- Forest Botany and Tree Physiology, University of Göttingen, Göttingen, Germany
Phosphorus (P) availability shows large differences among different soil types, affecting P nutrition of forest trees. Chemical binding of P to soil moieties affects partitioning of P between soil particles and solution, influencing soluble P concentrations upon which plants, their associated mycorrhizal symbionts, and microbes feed. The goal of this study was to characterize root P uptake by mycorrhizal and non-mycorrhizal root tips in competition with microbes in situ in the organic and mineral layer of a P-rich and a P-poor forest. We used intact soil cores (0.2 m depth) from beech (Fagus sylvatica) forests to tracing the fate of 33P in soil, plant and microbial fractions. We used the dilution of 33P in the rhizosphere of each soil layer to estimate the enrichment with new P in mycorrhizal and non-mycorrhizal root tips and root P uptake. In soil cores from P-rich conditions, 25 and 75% of root P uptake occurred in the organic and mineral layer, respectively, whereas in the P-poor forest, 60% occurred in the organic and 40% in the mineral layer. Mycorrhizal P efficiency, determined as enrichment of new P in mycorrhizal root tips, differed between soil layers. Root P uptake was correlated with mycorrhizal P efficiency and root tip abundance but not with root tip abundance as a single factor. This finding underpins the importance of the regulation of mycorrhizal P acquisition for root P supply. The composition of mycorrhizal assemblages differed between forests but not between soil layers. Therefore, differences in P efficiencies resulted from physiological adjustments of the symbionts. Non-mycorrhizal root tips were rare and exhibited lower enrichment with new P than mycorrhizal root tips. Their contribution to root P supply was negligible. Microbes were strong competitors for P in P-poor but not in P-rich soil. Understory roots were present in the P-rich soil but did not compete for P. Our results uncover regulation of mycorrhizal P efficiencies and highlight the complexity of biotic and abiotic factors that govern P supply to trees in forest ecosystems.
Introduction
Plants take up phosphorus (P) as inorganic phosphate (Pi, , or ) from the soil solution (Plassard and Dell, 2010). However, this P source is scarce because Pi has low diffusion rates in soil (Shen et al., 2011), is sequestered by soil minerals such as Fe and Al hydroxides (Lambers et al., 2015b; Prietzel et al., 2016) or is bound to organic matter (Ilg et al., 2008; Lambers et al., 2008). Besides, P input into soil by P deposition is extremely low (Peñuelas et al., 2013). Consequently, weathering and P utilization lead to a shift in P availability for plants (Walker and Syers, 1976; Callaway and Nadkarni, 1991; Vitousek et al., 2010; Vincent et al., 2013) and drive P reductions during soil geological aging (Turner and Condron, 2013). As a result, different soils vary drastically in P availability (Chadwick et al., 1999; Lang et al., 2016).
Since P is an essential element for plant nutrition, plants have to adjust their acquisition strategies to cope with different P availabilities (Vance et al., 2003; Lambers et al., 2008, 2015a,b). This requirement is especially important for tree species such as beech (Fagus sylvatica L.), which occur on a broad range of different soil types (Leuschner et al., 2006). It has been shown that beech ecosystems along a geosequence of different P availabilities adjust their nutritional strategies from P acquisition under high P availability to P recycling when P is scarce (Lang et al., 2017). Due to differences in soil structure and nutrient availability, beech trees in P-poor soil modify their root systems to explore the organic soil layer more intensely than trees in P-rich soil (Lang et al., 2017). They further exhibit extended seasonal P acquisition (Spohn et al., 2018), stronger recycling of P resources from internal storage tissues (Netzer et al., 2017; Zavišić and Polle, 2018), and reductions in growth and photosynthesis (Yang et al., 2016), which are released upon P-fertilization (Zavišić et al., 2018). In acidic, P-poor soils, forests build up a thick organic layer from which P can be re-mobilized by decomposition (Heuck and Spohn, 2016; George et al., 2018; Zederer and Talkner, 2018). In mesocosms with reconstructed soil layers composed of homogenized organic and mineral soil, the organic layer was important for the P supply to young beech saplings (Hauenstein et al., 2018). Under field conditions, understory vegetation is present and more abundant in P-rich than P-poor beech forests (Rieger et al., 2019). To assess beech P nutrition, the potential competition of understory for nutritional resources needs to be taken into account (Nambiar and Sands, 1993). Furthermore, soil microbes are important competitors for mineral nutrients (Kuzyakov and Xu, 2013; Zhu et al., 2017).
It is well-known that ectomycorrhizal fungi play a critical role for nutrition of forest trees in temperate ecosystems (Cairney, 2011; Becquer et al., 2014; Johri et al., 2015; Nehls and Plassard, 2018). Ectomycorrhizas produce hyphal networks that overcome zones of P depletion by hyphal tunneling (Jongmans et al., 1997; Hoffland et al., 2003; Jansa et al., 2011). Mycorrhizal fungi further contribute to bioweathering by secretion of organic acids (Wallander, 2000; Balogh-Brunstad et al., 2008; Jansa et al., 2011) and to the recycling of organic-bound P by production of acidic phosphatases (Hinsinger et al., 2015; Smith et al., 2015). Already early tracer studies with radioactive Pi showed that mycorrhizal roots of pine and beech accumulate more P than non-mycorrhizal roots (Kramer and Wilbur, 1949; Harley et al., 1954). In beech forests, the fraction of non-mycorrhizal root tips is generally small (Pena et al., 2010; Lang et al., 2017) but whether they play a role for P acquisition in natural forest soils, for example, in deeper soil layers, has not yet been studied. P acquisition strategies of distinct mycorrhizal communities in natural soils have mainly been studied empirically and not directly by tracing P uptake. It is therefore unknown if mycorrhizal fungal assemblages at different soil depth show physiological differences that adjust P acquisition to P availability in soil. It is further unclear whether differences in fungal assemblages and soil structures affect competition for P of beech with roots of understory plants and soil microbes. The present study was undertaken to investigate these open points.
The aims of this study were to quantify the contributions of mycorrhizal and non-mycorrhizal root tips to Pi acquisition in the organic layer and mineral top soil of a P-poor and a P-rich beech forest and to elucidate the competition between beech roots, microbes and other plants roots for P uptake. To address these goals, we excised intact soil cores from two well-characterized beech forests, which constitute the high-P (HP) and low-P (LP) ends of a geosequence in Central Europe (Germany), previously characterized by Lang et al. (2017). We irrigated the soil cores with 33P, traced the distribution of the label and measured the P contents in the soluble and insoluble fractions of bulk soil, rhizosphere, beech roots, mycorrhizal and non-mycorrhizal root tips, other roots, and microbes in the organic layer and mineral topsoil. As a methodological innovation, we determined the bioavailability of Pi not only in bulk but also in rhizosphere soil. We tested the following hypotheses: (i) the main P source for beech P supply is the mineral topsoil under P-rich and the organic layer under P-poor conditions. (ii) Mycorrhizal root tips exhibit different P efficiencies, thereby counteracting low P availability by relatively higher P uptake than under high P availability; the contribution of non-mycorrhizal root tips to P uptake is low. (iii) Under high P availability, beech roots do not compete with understory or microbes for Pi, whereas the competition is strong under low P availability.
Materials and Methods
Site Characteristics
Two beech (F. sylvatica L.) forests, which differ strongly in soil properties and P stocks (Supplementary Table S1) were selected for this study: Lüss, a low-P (LP) and Bad Brückenau, a high-P (HP) site (Zavišić et al., 2016; Lang et al., 2017). Briefly, the LP forest is located in the district Celle in Lower Saxony (R: 3585473 E, H: 5857057 N; 115 m above sea level) and stocked with about 132-year-old beech trees (Haußmann and Lux, 1997). The soil type is sandy-loam from silicate substrate, containing 164 g P m–2 to a depth of 1 m (Lang et al., 2017). The mean annual temperature was 8.0°C and the mean annual sum of precipitation 730 mm. The HP forest is situated in the biosphere reservation ‘Bayerische Rhön’ (R: 3566195 E, H: 5579975 N; 801 to 850 m above sea level). The mean long-term sum of annual precipitation was 1000 mm and the mean annual temperature 5.8°C. The soil type is basalt of volcanic origin, containing 904 g P m–2 to a depth of 1 m (Lang et al., 2017). The average age of the beech stand is 137 years (Haußmann and Lux, 1997). Additional information on the research sites has been compiled in Supplementary Table S1.
Collection of Soil Cores
We collected intact soil cores in the HP (27.6.2017) and the LP (19.6.2017) forest, respectively, using PVC pipes of 120 mm diameter and a length of 200 mm. Each pipe was hammered completely into the soil at a distance of about 0.2 m to a beech tree and was pulled up containing the soil and forest floor. The soil cores remained in the PVC pipes. They were immediately transported to laboratory, kept at room temperature, weighed, watered with 100 ml of tap water, and used the next day for labeling (n = 5 per forest). Soil cores of the same dimensions (n = 5 per forest) for mycorrhizal inspection were harvested on the same plots about 2–3 weeks later in the same manner.
Radioactive Labeling and Harvest
We used the soil cores for uptake studies of 33P into roots. Pretests with soil cores collected in the same manner in 2015 showed that the roots were still active exhibiting similar uptake of the label as roots of intact plants (Spohn et al., 2018). We labeled five soil cores per forest by addition of 1850 kBq of H333PO4 (Hartmann Analytic GmbH, Brunswick, Germany) in 40 ml of tap water to each soil core. The added 33P corresponded to 1.66 ng P per soil core. Each soil core was subsequently watered with 40 ml tap water to distribute the radioactive marker throughout the soil core. To avoid loss of label by through-flow a plastic saucer was placed underneath each soil core. Any through-flow was returned to the soil core. After 24 h incubation in the laboratory at 20°C, the soil cores were pushed out of the pipe and were immediately separated in organic and mineral topsoil. Each sample was further fractionated in bulk soil, rhizosphere soil, beech roots (fine roots: < 2 mm, coarse roots: > 2 mm), other roots (distinguished by color and surface structure, Hölscher et al., 2002), and residual materials (litter, fruits, twigs, and stones).
We defined rhizosphere soil as the soil adhering to roots. Our rhizosphere effectively represents the mycorrhizosphere since fungal hyphae also contribute to soil adherence (York et al., 2016) but for consistency, the term rhizosphere was used throughout this study. The rhizosphere soil was collected by streaking the adhering soil from the roots with a paintbrush. Afterward, fresh fine roots were cut into pieces of similar lengths and yielded one mixed sample per soil layer and soil core, which was immediately weighed. All other fractions were also immediately weighed. Well-mixed aliquots of soil and plant fractions were dried at 40°C for 1 week, weighed, and used for analyses of the dry mass per soil layer and plant fraction.
Analyses of Root Tips and Mycorrhizal Species
Approximately half of the fresh beech fine roots, extracted from each soil core, were used to determine the number of root tips and mycorrhizal colonization. The sample was weighed and the number of root tips in this sample was counted under a stereomicroscope (Leica M205 FA, Wetzlar, Germany). The total number of root tips per soil layer was calculated as:
Equation 1
Root tips were categorized according to their visual appearance as vital ectomycorrhizal, vital non-mycorrhizal or dry (Winkler et al., 2010). Mycorrhizal root tips and non-mycorrhizal root tips were collected separately from each sample. The mean weight of mycorrhizal and non-mycorrhizal root tips was determined by collecting 100 and 200 root tips, respectively, and weighing the sample after drying (40°C for 1 week, followed by freeze-drying for 4 days and equilibration at room temperature in a desiccator with silica gel). For the analyses of P and 33P, mycorrhizal root tips were collected for each soil layer and forest type separately. The mycorrhizal samples represented a mixture of morphotypes. Mycorrhizal colonization and root vitality were calculated as:
The abundance of different morphotypes was determined under the stereomicroscope (Leica MF205) using a simplified identification key (after Agerer, 1987–2012) as described by Pena et al. (2010). For mycorrhizal species identification, morphotypes of all species were collected, which comprised at least three root tips per sample. Mycorrhizal species identities were determined after DNA extraction and ITS sequencing using the protocol of Pena et al. (2017).
