How Microbial Biofilms Control the Environmental Fate of Engineered Nanoparticles?
- 1Department of Civil and Environmental Engineering, Northwestern University, Evanston, IL, United States
- 2Aix Marseille Univ, Centre National de la Recherche Scientifique (CNRS), Institut de Recherche pour le Dŕveloppement (IRD), Institut National de Recherche pourl’agriculture, l’alimentation et l’environnement (INRAE), Coll France, Centre Européen de Recherche et d’Enseignement en Géosciences de l’Environnement (CEREGE), Aix-en-Provence, France
- 3Université de Pau et des Pays de l’Adour, E2S Université de Pau et des Pays de l’Adour (UPPA), Centre National de la Recherche Scientifique (CNRS), Institut des Sciences Analytiques et de Physico-Chimie pour l’Environnement et les Matériaux (IPREM), UMR 5254, Pau, France
- 4Centre de coopération International en Recherche Agronomique pourle Développement (CIRAD), Unité Propre de Recherche (UPR) Recyclage et Risque, Montpellier, France
- 5Recyclage et Risque, University of Montpellier, Centre de coopération International en Recherche Agronomique pour le Développement (CIRAD), Montpellier, France
- 6Université de Paris, Institut de Physique du Globe de Paris, UMR 7154, Centre National de la Recherche Scientifique (CNRS), Paris, France
Predicting the fate of engineered nanoparticles (ENPs) once they are released in the environment is essential to evaluate their impacts to ecosystems. Microbial biofilms, as highly reactive compartments in soils and sediments, have the potential to impose strong controls on ENPs life cycle in natural settings. However, information regarding impacts of biofilms toward ENPs environmental fate are not easily accessible, and such evidences are collected and discussed in this review, in order to identify common trends and to better constrain the role played by these microbial structures. Biofilms are reported to exhibit important ENPs accumulation capacities, and short to long-term ENPs immobilization can thus be expected. Mechanisms that govern such accumulation and ENPs migration within biofilms depend strongly on electrostatic and hydrophobic interactions, as well as biofilm structural properties, such as density and permeability. They are a combination of key parameters that include ENPs size and surface properties, mineral substrate reactivity, ability to develop organic corona around ENPs, or formation of aggregates within the biofilm thickness. In addition, these microbial structures exhibit highly reactive microenvironments, and are consequently able to impose major ENPs transformations such as dissolution, through ligand- or redox-mediated pathways, as well as passivation or stabilization processes. Interestingly, exposure to toxic ENPs can even trigger a response from micro-organisms biofilms which has the potential to strongly modify ENPs speciation. Promising approaches to investigate the role of microbial biofilms for ENPs cycling in realistic systems are introduced through the use of mesocosms, medium-size replicated ecosystems that allow to integrate the complexity of natural settings. Finally, biofilm-mediated nanoparticles synthesis in man-impacted systems is presented. This raises important questions regarding biofilms role as secondary sources of nanoparticles.
Introduction
Since their generalized synthesis in the late 1980s, the use and production of engineered nanoparticles (ENPs) have grown steadily (Giese et al., 2018). This specific class of material, defined as particles with at least one dimension inferior to 100 nm, present an important variety of compositions (both organic and inorganic), shapes (spheres, rods, nanotubes), sizes, and functionalized capping agents at their surface (various types of polymers or inorganic coatings). Attached to their nanometric dimensions, the unique physico-chemical properties of ENPs have been widely exploited in numerous fields including electronics, optics, medicine, cosmetics, energy or informatics (Auffan et al., 2009; Piccinno et al., 2012). However, the increasing number of products incorporating ENPs over the past 20 years (Vance et al., 2015) combined to high production volumes of approximately 300,000 metric tons per year (Keller et al., 2013), have raised important concerns regarding the release of these highly reactive ENPs in the environment, and subsequently their potential impacts on ecosystems. Keller et al. (2013) estimated that 63–91% of all produced ENPs were entering landfills, soil, water, and air annually, either during their utilization or at the end of life of ENPs-containing products (Mueller and Nowack, 2008).
Although some studies have recently been able to directly measure the concentration of ENPs in rivers (Peters et al., 2018; Wang et al., 2020), there are still major technical limitations to quantify accurately ENPs presence in the environment. To circumvent these difficulties, material flow models have been developed in the last years (Mueller and Nowack, 2008; Gottschalk et al., 2009; Keller et al., 2013) in order to estimate the flows and sinks of different ENPs types in the environment. These approaches based on production volumes and, more recently, time-dependent ENPs release from nano-products provided estimations of ENPs release in natural systems (Sun et al., 2016; Wang and Nowack, 2018). Thanks to those models, landfills, soils and sediments have been identified as the main environmental compartments acting as sinks for ENPs (Sun et al., 2016; Wang and Nowack, 2018). However, these approaches are mostly designed to quantify fluxes entering environmental and technical compartments and do not provide any information regarding ENPs fate and impact once in the environment. The high degree of complexity inherent to soils and sediments significantly complexifies studies on ENPs transformations and transport in such environmental matrices (Levard et al., 2012; Montano et al., 2014; Layet et al., 2017; Xu, 2018). Rodrigues et al. (2016) listed the principal factors and processes that control the behavior of ENPs in those matrices, which are homoaggregation, heteroaggregation with organic matter (OM), pore straining, oxidation/dissolution reactions, complexation with OM, and interaction with the mineral surfaces. In particular, the latter is considered of first importance when considering metal(loid)s, OM, colloids and even ENPs cycling in the environment (Brown et al., 1999). Moreover, the development of a microorganisms-based coating at the mineral surfaces creates a highly reactive interface that strongly impacts the fate of toxic and essential elements in the environment (Brown, 2001; Templeton et al., 2001, 2003a). In all subsurface environments where they can develop, i.e., from soils to several kilometers depth below the surface, microorganisms tend to form biofilms, structures in which microorganisms are encased in highly hydrated 3D-matrix composed of exopolymeric substances (EPS) (Costerton et al., 1995; Ménez et al., 2012; Flemming and Wuertz, 2019). The transport limitations attached to these structures in addition with metabolic activity favor the creation of microenvironments within the biofilm thickness (Stewart, 2003), which, in association to the high density of functional groups, confers a very high and specific reactivity at the mineral/biofilm/solution interface.
While a large amount of studies exists on ENPs toxicity toward microorganisms and microbial biofilms (Fulaz et al., 2019b), research focusing on biofilms impacts on ENPs fate in the environment are scarce and systematic information is not easily accessible. Herein, we report the current knowledge relative to controls exerted by microbial biofilms on ENPs transformations in soils, sediments or sludge systems. A critical analysis of biofilm organization and reactivity is introduced, as well as a description of the physical interactions between microbial biofilms and ENPs. Mechanisms of ENPs dissolution, passivation, and stabilization when in contact with microbial biofilms, or their EPS matrix, are thoroughly discussed. Promising strategies based on the use of mesocosms to investigate biofilms impacts in realistic dynamic ecosystems are presented. Finally, the generation of ENPs by biofilms as a defense mechanism when associated with anthropogenic activities are also discussed.
Microbial Biofilms in Natural Systems
Occurrence, Structure, and Overall Reactivity
Bacteria and archaea constitute major phylogenetic groups with reported concentrations around 107–1012 cells/g of soils (Watt et al., 2006), and a total biomass estimated at 77 Gt C on Earth (Bar-On et al., 2018). They are ubiquitous in all environments, from subsurface aquatic systems to locations several kilometers deep in the lithosphere. In most cases, soil microorganisms are organized as communities called biofilms, where microbial cells are encased in a 3-dimensional organic matrix (Costerton et al., 1987; Flemming and Wuertz, 2019). However, despite their high occurrence in soils, biofilms are mostly concentrated in small scale microhabitats that encompass less than 1% of total soil volume (Young et al., 2008). There, biofilms are found as 2–10 μm thick structures, mainly located at the surfaces of soil pores (Young et al., 2008; Flemming and Wuertz, 2019). The resulting microbial hotspots and high cell densities (Kuzyakov and Blagodatskaya, 2015; Nunan, 2017) are correlated to specific reactive microenvironments (Figure 1), which are associated with either metabolic activities or to the specific organization of these structures (Kuzyakov and Blagodatskaya, 2015; Nunan, 2017).
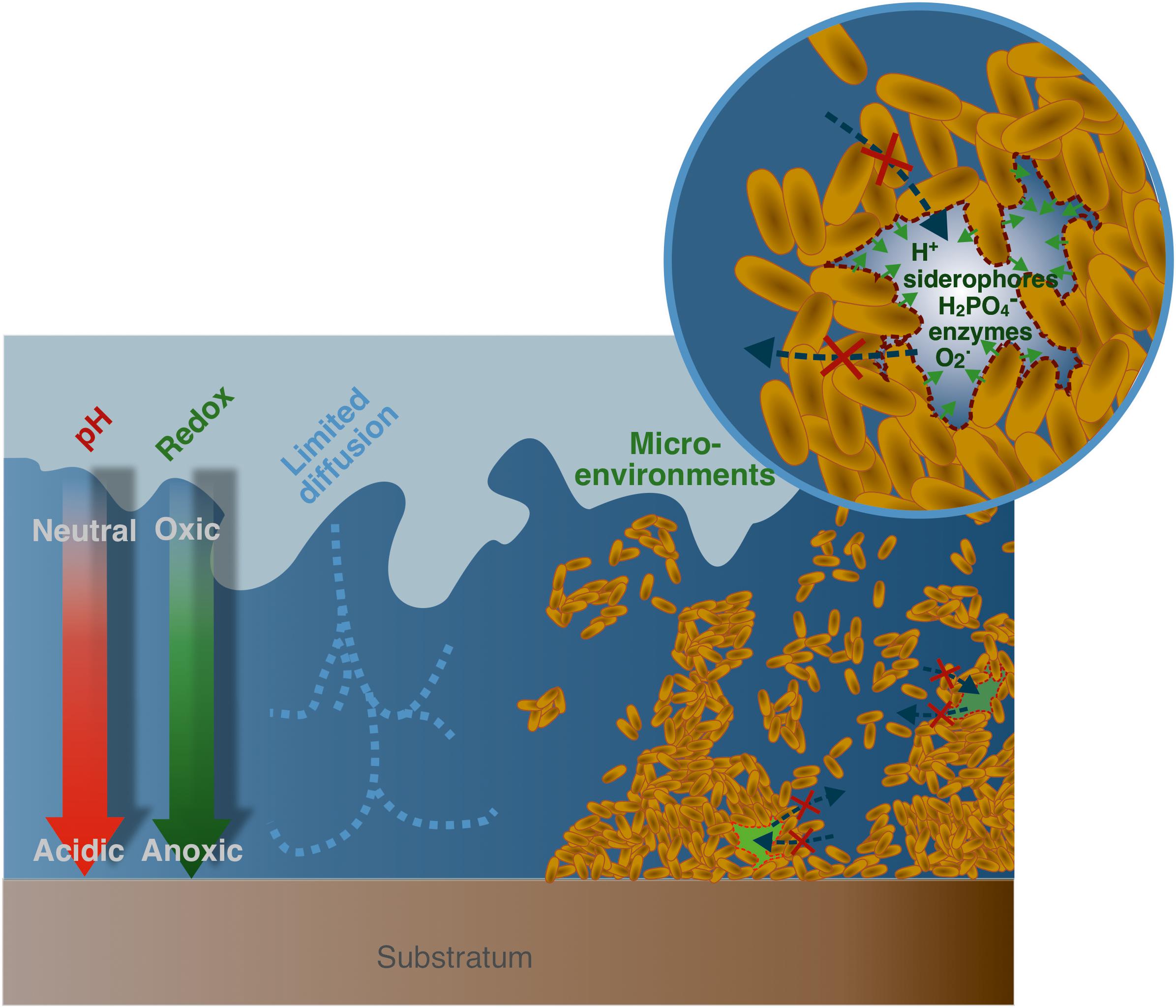
Figure 1. Physico-chemical properties of microbial biofilms. From left to right, existence of gradients in pH and redox conditions with depth; diffusion limited transport of nutriments, molecules (such as siderophores, enzymes), and metabolites; existence of microenvironments within the biofilm thickness that exhibit specific physico-chemical conditions (for instance low pH, high siderophore, superoxide, enzymes, or phosphate concentrations) at the local scale.
Indeed, microbial cell walls and membranes are known to exhibit very elevated specific surface areas, as well as high reactive site densities (Fein et al., 1998; Borrok et al., 2005). For bacteria, the overall surface charge is usually reported to originate from the presence of carboxyl moieties (pKa in the range 3–4.5) and phosphoryl groups (pKa in the range 7–8), both functions that exhibit a negative charge when deprotonated, as well as positively charged amino groups at neutral pH (pKa in the range 8–11). In addition, some recent studies highlighted a strong affinity of thiol groups toward soft metals (e.g., Hg, Cd), despite their lower density at the cell surfaces in comparison to other functional sites (Yu et al., 2014; Mishra et al., 2017). In general, the external surface charge of microbes exposed to the solution is dominated by negatively charged groups, with reported electrophoretic mobilities for bacteria suspensions generally negative at pH higher than 3 (Ha et al., 2010). Moreover, to create and maintain biofilms, microbial cells secrete an exopolymeric matrix (EPS), which in turns provides additional functional sites, mostly negatively charged (Tourney and Ngwenya, 2014), and confers specific density and permeability properties to the whole structure. Thus, biofilms and their EPS matrix as well as the substrate where they are attached to, can act as competing sorbents in all natural systems and are considered a major driver for various environmental processes such as metal(loid)s cycling (Ha et al., 2010; Wang et al., 2016a, b). As such, biofilms play a relevant role in metals and metalloids cycling, and are therefore of first importance from an environmental perspective.
Microenvironments Within Biofilm Thickness
Biofilms structure is intrinsically highly complex, and has been thoroughly investigated, especially in the context of biomedical applications (Costerton et al., 1999; Donlan, 2002). Their organization is highly variable, depending on the strain, growth condition, or even the substrate type on which these colonies develop (Sutherland, 2001). Microbial biofilm matrices are described as gel-like structures surrounding a high density of cells, where the diffusion of chemical elements is limited by the low porosity and permeability of the system (Warren and Haack, 2001). Consequently, because of their metabolic activities, microorganisms within these structures create and maintain micro-environments that are intrinsically different from the surrounding bulk solution (Figure 1). As a result, they impose concentration gradients for organic or inorganic compounds such as phosphate (Couasnon et al., 2019), enzymes or EPS (Flemming and Wingender, 2010), as well as physico-chemical gradients (pH, Eh, pO2…) (Hunter and Beveridge, 2005; Hidalgo et al., 2009). For instance, Pseudomonas fluorescens WCS 365 biofilms were reported to exhibit low pH regions (down to pH 5.1) in cells clusters located at the inner-core of microcolonies, which gradually evolved to neutral pH in vicinity of the bulk solution at the biofilm surface (Fulaz et al., 2019a). These pH variations within relatively short spatial scales could be explained by the fast production of metabolic residues combined with a limited solute transfer through the EPS matrix, resulting in a local accumulation of acidic by-products (Fulaz et al., 2019a). Multi-species biofilms, presenting an array of different metabolisms, could generate more acidic by-products under oxygen-limiting conditions compared to single-species biofilms, resulting in lower minimal pH values, reported between 4 and 5 for multi-species oral biofilms (Schlafer et al., 2018). In addition, extracellular medium within biofilms can be considered as an external digestive system, where extracellular enzymes are in close vicinity with cells, and can thus metabolize dissolved, colloidal and solid biopolymers (Flemming and Wingender, 2010). These physico-chemical modifications at the local scale in biofilms’ micro-environments constitute a key parameter regarding their reactivity, and they are known to impact the metal(loid)s cycling in environmental systems. For instance, numerous evidences point out the major control microbial communities exert on mineral and rock weathering. As such, a 20-fold increase in dissolution rates is reported for soil bacteria (Kalinowski et al., 2000), and up to 2 orders of magnitude for groundwater bacteria (Barker and Banfield, 1998). Local acidification as well as siderophore, organic acids, and metabolites produced in vicinity of microorganisms are usually invoked to explain this increase in weathering rates when microbial communities are present (Dehner et al., 2010; Gadd, 2010).
However, local physico-chemical conditions and the nature of gradients imposed, as well as metal(loid)s dynamic in biofilms, remain poorly understood and certainly need further investigation. It can be expected that the improvement of existing techniques to accurately probe specific patterns in biofilm microenvironments, such as confocal laser scanning microscopy (CLSM), will allow a better characterization and more precise description of the mechanisms of metal(loid)s dynamic in biofilms. Understanding these processes is particularly relevant in the case of ENPs that are potentially very sensitive to physico-chemical gradients. For example, these microbial structures can drive ENPs dissolution under low pH or high complexing ligands concentrations (ligand-assisted dissolution) but can also stabilize ENPs surfaces. Conversely, biofilms are also able to impose local oversaturations relative to mineral phases (Finlay et al., 1999; Templeton et al., 2003b; Nancharaiah et al., 2010; Couasnon et al., 2019), that were kept undersaturated in the overlying bulk solution, resulting in the formation of incidental NPs. As a result, biofilms may constitute an important accumulator for ENPs or a source of incidental NPs that can strongly impact their fate through a number of bio-physico-chemical processes that will be discussed in this review.
