- 1Guangling College, Yangzhou University, Yangzhou, China
- 2School of Chemistry and Chemical Engineering, Yangzhou University, Yangzhou, China
Herein, g-C3N4/CdS hybrids with controllable CdS nanoparticles anchoring on g-C3N4 nanosheets were constructed. The effects of CdS nanoparticles on photocatalytic H2 production and organic molecule degradation for g-C3N4/CdS hybrids were investigated. The maximum rate of H2 production for g-C3N4/CdS sample was 1,070.9 μmol g−1 h−1, which was about four times higher than that of the individual g-C3N4 nanosheet sample. The enhanced photocatalytic performance for prepared hybrids could be mainly attributed to the following causes: the formed heterojunctions can contribute to the light absorption and separation of photogenerated electrons and holes, the two-dimensional layered structure facilitates the transmission and transfer of electrons, and high specific surface area could provide more exposed active sites.
Introduction
Semiconductor materials have received widespread attention as promising photocatalysts for clean energy production and environmental problems. (Tong et al., 2012; Chang et al., 2016; Yu et al., 2017; Qi et al., 2018; Cao et al., 2019; Ng et al., 2021). Recently, g-C3N4 has received considerable attention as a promising photocatalyst, owing to its ease of preparation, high stability, low cost, clean and low toxicity, narrowed bandgap (∼2.7 eV), and special two-dimensional (2D) layered structure (Shi et al., 2016; Teng et al., 2017; Han et al., 2018; Deng et al., 2019; Ong et al., 2020; Vu et al., 2020; Yu et al., 2021); however, diverse drawbacks include poor efficiency of light utilization and low separation of photogenerated charges during the application, making g-C3N4 less attractive for photocatalyst construction. (Tahir et al., 2014; Liu et al., 2017; Al Marzouqi et al., 2019; Fu et al., 2019; Fan et al., 2020). In addition, bulk g-C3N4 with a low surface area and irregular morphology prepared through the conventional method leads to the low transfer rate of interfacial charge and poor photocatalytic activity (Teng et al., 2017; Niu et al., 2018).
For the sake of the improved photocatalytic performance of pure g-C3N4, many strategies have been made to develop cheaper and recyclable catalysts, such as nanostructure design (Sun et al., 2012; Bai et al., 2013; Tahir et al., 2014; Lin et al., 2016; Zhou et al., 2018; Yang et al., 2019), loading with noble metals (Zhang et al., 2016; Duan et al., 2019; Wan et al., 2020) or doping non-metal elements (Guo et al., 2017; Liu et al., 2018; Li et al., 2020), and heterojunction construction (Zhang et al., 2013; Shi et al., 2017; Ji et al., 2018). However, noble metals are rare and too expensive, and their stability is also a big challenge. Doping of non-metal elements would need high temperature and produce many noxious and odorous gases. While nanostructure design usually requires multiple synthetic steps, and templates are also required for special structures. Therefore, the enhanced photocatalytic performance of g-C3N4–based catalysts is still in urgent demand. As for heterojunction construction, the combination of g-C3N4 with another semiconductor that has well-matched band structures can not only expand the light absorption into the wide absorption region but also be formed between the two components, which effectively guarantee the separation of rapid charges and transfer in the contacted interface. As a visible light–responsive photocatalyst, CdS has also drawn great attention, and successfully applied in the fields of environmental protection, hydrogen evolution, and selective organic synthesis (Liu et al., 2015; Ren et al., 2019; Ai et al., 2020). Coupling g-C3N4 with CdS could be a feasible route to improve the photocatalytic activity. In previous reports, Chen et al. reported the synthesis of g-C3N4/CdS composites with a tunable density of CdS nanodots adopting the in situ photochemical deposition method (Chen et al., 2019). Cui et al. synthesized C3N4/CdS composites through a one-step calcination process at high temperatures (Cui, 2015). C3N4–CdS heterostructures were constructed by a precipitation–deposition route (Fu et al., 2013). All the reported g-C3N4/CdS composites showed higher photocatalytic activity and stability than individual g-C3N4 and CdS, ascribing to the synergic effect between g-C3N4 and CdS, which can effectively promote the charge separation and transfer. Although numerous heterostructure photocatalysts have been designed, the remarkable improvement of the photocatalytic effect has not been obtained. It was probably caused by large bulk volume or unreasonable contacted interfaces among the two or more components in photocatalysts. It has been investigated that the photocatalytic activity could be influenced by the following factors, such as particle sizes, morphology and structures, and preparation methods (Chen et al., 2010). Therefore, in order to optimize the photocatalytic performances of g-C3N4/CdS hybrids, some important factors should be taken into account, such as effective contact between two components, well-controlled morphology structure and particle size, and sufficiently exposed reactive active sites. However, few works have been found using the chemical deposition of CdS nanoparticles with controllable intensity and particle size onto g-C3N4 nanosheets to construct heterojunctions.
Herein, we realize the construction of the highly efficient heterostructured photocatalyst for the photocatalytic hydrogen evolution and degradation of pollutant molecules, where CdS nanoparticles with controllable particle sizes are obtained to modify g-C3N4 nanosheets through a facile chemical deposition process. It is found that the controllable CdS particle size has a significant effect on morphology, specific surface area, light absorption, and photocatalytic activity for prepared hybrids. The hydrogen generation rate of g-C3N4/CdS hybrids could reach up to 1,070.9 μmol g−1 h−1. The effects of CdS nanoparticles for the light absorption and photoinduced charge transport, and the enhanced photocatalytic activities were systematically discussed.
Experimental
Synthesis
First, g-C3N4 nanosheets were prepared according to the previous report (Fan et al., 2020). Briefly, the amount of urea was heated at 570°C under air for 3 h, and the yellow product was obtained for further use. Then 10.0 mg of g-C3N4 nanosheets were dispersed into 20.0 ml aqueous solution containing different amounts of CdCl2.2.5H2O (with 7.50, 15.0, and 22.5 mg CdCl2.2.5H2O, respectively) by ultrasonication for 30 min. Then 0.25 ml NH3·H2O and 5.0 ml thioacetamide (TAA) solution (with 5.5 mg TAA) was added, followed by a water bath at 60°C for 3 h. The resultant products were washed with water and ethanol three times and collected by centrifugation, followed by drying at 60°C for 4 h. After that, the as-prepared products were annealed at 300°C for 2 h in the nitrogen atmosphere by a tubular furnace to improve the crystallinity CdS. The as-prepared g-C3N4/CdS hybrids were denoted as g-C3N4/CdS-1, g-C3N4/CdS-2, and g-C3N4/CdS-3, respectively.
