Cover Cropping May Alter Legacy Phosphorus Dynamics Under Long-Term Fertilizer Addition
- 1Departamento de Ecologia Isotópica, Centro de Energia Nuclear na Agricultura (CENA), Universidade de São Paulo, Piracicaba, Brazil
- 2Lancaster Environment Centre, Lancaster University, Lancaster, United Kingdom
- 3Departamento de Ciência do Solo, Escola Superior de Agricultura Luiz de Queiroz (ESALQ), Universidade de São Paulo, Piracicaba, Brazil
- 4Departamento de Ciência do Solo, Universidade Tecnológica Federal do Paraná (UTFPR), Dois Vizinhos, Brazil
Use of cover crops in an integrated agricultural system can reduce demand of inorganic phosphorus (P) fertilizers, where the subsequent crops can take up P accumulated in cover crops biomass after the decomposition. In this research we hypothesized that some cover crops can take up P from less labile fractions and recycle it back to the soil through plant residues resulting in better P use efficiency of the system; cover crops are capable of P uptake from subsurface layers which leads to the accumulation of this P on the surface after the decomposition of their residues; and cover crop species respond differently to distinct inorganic P sources. To examine these hypotheses, a field experiment was conducted over nine successive years in South Brazil. The experimental treatments were established as a split-plot randomized block design, in a 3 × 6 factorial scheme, considering three P treatments [no-P, single superphosphate (SSP), and rock phosphate (RP)] as main plot and six cover crop treatments (common vetch, white lupin, fodder radish, ryegrass, black oat, and a fallow) as subplots. Soil samples were collected from the depths of 0–5, 5–10, and 10–15 cm and analyzed by Hedley P fractionation. In P-unfertilized cropping system, the amounts of labile and mod-labile P fractions were not modified by cover crops related to fallow. When inorganic P fertilizers were applied, the amount of labile and mod-labile P pools under fallow were higher than under cover crops in 5–10 cm depth. Black oat and common vetch cycled more labile P under SSP and RP, respectively. Common vetch and ryegrass resulted in the highest accumulation of organic P on the surface under SSP and RP, respectively. Black oat was capable to change P extracted by 1.0 M HCl to more labile forms. Fodder radish showed the highest P uptake in comparison with other cover crops. The higher P balance efficiency of the system was achieved under SSP in comparison with RP application but it seems that cover crops are more effective at improving the efficiency under RP compared to SSP.
Introduction
Phosphorus is the least mobile plant nutrient and a key limiting factor for crop development in agricultural systems (Hinsinger, 2001) and terrestrial ecosystems (Frossard et al., 1995). World P resources are finite and non-renewable, mostly concentrated in regions with territorial conflicts (Cordell and White, 2014). A sudden 800% rise in P fertilizer prices in 2008 gave rise to concern about the depletion of P resources (Cordell and White, 2011).
Phosphorus is being applied in high quantities to agricultural fields while the major part of it is being fixed by soil mineral particles and converted to soil pools with low plant availability over time (Takeda et al., 2009; Richardson et al., 2011), resulting in the high accumulation of legacy P in agricultural soils (Holford, 1997; Pearse et al., 2007). Withers et al. (2018) estimated that 106 Tg of legacy P will be accumulated in Brazilian agricultural lands by 2050 if current rate of inorganic P fertilizer use continues. Some plants like white lupin (Lupinus albus), pigeon pea (Cajanus cajan), rye (Secale cereale), and wheat (Triticum aestivum) are capable of using these less labile P fractions through different acquisition strategies and accumulate it in their aboveground plant parts (Braum and Helmke, 1995; Kamh et al., 1999; Raghothama, 1999; Wasaki et al., 2008, 2009). These crops can be used as cover crops in an integrated agricultural system (Vanlauwe et al., 2000; Horst et al., 2001), where the subsequent crops can take up P accumulated in their biomass after the decomposition, resulting in reduced demand of inorganic P fertilizers. Phosphorus released from cover crops after decomposition can be: (1) plant available [resin P and P extracted by 0.5 M NaHCO3 in Hedley P fractionation (Hedley et al., 1982)]; (2) immobilized in microbial biomass (moderately labile for plant uptake); (3) transformed into soil organic P (moderately to poorly available for plant uptake) (Daroub et al., 2000; Sugito et al., 2001); and (4) lost from soils via erosion, runoff or leaching (Maltais-Landry and Frossard, 2015). Iyamuremye et al. (1996) observed that most of P released to the soil after mineralization of crop residues was accumulated in NaOH extractable P derived from Hedley P fractionation. Cover crops which are capable of acquiring higher amounts of P and subsequently release it from their residues in plant-available forms are more efficient in stimulating soil P cycling (Damon et al., 2014). The contribution of cover crop residues to P uptake by the main crop depends on residue type and management, and soil conditions (Thibaud et al., 1988; Nachimuthu et al., 2009). Revealed by isotope labeling, this contribution can be less than 25% when the residues are not incorporated to the soil (Noack et al., 2014). Residues with high C:N ratio, high lignin and low cellulose contents like the species of the Poaceae family including black oat (Avena strigosa) and rye decompose slower and release smaller amounts of P (Ferreira et al., 2014), while residues with low C:N ratio, low lignin and high cellulose contents like the species of Brassicaceae family, including oilseed radish (Raphanus sativus) decompose faster and release higher amounts of P to the soil (Doneda et al., 2012).
We hypothesized that (1) some cover crops can take up P from less labile fractions and recycle it back to the soil through plant residues, resulting in better P use efficiency of the system; (2) cover crops are capable of P uptake from subsurface layers which leads to the accumulation of this P on the surface after the decomposition of their residues; and (3) cover crop species respond differently to distinct inorganic P sources, altering the dynamics of P in soil profile.
Materials and Methods
Experimental Design and Management Practices
A field experiment was conducted over nine successive years from 2009 to 2017 at the Federal Technological University of Paraná (UTFPR), Dois Vizinhos, located in Paraná State, South Brazil, in 25°44′05″S, 53°03′31″W and 509 m above the sea level. The soil is a clayey Rhodic Hapludox (Soil Survey Staff, 1999) with low pH (5.2–5.4) and high organic matter content (∼40 g kg–1) in the 0–10 cm depth layer (Pavinato et al., 2017). This soil is representative of large areas of soybean [Glycine max (L.) Merr.] and maize (Zea mays) cultivation in Brazil. The area had been cropped with summer grain crops (soybean/maize) and winter wheat or cover crops (oat) since 2001 under a no-tillage cultivation system. The available P level (anion exchange resin) in the soil at experiment establishment was considered low in the 0–5 (8.1 mg kg–1) and 5–10 (9.7 mg kg–1) cm layers, and very low in the 10–20 (4.8 mg kg–1) cm layer according to Raij et al. (1997). Iron oxide content, mineral assemblage by X-ray diffraction and P maximum adsorption capacity (2201 mg kg–1) were also determined in a soil sample collected from the 0–20 cm layer before trial establishment and the results are accessible at Teles et al. (2017).
The experimental treatments were established as a split-plot randomized block design, in a 3 × 6 factorial scheme (considering three P treatments as main plot and six cover crop treatments as subplots) with three replicates. Plots with the dimension of 5 × 5 m were established in January 2009. Phosphate fertilizer sources were always applied at sowing of summer crops (maize or beans), as rock phosphate (RP from Algeria: 9% soluble P2O5 and 29% total P2O5) and soluble phosphate (SSP: 18% soluble/total P2O5). Both phosphate fertilizers were broadcasted over the whole area without incorporation before establishment of every summer crop. A treatment without P addition was also included (Nil-P). Species of winter cover crops were: common vetch (Vicia sativa), white lupin (Lupinus albus), fodder radish (Raphanus sativus), ryegrass (Lolium multiflorum), black oat (Avena strigosa), and a fallow (occasional weeds, not identified).
In January 2009, the phosphate fertilizers were first applied to a common bean (Phaseolus vulgaris L.) crop at a rate of 135 kg ha–1 of soluble P2O5 either as SSP or RP according to the respective treatments. This rate included an allowance to build up the background level of plant-available P in the soil (<10 mg kg–1). Each subsequent summer, from 2010 to 2015, maize was cultivated with P fertilizer application at a rate of 105 kg ha–1 yr–1 of soluble P2O5 to meet the grain yield of 8.0 Mg ha–1, except in 2012/13 when soybean was established with the same fertilizer rate, although it was not harvested because of excessive rain in that season. In this way, the total amounts of P added were 335 and 1078 kg ha–1 by SSP and RP, respectively (Table 1). Winter cover crops did not receive P fertilizer, to enable assessment of the residual P uptake left by the preceding summer crop. Phosphorus fertilization was halted after 2015 as anion exchange resin P reached high levels (>50 mg kg–1). Potassium fertilizer was applied to summer crops as potassium chloride at sowing, according to standard recommendations (40 kg ha–1 of K2O annually), but was not applied to the winter cover crops since the soil K levels were considered high (Sociedade Brasileira de Ciência do Solo [SBCS], 2016). Winter cover crop species received N topdressing (40 kg ha–1 of N as urea), except vetch and lupin which are leguminous species and can fix atmospheric N2. Each maize crop received 120 kg ha–1 of N as urea, applied in three dressings of 40 kg ha–1 of N, at sowing, at V4–V5 stage and at V7–V8 stage.
