- 1Moon (Guangzhou) Biotech Co., Ltd., Guangzhou, China
- 2MoreMeat (Guangzhou) Biotech Co., Ltd., Guangzhou, China
Introduction: Alternative protein sources are urgently needed to address the environmental, ethical, and health challenges associated with traditional livestock-derived proteins. Mycoprotein, produced by fermenting filamentous fungi, is a high - quality option with meat - like texture, high protein content, rapid growth, low cost, and environmental sustainability, showing great potential to replace conventional proteins. However, both the research endeavors in the field of mycoprotein and the strain resources employed for mycoprotein production remain relatively scarce.
Methods: In this study, we identified Fusarium compactum MM-135, which was isolated from a humus soil sample in Shennongjia, China. This strain was recognized as a high-performing mycoprotein producer through rapid screening of over 270,000 microbial samples. The protein, fiber, fat, and allergen levels of the mycelium were analyzed biochemically. True protein digestibility (TD) and Protein Digestibility-Corrected Amino Acid Score (PDCAAS) were assessed according to AOAC Official Method 991.29 and FAO/WHO guidelines. Safety evaluations included mycotoxin analysis via HPLC-MS and toxicological tests (Ames, in vivo micronucleus, in vitro chromosome aberration) following the OECD Guidelines. A 14-day dietary study in rats evaluated potential adverse effects at intake levels up to 150,000 ppm, following a modified study protocol and relevant SOPs based on the OECD Guidelines.
Results: The strain produces mycelium with over 50% protein content, high fiber, low fat, and no allergens. It exhibits TD of 90.79% and a PDCAAS value of 1.00 for children aged 3-10 years and adults. Safety assessments revealed undetectable levels of most mycotoxins. The mycoprotein showed no mutagenicity or genotoxic effects in the Ames assay, in vivo mammalian erythrocyte micronucleus test, and in vitro mammalian chromosome aberration test. A 14-day dietary exposure study in rats demonstrated no adverse effects at intake levels up to 150,000 ppm, providing a strong basis for ongoing 90-day extended toxicity studies.
Discussion: This study highlights F. compactum MM-135 as a viable and sustainable protein source for future food systems, offering a robust solution to meet global protein demands while mitigating environmental impacts.
1 Introduction
With the global population projected to reach 10 billion by 2050, the demand for sustainable and nutritious protein alternatives is rapidly increasing (Onwezen et al., 2021). Traditional protein sources, particularly those derived from livestock, face significant challenges related to environmental impact, animal welfare, and health concerns associated with excessive animal protein consumption. As a result, there has been a surge of interest in alternative protein sources, including plant-based proteins, algae, insects, and fungi (Mishyna et al., 2021; Onwezen et al., 2021; Malek and Umberger, 2023; Lee et al., 2024). However, most plant proteins, except for soy protein, have unbalanced amino acid profiles, particularly lacking in lysine (Dimina et al., 2021; Tang et al., 2024). Their digestibility is generally below 90%, with some as low as 60–70%, far lower than that of the digestibility of animal proteins (usually above 95%) (Tang et al., 2024). Additionally, plant-based protein products often fail to simulate the fibrous structure and texture of meat. The processing equipment for plant proteins is also complex, costly, and faces technical bottlenecks (Timothy and Knob, 2020). Algae protein production requires complex harvesting and dehydration processes, which are costly and energy intensive. In their raw form, algae proteins are difficult to digest and absorb, necessitating extraction processes to enhance bioavailability (Ijaola et al., 2024). The relative digestibility of algae proteins is currently around 56%. Insect protein, mainly sourced from crickets, mealworms, and locusts, has issues with taste, appearance, and odor that do not meet consumer expectations. Many consumers, due to cultural background and traditional perceptions of insects, have psychological barriers to consuming insect protein (Lin et al., 2025). Moreover, allergens, natural toxins, heavy metals, and pesticide residues in insect protein significantly affect its acceptability (Traynor et al., 2024).
Fungal proteins, primarily sourced from yeasts, molds, and larger edible fungi, have high protein content (over 40% dry weight) and balanced amino acid profiles (Derbyshire et al., 2023; Lee et al., 2024). Certain fungal proteins and peptides offer health benefits such as immune modulation, cholesterol reduction, and antioxidant properties (Li et al., 2023; Pavis et al., 2024). Mycoprotein, produced by the fermentation of filamentous fungi, has significant comprehensive advantages (Derbyshire and Keith-Thomas, 2019). Compared to traditional plant proteins, mycoprotein has a fibrous texture similar to meat, a neutral taste, and is easy to process into a variety of meat and dairy alternatives. In contrast to yeast proteins, it offers superior taste and processing properties, with a broader range of product development possibilities. Nutritionally, mycoprotein is rich in essential amino acids, minerals, and dietary fiber, with low fat content, no cholesterol, and high digestibility, comparable to whey protein. It even shows superior performance in promoting muscle growth and lowering cholesterol levels (Pavis et al., 2024). In addition, mycoprotein can be produced through automated, intelligent, and large-scale processes, characterized by high growth rates and fermentation yields (Wang et al., 2023). With a short fermentation cycle (typically completed within 30 h), its production efficiency is thousands of times higher than that of traditional livestock and crop farming, with costs comparable to plant-based proteins and further potential for cost reduction. Therefore, the production of mycoprotein is cost-effective, requiring minimal land and water resources, and results in low carbon emissions (Sillman et al., 2020; Tong et al., 2024).
Currently, the main strains used to produce mycoproteins include Paecilomyces variotii, Aspergillus oryzae, Rhizopus oligosporus, Fusarium venenatum, Fusarium flavolapis and Flammulina velutipes (Muhammad Ijaz Ahmad et al., 2022). Fusarium venenatum is the most commonly used species for commercial mycoprotein production. It is a harmless soil fungus first discovered in a garden in the UK in the 1960s. Through fermentation technology, mycoprotein from F. venenatum is produced on a large scale, with its fibrous structure simulating the texture of meat and widely used in meat alternatives. It has been sold by Quorn® in the UK since January 1985, with sales expanding to other European countries starting in 1991. It has been consumed as a meat alternative in 17 countries for over 30 years, with an estimated 5 billion servings (Wiebe, 2002; Finnigan et al., 2019). Another Fusarium species used for commercial mycoprotein production is Fusarium flavolapis, which was initially discovered in a hot spring in Yellowstone National Park. Nature’s Fynd uses this species to produce mycoprotein products like Quorn®. Nature’s Fynd’s mycoprotein was granted Generally Recognized as Safe (GRAS) status in the USA in 2021. It subsequently received novel food approval from Health Canada in 2023 and obtained premarket approval from the Food Safety and Standards Authority of India (FSSAI) in 2024 (Chi, 2024). Currently, research on mycoprotein is relatively limited, and the strain resources available for mycoprotein production are also restricted. Specifically, Fusarium strains with natural high—protein production capabilities are extremely scarce. With the advancement of technology and the growth of market demand, the exploration of other Fusarium species for mycoprotein production potential is underway.
In this study, we identified Fusarium compactum as a potential producer of mycoprotein. A rapid screening technique was developed to evaluate 7,000 strains from 200 Fusarium species in a microbial library of over 270,000 strains. The strain MM-135 of Fusarium compactum was selected for its high protein content (over 50% in dry base) and rapid growth. The mycoprotein produced by MM-135 exhibited a complete amino acid profile, high fiber content, low fat, and no allergens. PDCAAS analysis revealed a TD of 90.79% and a score of 1.00 for both children (3–10 years) and adults (≥18 years). Safety assessments included mycotoxin testing, which detected enniatin and beauvericin within acceptable limits, and no genotoxic potential. Toxicological evaluations, including Ames tests, in vivo micronucleus tests, and in vitro chromosome aberration tests, showed no mutagenic or genotoxic effects. A 14-day dietary exposure study in rats demonstrated no adverse effects at intake levels up to 150,000 ppm, laying the groundwork for ongoing 90-day extended toxicity studies. Additionally, mycoprotein from F. compactum MM-135 has achieved self-affirmed GRAS status for use as a food ingredient and macronutrient through scientific procedures in 2024. These results indicate that mycoprotein from F. compactum MM-135 has the potential for safe use in food applications.
