C:N can result in rapid consumption of N by microbes, while low C:N can lead to ammonia accumulation and high pH values that inhibit microbial activity (Slopiecka et al., 2022). Fluctuating C:N can cause delayed digestion or system failure (Lin and Lay, 2004; Jiang et al., 2018). Likewise, high lipid or protein/nitrogen content in food waste can result in inhibitory ammonia concentrations causing process instability (Banks et al., 2011).
The hydraulic retention time (HRT) describes the period that the liquid portion of the feedstock remains in the digester and determines how long the microorganisms are exposed to the organic material. The optimum HRT for the AD of food waste depends on the feedstock composition and operating conditions such as temperature and ranges from 15 to 25 days (Dalke et al., 2021). Changes in pH, inhibitory levels of ammonia, suboptimal C:N ratios, and foaming can increase the HRT or lead to overall system failure (Xu et al., 2018; Xiao et al., 2022). The optimal pH for hydrolysis, acidogenesis, and acetogenesis is 5.5–6.5, while methanogenesis requires a pH range of 6.5–7.2 (Zhai et al., 2015).
2.3 Digester design
Three parameters classify digester designs: feedstock moisture, feedstock loading, and AD temperature.
• Moisture. A distinction between wet and dry digesters refers to the moisture content of the supplied feedstock. A wet digester processes feedstock with < 15% solids content, while dry digesters can process higher amounts of solids (EPA, 2024b).
• Loading. In a batch digester, feedstocks are loaded into the digester all at once, while in a continuous-flow digester, they are constantly fed into the digester while the digestate is removed (EPA, 2024b).
• Temperature. Microorganisms selectively thrive at different temperature ranges, affecting the pace of digestion and the amount and quality of outputs. Temperature ranges are typically 30–38°C for mesophilic and 50–60°C for thermophilic digesters (Dalke et al., 2021; Tiong et al., 2024b). Thermophilic digesters facilitate a higher pathogen mortality in the digestate (EPA, 2024b).
The pathways for methane production in AD, especially acetoclastic methanogenesis, common under mesophilic conditions, and syntrophic acetate oxidation, common in thermophilic digesters, are influenced by the temperature in the digester, which is regulated by the digester's design. These pathways determine methane production and process stability, with syntrophic acetate oxidation being more resilient to high ammonia levels (Treichel et al., 2019).
Decentralized AD solutions commonly rely on dry, continuous-flow, and mesophilic digesters and range between 0.65 and 150 m3 in volume (Almansa et al., 2023). Digesters with operating volumes at the upper end of this range are likely not suitable for households given their size and requirement for daily feedstock loadings (Lou et al., 2012; Vaneeckhaute et al., 2018). Common household AD systems include the fixed dome digester (a durable underground system that operates under constant pressure), the floating drum (a system with a movable drum that adjusts with gas production), and the plug flow digester (a flexible, above-ground unit), including the Home Biogas system used in this study (Surendra et al., 2014; Alexander et al., 2019). While nutrient recycling is effective in these systems, household-scale biodigesters usually cannot meet the entire energy requirements of a household (Jegede et al., 2019; Ellacuriaga et al., 2022). Another limitation of these digesters is that they cannot eliminate 100% of the pathogen load in the feedstock (Almansa et al., 2023). Finally, odor emissions from AD can hinder the adoption of smaller-scale systems. Across the various scales of AD designs, the emission of volatile organic compounds, such as formaldehyde and benzene, can cause multiple challenges, including nutrient loss, reactor instability, and public health concerns (O'Connor et al., 2022).
Household-scale AD systems are increasingly used around the world. For example, China had 41.8 million household-scale biogas plants in 2014 using livestock waste, domestic sewage, and agricultural wastes as feedstocks (Deng et al., 2017). India constructed over 90,000 family-size biogas digesters between 2017 and 2021; and Nepal, China, and Vietnam funded households to connect their toilet to an AD system (Almansa et al., 2023). Case studies from low and middle-income countries indicate that most of the biogas produced in household-scale AD systems is used for cooking, followed by lighting and heating (Kulkarni et al., 2021).
3 Methods
3.1 Case study
This community-based case study was conducted in Bozeman, a cold-climate town in Southwestern Montana, USA, with a population of ~53,000 residents (City of Bozeman, 2024), evolving into a small city (Warne et al., 2023). When we conducted our study, in 2021 and 2022, Bozeman did not have a municipal food waste recycling or composting program or a “Zero waste goal” (Rachal et al., 2022). Due to the lack of city food waste management and the number of residents, Bozeman is a representative location to conduct a case study on decentralized household-scale AD of food waste.
To evaluate the viability of household-scale AD to process food waste in Bozeman, we conducted a community-centered case study in 2021 and 2022 that explored technical aspects and user perceptions of biodigesters (Chan and Farrington, 2018). We also gathered quantitative data on household food waste composition and the chemical properties of the digestate. Households suitable for implementing this study were identified with assistance of the City of Bozeman and through an ad in a local newspaper. Households were selected based on (1) willingness to participate in the study (i.e., motivation to separate food waste from other garbage and its subsequent use in a digester), (2) ability to use the generated biofertilizer digestate (i.e., presence of a garden or flowerbeds), and (3) interest and ability to participate in community-centered research. Surveys were conducted following an MSU Institutional Review Board protocol approved by the MSU Office of Research Compliance (IRB #RE012121-EX). Out of 118 applications, we selected twelve households, obtaining a stratified sample of the overall population based on household income and number of residents per household.
3.2 Food waste collection and characterization
Systematically classifying food waste inputs and their chemical composition is essential to determine best practices for AD (Dalke et al., 2021), including digester design, feedstock load, and temperature regime. Other approaches to waste classification include classifications by origin, ingredients, packaging, degradation state, life cycle stage, edibility, biodegradability, complexity, treatment, and stage of the supply chain (Lebersorger and Schneider, 2011; Garcia-Garcia et al., 2017). Given the variability of household food waste in our study, classifying food waste by nutrient content was the most effective approach for household-scale digester operations to ensure balanced nutrients and optimal C:N ratios of the feedstock.
In 2021, the selected households received 5-gal lockable plastic bins for organic waste storage and specific instructions on their usage of food waste. We collected bins every 2 weeks for 3 consecutive summer months to determine household food waste composition. Following collection, the food waste was visually screened for composition and classified into 12 categories based on food type and nutrient content: (1) C-rich vegetables, (2) N-rich vegetables, (3) K-rich vegetables, (4) other vegetables, (5) citrus fruits, (6) N-rich fruits, (7) K-rich fruits, (8) other fruits, (9) coffee grounds, (10) bakery, (11) dried foods/powders, and (12) other, including prepared or processed dishes (Table 1). We weighted the fresh food waste in each category. After each of six collections, food waste in each category was shredded and mixed, and three 200 g samples per category (total of 36 samples) were frozen and subsequently analyzed for dry matter total solids (TS), organic matter (OM), pH, total N (TN), ammonium-N (NH3-N), organic-N (ON), P, K, Ca, Mg, S, Fe, Mn, Cu, Zn, and Na.
3.3 Digester comparison
In 2022, we compared two biodigester models. One model (Home Biogas hereafter) was a commercial Home Biogas 2 (Home Biogas, Beit Yanai, Israel), with a 1,200 L digester tank, and a capacity of storing up to 700 L biogas (Figure 1). The second model was a prototype designed by the research team (MSU model hereafter), a 114 L-capacity plastic drum built following a previous design (Ebel and Kissmann, 2019) that generated effluent (biofertilizer) but without capturing biogas (Figure 2). Six households received the Home Biogas and six received the MSU model. Together with the digesters, households obtained a brief introduction to use the biodigester and regular technical assistance.
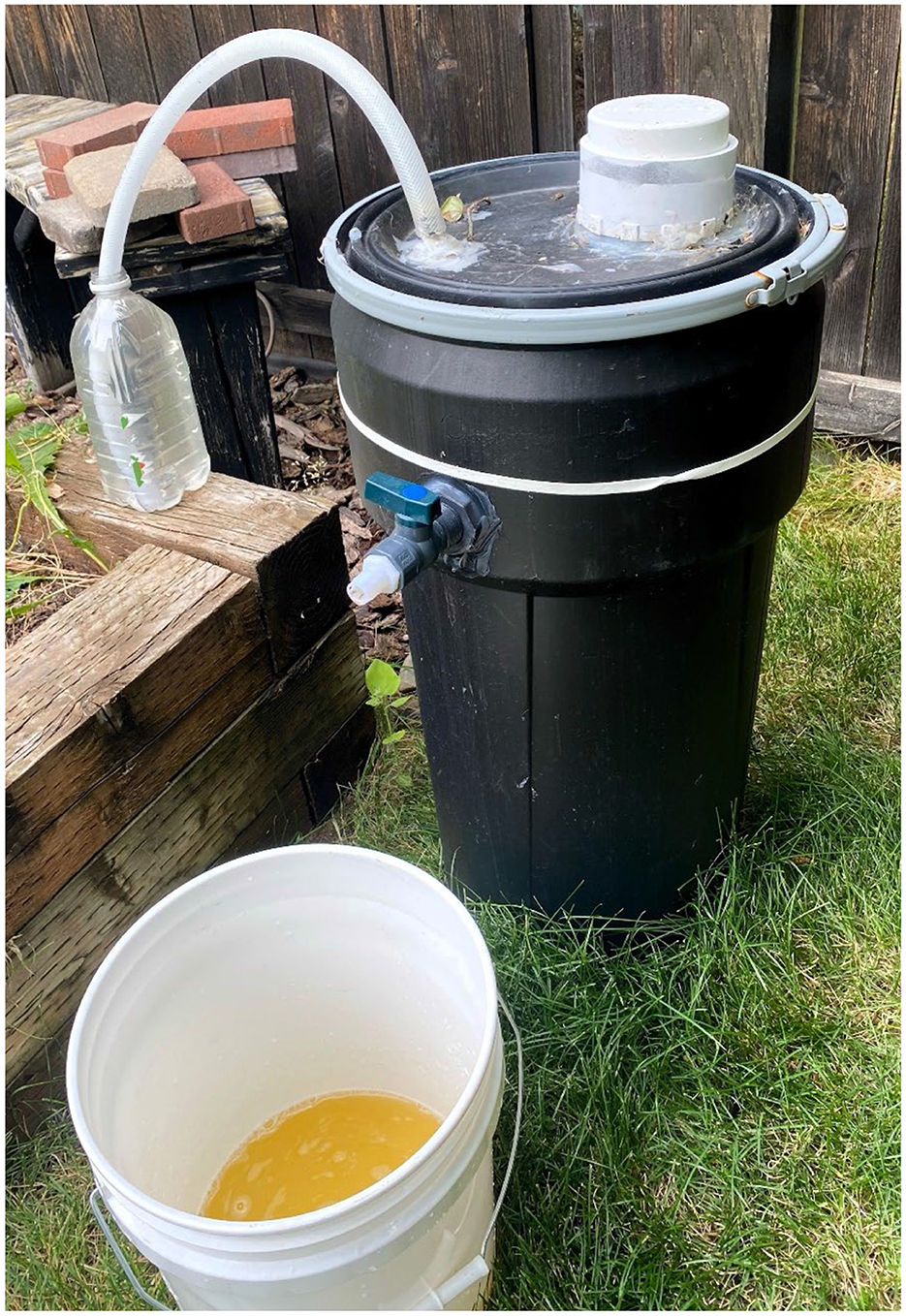
Figure 2. Custom-built (MSU) digester model in one household's backyard with recently collected effluent in the bucket in front.