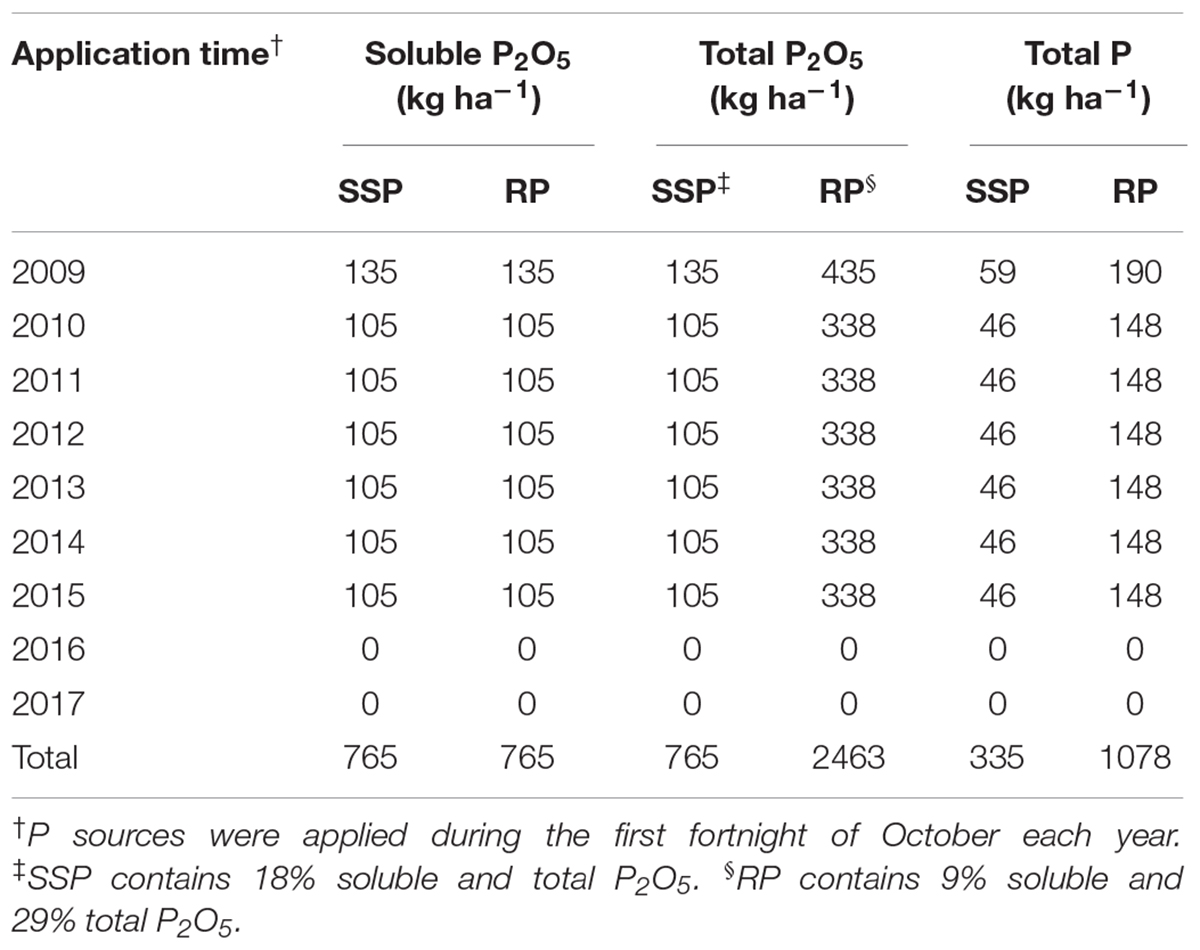
Table 1. Amounts of soluble and total P2O5 and total P applied with single superphosphate (SSP) and rock phosphate (RP) from 2009 to 2017.
Both winter and summer crops were established under a no-till cultivation system. Cover crops were established in the first half of May in all 9 years. Pure live seed rates were 70 kg ha–1 of common vetch, 100 kg ha–1 of white lupin, 20 kg ha–1 of fodder radish, 20 kg ha–1 of ryegrass, and 60 kg ha–1 of black oat. Row spacing was 20 cm between lines, except white lupin which was sown in 40-cm rows. Sowing lines were opened with a hand chisel and the seeds were distributed one by one along each row by hand. The cover crops biomass was desiccated with glyphosate [N-(phosphonomethyl) glycine] (2 L a.i. ha–1) in the first half of September and left on the soil surface. Maize in the summer was established in the first fortnight of October in each year, with a final density of about 60,000 plants per hectare. The hybrid CD308 (Coodetec, Dow Agrosciences, Cascavel, PR, Brazil) was cultivated in the first two years, and the hybrid AGN30A30 (Agromen, Agromen Sementes Ltda, Orlândia, SP, Brazil) in the other years. Control of weeds, pests, and diseases were performed according to the recommended protocols.
Soil and Plant Sampling and Analyses
Cover crop and maize tissue sampling was performed at flowering stage (for maize at VT stage), when the concentration of nutrients is normally at a high level, to determine dry matter (DM) accumulation and nutrient contents. For cover crops, aboveground biomass was collected from an area covering two row lines by 0.5 m long (0.40 m2 for white lupin and 0.20 m2 for other crops). Five subsamples were collected from each plot and thoroughly mixed to provide a composite plot sample. Maize was sampled by taking 10 representative plants from each plot. The crop samples were oven dried at 65°C for 72 h (or until constant weight), weighed (for DM determination), ground through a 2-mm sieve in a Wiley mill, and stored for later laboratory analysis. Concentration of P in plant tissues was determined according to the methodology described by Tedesco et al. (1995), and nutrient uptake (kg ha–1) was calculated based on the total DM. The maize harvest was performed manually in early April of each year, by taking an area of four rows by 4 m in each plot. Grain yields were obtained after threshing the ears in a mechanical machine and reported at 13% grain moisture, a standard procedure for Brazilian crops (Elmore and Roeth, 1999).
Changes in soil P pools were evaluated in the 0–5, 5–10, and 10–15 cm soil layers, collected in September 2017, at management of the ninth cover crop cultivation, right before glyphosate application. Four soil subsamples were collected from each plot in the depths of 0–5, 5–10, and 10–15 cm and thoroughly mixed to provide a composite plot sample from each depth (0.5 kg). The soil samples were air-dried for 72 h (or until constant weight), ground through a 2-mm sieve and stored for later laboratory analysis. Chemical P fractionation was performed according to the procedure described by Hedley et al. (1982), with modifications by Condron et al. (1985) changing the original sonication step by 0.5 M NaOH extraction. This technique uses chemical extractants sequentially in the same sample to remove progressively from the most available to the most stable fractions of inorganic (Pi) and organic P (Po). Thus, the pools determined sequentially were as follows (Cross and Schlesinger, 1995): (i) labile pool, corresponding to the inorganic P extracted by anion exchange resin (PAER) and the inorganic and organic P extracted by 0.5 M NaHCO3 at pH 8.5 (PiBIC and PoBIC); (ii) moderately labile (mod-labile) pool, corresponding to the inorganic and organic P extracted by 0.1 M NaOH (PiHID–0.1 and PoHID–0.1), and inorganic P extracted by 1.0 M HCl (PHCl); and (iii) non-labile pool, corresponding to the inorganic and organic P extracted by 0.5 M NaOH (PiHID–0.5 and PoHID–0.5), besides residual digestion with H2SO4 + H2O2 in the presence of saturated MgCl2 (Presidual). The P concentration in each extractant was measured according to Murphy and Riley (1962) procedure for acid extracts, and Dick and Tabatabai (1977) procedure for alkaline extracts. To measure organic P in alkali extracts, each fraction was digested with H2SO4 1:1 (acid:water) and ammonium persulfate 7.5%, and organic P was determined by the difference of concentration in the undigested (Pi) from the digested extract (total P).
Soil P pools to calculate P balance efficiency of the system were presented as kg ha–1 of P considering the soil bulk density of 1.2 g cm–3 to the depth of 15 cm. The amount of P exported from the field through the grains were calculated as a function of grain P concentration (%) and grain yield (kg ha–1). Phosphorus balance efficiency was calculated by the following equation (Simpson et al., 2011; Boitt et al., 2018):
To investigate the changes in the proportions of total P in different P fractions with time (Figure 1), data of 2009 and 2011 were obtained from Teles et al. (2017), data of 2014 were retrieved from Soltangheisi et al. (2018), and data of 2017 are the results of the current study. Each small square represents 1% of total P. Labile, mod-labile, and non-labile P fractions were shown with the shades of green, blue, and brown colors, respectively.
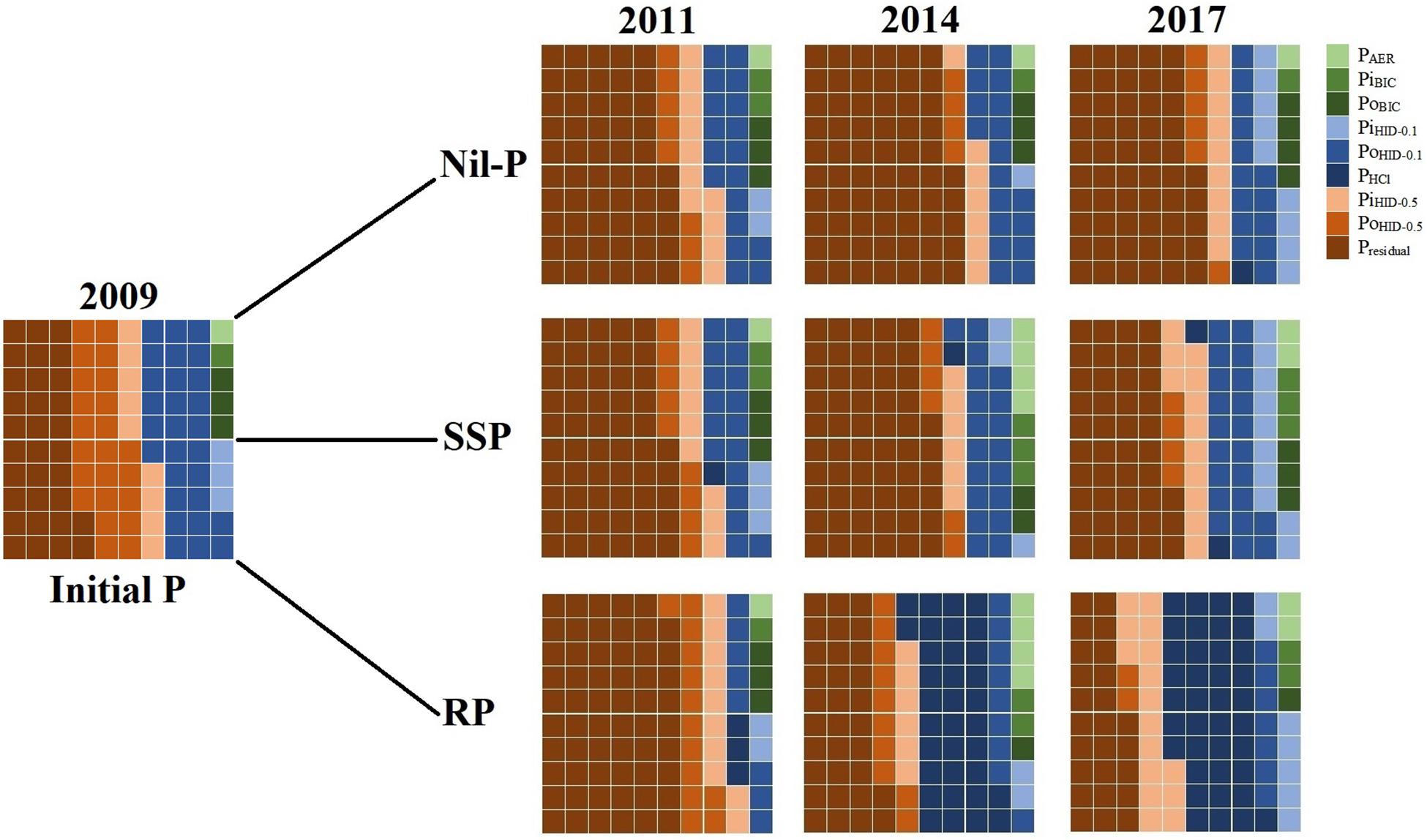
Figure 1. Proportions of each P fraction under phosphate sources in 0–5 cm soil layer from 2009 to 2017. Each small square represents 1% of total P. Labile, mod-labile, and non-labile P fractions were shown with the shades of green, blue, and brown colors, respectively. 2009 and 2011 data were obtained from Teles et al. (2017). 2014 data were obtained from Soltangheisi et al. (2018). Data of 2017 are the results of the current study.