Determination of Total and Soluble Phosphorus in Roots and Soil
Dry soil and coarse and fine root samples were milled in a ball mill (Retsch MN 400, Haan, Germany) to a fine powder. For determination of total P (Ptot), about 50 mg of the powder was weighed and extracted in 25 ml of 65% HNO3 at 160°C for 12 h according to Heinrichs et al. (1986). For Ptot determination of root tips, dry root tips, either mycorrhizal or non-mycorrhizal, with weights ranging from 0.2 to 30 mg were weighed and extracted in 2 ml of 65% HNO3 at 160°C for 12 h according to Heinrichs et al. (1986). For determination of soluble P (Psol) about 100 mg of plant or soil powder was extracted in 150 ml of Bray-1 solution (0.03 N NH4F, 0.025 N HCl) for 60 min on a shaker at 180 rpm (Bray and Kurtz, 1945). Microbial P was determined using the chloroform fumigation extraction method according to Brookes et al. (1985). About 5 g organic layer or 10 g mineral topsoil were fumigated for 24 h with chloroform (CHCl3). The fumigated samples were extracted for 60 min on a shaker at 180 rpm in 150 ml Bray-1 solution. After P measurement (described below), Pmic was calculated according to Brookes et al. (1982):
All extracts were filtered through phosphate free filter paper (MN 280 1/4, Macherey-Nagel, Düren, Germany). The Ptot and Bray-1 extracts were used for elemental analysis by inductively coupled plasma–optical emission spectroscopy (ICP-OES) (iCAP 7000 Series ICP–OES, Thermo Fisher Scientific, Dreieich, Germany). P was measured at the wavelength of 185.942 nm (axial) and calibrated with a series of dilutions (0.1 to 20 mg l–1) using the element standard for P (Einzelstandards, Bernd Kraft, Duisburg, Germany).
Determination of 33P by Scintillation Counting
The Ptot and Bray-1 extracts of all soil, plant and root tip samples were used to measure 33P. For this purpose, 3 ml of plant or soil extract or 1.5 ml of root tip extracts were mixed with 10 ml of scintillation cocktail (Rotiszint eco plus, Roth, Karlsruhe, Germany). The mixture was used for detection of 33P signals (DPM, disintegrations per minute) in a PerkinElmer scintillation counter (Tri-Carb TR/SL 3180, Waltham, MA, United States). The signal was corrected for the 33P half-life of 25.34 days using QuantSmart (version 4.00, PerkinElmer) to take the decay time between harvest and measurements into account. A background correction value was subtracted from all samples, which was obtained by measuring only scintillation cocktail and extraction solution. 33P concentrations in different fractions were expressed as 33P (Bq g–1 dry mass):
To determine the whole amount of 33P in a distinct soil or plant compartment (whole amount = WA), we multiplied the 33P concentration (Eq. 6) with the total mass of that compartment, for example for fine roots (FR):
The WA of 33P in coarse roots (CR), other roots, rhizosphere soil (Rhizo) in the organic layer (OL), rhizosphere soil in the mineral layer (ML), in bulk soil (Bulk) in the organic layer and in bulk soil in the mineral layer were determined correspondingly.
Calculation of P Uptake
Irrigation with 33P solution (33Ptot = total 33P) leads to distribution of 33P between soil solution (soluble 33P = 33Psol) and absorption to soil particles (bound 33P = 33Pbound). Furthermore, only a fraction of 33P will percolate through the organic layer into the mineral top soil, where it can be expected to result in another distribution factor between soil solution and soil particles than in the organic layer. As the consequence, 33Psol will vary depending on soil layer and forest type. Similarly, we expect that soluble P (Psol) present in our soil cores will also vary between soil layers and forest type. Therefore, the dilution of 33P in the P concentration of the soil will vary among different soil layer and forest types, resulting in different specific activities. We determined the specific activity of 33Psol (spec 33Psol) for each soil compartment separately using the P and 33P concentrations of the Bray-1 solution (Supplementary Table S2):
We used the specific activities to determine P uptake and enrichment in plant roots, microbes and mycorrhizal and non-mycorrhizal tips after 1 day using the whole amounts of these fractions in different soil layers (cv. Eq. 7) and the corresponding specific activities (cv. Eq. 8). The specific activity of 33Psol in the bulk soil was used to calculate P uptake of microbes separately for the organic and the mineral layer:
We used the specific activity of the rhizosphere to calculate P uptake of beech roots because roots absorb nutrients mainly from the rhizosphere (York et al., 2016):
We calculated P uptakebeech separately for the mineral (ML) and organic layer (OL). With P uptakebeech in OL and in ML, we calculated the relative contribution of each soil layer to P uptake of beech roots:
The relative P uptake in the mineral layer was determined correspondingly.
Mycorrhizal (EM) and non-mycorrhizal (NM) root tips take up nutrients for their own metabolism and translocation to the host. The 33P label in the tips should therefore not be termed “uptake” but represents enrichment of new P (ENP) as the result of P metabolism in the mycorrhiza and host allocation at a distinct time point, as exemplified previously for nitrogen (Pena and Polle, 2014; Leberecht et al., 2016). To calculate ENP in EM root tips, we used the 33P concentration in root tips (Eq. 6), the specific activity of 33Psol in the rhizosphere (Eq. 8) and the mass of an individual EM root tip:
ENP of non-mycorrhizal root tips was calculated accordingly. We determined and used the following masses for roots tips: 1 EM root tip in HP = 18.35 μg, in LP = 15.68 μg, and 1 NM root tip = 8.84 μg in both HP and LP.
To determine the whole amount of new P (WA ENP) in all mycorrhizal root tips of a distinct layer, we used EMP (Eq. 12) and the total number of root tips in that layer (Eq. 1):
WA ENP for EM in the mineral layer and for the non-mycorrhizal root tips in those layers were calculated correspondingly. With these values we calculated WA ENP in all root tips of the whole soil core:
Using the enrichment of new P of a distinct fraction of root tips (according to Eq. 12) and the total enrichment of new P in all root tips of the soil core (Eq. 13), we determined the relative enrichment of new P in distinct soil layer and types of root tips:
The relative enrichments of new P in EM of the ML and in NM root tips per soil layer were determined correspondingly.
Calculations of Relative Competition Intensities (RCI)
According to Grace (1995) the dynamics of mixtures can be accurately determined by the relative competition intensity. We adapted the concept originally used to compare the performance of plants in different ecological settings (Grace, 1995) to calculate the competition intensities for P between beech roots and microbes, or beech roots and other roots in our soil cores or beech roots and all competitors (= microbes + other roots). In line with Grace (1995), we reasoned that new P could end in any of these fractions and the relative amount of new P in beech roots compared to other fractions indicates their competitive strengths:
RCIbeech versus other roots and RCIbeech versus all competitors were calculated accordingly. Negative values of RCI indicate competition; positive values or values that do not deviate from zero indicate no competition. To test whether the values deviated from zero, we used a non-parametric signed rank test. We considered p-values < 0.05 to indicate significant effects.
Statistical Analyses
Data are shown as means (n = 5 to 8 per soil layer and forest type ± SE), if not indicated otherwise. Statistical analyses were conducted with R version 3.6.0 (R Core Development Team, 2012). Normal distribution and homogeneity of variances were tested by analyzing residuals of the models and performing a Shapiro–Wilk test. Data were logarithmically transformed to meet the criteria of normal distribution and homogeneity of variances, where necessary. General linear models were used to test the factor “Forest type” with the two layers (OL and ML) as random factors; otherwise analysis of variance (ANOVA) was used. Differences between means were tested by a post hoc test Tukey HSD (package: ‘multcomp’). Means between the two forest types were compared with Student’s t-test. Means were considered to be significantly different from each other when p ≤ 0.05. For relative values, a generalized linear model using Poisson distribution was used and data were considered to be significantly different from each other when p ≤ 0.05. To detect linear relationships between variables, Pearson’s correlation coefficient was determined.
For the analyses of diversity data (count data for mycorrhizal species), we used PAST version 3.22 (Hammer et al., 2001) to determine mean species abundance and to test differences in the fungal assemblages by a two-way PERMANOVA for the factors: forest type and soil layer. Bray–Curtis was used as the similarity measure.
Results
Biomass, Total P and 33P Distribution in Soil, Roots, and Microbes Differ Between Soil Layers of P-Rich and P-Poor Soils
One day after irrigation of intact soil cores from a HP and an LP forest with 33P, we determined the masses of soil and root fractions (Figures 1A,B), the amounts of bound and soluble P (Figures 1C,D), and the amounts of 33P present in the different fractions (Figures 1E,F, complete statistical information: Supplementary Tables S2, S3). Bulk soil was always the largest mass fraction, followed by residual materials (leaf litter and twigs in the organic layer and a large stone fraction in the HP mineral soil), and rhizosphere soil (Figures 1A,B). LP contained more rhizosphere soil in the organic layer than HP (p = 0.028), probably because the biomass of beech fine roots, from which the adhering soil was collected, was higher in the organic layer at LP than at HP (p < 0.001) (Figures 1A,B and Supplementary Table S2). Beside beech roots, the soil cores also contained roots from other plant species in both layers (Figures 1A,B). Other roots were more abundant than beech roots in the organic layer at HP (p = 0.014), while they constituted a smaller fraction to that of beech roots in the organic layer at LP (p < 0.001) (Figures 1A,B). In the mineral layer, beech roots strongly dominated over other roots at HP (p = 0.002) and were mostly absent at LP (p < 0.001) (Figures 1A,B).
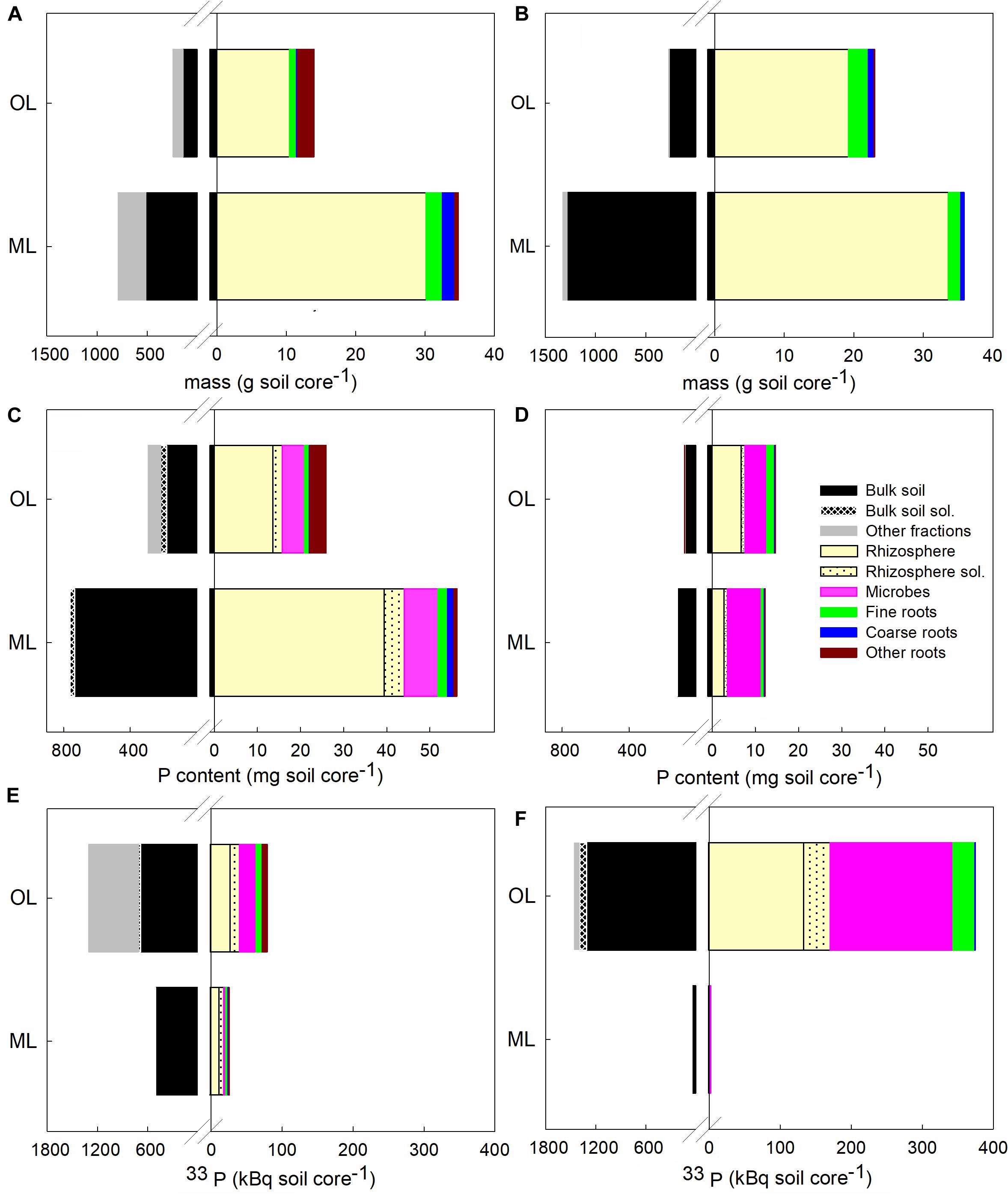
Figure 1. Distribution of biomass, P content and 33P activity in intact soil cores 24 h after label application. Soil cores were obtained from P-rich (A,C,E) and P-poor (B,D,F) forests. Soils from the organic layer (OL) and the mineral topsoil (ML) were analyzed separately. Data indicate means (n = 5). Standard errors and statistical information is shown in Supplementary Tables S2, S3. Colors of bars refer to bulk soil (black) with potential plant available Psol (white hatched), residual materials (gray), rhizosphere soil (light yellow) with potential plant available Psol (dotted), microbes (purple), fine roots (green), coarse roots (blue), and other roots (dark red).