Manure is commonly used as AD inoculum (Gu et al., 2014), but its sourcing, transport, and odor make it challenging to use in urban environments. Hence, we used locally sourced fresh raw cow milk as an inoculum (at 2% of digester volume) which can be challenging to source in the US but is easier to transport and more user-friendly to add to the digesters than manure (Xiao et al., 2022). Raw cow milk is an effective inoculant due to its rich microbial content, including lactic acid bacteria and lactobacilli, which enhance fermentation efficiency. Additionally, milk provides enzymes, fats, amino acids, vitamins, and minerals, which further support microbial growth and the AD process. The use of raw, unpasteurized milk is critical. Whey is a common alternative inoculant in larger systems, but milk is popular in simple small-scale digesters (Ebel and Kissmann, 2019; Meeske et al., 2002; Rivera and Hensel, 2009). While carrier materials are often added to AD systems to enhance methanogen colonization (Pilarska et al., 2021) they were not added to the digesters in this study since methane production was not a goal of the study. All feedstock was unprocessed food waste produced by households. In addition to milk and feedstock, we added water to 70% of the total digester volume.
For both digester models, food waste generated by each household was regularly fed to the digesters. After an initial digestion period of 21 days, we collected digestate samples from each digester every 2 weeks for analysis to evaluate pH, electric conductivity (EC), moisture, dry matter, OM, and nutrient content. Total N and C were measured by combustion, while and NO3 by the Kjeldahl method and cadmium reduction, respectively.
3.4 Survey
Participants completed a questionnaire during the final study period, June through October 2022, that assessed their reasons for joining the project, tracked food waste management practices, including the quantity and type of food waste, attitudes toward biogas usage, easiness of the biodigester handling, and recommendations for further household adoption. The questionnaire included open-ended, multiple-choice, Likert-type, ranking, and binary (Y/N) questions. We asked participants about the feasibility of using household-scale biodigesters, why they joined this study, and how their participation in this study shaped their perception of this technology and wider food waste issues.
The study sample (n = 28) included 39% males and 61% females from all 12 participating households. Nine (32%) participants were 16–19 years old, 54% were 19–70 years old, and 14% were older than 70 years. All received and analyzed survey responses were complete and consistent, with no exclusions required during survey processing. The survey data reliability was validated using Cronbach's Alpha test (Ferketich, 1990; Vaske, 2019). The resulting value of 0.71 is considered acceptable for this type of human subject research (Vaske et al., 2017).
3.5 Data processing
3.5.1 Statistical analysis
All statistical analyses were performed in R (R Core Team, 2023). We evaluated the food waste composition using analysis of variance (ANOVA). Spearman's rank correlation matrix was calculated with log-transformed nutrient concentrations and plotted with the R package PerformanceAnalytics (Carl and Peterson, 2020). Ordination was performed with principal components analysis (PCA) using the R packages vegan, stats, ggplot, and ggfortify (Tang et al., 2016; Oksanen et al., 2018; R Core Team, 2023). Compositional analysis was performed for C-rich vegetables, N-rich vegetables, K-rich vegetables, other vegetables, citrus fruits, K-rich fruits, and other fruits since household waste production in the remaining categories was insufficient for analysis. Estimated marginal means and standard errors were calculated for interactions between digester model and sample time using the emmeans function in the R package emmeans (Lenth, 2023; R Core Team, 2023). Differences between factors were examined using emmeans based on adjusted LSD tests. Additional post-hoc tests of interaction means were also conducted with emmeans to compare response variables separately for each digester design. Assumptions of homoscedasticity, normality, and influential observations were visually assessed (Schafer and Ramsey, 2003). Response variables were log-transformed to meet assumptions of normality. Degrees of freedom were determined by the Kenward-Roger method (Kenward and Roger, 1997) and p-values were adjusted for multiple comparisons using the Bonferroni test (Dunn, 1961).
3.5.2 Survey analysis
Closed-ended responses were analyzed using SPSS for Windows, v 26 (IBM, Armonk, NY; De Sá, 2007). Responses were transformed into numeric binary values (Brown, 2011). For all data, we conducted descriptive statistics, which included frequency and percentage response distributions as well as dispersion measures. For the analysis of open-ended questions, we conducted inductive, undirected content analysis to identify common themes in responses (Kuckartz, 2014). For the coding process, we used NVivo 14 (QSR International, Doncaster, Australia). We applied inductive coding, prioritizing in vivo codes to capture participants' own words. Condensed meaning units were summarized, and the frequency of meaning units for each code was calculated as a percentage of the total meaning units for each survey question (Leech and Onwuegbuzie, 2011; Saldaña, 2015).
4 Results and discussion
4.1 Food waste characterization
The average wet weight of food waste per collection period and household was 1.9 kg and varied from 0.4 to 5.3 kg, depending on household size. C-rich vegetables, N-rich vegetables, K-rich vegetables, K-rich fruits, and coffee grounds were the most abundant food waste categories across households, and food waste biomass in each category varied over time (Figure 3). Mean food waste generated across all categories and households was 180.0 ± 64.5 g per 14 days. Quantities of waste, categorized as “other vegetables,” citrus fruits, or “other fruits” were relatively consistent over time with a mean weight of 26.0 ± 18.6, 81.9 ± 40.6, and 58.8 ± 23.5 g, respectively. In contrast, K-rich vegetables, which was the highest-yielding waste category, showed highly variable weight, from 990.0 ± 897.7 g in week 2 to 168.0 ± 195.2 g in week 6 (Figure 3). Variability of food waste type and quantity over time is common and has been linked to seasonal variation in food sources (Xiao et al., 2022). Changes in food waste abundance in each category likely reflected food sources and household preferences, highlighting the challenge of maintaining a stable feedstock amount and composition in household-scale systems. Our results were derived under summer conditions and may differ in colder seasons, other climates, or areas with different food procurement habits.
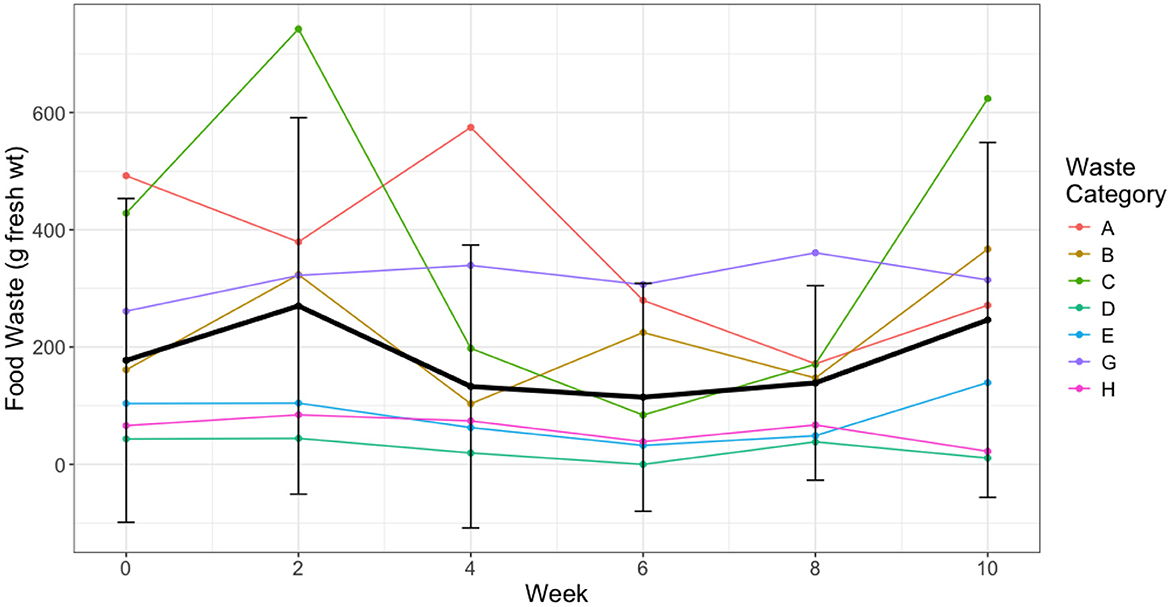
Figure 3. Mean household food waste fresh weight (g) by category for each collection period. Only waste categories used for nutrient analysis are shown. Black line shows mean food waste for all categories per collection period. A, C-rich vegetables, tubers, and bulbs. B, N-rich vegetables. C, K-rich vegetables and fungi. D, Other vegetables. E, Citrus fruits. G, K-rich fruits. H, Other fruits.