Data Analysis
Variance homogeneity and normality of data distribution were tested for each parameter before carrying out an analysis of variance (ANOVA). Data were transformed using Box-Cox techniques (Box and Cox, 1964) and outliers were removed when needed. Next, the data were submitted to ANOVA using PROC GLM to test the effect of phosphate fertilizer sources and cover crops on soil P fractions. When significant, means were compared using the t-test (LSD) (p < 0.05). When the interaction phosphate fertilizer source × cover crop was significant, means were tested using LSD (p < 0.05). All the statistical analyses were carried out using the SAS 9.3 program (Sas Institute, 2008).
Results
Soil P Lability
Labile, mod-labile and non-labile P pools were influenced by the interaction of cover crops × P sources in 0–5 and 5–10 cm depth layers (Table 2). In 10–15 cm soil layer, cover crops did not affect P lability, while mod-labile and non-labile P were increased by P application (Table 2).
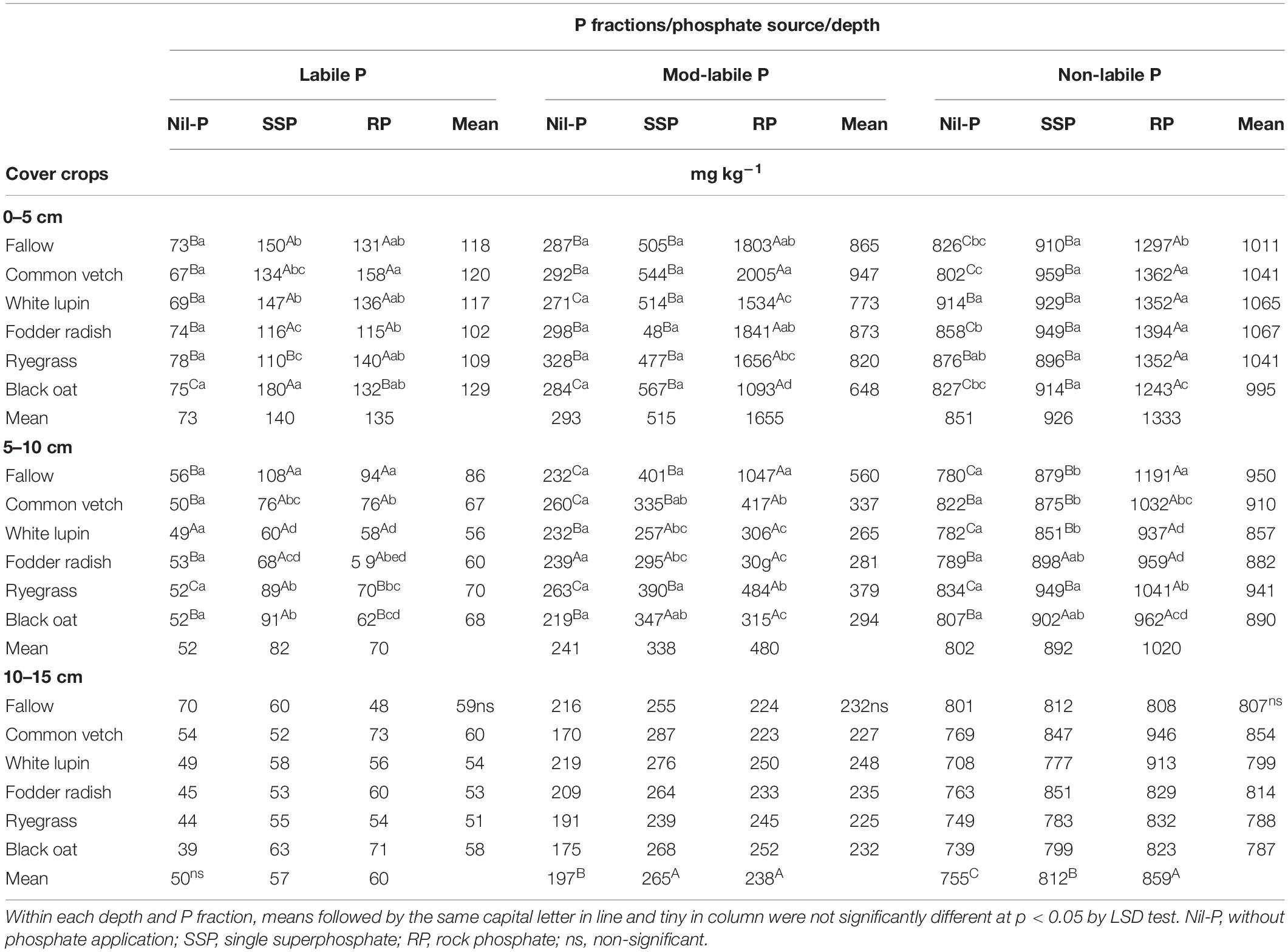
Table 2. Labile, mod-labile and non-labile P in three soil layers under phosphate sources and cover crops after the 9th cultivation cycle of successive cover crops and cash crops.
Labile P pool content increased with P application under all cover crops including fallow in both 0–5 and 5–10 cm layers, regardless of P sources (SSP and RP), except under white lupin in 5–10 cm which was similar to nil-P (Table 2). Under ryegrass cultivation, the highest value of labile P was recorded when RP applied, followed by SSP and nil-P in 0–5 cm depth, while in 5–10 cm the highest was observed under SSP, followed by RP and nil-P. Otherwise, for black oat it was the opposite, with higher values under SSP compared to RP in both layers. Cover crops did not modify the amount of labile P under nil-P in all depths evaluated. Under SSP, the highest labile P concentration was recorded under black oat cultivation in soil surface layer and under fallow in subsurface layer of 5–10 cm. Under RP application the highest labile P was observed under common vetch in 0–5 cm soil layer, and under fallow in 5–10 cm depth (Table 2).
Mod-labile P pool was the highest under RP application for all cover crop treatments in the top surface layer (Table 2). This P pool was changed by phosphate sources, with substantial increase under RP, followed by SSP application. Under nil-P, cover crops did not modify mod-labile P in all soil layers. The same trend was observed under SSP application in 0–5 and 10–15 cm depths, while in 5–10 cm layer the highest and the lowest mod-labile P pool was detected under fallow and white lupin, respectively. Under RP application, mod-labile P reached a maximum under common vetch cultivation in 0–5 cm, being 1.8 times higher than under black oat which was the lowest in this layer. In 5–10 cm depth, the highest mod-labile P was recorded under fallow, 2.6 times higher than the average of the cover crops. In 10–15 cm the mod-labile P was affected only by P sources being higher under P application compared to nil-P, irrespective of the source (Table 2).
In general, non-labile P pool was the highest under RP application for all soil depths investigated, except for fodder radish and black oat in 5–10 cm layer in which SSP and RP were similar, but both higher than nil-P (Table 2). Under nil-P, the highest amount of non-labile P pool in 0–5 cm was recorded for white lupin and the lowest for common vetch, while in 5–10 cm it was not affected by cover crops. Under SSP application non-labile P pool was not affected by cover crops in 0–5 and 10–15 cm layers, while it reached a maximum under ryegrass in 5–10 cm depth. Under RP application the lowest non-labile P was observed under black oat in 0–5 cm, while the highest was measured under fallow in 5–10 cm. Although cover crops did not influence non-labile P in 10–15 cm depth, it was affected by P sources, being the highest under RP followed by SSP and nil-P (Table 2).
Organic, Inorganic, and Total P
Inorganic P was affected by the interaction between P sources and cover crops in all depths (Table 3). The highest amount of inorganic P was measured under RP application for all cover crops and in all soil depths investigated, while in general significant differences were not observed between SSP and nil-P (Table 3). Cover crops did not influence inorganic P under nil-P in 0–5 and 5–10 cm soil layers, while in the deepest soil layer the highest amount was recorded under fallow (Table 3). While cover crops did not modify inorganic P under SSP application in soil surface layer, some small changes were observed in 5–10 and 10–15 cm depths. When RP was applied, the highest amount of inorganic P was detected under common vetch, 54% higher than under black oat which was the lowest in 0–5 cm soil layer. In 5–10 cm the amount of inorganic P under fallow was 1.8 times higher than the average of the cover crops (Table 3).