Photocatalytic Activity Tests
Photocatalytic degradation of organic pollutes was carried out in a photoreactor system (Xujiang XPA-7) with 5.0 mg of samples dispersed in a 25-ml target molecule solution (2.0 × 10−5 M). After that, a 400 W metal halide lamp with a filter (λ ˃400 nm) was irradiated to trigger the photocatalytic reaction. The concentration of the degraded solution was detected by a UV-vis spectrophotometer.
The photocatalytic H2 production was measured in a closed quartz reaction system (300 W Xe lamp with a filter as the light source, λ ˃400 nm) using triethanolamine as the sacrificial reagent. 100 ml solution (with 20% triethanolamine and dispersed 10.0 mg samples) was continuously stirred at a fixed speed. The amount of H2 was determined by the online gas chromatography (CEAULIGHT, GC-7920). During the photocatalytic tests, a cooling system was used to maintain the temperature constant.
Characterization
TEM (JEOL, JEM-2100) and HRTEM (FEI, Tecnai G2 F30 S-Twin TEM) were measured for imaging. The phase composition was measured by the Bruker D8 ADVANCE X-ray diffractometer (XRD). BET-specific surface areas and pore structures were determined by a Beishide 3H-2000PS2 system. XPS measurement was performed on a Thermo Scientific ESCALAB 250Xi spectrometer. The photocurrent response experiment was performed on a photoelectrochemical workstation (CIMPS-2, Zahner) with a three-electrode system; 300 W Xe lamp and Na2SO4 aqueous solution (0.1 M) were used as the light source and electrolyte solution, respectively. The hydroxyl radicals were detected using a fluorescence spectrophotometer (Hitachi F-7000).
Results and Discussions
Morphologies and Structural Characterization
The synthesis processes of g-C3N4/CdS hybrids are shown in Scheme 1. The morphology and structure of g-C3N4/CdS samples were characterized by SEM and TEM, as given in Figure 1. Supplementary Figure S1 is the TEM image of the pure g-C3N4 sample with a layered structure, which can offer a substrate for the arching of CdS nanoparticles. For the g-C3N4/CdS samples (Figure 1), it should be noted that the CdS nanoparticles are well loaded onto the g-C3N4 nanosheets. The amount and particle sizes of CdS nanoparticles can be tuned by changing the amount of the CdCl2 precursor. As demonstrated in Figures 1A,D, for the sample g-C3N4/CdS-1, CdS nanoparticles were evenly dispersed on g-C3N4 nanosheets with a size narrowed by about 11.5 nm. With increasing the amount of the CdCl2 precursor, more nanoparticles with a larger size, up to 18.0 nm, can be observed for sample g-C3N4/CdS-2 (Figures 1B,E). When the amount of CdCl2 was increased to 22.5 mg, for sample g-C3N4/CdS-3, the size distribution of CdS nanoparticles turns to be wider, and CdS nanoparticles with a diameter of ∼23.5 and ∼8.8 nm can be observed (Figures 1C,F). The statistical size distributions of CdS nanoparticles for g-C3N4/CdS-1/2/3 hybrids are also given in Figure 1.
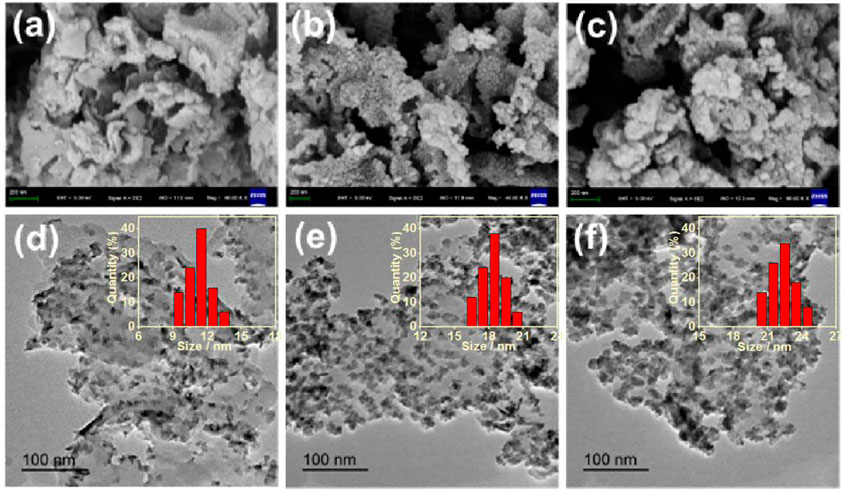
FIGURE 1. (A–C) SEM and (D–F) TEM images of (a, d) g-C3N4/CdS-1 (b, e) g-C3N4/CdS-2, and (c, f) g-C3N4/CdS-3 hybrids. Inset: The statistical size distributions of CdS nanoparticles for g-C3N4/CdS-1/2/3 hybrids.
As seen from Figures 2A–C, TEM, HRTEM, and EDX mapping indicated the successful loading of CdS nanoparticles on a g-C3N4 nanosheet. The TEM image (Figure 2A) shows a high distribution of CdS nanoparticles with average sizes of about ∼18 nm dispersed on g-C3N4 nanosheets. The HRTEM image (Figure 2B) gives two sets of distinct lattice fringes, whereas the spacing of about 0.316 and 0.321 nm, corresponding to the (101) and (002) planes of CdS and g-C3N4, respectively (Wang et al., 2017a; Zhang et al., 2017; Chiu et al., 2019). Obviously, EDX mappings demonstrate the existence of C, N, Cd, and S (Figures 2D–H), suggesting the uniform distribution of Cd and S elements from g-C3N4/CdS (Figure 2C), confirming the good connection between CdS and g-C3N4.
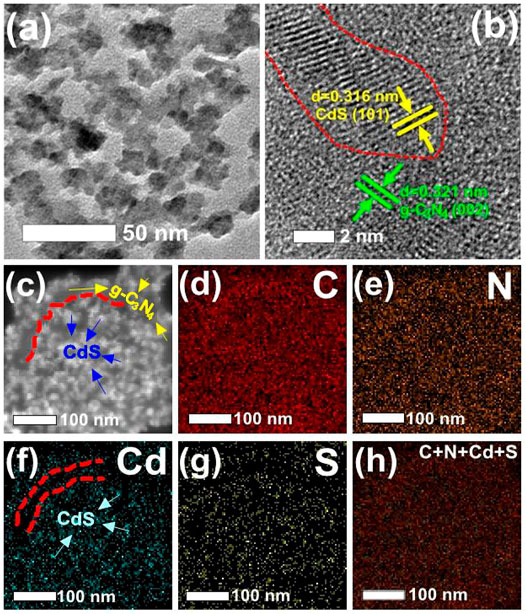
FIGURE 2. (A) TEM, (B) HRTEM, and (C) HAADF-STEM images of g-C3N4/CdS-2 hybrids. (D–G) Elemental mappings of (d) C, (e) N, (f) Cd, (g) S, and (h) C + N + Cd + S of g-C3N4/CdS-2 hybrids.
