- 1Pharmaceutical Biotechnology, Fraunhofer Institute for Toxicology and Experimental Medicine (ITEM), Braunschweig, Germany
- 2Institute of Microbiology, Braunschweig University of Technology, Braunschweig, Germany
- 3Leibniz Institute DSMZ-German Collection of Microorganisms and Cell Cultures GmbH (DSMZ), Braunschweig, Germany
- 4Department Trauma & Orthopedic Surgery, Septic & Reconstructive Surgery, Research and Treatment Center Septic Defect Wounds, Federal Armed Forces of Germany, Military Academic Hospital Berlin, Berlin, Germany
- 5Central Facility for Microscopy, Helmholtz Centre for Infection Research (HZI), Braunschweig, Germany
- 6Institute of Microbiology, Braunschweig Center of Systems Biology (BRICS), Braunschweig, Germany
Pseudomonas aeruginosa is an opportunistic pathogen causing severe infections of the lung, burn wounds and eyes. Due to its intrinsic high antibiotic resistance the bacterium is difficult to eradicate. A promising therapeutic option is the use of P. aeruginosa-specific bacteriophages. Thus, the implementation of a phage therapy requires their selection, production and systematic administration using multiple strains of the bacterial target. Here, we used 25 phages and tested their susceptibility on 141 different P. aeruginosa strains isolated from patients with different types of infection. Comparative host spectrum analyses were carried out using double agar overlay plaque assay (DPA) and planktonic killing assay (PKA), which resulted in 70% of the cases in the same host range. All phages were assigned to known phage genera, but some of the phages are new species. Isolated members of the genera Pakpunavirus, Pbunavirus (myoviruses), Pawinskivirus, Elvirus (myoviruses, jumbo phages), Litunavirus and Bruynoghevirus (podoviruses) demonstrated great therapeutic potential due to strong lysis behavior on diverse strains. Seven phages were excluded for therapeutic purposes due to genetic determinants that confer lysogenicity. Due to automation with lower time expenditure in execution and analysis, PKA has the higher potential for implementation in diagnostics. Finally, different combinations of phages were tested in silico with various P. aeruginosa strains. Highly efficient phage combinations eradicating multiple P. aeruginosa strains were found. Thus, a solid basis for the development of a broad host range phage therapy was laid.
1 Introduction
P. aeruginosa is a Gram-negative rod-shaped bacterium that is ubiquitous found in the environment. But, it also causes infectious diseases that often lead to long and expensive antibiotic treatments due to a large number of intrinsic antibiotic resistance and virulence factors (Aloush et al., 2006; Azam and Khan, 2019; Horcajada et al., 2019; Hilliam et al., 2020; Nazarov, 2022; Qin et al., 2022). In some cases, none of the eight antibiotic classes, including the reserve antibiotic class of carbapenems, commonly employed for P. aeruginosa infection treatment are effective anymore (Bassetti et al., 2018; Miller and Arias, 2024). For this reason P. aeruginosa is classified as high-risk pathogen on the World Health Organization’s global priority list for antibiotic-resistant bacteria (https://www.who.int/publications/i/item/9789240093461, accessed on 19 Nov 2024 (Miller and Arias, 2024; World Health Organization, 2024). Common sites of infection with P. aeruginosa in the human body are the respiratory tract, urinary tract, burn wounds, or the eyes (Poggio et al., 1989; Schein et al., 1989; Cheng et al., 1999; Serra et al., 2015; Prevaldi et al., 2016; La Rosa-Carrillo et al., 2022). Particularly in patients with predispositions like cystic fibrosis, bronchiectasis, chronic obstructive pulmonary disease (COPD), vascular diseases, diabetes, or immunocompromised patients there is a high risk of infection with P. aeruginosa (Prevaldi et al., 2016; La Rosa-Carrillo et al., 2022). Additionally, infections in difficult to reach sites in the body i. e. (eye background, sputum of cystic fibrosis lung) in combination with an intensive biofilm formation lead to poor pharmacokinetic distribution of applied antibiotics.
Consequently, other forms of bactericidal treatments are urgently required. One long-time known alternative and supplement to antibiotics is the use of bacteriophages (phages), viruses that infect bacteria. Phages show different pharmacokinetics and pharmacodynamics compared to classical drugs, as they multiply and self-regulate at the site of infection (Payne et al., 2000; Danis-Wlodarczyk et al., 2021b).
Around 1031 virus particles are estimated to exist on earth (Mushegian, 2020). But only a small part of these viruses, 2,818 genera, 84 subgenera and 11,273 species, have been classified by the International Committee for Taxonomy of Viruses (ICTV) in 2023 (International Committee of Taxonomy of Viruses, 2023). Of the sequences that can be unambiguously assigned to P. aeruginosa phages so far, about 2,148 nucleotide sequences, with 275 reference sequences and 55 different already classified genera have been deposited in the NCBI virus data base (https://www.ncbi.nlm.nih.gov/labs/virus/vssi/#/ accessed on 25 Oct 2024 (Hatcher et al., 2017; Alipour-Khezri et al., 2024). Due to the ubiquitous nature of P. aeruginosa, corresponding phages are found in soil, aquatic habitats, sewage, but also in humans and animals (Garbe et al., 2011; Amgarten et al., 2017; Shi et al., 2020; Aghaee et al., 2021; Alkalay-Oren et al., 2022; Hashemi Shahraki et al., 2023). Most P. aeruginosa phages described so far, including jumbo phages, belong to the Caudoviricetes (dsDNA genome) (Lecoutere et al., 2009; Yuan and Gao, 2017). A small part belongs to the Inoviridae (ssDNA), Fiersviridae (ssRNA) or Cystoviridae (dsRNA) (Sepúlveda-Robles et al., 2012; Pires et al., 2015; NCBI Virus, 2023). Shortly after the discovery of the phages by Frederick Twort and Félix Hubert d´Hérelle in the beginning of the last century, the latter developed the first phage therapeutic approaches together with the Georgian microbiologist Georgi Eliava. He founded the Bacteriological Institute Tiflis, later on renamed to the George Eliava Research Institute of Bacteriophage (Eliava Institute), today one of the leading institutions for phage therapy, even though most Western countries lost interest in phage therapy research during the time of the discovery and development of antibiotics. One major target of their research was the treatment of cystic fibrosis patients with P. aeruginosa infections (Parfitt, 2005; Chanishvili et al., 2022). Today, Pseudomonas phages have already shown their therapeutic potential against P. aeruginosa in several in vitro, in vivo and compassionate case studies on acute and respiratory infections, bacteremia, and wound infections (Debarbieux et al., 2010; Waters et al., 2017; Arumugam et al., 2022; Silva et al., 2022; Onallah et al., 2023; Rappo et al., 2023; Alipour-Khezri et al., 2024). The phages used therapeutically belong to twelve different phage genera - Pakpunavirus, Pbunavirus, Phikzvirus and Nankokuvirus (morphotype myovirus); Litunavirus, Bruynoghevirus, Paundecimvirus and Phikmvvirus (morphotype podovirus); Septimatrevirus and Nipunavirus (morphotype siphovirus) and Perrunavirus and Cystovirus (morphotype enveloped, spherical or icosahedral virion) (Rose et al., 2014; Ferry et al., 2021; Mabrouk et al., 2022; Silva et al., 2022; Alipour-Khezri et al., 2024; Pirnay et al., 2024; Pye et al., 2024). In general, prior to the treatments, phages were checked for an obligatory lytic lifecycle and the absence of virulence factors, toxins or antibiotic resistance genes in their genomes (Alipour-Khezri et al., 2024; Pirnay et al., 2024). Two generally different strategies exist for the application of phage therapy for the treatment of P. aeruginosa infections. Firstly, the use of a pre-composed phage cocktail such as practiced in the PhagoBurn project (Jault et al., 2019), composed of 12 phages directed against Pseudomonas infections of burn wounds, the various cocktails from the Eliava Institute in Georgia (Chanishvili, 2012) and from BiomX Gaithersburg, MD, USA (Rappo et al., 2023). In most cases their composition is not publicly available. The commercial cocktail of BiomX BX004-A in combination with antibiotics provided good tolerability and a bacterial reduction of 1.42 log in a clinical phase 1b/2a study of cystic fibrosis patients (Rappo et al., 2023). Secondly, a magistral preparation, as used in Belgium, Israel or Germany as part of the PhagoFlow project (Onallah et al., 2023; Pirnay and Verbeken, 2023; Willy et al., 2023), enables a customized selection of phages for each patient, often employed in combination with antibiotics (Onallah et al., 2023; Pirnay and Verbeken, 2023). Clinical improvement was reported for 77.2% of 100 cases of P. aeruginosa infections. In 61.3% of the cases a complete eradication of the targeted bacteria was found (Onallah et al., 2023; Pirnay et al., 2024).
A crucial factor during the establishment of phage therapeutic approaches is the quantitative determination of the efficiency phage activity using a phage susceptibility test (PST). Classically, a double agar overlay plaque assay (DPA) is performed that visualizes phage infection as plaques (Kropinski et al., 2009). Variations of DPA include the use of only one phage concentration or different dilutions applied as spots or discs with phages (RPST) (Skusa et al., 2023). Alternatively, phage lysis behavior can also be studied in liquids using a planktonic killing assay (PKA). To date, no standard conditions, cut-offs and breakpoints for bacterial lysis in the PST have been defined to determine the efficacy of phage lysis, which would contribute to comparability between laboratories (Parmar et al., 2023; Yerushalmy et al., 2023). It is already known from previous studies that the DPA and PKA methods lead to different results. One advantage of PKA is that the phages can be analyzed not only individually but also in combinations, which allows the identification of synergistic and antagonistic effects (Haines et al., 2021; Steffan et al., 2022).
Nevertheless, a systematic collection of P. aeruginosa phages tested in various combination on a broad spectrum of P. aeruginosa strain isolated from different types of infection is missing to generate generally applicable knowledge for P. aeruginosa phage therapies. This is necessary to select safe and broad host spectrum phages for a clinical magistral application to individually treat as many patients as possible. Thus, in this project, 25 phages and 141 P. aeruginosa strains were isolated, characterized, sequenced and their interaction investigated. In the context of phage susceptibility, DPA was compared with serial dilutions as spots and PKA on a large phage-host panel to reveal the advantages and disadvantages of each of the method.
2 Materials and methods
2.1 Bacterial strains and growth conditions
P. aeruginosa strains BWKH001–133 were isolated by the military hospital in Berlin and Hamburg (Germany) between 2015 and 2018. A description of the corresponding infection type (wounds, skin, repository tract, urinary tract, rectal, tissue) is given in Supplementary Data Sheet 1. Additionally, P. aeruginosa MH16, MH19, MH27, MH38 and RN21 were isolated from the urinary tract (Tielen et al., 2011, 2014). Five P. aeruginosa strains from patients with cystic fibrosis, five strains from patients with chronic obstructive pulmonary disease (COPD56-COPD129) and 14 strains of bronchiectasis (Bron08-Bron76) patients were provided by B. Tümmler (Medical School Hannover MHH, Germany) (Hamed et al., 2023). The model strains PAO1 (DSM 19880) and PA14 (DSM 19882) were obtained from the German Collection of Microorganisms and Cell Cultures (DSMZ Braunschweig, Germany) (Rahme et al., 1995; Stover et al., 2000; Mathee, 2018). All P. aeruginosa strains were cultivated aerobically in LB veggie medium (10 g/L veggie peptone, 5 g/L veggie yeast extract, 10 g/L NaCl (all Merck, Darmstadt, Germany)) at 37°C. For growth on agar plates 1.5 g/L agar (Merck, Darmstadt, Germany) was added.
2.2 Antibiograms of the P. aeruginosa strains
Bacterial strains were tested for antibiotic susceptibility using the disc diffusion method. Discs according to EUCAST concentrations were ordered by Mast Diagnostica GmbH, Reinfeld/Germany (Supplementary Data Sheet 2). Briefly, freshly streaked bacteria from cryo culture (LB veggie agar, 37°C, 18 h) were suspended in 10 mL 0.85% NaCl (Merck, Darmstadt, Germany) solution to an OD600nm of 0.25. A cotton swab was dipped once into the inoculum, squeezed, and streaked in three directions on Mueller Hinton agar plates (25 mL/dish, 38 g/L, Roth, Karlsruhe, Germany). Afterwards six antibiotic discs were placed per plate with a dispenser (Mast Diagnostica GmbH, Reinfeld, Germany). After an overnight growth (35°C, 17h ± 1h) the zone of inhibition was measured and compared to the EUCAST thresholds (European Committee on Antimicrobial Susceptibility Testing, 2022).
2.3 Phage isolation, purification, and propagation
For isolation of P. aeruginosa phages, enrichments from different environments in Germany, including clinical sewage, washing machines and garden compost were performed between 2007 and 2022 and detected by DPA as described by Kropinski et al. with minor changes (Kropinski et al., 2009). A list of the phages and their characteristic is given in Supplementary Data Sheet 3. Agar concentration in top agars for host range analyses was 0.5% (Sigma Aldrich, Darmstadt, Germany), while different top agar concentrations (0.3 to 0.7%) were applied for visualization of the individual phages (Supplementary Data Sheet 3). Clonal purification was performed by in least three consecutive rounds of single plaque picking and streaking out on double ager overlay plates. Plaque sizes were determined via ruler measurement. In general, phage lysates were prepared either in liquid form by infecting logarithmically growing P. aeruginosa cultures with phages at a multiplicity of infection (MOI) between 0.01 to 0.5 followed by an incubation at 37°C and 140 rpm until complete lysis or for 22 hours. Alternatively, top agar of double agar overlay plates were incubated with phages until semiconfluent lysis occurred, subsequently covered with SM buffer, and finally scraped off after incubation. After centrifugation (10,967 x g, 4°C, 10 min), the phage-containing supernatant was filtered through 0.2 µm syringe filters (cellulose acetate, Sartorius, Germany), quantified using DPA and stored at 4°C.
2.4 DNA isolation and determination of DNA concentration
For DNA isolation the “Phage DNA Isolation Kit” (Norgen, Thorold, Canada) was used following the manufacturer’s protocol and DNA was stored at 4°C. The concentration was determined using the Qubit® dsDNA broad range and 1x dsDNA high-sensitive assay kit (Thermo Fisher Scientific, Waltham, USA) following the manufacturer’s instructions.