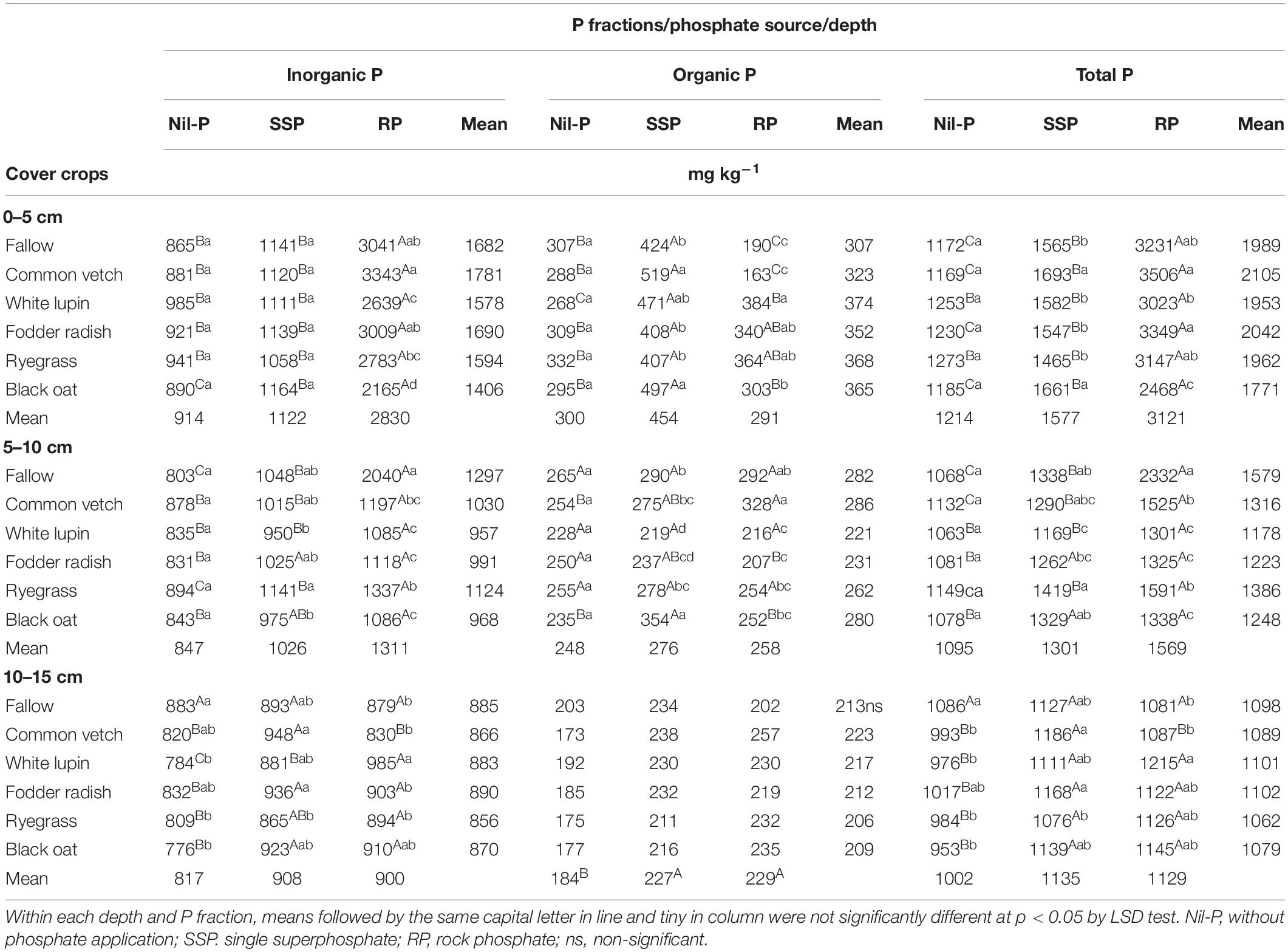
Table 3. Inorganic, organic and total P in three soil layers under phosphate sources and cover crops after the 9th cultivation cycle of successive cover crops and cash crops.
Organic P was influenced by P sources × cover crops interactions in 0–5 and 5–10 cm soil layers, while it was only affected by P sources in 10–15 cm (Table 3). In the 0–5 cm layer organic P was the highest under SSP for all the cover crop treatments in comparison with nil-P and RP. Under nil-P cover crops did not affect the amount of organic P in all depths. Under SSP the highest amount of organic P was recorded under common vetch and black oat in 0–5 cm, and under black oat in 5–10 cm, being 62% higher than under white lupin which showed the lowest content of organic P in this depth. Under RP organic P reached a maximum when ryegrass was cultivated, while the lowest was observed for common vetch in 0–5 cm. In 5–10 cm the highest and the lowest organic P were measured under common vetch and fodder radish, respectively (Table 3). While the content of organic P increased by phosphate application compared to nil-P in 10–15 cm soil layer, it was not different among P sources (SSP and RP) (Table 3).
Total P in soil surface layer followed the trend: RP > SSP > nil-P except under white lupin and ryegrass in which there were no differences between SSP and nil-P (Table 3). The same trend was observed in 5–10 cm depth except under white lupin in which there was not significant difference between SSP and nil-P, and under fodder radish and black oat in which significant differences were observed between SSP and RP (Table 3). In 10–15 cm the total P was similar among SSP and RP, while both P sources were higher than nil-P except for fallow in which total P was the same under P sources and nil-P. Under nil-P, cover crops did not influence total P in 0–5 and 5–10 cm depths, while in 10–15 cm the highest amount was under fallow compared to cover crops (Table 3). When SSP was applied, the highest total P content in soil surface layer was recorded under common vetch and black oat as cover crops. In 5–10 cm, the highest total P was measured under ryegrass, being 21% higher than white lupin which was the lowest one (Table 3). Under RP application, the highest and the lowest total P contents were observed for common vetch and black oat, respectively, with a difference of 1038 mg kg–1 in 0–5 cm. In 5–0 cm, total P under fallow was 1.6 times higher than the average of all cover crops. Among cover crops in this depth, total P under common vetch and ryegrass was higher than the others (Table 3).
Proportions of P Fractions
The proportion of total P in PAER was not changed under nil-P and constituted 1% of total P in all years averaged among cover crops (Figure 1). When phosphates were applied (SSP and RP), PAER proportion increased to 4% in 2014 and then decreased to 2% in 2017 for both P sources as a consequence of no P fertilization in the last 2 years. Proportion of PiBIC followed the same trend but it did not exceed 3% of total P (Figure 1). When RP was applied, PoBIC was reduced from 3% in 2009 to 1% of total P in 2014 and 2017. Labile P pool (sum of green colors) constituted 6, 8, and 5% of total P under nil-P, SSP, and RP in 2017, after seven consecutive years of P application and 2 years under the use of legacy P (Figure 1).
The proportion of PiHID–0.1 was enhanced from 3% in 2009 to 9, 10, and 7% of total P under nil-P, SSP, and RP, respectively, in 2017 (Figure 1). PoHID–0.1 proportion decreased from 2009 to 2017 in all years and under all P sources (nil-P, SSP, and RP) (Figure 1). PHCl was negligible in 2009 and its proportion was not affected by SSP application over the time, while RP increased its proportion to 3, 34, and 38% in 2011, 2014, and 2017, respectively (Figure 1). Proportion of mod-labile P pool (sum of the shades of blue colors) was reduced with time under nil-P, from 31% in 2009 to 24% in 2017 (Figure 1). When P sources were applied, this proportion was lessened in the first 2 years of P application (in 2011), and then enhanced reaching 33 and 52% of total P under SSP and RP, respectively, in 2017 (Figure 1).
The proportion of PiHID–0.5 was not changed from 2009 to 2017 under nil-P (9% of total P), while it was enhanced with P application (Figure 1).%PoHID–0.5 reduced with time from 23% in 2009 to 6, 4, and 2% under nil-P, SSP, and RP, respectively, in 2017 (Figure 1). Presidual constituted 32% of total P in 2009 (Figure 1). Under nil-P, its proportion increased to 55% after 9 years. When SSP was applied, its proportion increased to 57% after 2 years (in 2011), and then reduced with time reaching 42% of total P in 2017. Under RP application, %Presidual enhanced to 59% in 2011, and then reduced with time, reaching the same levels of the initial amount in 2014 (32%) and reducing to 25% in 2017, being 7% lower than the initial condition. Proportion of non-labile P pool (sum of the shades of brown colors) increased with time under nil-P from 64% of total P in 2009 to 70% in 2017 (Figure 1). When P sources were applied, it was increased in the first 2 years (2011), and then gradually reduced reaching 59 and 43% of total P under SSP and RP application, respectively, in 2017 (Figure 1). The latter was dropped more due to the proportional increase in mod-labile P pool under RP.
P Balance Efficiency and P Uptake by Cover Crops
In general, P balance efficiency was 2.2 times higher under SSP application (48% averaged among cover crops) compared to RP application (22% averaged among cover crops) (Table 4). Under SSP application, white lupin (55%) and ryegrass (54%) showed the best P balance efficiency, higher than other species and fallow, while under RP application all cover crops (>19%) had P balance efficiency higher than fallow (15%). Among cover crops, black oat resulted in the highest P balance efficiency (27%) under RP application compared to other species (Table 4).