The crystal phases of g-C3N4, CdS, and g-C3N4/CdS samples were analyzed by XRD. As shown in Figure 3A, all diffraction peaks for g-C3N4 and CdS can be observed. The two strong diffraction peaks clearly shown at 12.8o and 27.6o can be ascribed to (100) and (002) crystal planes of g-C3N4 (PDF#50-1,250), which can be associated with typical in-plane tri-s-triazine and graphitic stacking of g-C3N4 (Xu et al., 2020). Furthermore, diffraction peaks at 24.8o, 26.5o, and 28.3o are well matched for those of hexagonal CdS (PDF#41-1,049). The pore structures and specific surface areas for prepared samples were determined by BET measurements. The corresponding results of sample CdS nanoparticles, g-C3N4 nanosheets, and g-C3N4/CdS-(1-3) hybrids are given in Figure 3B and Supplementary Figure S2, suggesting the existence of mesoporous pores. The SBET of sample g-C3N4/CdS-2 is the highest SBET among all the prepared samples, which can provide more reactive sites and ensure better contact catalysts with reagents during the photocatalytic reactions. The light absorption of pristine g-C3N4, CdS nanoparticles, and the as-prepared g-C3N4/CdS samples are shown in Figures 3C,D. The absorption around 470 nm is assigned for pristine g-C3N4. It can be obviously observed that the absorption ranges of prepared g-C3N4/CdS samples were extended. The resulting values of Eg for CdS, g-C3N4, and g-C3N4/CdS-(1-3) samples were 2.16, 2.60, 2.11, 1.91, and 2.05 eV, respectively. The enhanced absorption for g-C3N4/CdS is probably due to the formation of heterojunctions. Sample g-C3N4/CdS-2 with appropriate CdS particle size has the lowest bandgap, the stronger background absorption could be ascribed to the synergistic interaction between g-C3N4 nanosheets and CdS nanoparticles, and the photoinduced electrons from the LUMO of CdS could adequately inject into the conduction band of g-C3N4, leading to the reduced initial bandgap values of CdS and g-C3N4, separately (Al Marzouqi et al., 2019).
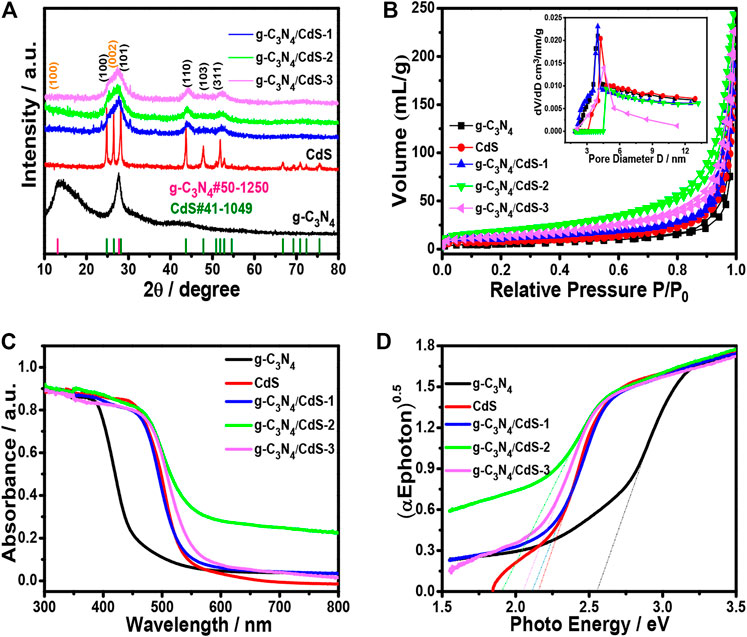
FIGURE 3. (A) XRD patterns and (B) nitrogen adsorption–desorption isotherms of g-C3N4, CdS, and g-C3N4/CdS. Inset in Figure 3B is the corresponding BJH pore size distribution. (C) UV-Vis of absorption spectra and (D) the corresponding (αEphoton)0.5 vs photon energy curves.
The surface composition of the g-C3N4, CdS, and g-C3N4/CdS samples was studied by XPS analyses, as shown in Figure 4. The survey XPS spectra (Figure 4A) provide the C 1s and N 1s peaks for g-C3N4, as well as S 2p and Cd 3 days peak for CdS. As shown in Figure 4B, the C 1s peak for g-C3N4/CdS at 284.3 eV, which is assigned to sp2 C-C bonds from graphitic carbon. The other two peaks at 286.2 and 288.1 eV are attributed to N-C=N and π-excitation, respectively (Gao et al., 2014; Liang et al., 2019; Xu et al., 2020), These peaks show a slight shift while compared with g-C3N4. In Figure 4C, the two peaks at 405.5 and 412.2 eV could be ascribed to Cd 3d5/2 and Cd 3d3/2, of CdS from g-C3N4/CdS. In addition, the peaks at 161.1 and 162.3 eV are associated with S 2p3/2 and S 2p1/2 of CdS, respectively (Figure 4D; Ren et al., 2019; Wang et al., 2019; Ai et al., 2020). These peaks show a slight shift while compared with pristine CdS or g-C3N4, which confirm the interaction between the heterojunctions.
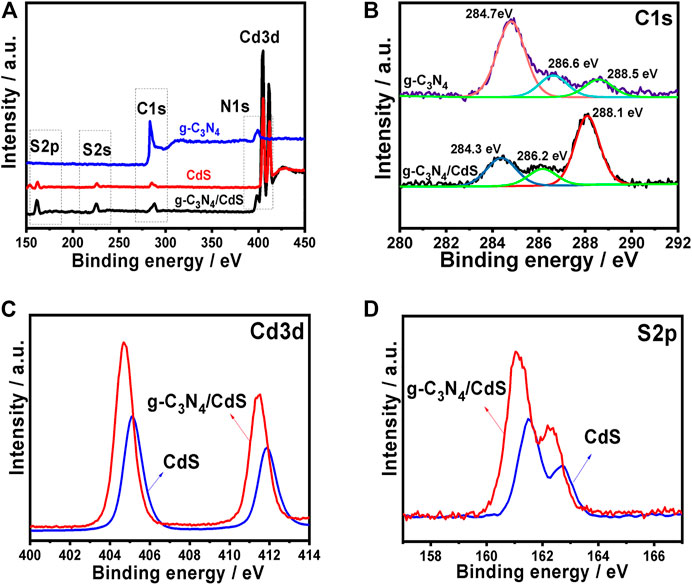
FIGURE 4. Fully scanned XPS spectra of (A) g-C3N4, CdS, and g-C3N4/CdS; high-resolution XPS plots of (B) C 1 s, (C) Cd 3 days, and (D) S 2p.
