- 1Environmental Geosciences, University of Basel, Basel, Switzerland
- 2Agroscope, Zürich, Switzerland
Peatland degradation impairs soil functions such as carbon storage and the existence of biodiversity hotspots. Therefore, and in view of the ongoing climate change, an efficient method of evaluating peatland hydrology and the success of restoration efforts is needed. To understand the role of microbial groups in biogeochemical cycling, gaseous loss and isotopic fractionation that lead to specific isotopic depth patterns (δ13C, δ15N), we integrated previously published stable isotope data with a membrane fatty acid (mFA) analysis related to various microbial groups that are known to be common in peatlands. We performed two sampling campaigns to verify the observed stable isotope depth trends in nutrient-poor peatlands in Northern Europe. Cores were taken from adjacent drained (or rewetted) and undrained sites. Fungal-derived mFA abundance was highest in the uppermost part of the drained layer. We found increasing bacterial-derived mFA concentrations with depth peaking in the middle of the drained layers, which correlates with a δ15N peak of bulk material. The results support our hypothesis that changing peatland hydrology induce a shift in microbial community and metabolism processes and is therefore also imprinted in stable isotope values. Under waterlogged conditions overall levels of microbial-derived mFAs were generally low. Drained layers showed simultaneous changes in microbial abundance and composition and depth trends in stable isotope bulk values. Bacteria, particularly acidobacteria, can be expected to dominate increased denitrification with low oxygen saturation accompanied by increased δ15N bulk values in the remaining substrate. Interestingly, cores from recent rewetted peatlands show no depth trend of δ15N in the layers grown under rewetting conditions; this is congruent with relatively low concentrations of microbial-derived mFAs. Hence, we conclude that stable isotopes, especially δ15N values, reflect changing microbial metabolic processes, which differ between drained and undrained - and especially also for recent rewetted–peatlands. As today stable isotope measurements are routine measurements, these findings enable us to get cost- and time efficient reliable information of drainage and restoration success.
Introduction
A unique biodiversity, slow rates of decomposition and the storage of significant quantities of carbon characterize wetland soils; this is especially true for nutrient-poor peatlands (Moore and Basiliko, 2006). The protection of biodiversity and successful peatland restoration could save 1.91 (0.31–3.38) gigatons (Gt) of CO2-equivalent greenhouse gas emissions (Leifeld and Menichetti, 2018). Furthermore, 6% of the greenhouse effect is contributed by N2O Schulze et al. (2009), which is also released by degraded peatlands due to impaired soil functioning (Palmer and Horn, 2015). For other ecosystems, microbial communities and their major role in biochemical cycling of carbon and nitrogen in soil are well documented, but little is known of the microbial community and its function in peatlands (Elliott et al., 2015). In particular, information about microbial community structures in different layers and their influence on biochemical processes under rewetting conditions is widely unknown (Elliott et al., 2015). Thus, more reliable information about peatland degradation and restoration success is needed.
Peat soil can be divided into three layers. In the uppermost part, the acrotelm, most biological metabolism and nutrient cycling takes place (Asada et al., 2005; Artz, 2013). In the lower layer, the anaerobic, water-saturated catotelm, microbial metabolism is suppressed due to the lack of oxygen (Asada et al., 2005; Artz, 2013; Lin et al., 2014). In between, the mesotelm is characterized by a fluctuating water table and facultative anaerobic conditions, which therefore leads to shifting levels of microbial abundance and activity (Asada et al., 2005; Artz, 2013; Lin et al., 2014). Drainage of peatlands expands the mesotelm, wherein formerly preserved organic substrates can be decomposed (Zedler and Kercher, 2005). If rewetting occurs, the former mesotelm will become anaerobic again, and aerobic microbial activity will be inhibited (Asada et al., 2005; Andersen et al., 2010).
Whereas microbial metabolism of carbon is discussed in several papers (see review of Blodau (2002)), nitrogen cycling in peatlands is less well studied. Nitrogen fixation has been reported to only occur in surface layers of peat, (Lin et al., 2014). However, the N2O producing microbial mediated nitrification and denitrification of organic matter as well as other chemical processes occur also in deeper layers (Bremner, 1997; Palmer and Horn, 2015). For peatlands, Palmer et al. (2010) report denitrification of organic matter as the main N2O source. Denitrification causes a reduction of nitrate and nitrite by converting them to nitric oxide (NO) and N2O and, ultimately, to dinitrogen (N2; Novák et al., 1999). Especially for the deep, anaerobic layer, Lin et al. (2014) reported extremely low values for denitrification and other N-cycling processes and, therefore, a conservation of the substrate.
Stable isotopes of carbon and nitrogen are known indicators of peatland hydrology (Alewell et al., 2011; Krüger et al., 2016; Groß-Schmölders et al., 2020; Kohl et al., 2015). For δ13C, Alewell et al. (2011), Krüger et al. (2016), Novak et al. (1999), Hobbie et al. (2017) and Biester et al. (2014) report an enrichment of 13C with depth due to an increasing degree of organic matter decomposition. Substrates have a natural and specific range of 13C values (Lerch et al., 2011). As lignin, cellulose and lipids are known to be depleted in 13C, glucose, amino acids, pectin and hemicellulose are enriched in 13C (Lerch et al., 2011). In undrained wetlands, the combination of these substrates is mostly preserved due to the waterlogged conditions. If drainage takes place, the original bulk soil δ13C value is changed by degradation and microbial metabolism processes. Kohl et al. (2015) state that an increasing δ13C depth trend is a consequence of a switch in dominant microbial decomposition, which has stronger effects than the residual enrichment of recalcitrant compounds such as lignin. Kohl et al. (2015) stated out, that fungi are main decomposer in the uppermost soil horizons and bacteria are more prominent in deeper horizons. With this switch of dominant microbial groups, also the decomposed material switches and therefore the 13C bulk values change.
Additionally, a positive correlation between increased microbial metabolism and δ15N was previously presented (Gro-Schmölders et al., 2020). Fractionation of stable isotopes during microbial metabolism of nitrate and ammonium occurs, since most organisms prefer the lighter and more frequently occurring 14N (Kohzu et al., 2003; Robinson et al., 1998). As a result, plants in particular incorporate and translocate the lighter 14N upwards to stem and foliar, which leads to an enrichment of heavier 15N in the remaining bulk material (Högberg et al., 1996). Additionally, the mycorrhizal uptake of lighter 14N into plants increases the δ15N values of bulk material (Hobbie and Högberg, 2012). Furthermore, with ongoing microbial metabolic processes in peat, the δ15N values increase as long as microbial metabolism occurs, and lighter 14N will be leached, translocated or lost via outgassing (Novák et al., 1999; Damman, 1988; Niemen, 1998). In 2010, Goldberg et al. (2010) showed, that increasing oxygen concentrations in drained fens leads to higher N2O release by nitrification, which is followed by increasing δ15N values in the remaining substrate. Thus, microbial abundance and stable isotope ratios are closely linked, especially for some microbial groups that are more active in nitrogen cycling than others and therefore play a greater role (Tfaily et al., 2014). Fungi have a low demand for nitrogen, making them less likely to be a main driver of increasing δ15N values (Thormann, 2005). In contrast, acidobacteria are one of the main bacterial groups in peat and are highly active in nitrogen cycling; in particular, they are involved in denitrification and N fixation (Ward et al., 2009). Accordingly, their abundance can be expected to have a close link to carbon and especially nitrogen stable isotope depth trends (Weijer et al., 2010).
To examine microbial abundance, we measured the concentrations of specific membrane fatty acids (mFAs). Membrane fatty acids are valid markers to indicate the abundance of specific microbial communities. Sundh et al. (1997) and Torres and Pancost (2016) demonstrated that mFAs are persistent and, to a high degree, insoluble compounds in peat soil. Membrane fatty acids vary based on their origin (plants, specific microbial groups, etc.; Bajerski et al., 2017; Finotti et al., 1993; Piotrowska-Seget and Mrozik, 2003; Reiffarth et al., 2016; Willers et al., 2015). Therefore, based on an analysis of the quantity of mFAs present, the relative abundance of certain microbial communities might be assessed (Piotrowska-Seget and Mrozik, 2003; Torres and Pancost, 2016). We tested the existence of four bacterial markers and one fungal marker:
- i-C15:0 and C16:1ω7c, which, in combination, are indicative of acidobacteria (Damast et al., 2011; Dedysh and Damsté, 2018; Myers and King, 2016);
- C14:0 and C17:0, which are generally indicative of bacteria (Willers et al., 2015; Zelles, 1997); and
- C18:2ω6c, which is indicative of saprotrophic fungi (Sundh et al., 1997; Elvert et al., 2003; Willers et al., 2015).
To differentiate between wetland soil functioning as carbon storage and biodiversity hotspots in undrained, rewetted and drained sites, we investigate the influence of drainage and rewetting on microbial-derived mFA abundance and stable isotopic values. We studied two peatlands with different drainage histories, using a high spatial resolution of 4 cm in the uppermost 50 cm of the peat columns. In both investigated peatlands’ ditches were installed to drain the sites for agricultural use (Mikkenen et al.m, 1999; Nielsson et al., 2008). In Lakkasuo, Southern Finland, we located a site that had experienced continuous drainage since 1961 (Minkkinen et al., 1999). In the Swedish Degerö Stormyr, ditches were installed at the beginning of the 20th century (Nielsson et al., 2008). They have filled up naturally with sphagnum over the last 20 years and sites are thus rewetting. We compared undrained with drained sites in Lakkasuo and undrained with rewetted sites in Degerö Stormyr. Furthermore, to verify our previous results regarding stable isotopes as markers for peatland hydrology and drainage history (Minkkinen et al., 1999), we investigated depth trends at two points in time (2013 and 2017) to verify pattern stability over time.