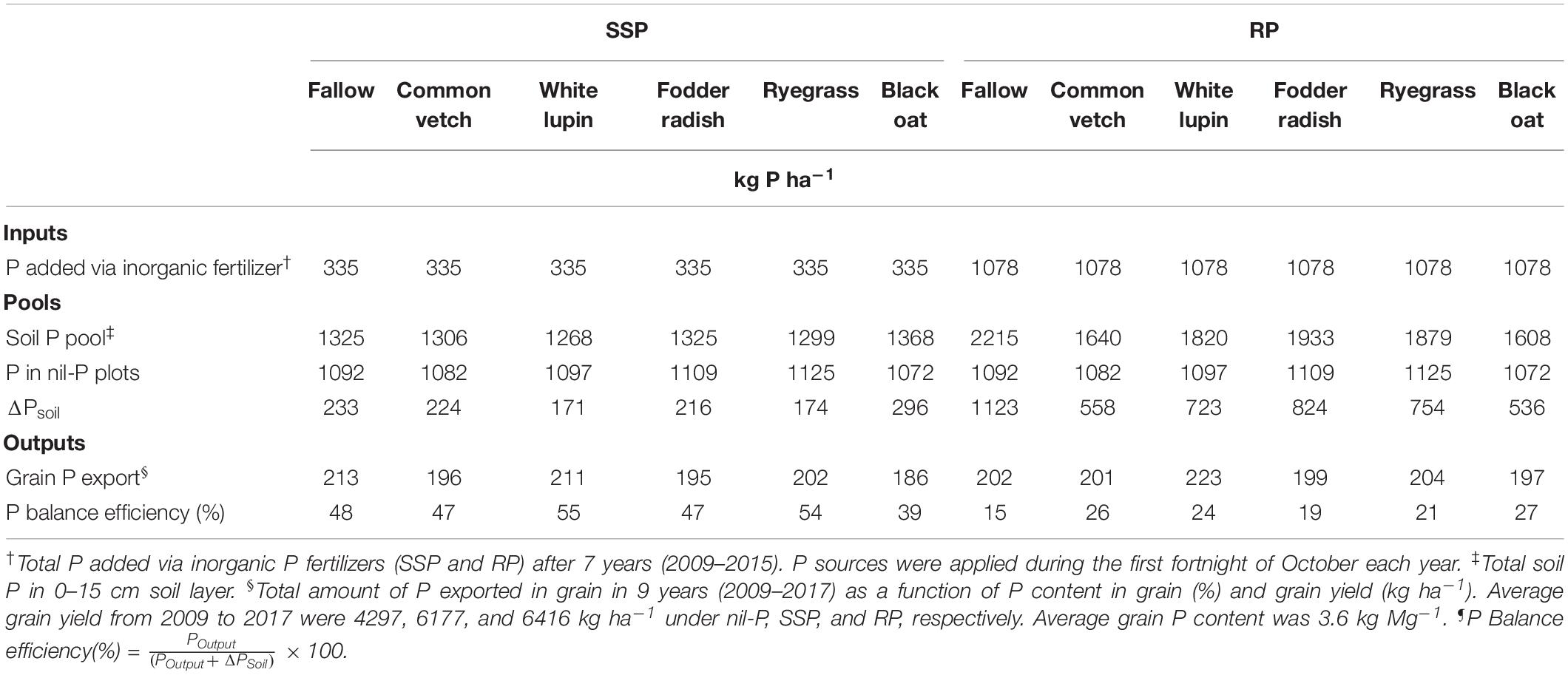
Table 4. P inputs, pools, outputs, and balance efficiency after 9 years in the cropping system receiving single superphosphate (SSP) and rock phosphate (RP).
Cumulative maize grain yield from 2009 to 2017 was enhanced with P application related to nil-P, while different P sources and cover crops did not influence it (Supplementary Table S1). Considering the efficiency of cover crops to take up and mineralize (recycle) P over the time, fodder radish was the most profitable one with taking up 232 kg ha–1 of P in plant tissue from 2009 to 2017, being 2.1 times higher than the average of the other cover crops (112 kg ha–1 of P), while P uptake was not different among other cover crops (Figure 2). Averaged among cover crops, P uptake by them under P application (150–160 kg ha–1) was higher than nil-P (100 kg ha–1), with no changes between SSP and RP (Figure 2).
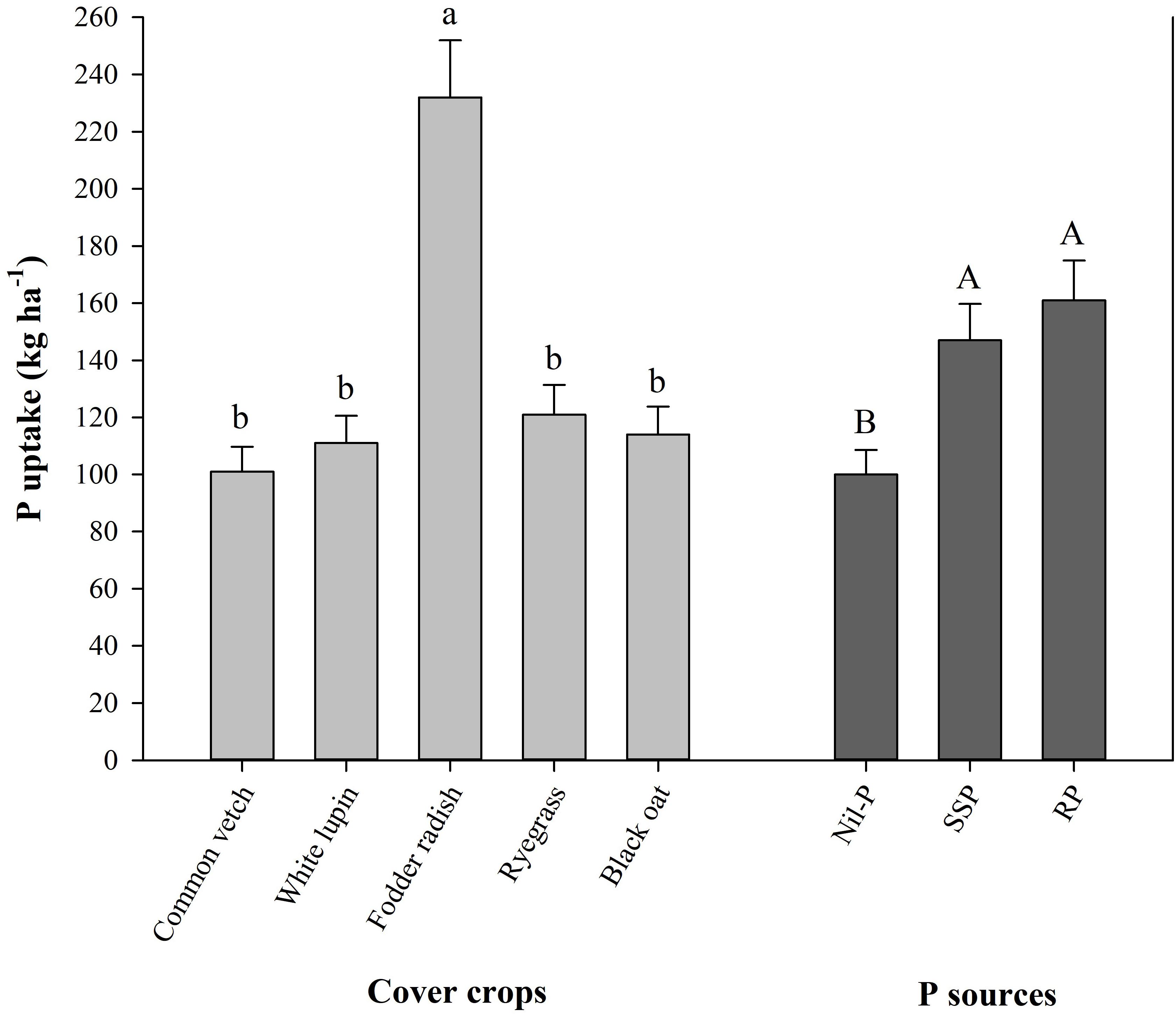
Figure 2. Cumulative shoot P uptake by winter cover crops from 2009 to 2017 affected by cover crops and P sources. Mean values followed by the same tiny letter in cover crops and capital letter in P sources do not differ by t-test (LSD, P < 0.05).
Discussion
In P-unfertilized cropping system, the amounts of labile and mod-labile P fractions were not modified by cover crops related to fallow, showing that cover crops did not take up P from these fractions or they took it up and recycled it back to the soil through plant residues. As cover crops did not show any difference with fallow for non-labile P, the second scenario is more plausible meaning that they took up P from labile and mod-labile fractions and refilled those pools through their biomass. It has been observed that some plants like wheat (Wang et al., 2008; Vu et al., 2010) and common bean (Li et al., 2008) can take up greater amount of available P compared to other crops due to their large root system which explores greater volumes of soil and reducing rhizosphere pH by releasing protons, which potentially can alter soil P lability over the time with crop residue deposition. Acquiring P from non-labile fractions by lupin (Braum and Helmke, 1995; Bais et al., 2006; Le Bayon et al., 2006; Shane et al., 2008; Wang et al., 2008) and ruzigrass (Urochloa ruziziensis) (Almeida and Rosolem, 2016) has been previously reported by many researchers, presumably due to the excretion of high amounts of organic acids (Le Bayon et al., 2006), which was not observed in this study as the amount of non-labile P under white lupin cultivation was higher than fallow in soil surface layer, under both P sources and even under nil-P.
When inorganic P fertilizers were applied, the amount of labile and mod-labile P pools under fallow were higher than under cover crops in the second soil layer evaluated (5–10 cm), showing that cover crops were able to take up P from less labile P fractions in subsurface layers and accumulate it on the surface after the decomposition of their residues (Table 2). Our research shows that their effectiveness is limited to 10 cm soil depth as we did not see changes in 10–15 cm depth. Black oat and common vetch cycled more labile P under SSP and RP, respectively. Contrary to our results, many studies did not observe the effect of cover crops on labile P fractions when available P was not limited (Kuo et al., 2005; Takeda et al., 2009; Rick et al., 2011).