Biofilms: Important Environmental Accumulators
As stated previously, biofilms have a 3-dimensional organization which is close to a gel-like structure, and exhibit high reactive site densities of cells and EPS. As a result, biofilms are frequently regarded as filters or even sinks for a variety of inorganic, organic and biological components (Ikuma et al., 2015). Actually, their sequestration capacity has been extensively studied for metal(loid)s by Templeton et al. (2003b) and Wang et al. (2016a; 2016b), that identified biofilms as accumulative compartments in the environment. Similarly, a large variety of ENPs are trapped in significant amounts by biofilms (Battin et al., 2009; Burns et al., 2013; Avellan et al., 2018). It was also observed in column transport experiments where retention of ENPs increases in presence of these microbial structures: latex NPs and CdSe/ZnS quantum dots (QDs) (Tripathi et al., 2012), zerovalent Fe-NPs (Lerner et al., 2012; Crampon et al., 2018), nano-ZnO (Jiang et al., 2013), biogenic nano-Se (Wang et al., 2019), nano-Ag (Xiao and Wiesner, 2013). This increased retention in column experiments is generally explained by changes in roughness, surface charge and hydrophobicity at the surface of aquifer grains (Donlan, 2002; Kurlanda-Witek et al., 2015) in addition to the intrinsic accumulation properties of these microbial structures.
In addition, a mesocosm-based study showed that microbial biofilms constituted the biggest NPs accumulation reservoir on a per mass basis for gold nanorods (Ferry et al., 2009). However, the reported degree of accumulation is variable, depending on the biofilm and ENPs types as well as parameters such as ENPs concentration, pH or presence of organic ligands. Nano-CeO2 accumulation in P. fluorescens biofilms and Mycobacterium smegmatis biofilms were estimated at approximately 20 and 50% respectively, for initial concentration ranging between 5 and 30 mg.L–1 (Jing et al., 2014). Multi-species biofilms seem to be more efficient nano-Ag accumulators compared to single-species biofilms, suggesting that higher structural heterogeneity and density in multi-species biofilm are important parameters for ENPs trapping (Walden and Zhang, 2018). Shewanella oneidensis MR1 biofilms exposed to 31.22 μg nano-Ag exhibit an accumulation extent of 1.7% at pH 7 (Desmau et al., 2018), while Pseudomonas putida exposed to 22.8 μg nano-Ag has an accumulation level of 0.4% at pH 7.5, and 5.3% at pH 6.0 (Fabrega et al., 2009). Interestingly, accumulation in P. putida biofilms becomes largely predominant when going to low ENPs concentrations, relevant to most expected concentrations in environmental systems (Gottschalk et al., 2009; Sun et al., 2016), reaching levels as high as 93% for an exposure to 20 μg.L–1 (total 2.28 μg) nano-Ag (Fabrega et al., 2009).
Regarding ENPs accumulation kinetics, a pseudo-first order was used to model adsorption and desorption rate of nano-CeO2 onto bacteria biofilms (Jing et al., 2014). Usually, interactions between ENPs and biofilms are reported as being fast, occurring in less than 30 min (Jing et al., 2014; Walden and Zhang, 2018). However, in some studies, the evolution at longer times varies among different experimental systems, either quickly reaching an apparent steady-state (Jing et al., 2014), or experiencing a continuous accumulation and ENPs migration at the mineral/biofilm/solution interface over 24 h (Desmau et al., 2018). A decrease in accumulation rate was also reported for nano-Fe3O4, with a maximum accumulation of 17% reached after 5 h of exposure, dropping to 0.5% after 24 h, potentially due to cells detachment (Herrling et al., 2016). Nevertheless, accumulation trends over longer time periods, on the order of months, remain mostly unexplored despite their high environmental importance.
The significant accumulation of ENPs in biofilms raises the question of considering biofilms as a potential secondary source for ENPs in other environmental compartments (Walden and Zhang, 2018). In soils, if biofilms are temporarily exposed to high ENPs concentrations on short time periods, they are likely to accumulate these objects to a relatively high extent. Then, biofilms could gradually release ENPs, or ENPs products of degradation, in ENPs-free pore waters. This process, potentially important when considering ENPs spreading in the environment on the longer term, specifically regarding their mobility and their associated toxicity to ecosystems, has remained largely overlooked up to now.
ENPs Retention and Migration Mechanisms Within Biofilms
Retention properties imposed by biofilms are critical when evaluating the environmental fate of ENPs. As a result, several reviews specifically report the types of interactions existing between ENPs and these microbial structures (Ikuma et al., 2015; Joo and Aggarwal, 2018; Walden and Zhang, 2018). Ikuma et al. (2015) provide an extensive description of such interactions following a three-step process: (1) ENPs transport to the vicinity of the biofilm, (2) ENPs attachment to the biofilm surface, (3) migration within the biofilm thickness. For the authors, if ENPs transport to the biofilm and its initial attachment are relatively well-documented, they point out the lack of comprehensive understanding regarding the processes that govern ENPs migration within biofilms. However, this last factor is absolutely essential since it determines to a large extent the retention properties imposed by biofilms, and is thus partly responsible for ENPs behavior in natural systems. As a result, the identified key parameters that control the migration and retention of ENPs within biofilm structures are reported here (Figure 2).
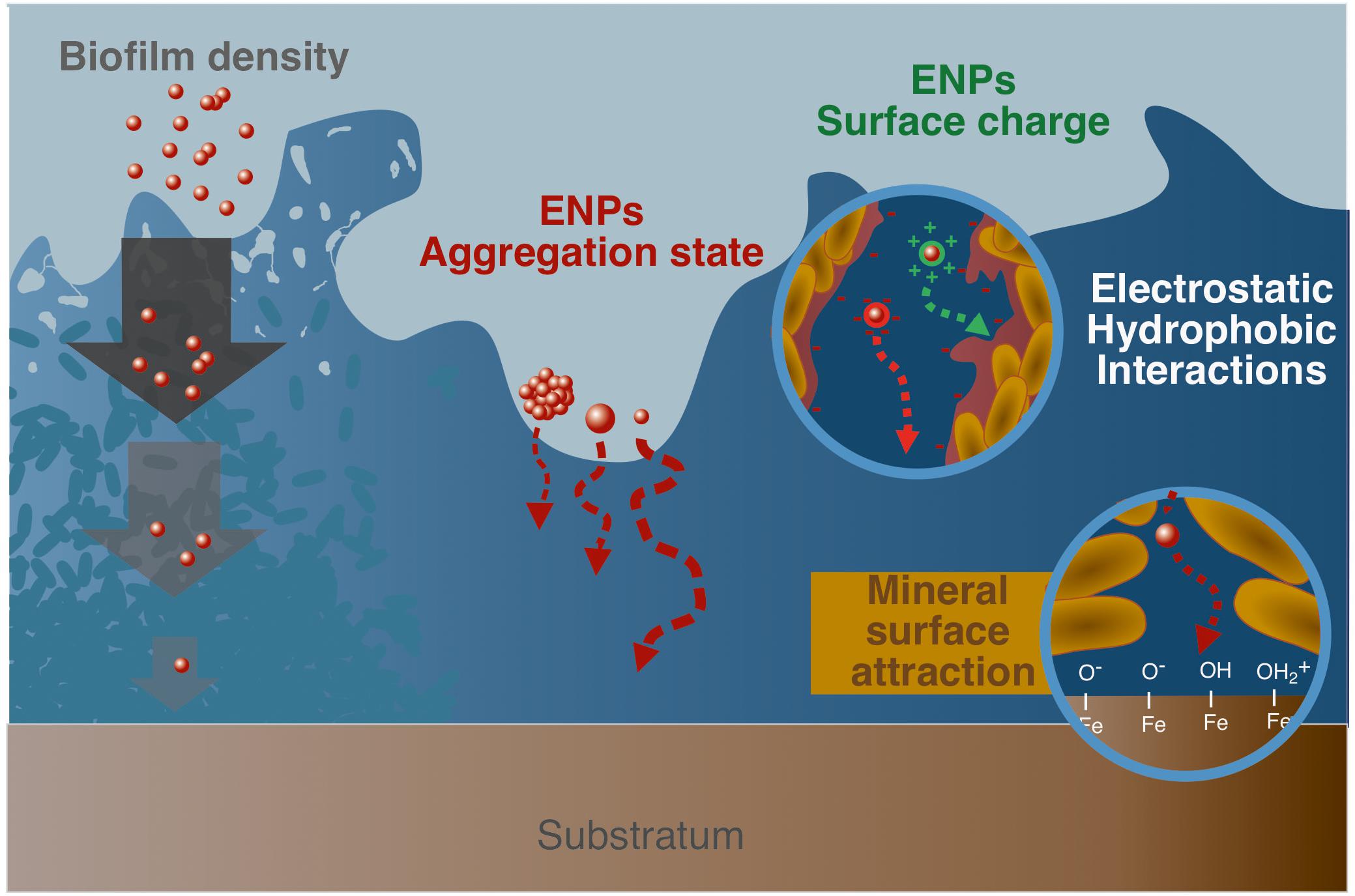
Figure 2. Parameters controlling ENPs migration within biofilms. From left to right, biofilm density and permeability that can limit access to the deepest layers; aggregation state and size of ENPs; ENPs surface charge that controls associations with the biofilm matrix (EPS and cells) through electrostatic and hydrophobic interactions, with for instance positively charged ENPs that tend to interact strongly with the negatively charged biofilm matrix; complexation of ENPs with the mineral substrate surface that partly drives ENPs transport through the biofilm thickness.
Biofilm Structural Properties and Effect on Size-Dependent ENPs Transport
Porosity and permeability of biofilms constitute major parameters for ENPs migration since they govern any solute transport within the structure. As such, ENPs diffusion coefficients are calculated to be much smaller (up to 50 times) than the values in aqueous solutions for a variety of model NPs (Au-, Ti-, latex-, and silicone-based NPs) and biofilms (P. fluorescens, Lactococcus lactis, Stenotrophonas maltophilia) (Guiot et al., 2002; Golmohamadi et al., 2013). Porosity and permeability are linked to the type, composition, thickness, age and roughness of the biofilm (Joo and Aggarwal, 2018). In more details, the presence of water channels, submitted to advective transport and simple diffusion, as well as smaller pores and conduits (Costerton et al., 1995) constitute the template that could allow the migration of ENPs at the mineral/biofilm/solution interface. In that case, the overall density variations in the biofilm, channel sections, but also ENPs size constitute critical factors, associated to size exclusion and diffusion limited processes (Golmohamadi et al., 2013).
For instance, when using silica NPs sensors as stains to generate high-resolution maps of Escherichia coli biofilms, only the smallest 10 nm diameter particles allowed to access fine details in biofilm structure, while 70 and 30 nm only displayed a limited access to this 3-dimensional organization (Hidalgo et al., 2009). Also, relative self-diffusion coefficients of 2 and 10 nm nano-Ag was shown to decrease exponentially with the square of the radius of the ENPs (Peulen and Wilkinson, 2011). This size effect as a control of diffusion was critical for nano-Ag interacting with biofilm, when investigating their migration through a S. oneidensis MR1 biofilm (Desmau et al., 2018). Actually, it is recognized that ENPs penetration is more efficient in less dense biofilms, or biofilm parts, since they exhibit higher pore space more readily accessible (Peulen and Wilkinson, 2011; Joo and Aggarwal, 2018). Similarly, more mature biofilms, usually displaying elevated structural densities, are expected to lower ENPs penetration (Mitzel and Tufenkji, 2014). However, there are evidence that ENPs tend to accumulate preferentially in dense rather than loosely attached biofilms (Peulen and Wilkinson, 2011), with higher structural heterogeneity and density being critical parameters for ENPs accumulation (Walden and Zhang, 2018). This may be explained by the fact that denser biofilms exhibit a more developed extracellular matrix (EPS) that fills the spaces between cells, composed of polysaccharides, proteins and other molecules, either hydrophilic or hydrophobic. As a result, dense biofilms are likely to display higher reactive site densities, enabling more efficient interactions with ENPs through electrostatic or chemical bonding in addition to steric immobilization, leading to strong ENPs trapping. On the contrary, if ENPs transport is favored in loose biofilms, a significant part of trapped ENPs is also submitted to advective transport out of the biofilm, thus disabling long term sequestration.
Electrostatic and Hydrophobic Interactions
Effect of Surface Properties of ENPs on ENPs-Biofilm Interactions
Interactions between ENPs and biofilms are usually described as governed by electrostatic and hydrophobic factors (Fulaz et al., 2019b). In this regard, a critical parameter to understand ENPs-biofilm interactions is surface properties of ENPs, such as hydrophobicity or type of coatings, and more specifically surface charge. The importance of the ENPs surface properties was demonstrated for different types of ENPs, (un)coated or with various capping agents. The uncoated ENPs showed a much higher retention compared to the coated ones (Li et al., 2013). The significantly higher attachment to Pseudomonas aeruginosa biofilms, for sulfate-functionalized model NPs compared to their carboxylated counterparts, illustrates how surface chemistry and NPs functionalization is an important parameter (Tripathi et al., 2012).
As noted earlier (cf. section “Occurrence, Structure, and Overall Reactivity”), microbial biofilms exhibit an overall negative charge (Ha et al., 2010; Tourney and Ngwenya, 2014). Based on electrostatic considerations, interactions with positively charged ENPs are thus expected to be favored, while ENPs of negative charge should only poorly interact with the biofilm. This general trend is globally observed in many ENPs-biofilms systems (Peulen and Wilkinson, 2011; Lerner et al., 2012; Ikuma et al., 2014, 2015; Dzumedzey et al., 2017; Crampon et al., 2018; Wang et al., 2019). Interestingly, electrostatic repulsion energy is also size dependent and is reported to be roughly proportional to the particle’s surface area. For instance, 70 nm particles are submitted to approximately a 50-fold increase in repulsion compared to 10 nm particles (Hidalgo et al., 2009). Functionalized QDs (Cdse/ZnS) of cationic charge were shown to fully penetrate into E. coli biofilms, while neutral and anionic QDs did not efficiently accumulate in the structure (Li et al., 2015). Similarly, a low retention of negatively charged nano-Ag [polyvinylpyrrolidone (PVP) coating] onto P. aeruginosa PAO1 biofilms was explained as the result of repulsive electrostatic forces (Mitzel and Tufenkji, 2014). Noticeably, although electrostatic interactions constituted a critical parameter in numerous studies, they could reveal to be inadequate to describe ENPs-biofilm interactions in some systems (Peulen and Wilkinson, 2011; Golmohamadi et al., 2013). Indeed, this electrostatic attraction or repulsion rule is not always accurate, and negatively charged ENPs have been shown to interact with biofilms despite their general negative charge (Tong et al., 2010; Tripathi et al., 2012; Desmau et al., 2018). This could be related to local positive charges or domains, e.g., the presence of positive functional moieties such as amino groups (Ha et al., 2010), or due to other attraction forces (attraction from the mineral surface, chemical binding and stabilization, hydrophobicity, advective transport).
In addition, ENPs hydrophobicity constitutes also an important parameter for their sequestration and subsequent migration within the biofilm thickness (Habimana et al., 2011; Xiao and Wiesner, 2013; Mitzel et al., 2016). This property is likely to be related to the existence of hydrophobic domains in biofilms (Aldeek et al., 2011; Jian-Zhou et al., 2015; Flemming et al., 2016), such as protein-rich zones for some microbial consortia (Xiao and Wiesner, 2013). Also, an increase in ENPs coating hydrophobicity could favor retention (Lerner et al., 2012). Li et al. (2015). reported distinct localization for hydrophobic and hydrophilic QDs throughout E. coli biofilms, with hydrophobic particles being more homogeneously distributed than hydrophilic ones. This example illustrates that dynamics of ENPs partitioning within different domains of biofilms can be, at least partly, impacted by hydrophobic interactions.
Effect of Biofilm and Corona-Formation on ENPs Aggregation
Aggregation state, which is related to ENPs surface properties, is a critical factor affecting ENPs’ reactivity, such as their penetration capacities in biofilms (Wirth et al., 2012). Indeed, nano-Ag were found to aggregate when exposed to E. coli, with an increase in average particle size by a factor of 40 in biofilms, and only by a factor of 15 for planktonic cells (Choi et al., 2010). Given the size of the aggregates, it is expected that aggregated ENPs will experience lower penetration and interaction with the biofilm (Peulen and Wilkinson, 2011). Within biofilm thickness, the presence of microenvironments is likely to modify the overall ENPs reactivity, and thus, their aggregation (Figure 3). For instance, nano-Ag suspensions are efficiently stabilized by EPS at low Ca(NO3)2 concentrations while at higher Ca(NO3)2 concentrations, the formation of EPS intermolecular bridging favors the connection of EPS-caped nano-Ag together (Fernando et al., 2020). The corona formation process, which consists in the association of organic (bio)molecules, natural OM or EPS to the ENPs surface (Navarro et al., 2009; Ikuma et al., 2015; Zhu et al., 2016; Ouyang et al., 2017), plays an important role in controlling ENPs aggregation by modifying the colloidal stability of the nanoparticles, and in turn their interactions properties with biofilms (Fabrega et al., 2009). This corona formation is likely to happen within the biofilm itself, given the existence of microenvironments along the biofilm thickness (cf. section “Occurrence, Structure, and Overall Reactivity”), that may locally expose ENPs to high concentrations of organic molecules (EPS, biopolymers, metabolic residues). The formation of an EPS coating layer surrounding the ENPs (Khan et al., 2011b), is reported to increase the ENPs hydrodynamic diameter (Kroll et al., 2014) but also to decrease the ENPs rate of aggregation (Khan et al., 2011a; Adeleye et al., 2014; Kroll et al., 2014; Miao et al., 2015; Wang and Nowack, 2018), In addition, the electrostatic repulsion between ENPs can be favored when the surface charge is becoming more negative in presence of EPS (Khan et al., 2011a; Wang and Nowack, 2018). Steric repulsions and hydrophobic interactions also need to be considered regarding the aggregation rate of ENPs in presence of EPS (Lin et al., 2016; Wei et al., 2019). One of the main consequences of the ENPs stabilization in presence of EPS would be the favored transport of ENPs in aquatic environments (Wang and Nowack, 2018).