We define a sudden directional change in the stable isotope depth patterns as “turning points,” according to Alewell et al. (2011) and Groß-Schmölders et al. (2020). The δ15N turning point is located in the middle of the mesotelm, where δ15N values are highest. In contrast, the δ13C turning point marks the bottom of the mesotelm and the onset of the underlying catotelm, above which the δ13C values start to decrease continuously up to the surface.
The contribution of this paper is to examine the microbial composition of peat soil with respect to stable isotope fractionation and test the persistence of stable isotope depth trends with a repeated sampling approach.
We hypothesize the following:
- Bacterial abundance, especially that of acidobacteria, is highest in the mesotelm.
- Bacterial abundance is the main driver of the nitrogen stable isotope depth trend in nutrient-poor peatlands.
- Stable isotope depth patterns are persistent over a time span of four years (2013–2017) and are therefore reliable indicators of drainage and rewetting.
Materials and Methods
Site Description
We investigated two nutrient-poor peatlands in northern Europe, both classified as fibric Histosol (HSf; IUSS, 2015; Table 1).

TABLE 1. Detailed information for the acrotelm/former mesotelm (only for Degerö rewetted) of the drained, rewetted and undrained sites of Degerö Stormyr and Lakkasuo at the surface (Nielsson et al., 2008; Mikkenen et al., 1999; Groß-Schmölders et al., 2020); av.: average, WT: water table below surface, C: carbon, N: nitrogen, CN: carbon:nitrogen ratio, BD bulk density [k m−3], von Post Indices (vP).
Degerö Stormyr (64°11′lat. 19°33′long.; 200 m above sea level (a.s.l.)) is situated in Northern Sweden, at the Kulbäcksliden Experimental Forest near Vindeln, between the rivers Umeälven and Vindelälven (Eurola et al., 1984). It is an acidic bog and consists of interconnected small mire patches divided by ridges of glacial till. The climate is characterized as cold with no dry seasons and cold summers (Dfc-zone after Köppen-Geiger classification; Peel et al., 2007). Mean annual temperature is +1.2°C and the annual precipitation has an average of 523 mm (Alexandersson et al., 1991). Ditches were installed in Degerö in the beginning of the 20th century but a natural reestablishment of sphagnum took place over the last few decades (>20 years). Therefore, we define this site as rewetted. In the undrained part the main moss species is Sphagnum majusNielsson et al. (2008) and the water table is near the surface (Table 1). The humification index (HI) after von Post is low (H1-H2) and macro residuals are highly visible (Table 1; Groß-Schmölders et al., 2020). Also, biochemical parameters indicate undrained conditions. The carbon:nitrogen ratio (CN) in the acrotelm is 89, and the bulk density (BD) is low (0.02 kg m−3), both is typical for undrained nutrient poor peatlands (Table 1; Groß-Schmölders et al., 2020). For the rewetted site the main moss species is Sphagnum balticum (Nielsson et al., 2008). The water table is near the surface, HI is low (H2) and macro-residuals are preserved well in the upper horizon (Table 1; Groß-Schmölders et al., 2020). In contrast the values of the former mesotelm indicate degradation: HI is higher (H3), less macro-residuals are visible, CN decreased to 41 and the BD increased (0.06 kg m−3; Table 1; Groß-Schmölders et al., 2020).
Lakkasuo (150 m a.s.l.) in Central Finland is an eccentric peatland complex with two parts. The southern part is a bog with ombrotrophic conditions; whereas the northern part is a fen (Minkkinen et al., 1999). Only samples of the ombrotrophic bog are included for this study. Lakkasuo is also located in the cold climate zone, with no dry seasons and cold summers (Dfc-zone after Köppen-Geiger classification; Peel et al., 2007). Mean annual temperature is +3°C and the average precipitation is 700 mm (Laine et al., 2004). Lakkasuo is still drained. Ditches installed in 1961 (70 cm depth, spacing of 40–60 m) affected approximately 50% of the peatland (Minkkinen et al., 1999). The main current moss species in undrained sites is Sphagnum angustifolia (Laine et al., 2004). HI is low (H2), a high number of macro-residuals is visible, and the water table is near the surface (<5 cm) (Table 1; Groß-Schmölders et al., 2020). Also, the biochemical parameters indicate undrained conditions: high CN (66) and low BD (0.02 kg m−3; Table 1; Groß-Schmölders et al., 2020). In the drained site Pleurozium spp., a moss species of drier environments, is the main moss species and a high number of pine trees is abundant. Macro-residuals are strongly affected by decomposition, HI (H3-H4) and BD (0.06 kg m−3) are high and CN (44) is low (Table 1; Groß-Schmölders et al., 2020).
Soil Sampling and Bulk Analyses
We drilled three volumetric peat cores per site in September 2013 and one in June 2017. Cores were drilled with a Russian peat corer (Eijkelkamp, Netherlands). Per site three Cores were taken (1–3). Cores were taken in the undrained parts (Degerö Stormyr (DU131-3, DU17); Lakkasuo (LU131-3, LU17)), and in 1-m distance to a drainage ditch (to 1-m depth) (Degerö Stormyr rewetted (DR131-3, DR17); Lakkasuo drained (LD131-3, LD17)). We drilled the cores in 2017 close to the location of 2013, with a GPS accuracy of 1.3 m, to monitor possible hydrology changes, which could be mirrored by stable isotope depth patterns and microbial FA abundance.
The cores were encased in hard plastic shells, stored in coolers, and transported to the laboratory. The cores were sliced in 2 cm sections for the isotope analyses. Every second section was analyzed, giving a 4 cm depth resolution. Samples were oven-dried at 40°C for 72 h, and homogenized with a vibrating ball mill (MM400, Retsch, Germany). Stable C and N isotopic signatures were measured an elemental analyzer combined with an isotope ratio mass spectrometer (EA-IRMS) (Inegra2, Sercon, Crewe, United Kingdom). Carbon isotopic composition (13C/12C) was expressed relative to Vienna Pee-Dee Belemnite (VPDB) standard and reported in delta notation (‰):
Rs and Rstd are the ratios of 13C/12C in the sample and VPDB standard (Rstd = 0.011182).
Nitrogen stable isotopes were expressed relative to atmospheric nitrogen and reported in delta notation (‰):
Rs and Rstd are the ratios of 15N/14N in the sample and atmospheric nitrogen (Rair = 0.0).
Fatty Acid Analyses
We aimed to extract total membrane FAs to distinguish between FAs of different bacterial groups, fungi, and plants. We processed 0.2–1.1 g of sample for the lipid extraction with a mixture of CH2Cl2: MeOH (9:1 ν/ν) in an Accelerated Solvent Extractor (Dionex ASE 350). 0.1 μg/ml of an internal standard with nonadecanoic acid was added before processing each sample.
The total lipid extracts (TLE) were saponified by adding 2 ml of KOH dissolved in MeOH (12%) and putting it in the oven for 3 h at 80°C.
Following the method of Elvert et al. (2003), the TLE were polarized with 1 ml KCl (0.1 mol), and the neutral fraction was extracted by rinsing three times with hexane, dried under a stream of N2, and stored in the refrigerator for later analysis. We acidified the rest of the TLE with fuming hydrochloric acid to a pH of 1. The acid fraction was extracted by rinsing again three times with hexane dried under a stream of N2. They were methylated by adding 1 ml Boron-Trifluoride (BF3) in MeOH (12–14%) and putting it in the oven for 1 h at 60°C. Afterwards the FA fraction was polarized with KCl (0.1 mol) and transferred in 4 ml vials by rinsing three times with hexane. After one day, in which residues could settle, we transferred the upper part with hexane in 2 ml vials to measure the FAs. The FAs were quantified with a Trace Ultra gas chromatograph (GC) equipped with a flame ionization detector (FID) (Thermo Scientific, Waltham, MA, United States). The carrier gas (helium) had a constant flow of 1.2 ml per minute and the GC-FID was set to splitless mode. Detector temperature was set to 320°C and the samples (dissolved in hexane) were injected at 300°C injector temperature. The starting temperature of the oven was 50°C and hold for 2 min. Then temperature was increased by 10°C per minute to 140°C. The temperature was held for 1 min before it was increased up to 300°C. This temperature was held for 63 min.
To identify the fungal and bacterial markers, we used the Bacterial Acid Methyl Esters standard (BAME, Supelco Mix). For bacteria, it includes the FAs C14:0 and C17:0 as general bacterial markers Zelles (1997), Willers et al. (2015)), i-C15:0 and C16:1ω7c for acidobacteria (Damasté et al., 2011; Dedysh and Damsté, 2018; Myers and King, 2016). The fatty acid C18:2ω9c was used as a marker for fungi (Andersen et al., 2010; Sundh et al., 1997; Zelles, 1997; O`Leary and Wilkinson, 1988; Vestal and White, 1989). All these markers are valid for overall membrane fatty acids and can be used to detect different microbial groups in soil (Finotti et al., 1993; Piotrowska-Seget and Mrozik, 2003; Bajerski et al., 2017). Quantification of the FAs was done using the injected internal standard C19:0 FA, after correcting for the methyl group, added during methylation reaction, normalized to dry weight of bulk material.
Statistical Analysis
For the FA analysis and the comparison of spatial variations for drained vs rewetted sites, we were interested in comparing the depth trends of all single profiles with each other. This was done by using the depth of the δ15N turning point in each drained profile as the anchor point serving as normalized depth (normD) and set them to 17 cm depth (normD = 17 cm) in each single core. Using the same procedure, δ13C trends were normalized using the same anchor point (e.g., δ15N turning point) (for more detailed information, please see Groß-Schmölders et al., 2020).
For the cores of 2013 (3 replicates), variance, standard deviations, and spearman correlation coefficient (R) were calculated with the software R (version 1.0.153). We define an R-value above 0.4 as a strong correlation following McGrew and Monroe (2000) and define significance with p < 0.05 (McCune and Grace, 2002).