The amount of organic P was also altered by the cultivation of distinct cover crops (Table 3). When SSP was applied, common vetch promoted the highest accumulation of organic P on the surface, while under RP application, this cover crop showed the lowest organic P level and ryegrass was responsible for the highest level on the soil surface layer. Nziguheba et al. (2000) and Sugito et al. (2001) observed an increase in organic P when plant residues were added to soil, while Tiecher et al. (2012) did not observe the effect of different winter crops on organic P measured by ignition method. Although not evaluated in this study, it seems likely that acid phosphatase enzyme activity under common vetch and RP was much higher than the other cover crops as organic P was less than half of the average of the other cover crops. Tiecher et al. (2012) detected the highest activity of this enzyme under vetch cultivation in comparison with oat, radish, lupin, wheat, and fallow. Tarafdar and Jungk (1987) also reported the depletion of organic P in the rhizosphere of wheat due to enhanced phosphatase activity. Common vetch is a non-mycorrhizal plant species which exudes higher amounts of acid phosphatase to compensate the lack of mycorrhizal fungi related to mycorrhizal plant species (Dalla Costa and Lovato, 2004; Kunze et al., 2011). Hallama et al. (2019) in a meta-analysis also showed that extracellular phosphatase activity was enhanced by 20% with cover cropping, being Fabaceae as one of the most effective plant families. Some studies have shown that besides inorganic P, organic P increases with continuous inorganic P application due to higher P uptake and consequently P addition to soil by cover crops and higher synthesis of organic P by soil microbiota (Stewart and Tiessen, 1987; George et al., 2007). This was observed in our study only when SSP was applied. Under RP application, organic P was reduced compared to nil-P. Averaged among cover crops, 38% of P was accumulated as PHCl fraction under RP application (Supplementary Table S2). This P fraction is stable in acid soils (Tiessen and Stewart, 1985; Beck and Sanchez, 1994). Black oat was capable to change this P fraction as the amount of PHCl reduced to half of the other cover crops in soil surface layer. Better RP utilization by black oat is also evidenced by its higher P balance efficiency (27%) compared to the others. Rapeseed was previously introduced as a crop which can efficiently utilize RP in calcareous soils (Habib et al., 1999; Chien et al., 2003), while we recommend black oat for clayey Oxisols; however, it is noteworthy that the interaction between P sources and cover crops is complex and site-specific recommendations are needed (Romanyà and Rovira, 2009; Hallama et al., 2019). The possible mechanism adapted by these crops to utilize RP is the higher proton release by their roots to improve the dissolution of RP in rhizosphere, as reported by many scientists (van Diest, 1981; Bekele et al., 1983; Ruiz and Arvieu, 1990; Hinsinger and Gilkes, 1995; Zoysa et al., 1998).
Averaged among cover crops, the amount of labile P in soil surface layer under phosphate sources was two times higher than nil-P which indicates that the adsorption sites have been saturated after 7 years of P application regardless of P source, resulting in weakening the binding energy of P. This was also observed by other studies in soil subsurface layer in no-tillage system (Rheinheimer et al., 2000; Tiecher et al., 2012; Wyngaard et al., 2012). Under SSP application, PiHID–0.1 and Presidual were sinks of applied P, while under RP application, mostly PHCl and to some extent PiHID (sum of PiHID–0.1 and PiHID–0.5) played this role (Supplementary Table S2). After 18 years of continuous P application in tropical Ultisols, Beck and Sanchez (1994) found out that PiHID–0.1 acts as the sink for fertilizer P added to soil. Stewart et al. (1987) and Dobermann et al. (2002) observed that the excess inorganic P fertilizer entered mostly to PiHID in Ultisols and Oxisols. Otherwise, in less weathered soils, PoHID, PHCl and Presidual were found as the primary sink of applied P (Schmidt et al., 1996; Zhang and MacKenzie, 1997).
Higher P uptake by some cover crops like sorghum, oat, rye, and vetch was previously observed by Karasawa and Takahashi (2015). Higher P uptake by fodder radish in comparison with other cover crops in our research is in accordance with Teles et al. (2017) and Soltangheisi et al. (2018) in a 3-years and 6-years data evaluation of this experiment, respectively. Hallama et al. (2019) also observed high P concentrations in Brassicaceae, like radish. According to Calegari et al. (2013), it is expected that cover crops with higher P uptake (black oat and blue lupin in their research) cycle a higher amount of P, resulting in the higher content of labile and mod-labile P fractions, while we did not observe it in the soil layers under fodder radish cultivation; however, its P uptake was 2.1 times higher than the average of the other cover crops after 9 years. It can be stated that fodder radish is a suitable cover crop when soluble P fertilizers are applied, as it accumulates high amount of P in its tissues and protect P from losses via erosion or leaching, without reducing available P for the summer cash crop. Other studies in Brazilian Oxisols showed that radish enhanced malic acid and soil P availability in comparison with other cover crops (Carvalho et al., 2008; Pavinato et al., 2008). The higher P uptake capacity of fodder radish can be the result of its different P acquisition strategy and needs more investigation. Under limited P condition, the uptake and consequently P release by cover crops was reduced significantly compared to high P condition, where the plots were fertilized. This was previously observed by Blair and Boland (1978) and many other studies. When soil P is restrictive, P released by cover crops would be immobilized by microbial biomass (Bünemann et al., 2012) which reinforces the need for P application for the subsequent crop.
Focusing on organic fractions, PoBIC:PoHID ratio under nil-P reached to 25% (Supplementary Table S2) which shows that Po is an important source of plant available P in the absence of P inputs (Kuo et al., 2005), while it was 14 and 15% under SSP and RP, respectively. Under nil-P, PoHID (sum of PoHID–0.1 and PoHID–0.5) was depleted by 31%, from 51% in 2009 to 20% in 2017, which indicates that these organic P fractions are acting as a source of available P over the time. As mentioned by some authors, without P fertilization, organic P contribution to supply P for plant uptake is higher than inorganic P (Daroub et al., 2001; Soltangheisi et al., 2019).
As expected, the higher P balance efficiency of the system was achieved under SSP in comparison with RP application but it seems that cover crops are more effective at improving the efficiency under RP as this improvement was not observed under SSP application considering the differences between fallow and cover crops. Simpson et al. (2011) presented phase farming as one of the management options to improve the P balance efficiency of farming systems; however, they mentioned that more field studies are required as the majority of the evidences came from glasshouse experiments. Our long-term field experiment confirmed their hypothesis.
Conclusion
Cover crops evaluated in our study were able to take up P from less labile P fractions in subsurface layers and accumulate it on the surface after the decomposition of their residues. Among them, black oat and common vetch cycled more labile P under SSP and RP, respectively. Organic P accumulation on the soil surface was also enhanced with common vetch and ryegrass cultivation under SSP and RP, respectively.
Under RP effect, black oat was capable to remobilize calcium-P to more labile P fractions as the amount of it reduced to half of the other cover crops in soil surface layer. Better RP utilization by black oat is also evidenced by its higher P balance efficiency compared to the other cover crops in our study in clayey Oxisol. Fodder radish took up the highest amount of P in comparison with other cover crops, being suitable when soluble P fertilizers are applied as it accumulates high amounts of P in its tissues and protect it from runoff/erosion losses.
The highest P balance efficiency was achieved under SSP compared to RP application. Otherwise, cover crops were more effective at improving the efficiency under RP considering the balance between fallow and cover crops.
Data Availability Statement
All datasets generated for this study are included in the article/Supplementary Material.
Author Contributions
AS conceived the data analysis and finalized all text, figures, and tables. LS collected plant and soil samples. PP and LS set up the experiment. AT guided the laboratory work. All authors contributed to the main text.
Funding
The authors are grateful to São Paulo Research Foundation (FAPESP), which supported the scholarship to AS (Grant Nos. 2017/11332-5 and 2019/21532-7).
Conflict of Interest
The authors declare that the research was conducted in the absence of any commercial or financial relationships that could be construed as a potential conflict of interest.
Acknowledgments
We thank the National Council for Scientific and Technological Development – CNPq for funding this research under project 574950/2008-7. We also thank the Federal Technology University of Paraná, Dois Vizinhos (UTFPR), and College of Agriculture “Luiz de Queiroz” – ESALQ/USP for providing the experimental and laboratory facilities and necessary materials for the research, development and analysis.
Supplementary Material
The Supplementary Material for this article can be found online at: https://www.frontiersin.org/articles/10.3389/fenvs.2020.00013/full#supplementary-material
References
Almeida, D. S., and Rosolem, C. A. (2016). Ruzigrass grown in rotation with soybean increases soil labile phosphorus. Agron. J. 108, 2444–2452. doi: 10.2134/agronj2015.0478
Bais, H. P., Weir, T. L., Perry, L. G., Gilroy, S., and Vivanco, J. M. (2006). The role of root exudates in rhizosphere interactions with plants and other organisms. Annu. Rev. Plant Biol. 57, 233–266. doi: 10.1146/annurev.arplant.57.032905.105159
Beck, M. A., and Sanchez, P. A. (1994). Soil phosphorus fraction dynamics during 18 years of cultivation on a Typic Paleudult. Soil Sci. Soc. Am. J. 58, 1424–1431. doi: 10.2136/sssaj1994.03615995005800050021x