Nutrient content varied between household food waste categories. The first axis of the PCA plot separated the waste based on food type (fruit or vegetable). Total variability in the data and PCA scores indicated that each food waste category formed a distinct cluster based on nutrient analysis with 27.3% of the variability explained by differences between C-rich vegetables and citrus as well as N-rich vegetables and K-rich fruits and vegetables (Figure 4). Visualization of the contribution of each nutrient to the PCA identified TC (total carbon), OM, TS, C:N, moisture, and pH as the most crucial factors contributing to the first axis. OM, TC, TS, and C:N were strongly associated with fruit waste while water content (%), pH, and NH3-N were associated with vegetable waste (Figure 4). These results are consistent with Mou et al. (2023) who found distinct nutrient profiles among food waste types.
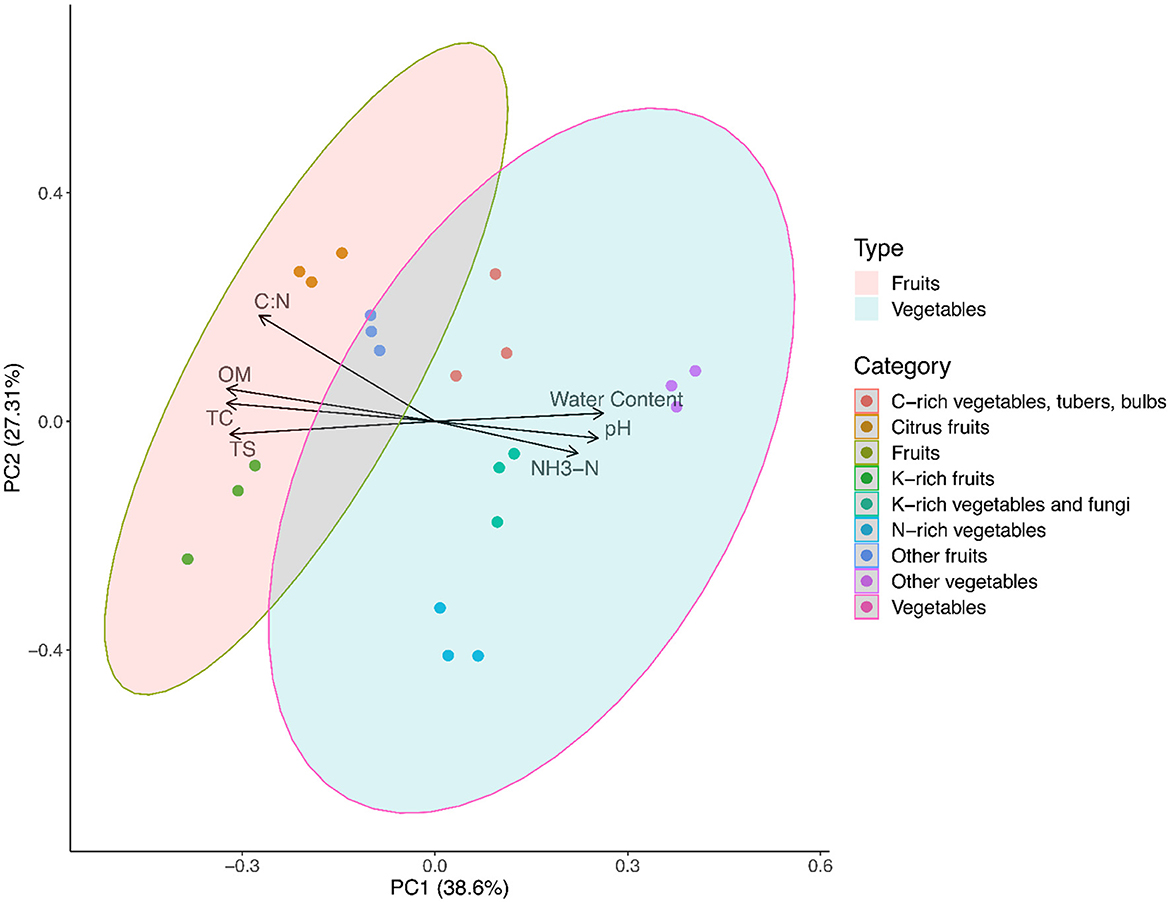
Figure 4. Biplot of PCA scores and loadings of the nutrient composition of food waste by waste category. Ellipses show 95% confidence intervals for food waste grouped by fruits or vegetables.
Total N varied from 18 ± 1.5 g kg−1 dry weight in K-rich fruits to 37.47 ± 1.55 g kg−1 dry weight in the “other vegetables” category (Tables 2, 3). Consistent with Zhang et al. (2007), TC varied from 43.3 ± 0.5% dry weight in N-rich vegetables to 46.1 ± 5.3% dry weight in the “other fruits” category. pH values differed considerably between food waste categories (Table 2). The C:N ratio is an indicator of nutrient availability and stability of the AD process (Villamil et al., 2018; Slopiecka et al., 2022), with values between 20 and 30 considered suitable (Xiao et al., 2022). Results of the food waste analysis indicated that vegetable waste had lower C:N (p < 0.001) compared to fruit waste (Tables 2, 3). C:N for vegetables ranged from 12.1 to 18.6, while for fruits, it ranged between 20.8 and 25.7, aligned with values for vegetable and fruit wastes from a broad range of sources (Slopiecka et al., 2022). Thus, the food waste generated by the households in this study was suitable for AD as long as fruit and vegetable fractions were balanced to minimize ammonia accumulation and nutritional imbalances for microbes (Capson-Tojo et al., 2016; Montoro et al., 2019).
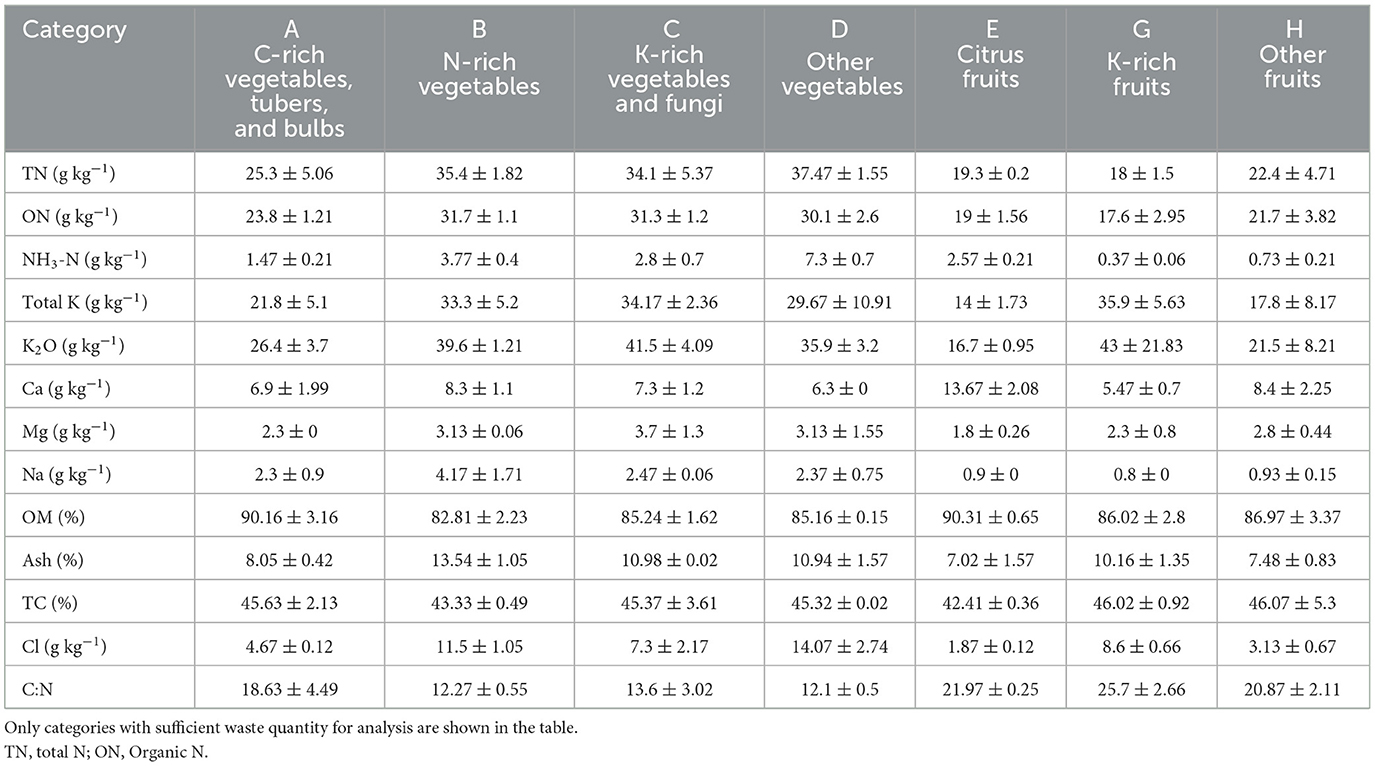
Table 3. Chemical characterization of feedstocks by waste category showing average values and standard deviations of dry samples across all households.