As expected (Lang et al., 2017), the vast majority of total P was sequestered in bulk soil and the amounts of P present in most of the analyzed fractions were higher at HP than at LP (Figures 1C,D and Supplementary Table S3). Still, we noted some exceptions: the total amounts of P bound by beech fine roots (p = 0.231), beech coarse roots (p = 0.522), and microbes (p = 0.114) in the organic layer of LP were similar to those at HP. In the mineral layer of LP, the amount of P in microbes was even higher than that of HP (p = 0.003). The Psol content of the rhizosphere, which we considered as the potentially plant-available P pool, was higher at HP than at LP in both soil layers (organic layer: p < 0.001, mineral layer: p < 0.001) (Figures 1C,D).
To investigate the fate of newly added Pi, we studied the distribution of 33P 1 day after labeling. The majority of the label was sequestered in bulk soil and was not soluble in mild acid at HP (p < 0.001) and LP (p < 0.012), suggesting that it was immediately removed from the easily available bioactive pool (Figures 1E,F). Furthermore, the distribution of the added 33P showed interesting differences between the organic layer and the mineral top soil at HP and LP (Figures 1E,F). At LP, only a relatively small fraction of the added 33P percolated into the mineral soil (Figure 1F, p < 0.001), while at HP, the organic layer including microbes and rhizosphere retained less 33P, resulting in a higher through flux of 33P into the mineral layer (Figure 1E). These distribution patterns also influenced the specific activities of 33Psol in the rhizosphere (Table 1), resulting in the highest specific activity in the organic layer and the lowest in mineral soil at LP, while HP assumed intermediate specific 33P activities (Table 1). Overall, the recovery of applied 33P was at 84.7% ± 5.1 at HP and 99.2% ± 0.4 at LP (calculated with the data in Supplementary Table S3). It is likely that the lower recovery at HP was caused by the stone fraction in the mineral layer, which could not be measured, but probably also absorbed a portion of the added 33P.
P Uptake and P Distribution in Beech Roots Differ Between Soil Layers in P-Rich and P-Poor Forests
The specific activities of 33Psol in rhizosphere per forest type and soil layer (Table 1) were used to estimate P uptake of beech roots in each layer separately. To compare the importance of soil layers for P supply at HP and LP, we normalized the data relative to P uptake of beech roots (Eq. 11 under materials and methods). We found that beech roots in our soil cores acquired about 75% of P from the mineral soil and 25% from the organic layer at HP, whereas beech roots at LP took up about 60% P from the organic and 40% from the mineral soil (Figure 2). These figures corresponded well to the distribution of total P in beech roots between the organic layer and mineral soil at HP (25% and 75%, respectively) and at LP (69% and 30%, respectively, calculated with the data in Supplementary Table S3).
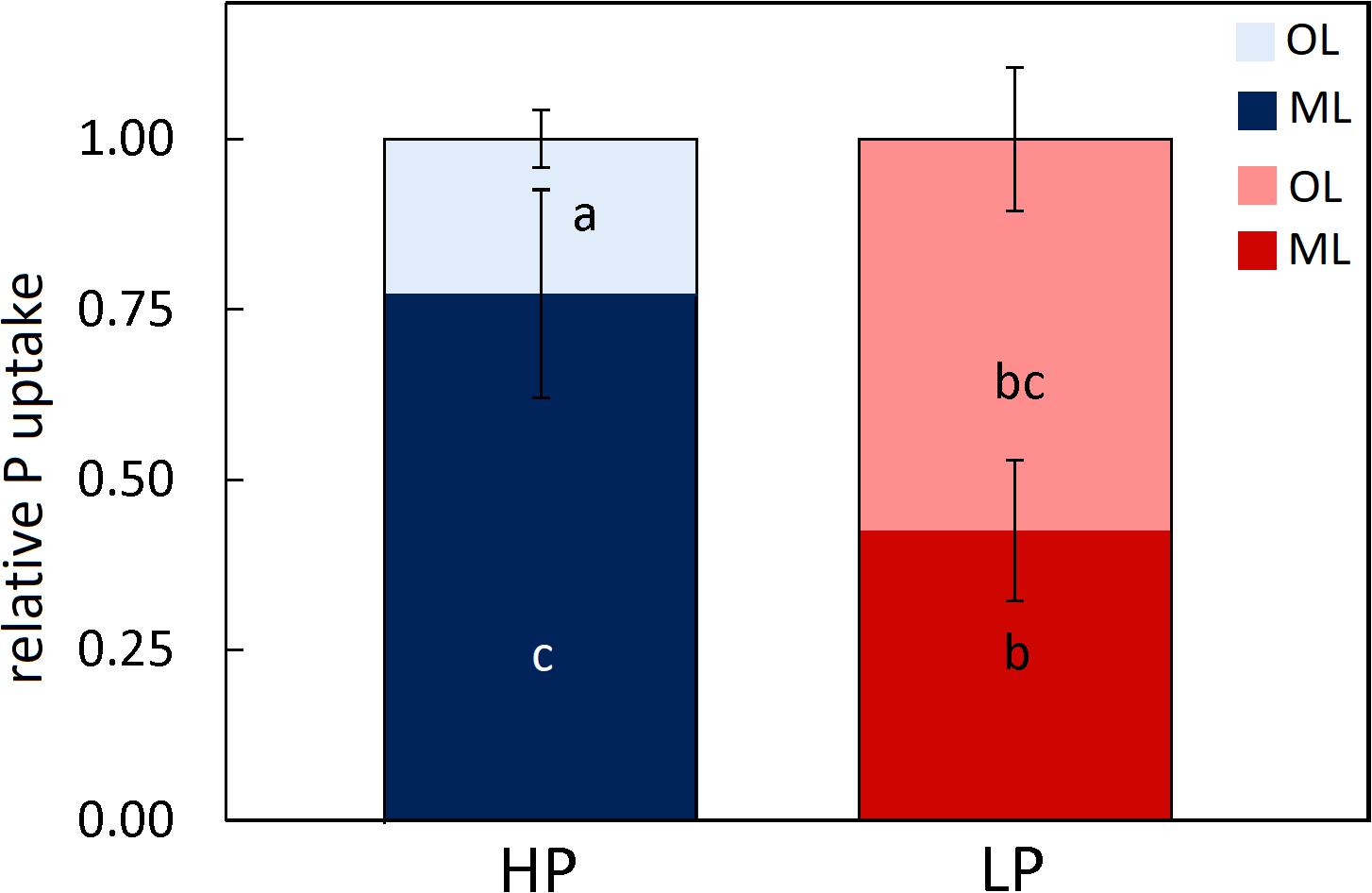
Figure 2. Relative P enrichment in beech roots in the organic layer and mineral layer topsoil of beech (Fagus sylvatica L.) forests. Intact soil cores were collected in a P-rich (HP: blue) and a P-poor (LP: red) forest, irrigated with 33P artificial soil solution and roots from the organic layer (light blue, light red) and the mineral topsoil (dark blue, dark red) were analyzed separately after 24 h of labeling. To determine P root uptake, the rhizospheric 33P dilutions per forest and layer were used as described under materials and methods. Data indicate means (n = 5 ± SE). Differences among means per forest and layer were tested by GLM using Poisson distribution. Different letters indicate significant differences at p ≤ 0.05.
Mycorrhizal P Efficiencies Differ Between Soil Layers in P-Rich and P-Poor Soil
Regardless of forest type and soil layer, 96% of the vital root tips were colonized by ectomycorrhizal fungi (Table 2). In soil cores from HP about 50% of the root tips were present in the organic layer and the other 50% in the mineral soil, whereas at LP 75% of the root tips were present in the organic layer and 25% in the mineral soil (Table 2). In HP soil, the P concentrations of mycorrhizal root tips were similar to those of non-mycorrhizal root tips in HP soil and did not differ between soil layers (Figure 3A). In LP soil, the P concentrations of mycorrhizal root tips were higher than those in HP soil (p = 0.002), whereas those of non-mycorrhizal root tips were by far lower than those of non-mycorrhizal root tips in HP soil (p = 0.015, Figure 3A).
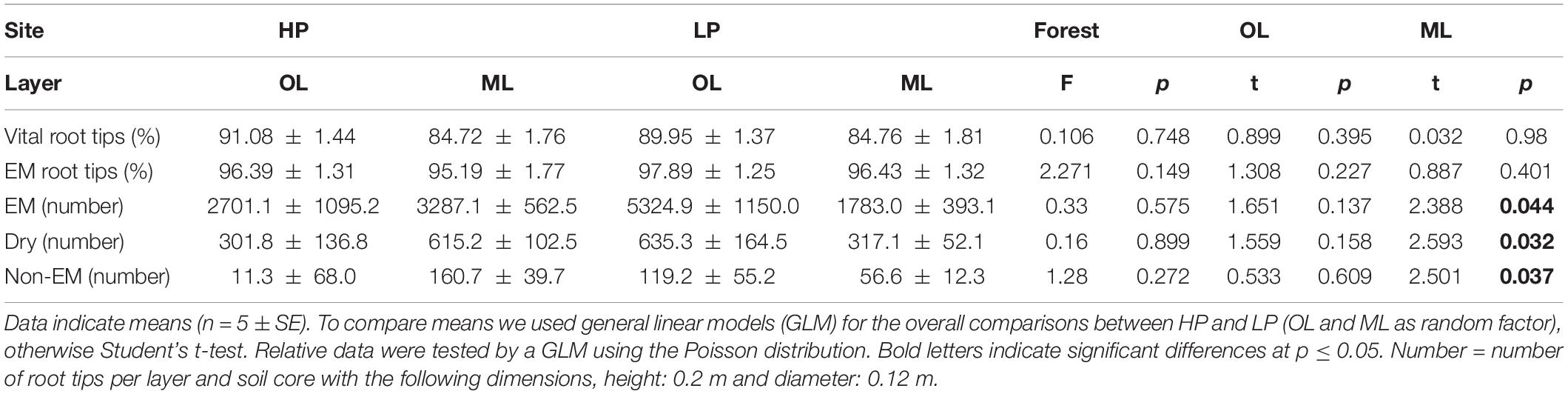
Table 2. Vitality, ectomycorrhizal (EM) colonization and number of beech root tips (soil core–1) in the organic layer (OL) and mineral topsoil (ML) from a P-rich (HP) and P-poor beech (Fagus sylvatica) forest.