2 Materials and methods
2.1 Material preparation
The raw materials used for mycoprotein fermentation are commonly used in the food, fermentation, and enzyme production industries and are all food-grade. Each batch was inspected according to internal procedures and accompanied by a certificate of analysis from the supplier. The production strain is inoculated into seed culture medium and enlarged by two runs of seed culturing. The first run is conducted in a 50-mL bioreactor, and the second run is then conducted in a 1,000-mL bioreactor, respectively. The seed culture medium includes glucose (Lihua Starch) as major carbon source, yeast extract (Angel Yeast, FM902) as major nitrogen source, as well as potassium dihydrogen phosphate (Zhixin Chemical), magnesium sulfate (Kelunduo Food), and calcium chloride (Kelunduo Food). Subsequently, culture broth was inoculated into the fermentation medium at a ratio of 5% of the fermentation medium volume. The fermentation medium primarily consists of the following components: 20 g/L corn starch (Lihua Starch), 3 g/L ammonium sulfate (Kelunduo Food), 5 mL/L ammonia solution (Qingjia Chemical), 3 g/L citric acid (Jinheboyuan Biochemical), 2 g/L potassium dihydrogen phosphate, 0.5 g/L magnesium sulfate, 0.03 g/L calcium chloride, and 0.5 mg/L biotin (Baixing Biotechnology). The medium was filtered through a 60-mesh sieve to remove impurities and sterilized at 121°C for 30 min. Biotin was sterilized by filtration through a 0.22-μm filter membrane (Jetbiofil, FMC201030) prior to being added to the fermentation medium. Under sterile conditions in a biological hood, the production strain master and working banks were prepared and tested for microbial purity by plating and observing the colony morphology under a microscope (Olympus, CX33). The mycelium obtained through cultivation on solid medium plates were then inoculated into a cooled fermentation medium. Fermentation was conducted in a closed system at 28°C with an agitation speed of 250 rpm, a ventilation rate of 0.5 VVM (volume per volume per minute), and a pH of 5.0, using the fermentation tank system (Saiduo Biology, China, model SD/F-1000 L). Post-fermentation, the fermentation broth is transferred to a heat treatment tank and is treated at 80°C for 30 min to inactivate F. compactum and enzymes while also reducing the RNA content. After heat treatment, the fermentation broth was filtered with vacuum to remove excess water and cool the product, and most of the residues of the raw material are removed in this process. Mycoprotein was then weighed and packaged in an aluminum foil bag, sealed under vacuum, and stored at −18°C before use.
2.2 Biomass and crude protein content determination
Mycelium was activated and inoculated into flasks containing potato dextrose broth (PDB) medium (HuanKai Microbial, 021053) and cultured at 200 rpm for 24–30 h at 28°C. After culturing, the fermentation broth was filtered through Whatman No. 1 filter paper to separate the mycelium, which was then thoroughly washed with distilled water and collected. The mycelium was dried at 60°C until a constant weight was achieved, and the dry weight was measured to determine the biomass. The biomass was calculated as the mass-to-volume ratio. The crude protein content was subsequently determined using the Kjeldahl method (Kjeldahl, 1883).
2.3 Identification of F. compactum
The identification of F. compactum was conducted using the Polyphasic identification method available on the FUSARIOID-ID online database (fusarium.mycobank.org; access date: May 6th, 2024). Relying solely on a single cloned sequence for identification or phylogenetic analysis can result in discrepancies, especially for fungal species. Therefore, utilizing multiple identification sequences is essential. The translation elongation factor I (TEF1), RNA polymerase I (RPB1), RNA polymerase II (RPB2), and internal transcribed spacer region (ITS) genes are commonly employed gene fragments in fungal systematics studies. For F. compactum MM-135, sequences of these four genes were up-loaded to the database, and the identification was performed using the ITS, TEF1, RPB1, and RPB2 sequences simultaneously.
To determine the evolutionary position of F. compactum MM-135, A multigene phylogenetic tree was con-structed. The sequences of the TEF1, RPB1, RPB2, and ITS genes from multiple strains of various subspecies within the Fusarium genus from the FUSARIOID-ID database1 were collected. The multigene phylogenetic tree analysis was conducted as follows: Multiple sequence alignments for each gene were performed using MAFFT (version 7.310). The aligned sequences were then refined using GBLOCKS (version 0.91b) to remove poorly aligned positions, followed by manual curation using AliView. The curated sequences of the four genes were subsequently concatenated. Finally, a multigene phylogenetic tree was constructed using IQ-TREE (version 2.2.5) based on the concatenated sequences. The resulting tree was visualized using ggtree (version 3.10.0).
2.4 Composition tests
Mycoprotein from F. compactum MM-135 was prepared as described in 2.1 material preparation and then three batches were tested for its composition with the methods shown in tables. The typical nutritional profile in mycoprotein from F. compactum MM-135 was conducted by Eurofins Technology Service (Guangzhou) Co., Ltd. (Guangzhou, China) using appropriate AOAC shown in Table 1. The amino acids in mycoprotein from F. compactum MM-135 were tested by SGS-CSTC Standards Technical Services Co., Ltd. (Guangzhou Branch, China) using GB 5009.124 and GB/T 18246 (Table 2). Food safety microbiological testing in mycoprotein from F. compactum MM-135 was tested by SGS-CSTC Standards Technical Services Co., Ltd. (Guangzhou Branch, China) using appropriate methods in USP General Chapter 61, 62 < 2022, 2023>, FDA BAM Chapter 5, FDA BAM Chapter 10 and internal methods (Supplementary Table S1). Heavy metals in mycoprotein from F. compactum MM-135 were tested by SGS-CSTC Standards Technical Services Co., Ltd. (Guangzhou Branch, China) using AOAC method (Supplementary Table S2) and mycotoxins were determined by Romer Labs Analytical Service (Wuxi) Ltd. (Wuxi, China) with the methods shown in Table 3.
2.5 Protein digestibility-corrected amino acid score (PDCAAS)
The study was conducted at Centre Testing International (Guangzhou) Co., Ltd. (Guangzhou, China). All animal experiments were carried out according to protocols approved by the Institutional Animal Care and Use Committee of Centre Testing International (Guangzhou) Co., Ltd., in compliance with the Guide for the Care and Use of Laboratory Animals. The research followed a modified study protocol and relevant SOPs based on AOAC Official Method 991.29 True Protein Digestibility of Foods and Food Ingredients. Eighteen weaned SD rats (21–30 days old, weighing 45–60 g) were purchased from the Guangdong Medical Laboratory Animal Center (Guangzhou, China). The rats were housed in rat cages at a room temperature of 18–26°C, relative humidity of 40–70%, and a 12-h light/dark cycle. After a 5-day quarantine period, the SD rats were randomly divided into three groups according to body weight (n = 6/group) using a random ranking table method, to ensure there were no statistically significant differences in the average body weight among the groups: a standard control (casein) group, a negative control (protein free) group, and a sample group, with six rats in each group. The custom diet for the standard control group used casein as the protein source, the custom diet for the negative control group contained no protein, and the custom diet for the sample group used the protein from the sample as the protein source, with the same nutritional components. The test diets and the positive control (casein) diet were formulated to contain 10% protein (N × 6.25) and levels of vitamins, minerals and calories to satisfy the requirements of the rats. The negative control (protein free) diet was formulated to contain the same levels of all nutrients except protein (e.g., protein was replaced with cornstarch). Diets were custom-made by the Guangdong Provincial Medical Laboratory Animal Center.
During the experimental period, the rats were housed in metabolic cages to allow for the weighing of feed and collection of feces. Each group was fed 15 grams per day of the corresponding custom diets till the end of the study and had free access to water, with daily weighing. Daily feed intake (the amount of feed added and the remaining amount) was measured. For the balance period of the study, feces were collected every 24 h. At the end of the balance period, the feces from each animal were mixed, dried, and analyzed for nitrogen content. The nitrogen content in the feces was determined by the Kjeldahl method. The TD was calculated using the following formula:
where Ni is the average nitrogen intake (g) of the animals in the sample group or the standard control group, Fn is the average fecal nitrogen excretion (g) of the animals in the sample group or the standard control group, and Mn is the average fecal nitrogen excretion (endogenous nitrogen content) of the animals in the negative control group.
Amino Acid Score (AAS) was calculated using the following formula:
Amino Acid Score (AAS) = [amino acid content in the sample protein (mg/g)]/[corresponding essential amino acid content in FAO/WHO scoring standard model (mg/g)]. Then, PDCAAS was calculated using the formula: PDCAAS = AAS × TD (%).
2.6 Bacterial reverse mutation (Ames) assay
To assess the mutagenic potential of mycoprotein, an Ames assay was conducted by Shanghai Municipal Center for Disease Control and Prevention (Shanghai, China) which is compliance with OECD principles of Good Laboratory Practice. The procedures followed were in accordance with the OECD Guideline for the Testing of Chemicals No. 471, specifically, the Bacterial Reverse Mutation Test from 2020. The assay utilized plate incorporation and pre-incubation methods to evaluate the mutagenic potential of a test substance on Salmonella typhimurium strains TA97a, TA98, TA100, TA102, and TA1535, all of which are histidine dependent. The evaluation was conducted with and without metabolic activation using a chemical-induced rat liver S9 mix.
The pre-experiment with plate incorporation method was conducted with mycoprotein at dose levels of 15.8,50,158,500, 1,580 and 5,000 μg/plate, serious precipitate occurred in the 5,000 μg/plate. For the confirmatory test, a preincubation modification of the plate incorporation method was used, and the highest concentration of the test substance is 1,580 μg/plate. In this approach, the test or control substances were first incubated with the bacterial suspension and S9/substitution buffer for approximately 30 min at approximately 37°C with gentle shaking to ensure thorough mixing. After the pre-incubation period, the mixture was combined with overlay agar and poured on minimal agar plates. The plates were handled in the same manner as in the initial test, with incubation at approximately 37°C until adequate growth was observed. The confirmatory test maintained the same study design as the initial test, including the use of the same bacterial strains and number of replicates. The highest concentration of the test substance in the confirmatory test was also 1,580 μg/plate, followed by lower concentrations of 316, 63.2, 12.6 and 2.5 μg/plate.