Photocatalytic Performances and Mechanism Discussion
The photocatalytic activities of g-C3N4, CdS, and g-C3N4/CdS samples were first evaluated with degradation of organic pollution, as given in Figure 5. It can be seen from Figures 5A,B that coupling with CdS shows a significant effect on RhB degradation for g-C3N4/CdS. The photocatalytic activity shows a trend of initially increasing and then decreasing with the increasing of CdS contents. The degradation efficiency of RhB can reach up to 99% within 40 min for g-C3N4/CdS-2 hybrids, which is remarkably enhanced than that for individual g-C3N4 nanosheets and CdS nanoparticles. The photocatalytic rate constant for the prepared g-C3N4/CdS-2 is 0.0849 min−1, about 5.5 times higher than that for g-C3N4. The photocatalytic results demonstrate that the anchoring of CdS nanoparticles onto g-C3N4 nanosheets can remarkably be conducive to photocatalytic performance. The enhanced activity for sample g-C3N4/CdS-2 can be attributed to well-contacted interfaces, effective charge separation, stronger light absorption, and a higher surface area. Furthermore, the TOC analyzer was measured to investigate the mineralization of RhB. The changed TOC values during the photocatalytic process were tested, as shown in Supplementary Table S1 and Supplementary Figure S3. The decayed TOC values suggest that RhB was decomposed into inorganic small molecules. As for MB (Figures 5C,D) and phenol (Figures 5E,F) degradation, g-C3N4/CdS-2 sample also exhibits much higher activity than others, where 96% of MB and 95% of phenol are degraded within 40 and 50 min, respectively. The degradation rate constants k for MB and phenol of prepared samples follow the same order with RhB degradation (Figure 6A). Moreover, the g-C3N4/CdS-2 sample can be recycled for six cycles without obviously decayed activity, and no scattering of CdS particles was found, indicating their excellent recyclability and stability (Figure 6B and Supplementary Figure S4).
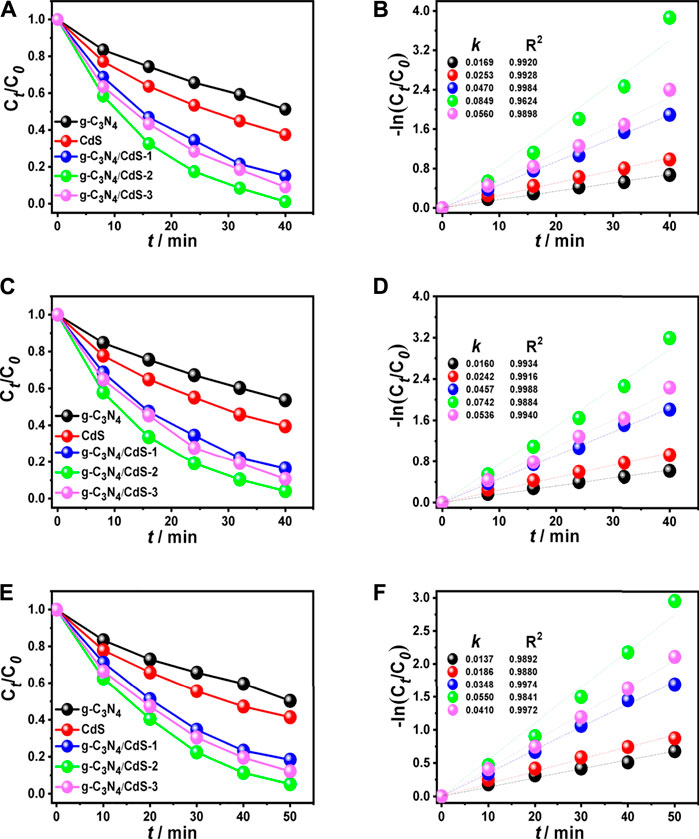
FIGURE 5. Photocatalytic degradation under visible light irradiation of (A) RhB, (C) MB, and (E) phenol, and (B,D,F) corresponding apparent reaction rates using g-C3N4, CdS, and g-C3N4/CdS hybrids.
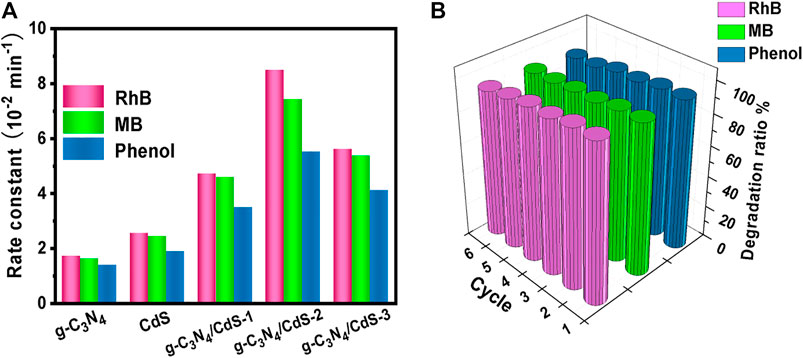
FIGURE 6. (A) Comparison of rate constants of g-C3N4, CdS, and g-C3N4/CdS hybrids for organic pollution degradation (RhB, MB, and phenol) under visible light irradiation. (B) Recyclability measurements of RhB, MB, and phenol degradation under visible light irradiation by g-C3N4/CdS hybrids.
The photocatalytic hydrogen evolution of prepared samples was investigated under visible-light irradiation with triethanolamine as the sacrificial reagent. As shown in Figures 7A,B, within 4 h measurement, pure g-C3N4 shows poor catalytic activity with the hydrogen generation rate of 237.8 μmol g−1 h −1, and the hydrogen generation rate using g-C3N4 nanosheets is obviously improved after anchoring with CdS. The hydrogen evolution rate is increased first and then decayed with increasing CdS content, and the g-C3N4/CdS-2 sample exhibits the best hydrogen evolution rate (1,070.9 μmol g−1 h −1), about 4 times as high as that for pure g-C3N4. The photocatalytic performance for g-C3N4/CdS-2 sample also surpasses the reported g-C3N4/CdS hybrids (Supplementary Table S2). In addition, the recyclability of photocatalytic hydrogen evolution was also measured, as shown in Supplementary Figure S5a. It can be seen that the hydrogen evolution performance of g-C3N4/CdS-2 shows no remarkable decline after 12 h, confirming its good stability.
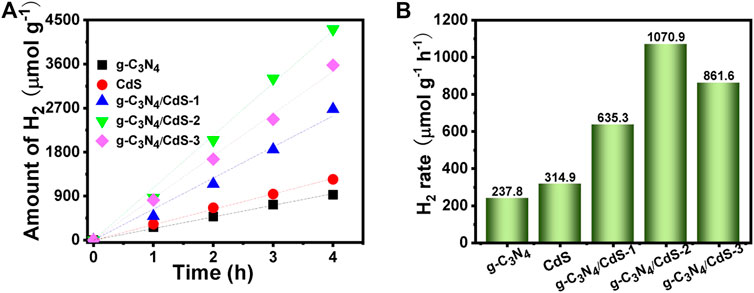
FIGURE 7. (A,B) Photocatalytic hydrogen evolution on g-C3N4, CdS, and g-C3N4/CdS hybrids under visible light.