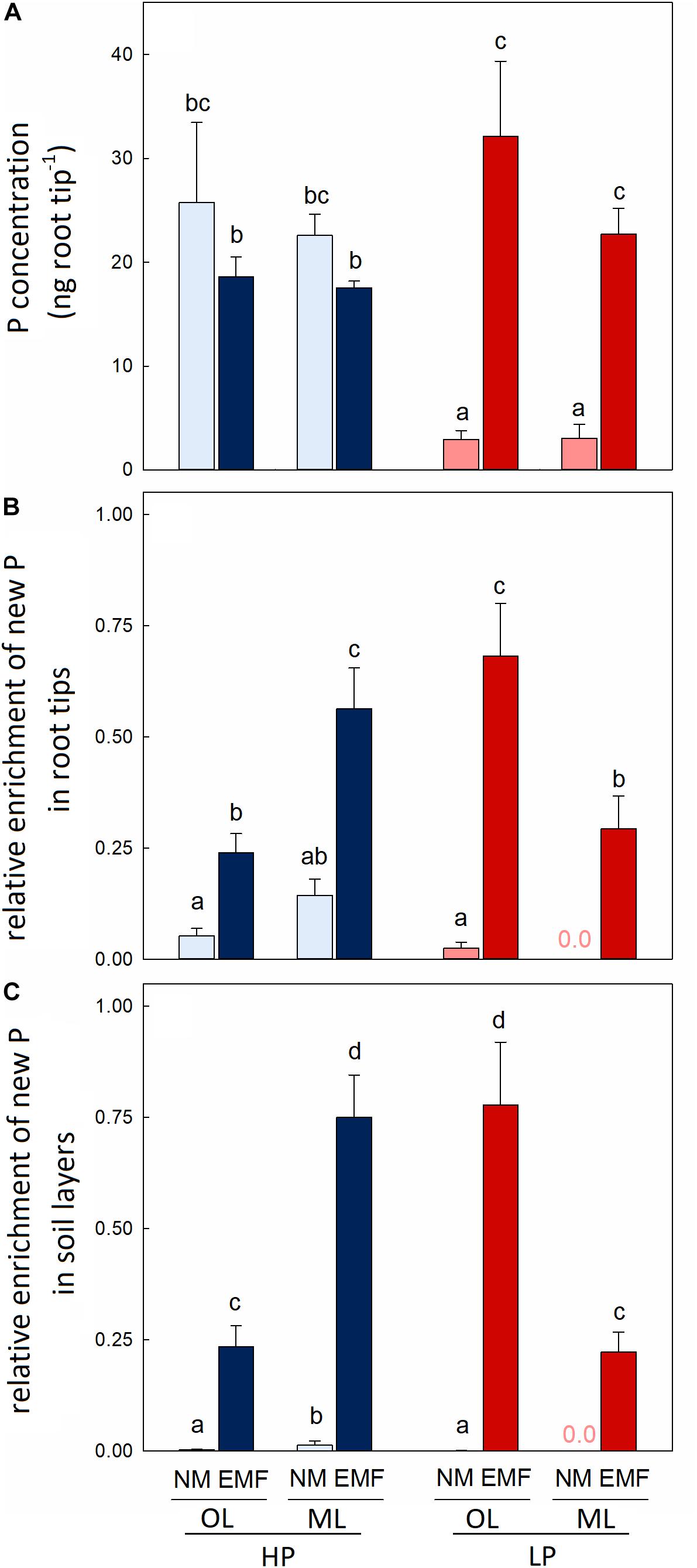
Figure 3. P concentration (A), relative net enrichment of new P in single root tips of beech trees (Fagus sylvatica L.) (B) and the relative net enrichment of new P in the sum of mycorrhizal and non-mycorrhizal root tips per forest type and soil layer (C). Intact soil cores were collected in a P-rich (HP: blue) and a P-poor (LP: red) forest, irrigated with 33P artificial soil solution and mycorrhizal (EMF) and non-mycorrhizal (NM) root tips from the organic layer (light blue, light red) and the mineral topsoil (dark blue, dark red) were analyzed separately after 24 h of labeling. To determine P enrichment, the rhizospheric 33P dilutions per forest and layer were used as described under materials and methods. Data indicate means (n = 5 ± SE). Differences among means per forest and layer were tested by three-way-ANOVA and Tukey HSD post hoc test (A) or by GLM using Poisson distribution (B,C). Different letters indicate significant differences at p ≤ 0.05.
To test whether these differences were caused by differences in the abilities for P acquisition in different soil layers, we determined the 33P enrichment of mycorrhizal and non-mycorrhizal root tips. We calculated the relative enrichment of new Proot tip with the specific activities of 33P in the rhizosphere of each soil layer and forest type (Table 1) as described under materials and methods (Eq. 12). The relative enrichment of new P per root tip indicates a mean value for the ability of the mycorrhizal root tips in that soil layer to acquire P (Eq. 15). We define this ability as mycorrhizal P efficiency. P accumulation in non-mycorrhizal root tips was treated correspondingly. The relative accumulation of new P per non-mycorrhizal root tip was low and did not differ between HP and LP (Figure 3B). In the mineral soil of LP, where only very little of the total added 33P was present (Figure 1F), non-mycorrhizal root tips showed no measurable 33P enrichment (Figure 3B). In contrast to non-mycorrhizal root tips, the relative enrichment per mycorrhizal root tip showed significant differences between soil layers and forest types (Figure 3B). In the organic layer, mycorrhizal P efficiency (per root tip) was higher at LP than at HP (Figure 3B). In the mineral layer, mycorrhizal P efficiency (per root tip) was lower at LP than at HP (Figure 3B). These differences were even more pronounced when the P enrichment in root tips was up-scaled to the whole soil core (Figure 3C). At HP, mycorrhizal root tips of the whole root system in the mineral layer exhibited 70% of P enrichment, followed by mycorrhizal root tips of the organic layer (26%), whereas non-mycorrhizal root tips of the mineral layer accumulated only about 4% and of the organic layer less than 0.1% (p < 0.001) of the total new P in root tips. At LP, mycorrhizal root tips of the whole root system in the organic layer contributed most to relative P accumulation in root tips (78%), followed by mycorrhizal root tips in the mineral layer (22%), whereas non-mycorrhizal root tips contributed less than 0.1% to P accumulation in root tips in the organic layer and nothing in the mineral layer (p = 0.003, Figure 3C).
If the enrichment of mycorrhizal root tips with new P was an indicator for root P supply, we expected that beech root P uptake is correlated with the abundance of mycorrhizal root tips and their specific enrichment with new P. In accordance with this suggestion, we found a strong relationship between the total amount of new P accumulated in all root tips of a soil layer and the estimated amount of new P taken up by beech roots in that layer (Figure 4). We did not find a correlation between the number of root tips and P uptake (R = 0.332, p = 0.164) indicating that the P efficiencies of mycorrhizal root tips are decisive for beech P acquisition.
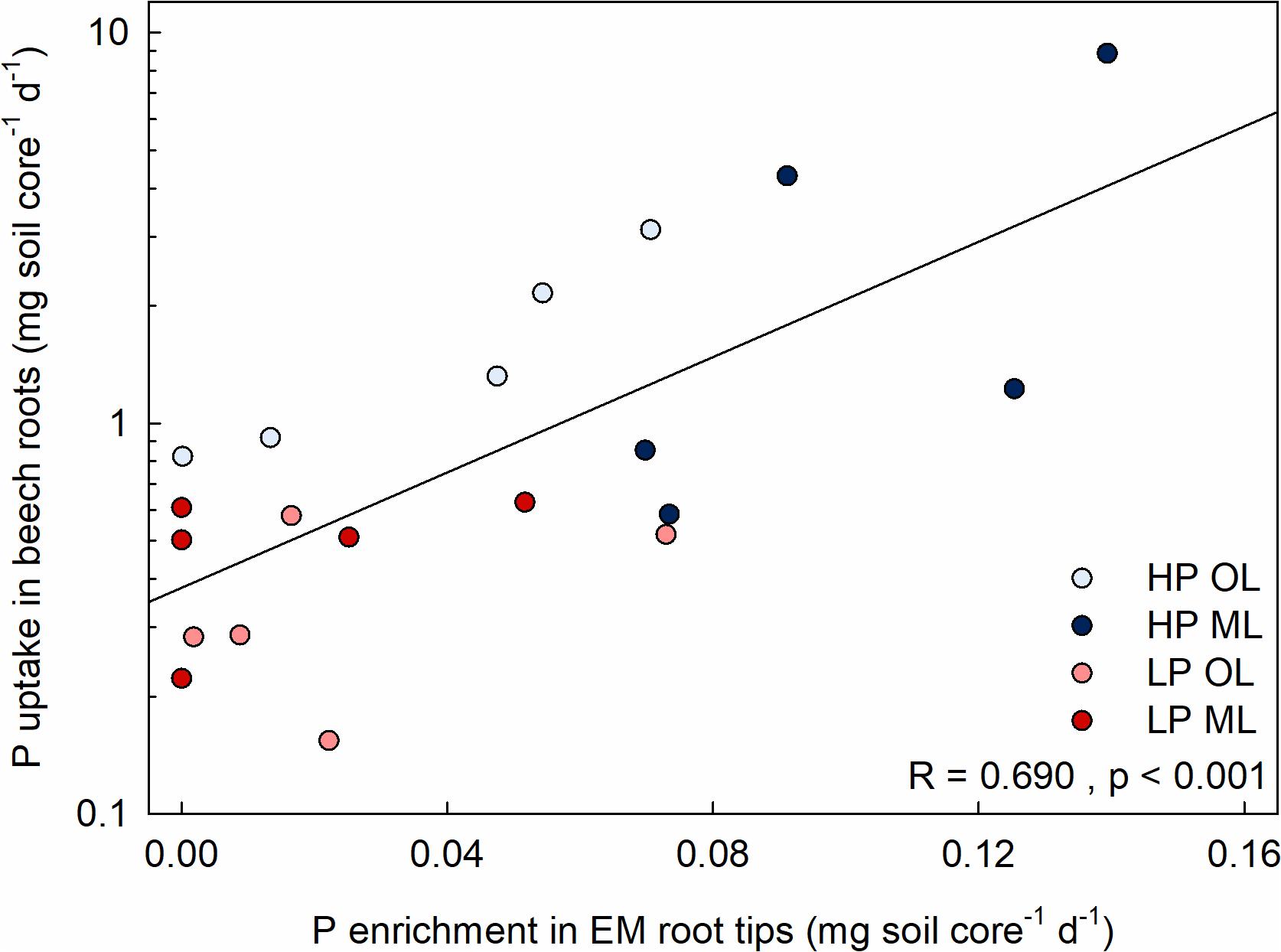
Figure 4. Relationship between the P enrichment in mycorrhizal (EM) root tips and P uptake of beech roots. Symbols indicate individual measurements in soil cores (diameter 0.12 m, height: 0.2 m) from a P-rich (HP) and a P-poor forest (LP) in the organic layer (OL) and in mineral top soil (ML). P uptake was determined 1 day (d) after application of 33P in artificial soil solution using the specific activities for 33Psol/Psol in the rhizosphere (Table 1) as described under materials and methods. Regression analysis was done determining Pearson correlation coefficient.
Mycorrhizal Community Structures Differ Between the HP and LP Forests but Not Between the Soil Layers
We investigated whether the observed differences in P acquisition were accompanied by differences in the mycorrhizal communities. Roots in HP and LP forests were colonized by different fungal assemblages (PERMANOVA, p(forest) = 0.012). Ascomycota (Hyaloscyphacae, Cenococcum geophilum) were more abundant in LP soil, whereas Basidiomycota of the orders of Russulales and Boletales (several Lactarius sp., Xerocomellus sp.) were more abundant in the HP soils (Figure 5). However, there were some exceptions, Lactarius blennius and Lactarius tabidus occurred only in the LP forests, whereas Genea hispidula was only found the HP forest (Figure 5).