2.5 Library preparation and whole genome sequencing
The protocols for library preparation and whole genome sequencing for PacBio RSII and Illumina were described before (Korf et al., 2019). Other long read sequencing of phage genomes was performed with Nanopore technology using the protocol, “Ligation sequencing gDNA - SQK-LSK109 version: GDE_9063_v109_revAP_25May2022” (ONT, Oxford, United Kingdom), flongles version 9.4.1 with default parameters and basecaller Guppy version 7.1.4 with high-accuracy model.
2.6 Genome assembly, annotation and comparison
Either SPAdes version 3.12.0 (PacBio and Illumina) or flye version 2.9.3-b1794 (ONT and Illumina) was used for genome assembly, before long reads were trimmed with porechop version 0.2.4 (threshold of 10). Short reads were mapped using BWA short reads version 0.7.17.5 with default parameters and polished using polypolish version 0.5.0 (default parameters). All genomes, including those already published, were annotated using pharokka version 1.7.1 and compared with clinker version v0.0.28. Phylogenomic tree was performed with VICTOR (Meier-Kolthoff and Göker, 2017), visualized with iTOL (Letunic and Bork, 2024) and annotation was generated with table2itol (https://github.com/mgoeker/table2itol).
2.7 Morphological analysis via transmission electron microscopy
For TEM analysis, phages were prepared for analysis as previously described (Korf et al., 2019). Briefly, thin carbon support films were prepared by evaporating a carbon thread onto a freshly cleaved mica surface. Small pieces of mica were then cut, and phages were negatively stained with 2% (w/v) aqueous uranyl acetate, pH 5.0. The samples were examined at an acceleration voltage of 80 kV/120 kV in a Zeiss EM 910 or Zeiss Libra120 Plus transmission electron microscope (Carl Zeiss, Oberkochen, Germany). The dimensions of heads and tails was determined for 3–10 different phage particles using ITEM software (Olympus Soft Imaging Solutions, Münster, Germany). The head dimension was estimated on the basis of head length x head width. The phenotypic classification was done using the morphological criteria of Ackermann (Ackermann and Eisenstark, 1974; Ackermann, 2011).
2.8 Host range analysis by DPA (double agar overlay plaque assay)
The phage host range was determined by spotting serial dilutions (adjusted to 1E9 PFU/ml) on double agar plates containing 100 µL of a logarithmic culture of the potential host. After incubation for 18h ± 2h at 37°C, the plates were examined for lysis. The host was categorized as sensitive if individual plaques could be detected (lysis), or as insensitive if either no visible plaques (no lysis) or reduced growth was observed.
2.9 Host range analysis by PKA (planktonic killing assay)
A PKA was performed to analyze the host range of the phages in liquid bacterial culture. A culture was inoculated with an overnight culture to an OD600nm of 0.05, allowed to grow above an OD600nm of 0.1 and finally adjusted to an OD600nm of 0.1. 200 µL adjusted culture were infected with 10 µL phage (2E8 PFU/mL) to reach an approximate MOI of 0.1. The optical density was measured in SpectraMax 250 (MWG-Biotech, Ebersberg, Germany) at 600 nm every 15 min for 24 h. The 96 well plate was mixed for 3 s before measurement. The host was classified as sensitive when the normalized area under curve (AUCnorm) was < 0.8 (lysis) and insensitive when AUCnorm was ≥ 0.8 (no lysis).
For all combinations that were classified as lytic, the time point of lysis was calculated. This value was set as the first time point, where AUCnorm was below 0.8.
2.10 Host range comparison of DPA and PKA
To compare DPA and PKA, corresponding results of their host spectra were merged in each possible combination. Resulting matches or deviations were grouped as follows: group 1 was “DPA and PKA tests resulted in no phage lysis”, group 2 was “DPA resulted reduced bacterial growth and PKA with no lysis”, group 3 was “DPA with lysis and PKA without lysis”, group 4 was “DPA with no lysis and PKA with lysis”, group 5 was “DPA with reduced growth and PKA with lysis”, and group 6 was “DPA and PKA with lysis”. The rows and columns of the heatmap were clustered using the distinct method in R software with resulting categories.
2.11 Determining the optimal phage combination
Optimal phage combinations based on host ranges identified by DPA and PKA were determined by Phage Cocktail Optimizer by Stephen T. Abedon (https://www.phage-therapy.org/calculators/cocktail_optimizer.html, accessed on 5 Jul 2024). “Lysis” determined as described above was rated as “positive” phage-host interaction.
2.12 MOI dependent planktonic killing assay and virulence index
The original bacterial host on which the phage was isolated was tested with different MOIs to determine the lysis behavior. The culture was inoculated from an overnight culture with OD600nm of 0.05 and grown to an OD600nm of 0.1. Serial dilutions of the culture at the time of infection were plated to determine the colony forming units (CFU) of the host P. aeruginosa. The phage was adjusted to 2E9 PFU/mL and serial diluted to 2E5 PFU/mL with LB veggie medium. 200 µL culture were infected with 10 µL phage dilutions in triplicates (final MOI between 1 to 0.0001). Optical density was measured using a SpectraMax 250 (MWG-Biotech, Ebersberg, Germany) every 15 min for 24 h. After incubation the triplicates were pooled and centrifuged (16,200 x g, 2 min, at room temperature) (Fresco 21, Thermo Scientific, Waltham, US). The phage titer t24h was determined by DPA from corresponding supernatants. Local virulence index (vi) was assessed as previously described (Storms et al., 2020). The beginning of the stationary phase was defined as the first local maximum with a threshold value of 5:
The global virulence index (vp) was calculated as the quotient of the area under the virulence curve (Ap) divided by the theoretical maximum area under the virulence curve (Amax) (Storms et al., 2020):
2.13 Ranking score
For the final ranking phages received score for the categories DPA, PKA, virulence index (vp) and safety according to Supplementary Data Sheet 4.
3 Result
3.1 Isolation and initial characterization of 25 P. aeruginosa phages
In order to systematically test multiple P. aeruginosa strains isolated from highly different infections corresponding phages were selected as a first step. For this purpose, phages were isolated from wastewater (partly with clinical proximity), garden compost and washing machines between 2004 and 2022 from locations in northern Germany. After isolation and propagation, phages were systematically characterized for their head, tail, plaque size, halo formation, plaque appearance, genome size, number of genes, GC content, virulence index and lysis behavior as shown in Figures 1, 2 and Supplementary Data Sheet 1 (complete dataset).
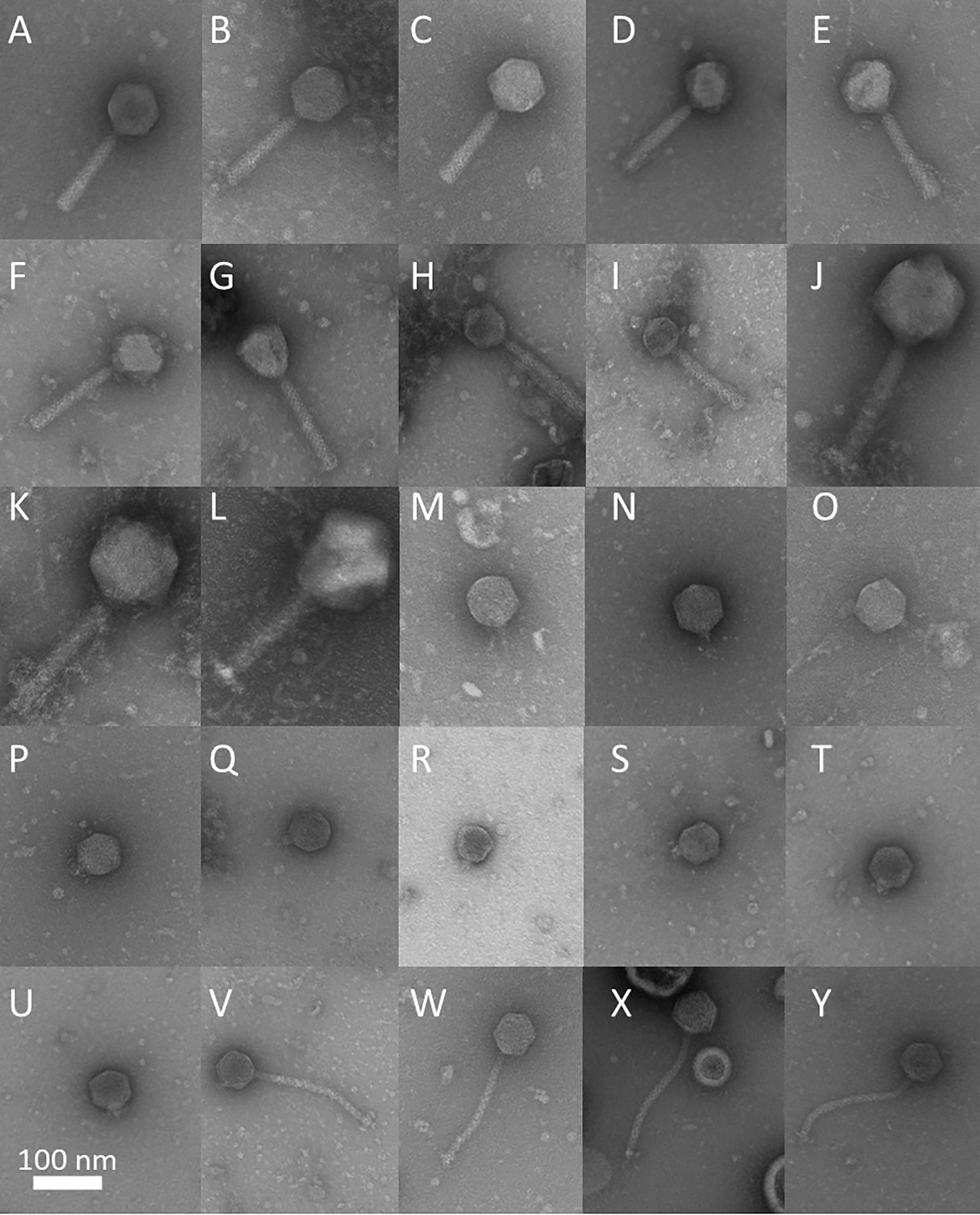
Figure 1. Electron micrographs of isolated phages with the morphotype myovirus [JG004 (A), HHBS9_1 (B), HHB18_1 (C), PTLAW1 (D), HHBS12_2 (E), HHBS42_2 (F), HHBS51_1 (G), Komp_PAO1_1 (H), BIBS67 (I), HHBS47_1 (J), HHBS8_1 (K), HHBS36_1 (L)], and morphotype podovirus [BWKH3_L8_1 (M), BWKH3_R8_1A (N), HHBS9_2 (O), HHBS10_2 (P), HHBS55_2 (Q), HHBS14_1 (R), Flu_PA14_3 (S), Flu_PA14_4 (T), Komp_PA14_gP (U)] and morphotype siphovirus (HHBS29_1 (V), 22043_B8_1 (W), Tom33 (X), Komp_PA14_H (Y). Bar represents 100 nm.
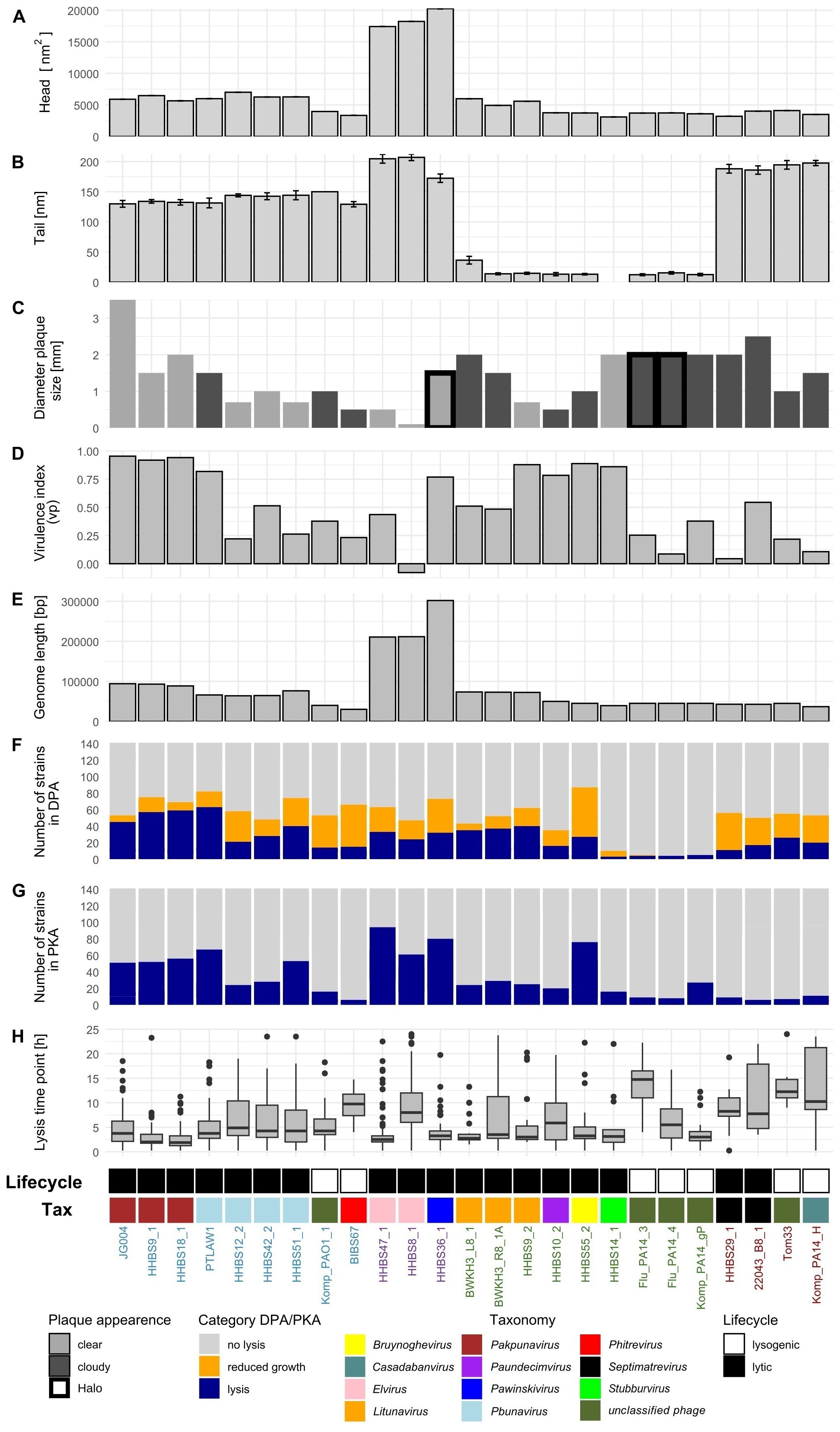
Figure 2. The diversity of the phages was assessed based on ten parameters. For all parameters phages are grouped based on morphotype (colored names at the bottom: myo- (blue) – jumbo phages (violet), podo- (green) or siphovirus (brown)) and taxonomy. Lysogenic phages are indicated in white, strictly lytic phages in black. Head size (head length x head width), tail length, plaque appearance, genome size, as well as virulence index (vp) are shown as barplots (A-E). Distribution of lysis behavior in DPA and PKA resulting in either no lysis, reduced growth or lysis are displayed in (F, G). Boxplots represent the time of lysis in PKA (first time normalized AUC was < 0.8) for all strains (H).