4.2 Digester operation and digestate characterization
In general, digestate nutrient content was lower than reported values (Wang et al., 2021; O'Connor et al., 2022; Mou et al., 2023), likely because of the shorter operating period and low temperatures. Mean total C and N content for all sampled digestates were 2,093.3 ± 1956.8 and 246.7 ± 206.6 mg l−1, respectively, and highly variable between samples (Table 4). This variability can be associated with the variability we observed in the food waste (Figure 3). With mean values below 300 mg l−1 of the digestate (Table 4), total N was more diluted in our samples than in other studies (with varying feedstock sources), which have reported concentrations of 460–2,930 mg l−1 (Loria et al., 2007; Mou et al., 2023; O'Connor et al., 2023); including 2100 mg l−1 from a simple thermophilic digester, similar to MSU model, though using a more manure-based feedstock (Ebel, 2020). The K content of 1212.5 mg l−1 we measured in the digestate of the MSU model was within the expected range of 1,200–11,500 mg l−1 for AD digestate in general, not discriminating between digester types and feedstocks (Moeller and Mueller, 2012). The K content of 857.14 we found in the Home Biogas model digestate was below this range and may be related to the higher dilution of the digestate in this model compared to the MSU one. It is important to note that the nutrient content values references cited here are based on the AD of agricultural feedstock or household waste managed through commercial waste systems, rather than decentralized household AD solutions.
Nutrient content was similar between the two digester designs for most nutrients, except for K (p < 0.05) and S (p = 0.06; Table 5). Because of the variability in household food waste inputs, it was not possible to determine whether these differences were due to food waste inputs or digester design. Likewise, the digestate content of volatile fatty acids, bicarbonates, and other factors potentially contributing to pH variation (Mir et al., 2016), varied across samples, most likely related to varying feedstock inputs rather than digester design.
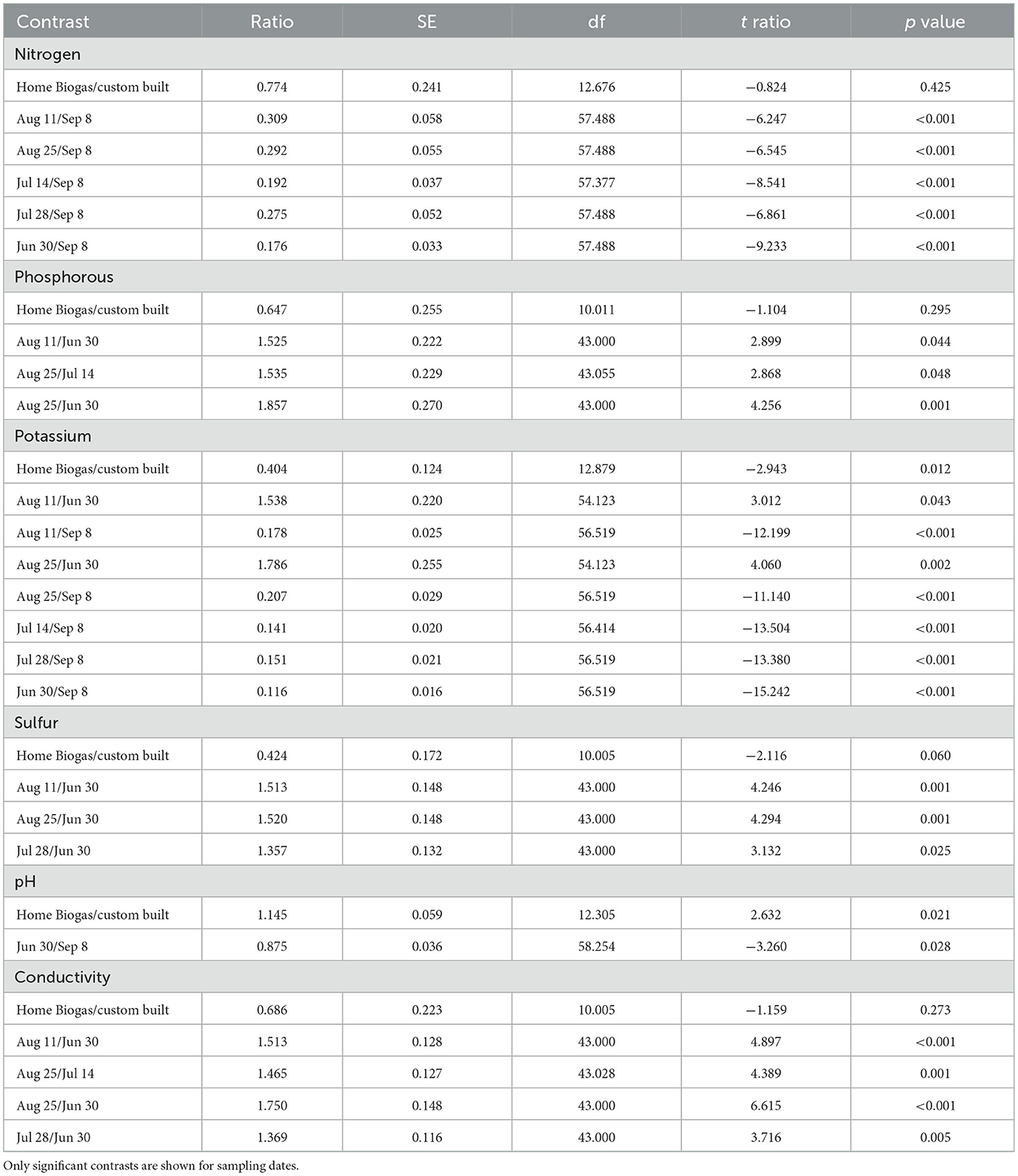
Table 5. Estimated marginal means contrasts of digestate nutrient content by digester design and sampling date.
Digestate nutrient content changed during digester operation with pH, N, P, K, S, and conductivity all increasing (p < 0.05; Table 5). The average ammonia concentration was 88.7 mg l−1 and not different between digester models (p = 0.46). Ammonia inhibition of methanogenesis can occur at ammonia concentrations above 650 mg l−1 (Banks et al., 2011). The low levels of ammonia observed in this study suggest that ammonia did not cause process instability or failure. While ammonia levels remained below inhibitory thresholds, the ratio of NH3-N to total N increased (p < 0.001) from 0.09 in the food waste to 0.25 in the digestate at the end of the treatment period. This indicates AD occurred as the degradation of organic material led to the accumulation of NH3-N.
The average digestate pH was 5.7 compared to a pH of 4.4 for the analyzed food waste. In contrast to our results, food waste digestates are usually alkaline at the end of the digestion process (O'Connor et al., 2022). The low digestate pH values we observed suggest that while digestion occurred, it was incomplete, likely due to the cool climate of our study site. Digestion also reduced the C:N ratio (p < 0.001) from 12.1 to 25.7 for food waste to 6.57 ± 2.23 and 10.25 ± 3.99 for the final digestate in the Home Biogas and MSU model digesters, respectively (Tables 4, 5). Food waste digestate C:N ratios can be highly variable, with reported values ranging from 6.4 to 28.4 (Wang et al., 2021; O'Connor et al., 2022). While we did not measure several important indicators of the digestion progress, including gas production volume and methane content, the reduction of the C:N ratio was likely due to the loss of C as CO2 and CH4 gases, as is reported by Iocoli et al. (2019). The PCA of digestate showed a shift in nutrient profiles between fresh food waste and digestate (Figure 5).
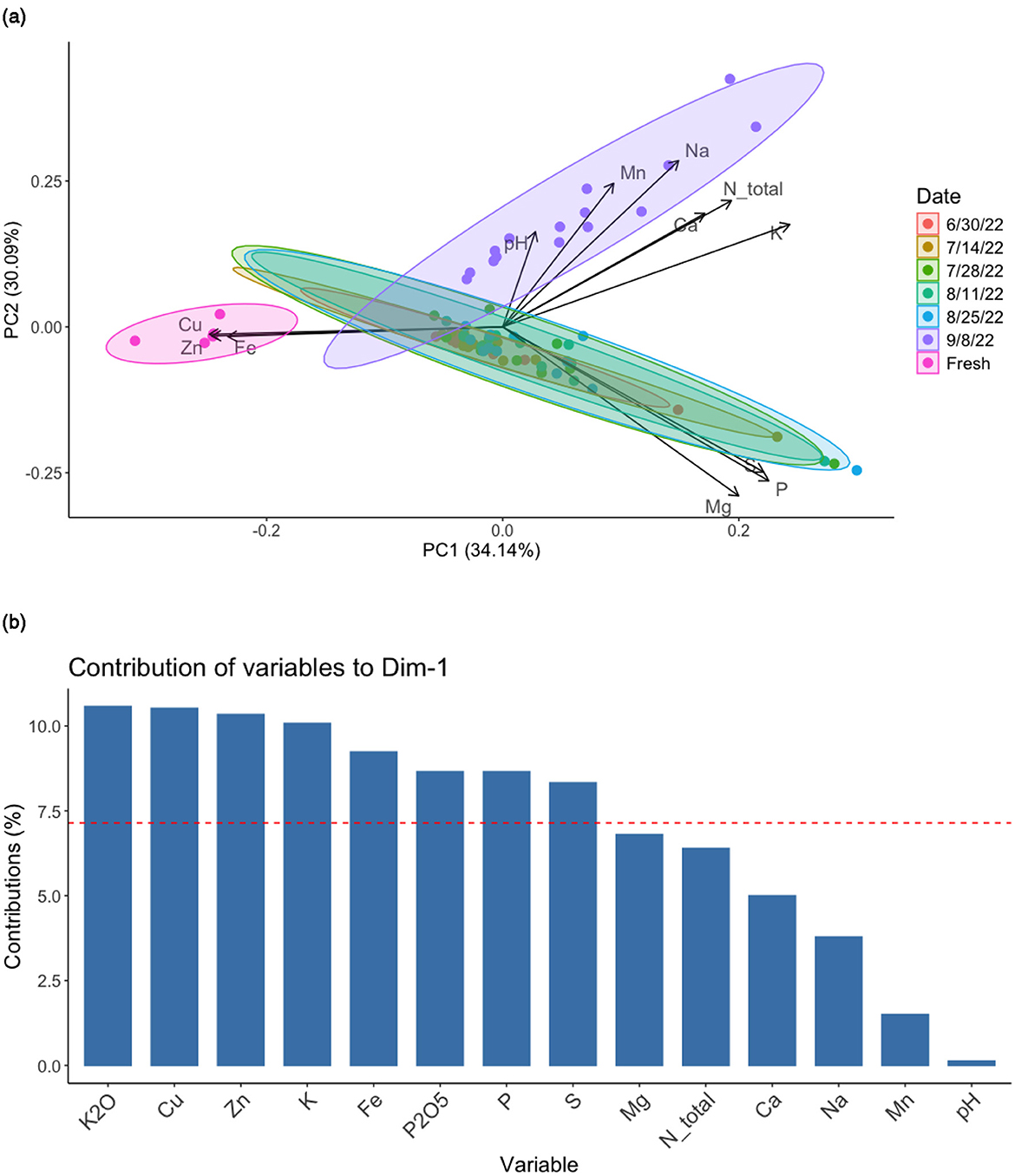
Figure 5. (a) Biplot of PCA scores and loadings of the nutrient content of fresh food waste and digestate at each sampling time point. Ellipses show 95% confidence intervals. (b) PCA contribution plot showing the contribution of nutrient composition at each timepoint to the first axis of the PCA plot (a). The dashed reference line corresponds to the expected value if the contributions were uniform and contributions above this line were considered important.