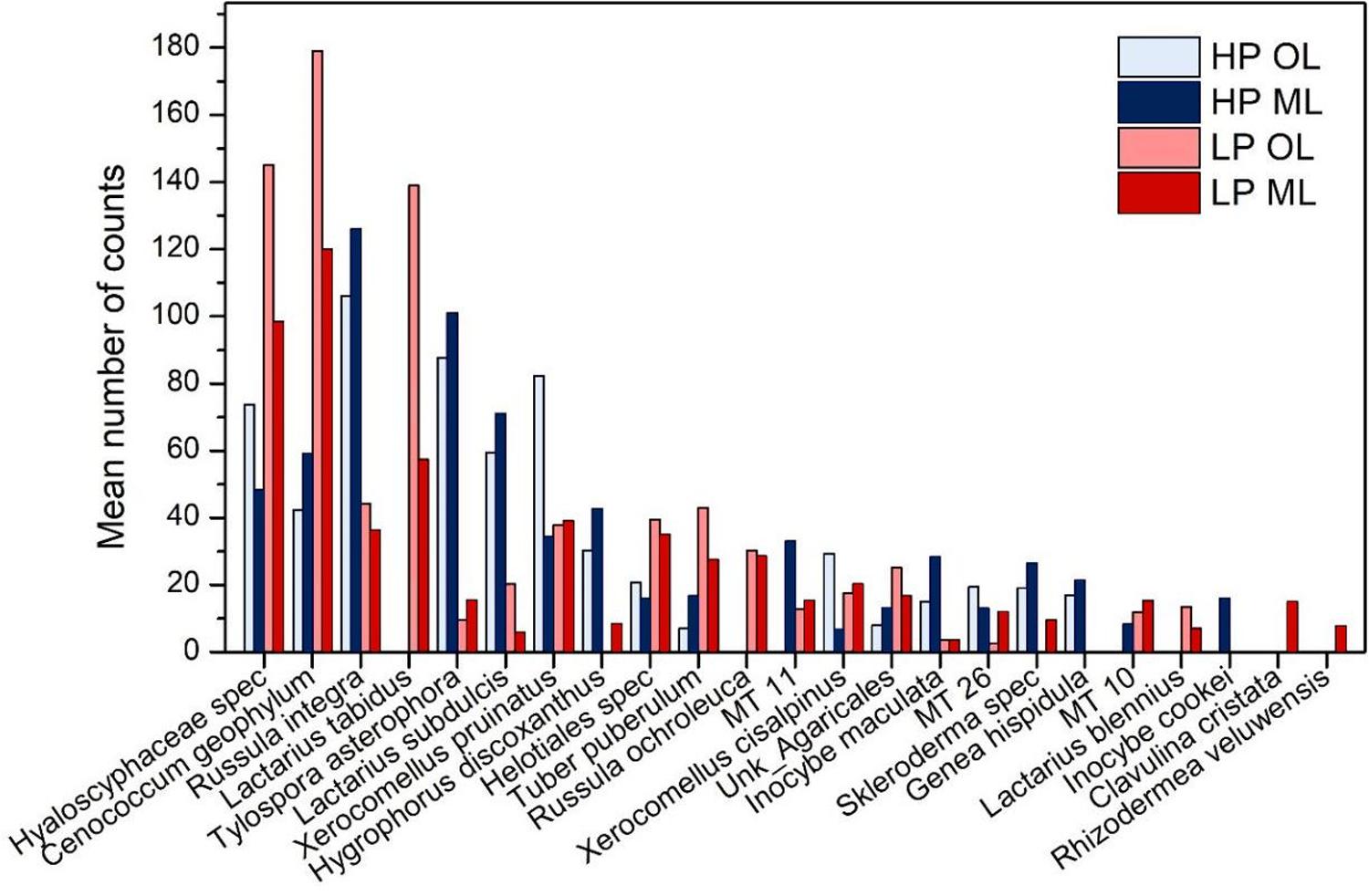
Figure 5. Ectomycorhizal species colonizing beech (Fagus sylvatica) roots in P-rich (HP: blue) and P-poor (LP: red) forest soil. Species were analyzed by morphotyping and ITS sequencing of the fungal morphotypes. Supplementary Table S4 shows additional information on species identification. Roots from the organic layer (OL, light blue, light red) and the mineral topsoil (ML: dark blue, dark red) were analyzed separately.
We further analyzed mycorrhizal community strutures in different soil layers. Clavulina cristata and Rhizodermea veluwensis occurred only in LP mineral (Figure 5) but these species were rare. Overall, no significant differences between the fungal assemblages in the organic layer and mineral top soil were detected (PERMANOVA, p(soil layer) = 0.747).
Competition of Beech Roots, Understory Roots, and Microbes for P Resources in Different Layers in P-Rich and P-Poor Forests
We expected that low P availability would result in strong competition of microbes and other roots with beech roots (i.e., negative values for RCI) and that high P availability would prevent competition. In line with this assumption, we found that the RCI for the competition of beech with microbes together with other roots at HP were not smaller than zero and higher than those at LP (Figure 6). Other roots, despite their relatively high abundance in the organic layer at HP (Figure 1A), did not significantly affect the competition of beech roots for Pi (vertical lines in the bars for HP in Figure 6). At LP, other roots were too rare to affect the competition between beech roots and microbes. At LP, microbes outcompeted beech roots in both soil layers (Figure 6).
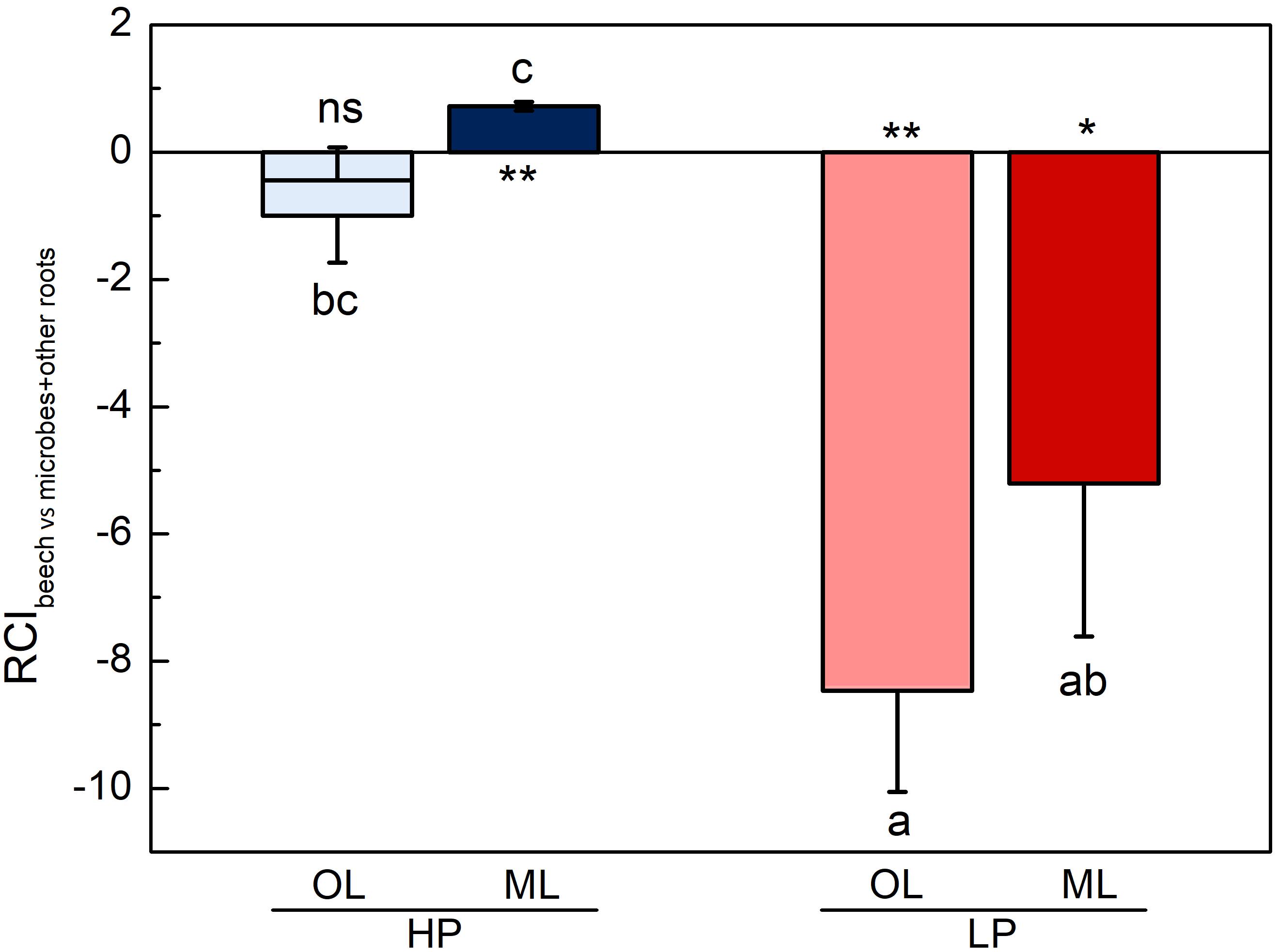
Figure 6. Relative competition indices (RCIs) for P uptake of beech (Fagus sylvatica) roots and their competitors (microbes + other roots) in P-rich (HP: blue) and P-poor (LP: red) forest soil. Analyses were conducted separately for the organic layer (OL, light blue, light red) and the mineral topsoil (ML: dark blue, dark red). Data show means (n = 5 ± SE per soil layer and forest type). Vertical line in bars show RCI for beech and other roots without microbes. Before ANOVA, data were normalized as follows: [log10((x−1)*−1)]. Significant deviation from zero are shown by different letters. To test for each variable, the non-parametric signed rank test was used and significant differences at p < 0.05 and p < 0.01 are indicated by * and **, respectively. ns = not significant.
Discussion
Soil Structure and Pi Availability Impact Beech Root P Uptake
In recent years, the concept of ecosystem nutrition has gained support, which proposes a gradual shift in nutritional strategies from P acquisition to P recycling when P soil stocks are decreasing from P-rich to P-poor forest sites (Lang et al., 2016). According to this model, we expected that bio-available P varied among soil layers of P-rich and P-poor forests, resulting in differences for root P supply. Using undisturbed soil horizons for P tracing, our results support our first hypothesis and comply with previous experiments in mesocosms with young beech plants (Brandtberg et al., 2004; Jonard et al., 2009; Hauenstein et al., 2018; Spohn et al., 2018; Zavišić and Polle, 2018). It should be noted that our approach might have some limitations because (i) the roots were severed at the edge of the soil cylinders and (ii) only the upper portion of the whole-rooted soil profile was included. Firstly, excised fresh roots are still highly active in P uptake and translocation (Noggle and Fried, 1960; Franklin, 1969; Mejstrik and Benecke, 1969; Rubio et al., 1997). Furthermore, P root uptake in the present study corresponded well to aboveground P translocation in young intact beech trees (Yang et al., 2016; Hauenstein et al., 2018; Zavišić and Polle, 2018). Therefore, we have no evidence for confounding effects of root damage in our experimental set-up. Secondly, based on our experimental unit “soil core,” we estimated a contribution of 25-to-75% of the organic layer-to-mineral layer to P root supply in the P-rich forest. Since a larger root fraction is present below the forest floor and the A horizon in HP (about 80%) than in LP soil (30%, Lang et al., 2017), the ratios for the contribution of soil layers to beech P supply may further diverge in favor of the mineral layer at HP. Our results, thus, underpin an at least twice higher contribution of the organic layer to root P acquisition (60-to-40%) under P-poor conditions. This result strongly supports that the organic layer is critical for P nutrition under P-poor conditions (Lang et al., 2017; Hauenstein et al., 2018) and underpins that our comparison of two sites concurs with field studies along a larger P gradient (Lang et al., 2017).