As observed in Figure 8A, the photocurrent response of prepared samples is detected by photoelectrochemical testing to study the charge separation. It can be found that g-C3N4/CdS heterostructured samples show much higher photocurrent intensity than individual CdS and g-C3N4, which can possibly be ascribed to the heterostructure-induced acceleration of charge separation and transfer. (Tian et al., 2017; Ren et al., 2018; Zhu et al., 2019; Xu et al., 2019; Putri et al., 2020). In order to conduct an in-depth study on photocatalytic degradation, the scavenger test was performed using methanol as the scavenger. As shown in Supplementary Figure S5b, the photocatalytic performance of the RhB photodegradation rate is remarkably decreased after adding methanol, suggesting that the photogenerated holes play an important role in the photocatalytic process. In view of the results, a possible mechanism for g-C3N4/CdS sample with enhanced photocatalytic performance is put forward. The narrowed bandgap semiconductors g-C3N4 and CdS can be excited under light irradiation. Briefly, the electrons produced from g-C3N4 will transfer to CdS owing to the well-contacted interface, and the excited holes can move from CdS to the VB of g-C3N4. Consequently, the separation of photoinduced electrons and holes is effectively improved, and the lifetime of charges is improved (Scheme 2). The transferred strongly oxidizing holes on the VB of g-C3N4 can not only effectively inhibit photocorrosive damage to CdS during the reaction process but also directly degrade the target molecules. In the process of photocatalytic reaction, the photoexcited electrons may reduce H+ into hydrogen. Meanwhile, the accumulated electrons on the surface of CdS can oxidize the adsorbed dissolved O2 to produce •O2− and subsequently form •OH. The formation of powerful reactive species (•O2− and •OH) can effectively degrade the organic pollutant molecules (Huo et al., 2012; Wu et al., 2018). The produced hydroxyl radicals can be measured by the fluorescence method with terephthalic acid as the probe reactant (Xiao et al., 2008; Yu et al., 2009; Wang et al., 2017b). The fluorescence spectra of all samples are shown in Figure 8B, where sample g-C3N4/CdS-2 can produce the largest amount of hydroxyl radicals. The obtained results are well consistent with those of the photocatalytic tests. The higher photocatalytic activity for g-C3N4/CdS sample can be ascribed to two main factors: 1) the significantly promoted charge separation by the constructed heterojunctions and 2) the higher specific surface area and excellent dispersion of prepared samples.
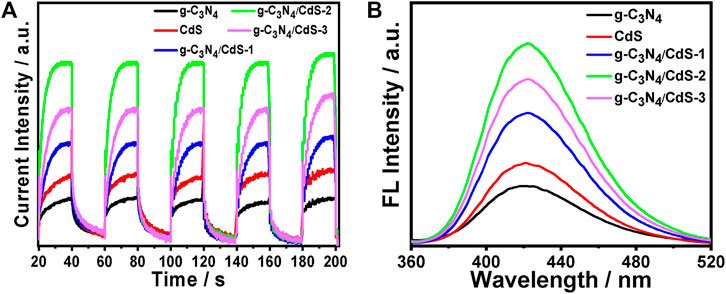
FIGURE 8. (A) Photocurrents of g-C3N4, CdS, and g-C3N4/CdS hybrids under visible light irradiation under 0.8 V versus Ag/AgCl electrode bias. (B) Fluorescence spectra of g-C3N4, CdS, and g-C3N4/CdS hybrids in a basic solution of terephthalic acid under visible light irradiation at a fixed time.
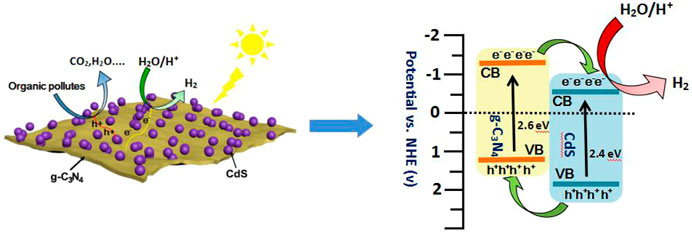
SCHEME 2. Schematic illustration of band structure diagram and photoinduced carrier transfer of g-C3N4/CdS hybrids in the piezoelectric field under visible light irradiation.
Conclusion
Heterostructured g-C3N4/CdS hybrids with controllable CdS nanoparticle anchoring on g-C3N4 nanosheets have been successfully prepared. The as-prepared samples show remarkably improved photocatalytic activity for organic pollute degradation and hydrogen generation under visible light. The experimental results demonstrate that the g-C3N4/CdS heterostructures exhibited superior photocatalytic hydrogen generation activity than individual g-C3N4 nanosheets and CdS nanoparticles, and the g-C3N4/CdS-2 sample has the highest visible light hydrogen generation rate as 1,070.9 μmol h−1 g−1, which is 4 times higher than that for pure g-C3N4. The enhanced activity and stability are attributed to the intimated heterojunction between g-C3N4 nanosheets and CdS nanoparticles, which promotes interfacial charge separation and transportation. Finally, the well-dispersed CdS nanoparticles offer more reactive sites. The g-C3N4/CdS hybrids demonstrate high photocatalytic activity, stability, and recyclability, and hold great promise for practical application. This work could provide new perspective into the construction and manufacture of heterojunctions with highly efficient charge separation and migration for the solar energy conversion.
Data Availability Statement
The original contributions presented in the study are included in the article/Supplementary Material; further inquiries can be directed to the corresponding authors.
Author Contributions
MGW performed the main experiment. JH conceived and directed the project. MGW, MW, and FP analyzed all the data and drew the figures. MGW wrote the manuscript with the help of XHS and JH. All authors agreed to the published version of the manuscript.
Funding
The authors gratefully acknowledge financial support from the National Natural Science Foundation of China (21922202), the Natural Science Research Project of Jiangsu Higher Education Institutions (19KJB150043 and 18KJB430031), and Qing Lan Project of Jiangsu Higher Education Institutions. We would also like to acknowledge the technical support received at the Testing Center of Yangzhou University.
Conflict of Interest
The authors declare that the research was conducted in the absence of any commercial or financial relationships that could be construed as a potential conflict of interest.
Publisher’s Note
All claims expressed in this article are solely those of the authors and do not necessarily represent those of their affiliated organizations, or those of the publisher, the editors, and the reviewers. Any product that may be evaluated in this article, or claim that may be made by its manufacturer, is not guaranteed or endorsed by the publisher.