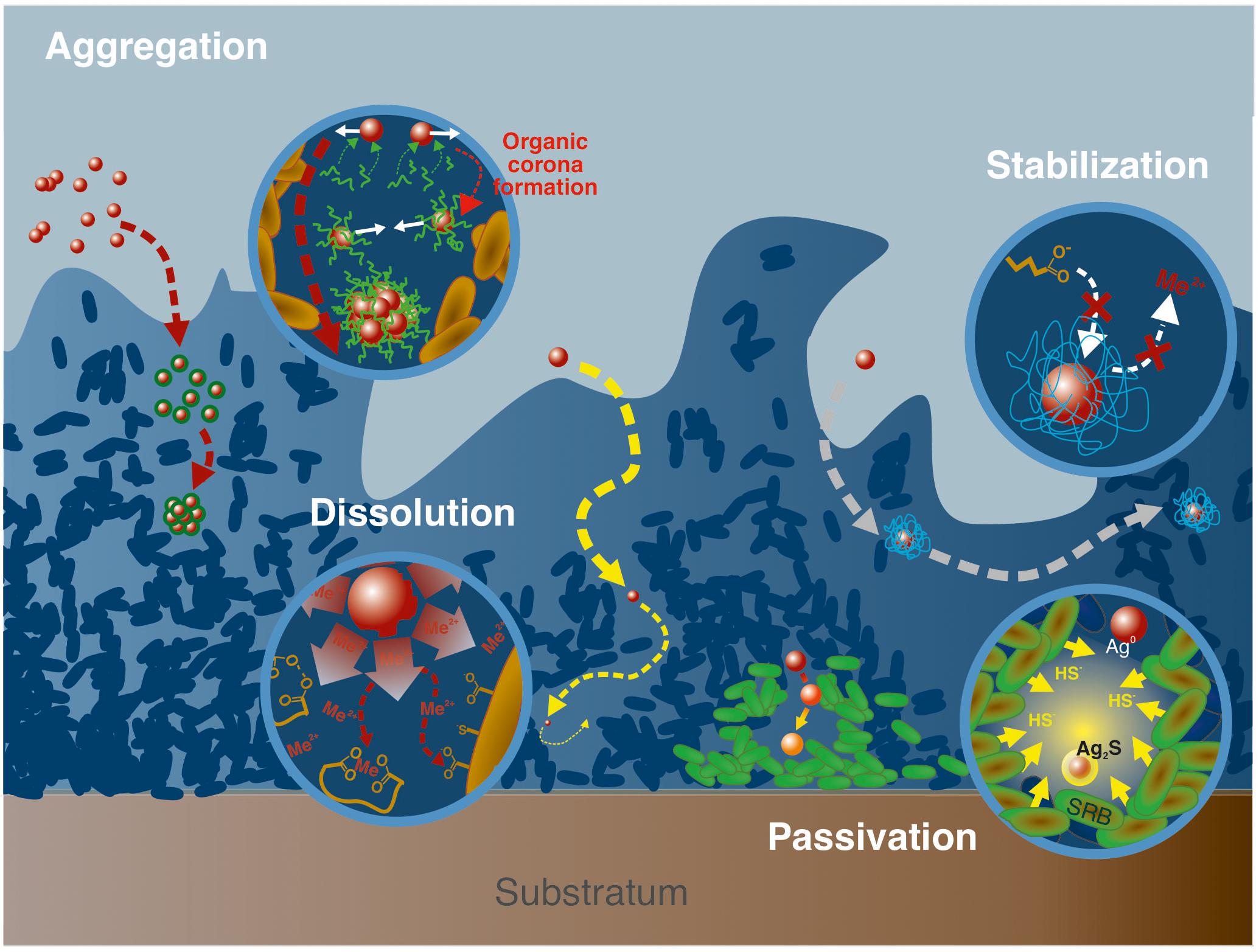
Figure 3. Examples of processes associated to ENPs transformations and aggregation. From left to right, aggregation mediated by the formation, in the biofilm matrix, of an organic corona, that modifies ENPs surface properties and promotes interactions between ENPs; ligand-mediated dissolution involving the presence of highly complexing ligands (such as siderophores) that complex free metals in solution and thus promotes ENPs dissolution; passivation of silver NPs in vicinity of sulfato-reducing bacteria (SRB) that increase locally HS- concentrations, and favors sulfidation processes at the ENPs surface; ENPs stabilization through the formation of an organic corona that isolates ENPs from the surrounding solution thus limiting dissolution processes.
The presence of functional groups in EPS, their composition (protein, carboxylate, polysaccharide, and lipid contents) and concentration will also strongly condition the interactions with ENPs (Adeleye and Keller, 2016; Xu et al., 2016; Zhou et al., 2016; Morelli et al., 2018; Wang and Nowack, 2018; Fernando et al., 2020). As such, investigations were conducted on the stabilization potential of three EPS types, soluble, loosely bound, and tightly bound EPS, characterized by different protein to polysaccharide ratio (Fernando et al., 2020). All three EPS-types were found to prevent nano-Ag aggregation, but loosely bound EPS were the most efficient, due to its lower content in hydrophilic dissolved OM. In addition, EPS hydrophobic properties can be important when considering sorption at ENPs surfaces (Wei et al., 2019).
Noticeably, EPS composition is strongly determined by the type of microorganisms considered (Sutherland, 2001; Flemming and Wingender, 2010), and conditions in which they develop. As a result, dynamic changes in ENPs reactivity when interacting with biofilms can be expected for some specific conditions, but such mechanism remains today largely overlooked.
Mineral Substrate Reactivity
Mineral surfaces are known to exhibit strong complexing capacities toward metal(loid)s and organic matter in most ecosystems (Brown et al., 1999). In soils, they can serve as substrates on which biofilms develop, but doing so, they maintain their high complexing capacities despite the overlying microbial community, as reported for different mineral/biofilm/solution interfaces (Templeton et al., 2001, 2003a,b; Wang et al., 2016a, b). In these studies, focused on the partitioning of metal(loid)s at the mineral/biofilm/solution interface, mineral substrates constitute a strong complexing compartment able to drive the dynamics of free metal transport within the whole interface, biofilm included. Regarding ENPs, a study by Desmau et al. (2018) on S. oneidensis MR1 biofilms exposed to nano-Ag shows that the mineral surface remains highly reactive and tends to accumulate negatively charged ENPs (PVP coated nano-Ag). As a result, the ENPs dynamics of accumulation and further transport at the mineral/biofilm/solution interface are strongly driven by the mineral surface. However, in most studies the reactivity of the mineral substrate was poorly or not considered and future research will need to elucidate its role.
ENPs Chemical Transformations in Biofilms
As previously discussed (cf. section “ENPs Retention and Migration Mechanisms Within Biofilms”), ENPs fate in natural systems is strongly controlled by retention mechanisms imposed by microbial biofilms. To this perspective, another critical factor that needs to be considered is the transformation that ENPs can undergo when in contact with these biological structures (Holden et al., 2016). At a first approximation, ENPs potential for speciation changes could be considered similar to that of free metals that are subjected to strong chemical modifications in biofilms (Templeton et al., 2003a; Couasnon et al., 2019). It is thus likely that ENPs experience similar transformations, driven by the high reactivity of local microenvironments and the important functional sites density spread within the biofilm thickness.
Dissolution and stabilization by surface passivation are reported here as the main processes able to drastically modify ENPs speciation (Figure 3). In addition, response to toxic stress from cells caused by ENPs exposure is discussed since the related processes are potentially impacting their environmental life cycle.
Dissolution
Toxicity of various ENPs comes from their dissolution and the subsequent exposure of organisms to free metals. This toxic pathway is usually evoked for known soluble ENPs such as nano-Ag or nano-ZnO (Franklin et al., 2007; Xia et al., 2008; Auffan et al., 2009; Gelabert et al., 2015; Le Ouay and Stellacci, 2015). In that case, ENPs in close vicinity of bacteria cell walls become a continuous source of novel metal species through dissolution processes. This can result in high concentrations of toxic ions and cause toxicity to the bacteria (Slavin et al., 2017). The extent of dissolution and the associated kinetics generally depend on the size, shape, surface characteristics of the ENPs, as well as the solvent properties (pH, ionic strength, presence of ligands), and is well-documented even in complex matrices (Gelabert et al., 2014; Sivry et al., 2014). In natural systems, dissolution kinetics can be largely enhanced in contact with highly reactive environmental compartments, such as biofilms. Even NPs known as stable could be submitted to dissolution. As such, some studies report a significant ENPs dissolution when in contact with microbial biofilms (Wirth et al., 2012; Gil-Allué et al., 2015; Avellan et al., 2018; Wang and Nowack, 2018; Alizadeh et al., 2019; McGivney et al., 2019).
Even if the associated processes remain poorly documented, two main factors are likely to be responsible for ENPs dissolution in microbial biofilms: (i) the high density of reactive ligands in these bio-structures favoring ligand assisted dissolution, and (ii) the strong redox activities of microorganisms. These dissolution processes are certainly favored in biofilm microenvironments that exhibit specific physico-chemical properties at the local scale.
Ligand-Mediated Dissolution
In microbial biofilms, the high reactive site densities result from the association of numerous functional sites present on the cell walls of microorganisms (Ngwenya et al., 2003; Borrok et al., 2004a, b, 2005), and on the EPS matrix (Ha et al., 2010; Tourney and Ngwenya, 2014). Regarding microbial surfaces, the compilation of several studies on Gram-positive and Gram-negative bacteria in planktonic suspension reported functional sites densities in the range of 4.5 ± 0.8.10–3 mol.g–1 (dry weight) (Yee and Fein, 2001). On the other hand, EPS reactivity is a function of the types of molecules included in their compositions, such as their polysaccharides/proteins ratio. Regarding ENPs forced dissolution, the high sites density in biofilms, cell walls and EPS included, tends to complex metal(loid)s to high extent (Tourney and Ngwenya, 2014; Wang et al., 2016a, b), resulting in a decrease in local concentrations of free metal(loid)s in solution within the biofilm matrix. In order to restore thermodynamics equilibria, ENPs will in turn dissolve and release free metal(loid)s (Bian et al., 2011; Wirth et al., 2012). If the density of complexing sites is in high excess, ENPs total dissolution could thus be expected. This process can be facilitated by formation of strong metal-organic complexes at the ENPs surface that weakens metal-oxygen bonds.
Focusing specifically on EPS reactivity in absence of biofilm, Adeleye et al. (2014) noted a more important dissolution of nano-CuO in presence of EPS after 90 days compared to EPS-free control. By extension, the EPS role is likely to be critical when investigating biofilm impact reactivity. As a result, EPS are considered by many authors a reasonably good analog to evaluate the biofilm impact onto ENPs fate (Tong et al., 2010; Jiang et al., 2013). However, it must be noted that since cells metabolic activity constitutes also an important part of the biofilms’ reactivity, which in association with their specific 3-dimensional structure that creates microenvironments, is likely to strongly impact the ENPs fate in the environment.
The extracellular matrix is known to contain enzymes and other proteins secreted by living cells in the biofilm, leading to the notion of “external digestion system” that partly controls organic molecules and polymers degradation, as well as certain extracellular redox process (Flemming and Wingender, 2010, Flemming et al., 2016). As a result, degradation of organic polymers that coat (and protect in a certain way) functionalized ENPs may arise from exposure to such enzymes (Ikuma et al., 2015). Interestingly, the type of capping agents constitutes an important factor regarding dissolution rate (Mitrano et al., 2014). Siderophores, metal-complexing molecules of high affinity, are also produced by bacteria in biofilms (Visca et al., 2007; Saha et al., 2013). Siderophore production by bacteria, including the ligands catecholate, hydroxamate, and carboxylate, is extensively reviewed (Springer and Butler, 2016; Albelda-Berenguer et al., 2019), and present the central role of this class of molecules for metal scavenging. Other highly complexing ligands are also secreted by bacteria, such as cysteine-containing molecules and glutathione that exhibit thiol groups of high affinity toward soft metals (Brown et al., 2006; Mugerfeld et al., 2009). Oxalic acid (Palmieri et al., 2019) produced by P. fluorescens (Hamel et al., 1999) or Burkholderia glumae (Nakata and He, 2010) were also reported. Presence of complexing ligands such as siderophores is frequently invoked when considering mineral dissolution mediated by bacteria, and can be extended to ENPs, with ligand-promoted dissolution kinetics being higher than proton-promoted dissolution above pH 4 (Kraemer, 2004; Reichard et al., 2007; Wang et al., 2015).
The biofilm structure is known to induce microenvironments formation (cf. section “Microenvironments Within Biofilm Thickness”), that are able to strongly impact local thermodynamics equilibria and favor both ligand-mediated and redox-mediated dissolution. Existence of gradients in pH or redox state are both reported, and take place at very short spatial scales within the biofilm structure (Yu and Bishop, 2001; Billings et al., 2015; Flemming et al., 2016; Kataky and Knowles, 2018). However, despite their importance, the nature and properties of such microenvironments are still poorly known regarding ligands or metal(loid)s concentrations, even if few studies suggest strong and dynamic changes in local physico-chemical conditions (Couasnon et al., 2019). Nonetheless, it can be anticipated that these “hot spots” of high reactivity exhibit low pHs, important redox changes, and locally concentrate to high extent siderophores and other complexing ligands as well as enzymes. Since metal-based ENPs dissolution is usually favored at low pH (Bian et al., 2011; Han et al., 2016) and in the presence of high affinity complexing molecules (cf. section “Ligand-Mediated Dissolution”), it is likely that microenvironments strongly impact ENPs dissolution.
Redox-Mediated Dissolution
As a second factor, many microorganisms are known to exhibit strong redox activities, thoroughly reported in numerous studies, as for Acidothiobacillus ferrooxidans which is able to oxidize sulfur species by direct contact or indirect mechanisms (Monachon et al., 2019). Generally, microorganisms in biofilms secrete a high variety of redox-active molecules associated to the EPS matrix (Tourney and Ngwenya, 2014), and some studies propose that some types of EPS could be electron shuttles in biofilms. Production of riboflavin or c-type cytochrome (Xiao et al., 2017; Kataky and Knowles, 2018), and cyanide residues (Avellan et al., 2018) have been reported. Also, the production of extracellular superoxide, a reactive oxygen species, has been reported for an important variety of microorganisms such as Firmicutes, Actinobacteria, Bacteroidetes, Gammaproteobacteria, and Alphaproteobacteria (Diaz et al., 2013). As a result, the collection of redox-active molecules in the extracellular matrix is also likely to play an important role for dissolution of redox-sensitive ENPs, such as nano-CeO2, nano-Ag or nano-Au, in microbial biofilms.
For instance, Nano-Au are thought to remain inert in abiotic conditions. However, presence of soil bacterium Chromobacterium violaceum, that produces cyanide residues, is able to provoke oxidative dissolution of nano-Au (McGivney et al., 2019). Similarly, nano-Au was shown to dissolve in contact with freshwater macrophyte biofilm through oxidation processes involving cyanide release (Avellan et al., 2018). Nano-CeO2 exposed to wastewater biofilm experience higher dissolution rates, following Ce(IV) reduction (Wang and Nowack, 2018), with associated mechanism involving either direct contact between the NPs and bacteria surface or liberation of electron shuttle molecules in the extracellular medium (Thill et al., 2006). It is also proposed that light-induced production of reactive oxygen species (ROS) at the EPS surface can also play a role for ENPs dissolution through oxidation of Ce(III) to Ce(IV) in solution, lowering the concentration of dissolved Ce(III) (Kroll et al., 2014).
ENPs Surface Passivation
Biofilms can favor ENPs dissolution as discussed above but can also induce surface passivation through either (i) chemical transformation of the surface to a more thermodynamically stable phase, or (ii) formation of a corona shell that will limit surface interactions with the surrounding environment.
Passivation of ENPs surface through phase transformation, lowering their reactivity and solubility, is observed when in contact with biofilm and microorganisms. For instance, in anaerobic conditions, typically at the bottom of biofilms, sulfate-reducing bacteria can reduce sulfate to bisulfide (HS–) which is in turn able to promote nano-Ag sulfidation at the surface of the NPs (Levard et al., 2011, 2013). Such sulfidation is reported in water resource recovery facilities biofilms (Alizadeh et al., 2019), with the creation of such an Ag2S passivation layer at the surface of nano-Ag that reduces the NP solubility, and consequently its toxicity (Levard et al., 2013).
Organic polymers originally present as coatings on ENPs can also be destabilized in the biofilm thickness by ligand exchange processes involving EPS molecules, with strong consequences on ENPs stability. Although the adsorption of EPS to the surface of ENPs can favor their dissolution or their aggregation, as presented previously, they may also promote their passivation. This depends on the type of EPS and complexing ligands considered, their charge and hydrophobic properties, as well as the mode of interaction with ENPs. Strong metal-organic complexes at the ENPs surface are expected to lower the strength of surrounding metal-oxygen bonds thus favoring dissolution, while weaker EPS interactions with ENPs can drastically modify ENPs surface properties, and potentially promote aggregation depending on local physico-chemical conditions (through intermolecular bridging for instance). Finally, ENPs enhanced passivation takes place when the organic corona formed by EPS either isolates ENPs from the surrounding solution, thus disabling their dissolution, or promotes repulsion among ENPs, lowering their aggregation.