3.2 Phage morphology
Using transmission electron microscopy (TEM), the 25 phages were classified as 13 myoviruses including three jumbo phages, four siphoviruses and eight podoviruses (Figure 1). All phages had a symmetrical hexagonal head and were categorized in A1 (myoviruses), B1 (siphoviruses) and C1 (podoviruses) according to Ackermann and Eisenstark, 1974. Prolonged heads were not observed. When analyzing phage dimensions, the myovirus morphotype showed the greatest diversity (myoviruses: mean head height 93 ± 29 nm; mean head width 87 ± 25 nm; mean tail length 151 ± 26 nm, podoviruses: 67 ± 8 nm, 65 ± 6 nm; 16 ± 8 nm and siphoviruses 62 ± 4 nm, 59 ± 3 nm; 192 ± 5 nm (Figures 2A, B). The phages HHBS36_1 (Pawinskivirus), HHBS8_1 and HHBS47_1 (both Elviruses) are representatives of the jumbo phages with head heights ranging from 139 to 147 nm. This means that they are not only larger in terms of their genome (see Chapter 3.5.1), but also in terms of their head and tail.
3.3 Plaque morphology and halo formation of the isolated phages
The diversity of phages can also be illustrated by their plaque morphology (Figure 2C, Supplementary Figure 1). DPA was performed with different concentrations of top agar (Supplementary Data Sheet 3). Plaque size ranged between 0.1 mm at 0.3% top agar concentration for HHBS8_1 (Elvirus) to 3.5 mm at 0.7% top agar concentration for JG004 (Pakpunavirus). Most myoviruses formed clear plaques, whereas the plaques of podoviruses and siphoviruses tended to be turbid. All temperate phages (Supplementary Data Sheet 3) produced turbid plaques. Halo structures could be found through all morphotypes. However, it should be noted that only 16% of all phages studied produced halos.
3.4 Lysis behavior of isolated phages
The actual virulence and efficacy of lysis were assessed in 96-well format using different MOIs and calculating the virulence index (vp) to compare the phages for later therapeutic potential (Figure 2D). The host bacteria used were the same as those used for the isolation. A high virulence index is characterized by rapid and complete lysis. Some phages of the morphotype myovirus (vp mean 0.58 ± 0.31) and podovirus (vp mean 0.57 ± 0.28) lysed in a short period and had a high virulence index of e. g. 0.95 - JG004 (Pakpunavirus) or 0.88 - HHBS9_2 (Litunavirus). On the other hand, there were also phages from the same morphotype that had a very low virulence index of 0.23 - BIBS67 (Phitrevirus) or 0.09 – Flu_PA14_4 (unclassified phage). For HHBS29_1 (Septimatrevirus – morphotype siphovirus) lysis was only observed at high MOI (1 and 0.1) so the virulence index was correspondingly low (vp 0.04). Two myoviruses JG004 and HHBS9_1 (both Pakpunavirus) underwent a second lysis after regrowth (Supplementary Figure 2A).
3.5 Genome of the isolated phages
3.5.1 General genetic properties
Phage sequencing was performed using PacBio or ONT (long reads) and Illumina (short reads) technologies for taxonomic classification and to exclude unwanted genes. Phage genome size ranged from 30,180 bp (BIBS67 - Phitrevirus) to 302,046 bp (HHBS36_1 – Pawinskivirus) (Figure 2E). Phages of the jumbo phage genera Elvirus and Pawinskivirus reached the upper limit in both physical and genome size (mean 246,208 bp). Other myoviruses also tended to have slightly larger genomes (mean 68,619 bp) than podoviruses (56,496 bp) and siphoviruses (41,912 bp). The GC content was between 44% and 64%, while all temperate phages had higher GC content (mean 61%) then lytic phages (mean 52%).
3.5.2 Phylogenetic tree of the isolated phages
The diversity of P. aeruginosa phages can also be demonstrated by examining their genome. At the time of the study, 18,673 reference genomes were deposited in the NCBI Virus Database (https://www.ncbi.nlm.nih.gov/labs/virus/vssi/#/ accessed on April 2nd, 2025). If all phage genomes whose host does not contain the search terms “Pseudomonas aeruginosa”, “Pseudomonas” or “Pseudomonas sp.” were removed, 275 reference genomes remained. At least one genome from each P. aeruginosa phage genus (complete reference sequence deposited in NCBI Virus Database) was selected and the relationships were presented in a phylogenetic tree (Figure 3). The distribution of morphotypes is very diverse, as it is spread across different clusters. While Pakpunavirus and Pbunavius are clearly separated, the jumbo phages all cluster together and have a relatively low GC content. Members of Bruynoghevirus, Paundecimvirus and Litunavirus share only individual genes with transcriptional regulatory or metabolic function. This makes them more closely related to each other than to Stubburvirus (e.g. HHBS14_1) or unclassified podoviruses (e.g. Flu_PA14_3). The unclassified phages including Tom33 form a very distinct cluster, most of which perform a lysogenic cycle and have a relative high GC content (Carballo-Ontiveros et al., 2020). But even within a genus, the phages were quite different, as only a nucleotide identity of 70% (coverage * identity) is required (Turner et al., 2021). Twelve out of 55 P. aeruginosa phage genera were covered by the phage panel characterized in this study. Some genera have already been described frequently and have many reference species. For example, 38 reference species are known for Pakpunavirus, 37 for Pbunavirus, 13 for Litunavirus and 20 for Septimatrevirus, while only one reference phage exists for relatively new species such as Elvirus and Pawinskivirus.
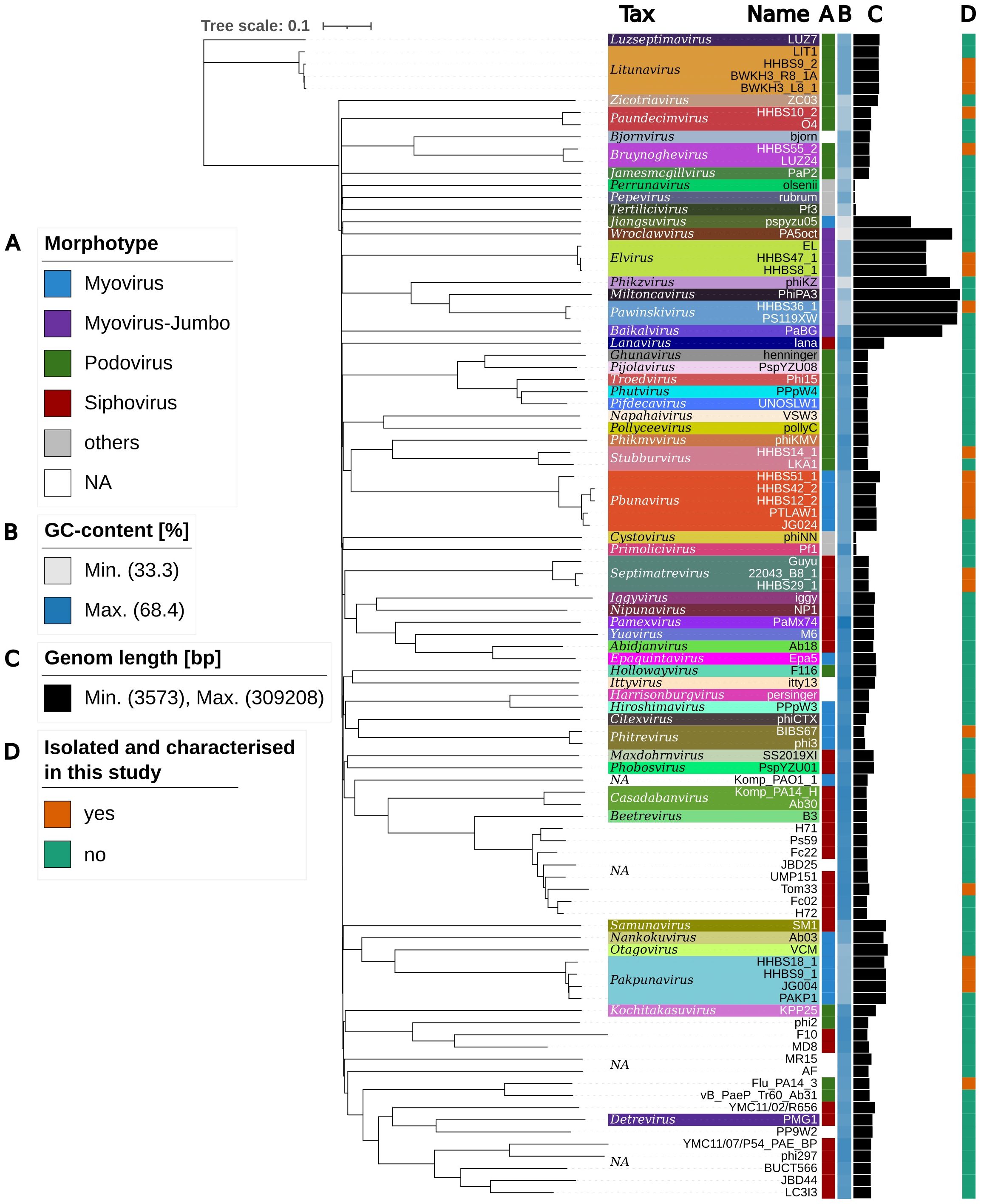
Figure 3. Phylogenomic tree of P. aeruginosa phages created with VICTOR and visualized with iTOL. At least one phage was selected from each P. aeruginosa phage genus uniquely colored (Tax). Morphotype and GC-content of phage are shown as color bars (A, B). Genome size is displayed as bar chart (C). All phages isolated and characterized in this study are labeled in orange (D).
However, despite the high sequence identity, the genome of phage species within these common genera sometimes differs considerably. The phages within Litunavirus and Pakpunavirus are more conserved than Pbunavirus, Bruynoghevirus and Septimatrevirus. In all genera, highly conserved genes (core genome) belong to proteins related to phage head and packaging, DNA, RNA and nucleotide metabolism (Supplementary Figures 3-8). When all phages are aligned with dnaapler at the same starting point (terL), the greatest variability is seen in proteins of the tail structures, such as the tail fiber protein, and at the end of the genome, where many small, hypothetical proteins are located. It is noteworthy that HHBS51_1 exhibits an insertion of 10 kb, which is not observed in other Pbunavirus. The insertion includes an endolysin, DNA primase and many small proteins of unknown function, but no genes that cause a lysogenic life cycle (Supplementary Figure 3).
3.6 Isolation and antibiotic sensitivity of 141 P. aeruginosa strains
Most P. aeruginosa isolates were isolated in the military hospitals in Berlin and Hamburg as well as at the Medical School in Hannover (MHH) in Germany from various types of infection, resulting in great diversity in terms of isolation time, geographical origin and indication (Supplementary Data Sheet 1). A detailed characterization of these strains will be subject of a different investigation. The susceptibility of P. aeruginosa isolates to different antibiotics (Supplementary Figure 9) was tested to compare susceptibility to antibiotics with susceptibility to phages. Twelve antibiotics including ceftazidime*, ceftazidime/avibactam, ceftolozane/tazobactam, cefiderocol (cephalosporins), piperacillin*, piperacillin/tazobactam (penicillins), imipenem*, meropenem* (carbapenems), azetreonam (monobactam), and ciprofloxacin* (fluoroquinolone), and some other antibiotics like amikacin and tobramycin (aminoglycosides) which are used as standard or reserved therapy for P. aeruginosa infections were chosen. All lead substances of antibiotic classes (underlined) were included (Supplementary Data Sheet 2). According to Robert-Koch-Institute in Germany, a distinction was made between 3 MRGN being resistant to three and 4 MRGN being resistant to four antibiotic classes (Kommission für Krankenhaushygiene und Infektionsprävention, 2019). Based on the antibiogram, 39% 3 MRGN (55/141 strains) and 61% 4 MRGN (86/141 strains) were identified (meropenem used as lead substance). Many wound isolates were only susceptible to cefiderocol, a last resort antibiotic. However, P. aeruginosa strains BWKH001, BWKH038 (both wound isolates) and Bron11 (respiratory tract) were even resistant to cefiderocol.