Supplementary Material
The Supplementary Material for this article can be found online at: https://www.frontiersin.org/articles/10.3389/fchem.2021.746031/full#supplementary-material
References
Ai, Z., Zhang, K., Shi, D., Chang, B., Shao, Y., Zhang, L., et al. (2020). Band-matching Transformation between CdS and BCNNTs with Tunable P-N Homojunction for Enhanced Photocatalytic Pure Water Splitting. Nano Energy 69, 104408. doi:10.1016/j.nanoen.2019.104408
Al Marzouqi, F., Kim, Y., and Selvaraj, R. (2019). Shifting of the Band Edge and Investigation of Charge Carrier Pathways in the CdS/g-C3n4 Heterostructure for Enhanced Photocatalytic Degradation of Levofloxacin. New J. Chem. 43, 9784–9792. doi:10.1039/c9nj01782h
Bai, X., Wang, L., Zong, R., and Zhu, Y. (2013). Photocatalytic Activity Enhanced via G-C3n4 Nanoplates to Nanorods. J. Phys. Chem. C. 117, 9952–9961. doi:10.1021/jp402062d
Cao, R., Yang, H., Zhang, S., and Xu, X. (2019). Engineering of Z-Scheme 2D/3D Architectures with Ni(OH)2 on 3D Porous G-C3n4 for Efficiently Photocatalytic H2 Evolution. Appl. Catal. B: Environ. 258, 117997. doi:10.1016/j.apcatb.2019.117997
Chang, X., Wang, T., and Gong, J. (2016). CO2 Photo-Reduction: Insights into CO2 Activation and Reaction on Surfaces of Photocatalysts. Energy Environ. Sci. 9, 2177–2196. doi:10.1039/c6ee00383d
Chen, L., Xu, Y., and Chen, B. (2019). In Situ photochemical Fabrication of CdS/g-C3n4 Nanocomposites with High Performance for Hydrogen Evolution under Visible Light. Appl. Catal. B: Environ. 256, 117848. doi:10.1016/j.apcatb.2019.117848
Chen, X., Shen, S., Guo, L., and Mao, S. S. (2010). Semiconductor-based Photocatalytic Hydrogen Generation. Chem. Rev. 110, 6503–6570. doi:10.1021/cr1001645
Chiu, Y.-H., Naghadeh, S. B., Lindley, S. A., Lai, T.-H., Kuo, M.-Y., Chang, K.-D., et al. (2019). Yolk-shell Nanostructures as an Emerging Photocatalyst Paradigm for Solar Hydrogen Generation. Nano Energy 62, 289–298. doi:10.1016/j.nanoen.2019.05.008
Cui, Y. (2015). In-situ Synthesis of C3N4/CdS Composites with Enhanced Photocatalytic Properties. Chin. J. Catal. 36, 372–379. doi:10.1016/s1872-2067(14)60237-0In
Deng, P., Xiong, J., Lei, S., Wang, W., Ou, X., Xu, Y., et al. (2019). Nickel Formate Induced High-Level In Situ Ni-Doping of G-C3n4 for a Tunable Band Structure and Enhanced Photocatalytic Performance. J. Mater. Chem. A. 7, 22385–22397. doi:10.1039/c9ta04559g
Duan, L., Liu, H., Muhammad, Y., Shi, L., Wu, H., Zhang, J., et al. (2019). Photo-mediated Co-loading of Highly Dispersed MnOx-Pt on G-C3n4 Boosts the Ambient Catalytic Oxidation of Formaldehyde. Nanoscale 11, 8160–8169. doi:10.1039/c8nr08731h
Fan, G., Du, B., Zhou, J., Yu, W., Chen, Z., and Yang, S. (2020). Stable Ag2O/g-C3n4 P-N Heterojunction Photocatalysts for Efficient Inactivation of Harmful Algae under Visible Light. Appl. Catal. B: Environ. 265, 118610. doi:10.1016/j.apcatb.2020.118610
Fu, J., Chang, B., Tian, Y., Xi, F., and Dong, X. (2013). Novel C3N4-CdS Composite Photocatalysts with Organic-Inorganic Heterojunctions: In Situ Synthesis, Exceptional Activity, High Stability and Photocatalytic Mechanism. J. Mater. Chem. A. 1, 3083–3090. doi:10.1039/c2ta00672c
Fu, J., Xu, Q., Low, J., Jiang, C., and Yu, J. (2019). Ultrathin 2D/2D WO3/g-C3n4 Step-Scheme H2-Production Photocatalyst. Appl. Catal. B: Environ. 243, 556–565. doi:10.1016/j.apcatb.2018.11.011
Gao, D., Xu, Q., Zhang, J., Yang, Z., Si, M., Yan, Z., et al. (2014). Defect-related Ferromagnetism in Ultrathin Metal-free G-C3n4 Nanosheets. Nanoscale 6, 2577–2581. doi:10.1039/c3nr04743a
Guo, S., Tang, Y., Xie, Y., Tian, C., Feng, Q., Zhou, W., et al. (2017). P-doped Tubular G-C3n4 with Surface Carbon Defects: Universal Synthesis and Enhanced Visible-Light Photocatalytic Hydrogen Production. Appl. Catal. B: Environ. 218, 664–671. doi:10.1016/j.apcatb.2017.07.022
Han, C., Li, J., Ma, Z., Xie, H., Waterhouse, G. I. N., Ye, L., et al. (2018). Black Phosphorus Quantum Dot/g-C3n4 Composites for Enhanced CO2 Photoreduction to CO. Sci. China Mater. 61, 1159–1166. doi:10.1007/s40843-018-9245-y
Huo, Y., Zhang, J., Miao, M., and Jin, Y. (2012). Solvothermal Synthesis of Flower-like BiOBr Microspheres with Highly Visible-Light Photocatalytic Performances. Appl. Catal. B: Environ. 111-112, 334–341. doi:10.1016/j.apcatb.2011.10.016
Ji, C., Yin, S.-N., Sun, S., and Yang, S. (2018). An In Situ Mediator-free Route to Fabricate Cu2O/g-C3n4 Type-II Heterojunctions for Enhanced Visible-Light Photocatalytic H2 Generation. Appl. Surf. Sci. 434, 1224–1231. doi:10.1016/j.apsusc.2017.11.233
Li, W., Guo, Z., Jiang, L., Zhong, L., Li, G., Zhang, J., et al. (2020). Facile In Situ Reductive Synthesis of Both Nitrogen Deficient and Protonated G-C3n4 Nanosheets for the Synergistic Enhancement of Visible-Light H2 Evolution. Chem. Sci. 11, 2716–2728. doi:10.1039/c9sc05060d
Liang, M., Borjigin, T., Zhang, Y., Liu, B., Liu, H., and Guo, H. (2019). Controlled Assemble of Hollow Heterostructured G-C3N4@CeO2 with Rich Oxygen Vacancies for Enhanced Photocatalytic CO2 Reduction. Appl. Catal. B: Environ. 243, 566–575. doi:10.1016/j.apcatb.2018.11.010