Bekele, T., Cino, B. J., Ehlert, P. A. I., Van der Maas, A. A., and Van Diest, A. (1983). An evaluation of plant-borne factors promoting the solubilization of alkaline rock phosphates. Plant Soil 75, 361–378. doi: 10.1007/bf02369971
Blair, G. J., and Boland, O. W. (1978). The release of phosphorus from plant material added to soil. Soil Res. 16, 101–111. doi: 10.1071/sr9780101
Boitt, G., Schmitt, D. E., Gatiboni, L. C., Wakelin, S. A., Black, A., Sacomori, W., et al. (2018). Fate of phosphorus applied to soil in pig slurry under cropping in southern Brazil. Geoderma 321, 164–172. doi: 10.1016/j.geoderma.2018.02.010
Braum, S. M., and Helmke, P. A. (1995). White lupin utilizes soil phosphorus that is unavailable to soybean. Plant Soil 176, 95–100. doi: 10.1007/bf00017679
Bünemann, E. K., Oberson, A., Liebisch, F., Keller, F., Annaheim, K. E., Huguenin-Elie, O., et al. (2012). Rapid microbial phosphorus immobilization dominates gross phosphorus fluxes in a grassland soil with low inorganic phosphorus availability. Soil Biol. Biochem. 51, 84–95. doi: 10.1016/j.soilbio.2012.04.012
Calegari, A., Tiecher, T., Hargrove, W. L., Ralisch, R., Tessier, D., de Tourdonnet, S., et al. (2013). Long-term effect of different soil management systems and winter crops on soil acidity and vertical distribution of nutrients in a Brazilian Oxisol. Soil Till. Res. 133, 32–39. doi: 10.1016/j.still.2013.05.009
Carvalho, A. M., Bustamante, M. M. C., Sousa Junior, J., and Vivaldi, L. J. (2008). Decomposição de resíduos vegetais em Latossolo sob cultivo de milho e plantas de cobertura. Rev. Bras. Cienc. Solo. 32, 2831–2838. doi: 10.1590/s0100-06832008000700029
Chien, S. H., Carmona, G., Henao, J., and Prochnow, L. I. (2003). Evaluation of rape response to different sources of phosphate rock in an alkaline soil. Commun. Soil Sci. Plant Anal. 34, 1825–1835. doi: 10.1081/css-120023217
Condron, L. M., Goh, K. M., and Newman, R. H. (1985). Nature and distribution of soil phosphorus as revealed by a sequential extraction method followed by 31P nuclear magnetic resonance analysis. J. Soil Sci. 36, 199–207. doi: 10.1111/j.1365-2389.1985.tb00324.x
Cordell, D., and White, S. (2011). Peak phosphorus: clarifying the key issues of a vigorous debate about long-term phosphorus security. Sustainability 3, 2027–2049. doi: 10.3390/su3102027
Cordell, D., and White, S. (2014). Life’s bottleneck: sustaining the world’s phosphorus for a food secure future. Ann. Rev. Env. Resour. 39, 161–188. doi: 10.1146/annurev-environ-010213-113300
Cross, A. F., and Schlesinger, W. H. (1995). A literature review and evaluation of the. Hedley fractionation: applications to the biogeochemical cycle of soil phosphorus in natural ecosystems. Geoderma 64, 197–214. doi: 10.1016/0016-7061(94)00023-4
Dalla Costa, M., and Lovato, P. E. (2004). Fosfatases na dinâmica do fósforo do solo sob culturas de cobertura com espécies micorrízicas e não micorrízicas. Pesq. Agropec. Bras. 39, 603–605. doi: 10.1590/s0100-204x2004000600013
Damon, P. M., Bowden, B., Rose, T., and Rengel, Z. (2014). Crop residue contributions to phosphorus pools in agricultural soils: a review. Soil Biol. Biochem. 74, 127–137. doi: 10.1016/j.soilbio.2014.03.003
Daroub, S. H., Ellis, B. G., and Robertson, G. P. (2001). Effect of cropping and low-chemical input systems on soil phosphorus fractions. Soil Sci. 166, 281–291. doi: 10.1097/00010694-200104000-00007
Daroub, S. H., Pierce, F. J., and Ellis, B. G. (2000). Phosphorus fractions and fate of phosphorus-33 in soils under plowing and no-tillage. Soil Sci. Soc. Am. J. 64, 170–176. doi: 10.2136/sssaj2000.641170x
Dick, W. A., and Tabatabai, M. A. (1977). Determination of orthophosphate in aqueous solutions containing labile organic and inorganic phosphorus compounds 1. J. Environ. Qual. 6, 82–85. doi: 10.2134/jeq1977.00472425000600010018x
Dobermann, A., George, T., and Thevs, N. (2002). Phosphorus fertilizer effects on soil phosphorus pools in acid upland soils. Soil Sci. Soc. Am. J. 66, 652–660. doi: 10.2136/sssaj2002.0652
Doneda, A., Aita, C., Giacomini, S. J., Miola, E. C. C., Giacomini, D. A., Schirmann, J., et al. (2012). Fitomassa e decomposição de resíduos de plantas de cobertura puras e consorciadas. Rev. Bras. Cienc. Solo. 36, 1714–1723. doi: 10.1590/s0100-06832012000600005
Elmore, R. W., and Roeth, F. W. (1999). Corn kernel weight and grain yield stability during post-maturity drydown. J. Produc. Agric. 12, 300–305. doi: 10.2134/jpa1999.0300
Ferreira, P. A. A., Girotto, E., Trentin, G., Miotto, A., Melo, G. W. D., Ceretta, C. A., et al. (2014). Biomass decomposition and nutrient release from black oat and hairy vetch residues deposited in a vineyard. Rev. Bras. Cienc. Solo. 38, 1621–1632. doi: 10.1590/s0100-06832014000500027
Frossard, E., Brossard, M., Hedley, M. J., and Metherell, A. (1995). Reactions Controlling the Cycling of P in Soils. Hoboken, NJ: John Wiley & Sons Ltd. doi: 10.1007/978-1-4613-8847-0_1
George, T. S., Simpson, R. J., Hadobas, P. A., Marshall, D. J., and Richardson, A. E. (2007). Accumulation and phosphatase-lability of organic phosphorus in fertilised pasture soils. Aus. J. Agric. Res. 58, 47–55. doi: 10.1071/ar06167
Habib, L., Chien, S. H., Carmona, G., and Henao, J. (1999). Rape response to a Syrian phosphate rock and its mixture with triple superphosphate on a limed alkaline soil. Commun. Soil Sci. Plant Anal. 30, 449–456. doi: 10.1080/00103629909370216
Hallama, M., Pekrun, C., Lambers, H., and Kandeler, E. (2019). Hidden miners–the roles of cover crops and soil microorganisms in phosphorus cycling through agroecosystems. Plant Soil 434, 7–45. doi: 10.1007/s11104-018-3810-7
Hedley, M. J., Stewart, J. W. B., and Chauhan, B. (1982). Changes in inorganic and organic soil phosphorus fractions induced by cultivation practices and by laboratory incubations 1. Soil Sci. Soc. Am. J. 46, 970–976. doi: 10.2136/sssaj1982.03615995004600050017x
Hinsinger, P. (2001). Bioavailability of soil inorganic P in the rhizosphere as affected by root-induced chemical changes: a review. Plant Soil 237, 173–195.
Hinsinger, P., and Gilkes, R. J. (1995). Root-induced dissolution of phosphate rock in the rhizosphere of lupins grown in alkaline soil. Soil Res. 33, 477–489. doi: 10.1071/sr9950477
Holford, I. C. R. (1997). Soil phosphorus: its measurement, and its uptake by plants. Soil Res. 35, 227–240. doi: 10.1071/s96047
Horst, W. J., Kamh, M., Jibrin, J. M., and Chude, V. O. (2001). Agronomic measures for increasing P availability to crops. Plant Soil. 237, 211–223. doi: 10.1023/a:1013353610570
Iyamuremye, F., Dick, R. P., and Baham, J. (1996). Organic amendments and phosphorus dynamics: II. Distribution of soil phosphorus fractions. Soil Sci. 161, 436–443. doi: 10.1097/00010694-199607000-00003
Kamh, M., Horst, W. J., Amer, F., Mostafa, H., and Maier, P. (1999). Mobilization of soil and fertilizer phosphate by cover crops. Plant Soil. 211:19. doi: 10.1007/978-3-663-01125-5_22
Karasawa, T., and Takahashi, S. (2015). Introduction of various cover crop species to improve soil biological P parameters and P uptake of the following crops. Nutr. Cycl. Agroecosyst. 103, 15–28. doi: 10.1007/s10705-015-9715-4
Kunze, A., Costa, M. D., Epping, J., Loffaguen, J. C., Schuh, R., and Lovato, P. E. (2011). Phosphatase activity in sandy soil influenced by mycorrhizal and non-mycorrhizal cover crops. Rev. Bras. Cien. Solo. 35, 705–711. doi: 10.1590/s0100-06832011000300005
Kuo, S., Huang, B., and Bembenek, R. (2005). Effects of long-term phosphorus fertilization and winter cover cropping on soil phosphorus transformations in less weathered soil. Biol. Fert. Soils 41, 116–123. doi: 10.1007/s00374-004-0807-6
Le Bayon, R. C., Weisskopf, L., Martinoia, E., Jansa, J., Frossard, E., Keller, F., et al. (2006). Soil phosphorus uptake by continuously cropped Lupinus albus: a new microcosm design. Plant Soil 283, 309–321. doi: 10.1007/s11104-006-0021-4
Li, H., Shen, J., Zhang, F., Clairotte, M., Drevon, J. J., Le Cadre, E., et al. (2008). Dynamics of phosphorus fractions in the rhizosphere of common bean (Phaseolus vulgaris L.) and durum wheat (Triticum turgidum durum L.) grown in monocropping and intercropping systems. Plant Soil 312, 139–150. doi: 10.1007/s11104-007-9512-1
Maltais-Landry, G., and Frossard, E. (2015). Similar phosphorus transfer from cover crop residues and water-soluble mineral fertilizer to soils and a subsequent crop. Plant Soil 393, 193–205. doi: 10.1007/s11104-015-2477-6
Murphy, J., and Riley, J. P. (1962). A modified single solution method for the determination of phosphate in natural waters. Anal. Chim. Acta 27, 31–36. doi: 10.1016/s0003-2670(00)88444-5
Nachimuthu, G., Guppy, C., Kristiansen, P., and Lockwood, P. (2009). Isotopic tracing of phosphorus uptake in corn from 33P labelled legume residues and 32P labelled fertilisers applied to a sandy loam soil. Plant Soil 314:303. doi: 10.1007/s11104-008-9730-1
Noack, S. R., McBeath, T. M., McLaughlin, M. J., Smernik, R. J., and Armstrong, R. D. (2014). Management of crop residues affects the transfer of phosphorus to plant and soil pools: results from a dual-labelling experiment. Soil Biol. Biochem. 71, 31–39. doi: 10.1016/j.soilbio.2013.12.022
Nziguheba, G., Merckx, R., Palm, C. A., and Rao, M. R. (2000). Organic residues affect phosphorus availability and maize yields in a Nitisol of western Kenya. Biol. Fert. Soils 32, 328–339. doi: 10.1007/s003740000256
Pavinato, P. S., Merlin, A., and Rosolem, C. A. (2008). Organic compounds from plant extracts and their effect on soil phosphorus availability. Pesqui. Agropecu. Bras. 43, 1379–1388. doi: 10.1590/s0100-204x2008001000017
Pavinato, P. S., Rodrigues, M., Soltangheisi, A., Sartor, L. R., and Withers, P. J. A. (2017). Effects of cover crops and phosphorus sources on maize yield, phosphorus uptake, and phosphorus use efficiency. Agron. J. 109, 1039–1047. doi: 10.2134/agronj2016.06.0323
Pearse, S. J., Veneklaas, E. J., Cawthray, G., Bolland, M. D., and Lambers, H. (2007). Carboxylate composition of root exudates does not relate consistently to a crop species’ ability to use phosphorus from aluminium, iron or calcium phosphate sources. New Phytol. 173, 181–190. doi: 10.1111/j.1469-8137.2006.01897.x
Raghothama, K. G. (1999). Phosphate acquisition. Annu. Rev. Plant Biol. 50, 665–693. doi: 10.1146/annurev.arplant.50.1.665
Raij, B. V., Cantarella, H., Quaggio, J. A., and Furlani, A. M. C. (1997). Recomendações de Adubação e Calagem Para o Estado de São Paulo. Campinas: Instituto Agronômico/Fundação IAC.