In the following we present only the normalized data. Raw data without normalization are available in the Supplementary Table.
Results
Microbial-Derived FA Quantities
Microbial-derived mFA abundance was found to be low over the whole profile in undrained sites, with an average of 16.05 μg/g (±7.4) in Lakkasuo (undrained; LU) and 14.74 μg/g (±4.7) in Degerö Stormyr (undrained; DU). In the acrotelm, the quantity is higher (LU: 21.8 μg/g; DU: 27.7 μg/g) than in the catotelm (LU: 13.5 μg/g, ±4.4; DU: 13.28 μg/g, 3.4; Figure 1).
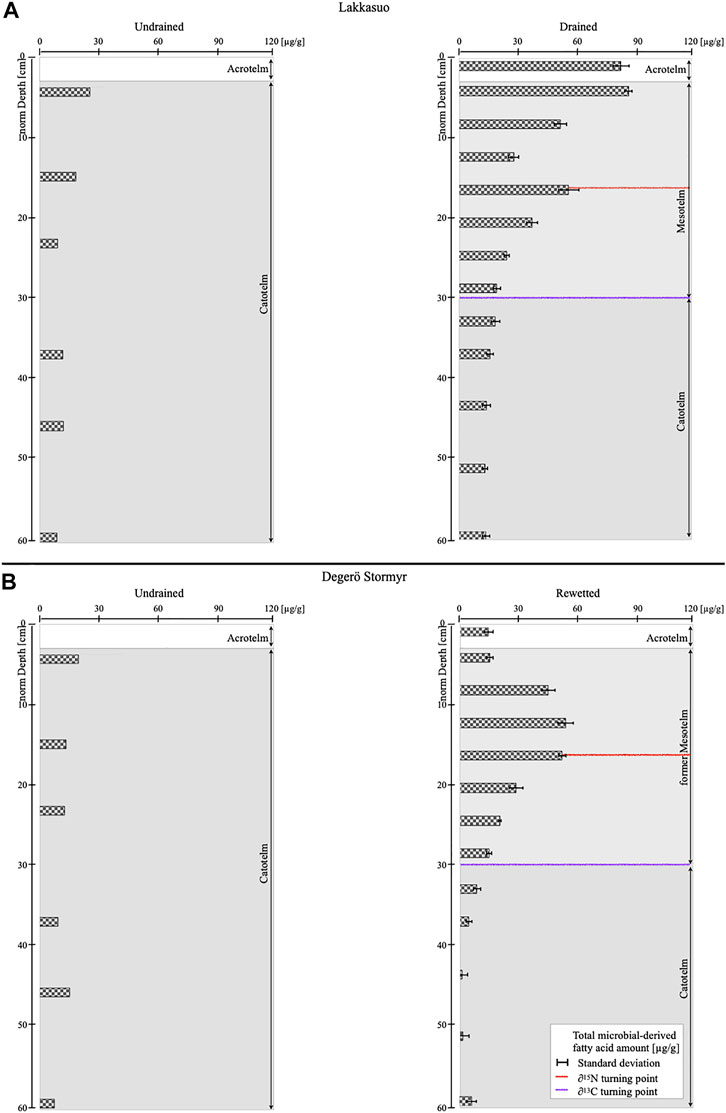
FIGURE 1. Average values of total microbial-derived membrane fatty acid concentrations [µg/g] (A) Lakkasuo: undrained (LU1-3) and drained (LD131-3) (B) Degerö Stormyr: undrained (DU1-3) and rewetted (DR131-3); Red reference line gives the δ15N turning point, violet reference line gives the δ13C turning point.
In the drained site at Lakkasuo (LD), we discovered a large quantity of microbial-derived mFAs in the acrotelm {LD131–3: 108.7 μg/g [mean, ±5.5 (standard deviation of n = 3]; Figure 1) and the mesotelm [62.27 μg/g (±3.7)]; all results from the 2013 sampling}. The highest microbial-derived mFA concentration was found at the δ15N turning point (73.73 μg/g, ±6.74; Figure 1). In the catotelm, the values of mFAs were low in the LD sites (21.01 μg/g, ±2.4). The concentration of fungal-derived mFAs is decreasing from 40.33 μg/g (±11.7; Figure 2) in the acrotelm of LD131–3, to very low values at the end of the mesotelm and in the catotelm (2.88 μg/g, ±1.4 μg/g; Figure 2). Also, the percent of fungal-derived mFAs are decreasing from 49% of all microbial-derived mFAs in the acrotelm to 14% in the catotelm. Contrary to the continually decreasing trend in depth of the fungal-derived mFAs under drained conditions, the bacterial-derived mFA concentration is highest in the middle of the mesotelm and peaks parallel to the δ15N turning point (mean 60.17 μg/g, ±10.2; Figure 2). Bacterial-derived mFAs comprise up to 85% of total microbial-derived mFAs at this depth.
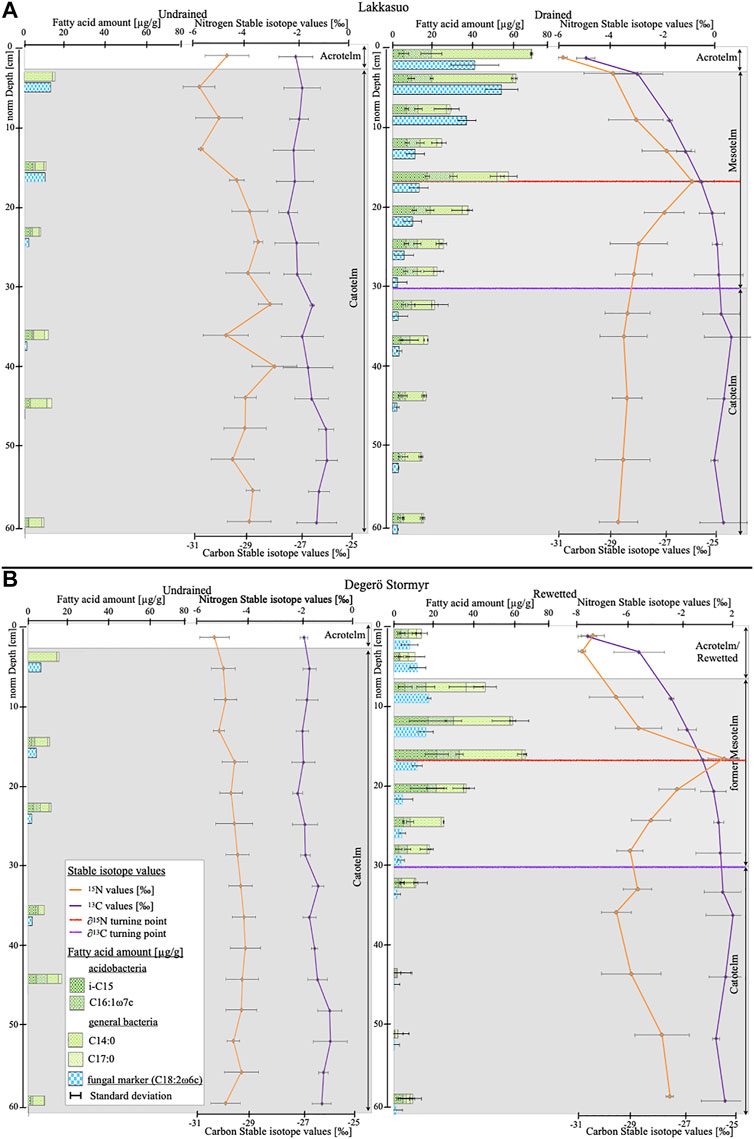
FIGURE 2. Stable isotope depth trends [‰] (orange: δ15N, violet: δ13C) and fatty acids marker concentrations [µg/g], separated by different microbial groups (A) Lakkasuo: undrained (LU1-3) and drained (LD131-3) (B) Degerö Stormyr: undrained (DU1-3) and rewetted (DR131-3); Red reference line gives the δ15N turning point, violet reference line gives the δ13C turning point.
For the rewetted site in Degerö Stormyr (DR131-3), we detected low values of microbial-derived mFAs in the acrotelm and in the uppermost part of the former mesotelm [20.61 μg/g (±2.6)]. This is expected, because of the wet conditions which are not suitable for high microbial abundance. Below, in the deeper part of the former mesotelm microbial-derived mFA quantities increases [53.44 μg/g (±2.93)]. As in Lakkasuo, the microbial-derived mFA quantity also peaks at the δ15N turning point in the middle of the formerly drained layer (71.55 μg/g, ±2.48; Figure 1). In the below catotelm, values were low for DR sites (8.80 μg/g, ±2.7; Figure 1).
In the rewetted cores DR131-3, we found that fungal-derived mFAs have the highest percentage of the mFAs detected in the acrotelm (35%) and in the upper part of the former mesotelm (32%, Figure 3). The highest total quantity of fungal-derived mFAs is in the upper mesotelm (16.75 μg/g, ±3.82; Figure 2). In the catotelm, the percentage (16%) and the total quantity of fungal-derived mFAs (2.42 μg/g, ±2.17; Figure 2/3) is low. With decreasing fungal-derived mFA percentage, the bacterial-derived mFA percentage increases, from 65% in the acrotelm to 84% in the catotelm (Figure 3). Overall, bacterial-derived mFA abundance is highest at the δ15N turning point (62.15 μg/g, ±5) and low in the catotelm (7.45 μg/g, ±3; Figure 2).