Lin, B., Yang, G., Yang, B., and Zhao, Y. (2016). Construction of Novel Three Dimensionally Ordered Macroporous Carbon Nitride for Highly Efficient Photocatalytic Activity. Appl. Catal. B: Environ. 198, 276–285. doi:10.1016/j.apcatb.2016.05.069
Liu, C., Huang, H., Ye, L., Yu, S., Tian, N., Du, X., et al. (2017). Intermediate-mediated Strategy to Horn-like Hollow Mesoporous Ultrathin G-C3n4 Tube with Spatial Anisotropic Charge Separation for superior Photocatalytic H2 Evolution. Nano Energy 41, 738–748. doi:10.1016/j.nanoen.2017.10.031
Liu, J., Fang, W., Wei, Z., Qin, Z., Jiang, Z., and Shangguan, W. (2018). Efficient Photocatalytic Hydrogen Evolution on N-Deficient G-C3n4 Achieved by a Molten Salt post-treatment Approach. Appl. Catal. B: Environ. 238, 465–470. doi:10.1016/j.apcatb.2018.07.021
Liu, Y., Zhang, P., Tian, B., and Zhang, J. (2015). Core-Shell Structural CdS@SnO2 Nanorods with Excellent Visible-Light Photocatalytic Activity for the Selective Oxidation of Benzyl Alcohol to Benzaldehyde. ACS Appl. Mater. Inter. 7, 13849–13858. doi:10.1021/acsami.5b04128
Ng, S. F., Lau, M. Y. L., and Ong, W. J. (2021). Engineering Layered Double Hydroxide-Based Photocatalysts toward Artificial Photosynthesis: State‐of‐the‐Art Progress and Prospects. Sol. RRL 5, 2000535. doi:10.1002/solr.202000535
Niu, P., Qiao, M., Li, Y., Huang, L., and Zhai, T. (2018). Distinctive Defects Engineering in Graphitic Carbon Nitride for Greatly Extended Visible Light Photocatalytic Hydrogen Evolution. Nano Energy 44, 73–81. doi:10.1016/j.nanoen.2017.11.059
Ong, W. J., Putri, L. K., and Mohamed, A. R. (2020). Rational Design of Carbon‐Based 2D Nanostructures for Enhanced Photocatalytic CO 2 Reduction: A Dimensionality Perspective. Chem. Eur. J. 26, 9710–9748. doi:10.1002/chem.202000708
Putri, L. K., Ng, B.-J., Ong, W.-J., Lee, H. W., Chang, W. S., Mohamed, A. R., et al. (2020). Energy Level Tuning of CdSe Colloidal Quantum Dots in Ternary 0D-2D-2D CdSe QD/B-rGO/O-gC3N4 as Photocatalysts for Enhanced Hydrogen Generation. Appl. Catal. B: Environ. 265, 118592. doi:10.1016/j.apcatb.2020.118592
Qi, J., Zhang, W., and Cao, R. (2018). Solar-to-hydrogen Energy Conversion Based on Water Splitting. Adv. Energ. Mater. 8, 1701620. doi:10.1002/aenm.201701620
Ren, J.-T., Yuan, K., Wu, K., Zhou, L., and Zhang, Y.-W. (2019). A Robust CdS/In2O3 Hierarchical Heterostructure Derived from a Metal-Organic Framework for Efficient Visible-Light Photocatalytic Hydrogen Production. Inorg. Chem. Front. 6, 366–375. doi:10.1039/c8qi01202d
Ren, X., Gao, P., Kong, X., Jiang, R., Yang, P., Chen, Y., et al. (2018). NiO/Ni/TiO2 Nanocables with Schottky/p-N Heterojunctions and the Improved Photocatalytic Performance in Water Splitting under Visible Light. J. Colloid Interf. Sci. 530, 1–8. doi:10.1016/j.jcis.2018.06.071
Shi, L., Chang, K., Zhang, H., Hai, X., Yang, L., Wang, T., et al. (2016). Drastic Enhancement of Photocatalytic Activities over Phosphoric Acid Protonated Porous G-C3N4Nanosheets under Visible Light. Small 12, 4431–4439. doi:10.1002/smll.201601668
Shi, X., Fujitsuka, M., Lou, Z., Zhang, P., and Majima, T. (2017). In Situ nitrogen-doped Hollow-TiO2/g-C3n4 Composite Photocatalysts with Efficient Charge Separation Boosting Water Reduction under Visible Light. J. Mater. Chem. A. 5, 9671–9681. doi:10.1039/c7ta01888f
Sun, J., Zhang, J., Zhang, M., Antonietti, M., Fu, X., and Wang, X. (2012). Bioinspired Hollow Semiconductor Nanospheres as Photosynthetic Nanoparticles. Nat. Commun. 3, 1139. doi:10.1038/ncomms2152
Tahir, M., Cao, C., Mahmood, N., Butt, F. K., Mahmood, A., Idrees, F., et al. (2014). Multifunctional G-C3n4 Nanofibers: A Template-Free Fabrication and Enhanced Optical, Electrochemical, and Photocatalyst Properties. ACS Appl. Mater. Inter. 6, 1258–1265. doi:10.1021/am405076b
Teng, Z., Lv, H., Wang, C., Xue, H., Pang, H., and Wang, G. (2017). Bandgap Engineering of Ultrathin Graphene-like Carbon Nitride Nanosheets with Controllable Oxygenous Functionalization. Carbon 113, 63–75. doi:10.1016/j.carbon.2016.11.030
Tian, Q., Wu, W., Liu, J., Wu, Z., Yao, W., Ding, J., et al. (2017). Dimensional Heterostructures of 1D CdS/2D ZnIn2S4 Composited with 2D Graphene: Designed Synthesis and superior Photocatalytic Performance. Dalton Trans. 46, 2770–2777. doi:10.1039/c7dt00018a
Tong, H., Ouyang, S., Bi, Y., Umezawa, N., Oshikiri, M., and Ye, J. (2012). Nano-photocatalytic Materials: Possibilities and Challenges. Adv. Mater. 24, 229–251. doi:10.1002/adma.201102752
Vu, N.-N., Kaliaguine, S., and Do, T.-O. (2020). Synthesis of the G-C3N4/CdS Nanocomposite with a Chemically Bonded Interface for Enhanced Sunlight-Driven CO2 Photoreduction. ACS Appl. Energ. Mater. 3, 6422–6433. doi:10.1021/acsaem.0c00656
Wan, C., Zhou, L., Sun, L., Xu, L., Cheng, D.-g., Chen, F., et al. (2020). Boosting Visible-Light-Driven Hydrogen Evolution from Formic Acid over AgPd/2D G-C3n4 Nanosheets Mott-Schottky Photocatalyst. Chem. Eng. J. 396, 125229. doi:10.1016/j.cej.2020.125229