Cellular Toxicity Response as a Strong Modifier of ENPs Speciation
ENPs toxicity to biofilm cells is clearly related to the penetration of these particles and the effectiveness of interaction with microbial cells. As a result, several studies linked ENPs toxicity to biofilm physico-chemical properties such as thickness or roughness (Thuptimdang et al., 2017). Actually, bacteria encased in biofilms structures are usually reported as more resistant to toxic substances than the corresponding planktonic analogs, and a significant amount of studies and reviews exist that discuss this topic (Wirth et al., 2012; Flemming et al., 2016). However, what is more important regarding the ENPs environmental fate is the microbial cells response after exposure to NPs, since some of them are known toxic agents.
Multi-species communities exposed to ENPs may experience important changes in microbial consortium structure with significant evolutions in microbial diversity (Binh et al., 2014, 2016; Echavarri-Bravo et al., 2015; Jomini et al., 2015; Tang et al., 2017; Miao et al., 2018; Zhu et al., 2018a, b, 2019). These remodeling of microbial consortia can potentially modify the types of metabolisms expressed in these communities and impact the environmental ENPs fate. Noticeably, mechanisms of cell toxicity resistance to ENPs will differ from one microorganism to the other, as is the case of E. coli and Pseudomonas aeruginosa exposed to nano-Ag (Saleh et al., 2015), or even among two different strains of the same bacteria, such as E. coli MG1655 and W3110 exposed to ZnO-NPs (Gelabert et al., 2015). This suggests the existence of numerous metabolic pathways, potentially generating the extracellular release of a variety of substances.
Nevertheless, whereas NPs impact on biofilms viability and toxicity have been extensively studied, only a few articles focus on ENPs effects on microbial biofilms functioning. Exposure to photocatalytic ENPs (CdS, TiO2, Fe2O3) result in different adaptations in peryphitic biofilms (Zhu et al., 2019). While no decrease in biomass, chlorophyll content and ATPase activity were detected, a significant increase in EPS production and secretion of superoxide dismutase have been observed. The microbial community is also evolving due to an increase in the proportion of phototrophic and high nutrient metabolic microorganisms. Such an evolution in microbial community composition was also shown for periphytic biofilms exposed to nano-TiO2, with an increase in the abundance of denitrifying bacteria (Zhu et al., 2018a) and a significant production of EPS enriched in proteins. Exposure to nano-TiO2 and nano-CeO2 also lead to changes in bacteria consortium for surficial sediment microbial communities, with an observed inhibition of microbial-mediated oxygen consumption, and strong changes in local redox state (Miao et al., 2018). Nano-CdS have been shown to induce an increase in overall metabolic activity (ATPase activity), and to stimulate EPS production (Zhu et al., 2018b), while exposure to nano-CuO resulted in the production of polysaccharides-rich loosely bound-EPS (Hou et al., 2015).
Interestingly, the production or inhibition of key molecules that could modify ENPs speciation were also reported. S. oneidensis MR-1 biofilms exposed to nano-TiO2 showed an increased production of riboflavin (Maurer-Jones et al., 2013). This molecule is related to metal reduction capacity in bacteria, accounting for approximately 75% of extracellular electron transfer to insoluble substrates by S. oneidensis (Kotloski and Gralnick, 2013). Similarly, a riboflavin production was reported for planktonic S. oneidensis exposed to lithium nickel manganese cobalt oxide nanosheets (Mitchell et al., 2019), whereas nano-Fe oxide did not induce any increase in riboflavin expression (Buchman et al., 2019). The production of pyoverdine, an important siderophore exhibiting a high affinity for Fe, was shown to be inhibited following exposure to nano-CuO on Pseudomonas chlororaphis 06 (Dimkpa et al., 2012), or to Te nanorods for P. aeruginosa (Mohanty et al., 2014, 2015). In bacterial communities, the activity of key extracellular enzymes such as alkaline phosphatase and ß-glucosidase was also restrained by the combined exposure to nano-TiO2 and Cu (Fan et al., 2016) or polystyrene NPs (Awet et al., 2018). However, denitrification enzyme activity and respiration were shown to increase after addition of nano-TiO2 (Ozaki et al., 2016). Importantly, a common metal-resistance mechanism by bacteria, such as S. oneidensis, to resist oxidative stress is the production of enzymes containing cysteine residues and glutathione (Brown et al., 2006; Mugerfeld et al., 2009), molecules that usually exhibit high affinities toward soft metals and could thus promote ligand-mediated dissolution for ENPs containing such metals.
Regarding specific impacts on EPS matrix, several studies report the extracellular production of molecules by microbes exposed to ENPs (Maurer-Jones et al., 2013; Zhu et al., 2018a, b, 2019). Particularly, the formation of more compacted biofilms and higher active site densities was observed, following a significant increase in EPS production after exposure to ENPs (Hou et al., 2015; Tang et al., 2017; Liu et al., 2018; Miao et al., 2018; Zhu et al., 2018a, b, 2019). Interestingly, this increased EPS production is thought to reduce cells damage (Hessler et al., 2012), particularly when most metal-bearing ENPs are known to produce ROS at their surface (Nel et al., 2006) which promotes oxidative stress at the cellular level. However, a denser and more functionalized EPS matrix provides more attachment sites to ENPs, thus drastically limiting their interactions with cell membranes and thus lowering exposure to ROS (Joshi et al., 2012; Zhou et al., 2016). Concurrently, even ROS generation at the ENPs surface is reported to be lowered in presence of an EPS coating, potentially due to a limited contact between NPs inorganic surface and solution (Hessler et al., 2012). However, if ENPs toxicity is too high, structural damages will occur and biofilms will, on the contrary, tend to get thinner and less dense.
All these studies show that ENPs can significantly impact microbial biofilms functioning by inducing changes in EPS composition and by promoting EPS production. Such changes can result in more packed biofilms and a significant increase in active site densities, simultaneously promoting ENPs trapping and an increased dissolution of metallic ENPs due to the presence of higher local concentrations of complexing ligands. Also, changes in the enzymes production as well as the synthesis of strong metal complexants such as cysteine residues, or molecules involved in metal reduction (e.g., flavins and riboflavins), can drastically govern changes in ENPs speciation through dissolution processes.
Long-Term Fate of ENPs in Natural Environment: the Mesocosm Approach
As stated previously, microbial biofilms control to high extents the environmental fate of ENPs. Most studies devoted to these questions of biofilm-ENPs interactions focus on model systems, providing invaluable information regarding the associated processes at the biofilm scale. However, these approaches lack the complexity of natural settings as well as the intricate interplay between the different environmental compartments. As such, in complement to these small-scale studies, more integrative approaches are required that evaluate the biofilms impact in global environmental systems.
Understanding the long-term fate and biotransformation of ENPs in the environment is challenging due to the complexity of the bio-physical-chemical interactions within natural systems including aggregation and sorption of (in)organic substances, oxidation and reduction, as well as ecological factors such as interacting organisms [e.g., microorganisms, (in)vertebrates, plants, fungi], trophic levels present (e.g., primary producer, primary consumer, secondary consumer) and trophic and transgenerational transfer potentials (Auffan et al., 2009). Taking into account this complexity requires a diverse collection of expertise, including but not limited to physical chemistry, (micro)biology, and ecology. Particularly well-suited experimental units with which to engage such multidisciplinary teams are mesocosms, that consist in medium-size replicated ecosystems (Crossland and La Point, 1992; Shaw and Kennedy, 1996; Food and Agriculture Organization, 2009). Mesocosms experiments can be designed to mimic any ecological scenarios of exposure (Auffan et al., 2019) and in the scope of ENP studies, have been defined as indoor or outdoor experimental systems containing a portion of the natural environment which is (i) self–sustaining once set up and acclimatized without any additional input of nutrients or resources, and (ii) that allows monitoring of all (or the maximum of) input and output parameters to measure changes in the environment, and in the concentration and speciation of ENPs over time, space, and ecosystem compartments (Figure 4).
This set of information is used to discern the kinetics of (bio)transformation, (bio)distribution and impacts of the ENPs to better inform studies on their fate and modeling of their environmental risks. Biotransformation of the ENPs exerted by micro-organisms, as well as macro-organisms, is one of the main drivers of the ENPs fate and impacts on the long-term. For instance, sulfidation in the sediments is a major transformation pathway for ENPs such as Ag and Cu, that are enhanced by the activity of microbial consortia as the modulation of anoxic/anaerobic conditions in soils/sediments, or also the release of exudates and organic material during metabolism, respiration or death (e.g., Bone et al., 2012; Lowry et al., 2012). Such a release of exudates by microbial biofilms and macro-organisms is important in inducing dissolution, homo- and hetero-aggregation, and by modulating their toxic effects on the mid- to long-term, as presented previously in this review. Although the formation of bio-corona shortly after introduction in mesocosms governed the persistency of ENPs in the water column (Espinasse et al., 2018), this will depend on the size and surface properties of the pristine ENPs (Unrine et al., 2012; Geitner et al., 2018). For instance, PVP coated Ag ENPs were colloidally stabilized by plant-derived and biofilm-derived dissolved organic matter. On the contrary, gum Arabic-coated Ag ENPs were rapidly removed from the water column at the same conditions through deposition or dissolution of the particles and subsequent binding of ions to plant or biofilm on the sediment surfaces (Bone et al., 2012; Unrine et al., 2012).
Macrophytes, surficial sediments and associated microorganisms were often reported as two major sinks for ENPs. Regarding aquatic plants, studies reported extensive change in the speciation of ENMs following their adsorption or internationalization by aquatic plants. For instance, the majority of nano-Au introduced in freshwater mesocosms (outdoor, 300 L volume, 180 d exposure) were found associated to the biofilm growing on Egeria densa, where the totality of Au was complexed with cyanide (43 ± 8%), hydroxide (38 ± 4%) and thiol (19 ± 4%) ligands (Avellan et al., 2018). The driving biodissolution mechanism was the Au oxidation and subsequent complexation with cyanide [Au(CN)2–], produced as secondary metabolites by the microbiome in the periphyton associated with leaves. The displacement of CN ligands by S-R groups, led to the formation of soluble thiol-Au species such as [R-S-Au-CN]– or [R-S2-Au]– complexes. These complexes were stable at the environmental physico-chemical conditions tested and likely represent major transformation pathways for other redox active ENPs. Stegemeier et al. (2017) also investigated the speciation of nano-Ag and -Cu in wetland mesocosms (outdoor, 400 L volume, 270 d exposure). Ag(0) ENPs added to the ecosystem were found in the plant tissues as Ag(0), Ag2S, and Ag bound to thiol whereas major transformation products for Cu ENPs were identified in Cu–O–R and Cu–S–R. Such products differed from those measured in the surrounding sediments compartment and the authors suggested that plants natural defense, such as glutathione, may play an important role. Similarly, in freshwater pond mesocosms contaminated with Ce ENPs, metals speciation and redox reactions were associated with the benthic (micro)biota activity and were not measured in the surrounding natural matrices (Tella et al., 2014). A protein storage mechanism for Cu ENPs was also proposed to explain the transformation of Cu(OH)2 nano-pesticide in Cu-S, and Cu-O/N-R products in plant tissues (Avellan et al., 2020). Changes in the redox state following interactions with the micro-organisms/macrophytes systems were also observed for CeO2 ENPs, where up to 57% of the pristine Ce(IV) atoms were reduced to Ce(III). In this case, the degree of reduction was observed to increase at decreasing ENPs size, likely due to an intrinsic higher reactivity of the smaller ENPs and their greater association with reducing microbes (Geitner et al., 2018).
These studies, conducted in complex realistic systems, confirm the important impact exerted by microbial biofilms toward ENPs fate, and allow investigating their role among the different reactive environmental compartments. However, it must be noted that although mesocosms experiments were able to provide comprehensive data regarding mid-term/long-term ENPs (bio)transformation in realistic environmental conditions, these strategies were seldom applied in environmental fate studies, in comparison with standard methodologies (e.g., single-species ecotoxicity tests) (Bour et al., 2015). Nonetheless, similar approaches are needed in order to investigate ENPs fate and effects that could not be observed in less complex systems, with shorter exposure periods and at higher ENPs doses (Avellan et al., 2018). These advantages can help investigating ENPs ecological processes at a higher level of complexity, and with regard to microbiota and biofilms. They can also help defining the role played by these microbial communities for ENPs fate in complex ecosystem submitted to realistic environmental exposure conditions. Mesocosms also offer many strengths when evaluating ecological hazards and could be seen as alternative testing strategies (ATS) for complementing existing procedures in the environmental risk assessment of ENPs (Hjorth et al., 2017). By simultaneously creating representative conditions for environmental transformation and ecosystem exposure, mesocosm platforms facilitate the integration of reliable exposure and hazard data into an environmental risk assessment framework. As a next step, mesocosms could also be used to study ENPs release from nano–enabled products (e.g., cement, paint, stains, diesel additives) throughout their life cycle, especially during their uses and their end of life. This could contribute to the development of nano–enabled products, which are safer by design, as well as innovative applications. Future aging protocols used to estimate the impact of such nano–enabled products should eventually be based on realistic exposure scenarios taking into account illumination, rainfall, temperature variation, leaching in biotic conditions. Mesocosms are, and will remain extremely useful on our way to understand the behavior of nano–products over the long term in realistic environments.
Bio-Mediated Formation and Stock of Incidental NPs in the Environment: the Case of ZnS NPs
If biofilms are key environmental compartments affecting the fate of ENPs, they are also able to generate NPs from secondary precipitation of metal(oid)s within their thickness, thus favoring and enhancing metal(loid)s immobilization in environmental systems. For instance, bacteria can release inorganic phosphates (Jarosławiecka and Piotrowska-Seget, 2014) that can be extracellularly trapped into the biofilm structure, and induce metal-bearing phosphate precipitation such as pyromorphite [Pb5(PO4)3(OH,Cl)] (Templeton et al., 2003b), HUO2PO4 (Finlay et al., 1999), or Cr(III)-phosphates (Nancharaiah et al., 2010). The dynamics of secondary minerals formation can also be strongly modified on short time scales, along with changes in micro-environments physico-chemical conditions. This has been reported for S. oneidensis MR1 biofilms simultaneously exposed to lead and molybdenum. In this case, although thermodynamics calculations predicted the formation of wulfenite (PbMoO4) only, a fast precipitation of nanoscale pyromorphite crystals was observed in hot spots distributed within the biofilm thickness, while wulfenite production was observed only after 7 days of exposure to lead (Couasnon et al., 2019). Another example concerns Sulfate-Reducing bacteria (SRB), mentioned before, that are known to play a major role in the precipitation of metal sulfide minerals through the reduction of sulfate to sulfide. Sulfides (mostly as HS- at neutral pH) readily precipitate with soft metals to form metal sulfides. Among the variety of bio-mediated NPs formed from anthropic metal(oid)s sources (also called incidental NPs), the case of ZnS NPs is a good example, relatively well-documented, and will be presented here as a case-study. As such, several examples are reported including ZnS NPs generated in abandoned mine sites, contaminated sediments and organic wastes.
Bio-Mediated Formation of ZnS-NPs in Contaminated Sites
Banfield’s group has been a pioneer in the discovery of ZnS NPs formed by SRB in abandoned tunnels and drainage system of the Piquette Pb-Zn mine in southwest Wisconsin (Labrenz et al., 2000; Druschel et al., 2002; Labrenz and Banfield, 2004; Moreau et al., 2004). ZnS NPs biofilm concentration was found to be about 106 times that of associated groundwater (Labrenz et al., 2000). ZnS NPs of the order of 3–5 nm, mostly sphalerite, were observed within micron-sized spheroids. The authors proposed that the spheroids were induced by microbially derived extracellular proteins, which potentially plays an important role in limiting NP dispersion in natural environments (Moreau et al., 2007). These proteins can interact with ZnS during and after their formation. Indeed, thiol containing compounds play a key role to limit ZnS NPs aggregation and was hypothesized to be a key factor contributing to their persistence in the environment (Lau and Hsu-Kim, 2008).
In another context, the formation of ZnS NPs in a variety of organic waste (OW) has long been overlooked. The main reason lies in the fact that extreme precaution should be given for sampling and storage of samples before their analyses to avoid change in Zn speciation (Le Bars et al., 2018). OWs, used as a fertilizer for crops in many countries, is a major source of input of NPs in the environment. While several studies investigated ENPs accumulation and transformation in wastewater sludges, it has recently been shown that a considerable amount of ZnS NPs was non-intentionally produced in a variety of OWs. Among them, sewage sludge and livestock manures used as amendment have relatively high concentrations of Zn (40–4000 mg.kg–1) (Alburquerque et al., 2012; Romeo et al., 2014; Zirkler et al., 2014; Alvarenga et al., 2015). Zn in OW is mostly anthropogenic. For example, high Zn concentrations in livestock manures are explained by important quantities of Zn added in animal feed compared to their ability to assimilate it (Legros et al., 2010). Nanosized ZnS is a major Zn species in raw liquid OWs and in anaerobically digested OWs (Le Bars et al., 2018). The reductive conditions combined with high sulfur content in these systems are a favorable environment for SRB (Santegoeds et al., 1999; Tang et al., 2004; Chen et al., 2008). Therefore, it is likely that these ZnS NPs are formed because of sulfide release by SRB. Crystallite size of ZnS formed in OW was estimated around 3 nm using X-ray Absorption Spectroscopy (Formentini et al., 2017; Le Bars et al., 2018). This is consistent with the identification of 2.5–7.5 nm ZnS in digested sewage sludge by Transmission Electron Microscopy (Kim et al., 2014). Interestingly, these particles transformed within months during composting (Donner et al., 2011; Lombi et al., 2012; Le Bars et al., 2018) or after land application (Formentini et al., 2017). These results suggest a high reactivity of bio-mediated ZnS NPs compared to micrometer ZnS analog which are stable over years in soils (Robson et al., 2014). The high reactivity of ZnS NPs formed in OW could be related to their small size. Indeed, Gilbert et al. (2004) highlighted contracted interatomic distances (1%) for ZnS NPs of this range of size (3.4 nm) compared to their bulk analog. Such structural disorder can increase NP surface reactivity (Yang et al., 2016) and enhance ZnS dissolution. A better understanding of potential impacts of ZnS NPs transformation on the ecosystem and in particular on cultivated lands is needed.