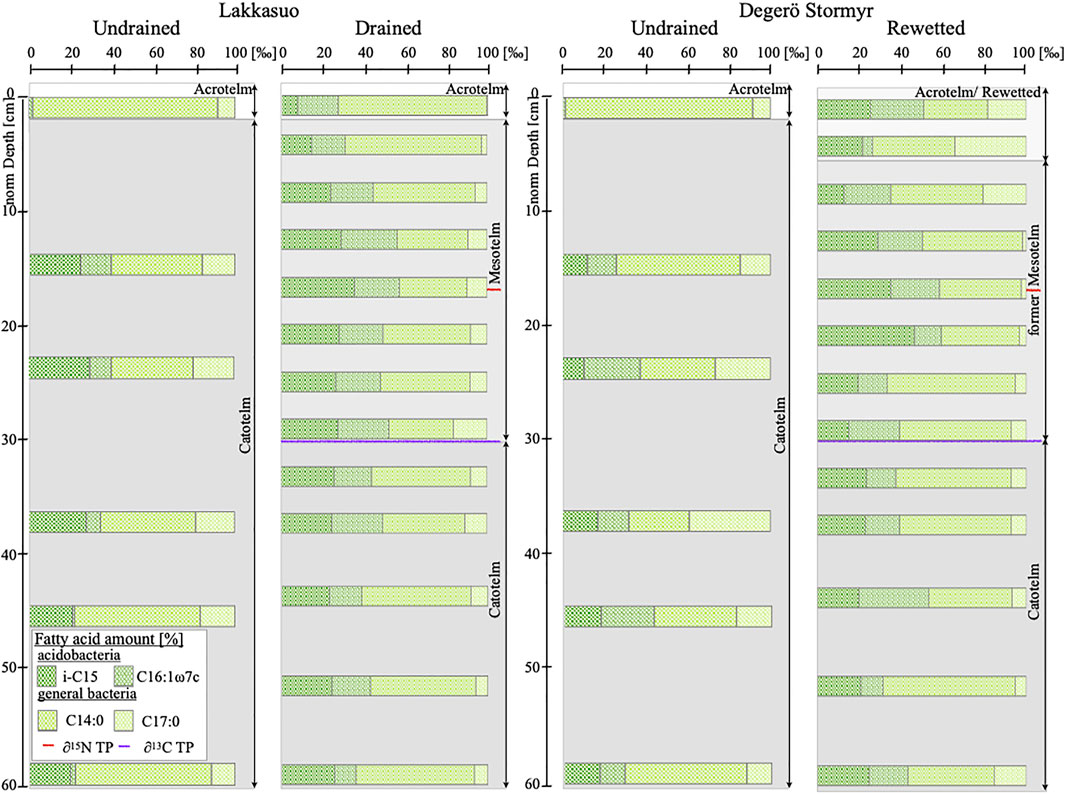
FIGURE 3. Relative amount of bacterial-derived membrane fatty acids, separated for general and acidobacterial markers Lakkasuo (undrained, drained) and Degerö Stormyr (undrained, rewetted) in the cores of 2013; Red reference line gives the δ15N turning point (TP; Statistical analysis), violet reference line gives the δ13C TP Statistical analysis).
Stable Isotope Values
Carbon and nitrogen isotope values in undrained sites in Lakkasuo (LU131–3, LU17) and Degerö Stormyr (DU131–3, DU17) show no depth trend, whereas carbon isotope values vary less than nitrogen stable isotope values (Figure 2).
In LU131-3 and LU17, carbon isotope values range between −24.47‰ and −28.36‰ with a mean of −26.43‰ (±0.85). Nitrogen stable isotope values in these cores are between 0.51‰ and −6.56‰ (mean −3.15‰, ±1.78).
In DU131–3 and DU17, carbon stable isotope values are between −22.84‰ and −27.38‰ (mean −24.52‰, ±0.94). For nitrogen, the values range between −0.71‰ and −9.31‰ (mean −3.99, ±2.27).
In the drained site in Lakkasuo (LD131–3, LD17) and the rewetted site in Degerö Stormyr (DR131–3, DR17), values show a distinct depth pattern and vary more than in undrained sites (Figure 2). In both sites (LD and DR), carbon stable isotope values show a decreasing trend in the upper layers (above 30 cm normD, Figure 2). In LD131–3, carbon isotope values range between −24.53‰ and −31.04‰ (mean -26.67‰, ±1.23), and in DR131–3, between −23.30‰ and −29.74‰ (mean −26.33, ±1.33).
Nitrogen values in drained and rewetted sites show a peak in the mesotelm (17 cm normD, Figure 2). In LD131–3, δ15N values are between 0.94‰ and −5.73‰ (mean 2.40, ±1.50). In DR131-3, δ15N values are between 1.83‰ and −10.64‰ (mean −3.42, ±2.38).
Discussion
New Insights to Microbial Abundance in Undrained, Rewetted and Drained Sites, Identified by Membrane Fatty Acids
In the undrained sites LU and DU, microbial-derived mFA concentrations were highest in the acrotelm, which is in line with other studies (Asada et al., 2005; Artz, 2013). It is the result of environmental conditions in this layer (aerobic, rich of primary plant material). But the values of the acrotelm in the undrained sites are low compared to those of the drained sites, which is a sign of the intact carbon storage function of peat soils and could be the result of 1) the overall nutrient-poor conditions of the investigated sites Minick et al. (2019) with a reduced quantity of nutrients and 2) the incorporation of C in living vegetation (Artz et al., 2008; Figure 1).
The highest microbial-derived mFA concentration discovered in the drained site of Lakkasuo is in line with our previous study Groß-Schmölders et al. (2020) and Peltoniemi et al. (2009), as the facultative aerobic mesotelm, with its high content of available organic matter, provides optimal conditions for microbial metabolism and thus contains the highest microbial-derived mFA concentrations (Figure 1). This is also in line with the findings of Wang et al. (2019), who showed that the mesotelm is a hot spot for microbial communities, with the highest microbial diversity.
For the rewetted site in Degerö Stormyr, we assumed that the highest mFA concentration in the former mesotelm (the newly established catotelm after rewetting; Figure 1) could be the result of former microbial metabolism preserved from the past aerobic conditions that occurred due to drainage. This correlation was also reported by Torres and Pancost (2016). The low microbial-derived mFA quantities in the uppermost part of DR1–3, similar to concentrations of DU (Figure 1) are likely a result of the recovery of undrained conditions.
Regarding microbial community assemblage, our results are congruent with previous studies (Groß-Schmölders et al. (2020), Thormann (2004)) demonstrating that fungal-derived mFA concentrations are dominant in the upper layers of drained sites (LD, Figure 2). This is congruent with the ecological niche for fungi being located near the surface, where there are large quantities of primary plant material and aerobic conditions (Thormann et al., 2004; Wallander et al., 2009; Strickland and Rousk, 2010). Here, fungal metabolism has a competitive advantage (De Boer et al., 2005; Thormann et al., 2003; Thormann, 2011).
In contrast to fungi, bacteria are better adapted to the facultative anaerobic conditions lower in the profile, (Winsborough and Basiliko, 2010). They are able to make use of a wider spectrum of substrates, which leads to an increase in ratios of bacterial-derived mFAs as depth increases within the mesotelm (Figure 2; Kohl et al., 2015).
The group of acidobacteria is of special interest here, as acidobacteria are highly abundant in soil, especially in peatlands (Hausmann et al., 2018; Damsté et al., 2015). They comprise 30% of all bacteria in nutrient-rich fens and up to 80% in pristine bogs (Serkebaeva et al., 2013). Acidobacteria are known to have a slow growth rate and are tolerant to depleted sites, which supports their abundance in nutrient-poor peatlands (Wang et al., 2019). Because of their capability to metabolize in facultative anaerobic to anaerobic conditions, acidobacteria are particularly visible in the mesotelm (Urbanova and Barta, 2014). Acidobacteria are always Gram-negative and exhibit a group of specific membrane compounds (Dedysh and Damsté, 2018). In particular acidobacteria mainly produce glycerol dialkyl glycerol tetraethers Weijers et al. (2010) and the membrane lipids i-C15:0, C16:1ω7c and 13,16-dimethyl octacosanedioic acid (Damsté et al., 2015; Damsté et al., 2018). The concentrations of these were linked to low pH values and decreasing oxygen availability (Weijers et al., 2010), which are typical conditions of the mesotelm. Our results are in line with these findings, as we found an increasing abundance of the mFAs i-C15:0 and C16:1ω7c near the δ15N turning point (Figures 2, 3).
Microbial-Derived Membrane Fatty Acid Quantities and Isotopic Values
In undrained sites, we saw no depth trend in the isotopic values compared to the strong decrease of microbial-derived mFA concentration in sublayers (Figure 2). We conclude that this is caused by the extreme environment of the catotelm, with low temperatures and anaerobic conditions. Hence, metabolism and decomposition processes are strongly inhibited, the mFA quantities and the isotopic fractionation rates are extremely low, and organic substrates are stored (Clymo, 1984; Frolking et al., 2001; Krüger et al., 2015).