2.7 In vivo mammalian erythrocyte micronucleus test in mice
The mammalian erythrocyte micronucleus test for mycoprotein from F. compactum MM-135 was conducted according to the OECD Guideline for the Testing of Chemicals-No. 474 mammalian erythrocyte micronucleus test (2016) by Shanghai Municipal Center for Disease Control and Prevention which is compliance with OECD principles of Good Laboratory Practice. Briefly, 50 specific-pathogen-free (SPF) Kunming mice, 6 weeks of age and with an equal sex distribution, were used in the experiment. The mice weighed between 25 and 27 g each. They were randomly allocated into five groups based on their body weight: three groups for testing different doses of the substance, one negative control group, and one positive control group. Animals were weighed and the prepared test solutions of different concentrations and the positive control solution were administered to each group of animals at a dose of 10 mL/kg body weight. Distilled water was administered to the negative control group via gavage. The test substance was administered to the animals for 2 days (with a 24-h interval). After the last administration, the animals were sacrificed, and the bone marrow from both femurs was removed, mixed with calf serum, and homogenized. Routine smears were prepared, fixed with methanol, and stained with Giemsa. Micronuclei in immature erythrocytes were observed under a microscope (ECLIPSE 80i, Nikon, Japan) and the number of micronucleated cells were counted.
2.8 In vitro mammalian chromosome aberration test
The in vitro mammalian chromosome aberration test of mycoprotein was conducted by Shanghai Municipal Center for Disease Control and Prevention following the OECD principles of Good Laboratory Practice and OECD Guideline No. 473. Chinese hamster lung cells (CHL, purchased from Wuhan Prosel Life Science and Technology Co., Ltd.) were digested with 0.25% Trypsin–EDTA and prepared into a 1 × 10^6/mL suspension with MEM culture medium containing 10% fetal bovine serum. Each 25 mL culture bottle was inoculated with 1 mL cell suspension, supplemented with 4 mL culture medium, and incubated at 37°C with 5% CO2 for 24 h. After 24 h, the medium was replaced with 500 μL test solution, 0.5 mL S9 mixture (or serum-free MEM medium if no activation was needed), and serum-free MEM to a total of 5 mL, then incubated for 4 h with a negative control. Mitomycin C (0.25 μg/mL) and cyclophosphamide (20 μg/mL) served as positive controls for tests with and without the S9 mix. For mycoprotein from F. compactum MM-135, without S9, concentrations were 2,500 μg/mL (high), 1,250 μg/mL (mid), and 625 μg/mL (low). With S9, concentrations were 5,000 μg/mL (high), 2,500 μg/mL (mid), and 1,250 μg/mL (low). Post-incubation, cells were washed, re-cultured in 5 mL MEM with 10% fetal bovine serum for 24 h, then digested, stained, and assessed for RICC (Relative Increase in Cell Count) and cytotoxicity. Additionally, a 24-h test was conducted with 0.1 μg/mL mitomycin C as a positive control, and doses of 1,250, 625, and 312.5 μg/mL were used for continuous treatment.
2.9 14-day dietary toxicity study in rats
The study was conducted at Medicilon Preclinical Research (Shanghai) LLC. The research followed a modified study protocol and relevant SOPs based on OECD Guidelines for Testing of Chemicals and Food Ingredients, Section 4 (Part 407): Health Effects, Repeated Dose 28-Day Oral Toxicity Study in Rodents (2008), and US FDA Redbook 2000, IV.C. 4. a. (2007). Forty SPF Sprague–Dawley rats (20 males and 20 females, purchased from Zhejiang Vital River Laboratory Animal Technology Co., Ltd.) were randomly divided into four groups (5/sex/group) and fed diets containing 0, 50,000, 100,000, and 150,000 ppm of mycoprotein. During acclimation, cage-side observations were conducted twice daily, with detailed clinical observations once. Body weights were measured on Days −5 and −1. During the experiment, twice-daily cage-side observations (morning and afternoon) were conducted, with detailed clinical observations once daily. Rats were weighed on Days 3, 7, 10, 14, and 15 (pre-necropsy). Food consumption was measured daily, and food efficiency was calculated as Food efficiency = (Mean Daily Body Weight Gain) / (Mean Daily Food consumption). Clinical pathology testing was performed on Day 15 for all groups, with blood samples collected by abdominal aortic puncture for hematology, coagulation, and serum biochemistry analysis. Necropsy and gross observation were performed on Day 15, with tissues and organs collected, weighed, and fixed for pathological examination. Data from different genders within each group were analyzed separately. Raw data were tabulated and mean, and standard deviation/category changes were calculated by group and sex. Treatment groups were compared with the control group using pair-wise comparisons for all indicators except leukocyte count, which was analyzed using log transformation of group pair-wise comparisons.
2.10 Statistical analysis
Data were presented as mean ± standard deviation (SD). Sample sizes and statistical methods were provided in figure or table legends. GraphPad software (version 8.0.1) or Provantis 10.5.0.4 were used for statistical analyses. The level of statistical significance was set at p < 0.05.
3 Results
3.1 F. compactum MM-135 identification
Based on our previously published research findings about the methods for screening marker proteins or marker genes associated with protein biomass in Fusarium species, along with methodologies for evaluating protein biomass (Patent No. CN118553304A) (Pu et al., 2024), and based on multiple criteria requirements, we screened over 7,000 strains from 200 Fusarium species in a microbial library of over 270,000 strains and ultimately selected one strain demonstrating high protein content, elevated biomass, rapid growth rate, and well-developed mycelial morphology. In detail, following the completion of strain fermentation, a specified volume of the fermentation broth was collected, dried and accurately weighed. The biomass was subsequently calculated as the mass-to-volume ratio. The protein content was determined using the Kjeldahl method. Examination of hyphal morphology was conducted under a microscope for detailed observation. The results revealed that the average biomass and protein content exhibit significant variation among different Fusarium species. Notably, F. compactum MM-135 exhibited high biomass and protein content, with values of 1.89 and 58.12%, respectively (Figure 1). Morphological observations indicate that F. compactum MM-135 cells form filamentous hyphae with separations between cells. Hyphae are 800–1,500 μm long, branched, and produce large conidia that are sickle-shaped or long cylindrical with multiple transverse septa. These hyphae can cross and entangle to form a reticulated structure (Figure 1).
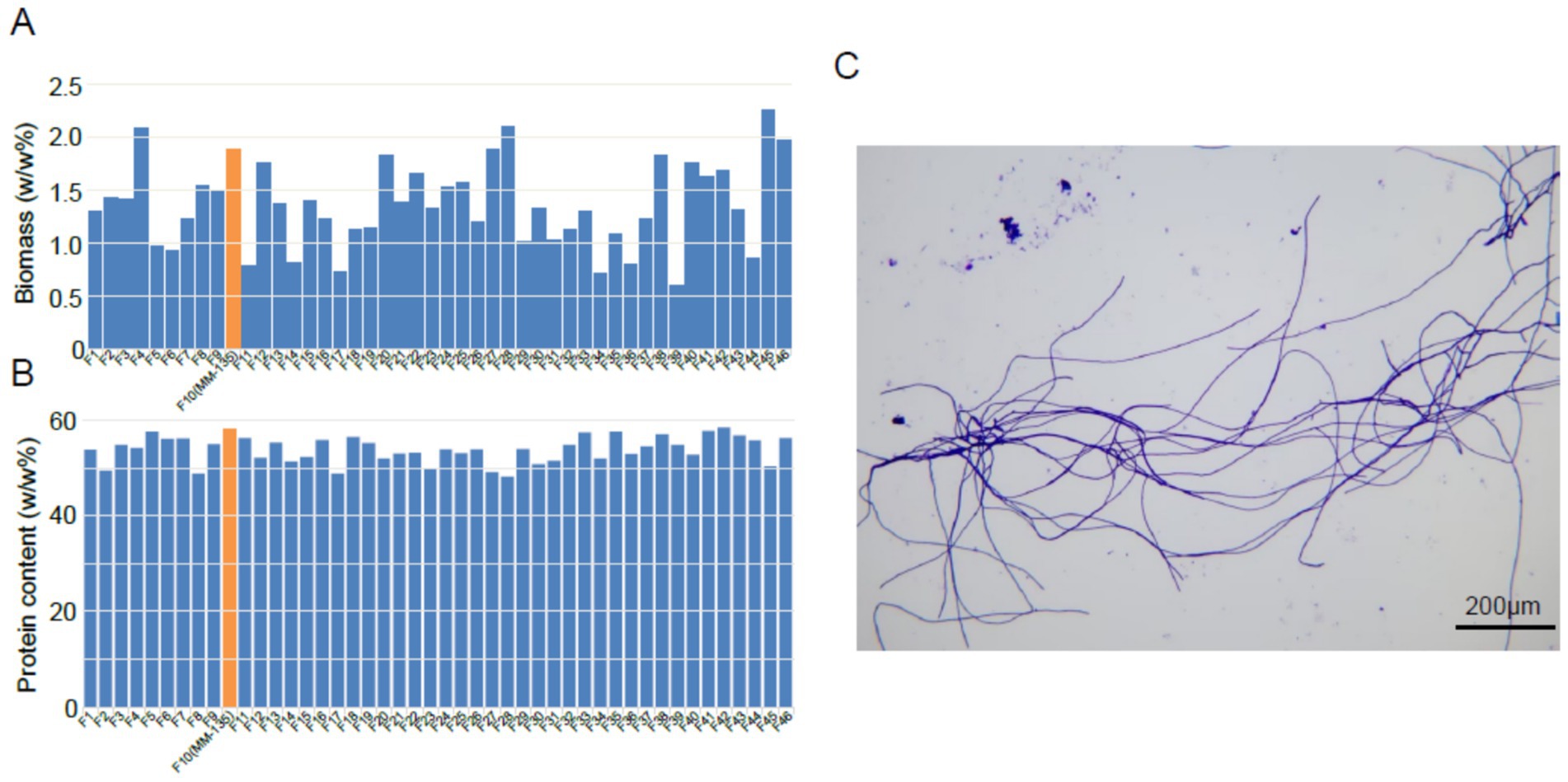
Figure 1. Biomass (A) and crude protein content (B) of Fusarium compactum MM-135 and other Fusarium spp., and microscope image of t (C).