3.7 Host range of the phages tested with the 141 P. aeruginosa strains
A classical host range analysis (DPA) was performed with the diverse phage panel (Figures 2F, 4). Pbunavirus and Pakpunavirus had a broad host range (15 – 45%) and clustered close together although they are not genetically related (Figure 3). PTLAW1 (Pakpunavirus) had the highest coverage with 45% (63/141 strains). HHBS36_1 (Pawinskivirus), HHBS47_1 and HHBS8_1 (both Elviruses) had a medium coverage of 21% (mean 30/142 strains), but phage-host interactions were often categorized as reduced growth, in 22% of interactions. Among the myoviruses, BIBS67, a temperate Phitrevirus, had a narrow host spectrum with a coverage of 11% (15/141 strains). In comparison to Pakpunavirus, the Litunavirus (podoviruses) grouped together more strongly and lysed other strains (see Figures 4, 5). Twelve out of 33 wound and seven out of 24 urinary tract isolates were covered by Litunavirus with at least one phage. Strikingly, the Bruynoghevirus HHBS55_2 showed reduced growth for a particularly large number of strains 43% (60/141 strains), whereas plaques were only determined for 19% of the strains (27/141 strains). In contrast, HHBS14_1 (Stubburvirus) was very specific and covered 2% of the tested strains (3/141 strains). Similarly, Flu_PA14_3, Flu_PA14_4 and Komp_PA14_H (unclassified phage) covered 3 - 4% of the strains. The two Septimatrevirus (siphoviruses) were quite different from each other. HHBS29_1 showed lytic behavior on 8% of the strains (11/141 strains), some of which were cystic fibrosis isolates, and 22043_B8_1 lysed 12% of the strains (17/141 strains) (see Figures 4, 5). Both phages tend to have many phage-host interactions that we classified as reduced growth. No plaques were detected in about 9% of the strains (13/141 strains) from different sampled sites. 10% (14/141 strains) of P. aeruginosa strains were only covered by one phage, showing visible plaques. On the other hand, there were also 10% (14/141 strains) of P. aeruginosa strains that could be covered by ten or more of the phages tested. Interestingly, the susceptibility of the P. aeruginosa strains against phages tended to correlate positively with the susceptibility of antibiotics (Supplementary Figure 10). Lytic behavior of phages could not be shown for P. aeruginosa strains BWKH17 (wound), BWKH23 (respiratory tract), BWKH24 (skin), BWKH35 (wound), BWKH68 (urinary tract), BWKH96 (rectal swab) or BWKH115 (rectal swab) with any method. All of them are highly resistant to antibiotics.
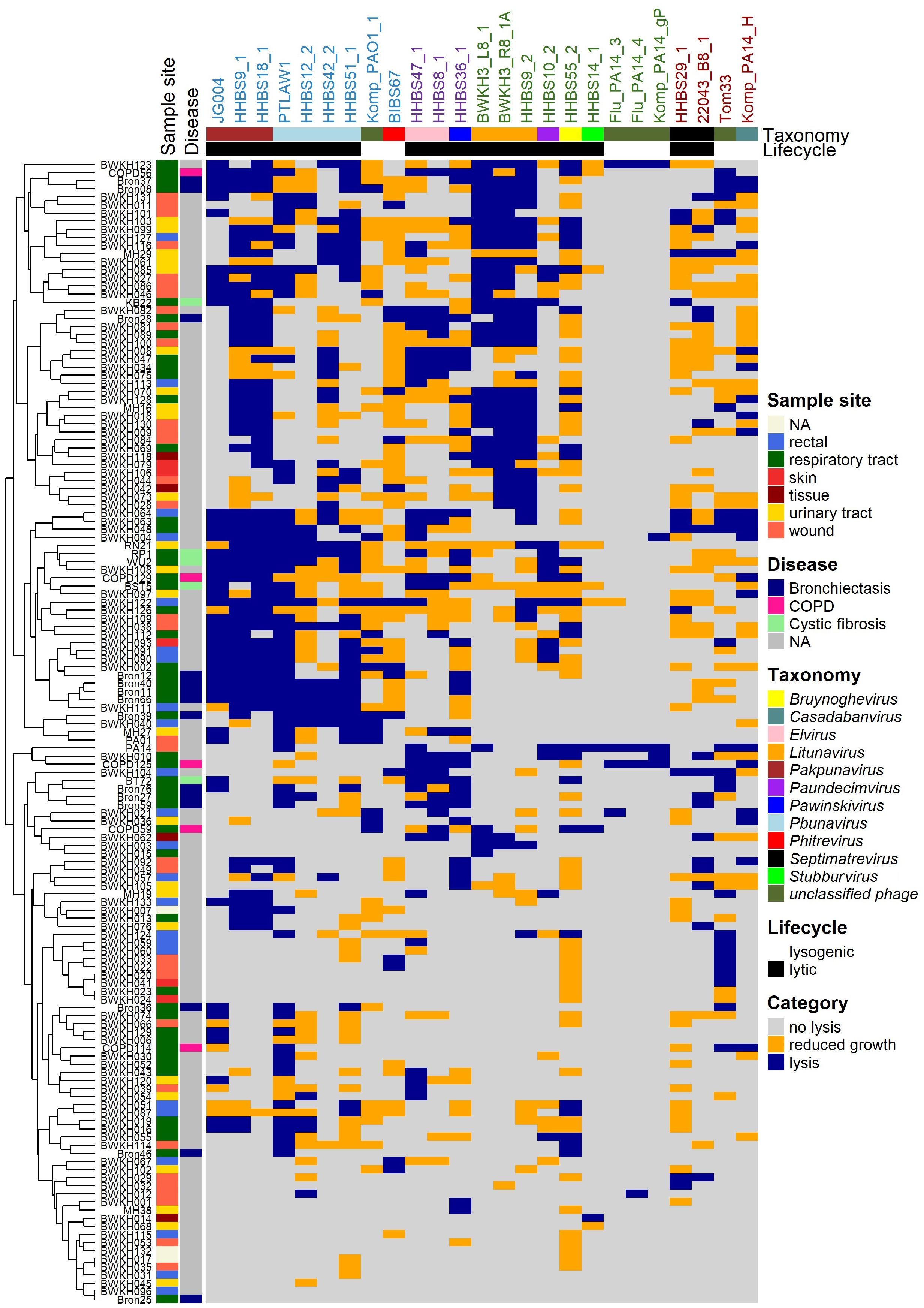
Figure 4. Host range analysis by DPA (top agar 0.5%) of 25 phages. Interaction is classified into “no lysis” (grey), “reduced growth” (orange) and “lysis” (blue). The used phages are colored based on their morphotype (colored names at the top: myo- (blue) – jumbo phages (violet), podo- (green) or siphovirus (brown)), taxonomic classification and lifestyle. Analyses was performed using 142 clinical isolates of P. aeruginosa sampled from different habitats and patients with different diseases (colored left). Clustering of rows is performed by distinct method of ComplexHeatmap package in R.
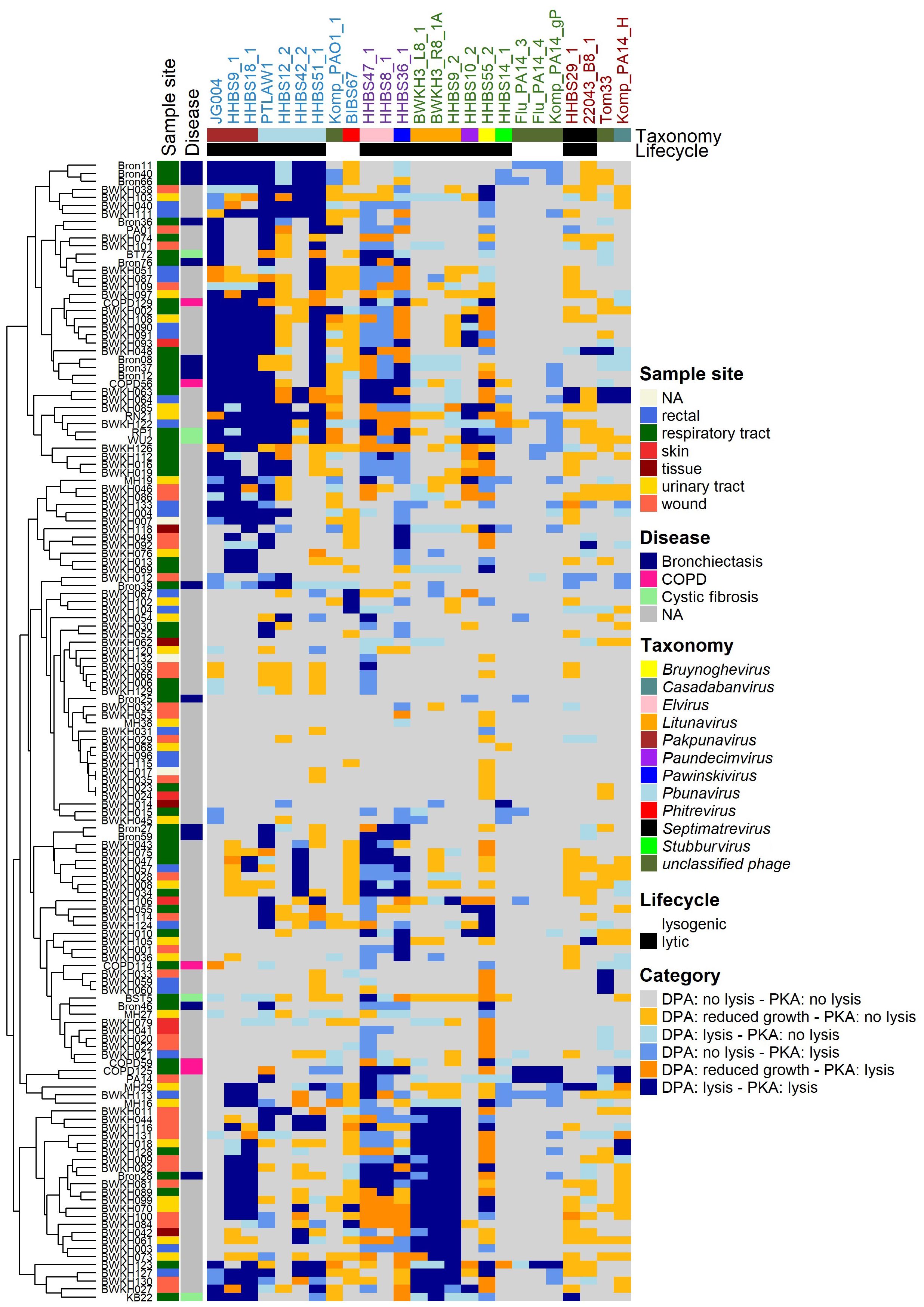
Figure 5. Comparison of host spectra determined by DPA and PKA respectively. Phages are colored based on their morphotype (colored names at the top: myo- (blue) – jumbo phages (violet), podo- (green) or siphovirus (brown)), genus and lifecycle (top) and the P. aeruginosa strains are shown with the sample site (left). DPA was performed using 0.5% top agar concentration and serial dilutions of phage lysates and classified as “no lysis”, “reduced growth” and “lysis”. PKA was performed in LB veggie medium at MOI 0.1. The growth curves of PKA are integrated for 24h and normalized against the control without phage. Normalized AUC ≥ 0.8 is classified as “no lysis” and normalized AUC < 0.8 as “lysis”. Both results are combined with the expressions DPA: lysis - PKA lysis in dark blue, DPA: reduced growth - PKA lysis in dark orange; DPA: no lysis - PKA lysis in mid blue; DPA: lysis - PKA no lysis in light orange; DPA: reduced growth - PKA no lysis in light blue and DPA: no lysis - PKA no lysis in grey. Heatmap is clustered with average methods of ComplexHeatmap (R) in rows and columns.
3.8 Comparison of the PKA and DPA methods
However, it is not yet clear which is the best in vitro method for determining the phage susceptibility that best predicts in vivo efficacy. Therefore, we determined the host range using both PKA and DPA method and systematically compared the results in order to contrast the advantages, disadvantages and limitations of both methods. The comparison of both methods revealed a correlation of 70%, of which 13% is attributable to a lytic interaction (dark green) and 57% to a non-lytic interaction (light green) (Supplementary Figure 11A). A high correlation of lytic and non-lytic interaction can be observed particularly in the genera Pbunavirus, Pakpunavirus and Litunavirus (Supplementary Figure 11B). At the same time, the PKA method tended to identify more lytic phage-host interactions for these genera than DPA. In 17% of the cases there were ambiguous results, which can be further distinguished. There are 5% of the cases in the category “DPA: reduced growth - PKA: lysis” (dark orange), in which the host range of phages was underestimated using the DPA method. Many of these cases were related to jumbo phages. This is due to the fact that members of Elvirus, Pawinskivirus and Bruynoghevirus in particular formed invisible, too tiny or not clearly defined individual plaques with 0.5% top agar. When the lysis behavior is tested with PKA, these phages sometimes achieve even better coverage (43 to 67%) than the other myoviruses (Figure 2G). On the other hand, 13% of the cases were classified as “DPA: reduced growth – PKA: no lysis” (light orange). In particular, phages with the morphotype siphovirus accounted for more than 21% in this category, which means that they were probably overestimated by the DPA. If the plaques were cloudy in DPA, often no lysis was observed in the PKA (Figure 5). No correlation was found in 6% of the cases with expression “DPA: no lysis - PKA: lysis” and 6% of the cases with the expression “DPA: lysis - PKA: no lysis”. The second case was particularly prevalent in Litunavirus and phages with the morphotype siphovirus (Figure 5). Since both methods lead to different results and this can have an influence on the selection of phages, both methods must be taken into account for the PST.
This study also investigated whether there are infection-specific phages by analyzing the host spectrum with regard to the isolation type of the hosts. Figure 6 represents the percentage coverage of the lysed strains depending on the type of infection of strains shown for DPA and PKA. A specificity of certain phages toward P. aeruginosa from specific habitats or diseases could not be determined in this study. The only finding was that Litunavirus lysed fewer strains from the respiratory tract than myoviruses.