We demonstrated that the function of the organic layer for root P supply occurred already at a short time scale of 1 day. One reason for this finding is probably that the sorption capacity for different P species is lower in the organic layer in P-poor than in P-rich soil (Lang et al., 2017), thus, resulting in relatively higher Pi bioavailability. Our results show that microbes benefited strongly but roots also obtained a higher share of P than one would expected on the basis of the low P stock in the P-poor forest. As the consequence of high plant and microbial activities (this study; Bergkemper et al., 2016; Hofmann et al., 2016) the P retention of the organic layer was high, despite its lower sorption capacity (Lang et al., 2017).
Our estimation of the relative P partitioning in roots in different layers and soil types was based on the premise that P uptake into roots occurs from the rhizosphere and therefore, we used the rhizospheric dilution of the P tracer for the estimation of root P uptake. This is a novel approach since previous studies considered either the dilution of added P in pooled soil samples (Jonard et al., 2015) or used more specifically tracer dilution in the organic layer and the mineral soil, respectively (Di et al., 2000; Hauenstein et al., 2018; Spohn et al., 2018; Zavišić and Polle, 2018). Our results indicate strong differences for soluble P and consequently for the specific dilution of the tracer (33P/mg Psol) between rhizosphere and bulk soil from the organic and mineral layers, respectively. The differences between bulk soil and rhizosphere might have been expected because plant roots and their associated mycorrhizal fungal symbionts exude organic acids, metal-chelating compounds and acid phosphatases that enhance solubilization of P (Leyval and Berthelin, 1993; Wallander, 2000; Casarin et al., 2004; Liu et al., 2007). These biotic activities result in an altered rhizosphere chemistry, in turn leading to higher P availability for mycorrhizal and root uptake (Wallander, 2000; Casarin et al., 2004; Liu et al., 2007). Consequently, we recommend that future studies should consider rhizospheric P availability when estimating root P uptake. Overall, our short-term tracer experiment with forest-grown roots in naturally stratified soil horizons highlight the prominent role of the organic layer for beech P nutrition under P poor conditions und uncovered heterogeneity for bioavailable P between rhizosphere and bulk soil in different soil layers and forest types.
Mycorrhizal P Efficiencies Regulate Beech P Uptake in Different Soil Layers but Do Not Prevent Competition With Microbes in P-Poor Soil
Roots of beech trees in temperate forests are usually almost completely colonized by a diverse spectrum of different mycorrhizal fungi (Courty et al., 2010; Pena et al., 2010, 2017). The taxonomic composition of ectomycorrhizal fungi differs between P-rich and P-poor beech forests (this study; Zavišić et al., 2016). Notably, the dominant ectomycorrhizal species present in both forest types were characterized by short hyphae of a few micrometers (short-distance exploration types) or by the absence of hyphae (contact types) (Weigt et al., 2012). Among the fungi in our study, only the Boletales, which were not very abundant (approximately 8% of the mycorrhizal root tips), produce long distance hyphae (Agerer, 1987) and may explore regions beyond the rhizosphere. Therefore, mycorrhizal P mobilization in P-rich and P-poor forests was mainly confined to the vicinity of roots. These considerations further underpin that for the assessment of P uptake by mycorrhizal root tips and plant roots, the specific activities of rhizospheric rather than those of bulk soil should be taken into account.
In our study forests, non-mycorrhizal root tips were rarely found and therefore were unimportant for gross host P provision. However, they provided insights into the benefits of P handling by mycorrhizal root tips in naturally composed fungal community. Drastically lower P concentrations in non-mycorrhizal root tips under low than under high P in soil highlight the superiority of mycorrhizas for P acquisition under P limitation. Although the beneficial influence of mycorrhiza for P provision is well-established (Nehls and Plassard, 2018), studies demonstrating mycorrhizal P facilitation in natural soils are scarce. An enhanced P enrichment of mycorrhizal root tips can be regarded as a measure for P efficiency. A relatively stronger enrichment with new P in mycorrhizal compared to non-mycorrhizal root tips, regardless of P availability in the soil, also indicated the predominance for P acquisition of mycorrhizal root tips. Despite lower P efficiency of non-mycorrhizal root tips in P-rich soil, their P concentrations, which are the result of more long-term processes, were similar to mycorrhizal root tips. This suggest that in contrast to P-limited conditions, mycorrhizal and non-mycorrhizal root tips do not compete for P under P-rich conditions.
An obvious question is whether differences in fungal community structures or differences in the regulation of P-uptake in the P-rich and P-poor forest were responsible for differences in plant supply. An ultimate answer to that question is not possible but our finding that similar fungal community structures in organic and mineral soil layers exhibited different P efficiencies suggests a strong impact of regulatory processes on P uptake. P-limitation leads to an increase in high-affinity P transporters to ensure P uptake, whereas under high P, low affinity transporters maintain P uptake (Wu et al., 2003; Desai et al., 2014; Kavka and Polle, 2016, 2017). Therefore, our initial hypothesis was that low P availability is counteracted by relatively higher P uptake efficiencies than under high P availability. However, in contrast to that assumption relatively higher P efficiencies of mycorrhizal root tips occurred in those soil layers that contributed most to P provision to roots and not in those with lower P availability. The most likely explanation for these findings is that both adaptedness of fungal assemblages to certain habitat conditions together with regulation of P supply in response to plant demand (Zavišić and Polle, 2018) were responsible for the observed root P supply. Since the abundance of root tips alone cannot predict root P uptake, it is obvious that the properties of the symbionts are essential for P acquisition. Here, any contribution of distinct taxa to P provision to the roots remained enigmatic because we used pooled samples. Previous studies have shown large differences among different fungal species for P translocation (Cumming, 1996; Brandes et al., 1998; Colpaert et al., 1999; Jentschke et al., 2001). Field studies in beech forests demonstrated high phosphatase activities in Lactarius sp., Russula sp., and Cenococcum sp. (Courty et al., 2005). These genera were also abundant in our investigation, suggesting a high potential to release Pi from organic material. In conclusion, the significant relationship between root P uptake and mycorrhizal P enrichment corroborates that mycorrhizal P efficiency governs P provision to the host.
Plant P supply is not only affected by the complex interactions of mycorrhizal properties and geochemical soil properties but usually also by competition with other biota (Curt et al., 2005; Zhu et al., 2016). When nutrients such as P are inadequately available in the soil of natural ecosystems to suffice plant and microorganism requirements, plants are thought to be inferior in the competition (Woodmansee et al., 1981) but some studies have found that plants compete successfully with soil microorganisms (Schimel and Bennett, 2004) or are even superior under specific circumstances (Wang and Lars, 1997). Our study did neither reveal competition between beech roots and microbes nor with other understory roots in P-rich soil, even though the biomass of other roots was considerable and the biomass of microbes about 10-fold higher in the P-rich than in the P-poor forest (Bergkemper et al., 2016; Lang et al., 2017). The absence of P competition in P rich soil underpins P sufficiency for biological processes. By contrast, the competition between beech roots and microbes was strong in P-poor soil and might have precluded the establishment of further understory species. An unexpected result was that the competition strength did not differ between organic and mineral soil layers. This observation was surprising since we anticipated lower competition in the organic layer with higher P availability than in the mineral soil where P availability was lower. Possibly, the regulation of P uptake into roots by different mycorrhizal efficiencies balances nutrient competition across different soil layers. This idea should be followed up in future studies.
Conclusion
This study reveals that P uptake by plant roots is not only based on soil P stocks but differs between different layers, underlies regulation by mycorrhizal efficiencies, and faces competition with soil microbes under P-limiting conditions. Revisiting our hypotheses, we confirmed that (i) the main P source for beech P supply is the mineral topsoil under P-rich and the organic layer under P-poor conditions. Our results pinpoint different mycorrhizal efficiencies as drivers for root P uptake. As expected non-mycorrhizal root tips did not contribute markedly to root P supply in natural forest soils. However, the simplistic idea (hypothesis ii) that the mycorrhizal P enrichment is relatively higher to compensate for lower P bioavailability can be refuted because the relative mycorrhizal P enrichments were highest in those layers that contributed most to root P uptake. In agreement with our hypothesis (iii) no competition for P was detected in soil of the P-rich forest, whereas microbes were strong competitors under P-poor conditions.
In accordance with previous studies (Lang et al., 2017; Hauenstein et al., 2018), we conclude that an intact forest floor is of tremendous importance under nutrient-poor conditions for uptake and recycling of P. Our study provides insights into the mechanisms that contribute to the adaptation of ecosystems to low P availability. Obviously, forests that rely on P recycling with the majority of roots in the upper layer are at risk under climate change. When the duration and intensity of summer droughts increase, the top layer roots and microbes will be the first to be negatively affected by water shortage. Further depletion of P is expected due to lower P mineralization rates (Schimel et al., 2007) and lower P mobility in drier soil (Schachtman et al., 1998; Kreuzwieser and Gessler, 2010). This study envisages decreasing P retention as the result of declining microbial biomass and declining mycorrhizal activities in future climate scenarios.
Data Availability Statement
The datasets generated for this study can be found in the Dryad digital repository (10.5061/dryad.4j0zpc87g). The sequences for identified mycorrhizal fungi are available in GenBank (accession numbers in Supplementary Table S4).
Author Contributions
SC and AP contributed to the conception and design of the study and contributed to manuscript revision and approved the final version. SC conducted the measurements, analyzed the data, and wrote the first draft of the manuscript. AP revised the draft.
Funding
The project was funded by Deutsche Forschungsgemeinschaft (DFG) and was part of SPP 1685 “Ecosystem Nutrition,” funding project Po362/22-2.
Conflict of Interest
The authors declare that the research was conducted in the absence of any commercial or financial relationships that could be construed as a potential conflict of interest.
Acknowledgments
We are grateful to M. Franke-Klein for ICP analyses, to T. Klein (Laboratory for Radio-Isotopes, University of Goettingen) for help with mycorrhizal sequencing and to G. Lehmann (Laboratory for Radio-Isotopes, University of Goettingen) for support with radioactive labeling experiment.
Supplementary Material
The Supplementary Material for this article can be found online at: https://www.frontiersin.org/articles/10.3389/ffgc.2020.00054/full#supplementary-material
References
Agerer, R. (ed.) (1987). Colour Atlas of Ectomycorrhizae: With Glossary. Delivery 2, 1th-5th del Edn. Schwäbisch Gmünd: Einhorn-Verlag.