After screening, fermentation optimization, and pilot-scale amplification, we obtained one strain, F. compactum MM-135. Using a single cloned sequence for identification or phylogenetic analysis may lead to sequence discrepancies in fungal species; therefore, multiple identification sequences are necessary. ITS, TEF1, RPB1, and RPB2 are gene fragments commonly used in fungal systematics studies. Consequently, we identified F. compactum MM-135 using the polyphasic identification method provided by FUSARIOID-ID online database (fusarium.mycobank.org, access date: May 6th, 2024), with ITS, TEF1, RPB1, and RPB2 sequences. The sequences of these four genes were uploaded to the database, and the results showed that F. compactum MM-135 was most closely relate to strain F. compactum LLC 2178, with a similarity of 99.82%. The phylogenetic tree in Figure 2 was constructed using genetic data from F. compactum MM-135, 35 additional Fusarium strains, and one outgroup, F. buharicum_CBS_178_35_ET, according to methods (Taylor et al., 2000). The phylogenetic tree and DNA comparison revealed that F. compactum MM-135 has a high genetic relationship with other Fusarium spp., with greater similarity to Fusarium venenatum A 3/5 than to Fusarium flavolapis MK7, based on the comparison of four different gene sequences. Furthermore, the production organism was not genetically modified.
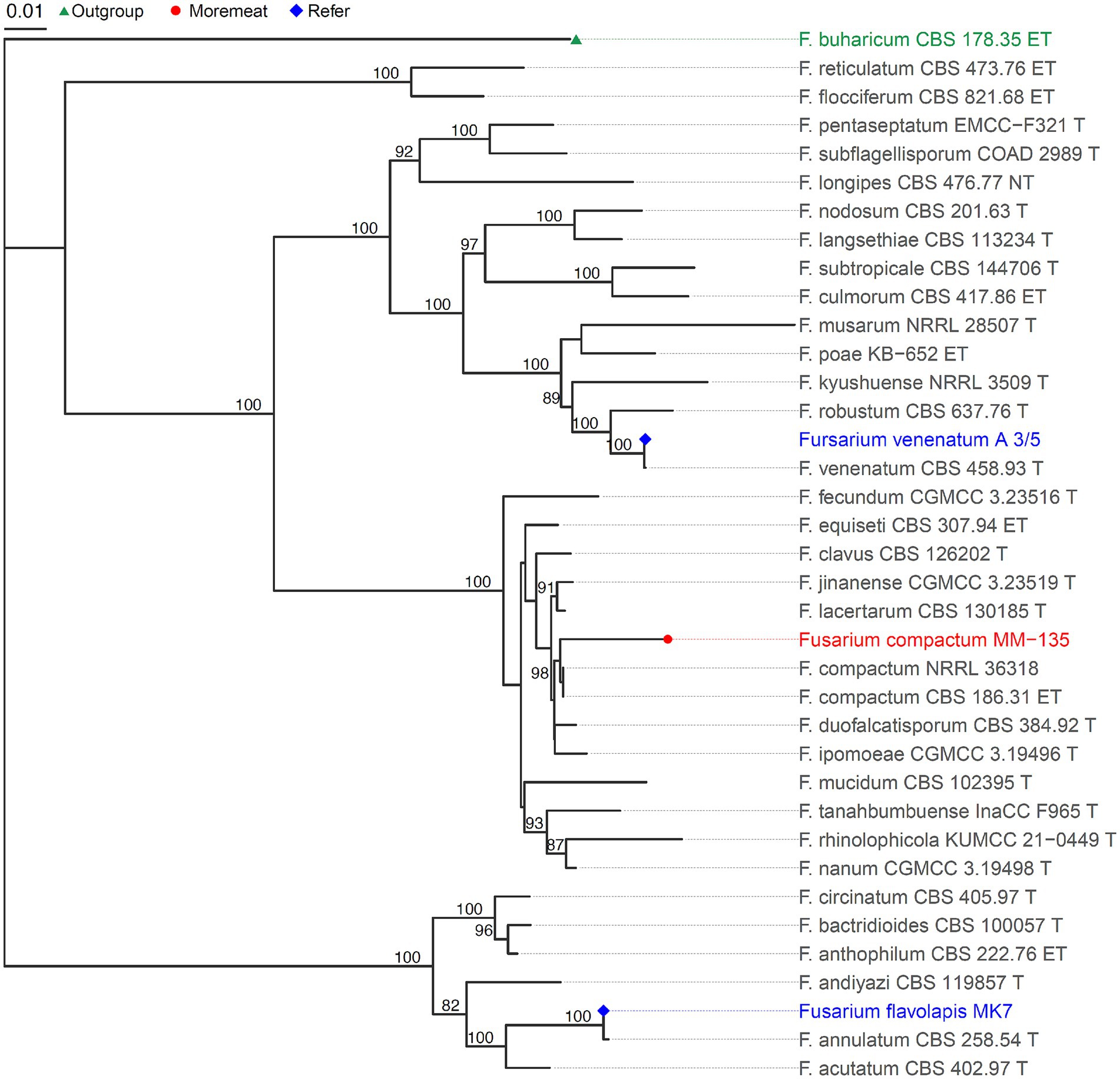
Figure 2. Phylogenetic tree showing relationship between Fusarium compactum MM-135 and other Fusarium spp.
3.2 Composition in mycoprotein from F. compactum MM-135
Nutritional analysis of three batches of mycoprotein derived from F. compactum MM-135 revealed that it contains 13 g protein, 8.1 g dietary fiber, low fat, and nearly no cholesterol per 100 g (Table 1). Amino acid analysis revealed that total amino acids account for 40–50% of the total dry weight, with all nine essential amino acids present, comprising 20–25% of the total dry weight and 40–50% of the total protein content (Table 2). Branched-chain amino acids (leucine, isoleucine, and valine) account for 8–15% of the dry weight (Table 2). Mycoprotein from F. compactum MM-135 is a complete protein source with no trans-unsaturated fatty acids.
Microbiological testing of three batches found no common foodborne pathogens, such as Listeria monocytogenes, Escherichia coli, Staphylococcus aureus, and Salmonella. Total aerobic microbes, yeast, and mold were less than 10 CFU per gram, indicating a suitable production process and high-quality control standards (Supplementary Table S1). Heavy metals testing of three batches showed levels of cadmium, lead, and arsenic were less than 20 μg/kg, and mercury less than 10 μg/kg, demonstrating low contamination (Supplementary Table S2).
Mycotoxin analysis covered items tested in previous mycoprotein GRAS notifications approved by the FDA, and the results showed that nearly all the tested mycotoxins were not detected, except for enniatin and beauvericin, which may originate from contaminants present in the raw material corn starch (Logrieco et al., 1993; Plattner and Nelson, 1994; Krska et al., 1996; Han et al., 2017) (Table 3). However, there are currently no international limits for enniatin and beauvericin, and the European Union (EU) is still in the process of assessment (EFSA Panel on Contaminants in the Food Chain (CONTAM Panel), 2014; Maranghi et al., 2018; EFSA Panel on Contaminants in the Food Chain (CONTAM), 2024). Referring to the NOAEL published by EFSA (Maranghi et al., 2018), the content of these compounds in our product is within a reasonable range. Furthermore, recent research conducted by EFSA has concluded that beauvericin (BEA) does not exhibit genotoxic potential (EFSA Panel on Contaminants in the Food Chain (CONTAM), 2024), with a NOAEL of 0.1 mg/kg b.w. per day, as assessed by EFSA in 2018 (Maranghi et al., 2018). Similarly, the NOAEL for enniatin is 0.18 mg/kg b.w. per day (Maranghi et al., 2018).
3.3 PDCAAS analysis
The PDCAAS method evaluates food protein quality based on human amino acid requirements and digestibility, predicting dietary protein utilization. The results indicated the TD of mycoprotein derived from F. compactum MM-135 was 90.79% with the first limiting amino acids were methionine + cysteine (Table 4). Based on the amino acid requirements of children aged 3–10 years (FAO, 2013), PDCAAS score was 1.00; based on the amino acid requirements of adults aged 18 years and above, PDCAAS score was 1.00 (Table 4). Compared to Fy protein (TD score 89.081, actual PDCAAS score 90.86), mycoprotein derived from F. compactum MM-135 has a comparable nutritional profile and amino acid content, but higher TD and PDCAAS score (Furey et al., 2022). Besides, it demonstrates that mycoprotein is a quality protein similar to other protein sources, such as beef (PDCAAS score 0.92) and pea protein concentrates (PDCAAS score 0.67) (van Vliet et al., 2015).