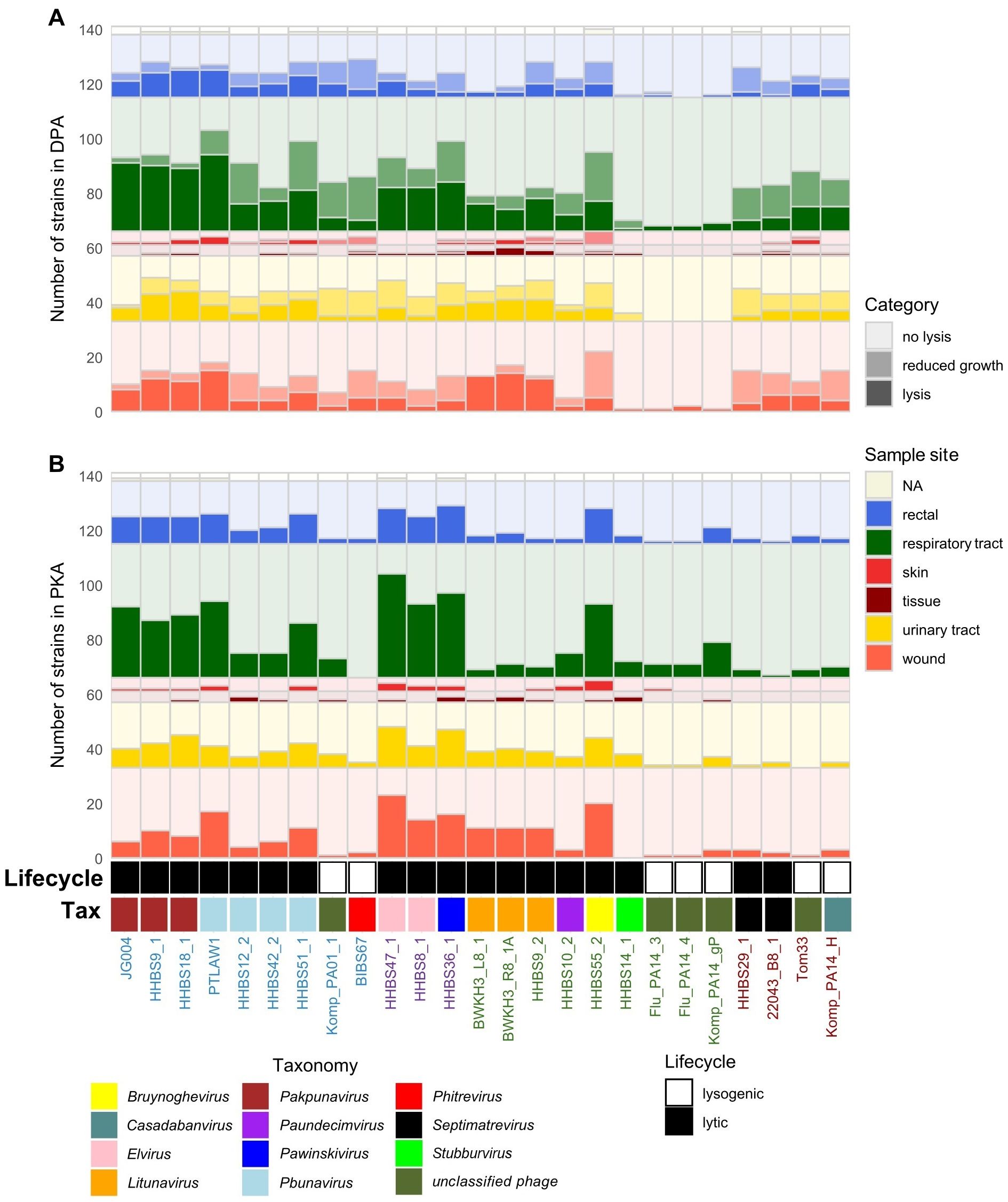
Figure 6. Percentage of lysed clinical P. aeruginosa strains depending on the phage and the PST. The phage-host interaction is shown with increasing intensity: DPA assay in three categories (no lysis, reduced growth, lysis) (A) and PKA assay in two categories (lysis: normalized AUC < 0.8 and no lysis: normalized AUC ≥ 0.8) (B). Both plots are grouped based on morphotype (colored names at the bottom: myo- (blue) – jumbo phages (violet), podo- (green) or siphovirus (brown)), genus and lifecycle of the phage. The habitats from which P. aeruginosa was sampled are light grey = not available, blue = rectal, dark green = respiratory tract, red = skin, brown = tissue, yellow = urinary tract and orange = wound.
3.9 Final ranking of phages suitable for a phage therapy
Two aspects need to be distinguished for a ranking of the phage concerning their application in a phage therapy. Which phages are suitable for (individual) therapy and which phages have favorable properties for production. Both aspects should be considered for the selection to ensure an efficient and safe treatment as well as successful production. Here we provide a ranking (Figure 7) with multiple factors like safety, host range determined by DPA and PKA and virulence index (vp) included.
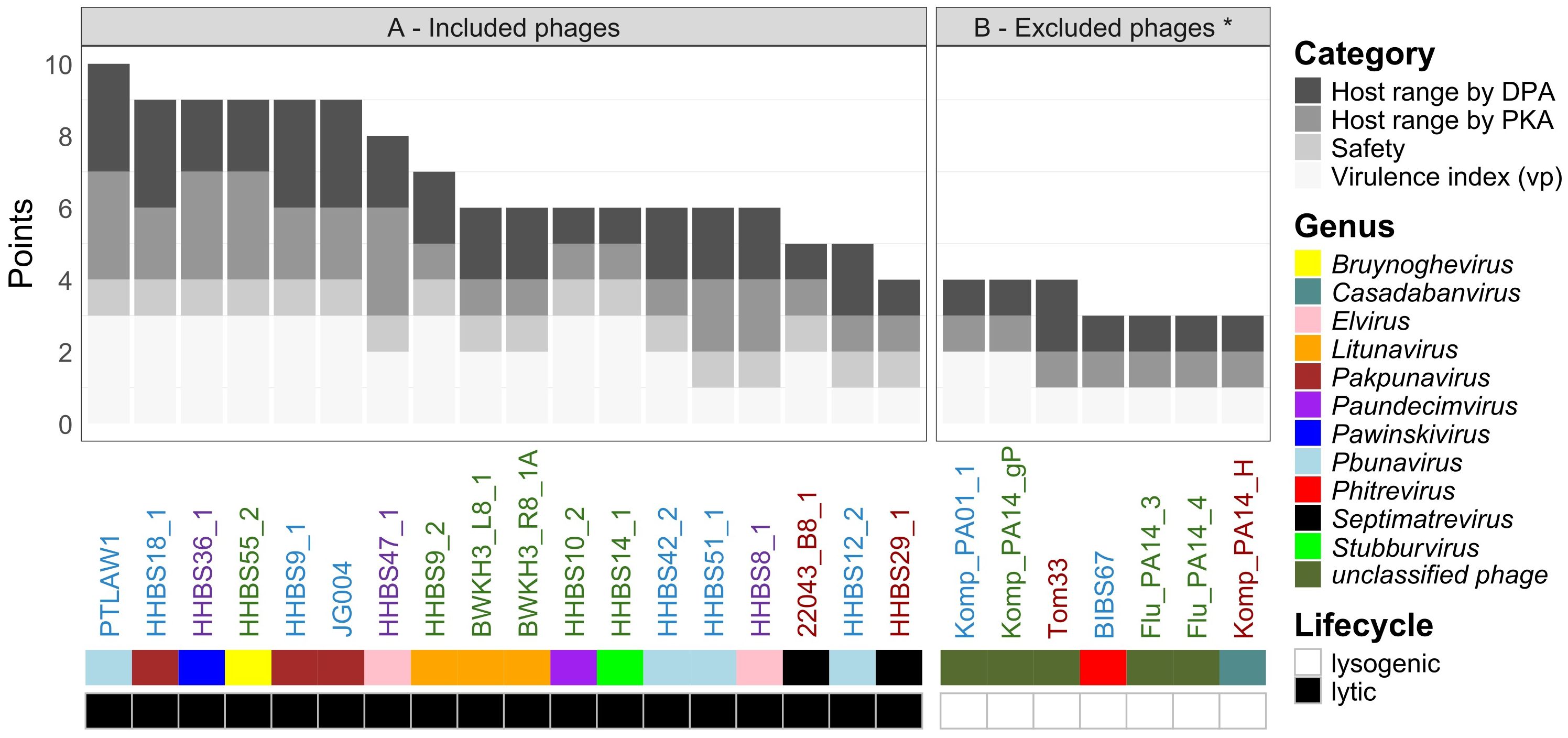
Figure 7. The ranking of phages regarding therapeutic potential and production is visualized by several categories in descending order and subdivided according to safety aspects. Both plots are shown with the morphotype (colored names: myo- (blue) – jumbo phages (violet), podo- (green) or siphovirus (brown)), genus and lifecycle of the phage. (A) shows all phages without safety concerns and (B) shows all phages with safety concerns due to a lysogenic life cycle *. In both diagrams, the distribution of points is determined as described in Supplementary Data Sheet 4. In detail, the points for DPA are as follows: 3 points for ≥ 42 lysed strains, 2 points for 21–42 lysed strains and 1 point for < 21 lysed strains. Classification of host range determined by PKA is: 3 points for ≥ 63 lysed strains, 2 points for 31–63 lysed strains and 1 point for < 31 lysed strains. Strictly lytic phages without toxins, antibiotic resistance genes or genes that induce a lysogenic cycle receive 1 point, otherwise 0 point*. Virulence index (vp) is classified like 3 points: vp ≥ 0.6, 2 points: vp 0.3-0.6 and 1 point: vp < 0.3. In addition, the phages are colored based on their genera, morphotype and lifecycle.
We suggest that phages with a broad (Pakpunavirus) or narrow (Stubburvirus) host spectrum should be assessed equally for therapy if they are effective on a patient isolate. Even more important are the safety aspects. In order to exclude risk factors for the patients, the genomes of the phages were examined. 18/25 phages were strictly lytic, do not encode any virulence factors or antibiotic resistance genes (analyzed with Virulence factor database (VFDB) (Liu et al., 2022) and AMRfinderPlus (Feldgarden et al., 2021) (Altschul et al., 1997; Feldgarden et al., 2021) and could be used for therapy purposes (Figure 7). All lysogenic phages (unclassified phages, Casadabanvirus and Phitrevirus) with integrase, transposase, excisionase or other DNA transposition proteins were excluded (Figure 7B) for therapeutic use.
Looking at our ranking, PTLAW1 (Pbunavirus) ranked the highest with ten final points. Other phages like HHBS18_1, JG004 and HHBS9_1 (Pakpunavirus with morphotype myovirus) received 9 points. They lysed their production host efficiently and fast (Figure 2H). HHBS55_2 (Bruynoghevirus) and HHBS36_1 (Pawinskivirus) were also promising candidates (9 points) because of their great host range in PKA. HHBS47_1 should be preferred (8 points) over HHBS8_1 (both Elvirus) (6 points) because of a higher virulence index. Various phages with the podovirus morphotype ranked in the midfield, including the promising phage HHBS9_2 (Litunavirus) (7 points). On average, siphoviruses ended up at the bottom of the ranking. The biggest influence was their low virulence index (vp). This made HHBS29_1 (Septimatrevirus) difficult to produce in liquid (Figure 7, Supplementary Data Sheet 3, Supplementary Figure 2A)).
3.10 Theoretical combination of phages for phage therapy
The effectiveness of phage therapy depends not only on the selection of individual phages but also on a combination of phages. Thus, the theoretical coverage of multiple phage applications was analyzed in silico using the Phage Cocktail Optimizer (Abedon, 2020) to identify the phage combination that maximizes coverage. Only safe phages that could be used for treatment were included in this calculation. The maximum coverage of 92% of all strains with at least one phage per bacterium was achieved with six phages, based on the PKA results. For the DPA results, the maximum coverage is 86% with a total of twelve phages combined (Table 1). The greatest theoretical coverage was always achieved when phages of different genera were combined. If three phages were theoretically combined in a cocktail, this would result in a coverage of over 74% (DPA) or 86% (PKA) (Table 1). Many possible combinations contain PTLAW1 (Pbunavirus) and HHBS47_1 (Elvirus) or HHBS55_2 (Bruynoghevirus).
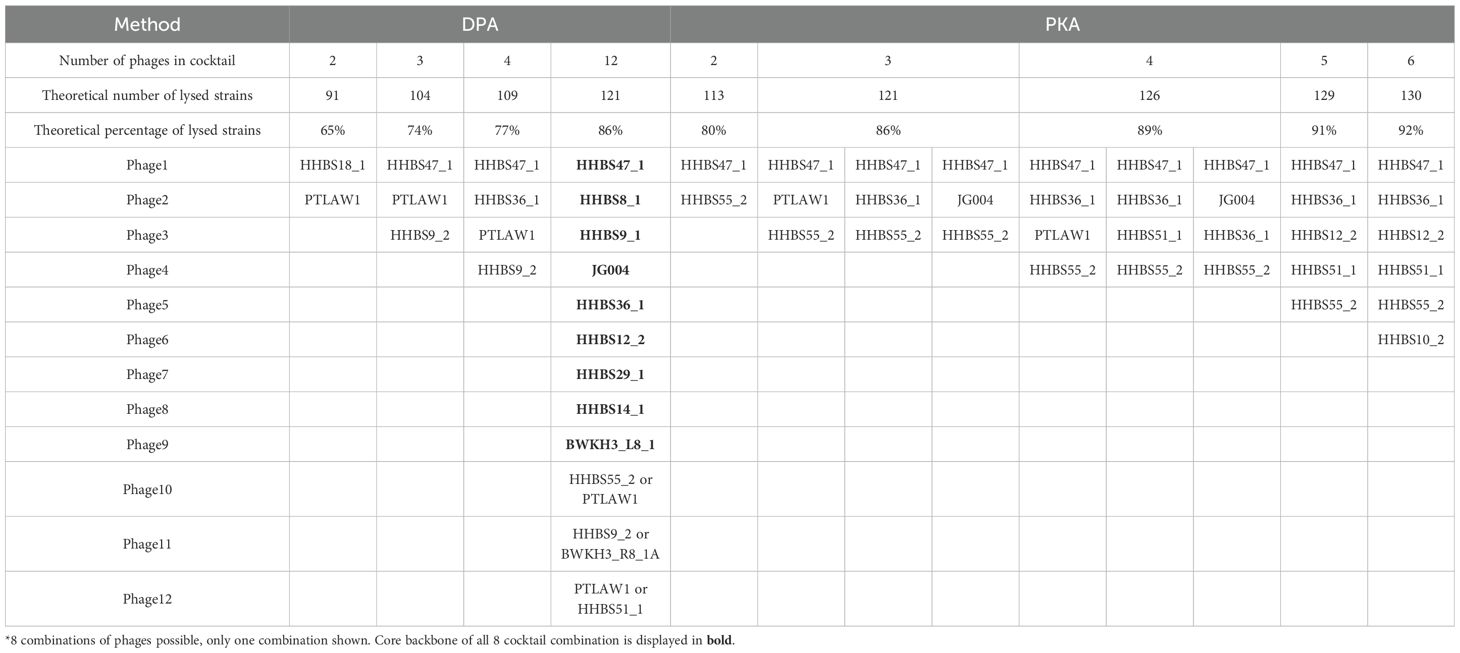
Table 1. Coverage of a theoretical phage combination determined with Phage Cocktail Optimizer (Abedon, 2020) depending on the method and the depth of the cocktail (only safe phages used).