Structural Properties of Biogenic ZnS NPs
It seems that SRB-induced ZnS NPs are bigger than ZnS-NPs formed in abiotic systems at similar conditions (Peltier et al., 2011; da Costa et al., 2012; Xu et al., 2016; Eskelsen et al., 2018). It could be explained by the progressive production of sulfide by SRB leading to a lower saturation index, since a lower saturation index favors particle growth (Mersmann, 1999). In the same way, ligands either initially present in the culture media, or produced metabolites by bacteria, could bind to Zn, thus lowering the saturation index. However, factors controlling the size of biogenic ZnS NPs are not clear and would require further investigation.
Biogenic ZnS NPs tend to exhibit structural defects. Indeed, Xu et al. (2016) showed stacking faults and twins on the (111) face of biogenic ZnS NPs, the only polar face, i.e., prone to interact with polar molecules such as water, phospholipids head or amino acids. This suggests that surface interaction with surrounding compounds influences ZnS NPs structure. Eskelsen et al. (2018) also observed structural defaults (twins) on the (111) face of biogenic ZnS NPs, to a lower extent because of the unfavorable particles’s orientation. The authors detected carbon traces in biogenic ZnS NPs aggregates and assumed a microbial metabolite interaction with ZnS NPs.
However, the effect of structural defects on their reactivity is unclear. For hematite NPs, Echigo et al. (2012) have shown that surface defects enhance their dissolution. Regarding ZnS NPs, Xu et al. (2016) highlighted by electronic microscopy that biogenic ZnS NPs dissolution starts where the structural defects are observed. Also, Eskelsen et al. (2018) have calculated a higher surface energy for biogenic ZnS NPs, compared with the abiotic ones, suggesting a higher reactivity. In contrast, smaller abiotic ZnS NPs have a higher available specific surface area for oxidative dissolution. Despite the gathered information regarding their properties, further investigations are needed to evaluate biotic influence on ZnS NPs reactivity, in order to predict their fate when released in the environment.
Biogenic ZnS NPs: An Underestimated Stock in the Environment That Needs Further Investigation
Although biofilms have been identified as a key compartment governing in part the environmental fate of ENPs, it also hosts favorable conditions for the formation of biogenic NPs in particular from anthropic metal(oid)s inputs. The latter is clearly overlooked despite concentrations that can reach the orders of magnitude of the expected ENPs environmental release. As an example, the concentrations of ZnS NPs observed in the studies described above are up to 4 orders of magnitudes higher than the predicted concentrations of ZnO ENPs in soils, sediments and biosolids (about 0.1, 0.1, and 10–100 mg.kg–1, respectively) (Gottschalk et al., 2013). Indeed, although ZnO ENPs was shown to transform into ZnS NPs in wastewater treatment plants (Ma et al., 2013, 2014), a large variety of other Zn sources can potentially be transformed into ZnS NPs within biofilms. In this regard, better understanding of biomediated formation of NPs in biofilms needs further attention in particular when associated with anthropic activities that potentially affect biofilm and therefore NPs stabilities. It includes the use of OW as amendments or sediment dredging for example that in both cases involve strong physico-chemical changes (e.g., oxidation) that can potentially release significant amounts of free Zn with unknown effects for ecosystems. Thus, in addition to the control they exert on ENPs fate, biofilms can also become secondary sources of NPs release in the environment, showing the central role these microbial structures are likely to play in general NPs cycling. However, numerous questions remain opened that deserve further investigation in order to better constrain their environmental impact.
Concluding Remarks
This review highlights the central role played by biofilms regarding ENPs fate in the environment. In details, these microbial structures exert a control on ENPs cycling at multiple levels, by exhibiting relatively high retention capacities and by enforcing ENPs transformations. Accumulation and migration properties within biofilms are governed by a combination of key parameters, including biofilm density, ENPs size and surface properties, creation of electrostatic and hydrophobic interactions, mineral substrate reactivity, and formation of aggregates within the biofilm thickness. Interestingly, the effects of some of these key parameters remain poorly defined despite evidence of their strong impact on ENPs accumulation and transport within biofilms. This is for instance the case of mineral substrates, on which biofilms develop, that are likely to exhibit strong complexation capacity toward ENPs. In addition, given the relatively high accumulation capacities of biofilms, these microbial structures could be considered as secondary sources of ENPs and products of ENPs transformations, able to release them gradually in ENPs-free pore waters. This problematic remains an open question of important environmental significance that needs further investigations.
ENPs are also submitted to speciation changes in biofilms. They can experience dissolution, either mediated by strong complexing ligands produced by microorganisms (siderophores, cysteine-containing molecules) or redox conditions, as well as changes in local physico-chemical properties in microenvironments. Passivation mechanisms can also happen through the precipitation of an inert secondary mineral layer, as well as through ENPs stabilization following the interactions with organic ligands from the EPS matrix. Finally, exposure to toxic ENPs can trigger a response from micro-organisms in biofilms (for instance riboflavin production), which in turn has the potential to strongly impact the ENPs fate within these microbial structures.
Mesocosms-based approaches allow to consider biofilms activity in complex ecosystems, in some ways similar to the environment, and submitted to realistic exposure conditions. As such, these approaches are extremely valuable to better constrain the role of microbial biofilms for ENPs fate, and certainly need to be developed for future research devoted to that topic.
Finally, the case of nano-ZnS shows that biofilms can also synthesize NPs in man-impacted systems. This raises important questions regarding the impact of such processes on a global NPs cycling in natural systems. However, these questions have been largely overlooked and certainly require further investigation.
Some other important points deserve direct attention. First, the general lack of established standardized protocols strongly limits inter-comparison between studies whether at the small-scale or in larger systems. Then, even if considering single-species biofilm as a model system provides important advantages to investigate complex mechanisms of interaction between ENPs and biofilms, this approach presents also some limitations. Natural biofilms function as important microbial consortia that usually include a high variety of strains. To that perspective, efforts should be conducted to investigate more realistic multi-species biofilms. Also, metagenomic approaches could help understanding the dynamic role of the different microbial communities and metabolisms in biofilms, and the complex interplay among them, during their interactions with ENPs. Finally, a vast majority of the studies compiled in this review were focused on the interactions between ENPs and bacteria biofilms. Nevertheless, in the environment, fungi and archaea play also important roles, and more studies should investigate their ability to impact ENPs cycling.
Author Contributions
MD, CL, and AG contributed to the conception of the study. MD and AG wrote the first draft of the manuscript. MA, AC, CL, and ML wrote sections of the manuscript. MB, AC, and ED revised it critically. All authors contributed to the article and approved the submitted version.
Funding
This work has received funding from Excellence Initiative of Aix – Marseille University – A∗MIDEX, a French “Investissements d’Avenir” program, through its associated Labex SERENADE project, and from the MAMBA ANR project (ANR-18-CE01-0001-01). This work was also a contribution to the OSU-Institut Pytheìas. The authors thank the PIREN-Seine program for the funding.
Conflict of Interest
The authors declare that the research was conducted in the absence of any commercial or financial relationships that could be construed as a potential conflict of interest.
Acknowledgments
We acknowledge the CNRS for the funding of the IRP iNOVE.
References
Adeleye, A. S., Conway, J. R., Perez, T., Rutten, P., and Keller, A. A. (2014). Influence of extracellular polymeric substances on the long-term fate, dissolution, and speciation of copper-based nanoparticles. Environ. Sci. Technol. 48, 12561–12568. doi: 10.1021/es5033426
Adeleye, A. S., and Keller, A. A. (2016). Interactions between algal extracellular polymeric substances and commercial TiO2 nanoparticles in aqueous media. Environ. Sci. Technol. 50, 12258–12265. doi: 10.1021/acs.est.6b03684
Albelda-Berenguer, M., Monachon, M., and Joseph, E. (2019). Siderophores: from natural roles to potential applications. Adv. Appl. Microbiol. 106, 193–225. doi: 10.1016/bs.aambs.2018.12.001
Alburquerque, J. A., de la Fuente, C., Ferrer-Costa, A., Carrasco, L., Cegarra, J., Abad, M., et al. (2012). Assessment of the fertiliser potential of digestates from farm and agroindustrial residues. Biomass Bioenergy 40, 181–189. doi: 10.1016/j.biombioe.2012.02.018
Aldeek, F., Mustin, C., Balan, L., Roques-Carmes, T., Fontaine-Aupart, M. P., and Schneider, R. (2011). Surface-engineered quantum dots for the labeling of hydrophobic microdomains in bacterial biofilms. Biomaterials 32, 5459–5470. doi: 10.1016/j.biomaterials.2011.04.019
Alizadeh, S., Ghoshal, S., and Comeau, Y. (2019). Fate and inhibitory effect of silver nanoparticles in high rate moving bed biofilm reactors. Sci. Total Environ. 647, 1199–1210. doi: 10.1016/j.scitotenv.2018.08.073
Alvarenga, P., Mourinha, C., Farto, M., Santos, T., Palma, P., Sengo, J., et al. (2015). Sewage sludge, compost and other representative organic wastes as agricultural soil amendments: benefits versus limiting factors. Waste Manage. 40, 44–52. doi: 10.1016/j.wasman.2015.01.027
Auffan, M., Masion, A., Mouneyrac, C., de Garidel-Thorona, C., Hendren, C. O., Thiery, A., et al. (2019). Contribution of mesocosm testing to a single-step and exposure-driven environmental risk assessment of engineered nanomaterials. Nanoimpact 13, 66–69. doi: 10.1016/j.impact.2018.12.005
Auffan, M., Rose, J., Bottero, J., Lowry, G. V., Jolivet, J., and Wiesner, M. R. (2009). Towards a definition of inorganic nanoparticles from an environmental, health and safety perspective. Nat. Nanotechnol. 4, 634–641. doi: 10.1038/NNANO.2009.242
Avellan, A., Simonin, M., Anderson, S. M., Geitner, N. K., Bossa, N., Spielman-Sun, E., et al. (2020). Differential reactivity of copper- and gold-based nanomaterials controls their seasonal biogeochemical cycling and fate in a freshwater wetland mesocosm. Environ. Sci. Technol. 54, 1533–1544. doi: 10.1021/acs.est.9b05097
Avellan, A., Simonin, M., McGivney, E., Bossa, N., Spielman-Sun, E., Rocca, J. D., et al. (2018). Gold nanoparticle biodissolution by a freshwater macrophyte and its associated microbiome. Nat. Nanotechnol. 13, 1072–1077. doi: 10.1038/s41565-018-0231-y
Awet, T. T., Kohl, Y., Meier, F., Straskraba, S., Grün, A. L., Ruf, T., et al. (2018). Effects of polystyrene nanoparticles on the microbiota and functional diversity of enzymes in soil. Environ. Sci. Eur. 30:11. doi: 10.1186/s12302-018-0140-6
Barker, W., and Banfield, J. F. (1998). Zones of chemical and physical interaction at interfaces between microbial communities and minerals. A model. Geomicrobiol. J. 15, 223–244. doi: 10.1080/01490459809378078
Bar-On, Y. M., Phillips, R., and Milo, R. (2018). The biomass distribution on Earth. Proc. Natl. Acad. Sci. U.S.A. 115, 6506–6511. doi: 10.1073/pnas.1711842115
Battin, T. J., Kammer, F. V. D., Weilhartner, A., Ottofuelling, S., and Hofmann, T. (2009). Nanostructured TiO2: transport behavior and effects on aquatic microbial communities under environmental conditions. Environ. Sci. Technol. 43, 8098–8104. doi: 10.1021/es9017046
Bian, S. W., Mudunkotuwa, I. A., Rupasinghe, T., and Grassian, V. H. (2011). Aggregation and dissolution of 4 nm ZnO nanoparticles in aqueous environments: influence of pH, Ionic Strength, Size, and Adsorption of Humic Acid. Langmuir 27, 6059–6068. doi: 10.1021/la200570n
Billings, N., Birjiniuk, A., Samad, T. S., Doyle, P. S., and Ribbeck, K. (2015). Material properties of biofilms - A review of methods for understanding permeability and mechanics. Rep. Prog. Phys. 78:036601. doi: 10.1088/0034-4885/78/3/036601
Binh, C. T. T., Adams, E., Vigen, E., Tong, T., Alsina, M. A., Gaillard, J.-F., et al. (2016). Chronic addition of a common engineered nanomaterial alters bio-mass, activity and composition of stream biofilm communities. Environ. Sci. Nano 3, 619–630. doi: 10.1039/c5en00274e
Binh, C. T. T., Tong, T., Gaillard, J.-F., Gray, K. A., and Kelly, J. J. (2014). Acute effects of TiO2 nanomaterials on the viability and taxonomic composition of aquatic bacterial communities assessed via high-throughput screening and next generation sequencing. PLos One 9:e106280. doi: 10.1371/journal.pone.0106280
Bone, A. J., Colman, B. P., Gondikas, A. P., Newton, K. M., Harrold, K. H., Cory, R. M., et al. (2012). Biotic and abiotic interactions in aquatic microcosms determine fate and toxicity of Ag nanoparticles: part 2-toxicity and Ag speciation. Environ. Sci. Technol. 46, 6925–6933. doi: 10.1021/es204683m
Borrok, D., Fein, J. B., and Kulpa, C. F. (2004a). Cd and proton adsorption onto bacterial consortia grown from industrial wastes and contaminated geologic settings. Environ. Sci. Technol. 38, 5656–5664. doi: 10.1021/es049679n
Borrok, D., Fein, J. B., and Kulpa, C. F. (2004b). Proton and Cd adsorption onto natural bacterial consortia: testing universal adsorption behavior. Geochim. Cosmochim. Acta 68, 3231–3238. doi: 10.1016/j.gca.2004.02.003
Borrok, D., Turner, B. F., and Fein, J. B. (2005). Universal surface complexation framework for modeling proton binding onto bacterial surfaces in geological settings. Am. J. Sci. 305, 826–853. doi: 10.2475/ajs.305.6-8.826
Bour, A., Mouchet, F., Silvestre, J., Gauthier, L., and Pinelli, E. (2015). Environmentally relevant approaches to assess nanoparticles ecotoxicity: a review. J. Hazard. Mater. 283, 764–777. doi: 10.1016/j.jhazmat.2014.10.021
Brown, G. E. (2001). How minerals react with water. Science 294, 67–69. doi: 10.1126/science.1063544
Brown, G. E., Henrich, V. E., Casey, W. H., Clark, D. L., Eggleston, C., Felmy, A., et al. (1999). Metal oxide surfaces and their interactions with aqueous solutions and microbial organisms. Chem. Rev. 99, 77–174. doi: 10.1021/cr980011z