Balogh-Brunstad, Z., Keller, C. K., Dickinson, J. T., Stevens, F., Li, C. Y., and Bormann, B. T. (2008). Biotite weathering and nutrient uptake by ectomycorrhizal fungus, Suillus tomentosus, in liquid-culture experiments. Geochim. Cosmochim. Acta 72, 2601–2618. doi: 10.1016/j.gca.2008.04.003
Becquer, A., Trap, J., Irshad, U., Ali, M. A., and Plassard, C. (2014). From soil to plant, the journey of P through trophic relationships and ectomycorrhizal association. Front. Plant Sci. 5:548. doi: 10.3389/fpls.2014.00548
Bergkemper, F., Schöler, A., Engel, M., Lang, F., Krüger, J., Schloter, M., et al. (2016). Phosphorus depletion in forest soils shapes bacterial communities towards phosphorus recycling systems: microbial phosphorus turnover in soil. Environ. Microbiol. 18, 1988–2000. doi: 10.1111/1462-2920.13188
Brandes, B., Godbold, D. L., Kuhn, A. J., and Jentschke, G. (1998). Nitrogen and phosphorus acquisition by the mycelium of the ectomycorrhizal fungus Paxillus involutus and its effect on host nutrition. New Phytol. 140, 735–743. doi: 10.1046/j.1469-8137.1998.00313.x
Brandtberg, P.-O., Bengtsson, J., and Lundkvist, H. (2004). Distributions of the capacity to take up nutrients by Betula spp. and Picea abies in mixed stands. Forest Ecol. Manage. 198, 193–208. doi: 10.1016/j.foreco.2004.04.012
Bray, R. H., and Kurtz, L. T. (1945). Determination of total, organic, and available forms of phosphorus in soils. Soil Sci. 59, 39–46. doi: 10.1097/00010694-194501000-00006
Brookes, P. C., Landman, A., Pruden, G., and Jenkinson, D. S. (1985). Chloroform fumigation and the release of soil nitrogen: a rapid direct extraction method to measure microbial biomass nitrogen in soil. Soil Biol. Biochem. 17, 837–842. doi: 10.1016/0038-0717(85)90144-0
Brookes, P. C., Powlson, D. S., and Jenkinson, D. S. (1982). Measurement of microbial biomass phosphorus in soil. Soil Biol. Biochem. 14, 319–329. doi: 10.1016/0038-0717(82)90001-3
Cairney, J. W. G. (2011). Ectomycorrhizal fungi: the symbiotic route to the root for phosphorus in forest soils. Plant Soil 344, 51–71. doi: 10.1007/s11104-011-0731-0
Callaway, R. M., and Nadkarni, N. M. (1991). Seasonal patterns of nutrient deposition in a Quercus douglasii woodland in central California. Plant Soil 137, 209–222. doi: 10.1007/BF00011199
Casarin, V., Plassard, C., Hinsinger, P., and Arvieu, J.-C. (2004). Quantification of ectomycorrhizal fungal effects on the bioavailability and mobilization of soil P in the rhizosphere of Pinus pinaster. New Phytol. 163, 177–185. doi: 10.1111/j.1469-8137.2004.01093.x
Chadwick, O. A., Derry, L. A., Vitousek, P. M., Huebert, B. J., and Hedin, L. O. (1999). Changing sources of nutrients during four million years of ecosystem development. Nature 397, 491–497. doi: 10.1038/17276
Colpaert, J. V., Van Tichelen, K. K., Van Assche, J. A., and Van Laere, A. (1999). Short-term phosphorus uptake rates in mycorrhizal and non-mycorrhizal roots of intact Pinus sylvestris seedlings. New Phytol. 143, 589–597. doi: 10.1046/j.1469-8137.1999.00471.x
Courty, P.-E., Buée, M., Diedhiou, A. G., Frey-Klett, P., Le Tacon, F., Rineau, F., et al. (2010). The role of ectomycorrhizal communities in forest ecosystem processes: NEw perspectives and emerging concepts. Soil Biol. Biochem. 42, 679–698. doi: 10.1016/j.soilbio.2009.12.006
Courty, P.-E., Pritsch, K., Schloter, M., Hartmann, A., and Garbaye, J. (2005). Activity profiling of ectomycorrhiza communities in two forest soils using multiple enzymatic tests: Methods. New Phytol. 167, 309–319. doi: 10.1111/j.1469-8137.2005.01401.x
Cumming, J. R. (1996). Phosphate-limitation physiology in ectomycorrhizal pitch pine (Pinus rigida) seedlings. Tree Physiol. 16, 977–983. doi: 10.1093/treephys/16.11-12.977
Curt, T., Coll, L., Prévosto, B., Balandier, P., and Kunstler, G. (2005). Plasticity in growth, biomass allocation and root morphology in beech seedlings as induced by irradiance and herbaceous competition. Ann. For. Sci. 62, 51–60. doi: 10.1051/forest:2004092
Desai, S., Naik, D., and Cumming, J. R. (2014). The influence of phosphorus availability and Laccaria bicolor symbiosis on phosphate acquisition, antioxidant enzyme activity, and rhizospheric carbon flux in Populus tremuloides. Mycorrhiza 24, 369–382. doi: 10.1007/s00572-013-0548-1
Di, H. J., Cameron, K. C., and McLaren, R. G. (2000). Isotopic dilution methods to determine the gross transformation rates of nitrogen, phosphorus, and sulfur in soil: a review of the theory, methodologies, and limitations. Aust. J. Soil Res. 38, 213–230. doi: 10.1071/SR99005
Franklin, R. E. (1969). Effect of adsorbed cations on phosphorus uptake by excised roots. Plant Physiol. 44, 697–700. doi: 10.1104/pp.44.5.697
George, T. S., Giles, C. D., Menezes-Blackburn, D., Condron, L. M., Gama-Rodrigues, A. C., Jaisi, D., et al. (2018). Organic phosphorus in the terrestrial environment: a perspective on the state of the art and future priorities. Plant Soil 427, 191–208. doi: 10.1007/s11104-017-3391-x
Grace, J. B. (1995). On the Measurement of Plant Competition Intensity. Ecology 76, 305–308. doi: 10.2307/1940651
Hammer, Ø., Harper, D. A. T., and Ryan, P. D. (2001). PAST: paleontological statistics software package for education and data analysis. Palaeontol. Electron. 4, 9–17.
Harley, J. L., Brierley, J. K., and Mccready, C. C. (1954). The uptake of phosphate by excised mycorrhizal roots of the beech. New Phytol. 53, 92–98. doi: 10.1111/j.1469-8137.1954.tb05225.x
Hauenstein, S., Neidhardt, H., Lang, F., Krüger, J., Hofmann, D., Pütz, T., et al. (2018). Organic layers favor phosphorus storage and uptake by young beech trees (Fagus sylvatica L.) at nutrient poor ecosystems. Plant Soil 432, 289–301. doi: 10.1007/s11104-018-3804-5
Haußmann, T., and Lux, W. (1997). Dauerbeobachtungsflächen zur Umweltkontrolle im Wald: Level II; Erste Ergebnisse. Bonn: BMELF (Bundesministerium für Ernährung, Landwirtschaft und Forsten).
Heinrichs, H., Brumsack, H.-J., Loftfield, N., and König, N. (1986). Verbessertes Druckaufschlußsystem für biologische und anorganische Materialien. Z. Pflanzenernaehr. Bodenk. 149, 350–353. doi: 10.1002/jpln.19861490313
Heuck, C., and Spohn, M. (2016). Carbon, nitrogen and phosphorus net mineralization in organic horizons of temperate forests: stoichiometry and relations to organic matter quality. Biogeochemistry 131, 229–242. doi: 10.1007/s10533-016-0276-7
Hinsinger, P., Herrmann, L., Lesueur, D., Robin, A., Trap, J., Waithaisong, K., et al. (2015). “Impact of roots, microorganisms and microfauna on the fate of soil phosphorus in the rhizosphere,” in Annual Plant Reviews, Vol. 48, eds W. C. Plaxton and H. Lambers (Hoboken, NJ: John Wiley & Sons, Inc), 375–407. doi: 10.1002/9781118958841.ch13
Hoffland, E., Giesler, R., Breemen, N., and van Jongmans, A. G. (2003). Feldspar tunneling by fungi along natural productivity gradients. Ecosystems 6, 739–746. doi: 10.1007/s10021-003-0191-3
Hofmann, K., Heuck, C., and Spohn, M. (2016). Phosphorus resorption by young beech trees and soil phosphatase activity as dependent on phosphorus availability. Oecologia 181, 369–379. doi: 10.1007/s00442-016-3581-x
Hölscher, D., Hertel, D., Leuschner, C., and Hottkowitz, M. (2002). Tree species diversity and soil patchiness in a temperate broad-leaved forest with limited rooting space. Flora Morphol. Distrib. Funct. Ecol. Plants 197, 118–125. doi: 10.1078/0367-2530-00021
Ilg, K., Dominik, P., Kaupenjohann, M., and Siemens, J. (2008). Phosphorus-induced mobilization of colloids: model systems and soils. Eur. J. Soil Sci. 59, 233–246. doi: 10.1111/j.1365-2389.2007.00982.x
Jansa, J., Finlay, R., Wallander, H., Smith, F. A., and Smith, S. E. (2011). “Role of mycorrhizal symbioses in phosphorus cycling,” in Phosphorus in Action, eds E. Bünemann, A. Oberson, and E. Frossard (Berlin: Springer), 137–168. doi: 10.1007/978-3-642-15271-9_6
Jentschke, G., Brandes, B., Kuhn, A. J., Schröder, W. H., and Godbold, D. L. (2001). Interdependence of phosphorus, nitrogen, potassium and magnesium translocation by the ectomycorrhizal fungus Paxillus involutus. New Phytol. 149, 327–337. doi: 10.1046/j.1469-8137.2001.00014.x
Johri, A. K., Oelmüller, R., Dua, M., Yadav, V., Kumar, M., Tuteja, N., et al. (2015). Fungal association and utilization of phosphate by plants: success, limitations, and future prospects. Front. Microbiol. 6:984. doi: 10.3389/fmicb.2015.00984
Jonard, M., Augusto, L., Morel, C., Achat, D. L., and Saur, E. (2009). Forest floor contribution to phosphorus nutrition: experimental data. Ann. For. Sci. 66, 510–510. doi: 10.1051/forest/2009039
Jonard, M., Fürst, A., Verstraeten, A., Thimonier, A., Timmermann, V., Potoèiæ, N., et al. (2015). Tree mineral nutrition is deteriorating in Europe. Glob. Change Biol. 21, 418–430. doi: 10.1111/gcb.12657
Jongmans, A. G., van Breemen, N., Lundström, U., van Hees, P. A. W., Finlay, R. D., Srinivasan, M., et al. (1997). Rock-eating fungi. Nature 389, 682–683. doi: 10.1038/39493
Kavka, M., and Polle, A. (2016). Phosphate uptake kinetics and tissue-specific transporter expression profiles in poplar (Populus × canescens) at different phosphorus availabilities. BMC Plant Biol. 16:206. doi: 10.1186/s12870-016-0892-3
Kavka, M., and Polle, A. (2017). Dissecting nutrient-related co-expression networks in phosphate starved poplars. PLoS One 12:e0171958. doi: 10.1371/journal.pone.0171958
Kramer, P. J., and Wilbur, K. M. (1949). Absorption of radioactive phosphorus by mycorrhizal roots of pine. Science 110, 8–9. doi: 10.1126/science.110.2844.8
Kreuzwieser, J., and Gessler, A. (2010). Global climate change and tree nutrition: influence of water availability. Tree Physiol. 30, 1221–1234. doi: 10.1093/treephys/tpq055
Kuzyakov, Y., and Xu, X. (2013). Competition between roots and microorganisms for nitrogen: mechanisms and ecological relevance. New Phytol. 198, 656–669. doi: 10.1111/nph.12235
Lambers, H., Clode, P. L., Hawkins, H.-J., Laliberté, E., Oliveira, R. S., Reddell, P., et al. (2015a). “Metabolic adaptations of the non-mycotrophic proteaceae to soils with low phosphorus availability,” in Annual Plant Reviews, Vol. 48, eds W. C. Plaxton and H. Lambers (Hoboken, NJ: John Wiley & Sons, Inc), 289–335. doi: 10.1002/9781118958841.ch11
Lambers, H., Martinoia, E., and Renton, M. (2015b). Plant adaptations to severely phosphorus-impoverished soils. Curr. Opin. Plant Biol. 25, 23–31. doi: 10.1016/j.pbi.2015.04.002
Lambers, H., Raven, J., Shaver, G., and Smith, S. (2008). Plant nutrient-acquisition strategies change with soil age. Trends Ecol. Evol. 23, 95–103. doi: 10.1016/j.tree.2007.10.008
Lang, F., Bauhus, J., Frossard, E., George, E., Kaiser, K., Kaupenjohann, M., et al. (2016). Phosphorus in forest ecosystems: new insights from an ecosystem nutrition perspective. J. Plant Nutr. Soil Sci. 179, 129–135. doi: 10.1002/jpln.201500541