AD is sensitive to temperature changes with mesophilic reactors functioning optimally at 35°C (Ward et al., 2008). While during our study, daytime temperatures reached this optimal, the average air temperature in Bozeman was 15.5°C in June of 2021, 20.7°C in July, 21.0°C in August, and 15.4°C in September of this year, indicating that the monthly average air temperatures throughout our period of digester use were similar to the 30-year temperature averages for these months in Bozeman (Montana Climate Office, 2025). The change in nutrient profiles near the end of the digestion period suggests that through the early summer, AD activity was low and did not increase until higher temperatures occurred. This challenge can be resolved through heating or insulating to improve AD pace and efficiency but increase complexity and cost (Wang, 2014).
4.3 Survey
Twenty percent of respondents (n = 28) reported having limited knowledge about biodigesters before this study, and 80% indicated they originally knew nothing about them. Participants' motivations for joining the research study were environmental concerns (67% of the respondents, multiple responses permitted), followed by a desire to learn more about food waste management (58%), and to support research on food systems (58%). Fifty percent of respondents participated in the study to contribute to their community, while 41% joined primarily to address their food waste disposal needs.
On average, before this study, 45% of food waste of participating households were regularly sent to a landfill, 14% were collected by an external organic waste service, 37% were composted, and 4% were processed through other methods. Upon completing the study, 64% of respondents reported a shift in their perception of food waste, with the majority (66%) noting increased awareness of the volume of food waste generated in their households. Participants also changed their perception of AD of food waste at the household level, yet responses differed depending on the digester model, as participants commented on the simplicity (MSU model), or difficulty (Home Biogas model) of operating the digesters. The perceptions of 73% of respondents regarding food recycling at the municipal level were also impacted, mainly on issues concerning scale “[This study] Helped me to see what issues a larger scale effort would require, it is hard to be on the same page” or “how much our house creates at the city scale is astonishing.” Regarding policies, 78% of respondents reported a shift in their understanding, particularly an increased awareness of the need for food waste management policies. However, only 44% of respondents indicated that their experience with biodigesters influenced their perception of climate change as an unresolved issue, while 82% of respondents expressed appreciation for community-based research to develop solutions for environmental challenges. Eighty-seven percent of respondents would continue using household-scale AD for their food waste with feasibility issues addressed. Half of the participating households did not specify how they used the biofertilizer they obtained, and its immediate use was not a requirement for project participation. Based on informal conversations, we learned that some of these households either did not use the biofertilizer at all or applied only a portion of it. Among the other half, the most common use was spraying the biofertilizer on lawns, followed by both basal and foliar applications on home garden vegetables, particularly leafy greens.
“Biodigestion is an effective way to reduce our footprint and to add organics back to nature. I did not have success with the gas, so I would not recommend that part of the digestion process. ‘Wasting' the gas is more than offset by the fertilizer and reduced landfill components.”
Participants reported convenience and feasibility challenges that affected their overall perception of household-scale AD systems.
“I love the concept, and I love how quickly the product is produced when the unit works. I like that you can continuously feed it and regularly extract the juice. Plus, it takes up so much less space than a traditional composter and the liquid would be so much easier to use!”
Participants reported that both biodigester models produced a smell that was occasionally perceived uncomfortable or slightly uncomfortable. Loading the biodigester was not considered challenging by any of the 12 participating households. However, handling the biodigester was frequently perceived complex, particularly during assembly, which 42% of respondents found difficult. The time for assembling, disassembling, maintaining, and extracting biofertilizer varied by the biodigester model, with the Home Biogas model requiring more time (i.e., it took about 1.5 h to assemble the Home Biogas, and 0.5 h for the MSU model). Additionally, 33% of respondents perceived disassembling the biodigester and extracting the biofertilizer as “somewhat difficult.” Overall, while assembling the AD system can be time-consuming, it is a one-time effort that, if done correctly, ensures long-term functionality without the need for repetition (Surendra et al., 2013). In contrast, dissembling, which was perceived as an uncomfortable task by households, was primarily necessary to prevent freezing of the digestate during the chilly winter months in Montana. This task is not required in tropical areas where household-scale AD use is more common (Rajendran et al., 2012). This situation emphasizes the need to enhance the cold-weather tolerance of these systems, such as using digester materials that withstand cooler temperatures or storing the in heated environments, even if digestion is paused for a few months. Additionally, disassembly and digestate extraction were more time-consuming for households using the Home Biogas system, suggesting that the smaller, easier-to-operate, and more affordable MSU model may be better suited for households in this region. Such households, typically consisting of 2–5 individuals, generate food waste volumes that do not necessitate a larger-scale model. Additionally, half of the individuals using the Home Biogas model reported being unable to extract biogas, negating the only potential advantage this system might have over the MSU model which does not capture gas.
5 Conclusions
One-third of food procured in the US is wasted (US Census Bureau, 2024), contributing to significant environmental, economic, and social challenges. AD presents a promising solution for upcycling food waste into valuable byproducts. AD of food waste can, therefore, contribute to a circular economy approach, i.e., a macroeconomic framework focused on minimizing waste and maximizing resource efficiency by ensuring that materials and products are continuously reused, repaired, recycled, or repurposed (Bongers and Casas, 2022; Bernal and Vanotti, 2023). In this context, AD creates a closed-loop system that reduces reliance on finite resources, minimizes environmental impact, and fosters economic sustainability. However, to be sustainable, AD solutions need to be implemented at appropriate scales and be practicable (Bridgens et al., 2018; Bakan et al., 2022).
Our study demonstrated that household-scale AD systems can effectively process food waste, even in cool climates, but it also highlighted the seasonality of this approach as digesters were not functional for over 6 months of the year due to cold temperatures. In cooler climates, insulation strategies can help optimize the operation of household-scale AD systems. Considering psychrophilic digesters, which function at 10–20°C (below the mesophilic temperature of the digesters we assessed) can be a promising research field (Akindolire et al., 2022).
We found that household food waste varies in composition and quantity, posing another challenge for maintaining stable digester operations. However, a balanced feedstock, comprised of fruits and vegetables, allows for ideal C:N ratios, a core parameter assuring AD efficiency. Yet, developing such solutions requires robust data on food waste composition, and the US lags other countries in collecting and analyzing such essential information (Dalke et al., 2021).
While most participants of our study appreciated the environmental benefits of AD, specifically reducing landfill waste and producing biofertilizer, challenges were reported concerning odor emissions and the seasonality of this technique in a colder climate. Though 87% of participants would recommend using a biodigester, they stressed the importance of convenience and ease of use in ensuring continued adoption. In this context, participants found the MSU custom-built digester model more feasible to use than the Home Biogas digester. Improving the materials of the custom-made units can extend their operational season in colder climates. Enhancing the sealing and adjusting the inlet are expected to be simple yet effective solutions for reducing odor emissions. In addition, design enhancements that either facilitate the disassembly and emptying of the system, or make this step unnecessary altogether, can contribute to user friendliness. Despite needing technical improvements for colder climates, household-scale AD systems show promise. Overall, our case study demonstrates the value of engaging communities in food waste processing and offers insights to improve biodigester design and inform policies for decentralized solutions.
Data availability statement
The original contributions presented in the study are included in the article/supplementary material, further inquiries can be directed to the corresponding author.
Ethics statement
The studies involving humans were approved by Montana State University Institutional Review Board. The studies were conducted in accordance with the local legislation and institutional requirements. The participants provided their written informed consent to participate in this study.
Author contributions
RE: Conceptualization, Formal analysis, Funding acquisition, Investigation, Methodology, Project administration, Supervision, Validation, Writing – original draft, Writing – review & editing. JE: Data curation, Formal analysis, Funding acquisition, Investigation, Methodology, Validation, Visualization, Writing – original draft, Writing – review & editing. BG: Data curation, Validation, Writing – original draft, Writing – review & editing. TS: Data curation, Validation, Visualization, Writing – original draft, Writing – review & editing, Investigation, Methodology. FM: Formal analysis, Funding acquisition, Investigation, Methodology, Supervision, Validation, Writing – original draft, Writing – review & editing.