4 Discussion
Our data highlights the diversity of P. aeruginosa phages. We were able to isolate 25 phages, 18 of which pursue a strictly lytic lifecycle (all isolated from wastewater samples), while only temperate phages could be extracted from the environmental samples (garden compost, tomato, washing machine). New phages should therefore be sequenced at an early stage of the work to determine the life cycle and avoid labor-intensive experiments. All lytic phages could be assigned to previously published genera, but many phages represent a potential new species (Supplementary Data Sheet 3). Some temperate phages, on the other hand, were assigned to following new genera: first new genus include Flu_PA14_3, Flu_PA14_4, Komp_PA14_gP, second new genus Komp_PAO1_1 and third genus Tom33.
Our study revealed a high diversity in particular among in the morphotype myoviruses of our P. aeruginosa phage panel. Although they all have a hexagonal head and tail, they differ considerably at the genome level. Therefore, it is more contemporary to compare phages within their genus and not their morphotypes (Turner et al., 2021). There are many variations within a phage genus, including insertions, deletions, and mutations within genes. Mutations within the tail proteins are primarily responsible for adsorption to the host (Gaborieau et al., 2024) and the resulting variability of host specificity.
Virus- and plaque morphologies are often used as a basic characterization as they are easy to compare and allow phage identification. The formation of large, clear plaques should be favored over cloudy plaques, as this often represents temperate phages. Small plaques are problematic as well, as they can be difficult to detect. However, phages should only be excluded after sequencing, as morphological characteristics only provide an initial indication of which phages have therapeutic potential. By determining the virulence index (vp), phages can be qualitatively compared for the first time (Storms et al., 2020). For this purpose, MOI-dependent lysis curves were recorded, the AUC was normalized against an uninfected control, and these local virulence indices (vi) are integrated a second time against the log MOI to obtain the virulence index (vp). A comparison of vp of different phages presents some difficulties, as almost all parameters must be identical for vp to be successfully calculated. Normally, only one parameter like growth medium or temperature may be changed in order to achieve optimal comparability. Nevertheless in our data, two parameters (phage and host) were adapted. Our aim was to determine which phage with its host is best suited for production. It has been shown that the production of Pakpunavirus, even at low MOIs, results in a significantly greater bacterial reduction than Litunavirus (Supplementary Data Sheet 3). Some of the phages (HHBS8_1 and HHBS47_1 (Elvirus)) lyse only after the onset of the steady-state phase, so that the vp is very small due to the official calculation that includes the time of the steady-state phase of the control. Therefore, for the determination of susceptibility in PKA, we calculated the AUC over 24 h rather than up to the steady-state phase to compensate for the different growth behavior of the strains. We consider that Peters et al, 2023 had similar difficulties in calculating the vp according to the original definition by Storms et al, 2020 and therefore set the cut-off to 8 hours and thus included early regrowth of the bacteria in the virulence index. Normally, phages with a high vp value should be favored. Nevertheless, we would like to raise the question of whether late-lysing phages with different lysis kinetics are even advantageous in combination with other early-lysing phages.
The phages can be differentiated according to their host spectrum. Using our broad phage and host panel, we compared 3525 phage-host interactions with DPA and PKA. We found that the comparability of the two methods strongly depends on the phage genus analyzed (Supplementary Figure 11). In particular, members of Pbunavirus, Pakpunavirus and Litunavirus can be compared very well (comparability of the methods on average > 76%), as they form very large plaques. The method DPA has some limitations if the plaque morphology is not unambiguous in the case of small or cloudy plaques. For example, the formation of small plaques of jumbo phages, which lead to the lytic interaction being undetected, could be avoided by decreasing the top agar concentration (Serwer et al., 2007; Yuan and Gao, 2017). However, other problems can then arise, such as the overgrowth of plaques with very mucous clinical isolates, and the plaques sometimes only grow very slowly (HHBS8_1 at 0.3% in Supplementary Figure 1) (Staudinger et al., 2014). Low adsorption rates favor the formation of small plaques not only in jumbo phages (Abedon and Culler, 2007). In contrast to the assumption that phages that do not lyse a liquid culture fail to form plaques as published by Haines et al., 2021, our results demonstrate that this behavior is exhibited by siphoviruses in particular. In addition, we were able to show that the plaque morphology of phages with regard to turbidity is dependent on the strain, especially in case of temperate phages. However, the boundaries between clear and cloudy plaques are difficult to distinguish by eye. HHBS55_2 (Bruynoghevirus), BWKH3_L8_1 and BWKH3_R8_1A (both Litunavirus), which are lytic phages, form cloudy plaques in their original strains (Figure 2C) and in some cases also on other strains (Figure 4, data not shown).
Considering all these arguments we propose to use PKA as the standard method for PST. It offers several advantages: an already successfully implemented automation, easy performance in replicates and with different MOIs, and quantitative measurements in a high throughput screening format. For most phage-host interactions, PKA is faster, it can detect possible lytic behavior after only a few hours. In addition, several phages can be tested simultaneously or in a combination of phages with antibiotics. However, it should be noted that the lysis behavior can also be caused by toxins, endolysins, tailocins etc. when using crude lysates and therefore purified lysates should be preferred and the results should be validated with those of the DPA (Kim et al., 2020). In the future it may also be possible to predict phage-host interaction in silico, as this has already been done for selected bacteria such as Staphylococcus aureus (Moller et al., 2021), Escherichia coli (Gaborieau et al., 2024) and Klebsiella pneumoniae (Boeckaerts et al., 2024). The prediction tools that are currently available, such as HostPhinder, DeepHost, do not yet differentiate between phage-host interaction in P. aeruginosa at the strain level (Villarroel et al., 2016; Ruohan et al., 2022). But regardless of whether DPA and PKA are used as PST, the more important comparison is with the in vivo data, so that we can understand which assay better mimics the situation in the patient. So far there is still little data available. In many studies, it would be helpful for the comparison of P. aeruginosa phages if both the phage name and the corresponding genus is mentioned.
There is a tendency for phage susceptibility in the classical host spectrum to correlate negatively with antibiotic resistance (Supplementary Figure 10). Despite repeated efforts, we were unable to find a matching phage for every clinical P. aeruginosa isolate with twelve of 37 known P. aeruginosa phage genera. Especially the wound isolates were less covered with the phage panel. To verify possible explanations like anti-phage defense mechanism of the bacteria, interacting prophages or missing receptors, the strains need to be sequenced (Makarova et al., 2020; Markwitz et al, 2022; Georjon and Bernheim, 2023). Since there are limitations regarding the selected phages and antibiotics, further experiments, including in vitro studies, need to be performed to verify the correlation.
Our ranking suggests that Pakpunavirus, Pbunavirus, Pawinskivirus and Elvirus (myoviruses) as well as Litunavirus and Bruynoghevirus (podoviruses) have great potential for an application in phage therapy because they lyse many strains and are easy to produce (Figure 7). In previous studies, these phage genera have often been used in vitro and in vivo in animals and humans against P. aeruginosa infections (Silva et al., 2022). In particular, various Pbunavirus such as Pa193, Pa204, Pb10, which are > 95% identical (coverage x identity) to PTLAW1, have already demonstrated their high potential for phage therapy against P. aeruginosa as single phages (Supplementary Figure 3) (Forti et al., 2018; Aslam et al., 2019; Alkalay-Oren et al., 2022; Shafigh Kheljan et al., 2023). These phages have also been used in phage cocktails. A metagenomic analysis in 2017 identified eight different phage genera in Pyo Bacteriophage™ (Georgia) against P. aeruginosa, which have many similarities with our phages (Pakpunavirus, Pbunavirus, Phikzvirus, Nankokuvirus, Phikmvvirus (myoviruses) and Bruynoghevirus, Litunavirus, unclassified phage (podoviruses)) (McCallin et al., 2018). For other phage cocktails, such as PP1131 (PhagoBurn) or BX004-A™ (BiomX, Israel), compositions have not yet been reported (Jault et al., 2019; Rappo et al., 2023). However, the use of different genera increases the likelihood that many strains/isolates can be treated (Figure 5). Not only do the host ranges of the individual phages often add up, there is also the chance of phage-phage synergy (PPS) (Schmerer et al., 2014). Whether synergistic or antagonistic effects exist for the best predicted cocktails with the specific phages would require further in vitro or biofilm analysis. In order to increase the overall coverage, phages with a narrow host range should also be included. Only a few siphoviruses have been used for therapy so far (Silva et al., 2022). We assume that this is due to their narrow host range (Figures 2F, G), and also due to the fact that the probability of finding lytic phages with the morphotype siphovirus is much lower, as the majority of P. aeruginosa prophages belong to the morphotype siphovirus (Johnson et al., 2022). However, this does not mean that no lytic siphoviruses are found (see 22043_B8_1 and HHBS29_1 (Septimatrevirus)).
In addition to safety and the suitable host spectrum, there are other factors that should be included in a ranking. Depending on the indication, efficacy against biofilms could also play a role in the selection. Among others the stability of the phages, which is often tested at different pH values and temperatures. Stability of the phages should be ensured regarding the various therapeutic indications (bladder, lungs, etc.). In addition, phages must be sequenced to exclude possible induced prophages from the host, as P. aeruginosa usually has more than one prophage (Johnson et al., 2022). Furthermore, combinations with other phages or antibiotics should be considered for therapy (Nikolic et al., 2022). Phage-antibiotic-antagonism (Gu Liu et al., 2020; Danis-Wlodarczyk et al., 2021a) and phage-phage antagonism should be avoided (Schmerer et al., 2014). At the same time, it should be tested whether phages of the same genus should be used together, as they probably have similar receptors (Pleteneva et al., 2008). In the best case the phages would be selected and combined in a way that it would make it difficult for the bacteria to adapt via the receptor or defense systems.
5 Conclusion
25 P. aeruginosa phages were characterized and compared showning a great diversity within all morphotypes. A combination of six phages could theoretically lyse 92% of clinical P. aeruginosa strains. Further, we showed that especially Pakpunavirus, Pbunavirus, Pawinskivirus, Elvirus (all myoviruses), Litunavirus and Bruynoghevirus (all podoviruses) showed the greatest potential in the proposed ranking and should be employed with priority, as they have already been used for phage therapy. Siphoviruses were less suitable. Phages with a lysogenic cycle also have less potential due to their narrow host range and efficiency (low vp value), as well as safety concerns regarding phage therapy.
We also compared the classic DPA (0.5% top agar) with the liquid PKA (MOI 0.1). Both methods are easy to perform but offer different limitations depending on the phage. Host analysis using DPA did not detect phages that form very small plaques, while PKA could not clearly state whether lysis by some siphoviruses and temperate phages was caused by phage activity. Overall, we suggest that PKA is the better method for phage susceptibly prediction because it is usually faster and less prone to individual interpretation. However, both methods should also be compared with in vivo data from patients in the future.
Data availability statement
The datasets presented in this study can be found in online repositories. The names of the repository/repositories and accession number(s) can be found in the article/Supplementary Material.
Author contributions
FR: Conceptualization, Data curation, Formal Analysis, Investigation, Validation, Visualization, Writing – original draft, Writing – review & editing. JW: Data curation, Investigation, Writing – review & editing. BB: Investigation, Writing – review & editing. CS: Investigation, Writing – review & editing. MH: Resources, Writing – review & editing. CW: Resources, Writing – review & editing. MM: Investigation, Writing – review & editing. HZ: Funding acquisition, Supervision, Writing – review & editing. IK: Conceptualization, Data curation, Funding acquisition, Investigation, Project administration, Supervision, Validation, Writing – original draft, Writing – review & editing. DJ: Conceptualization, Funding acquisition, Supervision, Writing – original draft, Writing – review & editing.
Funding
The author(s) declare that financial support was received for the research and/or publication of this article. This work received funding from “Gemeinsamer Bundesausschuss, Innovationsausschuss, Germany” under the grant “PTmHBP”, 01VSF18049 (to IK between 2019 and 2021, to MH between 2019 and 2024).
Acknowledgments
Authors thank Laura Wicke for isolating the phage PTLAW1, Ella Siegel for helping with the PKA - host spectrum and Stephanie Peter and Simone Schrader for generating phage libraries. Special thanks also go to Ina Brentop for EM sample preparation.
Conflict of interest
Author XY was employed by Leibniz Institute DSMZ, which is an independent, non-profit research infrastructure.
All authors declare that the research was conducted in the absence of any commercial or financial relationships that could be construed as a potential conflict of interest.
Generative AI statement
The author(s) declare that Generative AI was used in the creation of this manuscript. Deepl and ChatGPT were used to revise the text.
Publisher’s note
All claims expressed in this article are solely those of the authors and do not necessarily represent those of their affiliated organizations, or those of the publisher, the editors and the reviewers. Any product that may be evaluated in this article, or claim that may be made by its manufacturer, is not guaranteed or endorsed by the publisher.
Supplementary material
The Supplementary Material for this article can be found online at: https://www.frontiersin.org/articles/10.3389/fcimb.2025.1597009/full#supplementary-material
References
Abedon, S. T. (2020). Phage Cocktail Optimizer. https://www.phage-therapy.org/calculators/cocktail_optimizer.html (Accessed July 5, 2024).