Lang, F., Krüger, J., Amelung, W., Willbold, S., Frossard, E., Bünemann, E. K., et al. (2017). Soil phosphorus supply controls P nutrition strategies of beech forest ecosystems in Central Europe. Biogeochemistry 136, 5–29. doi: 10.1007/s10533-017-0375-0
Leberecht, M., Dannenmann, M., Tejedor, J., Simon, J., Rennenberg, H., and Polle, A. (2016). Segregation of nitrogen use between ammonium and nitrate of ectomycorrhizas and beech trees. Plant Cell Environ. 39, 2691–2700. doi: 10.1111/pce.12820
Leuschner, C., Meier, I. C., and Hertel, D. (2006). On the niche breadth of Fagus sylvatica: soil nutrient status in 50 Central European beech stands on a broad range of bedrock types. Ann. For. Sci. 63, 355–368. doi: 10.1051/forest:2006016
Leyval, C., and Berthelin, J. (1993). Rhizodeposition and net release of soluble organic compounds by pine and beech seedlings inoculated with rhizobacteria and ectomycorrhizal fungi. Biol. Fertil. Soils 15, 259–267. doi: 10.1007/BF00337210
Liu, Q., Loganathan, P., Hedley, M. J., and Grace, L. J. (2007). Effect of mycorrhizal inoculation on rhizosphere properties, phosphorus uptake and growth of pine seedlings treated with and without a phosphate rock fertilizer. J. Plant Nutr. 31, 137–156. doi: 10.1080/01904160701742063
Mejstrik, V., and Benecke, U. (1969). The ectotrophic mycorrhizas of Alnus ciridis (Chaix) D.C. and their significance in respect to phosphorus uptake. New Phytol. 68, 141–149. doi: 10.1111/j.1469-8137.1969.tb06427.x
Nambiar, E. K. S., and Sands, R. (1993). Competition for water and nutrients in forests. Can. J. For. Res. 23, 1955–1968. doi: 10.1139/x93-247
Nehls, U., and Plassard, C. (2018). Nitrogen and phosphate metabolism in ectomycorrhizas. New Phytol. 220, 1047–1058. doi: 10.1111/nph.15257
Netzer, F., Schmid, C., Herschbach, C., and Rennenberg, H. (2017). Phosphorus-nutrition of European beech (Fagus sylvatica L.) during annual growth depends on tree age and P-availability in the soil. Environ. Exp. Bot. 137, 194–207. doi: 10.1016/j.envexpbot.2017.02.009
Noggle, J. C., and Fried, M. (1960). A kinetic analysis of phosphate absorption by excised roots of millet, barley, and alfalfa. Soil Sci. Soc. Am. J. 24, 33–35. doi: 10.2136/sssaj1960.03615995002400010017x
Pena, R., Lang, C., Lohaus, G., Boch, S., Schall, P., Schöning, I., et al. (2017). Phylogenetic and functional traits of ectomycorrhizal assemblages in top soil from different biogeographic regions and forest types. Mycorrhiza 27, 233–245. doi: 10.1007/s00572-016-0742-z
Pena, R., Offermann, C., Simon, J., Naumann, P. S., Gessler, A., Holst, J., et al. (2010). Girdling affects ectomycorrhizal fungal (EMF) diversity and reveals functional differences in EMF community composition in a beech forest. Appl. Environ. Microbiol. 76, 1831–1841. doi: 10.1128/AEM.01703-09
Pena, R., and Polle, A. (2014). Attributing functions to ectomycorrhizal fungal identities in assemblages for nitrogen acquisition under stress. ISME J. 8, 321–330. doi: 10.1038/ismej.2013.158
Peñuelas, J., Poulter, B., Sardans, J., Ciais, P., van der Velde, M., Bopp, L., et al. (2013). Human-induced nitrogen–phosphorus imbalances alter natural and managed ecosystems across the globe. Nat. Commun. 4:2934. doi: 10.1038/ncomms3934
Plassard, C., and Dell, B. (2010). Phosphorus nutrition of mycorrhizal trees. Tree Physiol. 30, 1129–1139. doi: 10.1093/treephys/tpq063
Prietzel, J., Klysubun, W., and Werner, F. (2016). Speciation of phosphorus in temperate zone forest soils as assessed by combined wet-chemical fractionation and XANES spectroscopy. J. Plant Nutr. Soil Sci. 179, 168–185. doi: 10.1002/jpln.201500472
R Core Development Team (2012). R: A Language and Environment for Statistical Computing. Vienna: R Foundation for Statistical Computing. Available online at: https://www.r-project.org/
Rieger, I., Kowarik, I., Ziche, D., Wellbrock, N., and Cierjacks, A. (2019). Linkages between phosphorus and plant diversity in Central European forest ecosystems—complementarity or competition? Forests 10:1156. doi: 10.3390/f10121156
Rubio, G., Oesterheld, M., Alvarez, C. R., and Lavado, R. S. (1997). Mechanisms for the increase in phosphorus uptake of waterlogged plants: soil phosphorus availability, root morphology and uptake kinetics. Oecologia 112, 150–155. doi: 10.1007/s004420050294
Schachtman, D. P., Reid, R. J., and Ayling, S. M. (1998). Phosphorus uptake by plants: from soil to cell. Plant Physiol. 116, 447–453. doi: 10.1104/pp.116.2.447
Schimel, J., Balser, T. C., and Wallenstein, M. (2007). Microbial stress-response physiology and its implications for ecosystem function. Ecology 88, 1386–1394. doi: 10.1890/06-0219
Schimel, J. P., and Bennett, J. (2004). Nitrogen mineralization: challenges of a changing paradigm. Ecology 85, 591–602. doi: 10.1890/03-8002
Shen, J., Yuan, L., Zhang, J., Li, H., Bai, Z., Chen, X., et al. (2011). Phosphorus dynamics: from soil to plant. Plant Physiol. 156, 997–1005. doi: 10.1104/pp.111.175232
Smith, S. E., Anderson, I. C., and Smith, F. A. (2015). “Mycorrhizal associations and phosphorus acquisition: from cells to ecosystems,” in Annual Plant Reviews, Vol. 48, eds W. C. Plaxton and H. Lambers (Hoboken, NJ: John Wiley & Sons, Inc), 409–439. doi: 10.1002/9781118958841.ch14
Spohn, M., Zavišić, A., Nassal, P., Bergkemper, F., Schulz, S., Marhan, S., et al. (2018). Temporal variations of phosphorus uptake by soil microbial biomass and young beech trees in two forest soils with contrasting phosphorus stocks. Soil Biol. Biochem. 117, 191–202. doi: 10.1016/j.soilbio.2017.10.019
Turner, B. L., and Condron, L. M. (2013). Pedogenesis, nutrient dynamics, and ecosystem development: the legacy of T.W. Walker and J.K. Syers. Plant Soil 367, 1–10. doi: 10.1007/s11104-013-1750-9
Vance, C. P., Uhde-Stone, C., and Allan, D. L. (2003). Phosphorus acquisition and use: critical adaptations by plants for securing a nonrenewable resource. New Phytol. 157, 423–447. doi: 10.1046/j.1469-8137.2003.00695.x
Vincent, A. G., Vestergren, J., Gröbner, G., Persson, P., Schleucher, J., and Giesler, R. (2013). Soil organic phosphorus transformations in a boreal forest chronosequence. Plant Soil 367, 149–162. doi: 10.1007/s11104-013-1731-z
Vitousek, P. M., Porder, S., Houlton, B. Z., and Chadwick, O. A. (2010). Terrestrial phosphorus limitation: mechanisms, implications, and nitrogen–phosphorus interactions. Ecol. Appl. 20, 5–15. doi: 10.1890/08-0127.1
Walker, T. W., and Syers, J. K. (1976). The fate of phosphorus during pedogenesis. Geoderma 15, 1–19. doi: 10.3390/ijerph14121475
Wallander, H. (2000). Uptake of P from apatite by Pinus sylvestris seedlings colonised by different ectomycorrhizal fungi. Plant Soil 218, 249–256. doi: 10.1023/A:1014936217105
Wang, J., and Lars, B. R. (1997). Competition for nitrogen during mineralization of plant residues in soil: microbial response to C and N availability. Soil Biol. Biochem. 29, 163–170.
Weigt, R. B., Raidl, S., Verma, R., and Agerer, R. (2012). Exploration type-specific standard values of extramatrical mycelium–a step towards quantifying ectomycorrhizal space occupation and biomass in natural soil. Mycol. Prog. 11, 287–297. doi: 10.1007/s11557-011-0750-5
Winkler, J. B., Dannenmann, M., Simon, J., Pena, R., Offermann, C., Sternad, W., et al. (2010). Carbon and nitrogen balance in beech roots under competitive pressure of soil-borne microorganisms induced by girdling, drought and glucose application. Funct. Plant Biol. 37, 879–889. doi: 10.1071/FP09309
Woodmansee, R., Vallis, I., and Mott, J. J. (1981). Grassland nitrogen, terrestrial nitrogen cycles. Ecol. Bull. 33, 443–462.
Wu, P., Ma, L., Hou, X., Wang, M., Wu, Y., Liu, F., et al. (2003). Phosphate starvation triggers distinct alterations of genome expression in Arabidopsis roots and leaves. Plant Physiol. 132, 1260–1271. doi: 10.1104/pp.103.021022
Yang, N., Zavisic, A., Pena, R., and Polle, A. (2016). Phenology, photosynthesis, and phosphorus in European beech (Fagus sylvatica L.) in two forest soils with contrasting P contents. J. Plant Nutr. Soil Sci. 179, 151–158. doi: 10.1002/jpln.201500539
York, L. M., Carminati, A., Mooney, S. J., Ritz, K., and Bennett, M. J. (2016). The holistic rhizosphere: integrating zones, processes, and semantics in the soil influenced by roots. J. Exp. Bot. 67, 3629–3643. doi: 10.1093/jxb/erw108
Zavišić, A., Nassal, P., Yang, N., Heuck, C., Spohn, M., and Marhan, et al. (2016). Phosphorus availabilities in beech (Fagus sylvatica L.) forests impose habitat filtering on ectomycorrhizal communities and impact tree nutrition. Soil Biol. Biochem. 98, 127–137. doi: 10.1016/j.soilbio.2016.04.006
Zavišić, A., and Polle, A. (2018). Dynamics of phosphorus nutrition, allocation and growth of young beech (Fagus sylvatica L.) trees in P-rich and P-poor forest soil. Tree Physiol. 38, 37–51. doi: 10.1093/treephys/tpx146
Zavišić, A., Yang, N., Marhan, S., Kandeler, E., and Polle, A. (2018). Forest soil phosphorus resources and fertilization affect ectomycorrhizal community composition, beech P uptake efficiency, and photosynthesis. Front. Plant Sci. 9:463. doi: 10.3389/fpls.2018.00463
Zederer, D. P., and Talkner, U. (2018). Organic P in temperate forest mineral soils as affected by humus form and mineralogical characteristics and its relationship to the foliar P content of European beech. Geoderma 325, 162–171. doi: 10.1016/j.geoderma.2018.03.033
Zhu, Q., Riley, W. J., and Tang, J. (2017). A new theory of plant-microbe nutrient competition resolves inconsistencies between observations and model predictions. Ecol. Appl. 27, 875–886. doi: 10.1002/eap.1490
Keywords: ectomycorrhiza, rhizosphere, organic soil, mineral soil, phosphorus, radioactive labeling
Citation: Clausing S and Polle A (2020) Mycorrhizal Phosphorus Efficiencies and Microbial Competition Drive Root P Uptake. Front. For. Glob. Change 3:54. doi: 10.3389/ffgc.2020.00054
Received: 29 January 2020; Accepted: 14 April 2020;
Published: 19 May 2020.
Edited by:
Friederike Lang, University of Freiburg, GermanyReviewed by:
Tessa Camenzind, Freie Universität Berlin, GermanyLukas Kohl, University of Helsinki, Finland
Copyright © 2020 Clausing and Polle. This is an open-access article distributed under the terms of the Creative Commons Attribution License (CC BY). The use, distribution or reproduction in other forums is permitted, provided the original author(s) and the copyright owner(s) are credited and that the original publication in this journal is cited, in accordance with accepted academic practice. No use, distribution or reproduction is permitted which does not comply with these terms.
*Correspondence: Andrea Polle, YXBvbGxlQGd3ZGcuZGU=