Microbial-Derived Membrane Fatty Acid Quantities and Carbon Isotopic Values
Carbon stable isotope values increase with depth in all investigated drained sites by ∼2.48‰ from the acrotelm to the catotelm (Figure 2). This increase was also found previously Alewell et al. (2011), Krüger et al. (2014) and is in the same range of what other studies have found for peatland soils (Kohl et al., 2015; Hobbie et al., 2017). Wynn et al. (2006) reported, that this trend is caused by the microbial communities involved and their preference for substrates. If more enriched substrates are degraded, the δ13C values in the remaining substrate could decrease due to the enhanced gaseous loss of 13C enriched CO2 (Wynn et al., 2006). For example, glucose, pectin and hemicellulose, which are enriched in 13C, are some of the preferred substrates for microbial metabolism and are processed in the uppermost peat soil layers. As a result, δ13C values are low in the uppermost peat layers of degraded sites, as we see in LD (Figure 2). In contrast to the enriched δ13C values of CO2, methane (CH4) is depleted in δ13C, which could balance the effect of enriched CO2 in the remaining substrate. But as methane production mainly occurs in anerobic conditions, the effect of gaseous loss of depleted CH4 is expected to play a minor role in drained peatlands (Yang et al., 2019). In addition, Hornibrook et al. (1997) reported that the δ13C values of CH4 are increasing with decreasing depth in peatlands, which could also minor the effect of depleted CH4 in our sites. That the depleted δ13C values in top layers of peat are caused by the preferred cycling and therefore gaseous loss of heavy 13C isotopes can be verified by studies that reported that there is no preferred loss of lighter 12C during microbial metabolism (Lerch et al., 2011). Increasing values of δ13C in peat soil with depth are therefore related to a change in the processed substrates and their specific δ13C values (Kohl et al., 2015). With increasing depth, recalcitrant, δ13C-depleted substrates such as lignin are also processed, which leads to increasing mobilization of lighter 12C and further increasing δ13C values in the remaining bulk soil with depth (Lerch et al., 2011). Further Boström et al. (2007) assume that the 13C enrichment in drained soils with depth is a result of the increased contribution of microbial derived C with depth.
With regard to specific microbial groups, Kohl et al. reported in 2015 that a high fungal-to-bacterial ratio in the microbial community is negatively correlated with δ13C values in the remaining substrate; this is caused by changes in processed substrates and their carbon isotopic signals. Hence, fungal-to-bacterial ratio is decreasing, and δ13C values are increasing due to a change in microbial metabolism processes and substrates used (Kohl et al., 2015). As Kohl et al. (2015) showed, the δ13C values of bacteria (−40.1 to −30.6‰) and fungi (−31.1 to −24.6‰) stay the same in different depths of peat, but their ratios are changing, and with them, the δ13C values of the bulk material. This is in line with our data of a decreasing fungal-to-bacterial ratio and increasing δ13C values with depth (Figure 2).
With regard to acidobacteria, Weijers et al. (2010) found that acidobacterial-derived mFAs have enriched δ13C values compared to plants and in the same range then bulk. The reason for that could be the preferred cycling of glucose and pectin, which are enriched in δ13C (Pankratov et al., 2008; Lerch et al., 2011). As the highest quantity of acidobacterial-derived mFAs is found in the mesotelm (Figures 2, 3), we found that the increasing metabolism rate of acidobacteria could be linked to the increasing δ13C values (Figure 2). An increase in acidobacterial-derived mFAs is expected because acidobacteria are known to be autotrophs and are therefore able to assimilate CO2 from the soil (Wiesenberg et al., 2008). As soil CO2 is enriched in 13C Wiesenberg et al. (2008), the increasing metabolism by acidobacteria increases the δ13C values in the remaining substrate further.
Microbial-Derived Membrane Fatty Acid Quantities and Nitrogen Isotopic Values
Considering the parallel depth trend of the concentrations of bacterial-derived mFAs and δ15N values (Figure 4), we conclude that nitrogen stable isotope values appear to be linked more closely to bacterial abundance than to overall microbial abundance. This interpretation is supported by a higher Spearman correlation index of R = 0.54 for bacteria compared to R = 0.30 for all microbes. Peaks in bacterial-derived mFA abundance and at δ15N values occur in tandem and are visible for both sites investigated (Figure 2). The correlation is clear if we consider that the quantity of N in microbial biomass is a substantial part of N in bulk substrate of poor peatlands Lin et al. (2014), and thus, isotopic fractionation by microbes will influence the bulk isotopic value significantly.
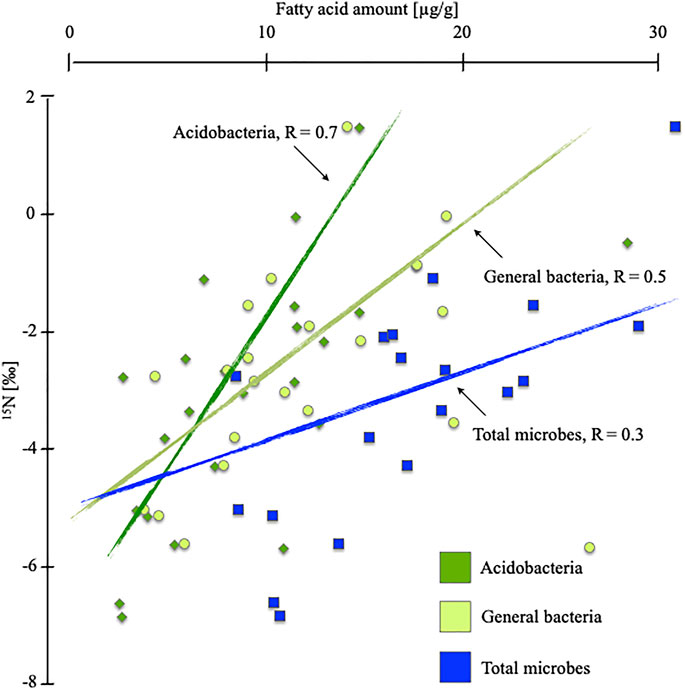
FIGURE 4. Spearman correlation index (R) and correlation of δ15N [‰] and microbial, general bacterial and acidobacterial membrane fatty acid marker amount [µg/g], for the rewetted site in Degerö Stormyr and the drained site in Lakkasuo.
We differentiated between acidobacterial and general bacterial markers. For both bacterial groups, the mFA concentrations increased towards the δ15N turning point and were lowest in the catotelm (Figure 2).
For both peatland sites, we found the highest concentration of acidobacterial-derived mFAs reaching approximately 50% of all bacterial-derived mFAs in the mesotelm (Figure 3). In the upper mesotelm and the catotelm, the percentage is approximately 40% (Figure 3). Hence, our results are congruent with the results of Artz in 2013, which point to a characteristic depth trend with a peak in the mesotelm of acidobacterial populations in nutrient-poor peatlands (Figures 2, 3).
Acidobacteria are closely involved in nitrogen cycling (Urbanova and Barta, 2014; Eichhorst et al., 2018; Kalam et al., 2020). For example, they are involved in denitrification processes (Urbanova and Barta, 2014). They reduce nitrate, nitrite and possibly nitric oxide (Ward et al., 2009; Eichhorst et al., 2018; Kalam et al., 2020). As Eichhorst et al. (2018) reported, there are also evidence for the mobilization of ammonium by acidobacteria and the gaseous loss of N2O. All these cycling processes are known to increase δ15N values in bulk material Denk et al. (2017) and are observed predominantly in the mesotelm (Palmer and Horn, 2015; Oshiki et al., 2016). Thus, acidobacteria could be the key to forming the δ15N depth trends in nutrient-poor peatlands. We assume that as the occurrence of acidobacteria increases, more nitrogen will be processed. Lighter 14N will be released in a gaseous state, leached or mineralized and then incorporated by plants and translocated upwards within the plants. These processes should lead to increasing δ15N values in the remaining substrate (Hausmann et al., 2018). This correlation is illustrated by our data. Acidobacterial-derived mFA markers, shown in absolute concentrations (Figure 2) and in relation to other microbial markers, are highest at the δ15N turning point (Figures 2, 3). At the δ15N turning point, the relative abundance of acidobacterial-derived mFAs in relation to all bacterial-derived mFAs is highest, with 49.92% for LD and 55.64% for DR (Figure 3). Overall investigated sites, δ15N values and the acidobacterial-derived mFAs are highly significantly correlated (R = 0.66, p < 0.05; Figure 4).
Depth Trend of Stable Isotopes in Drained and Rewetted Sites
The comparison of stable isotope depth trends (δ15N, δ13C) revealed specific differences between undrained, drained, and rewetted sites, which remained stable over both years. Regardless of the sampling year, none of the cores of undrained sites (DN131-3, DN17, LN131-3, LN17) showed any depth trend of stable isotopes (Figure 5). The cores of the drained site Lakkasuo (LD131–3, LD17) showed trends within the drained layer (acrotelm and mesotelm). δ13C values increased throughout the drained layer, and a peak of 15N values (the δ15N turning point) was visible in the mesotelm, which both is indicative for the ongoing loss of typical peatland soil functioning (e.g. carbon storage). In the rewetted site Degerö Stormyr (DR131–3, DR17), no depth trends in δ15N values were observed in the layer likely formed during rewetting conditions above the layer formed during former drainage (Figure 5). Below the rewetted layer, the δ15N depth trend of the former mesotelm was preserved in the newly established (>20 years of rewetting) catotelm. The decreasing trend of δ13C values in DR also seems to have slowed down in the rewetted core in 2017 (Figure 5).