Rheinheimer, D. S., Anghinoni, I., and Kaminski, J. (2000). Depletion of inorganic phosphorus fractions by successive extraction with resin in different soils and management systems. Rev. Bras. Cien. Solo. 24, 345–354. doi: 10.1590/s0100-06832000000200012
Richardson, A. E., Lynch, J. P., Ryan, P. R., Delhaize, E., Smith, F. A., Smith, S. E., et al. (2011). Plant and microbial strategies to improve the phosphorus efficiency of agriculture. Plant Soil 349, 121–156. doi: 10.1007/s11104-011-0950-4
Rick, T. L., Jones, C. A., Engel, R. E., and Miller, P. R. (2011). Green manure and phosphate rock effects on phosphorus availability in a northern Great Plains dryland organic cropping system. Org. Agric. 1, 81–90. doi: 10.1007/s13165-011-0007-2
Romanyà, J., and Rovira, P. (2009). Organic and inorganic P reserves in rain-fed and irrigated calcareous soils under long-term organic and conventional agriculture. Geoderma 151, 378–386. doi: 10.1016/j.geoderma.2009.05.009
Ruiz, L., and Arvieu, J. C. (1990). Measurement of pH gradients in the rhizosphere. Symbiosis 9, 71–75.
Schmidt, J. P., Buol, S. W., and Kamprath, E. J. (1996). Soil phosphorus dynamics during seventeen years of continuous cultivation: fractionation analyses. Soil Sci. Soc. Am. J. 60, 1168–1172. doi: 10.2136/sssaj1996.03615995006000040030x
Shane, M. W., Lambers, H., Cawthray, G. R., Kuhn, A. J., and Schurr, U. (2008). Impact of phosphorus mineral source (Al–P or Fe–P) and pH on cluster-root formation and carboxylate exudation in Lupinus albus L. Plant Soil 304:169. doi: 10.1007/s11104-007-9535-7
Simpson, R. J., Oberson, A., Culvenor, R. A., Ryan, M. H., Veneklaas, E. J., Lambers, H., et al. (2011). Strategies and agronomic interventions to improve the phosphorus-use efficiency of farming systems. Plant Soil 349, 89–120. doi: 10.1007/s11104-011-0880-1
Sociedade Brasileira de Ciência do Solo [SBCS]. (2016). Manual de calagem e adubação para os Estados do Rio Grande do Sul e Santa Catarina. Nucleo Regional Sul – (s.l.): Comissão de Química e Fertilidade do Solo 0 RS/SC. Viçosa, MG: SBCS, 376.
Soil Survey Staff, (1999). Soil Taxonomy: A Basic System of Soil Classification for Making and Interpreting Soil Surveys, 2nd Edn. Washington, DC: Natural Resources Conservation Service, 436.
Soltangheisi, A., de Moraes, M. T., Cherubin, M. R., Alvarez, D. O., de Souza, L. F., Bieluczyk, W., et al. (2019). Forest conversion to pasture affects soil phosphorus dynamics and nutritional status in Brazilian Amazon. Soil Till. Res. 194:104330. doi: 10.1016/j.still.2019.104330
Soltangheisi, A., Rodrigues, M., Coelho, M. J. A., Gasperini, A. M., Sartor, L. R., and Pavinato, P. S. (2018). Changes in soil phosphorus lability promoted by phosphate sources and cover crops. Soil Till. Res. 179, 20–28. doi: 10.1016/j.still.2018.01.006
Stewart, J. W. B., O’Halloran, I. P., and Kachanoski, R. G. (1987). Influence of texture and management practices on the forms and distribution of soil phosphorus. Can. J. Soil Sci. 67, 147–163. doi: 10.4141/cjss87-013
Stewart, J. W. B., and Tiessen, H. (1987). Dynamics of soil organic phosphorus. Biogeochemistry 4, 41–60. doi: 10.1007/bf02187361
Sugito, T., Yoshida, K., and Nitta, T. (2001). Changes of phosphorus forms in soil with organic matter application. Jpn. J. Soil Sci. Plant Nutr. 72, 195–205.
Takeda, M., Nakamoto, T., Miyazawa, K., and Murayama, T. (2009). Phosphorus transformation in a soybean-cropping system in Andosol: effects of winter cover cropping and compost application. Nutr. Cycl. Agroecosys. 85, 287–297. doi: 10.1007/s10705-009-9267-6
Tarafdar, J. C., and Jungk, A. (1987). Phosphatase activity in the rhizosphere and its relation to the depletion of soil organic phosphorus. Biol. Fert. Soils 3, 199–204. doi: 10.1007/bf00640630
Tedesco, M. J., Gianello, C., Bissani, C. A., Bohnen, H., and Volkweiss, S. J. (1995). Análises de solo, plantas e outros materiais. (Boletim Técnico, 5), 2 Edn. Porto Alegre: Universidade Federal do Rio Grande do Sul, 174.
Teles, A. P. B., Rodrigues, M., Bejarano Herrera, W. F., Soltangheisi, A., Sartor, L. R., Withers, P. J. A., et al. (2017). Do cover crops change the lability of phosphorus in a clayey subtropical soil under different phosphate fertilizers? Soil Use Manag. 33, 34–44. doi: 10.1111/sum.12327
Thibaud, M. C., Morel, C., and Fardeau, J. C. (1988). Contribution of phosphorus issued from crop residues to plant nutrition. Soil Sci. Plant Nutr. 34, 481–491. doi: 10.1080/00380768.1988.10416464
Tiecher, T., dos Santos, D. R., and Calegari, A. (2012). Soil organic phosphorus forms under different soil management systems and winter crops, in a long term experiment. Soil Till. Res. 124, 57–67. doi: 10.1016/j.still.2012.05.001
Tiessen, H., and Stewart, J. W. (1985). “The biogeochemistry of soil phosphorus,” in Proceedings of the 6th International Symposium on Environmental Biogeochemistry Planetary Ecology. Santa Fe, 463–472.
van Diest, A. (1981). Rock-phosphate mobilization induced by the alkaline uptake pattern of legumes utilizing symbiotically fixed nitrogen. Plant Soil 61, 27–42. doi: 10.1007/bf02277360
Vanlauwe, B., Diels, J., Sanginga, N., Carsky, R. J., Deckers, J., and Merckx, R. (2000). Utilization of rock phosphate by crops on a representative toposequence in the Northern Guinea savanna zone of Nigeria: response by maize to previous herbaceous legume cropping and rock phosphate treatments. Soil Biol. Biochem. 32, 2079–2090. doi: 10.1016/s0038-0717(00)00150-4
Vu, D. T., Tang, C., and Armstrong, R. D. (2010). Transformations and availability of phosphorus in three contrasting soil types from native and farming systems: a study using fractionation and isotopic labeling techniques. J. Soil Sediment. 10, 18–29. doi: 10.1007/s11368-009-0068-y
Wang, X., Tang, C., Guppy, C. N., and Sale, P. W. G. (2008). Phosphorus acquisition characteristics of cotton (Gossypium hirsutum L.), wheat (Triticum aestivum L.) and white lupin (Lupinus albus L.) under P deficient conditions. Plant Soil 312, 117–128. doi: 10.1007/s11104-008-9589-1
Wasaki, J., Kojima, S., Maruyama, H., Haase, S., Osaki, M., and Kandeler, E. (2008). Localization of acid phosphatase activities in the roots of white lupin plants grown under phosphorus-deficient conditions. Soil Sci. Plant Nutr. 54, 95–102. doi: 10.1111/j.1747-0765.2007.00207.x
Wasaki, J., Maruyama, H., Tanaka, M., Yamamura, T., Dateki, H., Shinano, T., et al. (2009). Overexpression of the LASAP2 gene for secretory acid phosphatase in white lupin improves the phosphorus uptake and growth of tobacco plants. Soil Sci. Plant Nutr. 55, 107–113. doi: 10.1111/j.1747-0765.2008.00329.x
Withers, P. J., Rodrigues, M., Soltangheisi, A., De Carvalho, T. S., Guilherme, L. R., Benites, V. D. M., et al. (2018). Transitions to sustainable management of phosphorus in Brazilian agriculture. Sci. Rep. 8:2537. doi: 10.1038/s41598-018-20887-z
Wyngaard, N., Echeverria, H. E., Vidaurreta, A., Picone, L. I., and Divito, G. A. (2012). Carbon and phosphorus in soil particulate fraction: effect of continuous agriculture, tillage and fertilization. Geophys. Res. Abstr. 14:3377.
Zhang, T. Q., and MacKenzie, A. F. (1997). Changes of phosphorous fractions under continuous corn production in a temperate clay soil. Plant Soil 192, 133–139. doi: 10.1023/a:1004232417658
Keywords: common vetch (Vicia sativa), white lupin (Lupinus albus), fodder radish (Raphanus sativus), ryegrass (Lolium multiflorum), black oat (Avena strigosa), Hedley P fractionation
Citation: Soltangheisi A, Teles APB, Sartor LR and Pavinato PS (2020) Cover Cropping May Alter Legacy Phosphorus Dynamics Under Long-Term Fertilizer Addition. Front. Environ. Sci. 8:13. doi: 10.3389/fenvs.2020.00013
Received: 30 October 2019; Accepted: 22 January 2020;
Published: 11 February 2020.
Edited by:
Dionisios Gasparatos, Aristotle University of Thessaloniki, GreeceReviewed by:
Jihui Tian, South China Agricultural University, ChinaJinyang Wang, Nanjing Agricultural University, China
Copyright © 2020 Soltangheisi, Teles, Sartor and Pavinato. This is an open-access article distributed under the terms of the Creative Commons Attribution License (CC BY). The use, distribution or reproduction in other forums is permitted, provided the original author(s) and the copyright owner(s) are credited and that the original publication in this journal is cited, in accordance with accepted academic practice. No use, distribution or reproduction is permitted which does not comply with these terms.
*Correspondence: Amin Soltangheisi, soltangheisi@usp.br; soltangheise@gmail.com