3.4 Genotoxicity study
3.4.1 Bacterial reverse mutation (Ames) assay
The average number of revertant colonies for each strain treated with the vehicle was within the expected or normal range (Supplementary Table S3), based on the lab’s historical control data or published values (Mortelmans and Zeiger, 2000; Gatehouse, 2012). The positive control chemicals induced a significant increase in revertant colony counts, regardless of whether S9 was used or not. These results confirmed the test’s sensitivity and the effectiveness of the S9 mix. The groups containing 5,000 μg/plate test substance in the pre-experiment exhibited severe precipitation during the experiment, precluding accurate determination. Therefore, these data were not included in the statistical analysis. At least, five different concentrations with the highest concentration of 1,580 μg/plate were tested for all strains, thus adequately evaluating bacterial mutagenicity. There were no concentration-related or substantial increases in revertant colonies with strains TA1535, TA97a, TA98, TA100, or TA102 regardless of whether S9 was used or not, using either the plate incorporation or pre-incubation method. Based on these results and the assessment criteria, mycoprotein derived from. Compactum MM-135 did not demonstrate mutagenic activity in the Ames test.
3.4.2 In vivo mammalian erythrocyte micronucleus test in mice
The in vivo micronucleus assay in mammals was performed to assess the genotoxic potential of mycoprotein from F. compactum MM-135 by detecting any chromosomal damage or effects on the mitotic apparatus. Comparisons of the micronucleated cell frequencies in the treated groups and the negative control revealed no significant differences (Supplementary Table S4). Consequently, mycoprotein from F. compactum MM-135, yielded a negative outcome in the mammalian erythrocyte micronucleus test, indicating no in vivo genotoxicity.
3.4.3 In vitro mammalian chromosome aberration test
Cell cultures were exposed to the test substance for 4 h. Without S9 mixture, the dose resulting in 45% ± 5% relative increase in chromosomal aberrations (RICC) was approximately 2,500 μg/mL. Therefore, the concentrations were 2,500 μg/mL (high), 1,250 μg/mL (middle), and 625 μg/mL (low) without the S9 mixture. With S9 mixture, the dose resulting in 45% ± 5% RICC was above 5,000 μg/mL. Therefore, with S9 mixture, the concentrations were 5,000 μg/mL in the high dosage group, 2,500 μg/mL in the middle dosage group, and 1,250 μg/mL in the low dosage group. Compared with the negative control group, the p value of the mammalian chromosome aberration rate for the high, medium, and low doses of the test sample was greater than 0.05, indicating no significant difference (Supplementary Table S5).
Cell cultures were also exposed to the test substance for 24 h. Without S9 mixture, the dose resulting in 45 ± 5% RICC was 1,250 μg/mL. Therefore, without S9 mixture, the concentrations were 1,250 μg/mL in the high dosage group, 625 μg/mL in the middle dosage group, and 312.5 μg/mL in the low dosage group. Compared with the negative control group, the p value for the mammalian chromosome aberration rate at the high, medium, and low doses were greater than 0.05, indicating no significant differences (Supplementary Table S5). Therefore, based on the in vitro mammalian chromosome aberration test, it is concluded that mycoprotein from F. compactum MM-135 does not exhibit mutagenicity related to clastogenicity and/or aneugenicity.
3.5 14-day dietary toxicity/palatability study in rats
The aim of this preliminary study was to assess the general toxicity of mycoprotein from F. compactum MM-135 in rats after a minimum of 14 days of dietary intake. Over the 14-day period, rats fed diets containing 50,000, 100,000, and 150,000 ppm of mycoprotein had average daily intakes of 3865.83, 8343.23, and 12,150.22 mg/kg/day for males, and 3833.80, 8434.32, and 12,499.29 mg/kg/day for females, respectively. There were no mortalities during the study. Blood biochemical indicators of total cholesterol (TCHO) were slightly decreased in all dose groups (Supplementary Table S7). Because these changes were dose-related and slight, they were considered to be test substance-related. This may be attributed to the fiber content in mycoprotein. Similar cholesterol-lowering effects have been observed in subchronic and chronic animal studies of mycoprotein from F. venenatum (Miller and Dwyer, 2001) and F. flavolapis (Furey et al., 2022), and as well as in human clinical trials (Coelho et al., 2021), all of which explored the dietary inclusion of mycoprotein.
Other test parameters, including clinical observations, body weight, food consumption, food efficiency, clinical pathology, and necropsy observations, showed no test substance-related changes. No clinical manifestations or symptoms associated with mycoprotein were observed in any of the animals. Body weight, daily weight gain, final body weight, food consumption, total food intake, and food efficiency ratio were similar between mycoprotein-treated rats and controls (Figure 3). Gross necropsy and organ weight observations revealed no abnormalities, except for a 14.38% increase in kidney weight in female rats of group 4 (Supplementary Table S6). The animals in group 4 exhibited normal serum biochemical indicators related to kidney function, and the slight increase in kidney weight was only observed during necropsy in female rats. This increase was attributed to individual differences or background changes and was not related to mycoprotein.
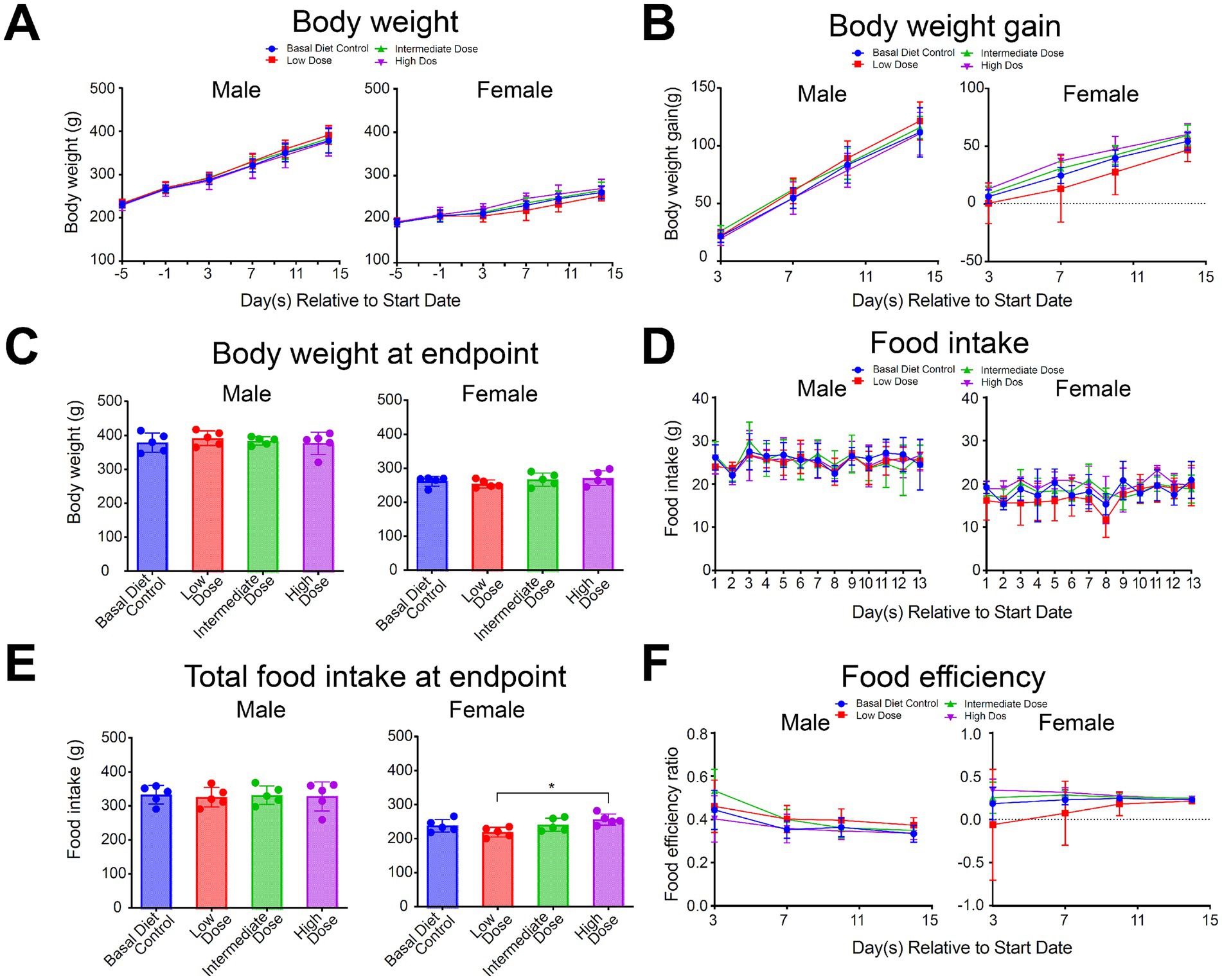
Figure 3. Effect of various doses of mycoprotein from Fusarium compactum MM-135 on (A) Body weight, (B) Body weight gain, (C) Body weight at endpoint, (D) Food intake, (E) Total food intake at endpoint and (F) Food efficiency in various groups (n = 5 per group). All data are expressed as mean ± SD and graphed and statistically analyzed using GraphPad Prism 9 software. Statistical analysis is performed by pairwise comparison using One-Way ANOVA (Dunnett’s multiple comparisons test). For testing two variables, Two-Way ANOVA (Dunnett’s multiple comparisons test) is used. ns: not significant, not shown; *: p < 0.05.