Funding
The author(s) declare that financial support was received for the research and/or publication of this article. This study was funded by the US Environmental Protection Agency (EPA) under the Supporting Anaerobic Digestion in Communities Grant (Award #EPA-OLEM-ORCR-20-02).
Acknowledgments
First and foremost, we would like to thank the 12 households in Bozeman, Montana, who actively participated in this study, for their passion and commitment. We further acknowledge the significant contributions to the project implementation by the following Montana State University students: Elizabeth Reiva, Gracelyn Abel, Katy Dolan, Kevin Sheridan, and Tyler Kulak.
Conflict of interest
The authors declare that the research was conducted in the absence of any commercial or financial relationships that could be construed as a potential conflict of interest.
Generative AI statement
The author(s) declare that no Gen AI was used in the creation of this manuscript.
Publisher's note
All claims expressed in this article are solely those of the authors and do not necessarily represent those of their affiliated organizations, or those of the publisher, the editors and the reviewers. Any product that may be evaluated in this article, or claim that may be made by its manufacturer, is not guaranteed or endorsed by the publisher.
References
Abel, G., and Ebel, R. (2022). A Quick Guide to Anaerobic Digesters for Small Farms. Bozeman, MT: Montana State University Extension.
Akindolire, M. A., Rama, H., and Roopnarain, A. (2022). Psychrophilic anaerobic digestion: a critical evaluation of microorganisms and enzymes to drive the process. Renew. Sustain. Energy Rev. 161:112394. doi: 10.1016/j.rser.2022.112394
Aktas, E., Sahin, H., Topaloglu, Z., Oledinma, A., Huda, A. K. S., Irani, Z., et al. (2018). A consumer behavioural approach to food waste. J. Enterprise Inf. Manage. 31, 658–673. doi: 10.1108/JEIM-03-2018-0051
Alexander, S., Harris, P., and McCabe, B. K. (2019). Biogas in the suburbs: an untapped source of clean energy? J. Clean. Prod. 215, 1025–1035. doi: 10.1016/j.jclepro.2019.01.118
Almansa, X. F., Starostka, R., Raskin, L., Zeeman, G., De Los Reyes, F. 3rd, Waechter, J., et al. (2023). Anaerobic digestion as a core technology in addressing the global sanitation crisis: challenges and opportunities. Environ. Sci. Technol. 57, 19078–19087. doi: 10.1021/acs.est.3c05291
Angouria-Tsorochidou, E., Teigiserova, D. A., and Thomsen, M. (2021). Limits to circular bioeconomy in the transition towards decentralized biowaste management systems. Resour. Conserv. Recycl. 164:105207. doi: 10.1016/j.resconrec.2020.105207
Anyaoku, C. C., and Baroutian, S. (2018). Decentralized anaerobic digestion systems for increased utilization of biogas from municipal solid waste. Renew. Sustain. Energy Rev. 90, 982–991. doi: 10.1016/j.rser.2018.03.009
Aschemann-Witzel, J. (2018). Consumer perception and preference for suboptimal food under the emerging practice of expiration date based pricing in supermarkets. Food Qual. Prefer. 63, 119–128. doi: 10.1016/j.foodqual.2017.08.007
Bakan, B., Bernet, N., Bouchez, T., Boutrou, R., Choubert, J.-M., Dabert, P., et al. (2022). Circular economy applied to organic residues and wastewater: research challenges. Waste Biomass Valorization 13, 1267–1276. doi: 10.1007/s12649-021-01549-0
Banks, C. J., Chesshire, M., Heaven, S., and Arnold, R. (2011). Anaerobic digestion of source-segregated domestic food waste: performance assessment by mass and energy balance. Bioresour. Technol. 102, 612–620. doi: 10.1016/j.biortech.2010.08.005
Bernal, M. P., and Vanotti, M. B. (2023). Editorial: Insights in waste management in agroecosystems: 2021. Front. Sustain. Food Syst. 7:1176007. doi: 10.3389/fsufs.2023.1176007
Bond, T., and Templeton, M. R. (2011). History and future of domestic biogas plants in the developing world. Energy Sustain. Dev. 15, 347–354. doi: 10.1016/j.esd.2011.09.003
Bongers, A., and Casas, P. (2022). The circular economy and the optimal recycling rate: a macroeconomic approach. Ecol. Econ. 199:107504. doi: 10.1016/j.ecolecon.2022.107504
Bortolotti, A., Kampelmann, S., and De Muynck, S. (2018). Decentralised organic resource treatments–classification and comparison through extended material flow analysis. J. Clean. Prod. 183, 515–526. doi: 10.1016/j.jclepro.2018.02.104
Bridgens, B., Powell, M., Farmer, G., Walsh, C., Reed, E., Royapoor, M., et al. (2018). Creative upcycling: reconnecting people, materials and place through making. J. Clean. Prod. 189, 145–154. doi: 10.1016/j.jclepro.2018.03.317
Brown, J. D. (2011). Likert Items and Scales of Measurement. Available online at: https://teval.jalt.org/test/bro_34.htm (accessed January 7, 2025).
Capson-Tojo, G., Rouez, M., Crest, M., Steyer, J.-P., Delgenès, J.-P., and Escudié, R. (2016). Food waste valorization via anaerobic processes: a review. Rev. Environ. Sci. Bio Technol. 15, 499–547. doi: 10.1007/s11157-016-9405-y
Chan, Y. E., and Farrington, C. J. (2018). Community-based research: engaging universities in technology-related knowledge exchanges. Inf. Org. 28, 129–139. doi: 10.1016/j.infoandorg.2018.08.001
City of Bozeman (2024). Our City. Available online at: https://www.bozeman.net/ (accessed August 8, 2024).
Coelho, J. J., Hennessy, A., Casey, I., Woodcock, T., and Kennedy, N. (2019). Responses of ryegrass, white clover, soil plant primary macronutrients and microbial abundance to application of anaerobic digestates, cattle slurry and inorganic N-fertiliser. Appl. Soil Ecol. 144, 112–122. doi: 10.1016/j.apsoil.2019.07.011
Dalke, R., Demro, D., Khalid, Y., Wu, H., and Urgun-Demirtas, M. (2021). Current status of anaerobic digestion of food waste in the United States. Renew. Sustain. Energy Rev. 151:111554. doi: 10.1016/j.rser.2021.111554
De Sá, J. P. M. (2007). Applied Statistics Using SPSS, Statistica, MatLab and R. Berlin, Heidelberg: Springer Science and Business Media.
Deng, L., Liu, Y., Zheng, D., Wang, L., Pu, X., Song, L., et al. (2017). Application and development of biogas technology for the treatment of waste in China. Renew. Sustain. Energy Rev. 70, 845–851. doi: 10.1016/j.rser.2016.11.265
Dunn, O. J. (1961). Multiple comparisons among means. J. Am. Stat. Assoc. 56, 52–64. doi: 10.1080/01621459.1961.10482090
Ebel, R. (2020). Yield response of a polycropping system with maize to fermented foliar fertilizers. CIENCIA ergo-sum: revista científica multidisciplinaria Universidad Autónoma Estado México 27, 401–415. doi: 10.30878/ces.v27n3a8
Ebel, R., and Kissmann, S. (2019). Fermented leaf fertilizers—principles and preparation. Org. Farm. 5, 14–22. doi: 10.12924/of2019.05010014
Ellacuriaga, M., González, R., and Gómez, X. (2022). Is decentralized anaerobic digestion a solution? Analyzing biogas production and residential energy demand. ENG 3, 662–676. doi: 10.3390/eng3040045
EPA (2023). Community Composting. US Environmental Protection Agency. Available online at: https://www.epa.gov/sustainable-management-food/community-composting (accessed August 8, 2024).
EPA (2024a). How Does Anaerobic Digestion Work?. Washington, DC: US Evironmental Protection Agency. Available online at: https://www.epa.gov/agstar/how-does-anaerobic-digestion-work (accessed November 26, 2024).
EPA (2024b). Types of Anaerobic Digesters. Washington, DC: US Environmental Protection Agency. Available online at: https://www.epa.gov/anaerobic-digestion/types-anaerobic-digesters (accessed August 10, 2024).
EPA (2024c). Wasted Food Scale. Washington, DC: United States Environmental Protection Agency. Available online at: https://www.epa.gov/sustainable-management-food/wasted-food-scale (accessed November 20, 2024).
Ferketich, S. (1990). Internal consistency estimates of reliability. Res. Nurs. Health 13, 437–440. doi: 10.1002/nur.4770130612
Galanakis, C. M. (2015). Food Waste Recovery: Processing Technologies and Industrial Techniques. Cambridge, MA: Academic Press.
Garcia-Garcia, G., Woolley, E., Rahimifard, S., Colwill, J., White, R., and Needham, L. (2017). A methodology for sustainable management of food waste. Waste Biomass Valorization 8, 2209–2227. doi: 10.1007/s12649-016-9720-0
Gu, Y., Chen, X., Liu, Z., Zhou, X., and Zhang, Y. (2014). Effect of inoculum sources on the anaerobic digestion of rice straw. Bioresour. Technol. 158, 149–155. doi: 10.1016/j.biortech.2014.02.011
Gunders, D., and Bloom, J. (2017). “Wasted: how America is losing up to 40 percent of its food from farm to fork to landfill,” in NRDC Issue Paper (Washington, DC: NRDC).