Abedon, S. T., Culler, R. R. (2007). Bacteriophage evolution given spatial constraint. J. Theor. Biol. 248, 111–119. doi: 10.1016/j.jtbi.2007.02.014
Ackermann, H. W., Eisenstark, A. (1974). The present state of phage taxonomy. Intervirology 3, 201–219. doi: 10.1159/000149758
Aghaee, B. L., Mirzaei, M. K., Alikhani, M. Y., Mojtahedi, A. (2021). Sewage and sewage-contaminated environments are the most prominent sources to isolate phages against Pseudomonas aeruginosa. BMC Microbiol. 21, 132. doi: 10.1186/s12866-021-02197-z
Alipour-Khezri, E., Skurnik, M., Zarrini, G. (2024). Pseudomonas aeruginosa bacteriophages and their clinical applications. Viruses 16, 1051. doi: 10.3390/v16071051
Alkalay-Oren, S., Yerushalmy, O., Adler, K., Khalifa, L., Gelman, D., Coppenhagen-Glazer, S., et al. (2022). Complete genome sequence of Pseudomonas aeruginosa bacteriophage PASA16, used in multiple phage therapy treatments globally. Microbiol. Resour Announc 11, e0009222. doi: 10.1128/mra.00092-22
Aloush, V., Navon-Venezia, S., Seigman-Igra, Y., Cabili, S., Carmeli, Y. (2006). Multidrug-resistant Pseudomonas aeruginosa: risk factors and clinical impact. Antimicrob. Agents Chemother. 50, 43–48. doi: 10.1128/aac.50.1.43-48.2006
Altschul, S. F., Madden, T. L., Schäffer, A. A., Zhang, J., Zhang, Z., Miller, W., et al. (1997). Gapped BLAST and PSI-BLAST: a new generation of protein database search programs. Nucleic Acids Res. 25, 3389–3402. doi: 10.1093/nar/25.17.3389
Amgarten, D., Martins, L. F., Lombardi, K. C., Antunes, L. P., de Souza, A. P. S., Nicastro, G. G., et al. (2017). Three novel Pseudomonas phages isolated from composting provide insights into the evolution and diversity of tailed phages. BMC Genomics 18, 346. doi: 10.1186/s12864-017-3729-z
Arumugam, S. N., Manohar, P., Sukumaran, S., Sadagopan, S., Loh, B., Leptihn, S., et al. (2022). Antibacterial efficacy of lytic phages against multidrug-resistant Pseudomonas aeruginosa infections in bacteraemia mice models. BMC Microbiol. 22, 187. doi: 10.1186/s12866-022-02603-0
Aslam, S., Courtwright, A. M., Koval, C., Lehman, S. M., Morales, S., Furr, C.-L. L., et al. (2019). Early clinical experience of bacteriophage therapy in 3 lung transplant recipients. Am. J. Transplant. 19, 2631–2639. doi: 10.1111/ajt.15503
Azam, M. W., Khan, A. U. (2019). Updates on the pathogenicity status of Pseudomonas aeruginosa. Drug Discovery Today 24, 350–359. doi: 10.1016/j.drudis.2018.07.003
Bassetti, M., Vena, A., Croxatto, A., Righi, E., Guery, B. (2018). How to manage Pseudomonas aeruginosa infections. Drugs Context 7, 212527. doi: 10.7573/dic.212527
Boeckaerts, D., Stock, M., Ferriol-González, C., Oteo-Iglesias, J., Sanjuán, R., Domingo-Calap, P., et al. (2024). Prediction of Klebsiella phage-host specificity at the strain level. Nat. Commun. 15, 4355. doi: 10.1038/s41467-024-48675-6
Carballo-Ontiveros, M. A., Cazares, A., Vinuesa, P., Kameyama, L., Guarneros, G. (2020). The Concerted Action of Two B3-Like Prophage Genes Excludes Superinfecting Bacteriophages by Blocking DNA Entry into Pseudomonas aeruginosa. J. Virol. 94, e00953-20. doi: 10.1128/jvi.00953-20
Chanishvili, N. (2012). Phage therapy–history from Twort and d’Herelle through Soviet experience to current approaches. Adv. Virus Res. 83, 3–40. doi: 10.1016/B978-0-12-394438-2.00001-3
Chanishvili, N., Myelnikov, D., Blauvelt, T. K. (2022). Professor Giorgi eliava and the eliava institute of bacteriophage. PHAGE 3, 71–80. doi: 10.1089/phage.2022.0016
Cheng, K. H., Leung, S. L., Hoekman, H. W., Beekhuis, W. H., Mulder, P. G., Geerards, A. J., et al. (1999). Incidence of contact-lens-associated microbial keratitis and its related morbidity. Lancet 354, 181–185. doi: 10.1016/S0140-6736(98)09385-4
Danis-Wlodarczyk, K., Cai, A., Chen, A., Gittrich, M. R., Sullivan, M. B., Wozniak, D. J., et al. (2021a). Friends or foes? Rapid determination of dissimilar colistin and ciprofloxacin antagonism of Pseudomonas aeruginosa phages. Pharmaceuticals 14, 1162. doi: 10.3390/ph14111162
Danis-Wlodarczyk, K., Dąbrowska, K., Abedon, S. T. (2021b). Phage therapy: the pharmacology of antibacterial viruses. Curr. Issues Mol. Biol. 40, 81–164. doi: 10.21775/cimb.040.081
Debarbieux, L., Leduc, D., Maura, D., Morello, E., Criscuolo, A., Grossi, O., et al. (2010). Bacteriophages can treat and prevent Pseudomonas aeruginosa lung infections. J. Infect. Dis. 201, 1096–1104. doi: 10.1086/651135
European Committee on Antimicrobial Susceptibility Testing (2022). Breakpoint tables for interpretation of MICs and zone diameters: Version 12.0. Available online at: http://www.eucast.org (Accessed July 10, 2023).
Feldgarden, M., Brover, V., Gonzalez-Escalona, N., Frye, J. G., Haendiges, J., Haft, D. H., et al. (2021). AMRFinderPlus and the Reference Gene Catalog facilitate examination of the genomic links among antimicrobial resistance, stress response, and virulence. Sci. Rep. 11, 12728. doi: 10.1038/s41598-021-91456-0
Ferry, T., Kolenda, C., Batailler, C., Gaillard, R., Gustave, C.-A., Lustig, S., et al. (2021). Case report: arthroscopic “Debridement antibiotics and implant retention” With local injection of personalized phage therapy to salvage a relapsing Pseudomonas aeruginosa prosthetic knee infection. Front. Med. 8. doi: 10.3389/fmed.2021.569159
Forti, F., Roach, D. R., Cafora, M., Pasini, M. E., Horner, D. S., Fiscarelli, E. V., et al. (2018). Design of a broad-range bacteriophage cocktail that reduces Pseudomonas aeruginosa biofilms and treats acute infections in two animal models. Antimicrob. Agents Chemother. 62, e02573-17. doi: 10.1128/aac.02573-17
Gaborieau, B., Vaysset, H., Tesson, F., Charachon, I., Dib, N., Bernier, J., et al. (2024). Prediction of strain level phage-host interactions across the Escherichia genus using only genomic information. Nat. Microbiol. 9, 2847–2861. doi: 10.1038/s41564-024-01832-5
Garbe, J., Bunk, B., Rohde, M., Schobert, M. (2011). Sequencing and characterization of Pseudomonas aeruginosa phage JG004. BMC Microbiol. 11, 102. doi: 10.1186/1471-2180-11-102
Georjon, H., Bernheim, A. (2023). The highly diverse antiphage defence systems of bacteria. Nat. Rev. Microbiol. 21, 686–700. doi: 10.1038/s41579-023-00934-x
Gu Liu, C., Green, S. I., Min, L., Clark, J. R., Salazar, K. C., Terwilliger, A. L., et al. (2020). Phage-antibiotic synergy is driven by a unique combination of antibacterial mechanism of action and stoichiometry. mBio 11, e01462-20. doi: 10.1128/mBio.01462-20
Haines, M. E. K., Hodges, F. E., Nale, J. Y., Mahony, J., van Sinderen, D., Kaczorowska, J., et al. (2021). Analysis of selection methods to develop novel phage therapy cocktails against antimicrobial resistant clinical isolates of bacteria. Front. Microbiol. 12. doi: 10.3389/fmicb.2021.613529
Hamed, M. M., Abdelsamie, A. S., Rox, K., Schütz, C., Kany, A. M., Röhrig, T., et al. (2023). Towards translation of PqsR inverse agonists: from in vitro efficacy optimization to in vivo proof-of-principle. Advanced Sci. 10, e2204443. doi: 10.1002/advs.202204443
Hashemi Shahraki, A., Vahed, M., Masood, S., Mirsaeidi, M. (2023). Complete genome sequence of Pseudomonas aeruginosa phage UF_RH6, isolated from human lung. Microbiol. Resour Announc 12, e0020623. doi: 10.1128/mra.00206-23
Hatcher, E. L., Zhdanov, S. A., Bao, Y., Blinkova, O., Nawrocki, E. P., Ostapchuck, Y., et al. (2017). Virus Variation Resource - improved response to emergent viral outbreaks. Nucleic Acids Res. 45, D482–D490. doi: 10.1093/nar/gkw1065
Hilliam, Y., Kaye, S., Winstanley, C. (2020). Pseudomonas aeruginosa and microbial keratitis. J. Med. Microbiol. 69, 3–13. doi: 10.1099/jmm.0.001110
Horcajada, J. P., Montero, M., Oliver, A., Sorlí, L., Luque, S., Gómez-Zorrilla, S., et al. (2019). Epidemiology and treatment of multidrug-resistant and extensively drug-resistant Pseudomonas aeruginosa infections. Clin. Microbiol. Rev. 32, e00031-19. doi: 10.1128/CMR.00031-19
International Committee of Taxonomy of Viruses (2023). International Committee of Taxonomy of Viruses: Current Taxonomy Release. Available online at: https://ictv.global/taxonomy (Accessed June 22, 2023).
Jault, P., Leclerc, T., Jennes, S., Pirnay, J. P., Que, Y.-A., Resch, G., et al. (2019). Efficacy and tolerability of a cocktail of bacteriophages to treat burn wounds infected by Pseudomonas aeruginosa (PhagoBurn): a randomised, controlled, double-blind phase 1/2 trial. Lancet Infect. Dis. 19, 35–45. doi: 10.1016/S1473-3099(18)30482-1
Johnson, G., Banerjee, S., Putonti, C. (2022). Diversity of Pseudomonas aeruginosa temperate phages. mSphere 7, e0101521. doi: 10.1128/msphere.01015-21
Kim, S., Lee, D.-W., Jin, J.-S., Kim, J. (2020). Antimicrobial activity of LysSS, a novel phage endolysin, against Acinetobacter baumannii and Pseudomonas aeruginosa. J. Glob Antimicrob. Resist. 22, 32–39. doi: 10.1016/j.jgar.2020.01.005
Korf, I. H. E., Meier-Kolthoff, J. P., Adriaenssens, E. M., Kropinski, A. M., Nimtz, M., Rohde, M., et al. (2019). Still Something to Discover: Novel Insights into Escherichia coli Phage Diversity and Taxonomy. Viruses 11, 454. doi: 10.3390/v11050454
KRINKO (2019). Ergänzung zur Empfehlung der KRINKO „Hygienemaßnahmen bei Infektionen oder Besiedlung mit multiresistenten gramnegativen Stäbchen” (2012) im Zusammenhang mit der von EUCAST neu definierten Kategorie „I” bei der Antibiotikaresistenzbestimmung: Konsequenzen für die Definition von MRGN. Epid Bull 9, 82–83. doi: 10.25646/5916
Kropinski, A. M., Mazzocco, A., Waddell, T. E., Lingohr, E., Johnson, R. P. (2009). Enumeration of bacteriophages by double agar overlay plaque assay. Methods Mol. Biol. 501, 69–76. doi: 10.1007/978-1-60327-164-6_7
La Rosa-Carrillo, D., Suárez-Cuartín, G., Golpe, R., Máiz Carro, L., Martinez-Garcia, M. A. (2022). Inhaled colistimethate sodium in the management of patients with bronchiectasis infected by Pseudomonas aeruginosa: A narrative review of current evidence. Infect. Drug Resist. 15, 7271–7292. doi: 10.2147/IDR.S318173
Lecoutere, E., Ceyssens, P.-J., Miroshnikov, K. A., Mesyanzhinov, V. V., Krylov, V. N., Noben, J.-P., et al. (2009). Identification and comparative analysis of the structural proteomes of phiKZ and EL, two giant Pseudomonas aeruginosa bacteriophages. Proteomics 9, 3215–3219. doi: 10.1002/pmic.200800727
Letunic, I., Bork, P. (2024). Interactive Tree of Life (iTOL) v6: recent updates to the phylogenetic tree display and annotation tool. Nucleic Acids Res. 52 (W1), W78–W82. doi: 10.1093/nar/gkae268
Liu, B., Zheng, D., Zhou, S., Chen, L., Yang, J. (2022). VFDB 2022: a general classification scheme for bacterial virulence factors. Nucleic Acids Res. 50, D912–D917. doi: 10.1093/nar/gkab1107
Mabrouk, S. S., Abdellatif, G. R., Abu Zaid, A. S., Aziz, R. K., Aboshanab, K. M. (2022). In Vitro and Pre-Clinical Evaluation of Locally Isolated Phages, vB_Pae_SMP1 and vB_Pae_SMP5, Formulated as Hydrogels against Carbapenem-Resistant Pseudomonas aeruginosa. Viruses 14, 2760. doi: 10.3390/v14122760
Makarova, K. S., Wolf, Y. I., Iranzo, J., Shmakov, S. A., Alkhnbashi, O. S., Brouns, S. J. J., et al. (2020). Evolutionary classification of CRISPR-Cas systems: a burst of class 2 and derived variants. Nat. Rev. Microbiol. 18, 67–83. doi: 10.1038/s41579-019-0299-x
Markwitz, P., Lood, C., Olszak, T., van Noort, V., Lavigne, R., Drulis-Kawa, Z. (2021). Genome-driven elucidation of phage-host interplay and impact of phage resistance evolution on bacterial fitness. ISME J. 16, 533–542. doi: 10.1038/s41396-021-01096-5
Mathee, K. (2018). Forensic investigation into the origin of Pseudomonas aeruginosa PA14 - old but not lost. J. Med. Microbiol. 67, 1019–1021. doi: 10.1099/jmm.0.000778