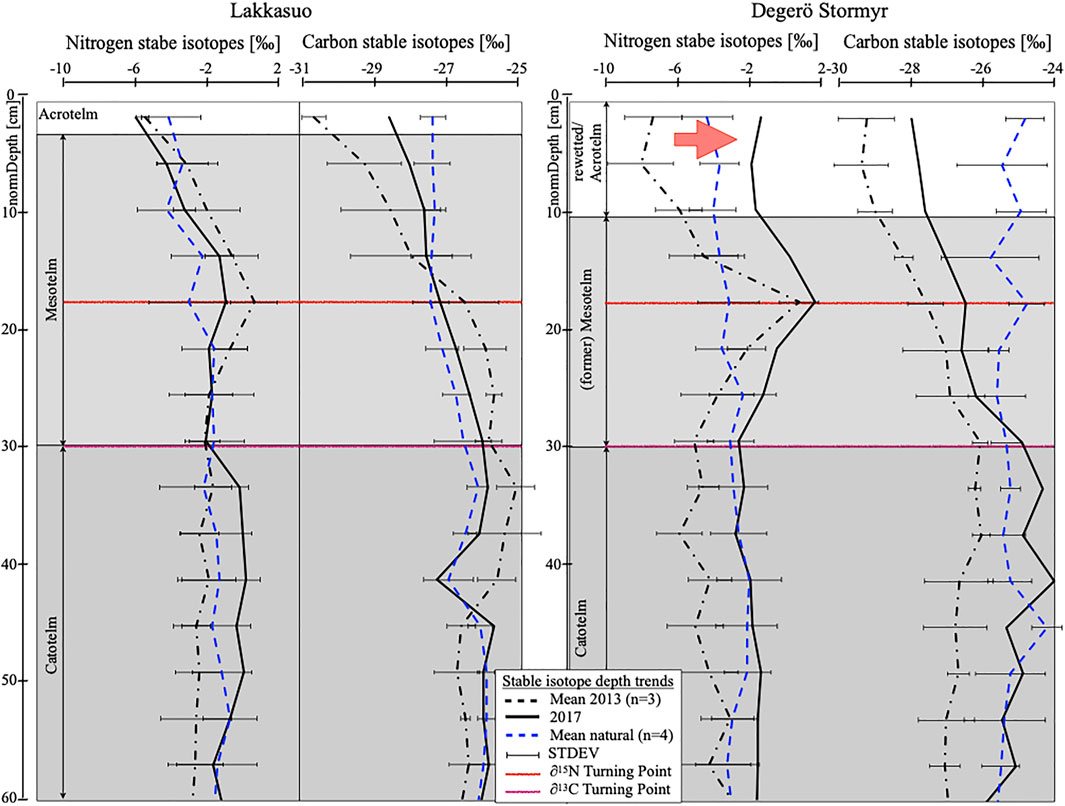
FIGURE 5. Depth-normalized stable isotope trends (nitrogen, carbon) for Degerö Stormyr and Lakkasuo, separated for drained sites (2013 (black dotted) and 2017 (black)) and undrained sites (blue dotted); Red reference line gives the δ15N turning point, violet reference line gives the δ13C turning point; Note the shift between the nitrogen isotope depth trend from 2013 to 2017 in the rewetted Degerö Stormyr (marked with red arrow).
The time-dependent sampling was devised to test the robustness of the stable isotope depth pattern as an indicator for peatland hydrology in relation to the onset, duration and ending of a drainage event. With one exception, nitrogen stable isotope depth trends in both years - 2013 and 2017 - are very similar, confirming our hypothesis (Figure 5). The exception is the δ15N depth trends in Degerö Stormyr, the site that was rewetted in recent decades, where a shift in the rewetted layer in 2017 towards more undrained depth trends compared to 2013 most likely indicates a successful restoration.
The changes in stable isotope values with rewetting occur simultaneously with changes in microbial-derived mFA quantities. This indicates that the changed environmental conditions lead to changing microbial community compositions and therefore changed metabolism processes (Asada et al., 2005). This is in line with the findings of Elliott et al. (2015), who also found distinct changes in microbial communities with rewetting. In drained peat sites, they also found increased values of acidobacteria, which we also found in our sites (Figures 2, 3). Elliott et al. (2015) also indicated that changes in microbial communities are rapid after rewetting and could therefore also indicate relatively short rewetting times and the recovery of typical peatland soil functioning.
Conclusion
Our results confirm that the existence of specific microbial groups is correlated to stable isotope depth trends (δ15N, δ13C) of nutrient-poor peatlands, particularly that of nitrogen isotopes. An analysis of mFA markers for general bacteria as well as specific mFA markers for acidobacteria, and fungi revealed a high abundance of fungal-derived mFAs in the aerobic acrotelm. The upper mesotelm showed a transition to decreasing fungal-derived and increasing bacterial-derived mFA abundance (especially that of acidobacteria). As such, the δ15N turning point seems to be driven in particular by the nitrogen cycling of bacterial metabolism, most prominently by acidobacteria. Downwards along the (former) mesotelm, δ15N values decreased, likely due to low microbial metabolic rates. Finally, in the permanent anaerobic catotelm, where microbial metabolism is strongly impeded, δ15N values show no further depth trend. Stable isotope depth trends (δ15N, δ13C) from two different years were able to confirm the persistence of these trends as indicators for ongoing drainage and therefore impaired soil functioning, e.g. as storage of carbon. Furthermore, δ15N seems to indicate former drainage followed by rewetting processes. In summary, we conclude that microbial abundance as indicated by group specific biomarkers can be confirmed as key for stable isotope depth trends and that differences in δ15N depth profiles may be indicative for drainage and rewetting.
Data Availability Statement
The original contributions presented in the study are included in the article/Supplementary Material, further inquiries can be directed to the corresponding author.
Author Contributions
MG-S conducted the sampling, measurements, evaluation and analysis of the data and cowrote the paper with CA. AB assisted with the measurements and helped with the analytics. KK added to the discussion. JL and CA had the project idea, supervised and added to the discussion.
Funding
This research has been supported by the Swiss National Science Foundation (SNF; grant no. 169556).
Conflict of Interest
The authors declare that the research was conducted in the absence of any commercial or financial relationships that could be construed as a potential conflict of interest.
Publisher’s Note
All claims expressed in this article are solely those of the authors and do not necessarily represent those of their affiliated organizations, or those of the publisher, the editors and the reviewers. Any product that may be evaluated in this article, or claim that may be made by its manufacturer, is not guaranteed or endorsed by the publisher.
Supplementary Material
The Supplementary Material for this article can be found online at: https://www.frontiersin.org/articles/10.3389/fenvs.2021.730106/full#supplementary-material
References
Alewell, C., Giesler, R., Klaminder, J., Leifeld, J., and Rollog, M. (2011). Stable Carbon Isotopes as Indicators for Environmental Change in Palsa Peats. Biogeosciences 8, 1769–1778. doi:10.5194/bg-8-1769-2011
Alexandersson, H., Karlström, C., and Larsson-Mccan, S. (1991). Temperature and Precipitation in Sweden 1961-1990. Reference Normals Meteorologi. 81. Norrköping, Sweden: Swedish Meteorological and Hydrological Institute (SMHI).
Andersen, R., Grasset, L., Thormann, M. N., Rochefort, L., and Francez, A.-J. (2010). Changes in Microbial Community Structure and Function Following Sphagnum Peatland Restoration. Soil Biol. Biochem. 42, 291–301. doi:10.1016/j.soilbio.2009.11.006
Artz, R. R. E., Chapman, S. J., Siegenthaler, A., Mitchell, E. A. D., Buttler, A., Bortoluzzi, E., et al. (2008). Functional Microbial Diversity in Regenerating Cutover Peatlands Responds to Vegetation Succession. J. Appl. Ecol. 45, 1799–1809. doi:10.1111/j.1365-2664.2008.01573.x
Artz, R. R. E. (2013). Microbial Community Structure and Carbon Substrate Use in Northern PeatlandsCarbon Cycling in Northern Peatlands. American Geophysical Union, 111–129. doi:10.1029/2008GM000806
Asada, T., Warner, B. G., and Aravena, R. (2005). Nitrogen Isotope Signature Variability in Plant Species from Open Peatland. Aquat. Bot. 82 (4), 297–307. doi:10.1016/j.aquabot.2005.05.005
Bajerski, F., Wagner, D., and Mangelsdorf, K. (2017). Cell Membrane Fatty Acid Composition of Chryseobacterium Frigidisoli PB4T, Isolated from Antarctic Glacier Forefield Soils, in Response to Changing Temperature and pH Conditions. Front. Microbiol. 8, 677. doi:10.3389/fmicb.2017.00677
Biester, H., Knorr, K.-H., Schellekens, J., Basler, A., and Hermanns, Y.-M. (2014). Comparison of Different Methods to Determine the Degree of Peat Decomposition in Peat Bogs. Biogeosciences 11, 2691–2707. doi:10.5194/bg-11-2691-2014
Blodau, C. (2002). Carbon Cycling in Peatlands - A Review of Processes and Controls. Environ. Rev. 10 (2), 111–134. doi:10.1139/a02-004
Boström, B., Comstedt, D., and Ekblad, A. (2007). Isotope Fractionation and 13C Enrichment in Soil Profiles during the Decomposition of Soil Organic Matter. Oecologia 153, 89–98. doi:10.1007/s00442-007-0700-8
Bremner, J. M. (1997). Sources of Nitrous Oxide in Soils. Nutrient Cycling Agroecosystem 49, 7–16. doi:10.1023/a:1009798022569
Chaves Torres, L., and Pancost, R. D. (2016). Insoluble Prokaryotic Membrane Lipids in a Sphagnum Peat: Implications for Organic Matter Preservation. Org. Geochem. 93, 77–91. doi:10.1016/j.orggeochem.2015.12.013
Clymo, R. S. (1984). The Limits to Peat Bog Growth. Phil. Trans. R. Soc. Lond. B 303, 605–654. doi:10.1098/rstb.1984.0002
Damman, A. W. H. (1988). Regulation of Nitrogen Removal and Retention in Sphagnum Bogs and Other Peatlands. OIKOS 51, 291–305. doi:10.2307/3565310
De Boer, W., and van der Wal, A. (2008). Chapter 8 Interactions between Saprotrophic Basidiomycetes and Bacteria. Br. Mycol. Soc. Symposia Ser. 28, 143–153. doi:10.1016/S0275-0287(08)80010-0
Dedysh, S. N., and Sinninghe Damsté, J. S. (2018). Acidobacteria. eLS. Chichester: John Wiley & Sons, 1–10. doi:10.1002/9780470015902.a0027685