Hagos, K., Zong, J., Li, D., Liu, C., and Lu, X. (2017). Anaerobic co-digestion process for biogas production: progress, challenges and perspectives. Renew. Sustain. Energy Rev. 76, 1485–1496. doi: 10.1016/j.rser.2016.11.184
Holohan, B. C., Duarte, M. S., Szabo-Corbacho, M. A., Cavaleiro, A. J., Salvador, A. F., Pereira, M. A., et al. (2022). Principles, advances, and perspectives of anaerobic digestion of lipids. Environ. Sci. Technol. 56, 4749–4775. doi: 10.1021/acs.est.1c08722
Iocoli, G. A., Zabaloy, M. C., Pasdevicelli, G., and Gomez, M. A. (2019). Use of biogas digestates obtained by anaerobic digestion and co-digestion as fertilizers: characterization, soil biological activity and growth dynamic of Lactuca sativa L. Sci. Total Environ. 647, 11–19. doi: 10.1016/j.scitotenv.2018.07.444
Jegede, A. O., Zeeman, G., and Bruning, H. (2019). A review of mixing, design and loading conditions in household anaerobic digesters. Crit. Rev. Environ. Sci. Technol. 49, 2117–2153. doi: 10.1080/10643389.2019.1607441
Jiang, J., Li, L., Cui, M., Zhang, F., Liu, Y., Liu, Y., et al. (2018). Anaerobic digestion of kitchen waste: The effects of source, concentration, and temperature. Biochem. Eng. J. 135, 91–97. doi: 10.1016/j.bej.2018.04.004
Kantor, L., and Blazejczyk, A. (2020). Food Availability (Per Capita) Data System - Glossary. Washington, DC: USDA. Available online at: https://www.ers.usda.gov/data-products/food-availability-per-capita-data-system/glossary/ (accessed August 22, 2024).
Kenward, M. G., and Roger, J. H. (1997). Small sample inference for fixed effects from restricted maximum likelihood. Biometrics 53, 983–997. doi: 10.2307/2533558
Kuckartz, U. (2014). “Three basic methods of qualitative text analysis,” in Qualitative Text Analysis: A Guide to Methods, Practice and Using Software, ed. U. Kuckartz (London: SAGE), 65–121. doi: 10.4135/9781446288719
Kulkarni, I., Zang, J. W., Leandro, W. M., Parikh, P., Adler, I., Da Fonseca-Zang, W. A., et al. (2021). Closed-loop biodigesters on small-scale farms in low-and middle-income countries: a review. Water 13:2744. foi: 10.3390/w13192744 doi: 10.3390/w13192744
Lebersorger, S., and Schneider, F. (2011). Discussion on the methodology for determining food waste in household waste composition studies. Waste Manag. 31, 1924–1933. doi: 10.1016/j.wasman.2011.05.023
Leech, N. L., and Onwuegbuzie, A. J. (2011). Beyond constant comparison qualitative data analysis: using NVivo. Sch. Psychol. Q. 26:70. doi: 10.1037/a0022711
Lin, C. Y., and Lay, C. H. (2004). Carbon/nitrogen-ratio effect on fermentative hydrogen production by mixed microflora. Int. J. Hydrogen Energy 29, 41–45. doi: 10.1016/S0360-3199(03)00083-1
Lohri, C. R., Diener, S., Zabaleta, I., Mertenat, A., and Zurbrügg, C. (2017). Treatment technologies for urban solid biowaste to create value products: a review with focus on low-and middle-income settings. Rev. Environ. Sci. Bio Technol. 16, 81–130. doi: 10.1007/s11157-017-9422-5
Loria, E. R., Sawyer, J. E., Barker, D. W., Lundvall, J. P., and Lorimor, J. C. (2007). Use of anaerobically digested swine manure as a nitrogen source in corn production. Agron. J. 99, 1119–1129. doi: 10.2134/agronj2006.0251
Lou, X. F., Nair, J., and Ho, G. (2012). Field performance of small scale anaerobic digesters treating food waste. Energy Sustain. Dev. 16, 509–514. doi: 10.1016/j.esd.2012.06.004
Malamis, D., Moustakas, K., Bourka, A., Valta, K., Papadaskalopoulou, C., Panaretou, V., et al. (2015). Compositional analysis of biowaste from study sites in Greek municipalities. Waste Biomass Valorization 6, 637–646. doi: 10.1007/s12649-015-9406-z
Meeske, R., Van der Merwe, G., Greyling, J., and Cruywagen, C. (2002). The effect of the addition of a lactic acid bacterial inoculant to maize at ensiling on silage composition, silage intake, milk production and milk composition. S. Afr. J. Anim. Sci. 32, 263–270.
Meng, Q., Liu, H., Zhang, H., Xu, S., Lichtfouse, E., and Yun, Y. (2022). Anaerobic digestion and recycling of kitchen waste: a review. Environ. Chem. Lett. 20, 1745–1762. doi: 10.1007/s10311-022-01408-x
Mickan, B. S., Ren, A.-T., Buhlmann, C. H., Ghadouani, A., Solaiman, Z. M., Jenkins, S., et al. (2022). Closing the circle for urban food waste anaerobic digestion: the use of digestate and biochar on plant growth in potting soil. J. Clean. Prod. 347:131071. doi: 10.1016/j.jclepro.2022.131071
Mir, M. A., Hussain, A., Verma, C., and Dubey, S. (2016). Design considerations and operational performance of anaerobic digester: a review. Cogent Eng. 3:1181696. doi: 10.1080/23311916.2016.1181696
Moeller, K., and Mueller, T. (2012). Effects of anaerobic digestion on digestate nutrient availability and crop growth: a review. Eng. Life Sci. 12, 242–257. doi: 10.1002/elsc.201100085
Montana Climate Office (2025). The Montana Mesonet Dashboard: Bozeman. Available online at: https://mesonet.climate.umt.edu/dash/acebozem (accessed January 10, 2025).
Montoro, S. B., Lucas, J., Santos, D. F. L., and Costa, M. S. S. M. (2019). Anaerobic co-digestion of sweet potato and dairy cattle manure: a technical and economic evaluation for energy and biofertilizer production. J. Clean. Prod. 226, 1082–1091. doi: 10.1016/j.jclepro.2019.04.148
Mou, J.-H., Qin, Z.-H., Yang, Y.-F., Liu, S.-F., Yan, W., Zheng, L., et al. (2023). Navigating practical applications of food waste valorisation based on the effects of food waste origins and storage conditions. Chem. Eng. J. 468:143625. doi: 10.1016/j.cej.2023.143625
Nghiem, L. D., Koch, K., Bolzonella, D., and Drewes, J. E. (2017). Full scale co-digestion of wastewater sludge and food waste: bottlenecks and possibilities. Renew. Sustain. Energy Rev. 72, 354–362. doi: 10.1016/j.rser.2017.01.062
Niles, M. T. (2020). Majority of rural residents compost food waste: policy and waste management implications for rural regions. Front. Sustain. Food Syst. 3:123. doi: 10.3389/fsufs.2019.00123
Ntostoglou, E., Khatiwada, D., and Martin, V. (2021). The potential contribution of decentralized anaerobic digestion towards urban biowaste recovery systems: a scoping review. Sustainability 13:13435. doi: 10.3390/su132313435
O'Connor, J., Mickan, B. S., Gurung, S. K., Siddique, K. H. M., Leopold, M., and Bolan, N. S. (2023). Enhancing nutrient recovery from food waste anaerobic digestate. Bioresour. Technol. 390:129869. doi: 10.1016/j.biortech.2023.129869
O'Connor, J., Mickan, B. S., Rinklebe, J., Song, H., Siddique, K. H. M., Wang, H., et al. (2022). Environmental implications, potential value, and future of food-waste anaerobic digestate management: a review. J. Environ. Manage. 318:115519. doi: 10.1016/j.jenvman.2022.115519
O'Connor, S., Ehimen, E., Pillai, S. C., Black, A., Tormey, D., and Bartlett, J. (2021). Biogas production from small-scale anaerobic digestion plants on European farms. Renew. Sustain. Energy Rev. 139:110580. doi: 10.1016/j.rser.2020.110580
Oksanen, J., Blanchet, F. G., Friendly, M., Kindt, R., Legendre, P., McGlinn, D., et al. (2018). Vegan: Community Ecology Package. R Package Version 2.5-3.
Pilarska, A., Wolna-Maruwka, A., Niewiadomska, A., Pilarski, K., Adamski, M., Grzyb, A., et al. (2021). Silica/Lignin carrier as a factor increasing the process performance and genetic diversity of microbial communities in laboratory-scale anaerobic digesters. Energies 14:4429. doi: 10.3390/en14154429
Platt, B., and Goldstein, N. (2014). State of composting in the U.S. BioCycle Magazine. Available online at: https://www.biocycle.net/state-of-composting-in-the-u-s/ (accessed January 8, 2025).
R Core Team (2023). R: A Language and Environment for Statistical Computing. R Foundation for Statistical Computing. Vienna: R Foundation for Statistical Computing.
Rachal, M., Rosengren, C., and Himmel, J. (2022). Mapping Zero Waste Cities: Where Local Governments Are Pursuing Waste Prevention and Diversion. Available online at: https://www.wastedive.com/news/zero-waste-cities-us-goal-tracker/635401/?epa-regions=region-8 (accessed August 8, 2024).
Rajendran, K., Aslanzadeh, S., and Taherzadeh, M. J. (2012). Household biogas digesters—a review. Energies 5, 2911–2942. doi: 10.3390/en5082911
Ren, A.-T., Abbott, L. K., Chen, Y., Xiong, Y.-C., and Mickan, B. S. (2020). Nutrient recovery from anaerobic digestion of food waste: impacts of digestate on plant growth and rhizosphere bacterial community composition and potential function in ryegrass. Biol. Fertil. Soils 56, 973–989. doi: 10.1007/s00374-020-01477-6
Rivera, J. R., and Hensel, J. (2009). Manual práctico de agricultura orgánica y panes de piedra. Jairo Restrepo Rivera. Available online at: https://www.academia.edu/download/31484546/Manual-Practico-de-Agricultura-Organica-y-Panes-de-Piedra.pdf (accessed April 15, 2025).