Histopathology of visceral organs revealed that mycoprotein administration from F. compactum MM-135 maintained the normal architecture of these organs, with no abnormalities (such as necrosis, inflammation, or hyperplastic changes) observed. Liver histology showed a normal central vein, bile ducts, hepatic artery/vein, and sinusoids. Kidney histology revealed that the glomerular space and Bowman’s capsule were intact, with normal proximal and distal convoluted tubules. In the spleen, lymphoid follicles and both white and red pulp appeared normal in the groups consuming mycoprotein from F. compactum MM-135. Colon tissue showed normal crypts, goblet cells, and no inflammation (Figure 4). Hematology data showed only a significant increase in MONO% in low-dose females, which was not considered test substance-related since no significant abnormalities were observed in absolute counts, and the changes were not dose-related (Supplementary Table S8). Coagulation indices showed no abnormalities associated with the test substance (Supplementary Table S8). The study concluded that there were no toxicological concerns related to mycoprotein administration, and the NOAEL was 150,000 ppm, equivalent to 12,150.22 and 12,499.29 mg/kg/day for male and female rats, respectively. These findings support the safety of F. compactum MM-135 mycoprotein for 90-day extended toxicity studies.
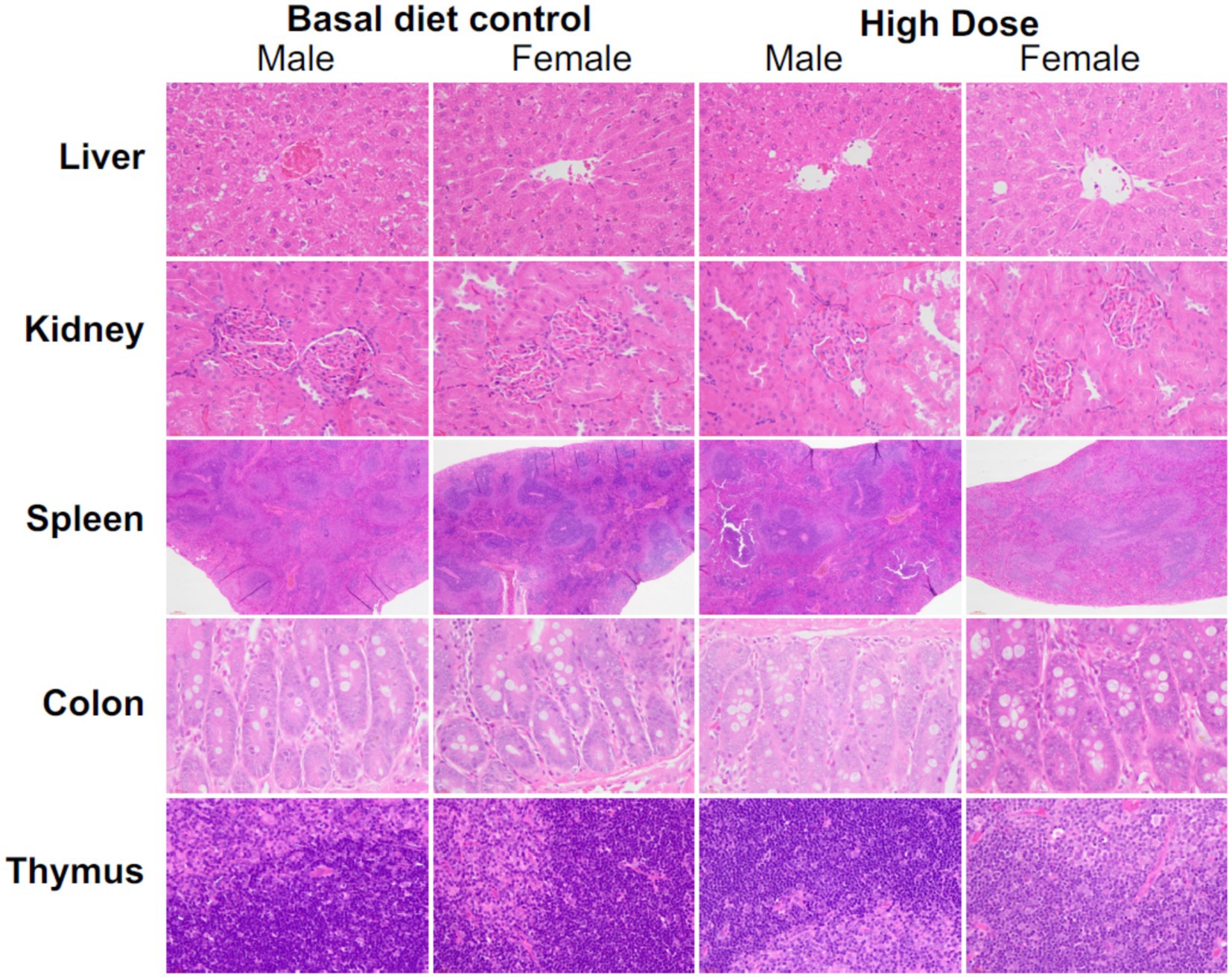
Figure 4. Histopathological analysis of the liver, kidney, spleen, colon, and thymus was conducted following a sub-acute toxicity test in rats treated with different doses of mycoprotein from Fusarium compactum MM-135. Representative H&E-stained photographs were shown. Magnification levels were as follows: 40x for the liver, kidney, colon, and thymus, and 4x for the spleen.
4 Discussion
To address the nutritional requirements of the expanding global population and meet global protein demands without causing undue environmental harm, there is an urgent need for sustainable and secure protein sources (Godfray, 2019). Mycoprotein, characterized by its high efficiency and low resource utilization, coupled with decreasing production costs due to technological advancements, emerges as a promising candidate (Finnigan et al., 2019; Souza Filho et al., 2019; Sillman et al., 2020; Muhammad Ijaz Ahmad et al., 2022; Derbyshire et al., 2023). The global fungal protein market size reached approximately $314 million in 2023 and is projected to reach $1,139 million by 2030, with a compound annual growth rate (CAGR) of 18.9% from 2024 to 2030 (Future Market Insights Inc, 2023; Reports and Insights Business Research Pvt Ltd, 2023). Despite the increasing research investment, the commercial availability of mycoprotein products remains limited. Currently, a few key suppliers, including Quorn Foods, 3F BIO, Meati Foods, MycoTechnology, and Mycorena, dominate the market with a combined market share exceeding 97%. Moreover, the published species utilized for mycoprotein production are restricted to F. venenatum in Quorn® and F. flavolapis in Nature’s Fynd’s product (Wiebe, 2002; Finnigan et al., 2019; Furey et al., 2022).
The identification of optimal fungal strains for mycoprotein production relies on extensive strain collections and advanced screening technologies. To meet the commercial criteria for strains—such as growth rate, protein yield, texture, and mycelial hue—we have developed an advanced screening technique that combines functional predictive analysis with high-throughput screening methods. Building on our previously published research on the bioinformatics-based screening and evaluation of protein biomass in the Fusarium genus (Pu et al., 2024), we have integrated key indicators, including protein content, biomass, growth rate, and well-developed mycelial morphology, to screen over 7,000 strains from our microbial strain library. More than 7,000 strains from 200 distinct Fusarium species in our microbial strain library have been subjected to multiple rounds of screening and evaluation in both laboratory and pilot facilities. We identified a novel high-performing mycoprotein-producing strain of Fusarium, designated as F. compactum MM-135. This strain demonstrates rapid growth and achieves a high mycelial protein content, exceeding 50% on a dry-weight basis, during liquid fermentation.
To comprehensively assess the nutrient composition of mycoprotein derived from F. compactum MM-135, three batches were analyzed for their nutritional profile, amino acid content, and PDCAAS. The results showed that this mycoprotein contains all nine essential amino acids, with total amino acids comprising 40–50% of the dry weight, essential amino acids representing 20–25% of the dry weight and 40–50% of the protein content and branched-chain amino acids (leucine, isoleucine, valine) accounting for 8–15% of the dry weight. Additionally, it is a complete protein source with high dietary fiber content and no trans-unsaturated fatty acids. Next, PDCAAS was used to evaluate product’s protein quality based on human amino acid requirements and digestibility, predicting dietary protein utilization. When evaluated based on the amino acid requirements of children aged 3–10 years and adults aged 18 years and above, mycoprotein derived from F. compactum MM-135 has a PDCAAS score of 1.00. This indicates that mycoprotein is a high-quality protein source, comparable to beef (PDCAAS 0.92) and superior to pea protein concentrates (PDCAAS 0.67) (van Vliet et al., 2015).