McCallin, S., Sarker, S. A., Sultana, S., Oechslin, F., Brüssow, H. (2018). Metagenome analysis of Russian and Georgian Pyophage cocktails and a placebo-controlled safety trial of single phage versus phage cocktail in healthy Staphylococcus aureus carriers. Environ. Microbiol. 20, 3278–3293. doi: 10.1111/1462-2920.14310
Meier-Kolthoff, J. P., Göker, M. (2017). VICTOR: genome-based phylogeny and classification of prokaryotic viruses. Bioinformatics 33, 3396–3404. doi: 10.1093/bioinformatics/btx440
Miller, W. R., Arias, C. A. (2024). ESKAPE pathogens: antimicrobial resistance, epidemiology, clinical impact and therapeutics. Nat. Rev. Microbiol. 22, 598–616. doi: 10.1038/s41579-024-01054-w
Moller, A. G., Winston, K., Ji, S., Wang, J., Hargita Davis, M. N., Solís-Lemus, C. R., et al. (2021). Genes influencing phage host range in Staphylococcus aureus on a species-wide scale. mSphere 6, e01263-20. doi: 10.1128/msphere.01263-20
Mushegian, A. R. (2020). Are there 1031 virus particles on earth, or more, or fewer? J. Bacteriol 202, e00052-20. doi: 10.1128/jb.00052-20
Nazarov, P. A. (2022). MDR pumps as crossroads of resistance: antibiotics and bacteriophages. Antibiotics 11, 734. doi: 10.3390/antibiotics11060734
Nikolic, I., Vukovic, D., Gavric, D., Cvetanovic, J., Aleksic Sabo, V., Gostimirovic, S., et al. (2022). An optimized checkerboard method for phage-antibiotic synergy detection. Viruses 14, 1542. doi: 10.3390/v14071542
Onallah, H., Hazan, R., Nir-Paz, R., Brownstein, M. J., Fackler, J. R., Horne, B., et al. (2023). Refractory Pseudomonas aeruginosa infections treated with phage PASA16: A compassionate use case series. Med 4, 600–611.e4. doi: 10.1016/j.medj.2023.07.002
Parfitt, T. (2005). Georgia: an unlikely stronghold for bacteriophage therapy. Lancet 365, 2166–2167. doi: 10.1016/S0140-6736(05)66759-1
Parmar, K., Komarow, L., Ellison, D. W., Filippov, A. A., Nikolich, M. P., Fackler, J. R., et al. (2023). Interlaboratory comparison of Pseudomonas aeruginosa phage susceptibility testing. J. Clin. Microbiol. 61, e0061423. doi: 10.1128/jcm.00614-23
Payne, R. J., Phil, D., Jansen, V. A. (2000). Phage therapy: the peculiar kinetics of self-replicating pharmaceuticals. Clin. Pharmacol. Ther. 68, 225–230. doi: 10.1067/mcp.2000.109520
Peters, D. L., Davis, C. M., Harris, G., Zhou, H., Rather, P. N., Hrapovic, S., et al. (2023). Characterization of virulent T4-like Acinetobacter baumannii bacteriophages DLP1 and DLP2. Viruses 15. doi: 10.3390/v15030739
Pires, D. P., Vilas Boas, D., Sillankorva, S., Azeredo, J. (2015). Phage therapy: a step forward in the treatment of pseudomonas aeruginosa infections. J. Virol. 89, 7449–7456. doi: 10.1128/JVI.00385-15
Pirnay, J.-P., Djebara, S., Steurs, G., Griselain, J., Cochez, C., Soir, S., et al. (2024). Personalized bacteriophage therapy outcomes for 100 consecutive cases: a multicentre, multinational, retrospective observational study. Nat. Microbiol. 9, 1434–1453. doi: 10.1038/s41564-024-01705-x
Pirnay, J.-P., Verbeken, G. (2023). Magistral phage preparations: is this the model for everyone? Clin. Infect. Dis. 77, S360–S369. doi: 10.1093/cid/ciad481
Pleteneva, E. A., Shaburova, O. V., Sykilinda, N. N., Miroshnikov, K. A., Kadykov, V. A., Krylov, S. V., et al. (2008). Study of the diversity in a group of phages of Pseudomonas aeruginosa species PB1 (Myoviridae) and their behavior in adsorbtion-resistant bacterial mutants. Russ J Genet. 44, 150–158. doi: 10.1134/S1022795408020051
Poggio, E. C., Glynn, R. J., Schein, O. D., Seddon, J. M., Shannon, M. J., Scardino, V. A., et al. (1989). The incidence of ulcerative keratitis among users of daily-wear and extended-wear soft contact lenses. N Engl. J. Med. 321, 779–783. doi: 10.1056/NEJM198909213211202
Prevaldi, C., Paolillo, C., Locatelli, C., Ricci, G., Catena, F., Ansaloni, L., et al. (2016). Management of traumatic wounds in the Emergency Department: position paper from the Academy of Emergency Medicine and Care (AcEMC) and the World Society of Emergency Surgery (WSES). World J. Emerg. Surg. 11, 30. doi: 10.1186/s13017-016-0084-3
Pye, H. V., Krishnamurthi, R., Cook, R., Adriaenssens, E. M. (2024). Phage diversity in one health. Essays Biochem. 68, 607–619. doi: 10.1042/EBC20240012
Qin, S., Xiao, W., Zhou, C., Pu, Q., Deng, X., Lan, L., et al. (2022). Pseudomonas aeruginosa: pathogenesis, virulence factors, antibiotic resistance, interaction with host, technology advances and emerging therapeutics. Signal Transduct Target Ther. 7, 199. doi: 10.1038/s41392-022-01056-1
Rahme, L. G., Stevens, E. J., Wolfort, S. F., Shao, J., Tompkins, R. G., Ausubel, F. M. (1995). Common virulence factors for bacterial pathogenicity in plants and animals. Science 268, 1899–1902. doi: 10.1126/science.7604262
Rappo, U., Cohen, A., Kario, E., Gold, J., Nevenzal, H. T., Levy-Saar, I., et al. (2023). 2891. Nebulized phage therapy for patients with cystic fibrosis with chronic Pseudomonas aeruginosa pulmonary infection: A phase 1b/2a randomized, double-blind, placebo-controlled, multicenter study. Open Forum Infect. Dis. 10, ofad500.2474. doi: 10.1093/ofid/ofad500.2474
Rose, T., Verbeken, G., Vos, D., Merabishvili, M., Vaneechoutte, M., Lavigne, R., et al. (2014). Experimental phage therapy of burn wound infection: difficult first steps. Int. J. Burns Trauma 4, 66–73.
Ruohan, W., Xianglilan, Z., Jianping, W., Shuai Cheng, L. I. (2022). DeepHost: phage host prediction with convolutional neural network. Brief Bioinform. 23, bbab385. doi: 10.1093/bib/bbab385
Schein, O. D., Glynn, R. J., Poggio, E. C., Seddon, J. M., Kenyon, K. R. (1989). The relative risk of ulcerative keratitis among users of daily-wear and extended-wear soft contact lenses. A case-control study. Microbial Keratitis Study Group. N Engl. J. Med. 321, 773–778. doi: 10.1056/NEJM198909213211201
Schmerer, M., Molineux, I. J., Bull, J. J. (2014). Synergy as a rationale for phage therapy using phage cocktails. PeerJ 2, e590. doi: 10.7717/peerj.590
Sepúlveda-Robles, O., Kameyama, L., Guarneros, G. (2012). High diversity and novel species of Pseudomonas aeruginosa bacteriophages. Appl. Environ. Microbiol. 78, 4510–4515. doi: 10.1128/AEM.00065-12
Serra, R., Grande, R., Butrico, L., Rossi, A., Settimio, U. F., Caroleo, B., et al. (2015). Chronic wound infections: the role of Pseudomonas aeruginosa and Staphylococcus aureus. Expert Rev. Anti Infect. Ther. 13, 605–613. doi: 10.1586/14787210.2015.1023291
Serwer, P., Hayes, S. J., Thomas, J. A., Hardies, S. C. (2007). Propagating the missing bacteriophages: a large bacteriophage in a new class. Virol. J. 4, 21. doi: 10.1186/1743-422X-4-21
Shafigh Kheljan, F., Sheikhzadeh Hesari, F., Aminifazl, M. S., Skurnik, M., Gholadze, S., Zarrini, G. (2023). Design of phage-cocktail-containing hydrogel for the treatment of Pseudomonas aeruginosa-infected wounds. Viruses 15, 803. doi: 10.3390/v15030803
Shi, X., Zhao, F., Sun, H., Yu, X., Zhang, C., Liu, W., et al. (2020). Characterization and Complete Genome Analysis of Pseudomonas aeruginosa Bacteriophage vB_PaeP_LP14 Belonging to Genus Litunavirus. Curr. Microbiol. 77, 2465–2474. doi: 10.1007/s00284-020-02011-5
Silva, C., Sá, S., Guedes, C., Oliveira, C., Lima, C., Oliveira, M., et al. (2022). The history and applications of phage therapy in Pseudomonas aeruginosa. Microbiol. Res. 13, 14–37. doi: 10.3390/microbiolres13010002
Skusa, R., Kohlen, J., Podbielski, A., Warnke, P. (2023). Introducing “Rapid phage susceptibility testing” (RPST): an accelerated lytic phage activity test for routine diagnostic laboratories within eight hours. Diagn. Microbiol. Infect. Dis. 107, 116054. doi: 10.1016/j.diagmicrobio.2023.116054
Staudinger, B. J., Muller, J. F., Halldórsson, S., Boles, B., Angermeyer, A., Nguyen, D., et al. (2014). Conditions associated with the cystic fibrosis defect promote chronic Pseudomonas aeruginosa infection. Am. J. Respir. Crit. Care Med. 189, 812–824. doi: 10.1164/rccm.201312-2142OC
Steffan, S. M., Shakeri, G., Kehrenberg, C., Peh, E., Rohde, M., Plötz, M., et al. (2022). Campylobacter bacteriophage cocktail design based on an advanced selection scheme. Antibiotics 11, 228. doi: 10.3390/antibiotics11020228
Storms, Z. J., Teel, M. R., Mercurio, K., Sauvageau, D. (2020). The virulence index: A metric for quantitative analysis of phage virulence. PHAGE 1, 27–36. doi: 10.1089/phage.2019.0001
Stover, C. K., Pham, X. Q., Erwin, A. L., Mizoguchi, S. D., Warrener, P., Hickey, M. J., et al. (2000). Complete genome sequence of Pseudomonas aeruginosa PAO1, an opportunistic pathogen. Nature 406, 959–964. doi: 10.1038/35023079
Tielen, P., Narten, M., Rosin, N., Biegler, I., Haddad, I., Hogardt, M., et al. (2011). Genotypic and phenotypic characterization of Pseudomonas aeruginosa isolates from urinary tract infections. Int. J. Med. Microbiol. 301, 282–292. doi: 10.1016/j.ijmm.2010.10.005
Tielen, P., Wibberg, D., Blom, J., Rosin, N., Meyer, A.-K., Bunk, B., et al. (2014). Genome sequence of the small-colony variant Pseudomonas aeruginosa MH27, isolated from a chronic urethral catheter infection. Genome Announc 2, e01174-13. doi: 10.1128/genomeA.01174-13
Turner, D., Kropinski, A. M., Adriaenssens, E. M. (2021). A roadmap for genome-based phage taxonomy. Viruses 13, 506. doi: 10.3390/v13030506
Villarroel, J., Kleinheinz, K. A., Jurtz, V. I., Zschach, H., Lund, O., Nielsen, M., et al. (2016). HostPhinder: A phage host prediction tool. Viruses 8, 116. doi: 10.3390/v8050116
Waters, E. M., Neill, D. R., Kaman, B., Sahota, J. S., Clokie, M. R. J., Winstanley, C., et al. (2017). Phage therapy is highly effective against chronic lung infections with Pseudomonas aeruginosa. Thorax 72, 666–667. doi: 10.1136/thoraxjnl-2016-209265
Willy, C., Bugert, J. J., Classen, A. Y., Deng, L., Düchting, A., Gross, J., et al. (2023). Phage therapy in Germany-update 2023. Viruses 15, 588. doi: 10.3390/v15020588
World Health Organization (2024). World Health Organization: Bacterial Priority Pathogens List 2024 Bacterial Pathogens of Public Health Importance, to Guide Research, Development, and Strategies to Prevent and Control Antimicrobial Resistance (Geneva: World Health Organization).
Yerushalmy, O., Braunstein, R., Alkalay-Oren, S., Rimon, A., Coppenhagn-Glazer, S., Onallah, H., et al. (2023). Towards standardization of phage susceptibility testing: the Israeli phage therapy center “Clinical phage microbiology”-A pipeline proposal. Clin. Infect. Dis. 77, S337–S351. doi: 10.1093/cid/ciad514
Keywords: bacteriophages, Pseudomonas aeruginosa, phage susceptibility testing, antibiotic resistance, phage selection
Citation: Rieper F, Wittmann J, Bunk B, Spröer C, Häfner M, Willy C, Müsken M, Ziehr H, Korf IHE and Jahn D (2025) Systematic bacteriophage selection for the lysis of multiple Pseudomonas aeruginosa strains. Front. Cell. Infect. Microbiol. 15:1597009. doi: 10.3389/fcimb.2025.1597009
Received: 20 March 2025; Accepted: 14 April 2025;
Published: 23 May 2025.
Edited by:
Mercedes Gonzalez Moreno, Leibniz Institute for Natural Product Research and Infection Biology, Hans Knoll Institute, GermanyReviewed by:
Yunxue Guo, Chinese Academy of Sciences (CAS), ChinaRobert Ramirez-Garcia, Imperial College London, United Kingdom
Copyright © 2025 Rieper, Wittmann, Bunk, Spröer, Häfner, Willy, Müsken, Ziehr, Korf and Jahn. This is an open-access article distributed under the terms of the Creative Commons Attribution License (CC BY). The use, distribution or reproduction in other forums is permitted, provided the original author(s) and the copyright owner(s) are credited and that the original publication in this journal is cited, in accordance with accepted academic practice. No use, distribution or reproduction is permitted which does not comply with these terms.
*Correspondence: Imke H.E. Korf, aW1rZS5rb3JmQGl0ZW0uZnJhdW5ob2Zlci5kZQ==; Dieter Jahn, ZC5qYWhuQHR1LWJzLmRl
†These authors have contributed equally to this work and share last authorship