Denk, T. R. A., Mohn, J., Decock, C., Lewicka-Szczebak, D., Harris, E., Butterbach-Bahl, K., et al. (2017). The Nitrogen Cycle: A Review of Isotope Effects and Isotope Modeling Approaches. Soil Biol. Biochem. 105, 121–137. doi:10.1016/j.soilbio.2016.11.015
Eichorst, S. A., Trojan, D., Roux, S., Herbold, C., Rattei, T., and Woebken, D. (2018). Genomic Insights into the Acidobacteria Reveal Strategies for Their success in Terrestrial Environments. Environ. Microbiol. 20 (3), 1041–1063. doi:10.1111/1462-2920.14043
Elliott, D. R., Caporn, S. J., Nwaishi, F., Nilsson, R. H., and Sen, R. (2015). Bacterial and Fungal Communities in a Degraded Ombrotrophic Peatland Undergoing Natural and Managed Re-vegetation. PLoS One 10, e0124726. doi:10.1371/journal.pone.0124726
Elvert, M., Boetius, A., Knittel, K., and Jørgensen, B. B. (2003). Characterization of Specific Membrane Fatty Acids as Chemotaxonomic Markers for Sulfate-Reducing Bacteria Involved in Anaerobic Oxidation of Methane. Geomicrobiology J. 20, 403–419. doi:10.1080/01490450303894
Eurola, S., Hicks, S. T., and Kaakinen, E. (1984). “Key to Finnish Mire Types,” in European Mires. Editor P. D. Moore (London, Great Britain): Academic Press), 1–117. doi:10.1016/b978-0-12-505580-2.50006-4
Finotti, E., Moretto, D., Marsella, R., and Mercantini, R. (1993). Temperature Effects and Fatty Acid Patterns in Geomyces Species Isolated from Antarctic Soil. Polar Biol. 13, 127–130. doi:10.1007/BF00238545
Frolking, S., Roulet, N. T., Moore, T. R., Richard, P. J. H., Lavoie, M., and Muller, S. D. (2001). Modeling Northern Peatland Decomposition and Peat Accumulation. Ecosystems 4, 479–498. doi:10.1007/s10021-001-0105-1
Goldberg, S. D., Knorr, K.-H., Blodau, C., Lischeid, G., and Gebauer, G. (2010). Impact of Altering the Water Table Height of an Acidic Fen on N2O and NO Fluxes and Soil Concentrations. Glob. Change Biol. 16, 220–233. doi:10.1111/j.1365-2486.2009.02015.x
Groß-Schmölders, M., von Sengbusch, P., Krüger, J. P., Klein, K., Birkholz, A., Leifeld, J., et al. (2020). Switch of Fungal to Bacterial Degradation in Natural, Drained and Rewetted Oligotrophic Peatlands Reflected in δ15N and Fatty Acid Composition. Soil 6, 299–313. doi:10.5194/soil-6-299-2020
Hausmann, B., Pelikan, C., Herbold, C. W., Köstlbacher, S., Albertsen, M., Eichorst, S. A., et al. (2018). Peatland Acidobacteria with a Dissimilatory Sulfur Metabolism. Isme J. 12 (7), 1729–1742. doi:10.1038/s41396-018-0077-1
Hobbie, E. A., Chen, J., Hanson, P. J., Iversen, C. M., McFarlane, K. J., Thorp, N. R., et al. (2017). Long-term Carbon and Nitrogen Dynamics at SPRUCE Revealed through Stable Isotopes in Peat Profiles. Biogeosciences 14, 2481–2494. doi:10.5194/bg-14-2481-2017
Hobbie, E. A., and Högberg, P. (2012). Nitrogen Isotopes Link Mycorrhizal Fungi and Plants to Nitrogen Dynamics. New Phytol. 196, 367–382. doi:10.1111/j.1469-8137.2012.04300.x
Högberg, P., Högbom, L., Schinkel, H., Högberg, M., Johannisson, C., and Wallmark, H. (1996). 15N Abundance of Surface Soils, Roots and Mycorrhizas in Profiles of European forest Soils. Oecologia 108, 207–214. doi:10.1007/BF00334643
Hornibrook, E. R. C., Longstaffe, F. J., and Fyfe, W. S. (1997). Spatial Distribution of Microbial Methane Production Pathways in Temperate Zone Wetland Soils: Stable Carbon and Hydrogen Isotope Evidence. Geochimica et Cosmochimica Acta 61 (4), 745–753. doi:10.1016/s0016-7037(96)00368-7
IUSS Working Group WRB (2015). “World Reference Base for Soil Resources 2014, Update 2015,”. World Soil Resources Reports 106 (Rome, Italy: Food and Agriculture Organization of the United Nations).
Kalam, S., Basu, A., Ahmad, I., Sayyed, R. Z., El-Enshasy, H. A., Dailin, D. J., et al. (2020). Recent Understanding of Soil Acidobacteria and Their Ecological Significance: A Critical Review. Front. Microbiol. 11, 580024. doi:10.3389/fmicb.2020.580024
Kohl, L., Laganière, J., Edwards, K. A., Billings, S. A., Morrill, P. L., Van Biesen, G., et al. (2015). Distinct Fungal and Bacterial δ13C Signatures as Potential Drivers of Increasing δ13C of Soil Organic Matter with Depth. Biogeochemistry 124, 13–26. doi:10.1007/s10533-015-0107-2
Kohzu, A., Matsui, K., Yamada, T., Sugimoto, A., and Fujita, N. (2003). Significance of Rooting Depth in Mire Plants: Evidence from Natural 15 N Abundance. Ecol. Res. 18, 257–266. doi:10.1046/j.1440-1703.2003.00552.x
Krüger, J. P., Alewell, C., Minkkinen, K., Szidat, S., and Leifeld, J. (2016). Calculating Carbon Changes in Peat Soils Drained for Forestry with Four Different Profile-Based Methods. For. Ecol. Manag. 381, 29–36. doi:10.1016/j.foreco.2016.09.006
Krüger, J. P., Leifeld, J., and Alewell, C. (2014). Degradation Changes Stable Carbon Isotope Depth Profiles in Palsa Peatlands. Biogeosciences 11, 3369–3380. doi:10.5194/bg-11-3369-2014
Krüger, J. P., Leifeld, J., Glatzel, S., Szidat, S., and Alewell, C. (2015). Biogeochemical Indicators of Peatland Degradation - a Case Study of a Temperate Bog in Northern Germany. Biogeosciences 12, 2861–2871. doi:10.5194/bg-12-2861-2015
Laine, J., Komulainen, V., Laiho, R., Minkkinen, K., Rasinmäki, A., Sallantus, T., et al. (2004). Lakkasuo: A Guide to a Mire Ecosystem. Helsinki: Department of Forest Ecology. University of Helsinki.
Leifeld, J., and Menichetti, L. (2018). The Underappreciated Potential of Peatlands in Global Climate Change Mitigation Strategies. Nat. Commun. 9, 1071. doi:10.1038/s41467-018-03406-6
Lerch, T. Z., Nunan, N., Dignac, M.-F., Chenu, C., and Mariotti, A. (2011). Variations in Microbial Isotopic Fractionation during Soil Organic Matter Decomposition. Biogeochemistry 106, 5–21. doi:10.1007/s10533-010-9432-7
Lin, X., Tfaily, M. M., Green, S. J., Steinweg, J. M., Chanton, P., Imvittaya, A., et al. (2014). Microbial Metabolic Potential for Carbon Degradation and Nutrient (Nitrogen and Phosphorus) Acquisition in an Ombrotrophic Peatland. Appl. Environ. Microbiol. 80, 3531–3540. doi:10.1128/AEM.00206-14
McCune, B. P., and Grace, J. B. (2002). Analysis of Ecological Communities. Gleneden Beach, United State of America: MjM Software Design.
McGrew, J., and Monroe, C. B. (2000). Statistical Problem Solving in Geography. Long Grove, United States of America: Waveland Press Inc.
Minick, K. J., Mitra, B., Li, X., Noormets, A., and King, J. S. (2019). Water Table Drawdown Alters Soil and Microbial Carbon Pool Size and Isotope Composition in Coastal Freshwater Forested Wetlands. Front. For. Glob. Change 2, 7. doi:10.3389/ffgc.2019.00007
Minkkinen, K., Vasander, H., Jauhiainen, S., Karsisto, M., and Laine, J. (1998). Post-drain- Age Changes in Vegetation Composition and Carbon Balance in Lakkasuo Mire, Central Finland. Plant and Soil 207, 107–120. doi:10.1023/A:1004466330076
Moore, T., and Basiliko, N. (2006). “Decomposition in Boreal Peatlands,” in Boreal Peatland Ecosystems, Ecological Studies (Analysis and Synthesis) (188. Editors R. K. Wieder, and D. H. Vitt (Berlin, Heidelberg, Germany: Springer).
Myers, M. R., and King, G. M. (2016). Isolation and Characterization of Acidobacterium Ailaaui Sp. nov., a Novel Member of Acidobacteria Subdivision 1, from a Geothermally Heated Hawaiian Microbial Mat. Int. J. Syst. Evol. Microbiol. 66, 5328–5335. doi:10.1099/ijsem.0.001516
Niemen, M. (1998). Changes in Nitrogen Cycling Following the Clearcutting of Drained Peatland Forests in Southern Finland. Boreal Environ. 31, 9–21. Available at: http://urn.fi/URN:NBN:fi-fe2016091423744.
Nilsson, M., Sagerfors, J., Buffam, I., Laudon, H., Eriksson, T., Grelle, A., et al. (2008). Contemporary Carbon Accumulation in a Boreal Oligotrophic Minerogenic Mire - a Significant Sink after Accounting for All C-Fluxes. Glob. Change Biol. 14 (10), 2317–2332. doi:10.1111/j.1365-2486.2008.01654.x
Novák, M., Buzek, F., and Adamová, M. (1999). Vertical Trends in δ13C, δ15N and δ34S Ratios in Bulk Sphagnum Peat. Soil Biol. Biochem. 31, 1343–1346. doi:10.1016/S0038-0717(99)00040-1
O`Leary, W. M., and Wilkinson, S. (1988). “Gram-positive Bacteria,” in Microbial Lipids. Editors C. Ratledge, and S. G. Wilkinson (London: Academic Press), 117–185.