Brown, S. D., Thompson, M. R., VerBerkmoes, N. C., Chourey, K., Shah, M., Zhou, J., et al. (2006). Molecular dynamics of the Shewanella oneidensis response to chromate stress. Mol. Cell. Proteomics 5, 1054–1071. doi: 10.1074/mcp.M500394-MCP200
Buchman, J. T., Pho, T., Rodriguez, R. S., Feng, Z. V., and Haynes, C. L. (2019). Coating iron oxide nanoparticles with mesoporous silica reduces their interaction and impact on S. oneidensis MR-1. Chemosphere 237:124511. doi: 10.1016/j.chemosphere.2019.124511
Burns, J. M., Pennington, P. L., Sisco, P. N., Frey, R., Kashiwada, S., Fulton, M. H., et al. (2013). Surface charge controls the fate of Au nanorods in saline estuaries. Environ. Sci. Technol. 47, 12844–12851. doi: 10.1021/es402880u
Chen, Y., Cheng, J. J., and Creamer, K. S. (2008). Inhibition of anaerobic digestion process: a review. Bioresour. Technol. 99, 4044–4064. doi: 10.1016/j.biortech.2007.01.057
Choi, O., Yu, C.-P., Fernandez, G. E., and Hu, Z. (2010). Interactions of nanosilver with Escherichia coli cells in planktonic and biofilm cultures. Water Res. 44, 6095–6103. doi: 10.1016/j.watres.2010.06.069
Costerton, J. W., Cheng, K. J., Geesey, G. G., Ladd, T. I., Nickel, J. C., Dasgupta, M., et al. (1987). Bacteria biofilms in nature and disease. Annu. Rev. Microbiol. 41, 435–464. doi: 10.1146/annurev.mi.41.100187.002251
Costerton, J. W., Lewandowski, Z., Caldwell, D. E., Korber, D. R., and Lappin-Scott, H. M. (1995). Microbial biofilms. Annu. Rev. Microbiol. 49, 711–745. doi: 10.1146/annurev.mi.49.100195.003431
Costerton, J. W., Stewart, P., and Greenberg, E. P. (1999). Bacterial biofilms: a common cause of persistent infections. Science 284, 1318–1322. doi: 10.1126/science.284.5418.1318
Couasnon, T., Gelabert, A., Menez, B., and Guyot, F. (2019). Experimental assessment of occurrences and stability of lead-bearing minerals in bacterial biofilms. Chem. Geol. 505, 23–35. doi: 10.1016/j.chemgeo.2018.11.023
Crampon, M., Hellal, J., Mouvet, C., Wille, G., Michel, C., Wiener, A., et al. (2018). Do natural biofilm impact nZVI mobility and interactions with porous media? A column study. Sci. Total Environ. 610, 709–719. doi: 10.1016/j.scitotenv.2017.08.106
Crossland, N. O., and La Point, T. W. (1992). The design of mesocosms experiments. Environ. Toxicol. Chem. 11, 1–4. doi: 10.1002/etc.5620110101
da Costa, J. P., Girao, A. V., Lourenco, J. P., Monteiro, O. C., Trindade, T., and Costa, M. C. (2012). Synthesis of nanocrystalline ZnS using biologically generated sulfide. Hydrometallurgy 117, 57–63. doi: 10.1016/j.hydromet.2012.02.005
Dehner, C. A., Awaya, J. D., Maurice, P. A., and DuBois, J. L. (2010). Roles of siderophores, oxalate, and ascorbate in mobilization of iron from hematite by the aerobic bacterium Pseudomonas mendocina. Appl. Environ. Microbiol. 76, 2041–2048. doi: 10.1128/AEM.02349-09
Desmau, M., Gélabert, A., Levard, C., Ona-Nguema, G., Vidal, V., Stubbs, J. E., et al. (2018). Dynamics of silver nanoparticles at the solution/biofilm/mineral interface. Environ. Sci. Nano 5, 2394–2405. doi: 10.1039/c8en00331a
Diaz, J. M., Hansel, C. M., Voelker, B. M., Mendes, C. M., Andeer, P. F., and Zhang, T. (2013). Widespread production of extracellular superoxide by Heterotrophic Bacteria. Science 340, 1223–1226. doi: 10.1126/science.123
Dimkpa, C. O., McLean, J. E., Britt, D. W., Johnson, W. P., Arey, B., Lea, S. A., et al. (2012). Nanospecific inhibition of pyoverdine siderophore production in Pseudomonas chlororaphis O6 by CuO nanoparticles. Chem. Res. Toxicol. 25, 1066–1074. doi: 10.1021/tx3000285
Donlan, R. M. (2002). Biofilms: microbial life on surfaces. Emerg. Infect. Dis. 8, 881–890. doi: 10.3201/eid0809.020063
Donner, E., Howard, D. L., Jonge, M. D. D., Paterson, D., Cheah, M. H., Naidu, R., et al. (2011). X-ray absorption and micro X-ray fluorescence spectroscopy investigation of copper and zinc speciation in biosolids. Environ. Sci. Technol. 45, 7249–7257. doi: 10.1021/es201710z
Druschel, G. K., Labrenz, M., Thomsen-Ebert, T., Fowle, D. A., and Banfield, J. F. (2002). Geochemical Modeling of ZnS in biofilms: an example of ore depositional processes. Econ. Geol. Bull. Soc. Econ. Geol. 97, 1319–1329. doi: 10.2113/gsecongeo.97.6.1319
Dzumedzey, Y., Labille, J., Cathala, B., Moreau, C., and Santaella, C. (2017). Polysaccharide coating on environmental collectors affects the affinity and deposition of nanoparticles. Nanoimpact 5, 83–91. doi: 10.1016/j.impact.2016.12.004
Echavarri-Bravo, V., Paterson, L., Aspray, T. J., Porter, J. S., Winson, M. K., Thornton, B., et al. (2015). Shifts in the metabolic function of a benthic estuarine microbial community following a single pulse exposure to silver nanoparticles. Environ. Pollut. 201, 91–99. doi: 10.1016/j.envpol.2015.02.033
Echigo, T., Aruguete, D. M., Murayama, M., and Hochella, M. F. Jr. (2012). Influence of size, morphology, surface structure, and aggregation state on reductive dissolution of hematite nanoparticles with ascorbic acid. Geochim. Cosmochim. Acta 90, 149–162. doi: 10.1016/j.gca.2012.05.008
Eskelsen, J. R., Xu, J., Chiu, M., Moon, J.-W., Wilkins, B., Graham, D. E., et al. (2018). Influence of structural defects on biomineralized ZnS nanoparticle dissolution: an in-situ electron microscopy study. Environ. Sci. Technol. 52, 1139–1149. doi: 10.1021/acs.est.7b04343
Espinasse, B. P., Geitner, N. K., Schierz, A., Therezien, M., Richardson, C. J., Lowry, G. V., et al. (2018). Comparative persistence of engineered nanoparticles in a complex aquatic ecosystem. Environ. Sci. Technol. 52, 4072–4078. doi: 10.1021/acs.est.7b06142
Fabrega, J., Renshaw, J. C., and Lead, J. R. (2009). Interactions of silver nanoparticles with Pseudomonas putida biofilms. Environ. Sci. Technol. 43, 9004–9009. doi: 10.1021/es901706j
Fan, W., Liu, T., Li, X., Peng, R., and Zhang, Y. (2016). Nano-TiO2 affects cu speciation, extracellular enzyme activity, and bacterial communities in sediments. Environ. Pollut. 218, 77–85. doi: 10.1016/j.envpol.2016.08.018
Fein, J. B., Daughney, C. J., Yee, N., and Davis, T. A. (1998). A chemical equilibrium model for metal adsorption onto bacterial surfaces. Geochim. Cosmochim. Acta 61, 3319–3328. doi: 10.1016/S0016-7037(97)00166-X
Fernando, I., Lu, D., and Zhou, Y. (2020). Interactive influence of extracellular polymeric substances (EPS) and electrolytes on the colloidal stability of silver nanoparticles. Environ. Sci. Nano 7, 186–197. doi: 10.1039/c9en00861f
Ferry, J. L., Craig, P., Hexel, C., Sisco, P., Frey, R., Pennington, P. L., et al. (2009). Transfer of gold nanoparticles from the water column to the estuarine food web. Nat. Nanotechnol. 4, 441–444. doi: 10.1038/NNANO.2009.157
Finlay, J. A., Allan, V. J. M., Conner, A., Callow, M. E., Basnakova, G., and Macaskie, L. E. (1999). Phosphate release and heavy metal accumulation by biofilm-immobilized and chemically-coupled cells of a citrobacter sp. pre-grown in continuous culture. Biotechnol. Bioeng. 63, 87–97. doi: 10.1002/(sici)1097-0290(19990405)63:1<87::aid-bit9>3.0.co;2-0
Flemming, H. C., and Wingender, J. (2010). The biofilm matrix. Nat. Rev. Microbiol. 8, 623–633. doi: 10.1038/nrmicro2415
Flemming, H.-C., Wingender, J., Szewzyk, U., Steinberg, P., Rice, S. A., and Kjelleberg, S. (2016). Biofilms: an emergent form of microbial life. Nat. Rev. Microbiol. 14, 563–575. doi: 10.1038/nrmicro.2016.94
Flemming, H. C., and Wuertz, S. (2019). Bacteria and archaea on Earth and their abundance in biofilms. Nat. Rev. Microbiol. 17, 247–260. doi: 10.1038/s41579-019-0158-9
Food and Agriculture Organization [FAO] (2009). Biosafety of Genetically Modified Organisms: Basic Concepts, Methods and Issues. Rome: FAO.
Formentini, T. A., Legros, S., Fernandes, C. V. S., Pinheiro, A., Le Bars, M., Levard, C., et al. (2017). Radical change of Zn speciation in pig slurry amended soil: Key role of nano-sized sulfide particles. Environ. Pollut. 222, 495–503. doi: 10.1016/j.envpol.2016.11.056
Franklin, N. M., Rogers, N. J., Apte, S. C., Batley, G. E., Gadd, G. E., and Casey, P. S. (2007). Comparative toxicity of nanoparticulate ZnO, bulk ZnO, and ZnCl2 to a freshwater microalga (Pseudokirchneriella subcapitata): the importance of particle solubility. Environ. Sci. Technol. 41, 8484–8490. doi: 10.1021/es071445r
Fulaz, S., Hiebner, D., Barros, C. H. N., Devlin, H., Vitale, S., et al. (2019a). Ratiometric imaging of the in situ pH distribution of biofilms by use of fluorescent mesoporous silica nanosensors. ACS Appl. Mater. Interfaces 11, 32679–32688. doi: 10.1021/acsami.9b09978
Fulaz, S., Vitale, S., Quinn, L., and Casey, E. (2019b). Nanoparticle–biofilm interactions: the role of the EPS matrix. Trends Microbiol. 27, 915–926. doi: 10.1016/j.tim.2019.07.004
Gadd, G. M. (2010). Metals, minerals and microbes: geomicrobiology and bioremediation. Microbiology 156, 609–643. doi: 10.1099/mic.0.037143-0
Geitner, N. K., Cooper, J. L., Avellan, A., Castellon, B. T., Perrotta, B. G., Bossa, N., et al. (2018). Size-based differential transport, uptake, and mass distribution of ceria (CeO2) nanoparticles in wetland mesocosms. Environ. Sci. Technol. 52, 9768–9776. doi: 10.1021/acs.est.8b02040
Gelabert, A., Sivry, Y., Ferrari, R., Akrout, A., Cordier, L., Nowak, S., et al. (2014). Uncoated and coated ZnO nanoparticles life cycle in synthetic seawater. Environ. Toxicol. Chem. 22, 341–349. doi: 10.1002/etc.2447
Gelabert, A., Sivry, Y., Gobbi, P., Mansour, N., Menguy, N., Brayner, R., et al. (2015). Testing nano effect onto model bacteria: impact of speciation and genotypes. Nanotoxicology 10, 216–25. doi: 10.3109/17435390.2015.1048323
Giese, B., Klaessig, F., Park, B., Kaegi, R., Steinfeldt, M., Wigger, H., et al. (2018). Risks, release and concentrations of engineered nanomaterial in the environment. Sci. Rep. 8:1565. doi: 10.1038/s41598-018-19275-4
Gil-Allué, C., Schirmer, K., Tlili, A., Gessner, M. O., and Behra, R. (2015). Silver nanoparticle effects on stream periphyton during short-term exposures. Environ. Sci. Technol. 49, 1165–1172. doi: 10.1021/es5050166
Gilbert, B., Huang, F., Zhang, H., Waychunas, G. A., and Banfield, J. F. (2004). Nanoparticles: strained and stiff. Science 305, 651–654. doi: 10.1126/science.1098454
Golmohamadi, M., Clark, R. J., Veinot, J. G. C., and Wilkinson, K. J. (2013). The role of charge on the diffusion of solutes and nanoparticles (silicon nanocrystals, nTiO2, nAu) in a biofilm. Environ. Chem. 10, 34–41. doi: 10.1071/EN12106
Gottschalk, F., Sonderer, T., Scholz, R. W., and Nowack, B. (2009). Modeled environmental concentrations of engineered nanomaterials (TiO2, ZnO, Ag, CNT, fullerenes) for Different Regions. Environ. Sci. Technol. 43, 9216–9222. doi: 10.1021/es9015553
Gottschalk, F., Sun, T., and Nowack, B. (2013). Environmental concentrations of engineered nanomaterials: review of modeling and analytical studies. Environ. Pollut. 181, 287–300. doi: 10.1016/j.envpol.2013.06.003
Guiot, E., Georges, P., Brun, A., Fontaine-Aupart, M. P., Bellon-Fontaine, M. N., and Briandet, R. (2002). Heterogeneity of diffusion inside microbial biofilms determined by fluorescence correlation spectroscopy under two-photon excitation. Photochem. Photobiol. 75, 570–578. doi: 10.1562/0031-8655(2002)0750570hodimb2.0.co2
Ha, J., Gelabert, A., Spormann, A., and Brown, G. E. Jr. (2010). Role of extracellular polymeric substances in metal ion complexation on Shewanella oneidensis: batch uptake, thermodynamic modeling, ATR-FTIR, and EXAFS study. Geochim. Cosmochim. Acta 74, 1–15. doi: 10.1016/j.gca.2009.06.031
Habimana, O., Steenkeste, K., Fontaine-Aupart, M. P., Bellon Fontaine, M. N., Kulakauskas, S., and Briandet, R. (2011). Diffusion of nanoparticles in biofilms is altered by bacterial cell wall hydrophobicity. Appl. Environ. Microbiol. 77, 367–368. doi: 10.1128/AEM.02163-10
Hamel, R., Levasseur, R., and Apana, V. D. (1999). Oxalic acid production and aluminum tolerance in Pseudomonas fluorescens. J. Inorg. Biochem. 76, 99–104. doi: 10.1016/S0162-0134(99)00120-8
Han, Y., Hwang, G., Kim, D., Bradford, S. A., Lee, B., Eom, I., et al. (2016). Transport, retention, and long-term release behavior of ZnO nanoparticle aggregates in saturated quartz sand: role of solution pH and biofilm coating. Water Res. 90, 247–257. doi: 10.1016/j.watres.2015.12.009
Herrling, M. P., Lackner, S., Tatti, O., Guthausen, G., Delay, M., Franzreb, M., et al. (2016). Short and long term biosorption of silica-coated iron oxide nanoparticles in heterotrophic biofilms. Sci. Total. Environ. 544, 722–729. doi: 10.1016/j.scitotenv.2015.11.174
Hessler, C. M., Wu, M. Y., Xue, Z., Choi, H., and Seo, Y. (2012). The influence of capsular extracellular polymeric substances on the interaction between TiO2 nanoparticles and planktonic bacteria. Water Res. 46, 4687–4696. doi: 10.1016/j.watres.2012.06.009
Hidalgo, G., Burns, A., Herz, E., Hay, A. G., Houston, P. L., Wiesner, U., et al. (2009). Functional tomographic fluorescence imaging of pH microenvironments in microbial biofilms by use of silica nanoparticle sensors. Appl. Environ. Microbiol. 75, 7426–7435. doi: 10.1128/AEM.01220-09
Hjorth, R., Holden, P. A., Hansen, S. F., Colman, B. P., Grieger, and Hendren, C. O. (2017). The role of alternative testing strategies in environmental risk assessment of engineered nanomaterials. Environ. Sci. Nano 4, 292–301. doi: 10.1039/c6en00443a
Holden, P. A., Gardea-Torresdey, J. L., Klaessig, F., Turco, R. F., Mortimer, M., Hund-Rinke, K., et al. (2016). Considerations of Environmentally Relevant Test Conditions for Improved Evaluation of Ecological Hazards of Engineered Nanomaterials. Environ. Sci. Technol. 50, 6124–6145. doi: 10.1021/acs.est.6b00608
Hou, J., Miao, L. Z., Wang, C., Wang, P. F., Ao, Y. H., and Lv, B. W. (2015). Effect of CuO nanoparticles on the production and composition of extracellular polymeric substances and physicochemical stability of activated sludge flocs. Bioresour. Technol. 176, 65–70. doi: 10.1016/j.biortech.2014.11.020
Hunter, R. C., and Beveridge, T. J. (2005). Application of a pH-sensitive fluoroprobe (C-SNARF-4) for pH microenvironment analysis in Pseudomonas aeruginosa biofilms. Appl. Environ. Microbiol. 71, 2501–2510. doi: 10.1128/AEM.71.5.2501-2510.2005
Ikuma, K., Decho, A. W., and Lau, B. L. T. (2015). When nanoparticles meet biofilms-interactions guiding the environmental fate and accumulation of nanoparticles. Front. Microbiol. 6:591. doi: 10.3389/fmicb.2015.00591
Ikuma, K., Madden, A. S., Decho, A. W., and Lau, B. L. T. (2014). Deposition of nanoparticles onto polysaccharide-coated surfaces: implications for nanoparticle–biofilm interactions. Environ. Sci. Nano 1, 117–122. doi: 10.1039/c3en00075c
Jarosławiecka, A., and Piotrowska-Seget, S. (2014). Lead resistance in micro-organisms. Microbiology 160, 12–25. doi: 10.1099/mic.0.070284-0