For basic quality control, we tested for common foodborne pathogens, aerobic microbes, mold and heavy metals in the samples. No common foodborne pathogens (e.g., Listeria monocytogenes, E. coli, Staphylococcus aureus, Salmonella) were detected. Levels of cadmium, lead, and arsenic were <20 μg/kg, and mercury was <10 μg/kg. Total aerobic microbes, yeast, and mold were <10 CFU/g, indicating high-quality production. To assess the toxicity, mutagenicity and genotoxicity of mycoprotein derived from F. compactum MM-135, a comprehensive series of studies have been conducted, including mycotoxins analysis, genotoxicity tests and a 14-day oral toxicity evaluation. Mycotoxin analysis revealed undetectable levels for most toxins, while certain mycotoxins, including enniatin and beauvericin (BEA), were found to be within acceptable regulatory limits. The EFSA Panel examined relevant information, including new data subsequent to the 2014 Scientific Opinion on the risks to human and animal health associated with the presence of BEA and enniatin in food and feed (EFSA Panel on Contaminants in the Food Chain (CONTAM), 2024). Recent in vitro studies conducted in mammalian cell lines did not provide substantial evidence for the induction of chromosomal damage or an increase in DNA strand breaks by BEA (Mallebrera et al., 2018). In vivo studies (Comet and Piga assays, micronucleus test) with BEA also yielded negative results (EFSA Panel on Contaminants in the Food Chain (CONTAM), 2024). The mycoprotein from F. compactum MM-135 demonstrated no mutagenicity in the Ames assay or genotoxicity in vitro and in vivo mammalian cell tests. A 14-day dietary exposure study in rats revealed no mortality and no test substance-related alterations in clinical observations, body weight, food consumption, food efficiency, clinical pathology, or necropsy observations. The safe dietary intake levels for rats are 12,150.22 mg/kg for males and 12,499.29 mg/kg for females, with no adverse effects observed at concentrations up to 150,000 ppm. These findings supported the initiation of a 90-day dietary exposure study in rats, which is currently underway, to further underscore the suitability of mycoprotein from F. compactum MM-135 for safe incorporation into food products. In summary, these findings support F. compactum MM-135 mycoprotein for potential food applications.
5 Conclusion
In this study, we identified a novel strain of Fusarium, F. compactum MM-135, which was recognized as a high-performing producer of mycoprotein through a rapid screening technique that assessed over 270,000 microbial samples. This comprehensive evaluation of mycoprotein sourced from F. compactum MM-135 revealed that it is a macronutrient and protein source providing all the essential amino acids and high amounts of dietary fiber, with no trans-unsaturated fatty acids. The true protein digestibility was 90.79%, and PDCAAS values was 1.00 for children aged 3–10 years and adults aged 18 and above. Batches of F. compactum MM-135 mycoprotein were devoid of microbial and heavy metal contamination, and detectable levels of most known mycotoxins. Furthermore, the absence of mutagenic potential was substantiated by negative results from the Ames test, and the mycoprotein did not exhibit genotoxicity in either the in vivo mammalian erythrocyte micronucleus assay or the in vitro mammalian chromosome aberration assay. Notably, even when administered at an elevated concentration of 150,000 ppm over a 14-day period in a dietary toxicity study with rats, the mycoprotein showed no adverse effects. Collectively, these results, along with the conclusion from a panel of experts that mycoprotein from F. compactum MM-135 is GRAS for its intended use as a food ingredient and macronutrient, support the safe incorporation of this mycoprotein into food products.
Data availability statement
The original contributions presented in the study are included in the article/Supplementary material, further inquiries can be directed to the corresponding authors.
Ethics statement
The animal study protocol of PDCAAS was approved by the Institutional Animal Care and Use Committee of Centre Testing International (Guangzhou) Co., Ltd., in compliance with the Guide for the Care and Use of Laboratory Animals (IACUC Number: CTI-IACUC-2024-12-003, approval date: 2024.12.26). The animal experiments involved in the study of mammalian erythrocyte micronucleus test were approved by the Institutional Animal Care and Use Committee (IACUC-SL-0010-2024, approval date: 2024.03.29) of Shanghai Municipal Center for Disease Control and Prevention. The study protocol of 14-Day Dietary Toxicity Study in Rats was approved by the Institutional Animal Care and Use Committee of Medicilon Preclinical Research (Shanghai) LLC (IACUC Number: 13035-24001-NG, effective date: 2023-05-15). The study was conducted in accordance with the local legislation and institutional requirements.
Author contributions
YX: Conceptualization, Data curation, Formal analysis, Methodology, Visualization, Writing – original draft, Writing – review & editing. LY: Data curation, Methodology, Project administration, Writing – review & editing. ZW: Investigation, Methodology, Writing – review & editing. DC: Investigation, Methodology, Writing – review & editing. ZS: Data curation, Investigation, Writing – review & editing. XJ: Conceptualization, Funding acquisition, Supervision, Writing – review & editing. LT: Conceptualization, Funding acquisition, Project administration, Supervision, Writing – review & editing.
Funding
The author(s) declare that financial support was received for the research and/or publication of this article. This work was supported by Moon Biotech, Inc. and MoreMeat Biotech, Inc. Moon Biotech, Inc. is an innovative biotechnology company focusing on the development and commercialization of microbial products and solutions. MoreMeat Biotech, Inc aim to produce fungi-based protein at large scale and at low cost sustainably to meet the growing human demand for alternative proteins and healthy foods.
Acknowledgments
The authors are thankful to the Moon Biotech Group (Moon Biotech, Inc.) and the MoreMeat Biotech Group (MoreMeat Biotech, Inc.) for supplying Fusarium strains for use in this study and financial resources. Especially, the authors are thankful to Yajun Liang of the Moon Biotech Group for identification and phylogenetic analysis of F. compactum MM-135 and Xingxiao Luo of the Moon Biotech Group for the establishment of detection methods.
Conflict of interest
YX and XJ were employed by the Moon (Guangzhou) Biotech Co., Ltd. LY, ZW, DC, ZS, and LT were employed by the MoreMeat (Guangzhou) Biotech Co., Ltd.
Generative AI statement
The authors declare that no Gen AI was used in the creation of this manuscript.
Publisher’s note
All claims expressed in this article are solely those of the authors and do not necessarily represent those of their affiliated organizations, or those of the publisher, the editors and the reviewers. Any product that may be evaluated in this article, or claim that may be made by its manufacturer, is not guaranteed or endorsed by the publisher.
Supplementary material
The Supplementary material for this article can be found online at: https://www.frontiersin.org/articles/10.3389/fsufs.2025.1583642/full#supplementary-material
Footnotes
References
AOAC Available online at: https://www.aoac.org/ (Accessed January 30, 2025).
Chi, M. D. (2024). Navigating the regulatory landscape for Fungi ingredients and Mycoprotein products: Insights from a food law expert. MycoStories.
Code of Federal Regulations. (2025). Available online at: https://www.ecfr.gov/current/title-21/chapter-I/subchapter-B/part-101/subpart-A/section-101.9 (Accessed April 22, 2025).
Coelho, M. O. C., Monteyne, A. J., Dirks, M. L., Finnigan, T. J. A., Stephens, F. B., and Wall, B. T. (2021). Daily mycoprotein consumption for 1 week does not affect insulin sensitivity or glycaemic control but modulates the plasma lipidome in healthy adults: a randomised controlled trial. Br. J. Nutr. 125, 147–160. doi: 10.1017/S0007114520002524
Derbyshire, E. A., and Keith-Thomas, A. (2019). Mycoprotein: nutritional and health properties. Nutr. Today 54, 7–15. doi: 10.1097/nt.0000000000000316
Derbyshire, E. J., Theobald, H., Wall, B. T., and Stephens, F. (2023). Food for our future: the nutritional science behind the sustainable fungal protein – mycoprotein. A symposium review. J. Nutr. Sci. 12:e44. doi: 10.1017/jns.2023.29
Dimina, L., Remond, D., Huneau, J. F., and Mariotti, F. (2021). Combining plant proteins to achieve amino acid profiles adapted to various nutritional objectives-An exploratory analysis using linear programming. Front. Nutr. 8:809685. doi: 10.3389/fnut.2021.809685
EFSA Panel on Contaminants in the Food Chain (CONTAM Panel) (2014). Scientific opinion on the risks to human and animal health related to the presence of beauvericin and enniatins in food and feed. EFSA J. 12:3802. doi: 10.2903/j.efsa.2014.3802
EFSA Panel on Contaminants in the Food Chain (CONTAM) (2024). Genotoxicity of beauvericin. EFSA J. 22:e9031. doi: 10.2903/j.efsa.2024.9031
FAO (2013). Dietary protein quality evaluation in human nutrition: Report of an FAO expert consultation. Auckland, New Zealand: FAO Food and Nutrition.
Finnigan, T. J. A., Wall, B. T., Wilde, P. J., Stephens, F. B., Taylor, S. L., and Freedman, M. R. (2019). Mycoprotein: the future of nutritious nonmeat protein. A symposium review. Curr. Dev. Nutr. 3:nzz021. doi: 10.1093/cdn/nzz021
Furey, B., Slingerland, K., Bauter, M. R., Dunn, C., Goodman, R. E., and Koo, S. (2022). Safety evaluation of Fy protein (nutritional Fungi protein), a macroingredient for human consumption. Food Chem. Toxicol. 166:113005. doi: 10.1016/j.fct.2022.113005
Future Market Insights Inc. (2023). Fungal Protein Market Size, Outlook and Forecast by 2033. London, United Kingdom.
Gatehouse, D. (2012). Bacterial mutagenicity assays: test methods. Methods Mol. Biol. 817, 21–34. doi: 10.1007/978-1-61779-421-6_2
Godfray, H. C. J. (2019). Meat: the future series-alternative proteins Report. Meat: The future series. Geneva, Switzerland: World Economic Forum.