Oshiki, M., Satoh, H., and Okabe, S. (2016). Ecology and Physiology of Anaerobic Ammonium Oxidizing Bacteria. Environ. Microbiol. 18 (9), 2784–2796. doi:10.1111/1462-2920.13134
Palmer, K., and Horn, M. A. (2015). Denitrification Activity of a Remarkably Diverse Fen Denitrifier Community in Finnish Lapland Is N-Oxide Limited. PLoS ONE 10, e0123123. doi:10.1371/journal.pone.0123123
Peel, M. C., Finlayson, B. L., and McMahon, T. A. (2007). Updated World Map of the Köppen-Geiger Climate Classification. Hydrol. Earth Syst. Sci. 11, 1633–1644. doi:10.5194/hess-11-1633-2007
Peltoniemi, K., Fritze, H., and Laiho, R. (2009). Response of Fungal and Actinobacterial Communities to Water-Level Drawdown in Boreal Peatland Sites. Soil Biol. Biochem. 41, 1902–1914. doi:10.1016/j.soilbio.2009.06.018
Piotrowska-Seget, Z., and Mrozik, A. (2003). Signature Lipid Biomarker (SLB) Analysis in Determining Changes in Communitiy Structure of Soil Microorganisms. Polish J. Environ. Stud. 12 (6), 669–675.
Reiffarth, D. G., Petticrew, E. L., Owens, P. N., and Lobb, D. A. (2016). Sources of Variability in Fatty Acid (FA) Biomarkers in the Application of Compound-specific Stable Isotopes (CSSIs) to Soil and Sediment Fingerprinting and Tracing: A Review. Sci. Total Environ. 565, 8–27. doi:10.1016/j.scitotenv.2016.04.137
Robinson, D., Handley, L. L., and Scrimgeour, C. M. (1998). A Theory for 15 N/14 N Fractionation in Nitrate-Grown Vascular Plants. Planta 205, 397–406. doi:10.1007/s004250050336
Schulze, E. D., Luyssaert, S., Luyssaert, S., Ciais, P., Freibauer, A., Janssens, I. A., et al. the CarboEurope Team (2009). Importance of Methane and Nitrous Oxide for Europe's Terrestrial Greenhouse-Gas Balance. Nat. Geosci. 2, 842–850. doi:10.1038/ngeo686
Serkebaeva, Y. M., Kim, Y., Liesack, W., and Dedysh, S. N. (2013). Pyrosequencing-based Assessment of the Bacteria Diversity in Surface and Subsurface Peat Layers of a Northern Wetland, with Focus on Poorly Studied Phyla and Candidate Divisions. PLoS One 8 (5), e63994–14. doi:10.1371/journal.pone.0063994
Sinninghe Damsté, J. S., Rijpstra, W. I. C., Hopmans, E. C., Weijers, J. W. H., Foesel, B. U., Overmann, J., et al. (2011). 13,16-Dimethyl Octacosanedioic Acid (Iso-Diabolic Acid), a Common Membrane-Spanning Lipid of Acidobacteria Subdivisions 1 and 3. Appl. Environ. Microbiol. 77, 4147–4154. doi:10.1128/AEM.00466-11
Strickland, M. S., and Rousk, J. (2010). Considering Fungal:bacterial Dominance in Soils - Methods, Controls, and Ecosystem Implications. Soil Biol. Biochem. 42, 1385–1395. doi:10.1016/j.soilbio.2010.05.007
Sundh, I., Nilsson, M., and Borgå, P. (1997). Variation in Microbial Community Structure in Two Boreal Peatlands as Determined by Analysis of Phospholipid Fatty Acid Profiles. Appl. Environ. Microbiol. 63 (4), 1476–1482. doi:10.1128/aem.63.4.1476-1482.1997
Tfaily, M. M., Cooper, W. T., Kostka, J. E., Chanton, P. R., Schadt, C. W., Hanson, P. J., et al. (2014). Organic Matter Transformation in the Peat Column at Marcell Experimental Forest: Humification and Vertical Stratification. J. Geophys. Res. Biogeosci. 119, 661–675. doi:10.1002/2013JG002492
Thormann, M. (2011). In Vitro decomposition of Sphagnum-Derived Acrotelm and Mesotelm Peat by Indigenous and Alien Basidiomycetous. Mires and Peat 8, 1–12.
Thormann, M. N., Currah, R. S., and Bayley, S. E. (2004). Patterns of Distribution of Microfungi in Decomposing Bog and Fen Plants. Can. J. Bot. 82, 710–720. doi:10.1139/b04-025
Thormann, M. N. (2006). Diversity and Function of Fungi in Peatlands: A Carbon Cycling Perspective. Can. J. Soil Sci. 86, 281–293. doi:10.4141/S05-082
Urbanová, Z., and Bárta, J. (2014). Microbial Community Composition Andin Silicopredicted Metabolic Potential Reflect Biogeochemical Gradients between Distinct Peatland Types. FEMS Microbiol. Ecol. 90, 633–646. doi:10.1111/1574-6941.12422
Vestal, J. R., and White, D. C. (1989). Lipid Analysis in Microbial Ecology. BioScience 39, 535–541. doi:10.2307/1310976
Wallander, H., Mörth, C.-M., and Giesler, R. (2009). Increasing Abundance of Soil Fungi Is a Driver for 15N Enrichment in Soil Profiles along a Chronosequence Undergoing Isostatic Rebound in Northern Sweden. Oecologia 160, 87–96. doi:10.1007/s00442-008-1270-0
Wang, M., Tian, J., Bu, Z., Lamit, L. J., Chen, H., Zhu, Q., et al. (2019). Structural and Functional Differentiation of the Microbial Community in the Surface and Subsurface Peat of Two Minerotrophic Fens in China. Plant Soil 437, 21–40. doi:10.1007/s11104-019-03962-w
Ward, N. L., Challacombe, J. F., Janssen, P. H., Henrissat, B., Coutinho, P. M., Wu, M., et al. (2009). Three Genomes from the Phylum Acidobacteria Provide Insight into the Lifestyles of These Microorganisms in Soils. Appl. Environ. Microbiol. 75 (7), 2046–2056. doi:10.1128/AEM.02294-08
Weijers, J. W. H., Wiesenberg, G. L. B., Bol, R., Hopmans, E. C., and Pancost, R. D. (2010). Carbon Isotopic Composition of Branched Tetraether Membrane Lipids in Soils Suggest a Rapid Turnover and a Heterotrophic Life Style of Their Source Organism(s). Biogeosciences 7, 2959–2973. doi:10.5194/bg-7-2959-2010
Wiesenberg, G. L. B., Schmidt, M. W. I., and Schwark, L. (2008). Plant and Soil Lipid Modifications under Elevated Atmospheric CO2 Conditions: I. Lipid Distribution Patterns. Org. Geochem. 39 (1), 91–102. doi:10.1016/j.orggeochem.2007.09.005
Willers, C., Jansen van Rensburg, P. J., and Claassens, S. (2015). Phospholipid Fatty Acid Profiling of Microbial Communities-A Review of Interpretations and Recent Applications. J. Appl. Microbiol. 119, 1207–1218. doi:10.1111/jam.12902
Winsborough, C., and Basiliko, N. (2010). Fungal and Bacterial Activity in Northern Peatlands. Geomicrobiology J. 27, 315–320. doi:10.1080/01490450903424432
Wynn, J. G., Harden, J. W., and Fries, T. L. (2006). Stable Carbon Isotope Depth Profiles and Soil Organic Carbon Dynamics in the Lower Mississippi Basin. Geoderma 131, 89–109. doi:10.1016/j.geoderma.2005.03.005
Yang, G., Tian, J., Chen, H., Jiang, L., Zhan, W., Hu, J., et al. (2019). Peatland Degradation Reduces Methanogens and Methane Emissions from Surface to Deep Soils. Ecol. Indicators 106, 105488. doi:10.1016/j.ecolind.2019.105488
Zedler, J. B., and Kercher, S. (2005). WETLAND RESOURCES: Status, Trends, Ecosystem Services, and Restorability. Annu. Rev. Environ. Resour. 30 (1), 39–74. doi:10.1146/annurev.energy.30.050504.144248
Keywords: peatland degradation, stable isotopes, membrane fatty acids, soil microbiology, biochemistry, element cycling
Citation: Groß-Schmölders M, Klein K, Birkholz A, Leifeld J and Alewell C (2021) Rewetting and Drainage of Nutrient-Poor Peatlands Indicated by Specific Bacterial Membrane Fatty Acids and a Repeated Sampling of Stable Isotopes (δ15N, δ13C) . Front. Environ. Sci. 9:730106. doi: 10.3389/fenvs.2021.730106
Received: 24 June 2021; Accepted: 19 August 2021;
Published: 01 September 2021.
Edited by:
Miriam Muñoz-Rojas, University of New South Wales, AustraliaReviewed by:
Jeffrey Chanton, Florida State University, United StatesYansheng Li, Northeast Institute of Geography and Agroecology (CAS), China
Copyright © 2021 Groß-Schmölders, Klein, Birkholz, Leifeld and Alewell. This is an open-access article distributed under the terms of the Creative Commons Attribution License (CC BY). The use, distribution or reproduction in other forums is permitted, provided the original author(s) and the copyright owner(s) are credited and that the original publication in this journal is cited, in accordance with accepted academic practice. No use, distribution or reproduction is permitted which does not comply with these terms.
*Correspondence: Miriam Groß-Schmölders, bWlyaWFtLmdyb3NzLXNjaG1vZWxkZXJzQHVuaWJhcy5jaA==