Jiang, X., Wang, X., Tong, M., and Kim, H. (2013). Initial transport and retention behaviors of ZnO nanoparticles in quartz sand porous media coated with Escherichia coli biofilm. Environ. Pollut. 174, 38–49. doi: 10.1016/j.envpol.2012.11.016
Jian-Zhou, H., Cheng-Cheng, L., Deng-Jun, W., and Zhou, D.-M. (2015). Biofilms and extracellular polymeric substances mediate the transport of graphene oxide nanoparticles in saturated porous media. J. Hazard. Mater. 300, 467–474. doi: 10.1016/j.jhazmat.2015.07.026
Jing, H., Mezgebe, B., Hassan, A. A., Sahle-Demessie, E., Sorial, G. A., and Bennett-Stamper, C. (2014). Experimental and modeling studies of sorption of ceria nanoparticle on microbial biofilms. Bioresour. Technol. 161, 109–117. doi: 10.1016/j.biortech.2014.03.015
Jomini, S., Clivot, H., Bauda, P., and Pagnout, C. (2015). Impact of manufactured TiO2 nanoparticles on planktonic and sessile bacterial communities. Environ. Pollut. 202, 196–204. doi: 10.1016/j.envpol.2015.03.022
Joo, S. H., and Aggarwal, S. (2018). Factors impacting the interactions of engineered nanoparticles with bacterial cells and biofilms: mechanistic insights and state of knowledge. J. Environ. Manag. 225, 62–74. doi: 10.1016/j.jenvman.2018.07.084
Joshi, N., Ngwenya, B. T., and French, C. E. (2012). Enhanced resistance to nanoparticle toxicity is conferred by overproduction of extracellular polymeric substances. J. Hazard. Mater. 241-242, 363–370. doi: 10.1016/j.jhazmat.2012.09.057
Kalinowski, B. E., Liermann, L. J., Givens, S., and Brantley, S. L. (2000). Rates of bacteria-promoted solubilization of Fe from minerals: a review of problems and approaches. Chem. Geol. 169, 357–370. doi: 10.1016/S0009-2541(00)00214-X
Kataky, R., and Knowles, E. (2018). Biofilm formation on abiotic surfaces and their redox activity. Curr. Opin. Electrochem. 12, 121–128. doi: 10.1016/j.coelec.2018.07.007
Keller, A. A., McFerran, S., Lazareva, A., and Suh, S. (2013). Global life cycle releases of engineered nanomaterials. J. Nanopart. Res. 15:1692. doi: 10.1007/s11051-013-1692-4
Khan, S. S., Mukherjee, A., and Chandrasekaran, N. (2011a). Impact of exopolysaccharides on the stability of silver nanoparticles in water. Water Res. 45, 5184–5190. doi: 10.1016/j.watres.2011.07.024
Khan, S. S., Srivatsan, P., Vaishnavi, N., Mukherjee, A., and Chandrasekaran, N. (2011b). Interaction of silver nanoparticles (SNPs) with bacterial extracellular proteins (ECPs) and its adsorption isotherms and kinetics. J. Hazard Mater. 192, 299–306. doi: 10.1016/j.colsurfa.2011.03.032
Kim, B., Levard, C., Murayama, M., Brown, G. E., and Hochella, M. F. (2014). Integrated approaches of X-ray absorption spectroscopic and electron microscopic techniques on zinc speciation and characterization in a final sewage sludge product. J. Environ. Qual. 43, 908–916. doi: 10.2134/jeq2013.10.0418
Kotloski, N. J., and Gralnick, J. A. (2013). Flavin electron shuttles dominate extracellular electron transfer by Shewanella oneidensis. mBio 4:e00553-12. doi: 10.1128/mBio.0055312
Kraemer, S. M. (2004). Iron oxide dissolution and solubility in the presence of siderophores. Aquat. Sci. 66, 3–18. doi: 10.1007/s00027-003-0690-5
Kroll, A., Behra, R., Kaegi, R., and Sigg, L. (2014). Extracellular polymeric substances (EPS) of freshwater biofilms stabilize and modify CeO2 and Ag nanoparticles. PLoS One 9:e110709. doi: 10.1371/journal.pone.0110709
Kurlanda-Witek, H., Ngwenya, B., and Butler, I. (2015). The influence of biofilms on the mobility of bare and capped zinc oxide nanoparticles in saturated sand and glass beads. J. Cont. Hydrol. 179, 160–170. doi: 10.1016/j.jconhyd.2015.06.009
Kuzyakov, Y., and Blagodatskaya, E. (2015). Microbial hotspots and hot moments in soil: concept & review. Soil Biol. Biochem. 83, 184–199. doi: 10.1016/j.soilbio.2015.01.025
Labrenz, M., and Banfield, J. F. (2004). Sulfate-reducing bacteria-dominated biofilms that precipitate ZnS in a subsurface circumneutral-pH mine drainage system. Microb. Ecol. 47, 205–217. doi: 10.1007/s00248-003-1025-8
Labrenz, M., Druschel, G. K., Thomsen-Ebert, T., Gilbert, B., Welch, S. A., Kemner, K. M., et al. (2000). Formation of Sphalerite (ZnS) deposits in natural biofilms of sulfate-reducing Bacteria. Science 290, 1744–1747. doi: 10.1126/science.290.5497.1744
Lau, B. L., and Hsu-Kim, H. (2008). Precipitation and growth of zinc sulfide nanoparticles in the presence of thiol-containing natural organic ligands. Environ. Sci. Technol. 42, 7236–7241. doi: 10.1021/es801360b
Layet, C., Auffan, M., Santaella, C., Chevassus-Rosset, C., Montes, M., Ortet, P., et al. (2017). Evidence that soil properties and organic coating drive the phytoavailability of cerium oxide nanoparticles. Environ. Sci. Technol. 51, 9756–9764. doi: 10.1021/acs.est.7b02397
Le Bars, M., Legros, S., Levard, C., Chaurand, P., Tella, M., Rovezzi, M., et al. (2018). Drastic change in zinc speciation during anaerobic digestion and composting: instability of nanosized zinc sulfide. Environ. Sci. Technol. 52, 12987–12996. doi: 10.1021/acs.est.8b02697
Le Ouay, B., and Stellacci, F. (2015). Antibacterial activity of silver nanoparticles: a surface science insight. Nano Today 10, 339–354. doi: 10.1016/j.nantod.2015.04.002
Legros, S., Doelsch, E., Masion, A., Rose, J., Borshneck, D., Proux, O., et al. (2010). Combining size fractionation, scanning electron microscopy, and X-ray absorption spectroscopy to probe zinc speciation in pig slurry. J. Environ. Qual. 39, 531–540. doi: 10.2134/jeq2009.0096
Lerner, R. N., Lu, Q., Zeng, H., and Liu, Y. (2012). The effects of biofilm on the transport of stabilized zerovalent iron nanoparticles in saturated porous media. Water Res. 46, 975–985. doi: 10.1016/j.watres.2011.11.070
Levard, C., Hotze, E. M., Colman, B. P., Dale, A. L., Truong, L., Yang, X. Y., et al. (2013). Sulfidation of silver nanoparticles: natural antidote to their toxicity. Environ. Sci. Technol. 47, 13440–13448. doi: 10.1021/es403527n
Levard, C., Hotze, E. M., Lowry, G. V., and Brown, G. E. (2012). Environmental Transformations of Silver nanoparticles: impact on stability and toxicity. Environ. Sci. Technol. 46, 6900–6914. doi: 10.1021/es2037405
Levard, C., Reinsch, B. C., Michel, F. M., Oumahi, C., Lowry, G. V., and Brown, G. E. Jr. (2011). Sulfidation processes of PVP-coated silver nanoparticles in aqueous solution: impact on dissolution rate. Environ. Sci. Technol. 45, 5260–5266. doi: 10.1021/es2007758
Li, X., Yeh, Y. C., Giri, K., Mout, R., Landis, R. F., Prakash, Y. S., et al. (2015). Control of nanoparticle penetration into biofilms through surface design. Chem. Commun. 51, 282–285. doi: 10.1039/c4cc07737g
Li, Z., Aly Hassan, A., Sahle-Demessie, E., and Sorial, G. A. (2013). Transport of nanoparticles with dispersant through biofilm coated drinking water sand filters. Water Res. 47, 6457–6466. doi: 10.1016/J.WATRES.2013.08.026
Lin, D., Story, S. D., Walker, S. L., Huang, Q., and Cai, P. (2016). Influence of extracellular polymeric substances on the aggregation kinetics of TiO2 nanoparticles. Water Res. 104, 381–388. doi: 10.1016/j.watres.2016.08.044
Liu, S., Cao, S., Guo, J., Luo, L., Zhou, Y., Lin, C., et al. (2018). Graphene oxide–silver nanocomposites modulate biofilm formation and extracellular polymeric substance (EPS) production. Nanoscale 10, 19603–19611. doi: 10.1039/c8nr04064h
Lombi, E., Donner, E., Tavakkoli, E., Turney, T. W., Naidu, R., Miller, B. W., et al. (2012). Fate of zinc oxide nanoparticles during anaerobic digestion of wastewater and post-treatment processing of sewage sludge. Environ. Sci. Technol. 46, 9089–9096. doi: 10.1021/es301487s
Lowry, G. V., Espinasse, B. P., Badireddy, A. R., Richardson, C. J., Reinsch, B. C., Lee, D. B., et al. (2012). Long-term transformation and fate of manufactured Ag nanoparticles in a simulated large scale freshwater emergent wetland. Environ. Sci. Technol. 46, 7027–7036. doi: 10.1021/es204608d
Ma, R., Levard, C., Judy, J. D., Unrine, J. M., Durenkamp, M., Martin, B., et al. (2014). Fate of zinc oxide and silver nanoparticles in a pilot wastewater treatment plant and in processed biosolids. Environ. Sci. Technol. 48, 104–112. doi: 10.1021/es403646x
Ma, R., Levard, C., Michel, F. M., Brown, G. E. Jr., and Lowry, G. V. (2013). Sulfidation mechanism for zinc oxide nanoparticles and the effect of sulfidation on their solubility. Environ. Sci. Technol. 47, 2527–2534. doi: 10.1021/es3035347
Maurer-Jones, M. A., Gunsolus, I. L., Meyer, B. M., Christenson, C. J., and Haynes, C. L. (2013). Impact of TiO2 nanoparticles on growth, biofilm formation, and flavin secretion in Shewanella oneidensis. Anal. Chem. 85, 5810–5818. doi: 10.1021/ac400486u
McGivney, E., Gao, X., Liu, Y., Lowry, G. V., Casman, E. A., and Gregory, K. B. (2019). Biogenic cyanide production promotes dissolution of gold nanoparticles in soil. Environ. Sci. Technol. 53, 1287–1295. doi: 10.1021/acs.est.8b05884
Ménez, B., Pasini, V., and Brunelli, D. (2012). Life in the hydrated suboceanic mantle. Nat. Geosci. 5, 133–137. doi: 10.1038/NGEO1359
Mersmann, A. (1999). Crystallization and precipitation. Chem. Eng. Process. 38, 345–353. doi: 10.1016/S0255-2701(99)00025-2
Miao, L., Wang, C., Hou, J., Wang, P., Ao, Y., Li, Y., et al. (2015). Enhanced stability and dissolution of CuO nanoparticles by extracellular polymeric substances in aqueous environment. J. Nanopart. Res. 17:404. doi: 10.1007/s11051-015-3208-x
Miao, L., Wang, P., Wang, C., Hou, J., Yao, Y., Liu, J., et al. (2018). Effect of TiO2 and CeO2 nanoparticles on the metabolic activity of surficial sediment microbial communities based on oxygen microelectrodes and high-throughput sequencing. Water Res. 129, 287–296. doi: 10.1016/j.watres.2017.11.014
Mishra, B., Shoenfelt, E., Yu, Q., Yee, N., Fein, J. B., and Myneni, S. C. B. (2017). Stoichiometry of mercury-thiol complexes on bacterial cell envelopes. Chem. Geol. 464, 137–146. doi: 10.1016/j.chemgeo.2017.02.015
Mitchell, S. L., Hudson-Smith, N. V., Cahill, M. S., Reynolds, B. N., Frand, S. D., Green, C. M., et al. (2019). Chronic exposure to complex metal oxide nanoparticles elicits rapid resistance in Shewanella oneidensis MR-1. Chem. Sci. 10, 9768–9781. doi: 10.1039/c9sc01942a
Mitrano, D. M., Ranville, J., Bednar, A., Kazor, K., Hering, A. S., and Higgins, C. (2014). Tracking dissolution of silver nanoparticles at environmentally relevant concentrations in laboratory, natural and processed waters using single particle ICP-MS (spICP-MS). Environ. Sci. Nano 1, 248–259. doi: 10.1039/c3en00108c
Mitzel, M. R., Sand, S., Whalen, J. K., and Tufenkji, N. (2016). Hydrophobicity of biofilm coatings influences the transport dynamics of polystyrene nanoparticles in biofilm-coated sand. Water Res. 92, 113–120. doi: 10.1016/j.watres.2016.01.026
Mitzel, M. R., and Tufenkji, N. (2014). Transport of Industrial PVP-Stabilized Silver Nanoparticles in Saturated Quartz Sand Coated with Pseudomonas aeruginosa PAO1 Biofilm of Variable Age. Environ. Sci. Technol. 48, 2715–2723. doi: 10.1021/es404598v
Mohanty, A., Kathawala, M. H., Zhang, J., Chen, W. N., Loo, J. S. C., Kjelleberg, S., et al. (2014). Biogenic tellurium nanorods as a novel antivirulence agent inhibiting pyoverdine production in Pseudomonas aeruginosa. Biotechnol. Bioeng. 111, 858–865. doi: 10.1002/bit.25147
Mohanty, A., Liu, Y., Yang, L., and Cao, B. (2015). Extracellular biogenic nanomaterials inhibit pyoverdine production in Pseudomonas aeruginosa: a novel insight into impacts of metal(loid)s on environmental bacteria. Appl. Microbiol. Biotechnol. 99, 1957–1966. doi: 10.1007/s00253-014-6097-5
Monachon, M., Albelda-Berenguer, M., and Joseph, E. (2019). Biological oxidation of iron sulfides. Adv. Appl. Microbiol. 107, 1–27. doi: 10.1016/bs.aambs.2018.12.002
Montano, M. D., Lowry, G. V., von der Kammer, F., Blue, J., and Ranville, J. F. (2014). Current status and future direction for examining engineered nanoparticles in natural systems. Environ. Chem. 11, 351–366. doi: 10.1071/EN14037
Moreau, J. W., Webb, R. I., and Banfield, J. F. (2004). Ultrastructure, aggregation-state, and crystal growth of biogenic nanocrystalline sphalerite and wurtzite. Am. Mineral. 89, 950–960. doi: 10.2138/am-2004-0704
Moreau, J. W., Weber, P. K., Martin, M. C., Gilbert, B., Hutcheon, I. D., and Banfield, J. F. (2007). Extracellular proteins limit the dispersal of biogenic nanoparticles. Science 316, 1600–1603. doi: 10.1126/science.1141064
Morelli, E., Gabellieri, E., Bonomini, A., Tognotti, D., Grassi, G., and Corsi, I. (2018). TiO2 nanoparticles in seawater: aggregation and interactions with the green alga Dunaliella tertiolecta. Ecotoxicol. Environ. Saf. 148, 184–193. doi: 10.1016/j.ecoenv.2017.10.024
Mueller, N. C., and Nowack, B. (2008). Exposure modeling of engineered nanoparticles in the environment. Environ. Sci. Technol. 42, 4447–4453. doi: 10.1021/es7029637
Mugerfeld, I., Law, B. A., Wickham, G. S., and Thompson, D. K. (2009). A putative azoreductase gene is involved in the Shewanella oneidensis response to heavy metal stress. Appl. Microbiol. Biotechnol. 82, 1131–1141. doi: 10.1007/s00253-009-1911-1
Nakata, P. A., and He, C. (2010). Oxalic acid biosynthesis is encoded by an operon in Burkholderia glumae. FEMS Microbiol. Lett. 304, 177–182. doi: 10.1111/j.1574-6968.2010.01895.x
Nancharaiah, Y. V., Dodge, C., Venugopalan, V. P., Narasimhan, S. V., and Francis, A. J. (2010). Immobilization of Cr(VI) and its reduction to Cr(III) phosphate by granular biofilms comprising a mixture of microbes. Appl. Environ. Microbiol. 76, 2433–2438. doi: 10.1128/AEM.02792-09
Navarro, D. A. G., Watson, D. F., Aga, D. S., and Banerjee, S. (2009). Natural organic matter-mediated phase transfer of quantum dots in the aquatic environment. Environ. Sci. Technol. 43, 677–682. doi: 10.1021/es8017623
Nel, A., Xia, T., Mädler, L., and Li, N. (2006). Toxic potential of materials at the nanolevel. Science 311, 622–627. doi: 10.1126/science.1114397
Ngwenya, B. T., Sutherland, I. W., and Kennedy, L. (2003). Comparison of the acid–base behaviour and metal adsorption characteristics of a Gram-negative bacterium with other strains. Appl. Geochem. 18, 527–538. doi: 10.1016/S0883-2927(02)00118-X
Nunan, N. (2017). Game changer in soil science The microbial habitat in soil: scale, heterogeneity and functional consequences. J. Plant Nutr. Soil Sci. 180, 425–429. doi: 10.1002/jpln.201700184
Ouyang, K., Yu, X. Y., Zhu, Y., Gao, C., Huang, Q., and Cai, P. (2017). Effects of humic acid on the interactions between zinc oxide nanoparticles and bacterial biofilms. Environ. Pollut. 231(Pt 1), 1104–1111. doi: 10.1016/j.envpol.2017.07.003
Ozaki, A., Adams, E., Binh, C., Tong, T. Z., Gaillard, J. F., Gray, K. A., et al. (2016). One-time addition of nano-TiO2 triggers short-term responses in benthic bacterial communities in artificial streams. Microb. Ecol. 71, 266–275. doi: 10.1007/s00248-015-0646-z
Palmieri, F., Estoppey, A., House, G. L., Lohberger, A., Bindschedler, S., Chain, P. S. G., et al. (2019). Chapter Two - Oxalic acid, a molecule at the crossroads of bacterial-fungal interactions. Adv. Appl. Microbiol. 106, 49–77. doi: 10.1016/bs.aambs.2018.10.001