Riviere, D., Desvignes, V., Pelletier, E., Chaussonnerie, S., Guermazi, S., Weissenbach, J., et al. (2009). Towards the definition of a core of microorganisms involved in anaerobic digestion of sludge. ISME J. 3, 700–714. doi: 10.1038/ismej.2009.2
Scarlat, N., Dallemand, J.-F., and Fahl, F. (2018). Biogas: developments and perspectives in Europe. Renew. Energy 129, 457–472. doi: 10.1016/j.renene.2018.03.006
Schafer, D. W., and Ramsey, F. L. (2003). Teaching the craft of data analysis. J. Statistics Educ. 11. doi: 10.1080/10691898.2003.11910692
Schoeber, M., Rahmann, G., and Freyer, B. (2021). Small-scale biogas facilities to enhance nutrient flows in rural Africa—relevance, acceptance, and implementation challenges in Ethiopia. Org. Agric. 11, 231–244. doi: 10.1007/s13165-020-00329-9
Sharma, P., Tiong, Y. W., Yan, M., Tian, H., Lam, H. T., Zhang, J., et al. (2023). Assessing Stachytarpheta jamaicensis (L.) Vahl growth response and rhizosphere microbial community structure after application of food waste anaerobic digestate as biofertilizer with renewable soil amendments. Biomass Bioenergy 178:106968. doi: 10.1016/j.biombioe.2023.106968
Siddiqua, A., Hahladakis, J. N., and Al-Attiya, W. A. K. A. (2022). An overview of the environmental pollution and health effects associated with waste landfilling and open dumping. Environ. Sci. Pollut. Res. 29, 58514–58536. doi: 10.1007/s11356-022-21578-z
Slopiecka, K., Liberti, F., Massoli, S., Bartocci, P., and Fantozzi, F. (2022). Chemical and physical characterization of food waste to improve its use in anaerobic digestion plants. Energy Nexus 5:100049. doi: 10.1016/j.nexus.2022.100049
Song, S., Lim, J. W., Lee, J. T. E., Cheong, J. C., Hoy, S. H., Hu, Q., et al. (2021). Food-waste anaerobic digestate as a fertilizer: the agronomic properties of untreated digestate and biochar-filtered digestate residue. Waste Manag. 136, 143–152. doi: 10.1016/j.wasman.2021.10.011
Stoknes, K., Scholwin, F., Krzesiński, W., Wojciechowska, E., and Jasińska, A. (2016). Efficiency of a novel “Food to waste to food” system including anaerobic digestion of food waste and cultivation of vegetables on digestate in a bubble-insulated greenhouse. Waste Manage. 56, 466–476. doi: 10.1016/j.wasman.2016.06.027
Strazzera, G., Battista, F., Andreolli, M., Menini, M., Bolzonella, D., and Lampis, S. (2021). Influence of different household food wastes fractions on volatile fatty acids production by anaerobic fermentation. Bioresour. Technol. 335:125289. doi: 10.1016/j.biortech.2021.125289
Surendra, K., Takara, D., Hashimoto, A. G., and Khanal, S. K. (2014). Biogas as a sustainable energy source for developing countries: opportunities and challenges. Renew. Sustain. Energy Rev. 31, 846–859. doi: 10.1016/j.rser.2013.12.015
Surendra, K. C., Takara, D., Jasinski, J., and Khanal, S. K. (2013). Household anaerobic digester for bioenergy production in developing countries: opportunities and challenges. Environ. Technol. 34, 1671–1689. doi: 10.1080/09593330.2013.824012
Szymkowiak, A., Borusiak, B., Pierański, B., Kotyza, P., and Smutka, L. (2022). Household food waste: the meaning of product's attributes and food-related lifestyle. Front. Environ. Sci. 10:918485. doi: 10.3389/fenvs.2022.918485
Tang, Y., Horikoshi, M., and Li, W. (2016). ggfortify: unified interface to visualize statistical result of popular R packages. R J. 8, 478–489. doi: 10.32614/RJ-2016-060
The Recycling Partnership (2023). Small-Town America's Part in Boosting U.S. Recycling Rates. Available online at: https://recyclingpartnership.org/small-town-america-part-in-boosting-us-recycling-rates/ (accessed August 8, 2024).
Tian, H., Wang, X., Lim, E. Y., Lee, J. T. E., Ee, A. W. L., Zhang, J., et al. (2021). Life cycle assessment of food waste to energy and resources: centralized and decentralized anaerobic digestion with different downstream biogas utilization. Renew. Sustain. Energy Rev. 150:111489. doi: 10.1016/j.rser.2021.111489
Tiong, Y. W., Sharma, P., Xu, S., Bu, J., An, S., Foo, J. B. L., et al. (2024a). Enhancing sustainable crop cultivation: the impact of renewable soil amendments and digestate fertilizer on crop growth and nutrient composition. Environ. Pollut. 342:123132. doi: 10.1016/j.envpol.2023.123132
Tiong, Y. W., Tian, H., Sharma, P., Yan, M., Lam, H. T., Zhang, J., et al. (2024b). Real-world assessment of a decentralized food waste anaerobic digestion system: a test-bedding case study application. J. Clean. Prod. 437:140752. doi: 10.1016/j.jclepro.2024.140752
Treichel, H., Fongaro, G., and Challenges, T. (2019). Improving Biogas Production. Springer,. doi: 10.1007/978-3-030-10516-7
US Census Bureau (2024). International Database (IDB). Washington, DC: U.S. Census Bureau International Programs Center. Available online at: https://www.census.gov/data-tools/demo/idb/#/dashboard (accessed August 22, 2024).
Vaneeckhaute, C., Styles, D., Prade, T., Adams, P., Thelin, G., Rodhe, L., et al. (2018). Closing nutrient loops through decentralized anaerobic digestion of organic residues in agricultural regions: a multi-dimensional sustainability assessment. Resour. Conserv. Recycl. 136, 110–117. doi: 10.1016/j.resconrec.2018.03.027
Vaske, J. J., Beaman, J., and Sponarski, C. C. (2017). Rethinking internal consistency in Cronbach's alpha. Leisure Sci. 39, 163–173. doi: 10.1080/01490400.2015.1127189
Villamil, J. A., Mohedano, A. F., Rodriguez, J. J., and de la Rubia, M. A. (2018). Valorisation of the liquid fraction from hydrothermal carbonisation of sewage sludge by anaerobic digestion. J. Chem. Technol. Biotechnol. 93, 450–456. doi: 10.1002/jctb.5375
Wang, J. (2014). Decentralized biogas technology of anaerobic digestion and farm ecosystem: opportunities and challenges. Front. Energy Res. 2:10. doi: 10.3389/fenrg.2014.00010
Wang, N., Huang, D., Zhang, C., Shao, M., Chen, Q., Liu, J., et al. (2021). Long-term characterization and resource potential evaluation of the digestate from food waste anaerobic digestion plants. Sci. Total Environ. 794:148785. doi: 10.1016/j.scitotenv.2021.148785
Ward, A. J., Hobbs, P. J., Holliman, P. J., and Jones, D. L. (2008). Optimisation of the anaerobic digestion of agricultural resources. Bioresour. Technol. 99, 7928–7940. doi: 10.1016/j.biortech.2008.02.044
Warne, T., Ebel, R., and Ahmed, S. (2023). Nourishing student success and wellbeing: unveiling the impact of food environments on student food security challenges through a case study from Montana. J. Agricult. Food Syst. Community Dev. 13, 313–333. doi: 10.5304/jafscd.2023.131.017
Xiao, Y., Zan, F., Zhang, W., and Hao, T. (2022). Alleviating nutrient imbalance of low carbon-to-nitrogen ratio food waste in anaerobic digestion by controlling the inoculum-to-substrate ratio. Bioresour. Technol. 346:126342. doi: 10.1016/j.biortech.2021.126342
Xu, F., Li, Y., Ge, X., Yang, L., and Li, Y. (2018). Anaerobic digestion of food waste - challenges and opportunities. Bioresour. Technol. 247, 1047–1058. doi: 10.1016/j.biortech.2017.09.020
Zhai, N., Zhang, T., Yin, D., Yang, G., Wang, X., Ren, G., et al. (2015). Effect of initial pH on anaerobic co-digestion of kitchen waste and cow manure. Waste Manage. 38, 126–131. doi: 10.1016/j.wasman.2014.12.027
Keywords: biofertilizer, biodigester, Home Biogas, decentralized anaerobic digestion, food waste composition
Citation: Ebel R, Eberly J, Grimberg BI, Seipel T and Menalled FD (2025) Household-scale anaerobic digestion of food waste—a community case study from Bozeman, Montana. Front. Sustain. Food Syst. 9:1561457. doi: 10.3389/fsufs.2025.1561457
Received: 15 January 2025; Accepted: 30 April 2025;
Published: 21 May 2025.
Edited by:
José Martinez, Institut National de recherche pour l'agriculture, l'alimentation et l'environnement (INRAE), FranceReviewed by:
Tsubasa Okaze, Institute of Science Tokyo, JapanBaranitharan Ethiraj, Saveetha University, India
Copyright © 2025 Ebel, Eberly, Grimberg, Seipel and Menalled. This is an open-access article distributed under the terms of the Creative Commons Attribution License (CC BY). The use, distribution or reproduction in other forums is permitted, provided the original author(s) and the copyright owner(s) are credited and that the original publication in this journal is cited, in accordance with accepted academic practice. No use, distribution or reproduction is permitted which does not comply with these terms.
*Correspondence: Roland Ebel, cm9sYW5kLmViZWxAbW9udGFuYS5lZHU=