Han, X., Xu, W., Zhao, X., Zhang, H., Zhagn, J., and Li, F. (2017). Development of high performance liquid chromatography tandem-mass spectrometry method for determination of beauvericin and enniatins in corn and wheat and their products. Chinese J. Food Hyg. 29, 633–640. doi: 10.13590/j.cjfh.2017.06.001
Ijaola, A. O., Akamo, D. O., George, T. T., Sengul, A., Adediji, M. Y., and Asmatulu, E. (2024). Algae as a potential source of protein: a review on cultivation, harvesting, extraction, and applications. Algal Res. 77:103329. doi: 10.1016/j.algal.2023.103329
Kjeldahl, J. (1883). Neue Methode zur Bestimmung des Stickstoffs in organischen Körpern. Z. Anal. Chem. 22, 366–382. doi: 10.1007/BF01338151
Krska, R., Schuhmacher, R., Grasserbauer, M., and Scott, P. M. (1996). Determination of the fusarium mycotoxin beauvericin at μg/kg levels in corn by high-performance liquid chromatography with diode-array detection. J. Chromatogr. A 746, 233–238. doi: 10.1016/0021-9673(96)00329-9
Lee, D. Y., Lee, S. Y., Yun, S. H., Lee, J., Mariano, E. Jr., Park, J., et al. (2024). Current technologies and future perspective in meat analogs made from plant, insect, and Mycoprotein materials: a review. Food Sci Anim Resour. 44, 1–18. doi: 10.5851/kosfa.2023.e51
Li, K., Qiao, K., Xiong, J., Guo, H., and Zhang, Y. (2023). Nutritional values and bio-functional properties of fungal proteins: applications in foods as a sustainable source. Food Secur. 12:4388. doi: 10.3390/foods12244388
Lin, J. W. X., Maran, N., Lim, A. J., Ng, S. B., and Teo, P. S. (2025). Current challenges, and potential solutions to increase acceptance and long-term consumption of cultured meat and edible insects – a review. Future Foods 11:100544. doi: 10.1016/j.fufo.2025.100544
Logrieco, A., Moretti, A., Ritieni, A., Chelkowski, J., Altomare, C., Bottalico, A., et al. (1993). Natural occurrence of beauvericin in preharvest fusarium subglutinans infected corn ears in Poland. J. Agric. Food Chem. 41, 2149–2152. doi: 10.1021/jf00035a061
Malek, L., and Umberger, W. J. (2023). Protein source matters: understanding consumer segments with distinct preferences for alternative proteins. Future Foods 7:100220. doi: 10.1016/j.fufo.2023.100220
Mallebrera, B., Prosperini, A., Font, G., and Ruiz, M. J. (2018). In vitro mechanisms of Beauvericin toxicity: a review. Food Chem. Toxicol. 111, 537–545. doi: 10.1016/j.fct.2017.11.019
Maranghi, F., Narciso, L., Tait, S., Rocca, C. L., Felice, G. D., Butteroni, C., et al. (2018). In vivo toxicity and genotoxicity of beauvericin and enniatins. Combined approach to study in vivo toxicity and genotoxicity of mycotoxins beauvericin (BEA) and enniatin B (ENNB). EFSA 15:1406. doi: 10.2903/sp.efsa.2018.EN-1406
Miller, S. A., and Dwyer, J. T. (2001). Evaluating the safety and nutritional value of Mycoprotein. Food Technol. 55, 42–47.
Mishyna, M., Keppler, J. K., and Chen, J. (2021). Techno-functional properties of edible insect proteins and effects of processing. Curr. Opin. Colloid Interface Sci. 56:101508. doi: 10.1016/j.cocis.2021.101508
Mortelmans, K., and Zeiger, E. (2000). The Ames Salmonella/microsome mutagenicity assay. Mutat. Res. 455, 29–60. doi: 10.1016/s0027-5107(00)00064-6
Muhammad Ijaz Ahmad, S. F., Alhamoud, Y., Li, C., and Zhang, H. (2022). A review on mycoprotein: history, nutritional composition, production methods, and health benefits. Trends Food Sci. Technol. 121, 14–29. doi: 10.1016/j.tifs.2022.01.027
Onwezen, M. C., Bouwman, E. P., Reinders, M. J., and Dagevos, H. (2021). A systematic review on consumer acceptance of alternative proteins: pulses, algae, insects, plant-based meat alternatives, and cultured meat. Appetite 159:105058. doi: 10.1016/j.appet.2020.105058
Pavis, G. F., Iniesta, R. R., Roper, H., Theobald, H. E., Derbyshire, E. J., Finnigan, T. J. A., et al. (2024). A four-week dietary intervention with mycoprotein-containing food products reduces serum cholesterol concentrations in community-dwelling, overweight adults: a randomised controlled trial. Clin. Nutr. 43, 649–659. doi: 10.1016/j.clnu.2024.01.023
Plattner, R. D., and Nelson, P. E. (1994). Production of beauvericin by a strain of fusarium proliferatum isolated from corn fodder for swine. Appl. Environ. Microbiol. 60, 3894–3896. doi: 10.1128/aem.60.10.3894-3896.1994
Pu, Z., Lan, Z., Zhang, Z., and Tian, L. (2024). Method for Screening Marker Proteins or Marker Genes Related to Protein Yield of Strains, and Method and Related Equipment for Evaluating Protein Yield of Strains. China Patent No CN 118553304. Beijing, China: A China National Intellectual Property Administration
Reports and Insights Business Research Pvt Ltd. (2023). Mycoprotein products market report 2024–2032: Industry analysis, Growth, Share, Trends, Size and Forecast. Kota, Rajasthan, India.
Sillman, J., Uusitalo, V., Ruuskanen, V., Ojala, L., Kahiluoto, H., Soukka, R., et al. (2020). A life cycle environmental sustainability analysis of microbial protein production via power-to-food approaches. Int. J. Life Cycle Assess. 25, 2190–2203. doi: 10.1007/s11367-020-01771-3
Souza Filho, P. F., Andersson, D., Ferreira, J. A., and Taherzadeh, M. J. (2019). Mycoprotein: environmental impact and health aspects. World J. Microbiol. Biotechnol. 35:147. doi: 10.1007/s11274-019-2723-9
Standard. Available online at: http://down.foodmate.net/standard/index.html (Accessed January 30, 2025).
Standards, E. (2017). Available online at: https://www.en-standard.eu/search/?q=BS+EN+16943%3A2017 (Accessed January 30, 2025).
Tang, J., Yao, D., Xia, S., Cheong, L., and Tu, M. (2024). Recent progress in plant-based proteins: from extraction and modification methods to applications in the food industry. Food Chem X 23:101540. doi: 10.1016/j.fochx.2024.101540
Taylor, J. W., Jacobson, D. J., Kroken, S., Kasuga, T., Geiser, D. M., Hibbett, D. S., et al. (2000). Phylogenetic species recognition and species concepts in fungi. Fungal Genet. Biol. 31, 21–32. doi: 10.1006/fgbi.2000.1228
Timothy, T. J., and Knob, J.. (2020). Equipment Considerations for Plant-Based Food Processing Available online at: https://www.leeind.com/blog/mixing-challenges/equipment-considerations-for-plant-based-food-processing (Accessed November 24, 2020).
Tong, S., Chen, W., Hong, R., Chai, M., Sun, Y., Wang, Q., et al. (2024). Efficient Mycoprotein production with low CO2 emissions through metabolic engineering and fermentation optimization of fusarium venenatum. J. Agric. Food Chem. 72, 604–612. doi: 10.1021/acs.jafc.3c08509
Traynor, A., Burns, D. T., Wu, D., Karoonuthaisiri, N., Petchkongkaew, A., and Elliott, C. T. (2024). An analysis of emerging food safety and fraud risks of novel insect proteins within complex supply chains. NPJ Sci. Food 8:7. doi: 10.1038/s41538-023-00241-y
van Vliet, S., Burd, N. A., and van Loon, L. J. (2015). The skeletal muscle anabolic response to plant-versus animal-based protein consumption. J. Nutr. 145, 1981–1991. doi: 10.3945/jn.114.204305
Wang, B., Shi, Y., Lu, H., and Chen, Q. (2023). A critical review of fungal proteins: emerging preparation technology, active efficacy and food application. Trends Food Sci. Technol. 141:104178. doi: 10.1016/j.tifs.2023.104178
Wiebe, M. G. (2002). Myco-protein from fusarium venenatum: a well-established product for human consumption. Appl. Microbiol. Biotechnol. 58, 421–427. doi: 10.1007/s00253-002-0931-x
Keywords: mycoprotein, Fusarium compactum MM-135, PDCAAS, safety, mutagenicity, genotoxicity
Citation: Xian Y, Yin L, Wu Z, Chen D, Sun Z, Jiang X and Tian L (2025) Characterization and preliminary safety evaluation of mycoprotein from Fusarium compactum MM-135. Front. Sustain. Food Syst. 9:1583642. doi: 10.3389/fsufs.2025.1583642
Edited by:
Reza Rastmanesh, American Physical Society, United StatesReviewed by:
Qisen Xiang, Zhengzhou University of Light Industry, ChinaTünde Pusztahelyi, University of Debrecen, Hungary
Copyright © 2025 Xian, Yin, Wu, Chen, Sun, Jiang and Tian. This is an open-access article distributed under the terms of the Creative Commons Attribution License (CC BY). The use, distribution or reproduction in other forums is permitted, provided the original author(s) and the copyright owner(s) are credited and that the original publication in this journal is cited, in accordance with accepted academic practice. No use, distribution or reproduction is permitted which does not comply with these terms.
*Correspondence: Lingzhi Tian, dGlhbmx6QG1vb25iaW8uY29t; Xianzhi Jiang, anh6QG1vb25iaW8uY29t