Peltier, E., Ilipilla, P., and Fowle, D. (2011). Structure and reactivity of zinc sulfide precipitates formed in the presence of sulfate-reducing bacteria. Appl. Geochem. 26, 1673–1680. doi: 10.1016/j.apgeochem.2011.04.024
Peters, R. J. B., van Bemmel, G., Milani, N. B. L., den Hertog, G. C. T., Undas, A. K., van der Lee, M., et al. (2018). Detection of nanoparticles in Dutch surface waters. Sci. Total Environ. 621, 210–218. doi: 10.1016/j.scitotenv.2017.11.238
Peulen, T. O., and Wilkinson, K. J. (2011). Diffusion of Nanoparticles in a Biofilm. Environ. Sci. Technol. 45, 3367–3373. doi: 10.1021/es103450g
Piccinno, F., Gottschalk, F., Seeger, S., and Nowack, B. (2012). Industrial production quantities and uses of ten engineered nanomaterials in Europe and the world. J. Nanopart. Res. 14:1109. doi: 10.1007/s11051-012-1109-9
Reichard, P., Kretzschmar, R., and Kraemer, S. (2007). Dissolution mechanisms of goethite in the presence of siderophores and organic acids. Geochim. Cosmochim. Acta 71, 5635–5650. doi: 10.1016/j.gca.2006.12.022
Robson, T. C., Braungardt, C. B., Rieuwerts, J., and Worsfold, P. (2014). Cadmium contamination of agricultural soils and crops resulting from sphalerite weathering. Environ. Pollut. 184, 283–289. doi: 10.1016/j.envpol.2013.09.001
Rodrigues, S. M., Trindade, T., Duarte, A. C., Pereira, E., Koopmans, G. F., and Römkens, P. F. A. M. (2016). A framework to measure the availability of engineered nanoparticles in soils: trends in soil tests and analytical tools. Trends Anal. Chem. 75, 129–140. doi: 10.1016/j.trac.2015.07.003
Romeo, A., Vacchina, V., Legros, S., and Doelsch, E. (2014). Zinc fate in animal husbandry systems. Metallomics 6, 1999–2009. doi: 10.1039/C4MT00062E
Saha, R., Saha, N., Donofrio, R. S., and Bestervelt, L. L. (2013). Microbial siderophores: a mini review. J. Basic Microbiol. 53, 303–317. doi: 10.1002/jobm.201100552
Saleh, N. B., Chambers, B., Aich, N., Plazas-Tuttle, J., Phung-Ngoc, H. N., and Kirisits, M. J. (2015). Mechanistic lessons learned from studies of planktonic bacteria with metallic nanomaterials: implications for interactions between nanomaterials and biofilm bacteria. Front. Microbiol. 6:677. doi: 10.3389/fmicb.2015.00677
Santegoeds, C. M., Damgaard, L. R., Hesselink, G., Zopfi, J., Lens, P., Muyzer, G., et al. (1999). Distribution of sulfate-reducing and methanogenic bacteria in anaerobic aggregates determined by microsensor and molecular analyses. Appl. Environ. Microbiol. 65, 4618–4629. doi: 10.1128/aem.65.10.4618-4629.1999
Schlafer, S., Baelum, V., and Dige, I. (2018). Improved pH-ratiometry for the three-dimensional mapping of pH T microenvironments in biofilms under flow conditions. J. Microbiol. Methods 152, 194–200. doi: 10.1016/j.mimet.2018.08.007
Shaw, J. L., and Kennedy, J. H. (1996). The use of aquatic field mesocosm studies in risk assessment. Environ. Toxicol. Chem. 15, 605–607. doi: 10.1002/etc.5620150501
Sivry, Y., Gelabert, A., Cordier, L., Ferrari, R., Lazar, H., Juillot, F., et al. (2014). Behavior and fate of industrial zinc oxide nanoparticles in a carbonate-rich river water. Chemosphere 95, 519–526. doi: 10.1016/j.chemosphere.2013.09.110
Slavin, Y. N., Asnis, J., Hafeli, U. O., and Bach, H. (2017). Metal nanoparticles: understanding the mechanisms behind antibacterial activity. J. Nanobiotechnology 15:65. doi: 10.1186/s12951-017-0308-z
Springer, S. D., and Butler, A. (2016). Microbial ligand coordination: consideration of biological significance. Coord. Chem. Rev. 306(Pt 2), 628–635. doi: 10.1016/j.ccr.2015.03.013
Stegemeier, J. P., Avellan, A., and Lowry, G. V. (2017). Effect of initial speciation of copper- and silver-based nanoparticles on their long-term fate and phytoavailability in freshwater wetland mesocosms. Environ. Sci. Technol. 51, 12114–12122. doi: 10.1021/acs.est.7b02972
Stewart, P. S. (2003). Diffusion in biofilms. J. Bacteriol. 185, 1485–1491. doi: 10.1128/JB.185.5.1485-1491.2003
Sun, T. Y., Bornhöft, N. A., Hungerbühler, K., and Nowack, B. (2016). Dynamic probabilistic modeling of environmental emissions of engineered nanomaterials. Environ. Sci. Technol. 50, 4701–4711. doi: 10.1021/acs.est.5b05828
Sutherland, I. W. (2001). The biofilm matrix – an immobilized but dynamic microbial environment. Trends Microbiol. 9, 222–227. doi: 10.1016/s0966-842x(01)02012-1
Tang, J., Zhu, N., Zhu, Y., Liu, J., Wu, C., Kerr, P., et al. (2017). Responses of periphyton to Fe2O3 nanoparticles: a physiological and ecological basis for defending nanotoxicity. Environ. Sci. Technol. 51, 10797–10805. doi: 10.1021/acs.est.7b02012
Tang, Y., Shigematsu, T., Morimura, S., and Kida, K. (2004). The effects of micro-aeration on the phylogenetic diversity of microorganisms in a thermophilic anaerobic municipal solid-waste digester. Water Res. 38, 2537–2550. doi: 10.1016/S0043-1354(04)00136-8
Tella, M., Auffan, M., Brousset, L., Issartel, J., Kieffer, I., Pailles, C., et al. (2014). Transfer, transformation, and impacts of ceria nanomaterials in aquatic mesocosms simulating a pond ecosystem. Environ. Sci. Technol. 48, 9004–9013. doi: 10.1021/es501641b
Templeton, A. S., Trainor, T. P., Spormann, A. M., and Brown, G. E. Jr. (2003a). Selenium speciation and partitioning within Burkholderia cepacia biofilms formed on α-Al2O3 surfaces. Geochim. Cosmochim. Acta 67, 3547–3557. doi: 10.1016/S0016-7037(03)00212-6
Templeton, A. S., Trainor, T. P., Spormann, A. M., Newville, M., Sutton, S. R., Dohnalkova, A., et al. (2003b). Sorption versus biomineralization of Pb(II) within Burkholderia cepacia biofilms. Environ. Sci. Technol. 37, 300–307. doi: 10.1021/es025972g
Templeton, A. S., Trainor, T. P., Traina, S. J., Spormann, A. M., and Brown, G. E. (2001). Pb(II) distributions at biofilm–metal oxide interfaces. Proc. Natl. Acad. Sci. U.S.A. 98, 11897–11902. doi: 10.1073/pnas.201150998
Thill, A., Zeyons, O., Spalla, O., Chauvat, F., Rose, J., Auffan, M., et al. (2006). Cytotoxicity of CeO2 nanoparticles for Escherichia coli: physico-chemical insight of the cytotoxicity mechanism. Environ. Sci. Technol. 40, 6151–6156. doi: 10.1021/es060999b
Thuptimdang, P., Limpiyakorn, T., and Khan, E. (2017). Dependence of toxicity of silver nanoparticles on Pseudomonas putida biofilm structure. Chemosphere 188, 199–207. doi: 10.1016/j.chemosphere.2017.08.147
Tong, M., Ding, J., Shen, Y., and Zhu, P. (2010). Influence of biofilm on the transport of fullerene (C60) nanoparticles in porous media. Water Res. 44, 1094–1103. doi: 10.1016/j.watres.2009.09.040
Tourney, J., and Ngwenya, B. T. (2014). The role of bacterial extracellular polymeric substances in geomicrobiology. Chem. Geol. 386, 115–132. doi: 10.1016/j.chemgeo.2014.08.011
Tripathi, S., Champagne, D., and Tufenkji, N. (2012). Transport behavior of selected nanoparticles with different surface coatings in granular porous media coated with Pseudomonas aeruginosa biofilm. Environ. Sci. Technol. 46, 6942–6949. doi: 10.1021/es202833k
Unrine, J. M., Colman, B. P., Bone, A. J., Gondikas, A. P., and Matson, C. W. (2012). Biotic and abiotic interactions in aquatic microcosms determine fate and toxicity of Ag nanoparticles, Part 1. Aggregation and Dissolution. Environ. Sci. Technol. 46, 6915–6924. doi: 10.1021/es204682q
Vance, M. E., Kuiken, T., Vejerano, E. P., McGinnis, S. P., Hochella, M. F. Jr., Rejeski, D., et al. (2015). Nanotechnology in the real world: redeveloping the nanomaterial consumer products inventory. Beilstein J. Nanotechnol. 6, 1769–1780. doi: 10.3762/bjnano.6.181
Visca, P., Imperi, F., and Lamont, I. L. (2007). Pyoverdine siderophores: from biogenesis to biosignificance. Trends Microbiol. 15, 22–30. doi: 10.1016/j.tim.2006.11.004
Walden, C., and Zhang, W. (2018). Bioaccumulation of silver nanoparticles in model wastewater biofilms. Environ. Sci. Water Res. Technol. 4, 1163–1171. doi: 10.1039/C8EW00102B
Wang, J.-L., Alasonati, E., Tharaud, M., Gelabert, A., Fisicaro, P., and Benedetti, M. F. (2020). Flow and fate of silver nanoparticles in small French catchments under different land-uses: the first one-year study. Water Res. 176, 115722. doi: 10.1016/j.watres.2020.115722
Wang, X., Liu, B., Pan, X., and Gadd, G. M. (2019). Transport and retention of biogenic selenium nanoparticles in biofilm-coated quartz sand porous media and consequence for elemental mercury immobilization. Sci. Total Environ. 692, 1116–1124. doi: 10.1016/j.scitotenv.2019.07.309
Wang, Y., Gélabert, A., Michel, F. M., Choi, Y., Gescher, J., Ona-Nguema, G., et al. (2016a). Effect of biofilm coatings at metal-oxide/water interfaces I: Pb(II) and Zn(II) partitioning and speciation at Shewanella oneidensis/metal-oxide/water interfaces. Geochim. Cosmochim. Acta 188, 368–392. doi: 10.1016/j.gca.2016.04.052
Wang, Y., Gélabert, A., Michel, F. M., Choi, Y., Peter, E., Spormann, A. M., et al. (2016b). Effect of biofilm coatings at metal-oxide/water interfaces II: competitive sorption between Pb(II) and Zn(II) at Shewanella oneidensis/metal-oxide/water interfaces. Geochim. Cosmochim. Acta 188, 396–406. doi: 10.1016/j.gca.2016.04.054
Wang, Y., and Nowack, B. (2018). Dynamic probabilistic material flow analysis of nano-SiO2, nano iron oxides, nano-CeO2, nano-Al2O3, and quantum dots in seven European regions. Environ. Pollut. 235, 589–601. doi: 10.1016/j.envpol.2018.01.004
Wang, Z., Schenkeveld, W. D., Kraemer, S. M., and Giammar, D. E. (2015). Synergistic effect of reductive and ligand-promoted dissolution of goethite. Environ. Sci. Technol. 49, 7236–7244. doi: 10.1021/acs.est.5b01191
Warren, L. A., and Haack, E. A. (2001). Biogeochemical controls on metal behaviour in freshwater environments. Earth Sci. Rev. 54, 261–320. doi: 10.1016/S0012-8252(01)00032-0
Watt, M., Hugenholtz, P., White, R., and Vinall, K. (2006). Numbers and locations of native bacteria on field-grown wheat roots quantified by fluorescence in situ hybridization (FISH). Environ. Microbiol. 8, 871–884. doi: 10.1111/j.1462-2920.2005.00973.x
Wei, L., Ding, J., Xue, M., Qin, K., Wang, S., Xin, M., et al. (2019). Adsorption mechanism of ZnO and CuO nanoparticles on two typical sludge EPS: effect of nanoparticle diameter and fractional EPS polarity on binding. Chemosphere 214, 210–219. doi: 10.1016/j.chemosphere.2018.09.093
Wirth, S. M., Lowry, G. V., and Tilton, R. D. (2012). Natural organic matter alters biofilm tolerance to silver nanoparticles and dissolved silver. Environ. Sci. Technol. 46, 12687–12696. doi: 10.1021/es301521p
Xia, T., Kovochich, M., Liong, M., Madler, L., Gilbert, B., Shi, H., et al. (2008). Comparison of the mechanism of toxicity of zinc oxide and cerium oxide nanoparticles based on dissolution and oxidative stress properties. ACS Nano 2, 2121–2134. doi: 10.1021/nn800511k
Xiao, Y., and Wiesner, M. R. (2013). Transport and retention of selected engineered nanoparticles by porous media in the presence of a biofilm. Environ. Sci. Technol. 47, 2246–2253. doi: 10.1021/es304501n
Xiao, Y., Zhang, E., Zhang, J., Dai, Y., Yang, Z., Christensen, H. E., et al. (2017). Extracellular polymeric substances are transient media for microbial extracellular electron transfer. Sci. Adv. 3:e1700623. doi: 10.1126/sciadv.1700623
Xu, F. (2018). Review of analytical studies on TiO2 nanoparticles and particle aggregation, coagulation, flocculation, sedimentation, stabilization. Chemosphere 212, 662–677. doi: 10.1016/j.chemosphere.2018.08.108
Xu, J., Murayama, M., Roco, C. M., Veeramani, H., Michel, F. M., Rimstidt, J. D., et al. (2016). Highly-defective nanocrystals of ZnS formed via dissimilatory bacterial sulfate reduction: a comparative study with their abiogenic analogues. Geochim. Cosmochim. Acta 180, 1–14. doi: 10.1016/j.gca.2016.02.007
Yang, S., Liu, F., Wu, C., and Yang, S. (2016). Tuning surface properties of low dimensional materials via strain engineering. Small 12, 4028–4047. doi: 10.1002/smll.201601203
Yee, N., and Fein, J. (2001). Cd adsorption onto bacterial surfaces: a universal adsorption edge? Geochim. Cosmochim. Acta 65, 2037–2042. doi: 10.1016/S0016-7037(01)00587-7
Young, I. M., Crawford, J. W., Nunan, N., Otten, W., and Spiers, A. (2008). Chapter 4 Microbial distribution in soils: physics and scaling. Adv. Agron. 100, 81–121. doi: 10.1016/S0065-2113(08)00604-4
Yu, Q., Szymanowski, J., Myneni, S. C. B., and Fein, J. B. (2014). Characterization of sulfhydryl sites within bacterial cell envelopes using selective site-blocking and potentiometric titrations. Chem. Geol. 373, 50–58. doi: 10.1016/j.chemgeo.2014.02.027
Yu, T., and Bishop, P. L. (2001). Stratification and oxidation–reduction potential change in an aerobic and sulfate-reducing biofilm studied using microelectrodes. Water Environ. Res. 73, 368–373. doi: 10.2175/106143001X139399
Zhou, K., Hu, Y., Zhang, L., Yang, K., and Lin, D. (2016). The role of exopolymeric substances in the bioaccumulation and toxicity of Ag nanoparticles to algae. Sci. Rep. 6:32998. doi: 10.1038/srep32998
Zhu, N., Tang, J., Tang, C., Duan, P., Yao, L., Wu, Y., et al. (2018a). Combined CdS nanoparticles-assisted photocatalysis and periphytic biological processes for nitrate removal. Chem. Eng. J. 353, 237–245. doi: 10.1016/j.cej.2018.07.121
Zhu, N., Wang, S., Tang, C., Duan, P., Yao, L., Tang, J., et al. (2019). Protection mechanisms of Periphytic biofilm to photocatalytic nanoparticle exposure. Environ. Sci. Technol. 53, 1585–1594. doi: 10.1021/acs.est.8b04923
Zhu, N., Wu, Y., Tang, J., Duan, P., Yao, L., Rene, E. R., et al. (2018b). A new concept of promoting nitrate reduction in surface waters: simultaneous supplement of denitrifiers, electron donor pool and electron mediators. Environ. Sci. Technol. 52, 8617–8626. doi: 10.1021/acs.est.8b01605
Zhu, T., Lawler, D. F., Chen, Y., and Lau, B. L. T. (2016). Effects of natural organic matter and sulfidation on the flocculation and filtration of silver nanoparticles. Environ. Sci. Nano 3, 1436–1446. doi: 10.1039/C6EN00266H
Keywords: biofilm, engineered nanoparticles, mesocosm, microenvironment, ZnS, passivation, dissolution
Citation: Desmau M, Carboni A, Le Bars M, Doelsch E, Benedetti MF, Auffan M, Levard C and Gelabert A (2020) How Microbial Biofilms Control the Environmental Fate of Engineered Nanoparticles? Front. Environ. Sci. 8:82. doi: 10.3389/fenvs.2020.00082
Received: 11 April 2020; Accepted: 25 May 2020;
Published: 03 July 2020.
Edited by:
Denise M. Mitrano, Swiss Federal Institute of Aquatic Science and Technology, SwitzerlandCopyright © 2020 Desmau, Carboni, Le Bars, Doelsch, Benedetti, Auffan, Levard and Gelabert. This is an open-access article distributed under the terms of the Creative Commons Attribution License (CC BY). The use, distribution or reproduction in other forums is permitted, provided the original author(s) and the copyright owner(s) are credited and that the original publication in this journal is cited, in accordance with accepted academic practice. No use, distribution or reproduction is permitted which does not comply with these terms.
*Correspondence: Alexandre Gelabert, gelabert@ipgp.fr