Wang, M., Han, J., Hu, Y., and GuoMesoporous, R. C. (2017). Mesoporous C, N-Codoped TiO2 Hybrid Shells with Enhanced Visible Light Photocatalytic Performance. RSC Adv. 7, 15513–15520. doi:10.1039/c7ra00985b
Wang, P., Wu, T., Wang, C., Hou, J., Qian, J., and Ao, Y. (2017). Combining Heterojunction Engineering with Surface Cocatalyst Modification to Synergistically Enhance the Photocatalytic Hydrogen Evolution Performance of Cadmium Sulfide Nanorods. ACS Sustain. Chem. Eng. 5, 7670–7677. doi:10.1021/acssuschemeng.7b01043
Wang, S., Zhu, B., Liu, M., Zhang, L., Yu, J., and Zhou, M. (2019). Direct Z-Scheme ZnO/CdS Hierarchical Photocatalyst for Enhanced Photocatalytic H2-Production Activity. Appl. Catal. B: Environ. 243, 19–26. doi:10.1016/j.apcatb.2018.10.019
Wu, Y., Wang, H., Tu, W., Wu, S., Liu, Y., Tan, Y. Z., et al. (2018). Petal-like CdS Nanostructures Coated with Exfoliated Sulfur-Doped Carbon Nitride via Chemically Activated Chain Termination for Enhanced Visible-Light-Driven Photocatalytic Water Purification and H2 Generation. Appl. Catal. B: Environ. 229, 181–191. doi:10.1016/j.apcatb.2018.02.029
Xiao, Q., Si, Z., Zhang, J., Xiao, C., and Tan, X. (2008). Photoinduced Hydroxyl Radical and Photocatalytic Activity of Samarium-Doped TiO2 Nanocrystalline. J. Hazard. Mater. 150, 62–67. doi:10.1016/j.jhazmat.2007.04.045
Xu, F., Mo, Z., Yan, J., Fu, J., Song, Y., El-Alami, W., et al. (2020). Nitrogen-rich Graphitic Carbon Nitride Nanotubes for Photocatalytic Hydrogen Evolution with Simultaneous Contaminant Degradation. J. Colloid Interf. Sci. 560, 555–564. doi:10.1016/j.jcis.2019.10.089
Xu, H., Wang, Y., Dong, X., Zheng, N., Ma, H., and Zhang, X. (2019). Fabrication of In2O3/In2S3 Microsphere Heterostructures for Efficient and Stable Photocatalytic Nitrogen Fixation. Appl. Catal. B: Environ. 257, 117932. doi:10.1016/j.apcatb.2019.117932
Yang, F., Liu, D., Li, Y., Cheng, L., and Ye, J. (2019). Salt-template-assisted Construction of Honeycomb-like Structured G-C3n4 with Tunable Band Structure for Enhanced Photocatalytic H2 Production. Appl. Catal. B: Environ. 240, 64–71. doi:10.1016/j.apcatb.2018.08.072
Yu, H., Shi, R., Zhao, Y., Bian, T., Zhao, Y., Zhou, C., et al. (2017). Alkali-assisted Synthesis of Nitrogen Deficient Graphitic Carbon Nitride with Tunable Band Structures for Efficient Visible-Light-Driven Hydrogen Evolution. Adv. Mater. 29, 1605148. doi:10.1002/adma.201605148
Yu, J., Wang, W., Cheng, B., and Su, B.-L. (2009). Enhancement of Photocatalytic Activity of Mesporous TiO2 Powders by Hydrothermal Surface Fluorination Treatment. J. Phys. Chem. C. 113, 6743–6750. doi:10.1021/jp900136q
Yu, X., Ng, S. F., Putri, L. K., Tan, L. L., Mohamed, A. R., and Ong, W. J. (2021). Point‐Defect Engineering: Leveraging Imperfections in Graphitic Carbon Nitride (g‐C 3 N 4 ) Photocatalysts toward Artificial Photosynthesis. Small, 2006851. doi:10.1002/smll.202006851
Zhang, G., Lan, Z.-A., Lin, L., Lin, S., and Wang, X. (2016). Overall Water Splitting by Pt/g-C3N4photocatalysts without Using Sacrificial Agents. Chem. Sci. 7, 3062–3066. doi:10.1039/c5sc04572j
Zhang, J., Wang, Y., Jin, J., Zhang, J., Lin, Z., Huang, F., et al. (2013). Efficient Visible-Light Photocatalytic Hydrogen Evolution and Enhanced Photostability of Core/Shell CdS/g-C3n4 Nanowires. ACS Appl. Mater. Inter. 5, 10317–10324. doi:10.1021/am403327g
Zhang, P., Liu, Y., Tian, B., Luo, Y., and Zhang, J. (2017). Synthesis of Core-Shell Structured CdS@CeO 2 and CdS@TiO 2 Composites and Comparison of Their Photocatalytic Activities for the Selective Oxidation of Benzyl Alcohol to Benzaldehyde. Catal. Today 281, 181–188. doi:10.1016/j.cattod.2016.05.042
Zhou, C., Shi, R., Shang, L., Wu, L.-Z., Tung, C.-H., and Zhang, T. (2018). Template-free Large-Scale Synthesis of G-C3n4 Microtubes for Enhanced Visible Light-Driven Photocatalytic H2 Production. Nano Res. 11, 3462–3468. doi:10.1007/s12274-018-2003-2
Keywords: G-C3N4, CdS, hybrids, photocatalyst, visible light
Citation: Wang M, Wang M, Peng F, Sun X and Han J (2021) Fabrication of g-C3N4 Nanosheets Anchored With Controllable CdS Nanoparticles for Enhanced Visible-Light Photocatalytic Performance. Front. Chem. 9:746031. doi: 10.3389/fchem.2021.746031
Received: 23 July 2021; Accepted: 02 September 2021;
Published: 14 October 2021.
Edited by:
Wee-Jun Ong, Xiamen University Malaysia, MalaysiaReviewed by:
Lutfi Kurnianditia Putri, Universiti Sains Malaysia (USM), MalaysiaLi Yuhan, Chongqing Technology and Business University, China
Copyright © 2021 Wang, Wang, Peng, Sun and Han. This is an open-access article distributed under the terms of the Creative Commons Attribution License (CC BY). The use, distribution or reproduction in other forums is permitted, provided the original author(s) and the copyright owner(s) are credited and that the original publication in this journal is cited, in accordance with accepted academic practice. No use, distribution or reproduction is permitted which does not comply with these terms.
*Correspondence: Jie Han, hanjie@yzu.edu.cn